- 1Institute of Plant Science and Resources, Okayama University, Okayama, Japan
- 2K.K. AB SCIEX, Tokyo, Japan
Methylobacterium and Methylorubrum species oxidize methanol via pyrroloquinoline quinone-methanol dehydrogenases (MDHs). MDHs can be classified into two major groups, Ca2+-dependent MDH (MxaF) and lanthanide (Ln3+)-dependent MDH (XoxF), whose expression is regulated by the availability of Ln3+. A set of a siderophore, TonB-dependent receptor, and an ABC transporter that resembles the machinery for iron uptake is involved in the solubilization and transport of Ln3+. The transport of Ln3+ into the cytosol enhances XoxF expression. A unique protein named lanmodulin from Methylorubrum extorquens strain AM1 was identified as a specific Ln3+-binding protein, and its biological function was implicated to be an Ln3+ shuttle in the periplasm. In contrast, it remains unclear how Ln3+ levels in the cells are maintained, because Ln3+ is potentially deleterious to cellular systems due to its strong affinity to phosphate ions. In this study, we investigated the function of a lanmodulin homolog in Methylobacterium aquaticum strain 22A. The expression of a gene encoding lanmodulin (lanM) was induced in response to the presence of La3+. A recombinant LanM underwent conformational change upon La3+ binding. Phenotypic analyses on lanM deletion mutant and overexpressing strains showed that LanM is not necessary for the wild-type and XoxF-dependent mutant’s methylotrophic growth. We found that lanM expression was regulated by MxcQE (a two-component regulator for MxaF) and TonB_Ln (a TonB-dependent receptor for Ln3+). The expression level of mxcQE was altered to be negatively dependent on Ln3+ concentration in ∆lanM, whereas it was constant in the wild type. Furthermore, when exposed to La3+, ∆lanM showed an aggregating phenotype, cell membrane impairment, La deposition in the periplasm evidenced by electron microscopy, differential expression of proteins involved in membrane integrity and phosphate starvation, and possibly lower La content in the membrane vesicle (MV) fractions. Taken together, we concluded that lanmodulin is involved in the complex regulation mechanism of MDHs and homeostasis of cellular Ln levels by facilitating transport and MV-mediated excretion.
Introduction
Methylobacterium and Methylorubrum species are ubiquitous in nature and can be found in a variety of habitats, including soil, dust, freshwater, lake sediments, leaf surfaces, and nodules (Green and Bousfield, 1983). They belong to the commensal type 2 methylotrophs, which utilize single-carbon substrates such as methanol and other methylated compounds for assimilation via the serine cycle (Whittenbury and Dalton, 1981). As a predominant bacterial member on the aerial surface (phyllosphere) of plants, they are involved in the global cycle of single-carbon compounds, as well as in important plant health–mediating symbionts (Vorholt, 2012).
The initial crucial step for methylotrophy is methanol oxidation catalyzed by pyrroloquinoline quinone (PQQ) methanol dehydrogenases (MDHs) in Methylobacterium species. The classic calcium-dependent MDH known as MxaFI was believed to be essential for methylotrophic growth in the laboratory conditions (Chistoserdova et al., 2009). It was found that the presence of a lanthanide ion (Ln3+) strongly induces a homologous XoxF-type MDH in Methylobacterium species (Hibi et al., 2011), including a well-studied model strain Methyorubrum extorquens strain AM1 (Nakagawa et al., 2012). This discovery has attracted a great deal of attention, since it was the first demonstration of the involvement of Ln in biological molecular functions, and Ln had previously been believed to be biologically unnecessary. In addition, XoxF-type MDHs are more common in nature, ecologically more relevant, and older from an evolutionary perspective (Keltjens et al., 2014; Chistoserdova, 2016). A variety of bacteria exhibits methanol-oxidation ability or methanol growth only in the presence of Ln owing to the presence of XoxF and absence of MxaF (Fitriyanto et al., 2011; Pol et al., 2014; Lv et al., 2018, 2020; Wang et al., 2019; Wegner et al., 2019). Methylobacterium extorquens strain AM1 has two homologues of XoxF MDHs type I (XoxF1 and XoxF2) as well as Ln-dependent ethanol dehydrogenase (ExaF, Good et al., 2016).
