- 1Department of Veterinary Microbiology and Preventive Medicine, Iowa State University, Ames, IA, United States
- 2Department of Food Science and Human Nutrition, Iowa State University, Ames, IA, United States
- 3Interdepartmental Microbiology Graduate Program, Iowa State University, Ames, IA, United States
Bovine mastitis is an inflammation of the udder tissue parenchyma that causes pathological changes in the glandular tissue and abnormalities in milk leading to significant economic losses to the dairy industry across the world. Mammary pathogenic Escherichia (E.) coli (MPEC) is one of the main etiologic agents of acute clinical mastitis in dairy cattle. MPEC strains have virulence attributes to resist the host innate defenses and thrive in the mammary gland environment. The association between specific virulence factors of MPEC with the severity of mastitis in cattle is not fully understood. Furthermore, the indiscriminate use of antibiotics to treat mastitis has resulted in antimicrobial resistance to all major antibiotic classes in MPEC. A thorough understanding of MPEC’s pathogenesis and antimicrobial susceptibility pattern is required to develop better interventions to reduce mastitis incidence and prevalence in cattle and the environment. This review compiles important information on mastitis caused by MPEC (e.g., types of mastitis, host immune response, diagnosis, treatment, and control of the disease) as well as the current knowledge on MPEC virulence factors, antimicrobial resistance, and the dilemma of MPEC as a new pathotype. The information provided in this review is critical to identifying gaps in knowledge that will guide future studies to better design diagnostic, prevent, and develop therapeutic interventions for this significant dairy disease.
Introduction
Bovine mastitis is an inflammation of the udder tissue parenchyma that may result in physical, chemical, and bacteriological changes in milk, and pathological alterations in the glandular tissue. Although mastitis can be caused by multiple events, e.g., traumatic, physiological, allergic, etc., infectious events are the most prevalent, as more than 140 species of microorganisms are involved in bovine mastitis (Radostitis et al., 2007). The disease results in significant financial losses owing to diagnostic tests, veterinary services, wasted milk and labor, and indirect expenses such as low reproduction rates and premature culling of affected animals (Heikkilä et al., 2018; Romero et al., 2018; Hogeveen et al., 2019). Mastitis-related economic losses in the United States are projected to be USD 2 billion annually (Rollin et al., 2015), with each mastitis case costing producers an average of USD 326 (Liang et al., 2017). In Israel, Holstein cows diagnosed with clinical mastitis caused by Escherichia coli had a decrease in daily milk yield of almost 15%, which is considered an average milk loss of about 200 liters per cow during the 305 days of the lactation period (Blum et al., 2014). In persistent infections, the loss of production is significantly higher; it is estimated at 1,500 liters of milk per cow with a decrease in daily production greater than 30% (Blum et al., 2014). In addition to its effects on dairy cattle, mastitis poses a threat to human health due to the risk of transmitting zoonotic pathogens through ingestion of contaminated milk or direct contact with infected cattle (Sugrue et al., 2019; Maity and Ambatipudi, 2021). Importantly, the emergence of antibiotic-resistant bacteria in milk and dairy products due to the indiscriminate use of antibiotics to treat bovine mastitis is a serious concern (Boireau et al., 2018; Singh et al., 2018). Notably, E. coli is a leading cause of acute clinical mastitis in dairy cattle worldwide (Shpigel et al., 2008; Blum et al., 2017; Gao et al., 2017).
Escherichia coli comprises a highly heterogeneous group of commensal residents of the gut; however, because of the flexibility of its genome (Méric et al., 2016; Blum et al., 2017; Denamur et al., 2021), this organism has evolved into pathogenic strains capable of causing diseases, including bovine mastitis following fecal contamination of the teat skin. Mammary pathogenic E. coli (MPEC) has been proposed as a new pathotype responsible for causing mastitis in dairy animals (Shpigel et al., 2008; Richards et al., 2015). The mammary gland is not a natural or primary habitat for E. coli because of the milk-associated innate immunity components, such as antimicrobial peptides, lysozyme, lactoferrin, and complement (Alamdari and Ehsani, 2017; Karav et al., 2017; Kuhi et al., 2021). However, some E. coli have acquired specific virulence factors (VFs) that might help the bacteria to invade the mammary gland, survive, and multiply in milk (Blum et al., 2008, 2015, 2018; Olson et al., 2018; Salamon et al., 2020; Zhou et al., 2021), turning the mammary gland into an amenable opportunistic habitat for these bacteria. Given the relevance of mastitis caused by E. coli, this paper reviews the important aspects of the disease, the characteristic of the etiologic agent E. coli, diagnosis, and treatments.
Clinical and Subclinical Mastitis
Based on disease symptoms, mastitis can be categorized into two different types, including clinical and subclinical. Clinical mastitis is characterized by visible abnormalities in the udder and milk (Oliveira et al., 2013). Physical examination of the affected mammary gland may reveal redness and pain upon palpation, swelling (edema), and induration (hardening) (Sepúlveda-Varas et al., 2016). Systemic symptoms and behavioral changes, including fever, anorexia, depression, lethargy, and a reduction in grooming might also occur (Medrano-Galarza et al., 2012; Dittrich et al., 2019). Cattle with clinical mastitis are also affected by poor milk quality, as evidenced by increased somatic cell count (SCC) and compositional changes in milk (Kayano et al., 2018; Malik et al., 2018; Skarbye et al., 2018). SCC is higher compared to the typical counts of under 200,000 cells/mL (Cobirka et al., 2020). The compositional changes in milk include the reduction of lactose content (Bezman et al., 2015; Costa et al., 2019), but little effect on fat and protein contents was observed (Kester et al., 2015; Tomazi et al., 2015). Clots and flakes can appear in milk, as well as clear serum-like or bloody discharges (Rainard and Riollet, 2006; Brandt et al., 2010). From 1996 to 2014, the reported incidence of clinical mastitis in United States dairy farms increased from 13% (USDA, 1996) to 25% (USDA, 2016). E. coli is one of the most common causes of clinical bovine mastitis, usually associated with acute symptoms such as dysstasia, diarrhea, and cool extremities (Bradley and Green, 2000; Blum et al., 2014; Hagiwara et al., 2014). E. coli causes predominantly acute infections, of short duration (10–30 days), mainly in the last 2 weeks of the dry period and in the first weeks postpartum, although reinfections may occur throughout lactation (Hogan and Smith, 2012; Wente et al., 2020). In most cases, E. coli is eliminated by the host immune response (Günther et al., 2017). However, severe cases with systemic involvement are difficult to treat and have a reserved prognosis (Günther et al., 2017).
Subclinical mastitis rarely poses an immediate threat to the animal’s life and is 15–40 times more common than clinical mastitis (Seegers et al., 2003). This form of the disease cannot be detected by visual inspection of the udder or milk since both appear normal (Ruegg, 2017). Consequently, due to the concealed nature of the disease, dairy farmers are unaware of the extent of milk quality loss and the risk of subclinical mastitis spreading to other cows in the herd. Subclinical mastitis is mostly caused by gram-negative bacteria, such as E. coli, Klebsiella pneumoniae, and Serratia marcescens (Schukken et al., 2012; Azevedo et al., 2016). These pathogens can be transmitted from an infected quarter to an uninfected quarter within the same animal or a different cattle by milking machine (e.g., teat cup liners), dirty milkers’ hands or gloves, and udder washcloths during the milking process (Klaas and Zadoks, 2018; Mohammed et al., 2020). Subclinical mastitis is the most important intramammary illness in cattle, and it costs the dairy farmer more than clinical mastitis due to the dramatic decrease in milk production and quality (Bobbo et al., 2017; Gonçalves et al., 2018).
The Dilemma of Mammary Pathogenic Escherichia coli as a New Pathotype
Extraintestinal pathogenic E. coli (ExPEC) include different sub-groups, e.g., uropathogenic E. coli (UPEC), meningitis-associated E. coli (MNEC), and sepsis-associated E. coli (SEPEC), causing urinary tract infection, meningitis, peritonitis, and septicemia, respectively (Croxen and Finlay, 2010; Mellata, 2013; Biran and Ron, 2018). This E. coli pathotype-based classification is based on the site of infection, symptoms of the disease, and types of VFs. A novel ExPEC pathotype known as MPEC, one of the most common etiologic agents of bovine mastitis, has been suggested (Shpigel et al., 2008). Compared to other bacteria like Campylobacter spp., Clostridium spp., and Ruminococcus spp. (Zhang et al., 2017), MPEC have undergone evolutionary adaptations (e.g., acquisition of VFs) that improve its colonization of the unique environmental niches found within the mammary gland (Shpigel et al., 2008). These niches include competing microbes, soluble and cellular antimicrobials in milk, e.g., lactoferrin and lactoperoxidase (Kawai et al., 2015; Koshiishi et al., 2017), and the innate response elicited by immune cells (Wellnitz and Bruckmaier, 2012). Consequently, E. coli with specific VFs selected within the mammary gland are better suited to cause bovine mastitis (Blum et al., 2015; Richards et al., 2015; Goldstone et al., 2016; Roussel et al., 2017; Sun et al., 2021; Zhou et al., 2021).
Some research studies found that E. coli isolated from cows with mastitis is less genotypically diverse than the environmental strains, and they mostly lack known E. coli VFs (Ghanbarpour and Oswald, 2010; Blum and Leitner, 2013; Aslam et al., 2021). This lack of VFs not only reflects the genome plasticity of E. coli but, most importantly, it suggests that mastitis-associated E. coli may have undergone some selective pressure based on the presence of specific VFs associated with the ability to survive in milk (Blum et al., 2015; Goldstone et al., 2016). However, despite recent publications focusing on E. coli genomes isolated from mastitis-affected cattle (Blum et al., 2015, 2018; Richards et al., 2015; Goldstone et al., 2016; Kempf et al., 2016; Olson et al., 2018; Aslam et al., 2021; Sun et al., 2021), researchers were unable to agree upon a common genetic group of putative VFs to all MPEC isolates. Importantly, genome studies on mastitis-associated E. coli have been questioned by some investigators, who argue the need to simultaneously analyze the host-pathogen interaction (Zadoks et al., 2011; Leimbach et al., 2017). For example, it is important to associate physiological traits with genomic data, including techniques such as dual RNA-Seq of the host and bacteria (Westermann et al., 2016, 2017), Tn-Seq to test virulence association of genes in vivo (Opijnen et al., 2009; Peek et al., 2020), comparative SNP analysis of orthologous genes and intergenic regions (Rocha et al., 2019), proteomics (Zhang H. et al., 2018; Bathla et al., 2020), and metabolomics (Tong et al., 2019; Hu et al., 2021).
Several research studies suggest that E. coli isolated from mastitis-affected cattle are naturally occurring commensals from the gastrointestinal tract of bovine (Le Gall et al., 2007; Tenaillon et al., 2010; Tourret and Denamur, 2016), mostly from phylogroups A and B1 (Suojala et al., 2011; Blum et al., 2018; Olson et al., 2018; Zhang D. et al., 2018; Guerra et al., 2019, 2020; Aslam et al., 2021; Bag et al., 2021). In other words, it is more likely that E. coli’s VFs implicated in bovine mastitis have their principal function in the colonization and survival in the highly diverse gastrointestinal environment (Leimbach et al., 2017). Consequently, E. coli isolated from mastitis-affected cattle are more likely to be opportunistic and might infect only cattle with a deficient immune system, particularly during the peripartum when leukocyte recruitment to the mammary gland is reduced (Leblanc, 2020). In this case, environmental variables and the cattle predispositions (e.g., innate immune system related to the health status, stage of lactation, and parity) (Burvenich et al., 2003; Wenz et al., 2006) are more important than the invading E. coli’s VFs in determining the severity of mastitis.
Table 1 shows studies on different virulence genes and VFs examined in E. coli isolates from bovine mastitis. Various virulence-related features have been suggested to explain the pathogenicity of E. coli in bovine mastitis, including but not limited to the activation of the innate immune system by pathogen-associated molecular patterns (PAMPs) (Schukken et al., 2011; Bhattarai et al., 2018; Petzl et al., 2018), resistance to serum (Blum and Leitner, 2013; Guerra et al., 2020; Aslam et al., 2021) and neutrophil killing (Kaipainen et al., 2002; Roussel et al., 2017), adhesion and invasion of mammary epithelial cells (Dogan et al., 2006, 2012), and survival and proliferation in milk though secretion system (Richards et al., 2015; Sun et al., 2021). However, it is important to note that although the aforementioned virulence-associated properties benefit E. coli to thrive in the mammary gland environment, they are not unique to mastitis-associated E. coli, but are also common in ExPEC. Moreover, E. coli strains isolated from cattle with mastitis usually do not present the same virulence characteristics, demonstrating the high genetic abundance and variability of mastitis-causing E. coli.
Interestingly, the iron scavenging by the ferric dicitrate system has been shown to be strongly associated with mastitis-associated E. coli (Blum et al., 2015; Goldstone et al., 2016; Kempf et al., 2016; Aslam et al., 2021). The Fec system is essential for the growth of E. coli in milk and may influence disease severity in infections (Olson et al., 2018). Iron in bovine milk is limited and kept at quantities below those required to enable E. coli growth because most iron is attached to citrate (Blum et al., 2018) and a lesser amount to lactoferrin (Karav et al., 2017), transferrin (Kupczyński et al., 2017), xanthine oxidase (Harrison, 2006), and certain caseins (Usami et al., 2011). To overcome the low amount of free iron in milk, E. coli expresses a variety of iron transport systems, such as the high-affinity siderophores (e.g., enterobactin, aerobactin, ferrichrome) and iron-regulated outer membrane proteins (IROMP) (van der Helm, 1998) that bind to ferric siderophore complexes for transportation into the bacterial cell. In a population-level genomic analysis, Blum et al. (2018) showed that the Fec system is required for the pathogenicity of E. coli in the udder environment, as the prototypical MPEC strain P4 was unable to induce mastitis without the system, and the non-mammary gland-pathogenic K71 strain gained MPEC capability with its addition. Importantly, while additional research is needed to fully comprehend the significance of the Fec system in mastitis-associated E. coli, this critical structural component is a promising target for novel chemotherapeutics, enhanced mastitis therapy medicines, and novel vaccines.