Ln regulates the expression of these alcohol dehydrogenases in the organisms that possess both (“Ln-switch,” Vu et al., 2016; Masuda et al., 2018). mxaF expression is dependent on the presence of xoxF in addition to dual two-component regulatory systems, MxcQE and MxbDM. Further research found that Ln uptake into the cytosol, mediated by a system consisting of a TonB-dependent receptor (TBDR) and an ABC transporter, which is similar to the iron acquisition system. Once the Ln enters the cytosol it induces the expression of XoxF1-MDH in M. extorquens strain AM1 (Roszczenko-Jasińska et al., 2020) as well as in M. extorquens strain PA1 (Ochsner et al., 2019). The uptake system is encoded in an Ln utilization and transport (lut) gene cluster (META1_1778 to META1_1787) in strain AM1. Furthermore, a periplasmic protein encoded by META1_p1781 (lutD) in the lut cluster was shown to bind Ln3+ (Mattocks et al., 2019). In addition, an Ln3+-chelator biosynthetic gene cluster called Lanthanide Chelation Clusters (LCC, META1p4129 to META1p4138) in M. extorquens strain AM1, which encode a TBDR and non-ribosomal peptide synthetase (NRPS) enzymes, was reported. The NRPS proteins are similar to those for siderophore aerobactin synthesis, and chemically synthesized aerobactin was demonstrated to bind Ln3+, however, aerobactin does not have an effect in vivo, denoting that the product of LCC is not aerobactin. In addition, expression of LCC in trans promoted the bioaccumulation of Ln, but not Fe, indicating that the product of LCC is a novel Ln3+ chelator (lanthanophore, Zytnick et al., 2022). Thus, the Ln uptake mechanism consists of the lanthanophore, TBDR, ABC transporter, and many other uncharacterized proteins comprising a “lanthanome” in Methylobacterium/Methylorubrum species (Mattocks et al., 2019).
Methylobacterium aquaticum strain 22A is a plant growth–promoting bacterium isolated from a moss (Tani et al., 2011). The strain belongs to clade C in phenotypically heterologous genera Methylobacterium/Methylorubrum species (Green and Ardley, 2018; Alessa et al., 2021). The strain also has MxaF and XoxF as well as ExaF that are regulated by Ln, but its second XoxF (XoxF2) is a pseudogene (Masuda et al., 2018). We found that strain 22A also switches between mxaF and xoxF expression depending on the presence of La3+, and that several unknown genes were upregulated (Masuda et al., 2018). We found that a gene, Maq22A_c02050 within them, which encodes a protein annotated as “histidine kinase,” was upregulated by La3+. The amino acid sequence of the gene contains a signal peptide and EF-hand calcium-binding motifs, but not a kinase domain. Since the EF-hand motif is reported to bind Ln3+ (Ye et al., 2005), we are curious about the function of the protein.
This putative Ln3+-binding protein homolog was first characterized in M. extorquens strain AM1 as a high-affinity Ln3+-binding protein (Cotruvo et al., 2018; Cook et al., 2019). The protein was named lanmodulin (LanM) and its metal coordination motifs enable 100 million times more selective binding for Ln3+ and Y3+ than for Ca2+. The protein undergoes a metal-dependent conformational change. LanM may function as a periplasmic Ln-binding protein that acts in association with TBDR (Cotruvo et al., 2018; Chistoserdova, 2019). The LanM gene (lanM, META1_1786) is part of the lut cluster in strain AM1 and based on annotation, it locates in the periplasm, however, no phenotype has been found when lacking. The genetic knockout of a homolog (Mext_1854) in an M. extorquens strain PA1 ∆mxaF background did not result in a growth defect on methanol in the presence of La3+ (Ochsner et al., 2019). Thus, the function of LanM as an Ln3+-binding protein in the methylotrophy and physiology of Methylobacterium species is yet to be characterized. In this study, we performed a functional analysis of LanM in order to obtain clues on the involvement of this protein in the mechanism of Ln3+ transport and methylotrophy in strain 22A.
Materials and Methods
Strains and Culture Conditions
Methylobacterium aquaticum strain 22A (FERM-BP11078; Tani et al., 2012) was used in this study. Strain 22A was grown on R2A medium or mineral medium (MM; Alamgir et al., 2015) containing 0.5% methanol or 0.5% succinate, and both at 28°C. Escherichia coli DH5α was used for plasmid construction and E. coli S17-1 was used for conjugation; they were grown on LB medium at 37°C. Kanamycin (Km, 25 mg/l) and LaCl3 of various concentrations were added when necessary. We exclusively used plastics to prepare LaCl3-containing medium since La3+ binds to glass surfaces. For growth experiments, strain 22A and its derivatives were grown in 200 μl medium prepared in 96-well plates. To facilitate aeration, we bored 35 holes (Φ5 mm) between the wells on the bottom side, and removed the side skirts. In this condition, the plates were rotary-shaken at 300 rpm at 28°C. The growth was monitored by measuring OD600 using a microplate reader (PowerScan HT, DS Pharma).
Recombinant LanM Expression and Purification
Alignment of protein sequences and signal peptide detection were done at EMBOSS water pairwise alignment1 and SignalIP-5.0 server,2 respectively. The strain 22A lanM (Maq22A_c02050) ORF encoding mature LanM without the signal peptide was PCR-generated using the primers listed in Supplementary Table S1. The product was cloned into the NdeI-XbaI site of pCold-I vector (Takara Bio Co.). The plasmid, pCold-lanM, was transformed into E. coli DH5α. E. coli DH5α (pCold-lanM) was cultured in 100 ml LB medium containing 25 mg/l ampicillin at 37°C until the culture OD600 became 0.5. Further cultivation was performed after the addition of 1 mM IPTG at 16°C for 18 h. The cells were collected, suspended in buffer A (20 mM Tris–HCl, pH 7.4, 70 mM NaCl and 20 mM imidazole), and disrupted with a bead beater (BioSpec 3110BX; Ieda Trading Corporation). The supernatant (centrifuged at 20,400 × g at 4°C for 15 min) was designated as a cell-free extract, and applied onto a Ni-NTA column (3.5 ml, His-Accept, Nacalai Tesque). The column was washed with 35 ml buffer A, and the His-tagged LanM (His-LanM) was eluted with buffer A containing 300 mM imidazole.
The ORF for mature lanM was also cloned into the BamHI site of pGEX-6p-1 (GE Healthcare) to generate pGEX-lanM, and expressed as a GST-fusion protein (GST-LanM). DH5α (pGEX-lanM) was cultured in 100 ml 2xYT medium (16 g/l polypeptone, 10 g/l yeast extract, and 5 g/l NaCl) at 25°C until the culture OD600 became 0.7. Further cultivation was performed after the addition of 0.1 mM IPTG for 3 h. The cells were harvested, suspended in buffer B (20 mM Tris–HCl pH 7.5), and disrupted as above. The cell-free extract was applied onto a GST-ACCEPT column (1 ml, Nacalai Tesque). The column was washed with 10 ml buffer B, and the protein was eluted with buffer B containing PreScission protease (GE Healthcare).
The purified His-LanM and LanM (GST-cleaved) were buffer-exchanged to 40 mM acetate buffer, pH 4.1 containing 0.05% sodium azide with a 3 kDa cutoff filter (Amicon Ultra 3,000, Merck), and kept at 4°C until use. The concentration of protein was measured according to the Lowry method (Lowry et al., 1951) using bovine serum albumin as the standard.
Biochemical Characterization of the Recombinant LanM
The purified His-LanM (86 μM) and LanM (43 μM) were subjected to gel filtration chromatography using 50 mM acetate-KOH buffer (pH 4.1) containing 100 mM NaCl and 0.05% sodium azide (TSK-GEL Super SW3000, 4.6 mm × 30 cm, flow rate 0.35 ml/min, injection 100 μl, detection 220 and 280 nm, fractionation 100 μl). The samples mixed with LaCl3 (final concentration 3.4 mM and 1.7 mM for His-LanM and LanM, respectively) were also analyzed. A fraction of the protein peak of LanM mixed with LaCl3 was selected (4.0–4.1 ml), mixed with nitric acid, and analyzed with inductively coupled plasma mass spectrometry (ICP-MS; Agilent Technologies 7500cx).