Virulence Attributes of Escherichia coli Associated With the Severity of Mastitis
The impact of known virulence genes on the severity of bovine mastitis caused by E. coli is currently unclear (Zadoks et al., 2011), and numerous virulence gene profiles have been found in both persistent and transient isolates, suggesting a high genotypic variability of mastitis-associate E. coli (Ghanbarpour and Oswald, 2010; Fernandes et al., 2011; Dogan et al., 2012; Fairbrother et al., 2015). Importantly, clinical symptoms of E. coli mastitis may be related to genes that have yet to be discovered or to characteristics related to the host (e.g., stage of lactation, number and function of circulating neutrophils, SCC, age of the cattle, nutritional and metabolic status, and genetic resistance) (Burvenich et al., 2003). However, persistent infections seem to be related to particular traits (Lippolis et al., 2014), especially the ability of E. coli to transfer from milk to mammary epithelial cells and survive intracellularly (White et al., 2010). Epithelial cell adhesion and invasion are important events in chronic or persistent infection (Finlay and Cossart, 1997). In vitro studies demonstrated that persistent mastitis-associated E. coli strains have a higher ability to adhere, invade and survive in bovine mammary epithelial cells line MAC-T than transient strains (Dogan et al., 2006), are more capable of penetrating an endosome-like compartment of mammary epithelial cells (Passey et al., 2008), and have a different intracellular trafficking mechanism than transient strains (Almeida et al., 2011). Moreover, it was shown that persistent E. coli isolates were more likely to carry lpf genes (encodes for long polar fimbriae), which correlates with E. coli’s ability to invade mammary epithelial cells (Dogan et al., 2012). Interestingly, in a host-pathogen gene expression study conducted by Kerro Dego et al. (2012), both acute and persistent E. coli mastitis strains boosted immune response gene expression (e.g., IRF1, CD83, IL-1α, IL-6, IL-8, CCL20, CXCL 2, MHC 1, Casp 3, CFB), but the immune response increase was substantially greater in cells cocultured with the acute E. coli strain than in cells cocultured with the persistent strain. Similarly, other investigations reported greater up-regulation in immune response functions, inflammation, and antigen processing and presentation in the acute phase than in the chronic phase of bovine mastitis (Mitterhuemer et al., 2010; Rinaldi et al., 2010; Buitenhuis et al., 2011). The induction of a modest immune response by persistent strain is insufficient to eradicate the infection, allowing E. coli to survive in the mammary gland for a lengthy period.
Host Immune Response to Escherichia coli
Innate and Acquired Immunity of the Mammary Gland
Bovine innate immunity comprises physical barriers at the tip of the teat and elements such as neutrophils, macrophages, cytokines, natural killer (NK) cells, lactoferrin, and complement, that act predominantly in the early stages of E. coli infections. This variety of immune cell types constitutes the somatic cells (SC), with neutrophils being the predominant type at the beginning of inflammation, representing about 90% of the SC increase (Ingvartsen and Moyes, 2015; Becheva et al., 2017). When pathogens invade the mammary gland, they face the first line of cellular defense, such as phagocytosis by neutrophils, which usually respond promptly to the inflammatory process (Mehrzad et al., 2004; Aitken et al., 2011; Bassel and Caswell, 2018). As a result, the severity of mastitis is determined by the speed with which neutrophils are recruited to the site of infection and how effectively this cell type phagocytes the pathogen (Mehrzad et al., 2004; Alhussien et al., 2016; Chengolova et al., 2021). Importantly, the efficacy of the innate response influences the incidence of new intramammary infections (Sordillo, 2018), clinical severity (Pezeshki et al., 2011), and duration of the case (Blum et al., 2017). Another crucial component of the host’s innate immunity is lactoferrin, an iron-tropic glycoprotein with bacteriostatic properties produced by epithelial cells and leukocytes (Shimazaki and Kawai, 2017). In ruminants, lactoferrin has been shown to inhibit the multiplication of E. coli by binding to iron ions, making them unavailable for bacterial growth (Rybarczyk et al., 2017; Kieckens et al., 2018). Additionally, cytokines including interleukins (IL), colony-stimulating factor (CSF), interferon (IFN), and tumor necrosis factor (TNF) are crucial in the mammary gland innate defense (Bhattarai et al., 2018; Vitenberga-verza et al., 2022). Cytokines are responsible for local signs of inflammation such as swelling, redness, pain, and systemic symptoms such as fever, tachycardia, increased respiratory rate, anorexia, and depression (Sordillo et al., 1997; Thompson-Crispi et al., 2014). When innate immunity is not efficient in fighting the infection, the specific (or acquired) immunity mediated by lymphocytes occurs. In response to the inflammation, antibodies produced by lymphocytes pass from the bloodstream to the milk due to increased vascular permeability of the gland. The inflammatory response to mastitis aims to eradicate the microorganism responsible for the infection, neutralize the pathogen’s toxins, and then regenerate injured udder tissue so that the volume of milk normally produced is quickly recovered.
Mammary Pathogenic Escherichia coli-Elicited Immune Response
The onset of mastitis occurs when MPEC penetrates through the teat canal, multiplies in the teat and gland cisterns, and spreads throughout the milk-producing glandular tissue (Figure 1A). Once inside the mammary gland, MPEC ferment lactose (Blum et al., 2008; Lippolis et al., 2009) and use it as an energy source to multiply without adhering to the gland’s epithelial surface, remaining in the cisterns, ducts, and alveolar lumen (Roussel et al., 2017; Bianchi et al., 2019). Some MPEC strains can adhere to the udder epithelium, causing chronic intramammary infections and recurrent cases of clinical mastitis (Dogan et al., 2006; Roussel et al., 2017). The multiplication and lysis of MPEC release LPS from the bacteria’s outer membrane (Figure 1B). LPS binds to the LPS binding protein (LBP), and the LPS-LBP complex binds to the cluster of differentiation (CD)-14, which leads the LPS to a toll-like receptor-4 (TLR) on the surface of macrophages (Bhattarai et al., 2018; Eckel and Ametaj, 2020; Figure 1C). When LPS binds to its respective TLR, an intracellular signaling cascade is activated, including the activation of nuclear factor kappa-beta (NF-κβ), which induces macrophages and mammary epithelial cells to synthesize pro-inflammatory cytokines [e.g., interleukin (IL)-1β, IL-1, IL-6, IL-8, and tumor necrosis factor (TNF-α)] and acute-phase proteins (e.g., serum amyloid A and haptoglobin) (Fitzgerald et al., 2007; Porcherie et al., 2012; Roussel et al., 2015; Günther et al., 2017; Curone et al., 2018; Akhtar et al., 2020; Bronzo et al., 2020; Khan et al., 2020; Figure 1D). Importantly, TNF-α is the main mediator of endotoxic shock that occurs in cases of super-acute mastitis caused by coliforms (Hogan and Smith, 2003; Shaheen et al., 2020).
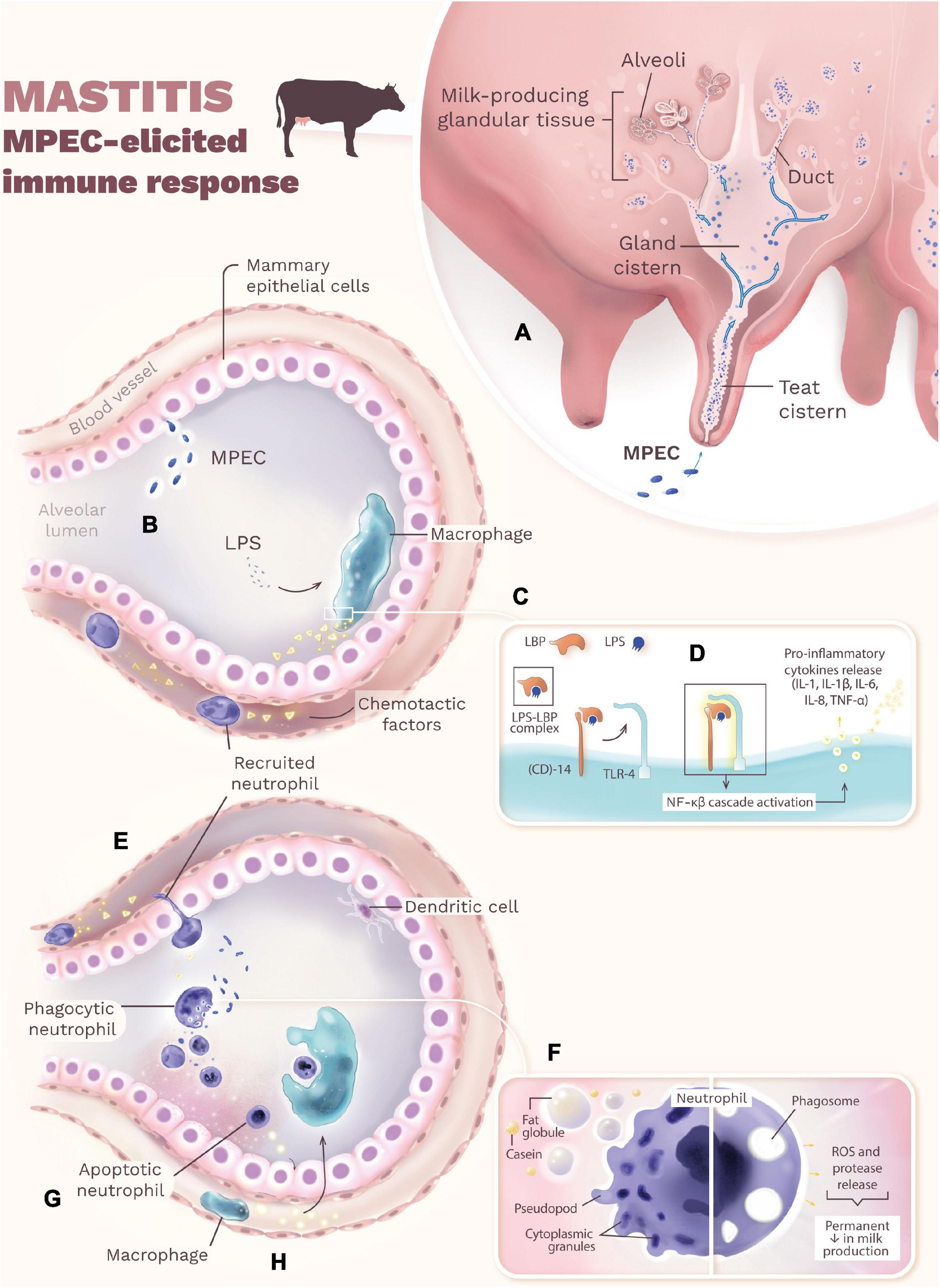
Figure 1. The onset of mastitis occurs when MPEC penetrates through the teat canal, multiplies in the teat and gland cisterns, and spreads throughout the milk-producing glandular tissue (A). The multiplication and lysis of MPEC release LPS from the bacteria’s outer membrane (B). LPS binds to the LBP, and the LPS-LBP complex binds to the CD-14, which leads the LPS to a TLR-4 on the surface of macrophage (C). When LPS binds to its respective TLR, an intracellular signaling cascade is activated (NF-κβ), which induces macrophages and mammary epithelial cells to synthesize pro-inflammatory cytokines and acute-phase proteins (D). Chemotactic factors, especially IL-8, then recruit neutrophils from the bloodstream that function as phagocytes at the site of infection in the alveoli (E). The exposure of neutrophils to fat globules and casein leads to loss of cytoplasmic granules (reduced bactericidal activities) and altered morphology (rounding), eliminating pseudopods needed for phagocytosis (F). During phagocytosis, neutrophils release chemicals that eliminate MPEC but also injure mammary epithelial cells, resulting in a permanent decrease in milk production (F). To minimize mammary tissue damage, neutrophils undergo programmed cell death (apoptosis) and release chemokines that attract macrophages to the site of infection (Paape et al., 2003) (G). In the last step of the inflammatory reaction, macrophages quickly phagocytose apoptotic neutrophils (efferocytosis), minimizing the release of granular contents that damage the tissue (H). Interestingly, whereas in the mammary alveolar, dendritic cells are generally non-responsive to MPEC infection.
Chemotactic factors, especially IL-8, recruit neutrophils from the bloodstream that function as phagocytes at the site of infection in the alveoli (Zaatout, 2022; Figure 1E). The efficiency of neutrophils’ diapedesis and phagocytosis directly influence the evolution of the local inflammatory process and the severity of mastitis (Elazar et al., 2010; Roussel et al., 2017; Bassel and Caswell, 2018). Although opsonization is not essential for phagocytosis, immunoglobulins (Ig) such as IgG2 and IgM recognize MPEC through Fab-regions and bind to neutrophils via Fc-receptors on the plasma membrane, leading to phagocytosis in a “zipper mechanism” (Burvenich et al., 2003). The exposure of neutrophils to fat globules and casein leads to loss of cytoplasmic granules (reduced bactericidal activities) and altered morphology (rounding), eliminating pseudopods needed for phagocytosis (Paape et al., 2003; Swain et al., 2014; Sordillo, 2018; Panda et al., 2019; Figure 1F). Consequently, many neutrophils are necessary to cope with the reduced phagocytic capabilities and control the infection. During phagocytosis, neutrophils release chemicals, e.g., reactive oxygen species (ROS) (Lauzon et al., 2005) and proteases (Mehrzad et al., 2005), that eliminate MPEC but also injure mammary epithelial cells, resulting in a permanent decrease in milk production (Zhao and Lacasse, 2008; Eckel and Ametaj, 2020; Figure 1F). To minimize mammary tissue damage, neutrophils undergo programmed cell death (apoptosis) and release chemokines that attract macrophages to the site of infection (Sladek et al., 2005; Figure 1G). In the last step of the inflammatory reaction, macrophages quickly phagocytose apoptotic neutrophils (efferocytosis), minimizing the release of granular contents that damage the tissue (Greenlee-Wacker, 2016; Figure 1H); whereas in the mammary alveolar, dendritic cells are generally non-responsive to MPEC infection. However, when efferocytosis done by macrophages is ineffective, there is increased clearing of apoptotic neutrophils by dendritic cells (Tian et al., 2016). Curing of the mammary gland is only successful if minimal tissue damage occurs (Gonen et al., 2007).