Construction of Mutants
The gene deletion mutant of lanM was generated using an allele-replacement vector pK18mobSacB (Schäfer et al., 1994), as previously reported (Alamgir et al., 2015). In brief, each 1 kb upstream and downstream region of the target gene was polymerase chain reaction (PCR)-amplified and cloned in tandem into the EcoRI site of the vector and the In-Fusion Cloning kit (Takara Bio Co.). The vector was introduced into strain 22A and its derivatives by conjugation using E. coli S17-1. Single-crossover mutants were selected by Km resistance, and double-crossover mutants were selected by 10% sucrose resistance. PCR diagnosis was carried out as previously described (Alamgir et al., 2015). The lanM deletion mutant was designated as ΔlanM. ΔmxaF, ΔxoxF1, and ΔxoxF1Sup were generated in our previous study (Masuda et al., 2018). ΔxoxF1SupΔmxaF, ΔxoxF1SupΔexaF, and ΔmxaFΔexaF were generated in this study using the gene deletion vectors made in our previous study (Yanpirat et al., 2020). In addition, we also constructed ΔtonB_Ln (Maq22A_c14845) and ΔmxcQE (Maq22A_c14840 and Maq22A_c14835).
Vector Construction
We generated pCM130KmC for general cloning purposes that operates in strain 22A from pCM130 (Addgene plasmid #45828, Marx and Lidstrom, 2001) in our previous study (Yanpirat et al., 2020). A fragment containing an EcoRI site and His-tag coding sequence generated by a pair of complementary oligonucleotides (pCM130KmCinsert1 and 2, Supplementary Table S1) was inserted into the EcoRI site of pCM130KmC with the In-Fusion Cloning kit to generate pAT01. The vector enables His-tagged protein expression. Then we cloned PCR fragments of formaldehyde-activating enzyme promoter (Pfae1, 220 bp upstream region of the fae1 ORF, Maq22A_c16490) and mxaF promoter (PmxaF, 572 bp upstream region of the mxaF ORF, Maq22A_1p33165) into the EcoRI site, and named them pAT02f and pAT02m, respectively. These vectors enable His-tagged protein expression under Pfae1 and PmxaF. The PCR-amplified lanM ORF was cloned into pAT02f to express LanM, and introduced into ΔlanM.
To assess the promoter activity, PCR-generated green fluorescent protein (GFP) ORF from pHC42 (Chou et al., 2009) was cloned into each vector to generate pAT02f-GFP and pAT02m-GFP, using the primers listed in Supplementary Table S1. These vectors were introduced into strain 22A by conjugation and their fluorescence was measured to compare the expression level. The strains were grown on R2A medium containing 25 mg/l Km at 28°C for 3 days. The cells were harvested, washed with saline, and suspended in methanol medium at OD600 = 0.4. The suspension was aliquoted (200 μl) into black and transparent 96-well plates, and the cells were allowed to grow at 28°C, 300 rpm. The fluorescence (excitation 440/40, emission 528/20, sensitivity 120) and cell density (OD600) were measured with a microplate reader. The fluorescence was normalized to the cell density (OD600). The wild-type strain 22A harboring pAT02f-GFP or pAT02m-GFP showed higher GFP fluorescence when cultured on methanol than on succinate. The expression level of GFP in methanol in the absence of La3+ was higher than that in the presence of La3+ (Supplementary Figure S3). Based on these results, we used pAT02f as an expression vector for methanol-inducible lanM expression.
We constructed a GFP-tagged LanM expression vector based on pHC42 that has a GFP gene as a reporter. The ribosome binding site of the vector was eliminated by PCR with InversePHC42F2 and InversePHC42R2–2 primers and self-circularization of the product, and a new EcoRV site was concomitantly introduced. The vector was named pHC42m. Then, lanM with its putative promoter region (1,077 bp upstream region of the ORF) was PCR-amplified with lanM-promoter5’ and lanM-ORF3’ primers and cloned into the EcoRV site of pHC42m (pHC42m-lanM).
We also constructed a luciferase-reporter vector (pAT06-Lux) by replacing the PmxaF and GFP gene in pAT02m-GFP with bacterial luciferase (Lux) genes, which were PCR-amplified from pUC18-mini-Tn7T-Gm-lux (Wehrmann et al., 2017) with LuxC-F and LuxE-R2 primers. The promoter regions of lanM and mxcQ were PCR-amplified, and inserted into pAT06-Lux to generate pAT06-Lux-PlanM and pAT06-Lux-PmxcQ.
qPCR
The total RNA was purified using Trizol (Sigma) from the exponentially growing culture of strain 22A wild type on methanol in the absence/presence of 30 μM LaCl3. The RNA samples were treated with Promega RQ DNase I (Promega). cDNA synthesis was carried out with ReverTra Ace (Toyobo) and a random hexamer primer. qPCR was performed with a CFX Connect Real-Time PCR detection system (Bio-Rad), Thunderbird SYBR green kit (Toyobo), and primers designed for quantitative (q) PCR (Supplementary Table S1). The thermal program was as follows: 95°C for 1 min, and 45 cycles of 95°C for 15 s and 62°C for 30 s, followed by dissociation curve analysis. The PCR-generated amplicon of the target region using the genome as a template was serially diluted and used for the generation of a standard curve. Data acquisition and analysis were performed with CFX Manager ver. 3.1 (Bio-Rad). The expression level of lanM was evaluated as a relative expression against rpoC (Maq22A_c27065), whose expression level is stable (Masuda et al., 2018).
Luciferase Reporter Assay
Strain 22A and its derivatives transformed with pAT06-Lux derivatives were grown for 3 days at 28°C on R2A solid medium containing 25 mg/l Km. The cells were collected, washed with saline, and suspended in methanol medium, succinate medium, or methanol succinate medium at OD600 = 0.02. The medium suspension was aliquoted (200 μl) into 96-well white (for luminescence measurement, sensitivity 135) and transparent (for OD600 measurement) plates, and the plates were rotary-shaken at 28°C, 300 rpm. Promoter activity was evaluated as luminescence normalized to cell density (OD600). The expression level was regarded as the maximum luminescence value normalized by the OD600 value at that time.
Observation of Cell Aggregation and Live/Dead Staining
The wild-type and ΔlanM cells grown on solid MM containing 0.5% methanol for 3 days were suspended in 10 mM HEPES, pH 7.0 (OD60 0 = 0.5). Then, LaCl3 was added (final concentration, 30 μM) and after 1 min, the cells were subjected to live/dead staining using a Viability/Cytotoxicity Assay kit for Bacteria (BTI Biotium Inc.) that contains DMAO (green fluorescent nucleic acid dye for staining live and dead bacteria) and ethidium homodimer (EthD-III, for staining dead bacteria). The cells were observed with a fluorescent microscope (Keyence BZ-X700). At the same time, the cell suspensions before/after 10 min addition of LaCl3 were serially diluted and spread onto R2A medium to count the number of colony forming units (CFUs).
Cellular Uptake of La3+
The wild type, ΔlanM, and ΔlanM (pAT02f-lanM)(hereafter referred to as lanM-OX) grown on solid MM containing 0.5% methanol for 3 days were suspended in saline at OD600 = 0.3. The suspensions of 500 μl were aliquoted into 2 ml tubes and LaCl3 was added (final concentration, 30 μM). The suspensions were shaken (28°C, 160 rpm), and three tubes were centrifuged (20,400 × g, 4°C, 15 min) at the timing of 0, 4, 24, 48, 72, and 96 h. The supernatant (400 μl) was taken as a supernatant fraction. The cells and residual saline (100 μl) were mixed, transferred into a new tube, and designated as the precipitate fraction. Then the materials remaining on the wall of the emptied tubes were regarded as the tube wall fraction. The samples were mixed with 61% HNO3, heated at 100°C for 1 h, appropriately diluted, and analyzed with ICP-MS to measure the La content. The precipitate fraction was regarded as containing La associated with the cells and soluble La with an equal concentration as the supernatant fraction. Therefore, the soluble La content was subtracted from that of the precipitate fraction to give the cell-associated La content. As a control, saline without cells was prepared and treated in the same manner. The cell concentration of the samples before the addition of LaCl3 was determined by CFU measurement on R2A medium.