Bovine Mastitis Diagnosis
Clinical mastitis is easily detected by visible abnormalities in the udder (e.g., redness, swelling, increased temperature, and pain upon physical palpation) and milk (e.g., presence of clots to clear, serum-like, or bloody secretions). In subclinical mastitis, however, indirect inflammation measures must be used to detect the disease. Contrarily to clinical mastitis, the only visible sign of animals with subclinical mastitis is reduced milk production (Bobbo et al., 2017; Martins et al., 2020). Consequently, the diagnostic requires complementary tests based on the SCC of milk (Adkins and Middleton, 2018). The SCC is used as an indicator of mammary gland health and milk quality. Usually, healthy cows produce milk with less than 100,000 SCC/mL, while cows with mastitis have a minimum SCC of 200,000 SCC/mL (Kelly et al., 2011). The California Mastitis Test (CMT) estimates the SCC on milk and can be performed at the cow-side to detect mastitis (Bhutto et al., 2012; Godden et al., 2017). Electronic SCC is another test performed on the total mixed (composite) milk from all quarters of each cow, and it helps track the progress of control programs (Alhussien and Dang, 2018). Microbiologic culture of milk from individual quarters or composite samples of individual animals is another tool to identify E. coli involved in clinical mastitis (Royster et al., 2014; Ferreira et al., 2018). However, since the number of bacteria may be too small to be identified by standard methods, bacteria are not detected in roughly 30% of samples (Taponen et al., 2009; El-Sayed et al., 2017; Singh et al., 2018). Repeated culture and specialized culture techniques may be required in these cases.
Improved Diagnosis of Escherichia coli Mastitis: Challenges and Future Approaches
Because of the high costs of E. coli mastitis and the importance of maintaining cattle’s health and well-being, early diagnosis is critical. As previously mentioned in Section “Bovine Mastitis Diagnosis,” SCC and CMT are techniques commonly used to diagnose bovine mastitis. Unfortunately, both techniques have drawbacks, including subjective interpretation of CMT results (Swinkels et al., 2021), which could result in false positives and negatives (Fosgate et al., 2013), and factors such as lactation number, milk yield, stress, season, and breed that could influence with SCC’s interpretation (Alhussien and Dang, 2018). As a result, genotypic approaches are also used to supplement phenotypic identification of bovine mastitis, providing a viable option for resolving issues such as false-negative results. For example, approximately 30% of clinical mastitis samples do not grow in bacterial culture (Taponen et al., 2009); however, polymerase chain reaction (PCR) is sensitive to detect growth-inhibited and reduces false-negative results (El-Sayed et al., 2017). Other molecular typing methods used for the identification of bovine mastitis pathogen at the species level include ribotyping (Zadoks et al., 2011) and amplified fragment length polymorphism (AFLP) (Leung et al., 2004); at the strain level, include restriction fragment length polymorphism (RFLP) (Ombarak et al., 2018), pulsed-field gel electrophoresis (PFGE) typing (Freitag et al., 2017), and multiple-locus variable-number tandem repeat analysis (MLVA) (Elsayed et al., 2021); and at both species and strain levels include transfer DNA intergenic spacer length polymorphism (Chakraborty et al., 2019) and DNA sequencing of housekeeping genes (Ali et al., 2017). Another genotypic method is microarray technology, which can detect seven common species of mastitis-causing pathogens in as little as 6 h, with a sensitivity of 94.1% and a specificity of 100% (Lee et al., 2008).
New biomarkers for high sensitivity and specificity, rapid and efficient with a “cow-side” application, are among the development prospects for novel E. coli mastitis diagnosis techniques (Duarte et al., 2015; Sharifi et al., 2018; Li L. et al., 2019). For example, proteomic research has yielded data on protein expression patterns that can be used to find novel therapeutic targets (e.g., bacterial immunogenic proteins for vaccines) and diagnostic biomarkers for bovine mastitis (Ceciliani et al., 2014). Importantly, a detection method’s suitability for routine mastitis diagnosis is determined by its specificity, sensitivity, cost, processing time, and ability to handle a large number of milk samples. Proteomic technique for accurate biomarkers is feasible for early identification of mastitis and treatment efficacy, as well as the discovery of possibly novel targets for alternative therapy development. These advancements, however, are still not suitable for routine diagnosis of bovine mastitis.
Bovine Mastitis Treatment and Control
Since mastitis results from the interaction of many different factors, especially milking procedures and cleanliness of the environment, there is no single way to treat and control the disease. The control of environmental mastitis is especially challenging because E. coli is ubiquitous in the cattle’s environment. Thus, good herd health management and environmental hygiene are the most critical factors in preventing this type of mastitis (Klaas and Zadoks, 2018; Zigo et al., 2021). Except for severe infections during the puerperal period, antibiotic therapy for coliform mastitis is not recommended due to the high spontaneous cure rates (Suojala et al., 2013; Ruegg, 2017) and the risk of antibiotic contamination of the bulk milk (Tang et al., 2017). Supportive care such as fluid therapy and treatment with steroidal or non-steroidal anti-inflammatory drugs (e.g., dexamethasone) should be the first treatment option (Barlow, 2011; Kayitsinga et al., 2017; Sintes et al., 2020). Parenteral administration of fluoroquinolones (e.g., enrofloxacin, danofloxacin, marbofloxacin) or third-generation cephalosporin (e.g., ceftiofur) is recommended to treat severe E. coli mastitis (Suojala et al., 2013; Krömker and Leimbach, 2017).
Another approach to prevent coliform mastitis is to boost the animal’s specific immunity through vaccination (Ismail, 2017; Rainard et al., 2021a), particularly with the E. coli J5 vaccine (Suojala et al., 2013; Pomeroy et al., 2016; Herry et al., 2017). While the teat canal is the first line of defense against intramammary infections, milk neutrophils are the major immune defense when E. coli has already invaded the teat canal (Sordillo, 2005; Lind et al., 2015; Bassel and Caswell, 2018). When intramammary infections are not quickly controlled by neutrophils, allowing LPS and lipoproteins to escape into the bloodstream, antibodies in the blood serum must be present to neutralize these potent toxins. Thus, preventing coliform mastitis through vaccination aims to achieve high levels of effective anti-coliform antibodies and antitoxins, both in milk and in the bloodstream, and around the teat canal (Erskine et al., 2007; Wilson et al., 2009; Kawai et al., 2020). Two mutant strains of bacteria, E. coli O111:B4 (J5) and Salmonella Typhimurium Re-17 have been used in commercial preparations to immunize cows against coliform mastitis (Erskine, 2012; El-Sayed and Kamel, 2021). E. coli O111:B4 (J5) is a rough mutant strain with an incomplete “O” polysaccharide chain in the cell wall, exposing the homologous half of the LPS core antigen (Zaatout, 2021). When this antigen is exposed, it stimulates the synthesis of immunoglobulins (e.g., IgM, IgG1, IgG2) that cross-react with other bacteria’s core antigens, resulting in immunity and protection against a wide range of bacterial genera and strains (Rainard et al., 2021a,b). The J5 vaccination boosts blood and milk titers of specific antibodies against E. coli LPS, enhancing its opsonization (Brade et al., 2013; Steele et al., 2019). Importantly, neutrophils can phagocyte bacteria more effectively when pathogens are opsonized by antibodies (Rainard et al., 2022). Vaccinations are often timed to correspond with the highest risk of acquiring mastitis by coliforms (e.g., pre- and immediate postpartum), with immunizations being carried out 30 days before delivery and in the first week following delivery (Rainard et al., 2021a). Increasing the cattle’s natural ability to fight infections in the mammary gland during periods of increased susceptibility is one alternative for reducing mastitis in dairy cows.
The outer membrane protein A (OmpA) has also been considered a potential antigen in the bovine mastitis vaccine associated with E. coli (Rainard et al., 2017; Chen et al., 2020; Liu et al., 2021). OmpA is a crucial virulence factor in the pathogenesis of E. coli, playing a key role in pore and biofilm formation, host cell invasion, and multidrug resistance (Confer and Ayalew, 2013). For example, recombinant OmpA protein fragments could regulate the expression of cytokines, chemokines, nitric oxide synthase, and cyclooxygenase-2, preventing E. coli meningitis in mice (Hsieh et al., 2016). Moreover, OmpA has been found to reduce complement-dependent or phagocytic-dependent killing of OmpA-positive E. coli (Krishnan and Prasadarao, 2012). Therefore, OmpA is a potential antigen in vaccine development and for the prevention of E. coli infection. However, despite current investigations on mastitis vaccine, there is limited information on OmpA as a viable vaccine antigen in mastitis-associated E. coli. Among the few studies on OmpA as a vaccine candidate in E. coli mastitis, Rainard et al. (2017) investigated the immunogenicity of OmpA by immunizing cows with a recombinant protein (rEcOmpA) to potentially stimulate antibodies and cell-mediated immune responses. Interestingly, the intramammary immunization elicited antibodies (e.g., IgG1 and IgG2), but the antibodies could not interact with the mastitis-causing strain P4, implying that they were unable to reach the accessible regions of OmpA molecules located at the outside leaflet of the bacteria external membrane. Collectively, the aforementioned studies show that the potential of OmpA as a vaccine to prevent E. coli-associated bovine mastitis has yet to be determined.
Antimicrobial Resistance of Mastitis-Associated Escherichia coli
Antibiotics are crucial in curing serious cases of bovine mastitis caused by E. coli (Bengtsson et al., 2009; Suojala et al., 2013). Several antimicrobial agents have been approved in the United States to treat E. coli mastitis, such as tetracyclines, macrolides, sulphonamides, quinolones, and β-lactams (Mathew et al., 2007; Bengtsson et al., 2009). Unfortunately, antibiotic therapy has frequently been demonstrated to have little or no effect in treating clinical or subclinical mastitis caused by coliforms in cattle (Suojala et al., 2010; Ruegg, 2018). This fact is evidenced by the increase in antimicrobial-resistant isolates (Skočková et al., 2015; Zhang D. et al., 2018; Cao et al., 2019; Ngaywa et al., 2019; Yu et al., 2020; Majumder et al., 2021) and the growing number of recurrent and persistent mastitis cases (Fairbrother et al., 2015; Bag et al., 2021; Taniguchi et al., 2021), often caused by the same E. coli isolate. E. coli isolated from the milk of mastitis-affected cattle is resistant to numerous antibiotic classes, including but not limited to aminopenicillin (e.g., cloxacillin) (Holko et al., 2019; Guerra et al., 2020), polypeptide (e.g., bacitracin) (Tanih et al., 2015), lincosamide (e.g., lincomycin) (Fazel et al., 2019), and macrolide (e.g., erythromycin) (Yu et al., 2020; Ahmed et al., 2021). In clinical mastitis, additional caution is advised when determining whether or not to treat cattle with antimicrobials, as antibiotics are not always essential, such as in cases of negative cultures and minor coliform infections (Makovec and Ruegg, 2003; Suojala et al., 2011; Oliveira and Ruegg, 2014; Fuenzalida and Ruegg, 2019). However, severe cases of mastitis caused by E. coli must be treated immediately following diagnosis due to endotoxin-induced shock (Persson et al., 2015).
Pathologic alterations in the mammary gland, such as necrosis and ischemia, might obstruct antimicrobial distribution in the udder parenchyma, preventing the antimicrobial from reaching the site of infection or reaching sub-inhibitory concentrations (Akers and Nickerson, 2011). Importantly, the ability of E. coli to form biofilms on the tissue surface of the infected mammary gland, thereby establishing an inherent resistance to several antimicrobials treatment, is one of the hypotheses to explain the emergence of recurring mastitis (Olson et al., 2002; Melchior et al., 2006; Pedersen et al., 2021; Rudenko et al., 2021). E. coli is notorious for being a reservoir for antimicrobial resistance genes, and it has the ability to horizontally transmit such genes to other pathogenic bacteria (Freitag et al., 2017; Zhang et al., 2020). Investigations have shown that the most prevalent resistance genes found in mastitis-associated E. coli encode resistance to aminoglycoside (e.g., aadA), streptomycin (e.g., strA, strB), tetracycline (e.g., tetA, tetC), sulfonamide (e.g., sulI, sulII), ampicillin (e.g., ampC), and β-lactams (e.g., blaTEM, blaCTX-M) (Srinivasan et al., 2007; Freitag et al., 2017; Todorović et al., 2018; Fazel et al., 2019; Yu et al., 2020). Importantly, the extended-spectrum β-lactamase enzymes (ESBL) are a major concern in the antibiotic resistance mechanism of E. coli mastitis (Schmid et al., 2013; Klaas and Zadoks, 2018; Yang et al., 2018). These enzymes are located in plasmids and can hydrolyze penicillin (Padmini et al., 2017), third and fourth-generation cephalosporins (Li Q. et al., 2019), and monobactam antibiotics (Jacoby, 2009; Bush and Jacoby, 2010). The detection of ESBL-producing isolates is of concern due to the direct association between multi-resistant bacteria and high mortality rates in humans (Bengtsson et al., 2009). There is a clear increase in the prevalence of ESBL E. coli intestinal carriage among healthy individuals worldwide (2.6% in 2003–2005 to 21.1% in 2015–2018; an average increase of 1.2% per year) (Bezabih et al., 2021). Importantly, several countries, including China (Ali et al., 2016), Egypt (Ahmed et al., 2021), Germany (Eisenberger et al., 2018), and Greece (Filioussis et al., 2020), have been reporting the occurrence of ESBL-producing E. coli in cattle with mastitis. Moreover, the presence of ESBL-producing E. coli in milk raises concerns about the risks of ingesting unpasteurized milk and dairy products, potentially transmitting ESBL-producing E. coli to humans (Freitag et al., 2017). The prevalence of these isolates in milk has ranged from 0.4% (Freitag et al., 2017) to 25.4% (Dahmen et al., 2013).
Tests used to verify the antimicrobial sensitivity of pathogens causing mastitis, including antibiogram, disc diffusion technique, and minimum inhibitory concentration (MIC), are alternatives for the rational use of antimicrobials, aiming to support the treatment only of quarters infected by agents with the possibility of cure. Nowadays, laboratories’ most often used method is the MIC, which produces more accurate findings than the antibiogram (Cheng et al., 2019; Bolte et al., 2020). This technique, however, does not yield reliable results when assessing sessile cells since sensitivity studies on bacteria organized in biofilms reveal that the concentration of antibiotics necessary for biofilm eradication is several times larger than that determined by MIC (Li et al., 2020; Pedersen et al., 2021).
Conclusion
Under field conditions, colonization of the mammary gland is a polymicrobial event. Since milk is an excellent growth medium, many species of bacteria grow on it, colonize the mammary gland, and elicit inflammation and disease. Nevertheless, most mastitis cases are attributed to a single bacterial species or even to a single strain or clone of a species. The ability of E. coli to acquire exogenous DNA, including virulence genes, contributes to the development of pathogenic strains adapted to the mammary gland and potentially cause mastitis. MPEC has been proposed as a new pathotype responsible for causing mastitis in dairy animals. Some mastitis-associated E. coli strains evolved mechanisms that enable them to compete with other bacteria co-infecting the gland and evading the protective mechanisms of the host. However, it is important to note that although the aforementioned virulence-associated properties benefit E. coli to thrive in the mammary gland environment, they are not unique to mastitis-associated E. coli, but are also common in ExPEC. It is crucial to understand the principles behind the infection strategy of E. coli so that new approaches to treat and prevent mastitis by this bacteria and perhaps other mammary pathogens can be developed. The use of antibiotics in farm animals and specifically to treat mastitis is gradually precluded, and thus new treatment and prevention strategies are needed.
Author Contributions
DG and MM wrote the manuscript. DG developed figure and table. MM revised manuscript. Both authors contributed to the article and approved the submitted version.
Funding
The open access publication fees for this article were covered by the Iowa State University Library.
Conflict of Interest
The authors declare that the research was conducted in the absence of any commercial or financial relationships that could be construed as a potential conflict of interest.
Publisher’s Note
All claims expressed in this article are solely those of the authors and do not necessarily represent those of their affiliated organizations, or those of the publisher, the editors and the reviewers. Any product that may be evaluated in this article, or claim that may be made by its manufacturer, is not guaranteed or endorsed by the publisher.
References
Adkins, P. R. F., and Middleton, J. (2018). Methods for diagnosing mastitis. Vet. Clin. N. Am. Food Anim. Pr. 34, 479–491. doi: 10.1016/j.cvfa.2018.07.003
Ahmed, W., Neubauer, H., Tomaso, H., El Hofy, F. I., Monecke, S., and El-Tawab, et al. (2021). Characterization of enterococci-and ESBL-producing Escherichia coli isolated from milk of bovides with mastitis in Egypt. Pathogens 10, 97. doi: 10.3390/pathogens10020097
Aitken, S. L., Corl, C. M., and Sordillo, L. M. (2011). Immunopathology of mastitis: insights into disease recognition and resolution. J. Mammary Gland Biol. Neoplasia 16, 291–304. doi: 10.1007/s10911-011-9230-4
Akers, R. M., and Nickerson, S. C. (2011). Mastitis and its impact on structure and function in the ruminant mammary gland. J. Mammary Gland Biol. Neoplasia 16, 275–289. doi: 10.1007/s10911-011-9231-3
Akhtar, M., Guo, S., Guo, Y., Zahoor, A., Shaukat, A., Chen, Y., et al. (2020). Upregulated-gene expression of pro-inflammatory cytokines (TNF-α, IL-1β and IL-6) via TLRs following NF-κB and MAPKs in bovine mastitis. Acta Trop. 207:105458. doi: 10.1016/j.actatropica.2020.105458
Alamdari, E. K., and Ehsani, M. R. (2017). Antimicrobial peptides derived from milk: a review. J. Food Biosci. Technol. 7, 49–56.
Alhussien, M. N., and Dang, A. K. (2018). Milk somatic cells, factors influencing their release, future prospects, and practical utility in dairy animals: an overview. Vet. World 11, 562–577. doi: 10.14202/vetworld.2018.562-577
Alhussien, M., Manjari, P., Mohammed, S., Sheikh, A. A., Reddi, S., and Dixit, S. (2016). Incidence of mastitis and activity of milk neutrophils in Tharparkar cows reared under semi-arid conditions. Trop. Anim. Heal. Prod. 48, 1291–1295. doi: 10.1007/s11250-016-1068-8
Ali, T., Rahman, S., Zhang, L., Shahid, M., Zhang, S., Liu, G., et al. (2016). ESBL-producing Escherichia coli from cows suffering mastitis in China contain clinical class 1 integrons with CTX-M linked to ISCR1. Front. Microbiol. 7:1931. doi: 10.3389/fmicb.2016.01931
Ali, T., Rahman, S., Zhang, L., Shahid, M., and Han, D. (2017). Characteristics and genetic diversity of multi-drug resistant Escherichia coli isolated from bovine mastitis. Oncotarget 8, 90144–90163. doi: 10.18632/oncotarget.21496
Almeida, R. A., Dogan, B., Klaessing, S., Schukken, Y. H., and Oliver, S. P. (2011). Intracellular fate of strains of Escherichia coli isolated from dairy cows with acute or chronic mastitis. Vet. Res. Commun. 35, 89–101. doi: 10.1007/s11259-010-9455-5
Aslam, N., Khan, S. U. H., Usman, T., and Ali, T. (2021). Phylogenetic genotyping, virulence genes and antimicrobial susceptibility of Escherichia coli isolates from cases of bovine mastitis. J. Dairy Res. 88, 78–79. doi: 10.1017/S002202992100011X
Azevedo, C., Pacheco, D., Soares, L., Romão, R., Moitoso, M., Maldonado, J., et al. (2016). Prevalence of contagious and environmental mastitis-causing bacteria in bulk tank milk and its relationships with milking practices of dairy cattle herds in São Miguel Island (Azores). Trop. Anim. Health Prod. 48, 451–459. doi: 10.1007/s11250-015-0973-6
Bag, M. A. S., Khan, M. S. R., Sami, M. D. H., Begum, F., Islam, M. S., Rahman, M. M., et al. (2021). Virulence determinants and antimicrobial resistance of E. coli isolated from bovine clinical mastitis in some selected dairy farms of Bangladesh. Saudi J. Biol. Sci. 28, 6317–6323. doi: 10.1016/j.sjbs.2021.06.099
Barlow, J. (2011). Mastitis therapy and antimicrobial susceptibility: a multispecies review with a focus on antibiotic treatment of mastitis in dairy cattle. J Mammary Gland Biol. Neoplasia 16, 383–407. doi: 10.1007/s10911-011-9235-z
Bassel, L. L., and Caswell, J. L. (2018). Bovine neutrophils in health and disease. Cell Tissue Res. 371, 617–637. doi: 10.1007/s00441-018-2789-y
Bathla, S., Sindhu, A., Kumar, S., Dubey, S. K., Pattnaik, S., Rawat, P., et al. (2020). Tandem Mass Tag (TMT)-based quantitative proteomics reveals potential targets associated with onset of Sub-clinical Mastitis in cows. Sci. Rep. 10, 1–10. doi: 10.1038/s41598-020-66211-6
Bean, A., Williamson, J., and Cursons, R. T. (2004). Virulence genes of Escherichia coli strains isolated from mastitic milk. J. Vet. Med. B Infect. Dis. Vet. Public Health 51, 285–287. doi: 10.1111/j.1439-0450.2004.00772.x
Becheva, Z., Gabrovska, K., and Godjevargova, T. (2017). Immunofluorescence microscope assay of neutrophils and somatic cells in bovine milk. Food Agric. Immunol. 28, 1196–1210. doi: 10.1080/09540105.2017.1332012
Bengtsson, B., Unnerstad, H. E., Ekman, T., Artursson, K., Nilsson-Öst, M., and Waller, K. P. (2009). Antimicrobial susceptibility of udder pathogens from cases of acute clinical mastitis in dairy cows. Vet. Microbiol. 136, 142–149. doi: 10.1016/j.vetmic.2008.10.024
Bezabih, Y. M., Sabiiti, W., Alamneh, E., Bezabih, A., Peterson, G. M., Bezabhe, W. M., et al. (2021). The global prevalence and trend of human intestinal carriage of ESBL-producing Escherichia coli in the community. J. Antimicrob. Chemother. 76, 22–29. doi: 10.1093/JAC/DKAA399
Bezman, D., Lemberskiy-Kuzin, L., Katz, G., Merin, U., and Leitner, G. (2015). Influence of intramammary infection of a single gland in dairy cows on the cow’s milk quality. J. Dairy Res. 82, 304–311. doi: 10.1017/S002202991500031X
Bhattarai, D., Worku, T., Dad, R., Rehman, Z. U., Gong, X., and Zhang, S. (2018). Mechanism of pattern recognition receptors (PRRs) and host pathogen interplay in bovine mastitis. Microb. Pathog. 120, 64–70. doi: 10.1016/j.micpath.2018.04.010
Bhutto, A. L., Murray, R. D., and Woldehiwet, Z. (2012). California mastitis test scores as indicators of subclinical intra-mammary infections at the end of lactation in dairy cows. Res. Vet. Sci. 92, 13–17. doi: 10.1016/j.rvsc.2010.10.006
Bianchi, R. M., Schwertz, C. I., de Cecco, B. S., Panziera, W., De Lorenzo, C., Heck, L. C., et al. (2019). Pathological and microbiological characterization of mastitis in dairy cows. Trop. Anim. Heal. Prod. 51, 2057–2066. doi: 10.1007/s11250-019-01907-0
Biran, D., and Ron, E. Z. (2018). “Extraintestinal pathogenic Escherichia coli’,” in Escherichia Coli, a Versatile Pathogen, eds G. Frankel and E. Z. Ron (Switzerland: Springer), 149–161.
Blum, S. E., and Leitner, G. (2013). Genotyping and virulence factors assessment of bovine mastitis Escherichia coli. Vet. Microbiol. 163, 305–312. doi: 10.1016/j.vetmic.2012.12.037
Blum, S. E., Goldstone, R. J., Connolly, J. P. R., Répérant-Ferter, M., Germon, P., Inglis, N. F., et al. (2018). Postgenomics characterization of an essential genetic determinant of mammary pathogenic Escherichia coli. MBio 9:e423–e418. doi: 10.1128/mBio.00423-18
Blum, S. E., Heller, E. D., and Leitner, G. (2014). Long term effects of Escherichia coli mastitis. Vet. J. 201, 72–77. doi: 10.1016/j.tvjl.2014.04.008
Blum, S. E., Heller, E. D., Jacoby, S., Krifucks, O., and Leitner, G. (2017). Comparison of the immune responses associated with experimental bovine mastitis caused by different strains of Escherichia coli. J. Dairy Res. 84, 190–197. doi: 10.1017/S0022029917000206
Blum, S. E., Heller, E. D., Krifucks, O., Sela, S., Hammer-Muntz, O., and Leitner, G. (2008). Identification of a bovine mastitis Escherichia coli subset. Vet. Microbiol. 132, 135–148. doi: 10.1016/j.vetmic.2008.05.012
Blum, S. E., Heller, E. D., Sela, S., Elad, D., Edery, N., and Leitner, G. (2015). Genomic and phenomic study of mammary pathogenic Escherichia coli. PLoS One 10:e0136387. doi: 10.1371/journal.pone.0136387
Bobbo, T., Ruegg, P. L., Stocco, G., Fiore, E., Gianesella, M., Morgante, M., et al. (2017). Associations between pathogen-specific cases of subclinical mastitis and milk yield, quality, protein composition, and cheese-making traits in dairy cows. J. Dairy Sci. 100, 4868–4883. doi: 10.3168/jds.2016-12353
Boireau, C., Cazeau, G., Jarrige, N., Calavas, D., Madec, J. Y., Leblond, A., et al. (2018). Antimicrobial resistance in bacteria isolated from mastitis in dairy cattle in France, 2006–2016. J. Dairy Sci. 101, 9451–9462. doi: 10.3168/jds.2018-14835
Bolte, J., Zhang, Y., Wente, N., and Krömker, V. (2020). In vitro susceptibility of mastitis pathogens isolated from clinical mastitis cases on northern german dairy farms. Vet. Sci. 7, 1–16. doi: 10.3390/vetsci7010010
Brade, L., Hensen, S., and Brade, H. (2013). Evaluation of a LPS-based glycoconjugate vaccine against bovine Escherichia coli mastitis: formation of LPS Abs in cows after immunization with E. coli core oligosaccharides conjugated to hemocyanine. Innate Immun. 19, 368–377. doi: 10.1177/1753425912462615
Bradley, A. J., and Green, M. J. (2000). A study of the incidence and significance of intramammary enterobacterial infections acquired during the dry period. J. Dairy Sci. 83, 1957–1965. doi: 10.3168/jds.S0022-0302(00)75072-7
Brandt, M., Haeussermann, A., and Hartung, E. (2010). Invited review: technical solutions for analysis of milk constituents and abnormal milk. J. Dairy Sci. 93, 427–436. doi: 10.3168/jds.2009-2565
Bronzo, V., Lopreiato, V., Riva, F., Amadori, M., Curone, G., Addis, M. F., et al. (2020). The role of innate immune response and microbiome in resilience of dairy cattle to disease: the mastitis model. Animals 10, 1–20. doi: 10.3390/ani10081397
Buitenhuis, B., Røntved, C. M., Edwards, S. M., Ingvartsen, K. L., and Sørensen, P. (2011). In depth analysis of genes and pathways of the mammary gland involved in the pathogenesis of bovine Escherichia coli-mastitis. BMC Genom. 12:130. doi: 10.1186/1471-2164-12-130
Burvenich, C., Merris, V., Mehrzad, J., Diez-Fraile, A., and Duchateau, L. (2003). Severity of E. coli mastitis is mainly determined by cow factors. Vet. Res. 34, 521–564. doi: 10.1051/vetres:2003023
Bush, K., and Jacoby, G. A. (2010). Updated functional classification of β-lactamases. Antimicrob. Agents Chemother. 54, 969–976. doi: 10.1128/AAC.01009-09