The tube wall fractions of 96 h samples prepared by another independent experiment were subjected to liquid chromatography (LC)-MS analysis. The tubes were washed twice with water, and vortexed after the addition of 1 ml acetonitrile. The contents were transferred into new tubes, centrifuged, dried in vacuo, dissolved in a buffer (8 M urea, 0.5 M Tris–HCl pH 8.5, and 25 mM EDTA), treated with 5 mg/ml DTT and 10 mg/ml iodoacetamide, and digested with trypsin. The samples were analyzed with a high performance LC-Chip/quantitative time of flight mass spectrometer (Agilent Technologies) at the Advanced Science Research Center, Okayama University, Okayama, Japan. The data were analyzed using Mascot (Matrix Science). The amino acid sequences of the CDSs extracted from strain 22A genome data served as the database.
Confocal Laser Scanning Microscopy
Strain 22A ΔlanM transformed with pHC42m and pHC42m-lanM was grown in liquid MM containing 0.5% methanol in the absence/presence of 30 μM LaCl3 for 2 days, suspended in 5% glycerol, and observed with a confocal scanning microscope (FLUOVIEW FV1000, Olympus, ×100 objective lens) to observe the cellular localization of GFP-tagged LanM.
Transmission Electron Microscopy
The wild type, ΔlanM, and lanM-OX grown in liquid MM containing 0.5% methanol in the absence/presence of 30 μM LaCl3 for 1 and 3 days were harvested, washed, and suspended in 2.5% glutaraldehyde in 0.1 M cacodylate buffer. The cells were washed with 0.1 M phosphate-buffered saline (PBS) four times, and mixed with 3% agarose. Then the samples were cut into 1 mm dice, fixed with 1.5% OsO4 in PBS for 1.5 h, washed with PBS three times, and dehydrated with ethanol (series of 50–100%, each 15 min). Ethanol was replaced with propylene oxide. The samples were embedded in Spurr resin (Polysciences), sliced with an ultramicrotome (Reichert-Jung), and stained with 3% uranyl acetate for 10 min and lead citrate for 2 min. Transmission electron microscopy (TEM) analysis was carried out with a JEM-1400 (JEOL, Ltd.). The electron-dense particles observed in the non-stained samples were also analyzed with a 200 kV TEM (JEM-2010) equipped with energy-dispersive X-ray spectroscopy (EDX; JED-2300, JEOL, Ltd.).
Proteome Analysis for ΔlanM
The wild-type and ΔlanM cells grown in 100 ml MM containing 0.5% methanol in the absence/presence of 30 μM LaCl3 for 4 days in triplicate were harvested, washed, and suspended in 500 μl 8 M Urea containing 5 mM phenylmethylsulfonyl fluoride. The samples were processed similarly to the above for LC–MS analysis. The dried samples were dissolved in 50 μl 0.1% formic acid, and analyzed with TripleTOFR6600 (AB SCIEX, Tokyo Japan) equipped with Eksigent™ nanoLC. The Sequential Windowed Acquisition of all THeoretical fragment ions Mass Spectrometry (SWATH-MS) technique (Gillet et al., 2012) was utilized to identify and quantify the proteins. ProteinPilot software (Shilov et al., 2007) identified 18,061 peptides and 1,693 proteins (FDR 1%) through merge analysis of all samples, and they are used as a library for SWATH analysis. The data of peak area were normalized, and differentially expressed proteins were extracted under the following condition: fold change, 3 in comparison of the gene deletion effect (wild type against ΔlanM); value of p <0.05, pairwise-corrected t-test.
MV Extraction and Proteome Analysis
Strain 22A wild-type (pAT02f), ΔlanM(pAT02f), and lanM-OX strains were grown in 30 ml of 0.5% methanol medium supplemented with 30 μM LaCl3 for 6 days with shaking at 28°C, 130 rpm in triplicate. The cultures were centrifuged twice at 5000 × g for 20 min at 4°C to remove bacterial cells, and then the supernatant was filtered through a 0.45 μm syringe filter. From these supernatants, Membrane Vesicles (MVs) were extracted according to the instructions in the ExoBacteria OMV Isolation Kit (SBI, code; EXOBAC100A-1). Of the 1.5 ml of each obtained MV extract, 400 μl was subjected to ICP-MS for quantification of La. The remaining extracts were used for protein concentration measurement by the BCA method and confocal microscopy. Each elemental data was normalized to the protein concentration of the respective MV extracts.
For proteome analysis of the MV fractions, the same strains were grown in 1 l medium similarly and the culture supernatant was filtered through a 0.45 μm syringe filter. These filtrates were ultracentrifuged (217,000 × g, 1 h, and 4°C, Beckman Coulter, Optima L-100 K), and the precipitated portions suspended in a buffer (12 mM sodium deoxycholate, 12 mM sodium N-lauroyl sarcosinate, 100 mM Tris–HCl pH 9.0; Masuda and Ishihara, 2016), and heated at 95°C for 5 min. The protein concentration was quantified using a BCA assay kit. DTT (final concentration 10 mM) was added to 300 μl of the samples. After 30 min, IAA (final concentration, 50 mM) was added. Then 1.2 ml of 50 mM ammonium hydrogen carbonate solution was added after 30 min incubation in the dark. For sodium N-lauroyl sarcosinate removal, 1.5 ml of ethyl acetate and TFA (final concentration 0.5%) were added, and the mixture was vortexed for 1 min. The upper ethyl acetate layer was removed by centrifugation. The remaining solution was dried in vacuo, and suspended in 100 μl of 5% ACN 0.5% TFA aqueous solution. The samples were desalted with C18-Tip, dried, and resuspended in 100 μl of 5% ACN 0.1% TFA for LC–MS analysis.
Results
LanM Sequence Characteristics and Its Upregulation in the Presence of La3+
lanM is encoded in the strain 22A chromosome, associated with the genes for purine biosynthesis, a hemolysin, a hypothetical protein, an adenylate cyclase, and an MucR transcriptional regulator (Supplementary Figure S2A). This approximately 4 kb region containing lanM is also conserved in the genomes of several Methylobacterium species, including Methylobacterium sp. strain 17Sr1-1 (CP029552.1), strain 17Sr1-28 (CP029553.1), and M. currus strain PR1016A (CP028843.1) that are phylogenetically close to strain 22A. The lanMs in strains AM1 and PA1 are clustered with lut genes related to ABC transporter and TBDR, which are important for Ln3+-dependent growth (Ochsner et al., 2019; Roszczenko-Jasińska et al., 2020). lanM in strain 22A appeared to be clustered with genes that are functionally unrelated to Ln transport.
The propeptide of LanM (134 amino acids) from strain 22A shares 58.5% identity and 68.9% similarity with that from strain AM1. A signal peptide cleavage site is detected at E23 or K25. Four repeats of the EF-hand motif (D(orE)xDxDxxxxxxE) were also detected. Unlike in the strain AM1 LanM holding proline at second amino acid of the four EF-hand motifs (Cotruvo et al., 2018), strain 22A LanM has T and K, respectively, in the first two motifs (Supplementary Figure S2B). These residues are not present in the structural homologs, calmodulin, and suggested to contribute to Ln selectivity.
In our RNA-sequencing experiment, lanM expression is 4.25-fold upregulated when the cells were grown on methanol in the presence of La3+, compared to the absence of La3+ (q < 0.001, two-way ANOVA followed by Tukey’s multiple comparison test, Masuda et al., 2018). We confirmed its upregulation with qPCR and found that the expression level is 10.3-fold upregulated in the presence of La3+, suggesting the importance of lanM for the growth in the presence of La3+ (Figure 1).