Cao, H., Pradhan, A. K., Karns, J. S., Hovingh, E., Wolfgang, D. R., Vinyard, B. T., et al. (2019). Age-associated distribution of antimicrobial-resistant salmonella enterica and Escherichia coli isolated from dairy herds in Pennsylvania, 2013-2015. Foodborne Pathog. Dis. 16, 60–67. doi: 10.1089/fpd.2018.2519
Ceciliani, F., Eckersall, D., Burchmore, R., and Lecchi, C. (2014). Proteomics in veterinary medicine: applications and trends in disease pathogenesis and diagnostics. Vet. Pathol. 51, 351–362. doi: 10.1177/0300985813502819
Chakraborty, S., Dhama, K., Tiwari, R., Iqbal Yatoo, M., Khurana, S. K., Khandia, R., et al. (2019). Technological interventions and advances in the diagnosis of intramammary infections in animals with emphasis on bovine population—a review. Vet Q 39, 76–94. doi: 10.1080/01652176.2019.1642546
Chen, Chen, Wu, N., Rong, N., Kang, C., and Chen, et al. (2020). Immunoprotective evaluation of Escherichia coli outer membrane protein A against the main pathogens of animal mastitis. Trop. J. Pharm. Res. 19, 155–162. doi: 10.4314/tjpr.v19i1.23
Cheng, J., Qu, W., Barkema, H. W., Nobrega, D. B., Gao, J., Liu, G., et al. (2019). Antimicrobial resistance profiles of 5 common bovine mastitis pathogens in large Chinese dairy herds. J. Dairy Sci 102, 2416–2426. doi: 10.3168/jds.2018-15135
Chengolova, Z., Atanasova, M., and Godjevargova, T. (2021). Neutrophil and CD4+milk cell count related to natural incidence of mastitis in Jersey cattle. J. Dairy Sci 88, 334–336. doi: 10.1017/S0022029921000510
Cobirka, M., Tancin, V., and Slama, P. (2020). Epidemiology and classification of mastitis. Animals 10, 1–17. doi: 10.3390/ani10122212
Confer, A. W., and Ayalew, S. (2013). The OmpA family of proteins: roles in bacterial pathogenesis and immunity. Vet. Microbiol 163, 207–222. doi: 10.1016/j.vetmic.2012.08.019
Costa, A., Lopez-Villalobos, N., Sneddon, N. W., Shalloo, L., Franzoi, M., De Marchi, M., et al. (2019). Invited review: milk lactose-current status and future challenges in dairy cattle. J. Dairy Sci. 102, 5883–5898. doi: 10.3168/jds.2018-15955
Croxen, M. A., and Finlay, B. B. (2010). Molecular mechanisms of Escherichia coli pathogenicity. Nat. Rev. Microbiol. 8, 26–38. doi: 10.1038/nrmicro2265
Curone, G., Filipe, J., Cremonesi, P., Trevisi, E., Amadori, M., Pollera, C., et al. (2018). What we have lost: mastitis resistance in Holstein Friesians and in a local cattle breed. Res. Vet. Sci. 116, 88–98. doi: 10.1016/j.rvsc.2017.11.020
Dahmen, S., Métayer, V., Gay, E., Madec, J. Y., and Haenni, M. (2013). Characterization of extended-spectrum beta-lactamase (ESBL)-carrying plasmids and clones of Enterobacteriaceae causing cattle mastitis in France. Vet. Microbiol. 162, 793–799. doi: 10.1016/j.vetmic.2012.10.015
Denamur, E., Clermont, O., Bonacorsi, S., and Gordon, D. (2021). The population genetics of pathogenic Escherichia coli. Nat. Rev. Microbiol. 19, 37–54. doi: 10.1038/s41579-020-0416-x
Dittrich, I., Gertz, M., and Krieter, J. (2019). Alterations in sick dairy cows’ daily behavioural patterns. Heliyon 5:e02902. doi: 10.1016/j.heliyon.2019.e02902
Dogan, B., Klaessig, S., Rishniw, M., Oliver, S., Simpson, K., and Schukken, Y. (2006). Adherent and invasive Escherichia coli are associated with persistent bovine mastitis. Vet. Microbiol. 116, 270–282. doi: 10.1016/j.vetmic.2006.04.023
Dogan, B., Rishniw, M., Bruant, G., Harel, J., Schukken, Y. H., and Simpson, K. W. (2012). Phylogroup and lpfA influence epithelial invasion by mastitis associated Escherichia coli. Vet. Microbiol. 159, 163–170. doi: 10.1016/j.vetmic.2012.03.033
Duarte, C. M., Freitas, P. P., and Bexiga, R. (2015). Technological advances in bovine mastitis diagnosis: an overview. J. Vet. Diagn. Investig. 27, 665–672. doi: 10.1177/1040638715603087
Eckel, E. F., and Ametaj, B. N. (2020). Bacterial endotoxins and their role in periparturient diseases of dairy cows: mucosal vaccine perspectives. MDPI Dairy 1, 61–90. doi: 10.3390/dairy1010006
Eisenberger, D., Carl, A., Balsliemke, J., Kämpf, P., Nickel, S., Schulze, G., et al. (2018). Molecular characterization of extended-spectrum β-lactamase-producing Escherichia coli isolates from milk samples of dairy cows with mastitis in Bavaria, Germany. Microb. Drug Resist. 24, 505–510. doi: 10.1089/mdr.2017.0182
Elazar, S., Gonen, E., Livneh-Kol, A., Rosenshine, I., and Shpigel, N. Y. (2010). Essential role of neutrophils but not mammary alveolar macrophages in a murine model of acute Escherichia coli mastitis. Vet. Res 41:53. doi: 10.1051/vetres/2010025
El-Sayed, A., and Kamel, M. (2021). Bovine mastitis prevention and control in the post-antibiotic era. Trop. Anim. Heal. Prod. 53:236. doi: 10.1007/s11250-021-02680-9
El-Sayed, A., Awad, W., Abdou, N. E., and Castañeda Vázquez, H. (2017). Molecular biological tools applied for identification of mastitis causing pathogens. Int. J. Vet. Sci. 5, 89–97. doi: 10.1016/j.ijvsm.2017.08.002
Elsayed, M. S. A. E., Eldsouky, S. M., Roshdy, T., Bayoume, A. M. A., Nasr, G. M., Salama, A. S. A., et al. (2021). Genetic and antimicrobial resistance profiles of non-O157 shiga toxin-producing Escherichia coli from different sources in Egypt. BMC Microbiol. 21:1–19. doi: 10.1186/s12866-021-02308-w
Erskine, R. J. (2012). Vaccination strategies for mastitis. Vet. Clin. N. Am. - Food Anim. Pr. 28, 257–270. doi: 10.1016/j.cvfa.2012.03.002
Erskine, R. J., VanDyk, E. J., Bartlett, P. C., Burton, J. L., and Boyle, M. C. (2007). Effect of hyperimmunization with an Escherichia coli J5 bacterin in adult lactating dairy cows. J. Am. Vet. Med. Assoc. 231, 1092–1097. doi: 10.2460/javma.231.7.1092
Fairbrother, J. H., Dufour, S., Fairbrother, J. M., Francoz, D., Nadeau, É, and Messier, S. (2015). Characterization of persistent and transient Escherichia coli isolates recovered from clinical mastitis episodes in dairy cows. Vet. Microbiol. 176, 126–133. doi: 10.1016/j.vetmic.2014.12.025
Fazel, F., Jamshidi, A., and Khoramian, B. (2019). Phenotypic and genotypic study on antimicrobial resistance patterns of E. coli isolates from bovine mastitis. Microb. Pathog. 132, 355–361. doi: 10.1016/j.micpath.2019.05.018
Fernandes, J. B. C., Zanardo, L. G., Galvão, N. N., Carvalho, I. A., Nero, L. A., and Moreira, M. A. S. (2011). Escherichia coli from clinical mastitis: serotypes and virulence factors. J. Vet. Diagn. Investig. 23, 1146–1152. doi: 10.1177/1040638711425581
Ferreira, J. C., Gomes, M. S., Bonsaglia, E. C. R., Canisso, I. F., Garrett, E. F., Stewart, J. L., et al. (2018). Comparative analysis of four commercial on-farm culture methods to identify bacteria associated with clinical mastitis in dairy cattle. PLoS One 13:e0194211. doi: 10.1371/journal.pone.0194211
Filioussis, G., Kachrimanidou, M., Christodoulopoulos, G., Kyritsi, M., Hadjichristodoulou, C., Adamopoulou, M., et al. (2020). Short communication: bovine mastitis caused by a multidrug-resistant, mcr-1-positive (colistin-resistant), extended-spectrum β-lactamase–producing Escherichia coli clone on a Greek dairy farm. J. Dairy Sci. 103, 852–857. doi: 10.3168/jds.2019-17320
Finlay, B. B., and Cossart, P. (1997). Exploitation of mammalian host cell functions by bacterial pathogens. Science 276, 718–725. doi: 10.1126/science.276.5313.718
Fitzgerald, D. C., Meade, K. G., McEvoy, A. N., Lillis, L., Murphy, E. P., MacHugh, D. E., et al. (2007). Tumour necrosis factor-α (TNF-α) increases nuclear factor κB (NFκB) activity in and interleukin-8 (IL-8) release from bovine mammary epithelial cells. Vet. Immunol. Immunopathol. 116, 59–68. doi: 10.1016/j.vetimm.2006.12.008
Fosgate, G. T., Petzer, I. M., and Karzis, J. (2013). Sensitivity and specificity of a hand-held milk electrical conductivity meter compared to the California mastitis test for mastitis in dairy cattle. Vet. J. 196, 98–102. doi: 10.1016/j.tvjl.2012.07.026
Freitag, C., Michael, G. B., Kadlec, K., Hassel, M., and Schwarz, S. (2017). Detection of plasmid-borne extended-spectrum β-lactamase (ESBL) genes in Escherichia coli isolates from bovine mastitis. Vet. Microbiol. 200, 151–156. doi: 10.1016/j.vetmic.2016.08.010
Fuenzalida, M. J., and Ruegg, P. L. (2019). Negatively controlled, randomized clinical trial to evaluate intramammary treatment of nonsevere, gram-negative clinical mastitis. J. Dairy Sci. 102, 5438–5457. doi: 10.3168/jds.2018-16156
Gao, J., Barkema, H. W., Zhang, L., Liu, G., Deng, Z., Cai, L., et al. (2017). Incidence of clinical mastitis and distribution of pathogens on large Chinese dairy farms. J. Dairy Sci. 100, 4797–4806. doi: 10.3168/jds.2016-12334
Ghanbarpour, R., and Oswald, E. (2010). Phylogenetic distribution of virulence genes in Escherichia coli isolated from bovine mastitis in Iran. Res. Vet. Sci. 88, 6–10. doi: 10.1016/j.rvsc.2009.06.003
Godden, S. M., Royster, E., Timmerman, J., Rapnicki, P., and Green, H. (2017). Evaluation of an automated milk leukocyte differential test and the California Mastitis Test for detecting intramammary infection in early- and late-lactation quarters and cows. J. Dairy Sci. 100, 6527–6544. doi: 10.3168/jds.2017-12548
Goldstone, R. J., Harris, S., and Smith, D. G. E. (2016). Genomic content typifying a prevalent clade of bovine mastitis-associated Escherichia coli. Sci. Rep. 6, 1–15. doi: 10.1038/srep30115
Gonçalves, J. L., Kamphuis, C., Martins, C. M. M. R., Barreiro, J. R., Tomazi, T., Gameiro, A. H., et al. (2018). Bovine subclinical mastitis reduces milk yield and economic return. Livest. Sci 210, 25–32. doi: 10.1016/j.livsci.2018.01.016
Gonen, E., Vallon-Eberhard, A., Elazar, S., Harmelin, A., Brenner, O., Rosenshine, I., et al. (2007). Toll-like receptor 4 is needed to restrict the invasion of Escherichia coli P4 into mammary gland epithelial cells in a murine model of acute mastitis. Cell Microbiol. 9, 2826–2838. doi: 10.1111/j.1462-5822.2007.00999.x
Greenlee-Wacker, M. C. (2016). Clearance of apoptotic neutrophils and resolution of inflammation. Immunol. Rev. 273, 357–370. doi: 10.1111/imr.12453
Guerra, S. T., Orsi, H., Joaquim, S. F., Guimarães, F. F., Lopes, B. C., Dalanezi, F. M., et al. (2020). Short communication: investigation of extra-intestinal pathogenic Escherichia coli virulence genes, bacterial motility, and multidrug resistance pattern of strains isolated from dairy cows with different severity scores of clinical mastitis. J. Dairy Sci. 103, 3606–3614. doi: 10.3168/jds.2019-17477
Guerra, S., Dalanezi, F., Paula, C., Hernandes, R., Pantoja, J., Listoni, F., et al. (2019). Putative virulence factors of extra-intestinal Escherichia coli isolated from bovine mastitis with different clinical scores. Lett. Appl. Microbiol. 68, 403–408. doi: 10.1111/lam.13113
Günther, J., Petzl, W., Bauer, I., Ponsuksili, S., Zerbe, H., Schuberth, H. J., et al. (2017). Differentiating Staphylococcus aureus from Escherichia coli mastitis: s. aureus triggers unbalanced immune-dampening and host cell invasion immediately after udder infection. Sci. Rep. 7, 1–14. doi: 10.1038/s41598-017-05107-4
Hagiwara, S., Mori, K., Okada, H., Oikawa, S., and Nagahata, H. (2014). Acute Escherichia coli mastitis in dairy cattle: diagnostic parameters associated with poor prognosis. J. Vet. Med. Sci. 76, 1431–1436. doi: 10.1292/jvms.13-0610
Harrison, R. (2006). Milk xanthine oxidase: properties and physiological roles. Int. Dairy J. 16, 546–554. doi: 10.1016/j.idairyj.2005.08.016
Heikkilä, A. M., Liski, E., Pyörälä, S., and Taponen, S. (2018). Pathogen-specific production losses in bovine mastitis. J. Dairy Sci. 101, 9493–9504. doi: 10.3168/jds.2018-14824
Herry, V., Gitton, C., Tabouret, G., Répérant, M., Forge, L., Tasca, C., et al. (2017). Local immunization impacts the response of dairy cows to Escherichia coli mastitis. Sci. Rep. 7, 1–18. doi: 10.1038/s41598-017-03724-7
Hogan, J., and Smith, K. (2003). Coliform mastitis. Vet. Res. 34, 507–519. doi: 10.1051/vetres:2003022
Hogan, J., and Smith, K. L. (2012). Managing environmental mastitis. Vet. Clin. North Am. Food. Anim. Pr. 28, 217–224. doi: 10.1016/j.cvfa.2012.03.009
Hogeveen, H., Steeneveld, W., and Wolf, C. A. (2019). Production diseases reduce the efficiency of dairy production: a review of the results, methods, and approaches regarding the economics of mastitis. Annu. Rev. Resour. Econ. 11, 289–312. doi: 10.1146/annurev-resource-100518-093954
Holko, I., Tančin, V., Vršková, M., and Tvarožková, K. (2019). Prevalence and antimicrobial susceptibility of udder pathogens isolated from dairy cows in Slovakia. J. Dairy Res. 86, 436–439. doi: 10.1017/S0022029919000694
Hsieh, W. S., Yang, Y. Y., Lin, P. H., Chang, C. C., and Wu, H. H. (2016). Recombinant OmpA protein fragments mediate interleukin-17 regulation to prevent Escherichia coli meningitis. J. Microbiol. Immunol. Infect. 49, 843–850. doi: 10.1016/j.jmii.2015.05.019
Hu, H., Fang, Z., Mu, T., Wang, Z., Ma, Y., and Ma, Y. (2021). Application of metabolomics in diagnosis of cow mastitis: a review. Front. Vet. Sci. 8:747519. doi: 10.3389/fvets.2021.747519
Ingvartsen, K. L., and Moyes, K. M. (2015). Factors contributing to immunosuppression in the dairy cow during the periparturient period. J. Vet. Res. 63, 15–24. doi: 10.14943/jjvr.63.suppl.s15
Ismail, Z. B. (2017). Mastitis vaccines in dairy cows: recent developments and recommendations of application. Vet. World 10, 1057–1062. doi: 10.14202/vetworld.2017.1057-1062
Jacoby, G. A. (2009). AmpC B -Lactamases. Clin. Microbiol. Rev. 22, 161–182. doi: 10.1128/CMR.00036-08
Kaipainen, T., Pohjanvirta, T., Shpigel, N. Y., Shwimmer, A., Pyörälä, S., and Pelkonen, S. (2002). Virulence factors of Escherichia coli isolated from bovine clinical mastitis. Vet. Microbiol. 85, 37–46. doi: 10.1016/S0378-1135(01)00483-7
Karav, S., German, J. B., Rouquié, C., Le Parc, A., and Barile, D. (2017). Studying lactoferrin N-glycosylation. Int. J. Mol. Sci. 18, 1–14. doi: 10.3390/ijms18040870
Kawai, K., Kondo, Y., Shinozuka, Y., Kawata, R., Kaneko, S., Iwano, H., et al. (2020). Immune response during the onset of coliform mastitis in dairy cows vaccinated with STARTVAC®. J. Anim. Sci. 92:e13502. doi: 10.1111/asj.13502
Kawai, K., Korematsu, K., Akiyama, K., Okita, M., Yoshimura, Y., and Isobe, N. (2015). Dynamics of lingual antimicrobial peptide, lactoferrin concentrations and lactoperoxidase activity in the milk of cows treated for clinical mastitis. Anim. Sci. J. 86, 153–158. doi: 10.1111/asj.12269
Kayano, M., Itoh, M., Kusaba, N., Hayashiguchi, O., Kida, K., Tanaka, Y., et al. (2018). Associations of the first occurrence of pathogen-specific clinical mastitis with milk yield and milk composition in dairy cows. J. Dairy Res. 85, 309–316. doi: 10.1017/S0022029918000456
Kayitsinga, J., Schewe, R. L., Contreras, G. A., and Erskine, R. J. (2017). Antimicrobial treatment of clinical mastitis in the eastern United States: the influence of dairy farmers’ mastitis management and treatment behavior and attitudes. J. Dairy Sci. 100, 1388–1407. doi: 10.3168/jds.2016-11708
Kelly, A. L., Leitner, G., and Merin, U. (2011). “Milk quality and udder health: Test methods and standards’,” in Encyclopedia of Dairy Science, eds J. W. Fuquay, P. F. Fox, and P. L. H. McSweeney (Cambridge, MA: Academic Press), 894–901. doi: 10.1016/B978-0-12-374407-4.00353-8
Kempf, F., Slugocki, C., Blum, S. E., Leitner, G., and Germon, P. (2016). Genomic comparative study of bovine mastitis Escherichia coli. PLoS One 11:e0147954. doi: 10.1371/journal.pone.0147954
Kerro Dego, O., Oliver, S. P., and Almeida, R. A. (2012). Host-pathogen gene expression profiles during infection of primary bovine mammary epithelial cells with Escherichia coli strains associated with acute or persistent bovine mastitis. Vet. Microbiol. 155, 291–297. doi: 10.1016/j.vetmic.2011.08.016
Kester, H. J., Sorter, D. E., and Hogan, J. S. (2015). Activity and milk compositional changes following experimentally induced Streptococcus uberis bovine mastitis. J. Dairy Sci. 98, 999–1004. doi: 10.3168/jds.2014-8576
Khan, M. Z., Khan, A., Xiao, J., Ma, J., Ma, Y., Chen, T., et al. (2020). Overview of research development on the role of NF-κB signaling in mastitis. Animals 10, 1–16. doi: 10.3390/ani10091625
Kieckens, E., Rybarczyk, J., Cox, E., and Vanrompay, D. (2018). Antibacterial and immunomodulatory activities of bovine lactoferrin against Escherichia coli O157:H7 infections in cattle. BioMetals 31, 321–330. doi: 10.1007/s10534-018-0082-x
Klaas, I. C., and Zadoks, R. N. (2018). An update on environmental mastitis: challenging perceptions. Transbound. Emerg. Dis. 65, 166–185. doi: 10.1111/tbed.12704
Koshiishi, T., Watanabe, M., Miyake, H., Hisaeda, K., and Isobe, N. (2017). Cellular and soluble components decrease the viable pathogen counts in milk from dairy cows with subclinical mastitis. J. Vet. Med. Sci. 79, 1389–1393. doi: 10.1292/jvms.17-0269
Krishnan, S., and Prasadarao, N. V. (2012). Outer membrane protein A and OprF: versatile roles in Gram-negative bacterial infections. FEBS J. 279, 919–931. doi: 10.1111/j.1742-4658.2012.08482.x
Krömker, V., and Leimbach, S. (2017). Mastitis treatment—Reduction in antibiotic usage in dairy cows. Reprod. Domest. Anim. 52, 21–29. doi: 10.1111/rda.13032
Kuhi, M., Aminlari, M., and Tavana, M. (2021). Antibacterial action of dextran conjugated lysozyme against bacteria involved in bovine mastitis. Adv. Dairy. Res. 9, 1–6.
Kupczyński, R., Bednarski, M., Śpitalniak, K., and Pogoda-Sewerniak, K. (2017). Effects of protein-iron complex concentrate supplementation on iron metabolism, oxidative and immune status in preweaning calves. Int. J. Mol. Sci. 18, 1501–1512. doi: 10.3390/ijms18071501
Lauzon, K., Zhao, X., Bouetard, A., Delbecchi, L., Paquette, B., and Lacasse, P. (2005). Antioxidants to prevent bovine neutrophil-induced mammary epithelial cell damage. J. Dairy Sci. 88, 4295–4303. doi: 10.3168/jds.S0022-0302(05)73115-5
Le Gall, T., Clermont, O., Gouriou, S., Picard, B., Nassif, X., Denamur, E., et al. (2007). Extraintestinal virulence is a coincidental by-product of commensalism in b2 phylogenetic group Escherichia coli strains. Mol. Biol. Evol. 24, 2373–2384. doi: 10.1093/molbev/msm172
Leblanc, S. J. (2020). Relationships between metabolism and neutrophil function in dairy cows in the peripartum period. Animal 14, 44–54. doi: 10.1017/S1751731119003227
Lee, K. H., Lee, J. W., Wang, S. W., Liu, L. Y., Lee, M. F., Chuang, S. T., et al. (2008). Development of a novel biochip for rapid multiplex detection of seven mastitis-causing pathogens in bovine milk samples. J. Vet. Diagn. Investig. 20, 463–471. doi: 10.1177/104063870802000408
Leimbach, A., Poehlein, A., Vollmers, J., Görlich, D., Daniel, R., and Dobrindt, U. (2017). No evidence for a bovine mastitis Escherichia coli pathotype. BMC Genom. 18:1–22. doi: 10.1186/s12864-017-3739-x
Leung, K. T., Mackereth, R., Tien, Y. C., and Topp, E. (2004). A comparison of AFLP and ERIC-PCR analyses for discriminating Escherichia coli from cattle, pig and human sources. FEMS Microbiol. Ecol. 47, 111–119. doi: 10.1016/S0168-6496(03)00254-X
Li, L., Chen, X., and Chen, Z. (2019). Identification of key candidate genes in dairy cow in response to Escherichia coli mastitis by bioinformatical analysis. Front. Genet. 10:1251. doi: 10.3389/fgene.2019.01251
Li, Q., Chang, W., Zhang, H., Hu, D., and Wang, X. (2019). The role of plasmids in the multiple antibiotic resistance transfer in ESBLs-producing Escherichia coli isolated from wastewater treatment plants. Front. Microbiol. 10:633. doi: 10.3389/fmicb.2019.00633
Li, W., Xue, M., Yu, L., Qi, K., Ni, J., Chen, X., et al. (2020). QseBC is involved in the biofilm formation and antibiotic resistance in Escherichia coli isolated from bovine mastitis. Peer J. 8, 1–18. doi: 10.7717/peerj.8833
Liang, D., Arnold, L. M., Stowe, C. J., Harmon, R. J., and Bewley, J. M. (2017). Estimating US dairy clinical disease costs with a stochastic simulation model. J. Dairy Sci. 100, 1472–1486. doi: 10.3168/jds.2016-11565
Lind, M., Sipka, A. S., Schuberth, H. J., Blutke, A., Wanke, R., Sauter-Louis, C., et al. (2015). Location-specific expression of chemokines. TNF-α and S100 proteins in a teat explant model. Innate Immun. 21, 322–331. doi: 10.1177/1753425914539820
Lippolis, J. D., Bayles, D. O., and Reinhardt, T. A. (2009). Proteomic changes in Escherichia coli when grown in fresh milk versus laboratory media. J. Proteome Res. 8, 149–158. doi: 10.1021/pr800458v
Lippolis, J. D., Brunelle, B. W., Reinhardt, T. A., Sacco, R. E., Nonnecke, B. J., Dogan, B., et al. (2014). Proteomic analysis reveals protein expression differences in Escherichia coli strains associated with persistent versus transient mastitis. J. Proteom. 108, 373–381. doi: 10.1016/j.jprot.2014.06.008
Liu, X., Sun, W., Wu, N., Rong, N., Kang, C., Jian, S., et al. (2021). Synthesis of Escherichia coli ompA oral nanoparticles and evaluation of immune functions against the major etiologic agent of cow mastitis. Vaccines 9:304. doi: 10.3390/vaccines9030304
Liu, Y., Liu, G., Liu, W., Liu, Y., Ali, T., Chen, W., et al. (2014). Phylogenetic group, virulence factors and antimicrobial resistance of Escherichia coli associated with bovine mastitis. Res. Microbiol. 165, 273–277. doi: 10.1016/j.resmic.2014.03.007
Maity, S., and Ambatipudi, K. (2021). Mammary microbial dysbiosis leads to the zoonosis of bovine mastitis: a One-Health perspective. FEMS Microbiol. Ecol. 97, fiaa241. doi: 10.1093/femsec/fiaa241
Majumder, S., Jung, D., Ronholm, J., and George, S. (2021). Prevalence and mechanisms of antibiotic resistance in Escherichia coli isolated from mastitic dairy cattle in Canada. BMC Microbiol. 21:1–14. doi: 10.1186/s12866-021-02301-3
Makovec, J. A., and Ruegg, P. L. (2003). Results of milk samples submitted for microbiological examination in Wisconsin from 1994 to 2001. J. Dairy Sci. 86, 3466–3472. doi: 10.3168/jds.S0022-0302(03)73951-4
Malik, T. A., Mohini, M., Mir, S. H., Ganaie, B. A., Singh, D., Varun, T. K., et al. (2018). Somatic cells in relation to udder health and milk quality-A review. J. Anim. Heal. Prod. 6, 18–26. doi: 10.17582/journal.jahp/2018/6.1.18.26
Martins, L., Barcelos, M. M., Cue, R. I., Anderson, K. L., and Santos, M. V. (2020). Chronic subclinical mastitis reduces milk and components yield at the cow level. J. Dairy Res. 87, 298–305. doi: 10.1017/S0022029920000321
Mathew, A. G., Cissell, R., and Liamthong, S. (2007). Antibiotic resistance in bacteria associated with food animals: a United States perspective of livestock production. Foodborne Pathog. Dis. 4, 115–133. doi: 10.1089/fpd.2006.0066
Medrano-Galarza, C., Gibbons, J., Wagner, S., de Passillé, A. M., and Rushen, J. (2012). Behavioral changes in dairy cows with mastitis. J. Dairy Sci. 95, 6994–7002. doi: 10.3168/jds.2011-5247
Mehrzad, J., Desrosiers, C., Lauzon, K., Robitaille, G., Zhao, X., and Lacasse, P. (2005). Proteases involved in mammary tissue damage during endotoxin-lnduced mastitis in dairy cows. J. Dairy Sci. 88, 211–222. doi: 10.3168/jds.S0022-0302(05)72679-5
Mehrzad, J., Duchateau, L., and Burvenich, C. (2004). Viability of milk neutrophils and severity of bovine coliform mastitis. J. Dairy Sci. 87, 4150–4162. doi: 10.3168/jds.S0022-0302(04)73558-4
Melchior, M. B., Vaarkamp, H., and Fink-Gremmels, J. (2006). Biofilms: a role in recurrent mastitis infections? Vet. J. 171, 398–407. doi: 10.1016/j.tvjl.2005.01.006
Mellata, M. (2013). Human and avian extraintestinal pathogenic Escherichia coli: infections, zoonotic risks, and antibiotic resistance trends. Foodborne Pathog. Dis. 10, 916–922. doi: 10.1089/fpd.2013.1533
Méric, G., Hitchings, M. D., Pascoe, B., and Sheppard, S. K. (2016). From Escherichia to the Escherichia coli genome. Lancet Infect. Dis. 16, 634–636. doi: 10.1016/S1473-3099(16)30066-4
Mitterhuemer, S., Petzl, W., Krebs, S., Mehne, D., Klanner, A., Wolf, E., et al. (2010). Escherichia coli infection induces distinct local and systemic transcriptome responses in the mammary gland. BMC Genom. 11:138. doi: 10.1186/1471-2164-11-138
Mohammed, A. N., Radi, A. M., Khaled, R., Abo El-Ela, F. I., and Kotp, A. A. (2020). Exploitation of new approach to control of environmental pathogenic bacteria causing bovine clinical mastitis using novel anti-biofilm nanocomposite. Environ. Sci. Pollut. Res. 27, 42791–42805. doi: 10.1007/s11356-020-10054-1
Momtaz, H. (2010). Investigation of virulence factors in Escherichia coli isolated from clinical and subclinical bovine mastitis. Bulg. J. Vet. Med. 13, 122–126.
Momtaz, H., Safarpoor Dehkordi, F., Taktaz, T., Rezvani, A., and Yarali, S. (2012). Shiga toxin-producing Escherichia coli isolated from bovine mastitic milk: serogroups, virulence factors, and antibiotic resistance properties. Sci. World J. 2012:618709. doi: 10.1100/2012/618709
Ngaywa, C., Aboge, G. O., Obiero, G., Omwenga, I., Ngwili, N., Wamwere, G., et al. (2019). Antimicrobial resistant Escherichia coli isolates detected in raw milk of livestock in pastoral areas of northern Kenya. Food Control 102, 173–178. doi: 10.1016/j.foodcont.2019.03.008
Oliveira, L., and Ruegg, P. L. (2014). Treatments of clinical mastitis occurring in cows on 51 large dairy herds in Wisconsin. J. Dairy Sci. 97, 5426–5436. doi: 10.3168/jds.2013-7756
Oliveira, L., Hulland, C., and Ruegg, P. L. (2013). Characterization of clinical mastitis occurring in cows on 50 large dairy herds in Wisconsin. J. Dairy Sci. 96, 7538–7549. doi: 10.3168/jds.2012-6078
Olson, M. A., Siebach, T. W., Griffitts, J. S., Wilson, E., Erickson, D. L., and Pinheiro, J. O. O. (2018). Genome-wide identification of fitness factors in mastitis-associated Escherichia coli. Appl. Environ. Microbiol. 84:e02190-17. doi: 10.1128/AEM.02190-17
Olson, M. E., Ceri, H., Morck, D. W., Buret, A. G., and Read, R. R. (2002). Biofilm bacteria: formation and comparative susceptibility to antibiotics. Can. J. Vet. Res. 66, 86–92.
Ombarak, R. A., Hinenoya, A., Elbagory, A. R. M., and Yamasaki, S. (2018). Prevalence and molecular characterization of antimicrobial resistance in Escherichia coli isolated from raw milk and raw milk cheese in Egypt. J. Food Prot. 81, 226–232. doi: 10.4315/0362-028X.JFP-17-277
Opijnen, T., Bodi, K. L., and Camilli, A. (2009). Tn-seq: high-throughput parallel sequencing for fitness and genetic interaction studies in microorganisms. Nat. Methods 6, 767–772. doi: 10.1038/nmeth.1377
Paape, M., Bannerman, D., Zhao, X., and Lee, J. (2003). The bovine neutrophil: structure and function in blood and milk. Vet. Res. 34, 597–627.
Padmini, N., Ajilda, A. A. K., Sivakumar, N., and Selvakumar, G. (2017). Extended spectrum β-lactamase producing Escherichia coli and Klebsiella pneumoniae: critical tools for antibiotic resistance pattern. J. Basic Microbiol. 57, 460–470. doi: 10.1002/jobm.201700008
Panda, B. S. K., Mohapatra, S. K., Alhussien, M. N., and Dang, A. K. (2019). Amount of milk neutrophil percentage and associated CD molecular changes on the compositional and technological properties of milk. Open Biotechnol. J. 13, 129–136. doi: 10.2174/187407070190130129
Passey, S., Bradley, A., and Mellor, H. (2008). Escherichia coli isolated from bovine mastitis invade mammary cells by a modified endocytic pathway. Vet. Microbiol. 130, 151–164. doi: 10.1016/j.vetmic.2008.01.003
Pedersen, R. R., Krömker, V., Bjarnsholt, T., Dahl-Pedersen, K., Buhl, R., and Jørgensen, E. (2021). Biofilm research in bovine mastitis. Front. Vet. Sci. 8:656810. doi: 10.3389/fvets.2021.656810
Peek, C. T., Ibberson, C. B., and Cassat, J. E. (2020). “Identification of virulence determinants during host-pathogen interaction using Tn-Seq technology’,” in Methicillin-Resistant Staphylococcus Aureus (MRSA) Protocols-Methods in Molecular Biology, ed. Y. Ji (New York, NY: Humana), 155–175. doi: 10.1007/978-1-4939-9849-4_12
Persson, Y., Katholm, J., Landin, H., and Mörk, M. J. (2015). Efficacy of enrofloxacin for the treatment of acute clinical mastitis caused by Escherichia coli in dairy cows. Vet. Rec. 176:673. doi: 10.1136/vr.102667
Petzl, W., Zerbe, H., Günther, J., Seyfert, H. M., Hussen, J., and Schuberth, H. J. (2018). Pathogen-specific responses in the bovine udder. Models and immunoprophylactic concepts. Res. Vet. Sci. 116, 55–61. doi: 10.1016/j.rvsc.2017.12.012
Pezeshki, A., Stordeur, P., Wallemacq, H., Schynts, F., Stevens, M., Boutet, P., et al. (2011). Variation of inflammatory dynamics and mediators in primiparous cows after intramammary challenge with Escherichia coli. Vet. Res. 42, 15. doi: 10.1186/1297-9716-42-15
Pomeroy, B., Gurjar, A., Sipka, A., Klaessig, S., Salmon, S., Quesnell, R., et al. (2016). Intramammary immunization with ultraviolet-killed Escherichia coli shows partial protection against late gestation intramammary challenge with a homologous strain. J. Dairy Sci. 99, 9014–9026. doi: 10.3168/jds.2016-11149
Porcherie, A., Cunha, P., Trotereau, A., Roussel, P., Gilbert, F. B., Rainard, P., et al. (2012). Repertoire of Escherichia coli agonists sensed by innate immunity receptors of the bovine udder and mammary epithelial cells. Vet. Res. 43:14. doi: 10.1186/1297-9716-43-14
Radostitis, O., Gay, C., Hinchcliff, K., and Constable, P. (2007). Veterinary Medicine: A Textbook of the Diseases of Cattle, Sheep, Pigs, Goats and Horses. Philadelphia: Saunders, 2180.
Rainard, P., and Riollet, C. (2006). Innate immunity of the bovine mammary gland. Vet. Res. 37, 369–400. doi: 10.1051/vetres:2006007
Rainard, P., Gilbert, F. B., Germon, P., and Foucras, G. (2021a).. Invited review: a critical appraisal of mastitis vaccines for dairy cows. J. Dairy Sci. 104, 10427–10448. doi: 10.3168/jds.2021-20434
Rainard, P., Gilbert, F. B., Martins, R. P., Germon, P., and Foucras, G. (2022). Progress towards the elusive mastitis vaccines. Vaccines 10, 1–24. doi: 10.3390/vaccines10020296
Rainard, P., Repérant-Ferter, M., Gitton, C., and Germon, P. (2021b). Shielding effect of Escherichia coli O-antigen polysaccharide on J5-induced cross-reactive antibodies. mSphere 6:e1227–e1220. doi: 10.1128/msphere.01227-20
Rainard, P., Répérant-Ferter, M., Gitton, C., Gilbert, F. B., and Germon, P. (2017). Cellular and humoral immune response to recombinant Escherichia coli OmpA in cows. PLoS One 12:e0187369. doi: 10.1371/journal.pone.0187369
Richards, V. P., Lefébure, T., Bitar, P. D. P., Dogan, B., Simpson, K. W., Schukken, Y. H., et al. (2015). Genome based phylogeny and comparative genomic analysis of intra-mammary pathogenic Escherichia coli. PLoS One 10:e0119799. doi: 10.1371/journal.pone.0119799
Rinaldi, M., Li, R. W., Bannerman, D. D., Daniels, K. M., Evock-Clover, C., Silva, M. V. B., et al. (2010). A sentinel function for teat tissues in dairy cows: dominant innate immune response elements define early response to E. coli mastitis. Funct. Integr. Genom. 10, 21–38. doi: 10.1007/s10142-009-0133-z
Rocha, L. S., Silva, D. M., Silva, M. P., Vidigal, P. M. P., Silva, J. C. F., Guerra, S. T., et al. (2019). Comparative genomics of Staphylococcus aureus associated with subclinical and clinical bovine mastitis. PLoS One 14:e0220804. doi: 10.1371/journal.pone.0220804
Rollin, E., Dhuyvetter, K. C., and Overton, M. W. (2015). The cost of clinical mastitis in the first 30 days of lactation: an economic modeling tool. Prev. Vet. Med. 122, 257–264. doi: 10.1016/j.prevetmed.2015.11.006
Romero, J., Benavides, E., and Meza, C. (2018). Assessing financial impacts of subclinical mastitis on Colombian dairy farms. Front. Vet. Sci 5:273. doi: 10.3389/fvets.2018.00273
Roussel, P., Cunha, P., Porcherie, A., Petzl, W., Gilbert, F. B., Riollet, C., et al. (2015). Investigating the contribution of IL-17A and IL-17F to the host response during Escherichia coli mastitis. Vet. Res. 46:56. doi: 10.1186/s13567-015-0201-4
Roussel, P., Porcherie, A., Répérant-Ferter, M., Cunha, P., Gitton, C., Rainard, P., et al. (2017). Escherichia coli mastitis strains: in vitro phenotypes and severity of infection in vivo. PLoS One 12:e0178285. doi: 10.1371/journal.pone.0178285
Royster, E., Godden, S., Goulart, D., Dahlke, A., Rapnicki, P., and Timmerman, J. (2014). Evaluation of the Minnesota Easy Culture System II Bi-Plate and Tri-Plate for identification of common mastitis pathogens in milk. J. Dairy Sci. 97, 3648–3659. doi: 10.3168/jds.2013-7748
Rudenko, P., Sachivkina, N., Vatnikov, Y., Shabunin, S., Engashev, S., Kontsevaya, S., et al. (2021). Role of microorganisms isolated from cows with mastitis in Moscow region in biofilm formation. Vet. World 14, 40–48. doi: 10.14202/VETWORLD.2021.40-48
Ruegg, P. L. (2017). A 100-Year Review: mastitis detection, management, and prevention. J. Dairy Sci. 100, 10381–10397. doi: 10.3168/jds.2017-13023
Ruegg, P. L. (2018). Making antibiotic treatment decisions for clinical mastitis. Vet. Clin. N. Am. - Food Anim. Pr. 34, 413–425. doi: 10.1016/j.cvfa.2018.06.002
Rybarczyk, J., Kieckens, E., Vanrompay, D., and Cox, E. (2017). In vitro and in vivo studies on the antimicrobial effect of lactoferrin against Escherichia coli O157:H7. Vet. Microbiol. 202, 23–28. doi: 10.1016/j.vetmic.2016.05.010
Salamon, H., Nissim-Eliraz, E., Ardronai, O., Nissan, I., and Shpigel, N. Y. (2020). The role of O-polysaccharide chain and complement resistance of Escherichia coli in mammary virulence. Vet. Res. 51:77. doi: 10.1186/s13567-020-00804-x
Schmid, A., Hörmansdorfer, S., Messelhäusser, U., Käsbohrer, A., Sauter-Louis, C., and Mansfeld, R. (2013). Prevalence of extended-spectrum β-lactamase-producing Escherichia coli on Bavarian dairy and beef cattle farms. Appl. Environ. Microbiol. 79, 3027–3032. doi: 10.1128/AEM.00204-13
Schukken, Y., Chuff, M., Moroni, P., Gurjar, A., Santisteban, C., Welcome, F., et al. (2012). The “other” gram-negative bacteria in aastitis: Klebsiella, Serratia, and more. Vet. Clin. North Am. Food Anim. Pract. 28, 239–256. doi: 10.1016/j.cvfa.2012.04.001
Schukken, Y. H., Günther, J., Fitzpatrick, J., Fontaine, M. C., Goetze, L., Holst, O., et al. (2011). Host-response patterns of intramammary infections in dairy cows. Vet. Immunol. Immunopathol. 144, 270–289. doi: 10.1016/j.vetimm.2011.08.022
Seegers, H., Fourichon, C., and Beaudeau, F. (2003). Production effects related to mastitis and mastitis economics in dairy cattle herds. Vet. Res. 34, 475–491. doi: 10.1051/vetres:2003027
Sepúlveda-Varas, P., Proudfoot, K. L., Weary, D. M., and von Keyserlingk, M. A. G. (2016). Changes in behaviour of dairy cows with clinical mastitis. Appl. Anim. Behav. Sci. 175, 8–13. doi: 10.1016/j.applanim.2014.09.022
Shaheen, T., Bilal Ahmad, S., Rehman, M. U., Muzamil, S., Razak Bhat, R., Hussain, I., et al. (2020). Investigations on cytokines and proteins in lactating cows with and without naturally occurring mastitis. J. King Saud. Univ. Sci. 32, 2863–2867. doi: 10.1016/j.jksus.2020.07.009
Sharifi, S., Pakdel, A., Ebrahimi, M., Reecy, J. M., Farsani, S. F., and Ebrahimie, E. (2018). Integration of machine learning and metaanalysis identifies the transcriptomic bio-signature of mastitis disease in cattle. PLoS One 13:e0191227. doi: 10.1371/journal.pone.0191227
Shimazaki, K. I., and Kawai, K. (2017). Advances in lactoferrin research concerning bovine mastitis. Biochem. Cell Biol. 95, 69–75. doi: 10.1139/bcb-2016-0044
Shpigel, N. Y., Elazar, S., and Rosenshine, I. (2008). Mammary pathogenic Escherichia coli. Curr. Opin. Microbiol. 11, 60–65. doi: 10.1016/j.mib.2008.01.004
Singh, K., Chandra, M., Kaur, G., Narang, D., and Gupta, D. K. (2018). Prevalence and antibiotic resistance pattern among the mastitis causing microorganisms. Open J. Vet. Med. 8, 54–64. doi: 10.4236/ojvm.2018.84007
Sintes, G. F., Bruckmaier, R. M., and Wellnitz, O. (2020). Nonsteroidal anti-inflammatory drugs affect the mammary epithelial barrier during inflammation. J. Dairy Sci. 103, 10742–10753. doi: 10.3168/jds.2020-18818
Skarbye, A. P., Krogh, M. A., and Sørensen, J. T. (2018). The effect of individual quarter dry-off in management of subclinical mastitis on udder condition and milk production in organic dairy herds: a randomized field trial. J. Dairy Sci. 101, 11186–11198. doi: 10.3168/jds.2018-14794
Skočková, A., Bogdanovičová, K., Koláčková, I., and Karpíšková, R. (2015). Antimicrobial-resistant and extended-spectrum β-lactamase-producing Escherichia coli in raw cow’s milk. J. Food Prot. 78, 72–77. doi: 10.4315/0362-028X.JFP-14-250
Sladek, Z., Rysanek, D., Ryznarova, H., and Faldyna, M. (2005). Neutrophil apoptosis during experimentally induced Staphylococcus aureus mastitis. Vet. Res. 36, 629–643. doi: 10.1051/vetres:2005023
Sordillo, L. M. (2005). Factors affecting mammary gland immunity and mastitis susceptibility. Livest. Prod. Sci. 98, 89–99. doi: 10.1016/j.livprodsci.2005.10.017
Sordillo, L. M. (2018). Mammary gland immunobiology and resistance to mastitis. Vet. Clin. N. Am. - Food Anim. Pr. 34, 507–523. doi: 10.1016/j.cvfa.2018.07.005
Sordillo, L. M., Shafer-Weaver, K., and DeRosa, D. (1997). Immunobiology of the mammary gland. J. Dairy Sci. 80, 1851–1865. doi: 10.3168/jds.S0022-0302(97)76121-6
Srinivasan, V., Gillespie, B. E., Lewis, M. J., Nguyen, L. T., Headrick, S. I., Schukken, Y. H., et al. (2007). Phenotypic and genotypic antimicrobial resistance patterns of Escherichia coli isolated from dairy cows with mastitis. Vet. Microbiol. 124, 319–328. doi: 10.1016/j.vetmic.2007.04.040
Steele, N. M., Swartz, T. H., Enger, K. M., Schramm, H., Cockrum, R. R., Lacy-Hulbert, S. J., et al. (2019). The effect of J5 bacterins on clinical, behavioral, and antibody response following an Escherichia coli intramammary challenge in dairy cows at peak lactation. J. Dairy Sci. 102, 11233–11249. doi: 10.3168/jds.2019-16549
Sugrue, I., Tobin, C., Ross, R. P., Stanton, C., and Hill, C. (2019). “Foodborne pathogens and zoonotic diseases’,” in Raw Milk - Balance Between Hazards and Benefits, eds L. A. Nero and A. F. Carvalho (London, UK: Academic Press), 259–272.
Sun, M., Gao, X., Zhao, K., Ma, J., Yao, H., and Pan, Z. (2021). Insight into the virulence related secretion systems, fimbriae, and toxins in O2:K1 Escherichia coli isolated from bovine mastitis. Front. Vet. Sci. 8:622725. doi: 10.3389/fvets.2021.622725
Suojala, L., Kaartinen, L., and Pyörälä, S. (2013). Treatment for bovine Escherichia coli mastitis - An evidence-based approach. J. Vet. Pharmacol. Ther. 36, 521–531. doi: 10.1111/jvp.12057
Suojala, L., Pohjanvirta, T., Simojoki, H., Myllyniemi, A. L., Pitkälä, A., Pelkonen, S., et al. (2011). Phylogeny, virulence factors and antimicrobial susceptibility of Escherichia coli isolated in clinical bovine mastitis. Vet. Microbiol. 147, 383–388. doi: 10.1016/j.vetmic.2010.07.011
Suojala, L., Simojoki, H., Mustonen, K., Kaartinen, L., and Pyörälä, S. (2010). Efficacy of enrofloxacin in the treatment of naturally occurring acute clinical Escherichia coli mastitis. J. Dairy Sci. 93, 1960–1969. doi: 10.3168/jds.2009-2462
Swain, D. K., Kushwah, M. S., Kaur, M., Patbandha, T. K., Mohanty, A. K., and Dang, A. K. (2014). Formation of NET, phagocytic activity, surface architecture, apoptosis and expression of toll like receptors 2 and 4 (TLR2 and TLR4) in neutrophils of mastitic cows. Vet. Res. Commun. 38, 209–219. doi: 10.1007/s11259-014-9606-1
Swinkels, J. M., Leach, K. A., Breen, J. E., Payne, B., White, V., and Green, et al. (2021). Randomized controlled field trial comparing quarter and cow level selective dry cow treatment using the California Mastitis Test. J. Dairy Sci. 104, 9063–9081. doi: 10.3168/jds.2020-19258
Tang, K. L., Caffrey, N. P., Nóbrega, D. B., Cork, S. C., Ronksley, P. E., Barkema, H. W., et al. (2017). Restricting the use of antibiotics in food-producing animals and its associations with antibiotic resistance in food-producing animals and human beings: a systematic review and meta-analysis. Lancet Planet. Heal. 1, 316–327. doi: 10.1016/S2542-5196(17)30141-9
Taniguchi, T., Latt, K. M., Tarigan, E., Yano, F., Sato, H., Minamino, T., et al. (2021). A 1-year investigation of extended-spectrum beta-lactamase-producing Escherichia coli and Klebsiella pneumoniae isolated from bovine mastitis at a large-scale dairy farm in Japan. Microb. Drug Resist. 27, 1450–1454. doi: 10.1089/mdr.2020.0481
Tanih, N. F., Sekwadi, E., Ndip, R. N., and Bessong, P. O. (2015). Detection of pathogenic Escherichia coli and Staphylococcus aureus from cattle and pigs slaughtered in abattoirs in Vhembe District. South Africa. Sci. World J. 2015:195972. doi: 10.1155/2015/195972
Taponen, S., Salmikivi, L., Simojoki, H., Koskinen, M. T., and Pyörälä, S. (2009). Real-time polymerase chain reaction-based identification of bacteria in milk samples from bovine clinical mastitis with no growth in conventional culturing. J. Dairy Sci. 92, 2610–2617. doi: 10.3168/jds.2008-1729
Tenaillon, O., Skurnik, D., Picard, B., and Denamur, E. (2010). The population genetics of commensal Escherichia coli. Nat. Rev. Microbiol. 8, 207–217. doi: 10.1038/nrmicro2298
Thompson-Crispi, K., Atalla, H., Miglior, F., and Mallard, B. A. (2014). Bovine mastitis: frontiers in immunogenetics. Front. Immunol. 5:493. doi: 10.3389/fimmu.2014.00493
Tian, L., Choi, S. C., Lee, H. N., Murakami, Y., Qi, C. F., Sengottuvelu, M., et al. (2016). Enhanced efferocytosis by dendritic cells underlies memory T-cell expansion and susceptibility to autoimmune disease in CD300f-deficient mice. Cell Death. Differ. 23, 1086–1096. doi: 10.1038/cdd.2015.161
Todorović, D., Velhner, M., Grego, E., Vidanović, D., Milanov, D., Krnjaić, D., et al. (2018). Molecular characterization of multidrug-resistant Escherichia coli isolates from bovine clinical mastitis and pigs in the Vojvodina province. Serbia. Microb. Drug Resist. 24, 95–103. doi: 10.1089/mdr.2017.0016
Tomazi, T., Gonçalves, J. L., Barreiro, J. R., Arcari, M. A., and dos Santos, M. V. (2015). Bovine subclinical intramammary infection caused by coagulase-negative staphylococci increases somatic cell count but has no effect on milk yield or composition. J. Dairy Sci. 98, 3071–3078. doi: 10.3168/jds.2014-8466
Tong, J., Zhang, H., Zhang, Y., Xiong, B., and Jiang, L. (2019). Microbiome and metabolome analyses of milk from dairy cows with subclinical Streptococcus agalactiae mastitis—Potential biomarkers. Front. Microbiol. 10:2547. doi: 10.3389/fmicb.2019.02547
Tourret, J., and Denamur, E. (2016). Population phylogenomics of extraintestinal pathogenic Escherichia coli. Microbiol. Spectr 4, doi: 10.1128/microbiolspec.UTI-0010-2012
Usami, A., Tanaka, M., Yoshikawa, Y., Watanabe, K., Ohtsuka, H., and Orino, K. (2011). Heme mediated binding of α-casein to ferritin: evidence for preferential α-casein binding to ferrous iron. BioMetals 24, 1217–1224. doi: 10.1007/s10534-011-9470-1
USDA. (2016). Dairy 2014, Milk Quality, Milking Procedures and Mastitis in the United States. Fort Collins, CO: USDA.
van der Helm, D. (1998). The physical chemistry of bacterial outer-membrane siderophore receptor proteins. Met. Ions Biol. Syst. 35:355.
Vitenberga-verza, Z., Melderis, I., and Gontar, Ł (2022). Identification of inflammatory and regulatory cytokines IL-1α-, IL- 4-, IL- 6-, IL- 12-, IL- 13-, IL- 17A-, TNF-α-, and IFN-γ-producing cells in the milk of dairy cows with subclinical and clinical mastitis. Pathogens 11, 1–23. doi: 10.3390/pathogens11030372
Wellnitz, O., and Bruckmaier, R. M. (2012). The innate immune response of the bovine mammary gland to bacterial infection. Vet. J. 192, 148–152. doi: 10.1016/j.tvjl.2011.09.013
Wente, N., Grieger, A. S., Klocke, D., Paduch, J. H., Zhang, Y., Leimbach, S., et al. (2020). Recurrent mastitis–persistent or new infections? Vet. Microbiol. 244:108682. doi: 10.1016/j.vetmic.2020.108682
Wenz, J. R., Barrington, G. M., Garry, F. B., Ellis, R. P., and Magnuson, R. J. (2006). Escherichia coli isolates’ serotypes, genotypes, and virulence genes and clinical coliform mastitis severity. J. Dairy Sci. 89, 3408–3412. doi: 10.3168/jds.S0022-0302(06)72377-3
Westermann, A. J., Barquist, L., and Vogel, J. (2017). Resolving host–pathogen interactions by dual RNA-seq. PLoS Pathog. 13:e1006033. doi: 10.1371/journal.ppat.1006033
Westermann, A. J., Förstner, K. U., Amman, F., Barquist, L., Chao, Y., Schulte, L. N., et al. (2016). Dual RNA-seq unveils noncoding RNA functions in host-pathogen interactions. Nature 529, 496–501. doi: 10.1038/nature16547
White, L. J., Schukken, Y. H., Dogan, B., Green, L., Döpfer, D., Chappell, M. J., et al. (2010). Modelling the dynamics of intramammary E. coli infections in dairy cows: understanding mechanisms that distinguish transient from persistent infections. Vet. Res. 41:13. doi: 10.1051/vetres/2009061
Wilson, D. J., Mallard, B. A., Burton, J. L., Schukken, Y. H., and Grohn, Y. T. (2009). Association of Escherichia coli J5-specific serum antibody responses with clinical mastitis outcome for J5 vaccinate and control dairy cattle. Clin. Vaccine Immunol. 16, 209–217. doi: 10.1128/CVI.00324-08
Yang, F., Zhang, S., Shang, X., Wang, X., Wang, L., Yan, Z., et al. (2018). Prevalence and characteristics of extended spectrum β-lactamase-producing Escherichia coli from bovine mastitis cases in China. J. Integr. Agric. 17, 1246–1251. doi: 10.1016/S2095-3119(17)61830-6
Yu, W. J., Ho, H., Wang, Y. T., Huang, S. N., and Han, R. W. (2020). Prevalence and antimicrobial-resistance phenotypes and genotypes of Escherichia coli isolated from raw milk samples from mastitis cases in four regions of China. J. Glob. Antimicrob. Resist 22, 94–101. doi: 10.1016/j.jgar.2019.12.016
Zaatout, N. (2022). An overview on mastitis-associated Escherichia coli: pathogenicity, host immunity and the use of alternative therapies. Microbiol. Res. 256:126960. doi: 10.1016/j.micres.2021.126960
Zadoks, R. N., Middleton, J. R., McDougall, S., Katholm, J., and Schukken, Y. H. (2011). Molecular epidemiology of mastitis pathogens of dairy cattle and comparative relevance to humans. J. Mammary Gland Biol. Neoplasia 16, 357–372. doi: 10.1007/s10911-011-9236-y
Zhang, D., Zhang, Z., Huang, C., Gao, X., Wang, Z., Liu, Y., et al. (2018). The phylogenetic group, antimicrobial susceptibility, and virulence genes of Escherichia coli from clinical bovine mastitis. J. Dairy Sci. 101, 572–580. doi: 10.3168/jds.2017-13159
Zhang, H., Jiang, H., Fan, Y., Chen, Z., Li, M., Mao, Y., et al. (2018). Transcriptomics and iTRAQ-proteomics analyses of bovine mammary tissue with Streptococcus agalactiae-induced mastitis. J. Agric. Food Chem. 66, 11188–11196. doi: 10.1021/acs.jafc.8b02386
Zhang, J., Xu, C., Huo, D., Hu, Q., and Peng, Q. (2017). Comparative study of the gut microbiome potentially related to milk protein in Murrah buffaloes (Bubalus bubalis) and Chinese Holstein cattle. Sci. Rep 7:42189. doi: 10.1038/srep42189
Zhang, S., Abbas, M., Rehman, M. U., Huang, Y., Zhou, R., Gong, S., et al. (2020). Dissemination of antibiotic resistance genes (ARGs) via integrons in Escherichia coli: a risk to human health. Environ. Pollut. 266:115260. doi: 10.1016/j.envpol.2020.115260
Zhao, X., and Lacasse, P. (2008). Mammary tissue damage during bovine mastitis: causes and control. J. Anim. Sci. 86, 57–65. doi: 10.2527/jas.2007-0302
Zhou, M., Yang, Y., Wu, M., Ma, F., Xu, Y., Deng, B., et al. (2021). Role of long polar fimbriae type 1 and 2 in pathogenesis of mammary pathogenic Escherichia coli. J. Dairy Sci. 104, 8243–8255. doi: 10.3168/jds.2021-20122
Keywords: mammary pathogenic Escherichia coli, antimicrobial resistance, virulence, cattle, mastitis pathogenesis, host-pathogen interaction, milk, immune response
Citation: Goulart DB and Mellata M (2022) Escherichia coli Mastitis in Dairy Cattle: Etiology, Diagnosis, and Treatment Challenges. Front. Microbiol. 13:928346. doi: 10.3389/fmicb.2022.928346
Received: 25 April 2022; Accepted: 13 June 2022;
Published: 07 July 2022.
Edited by:
Sónia Silva, University of Minho, PortugalReviewed by:
Zuzana Farkašová, University of Veterinary Medicine and Pharmacy in Košice, SlovakiaUlrich Dobrindt, University of Münster, Germany
Maria José Saavedra, Universidade de Trás-os-Montes e Alto Douro, Portugal
Copyright © 2022 Goulart and Mellata. This is an open-access article distributed under the terms of the Creative Commons Attribution License (CC BY). The use, distribution or reproduction in other forums is permitted, provided the original author(s) and the copyright owner(s) are credited and that the original publication in this journal is cited, in accordance with accepted academic practice. No use, distribution or reproduction is permitted which does not comply with these terms.
*Correspondence: Débora Brito Goulart, ZGdvdWxhcnRAaWFzdGF0ZS5lZHU=; Melha Mellata, bW1lbGxhdGFAaWFzdGF0ZS5lZHU=