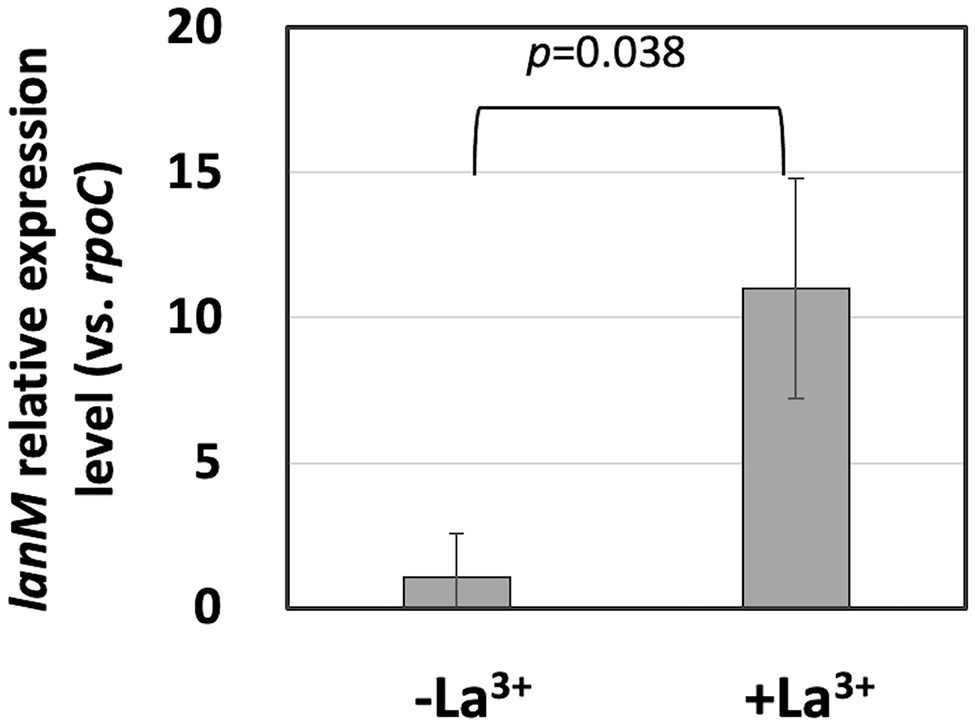
Figure 1. lanM expression level in Methylobacterium aquaticum strain 22A grown on methanol in the presence/absence of 30 μM La3+, quantified by qPCR. lanM expression level is shown as the relative expression level compared to that of rpoC as a reference. Data were analyzed with Student’s t-test.
LanM Binds La3+
To biochemically characterize LanM from M. aquaticum strain 22A, we purified the recombinant mature LanM (E23 was regarded as the cleaving site, and its theoretical molecular mass is 11.85 kDa) expressed in E. coli as an N-terminal His-tagged protein (His-LanM, theoretical mass 14.0 kDa) and N-terminal GST-tagged protein (GST-LanM, 36.7 kDa; Supplementary Figure S3). The GST-LanM was cleaved to remove the GST portion (LanM, 12.3 kDa). The purified His-LanM and LanM were mixed with La3+ or Ca2+ and analyzed with gel filtration chromatography (Figure 2). The apparent native molecular mass was 28.1 kDa (His-LanM) and 30.8 kDa (LanM), which shifted to 19.0 kDa and 22.1 kDa when mixed with La3+, respectively. That of His-LanM mixed with Ca2+ also shifted to 25.1 kDa. The His-LanM and that with Ca2+ showed an additional peak at 53.3 and 49.5 kDa, respectively, which disappeared upon the addition of La3+. These higher molecular peaks did not appear in the LanM preparation. The peak fraction of LanM (4.0–4.1 ml) mixed with La3+ was analyzed with ICP-MS. The protein concentration was 25.3 μM and the La3+ content was 92.5 μM, suggesting that LanM binds 3.65 mol La3+ per LanM molecule. La3+ was not detected in the experiment without LanM and that without added La3+, at the fraction of the same elution volume (Data not shown). These results suggested that LanM alters its conformation to a more compact form upon binding La3+, as already shown for the strain AM1 LanM (Cotruvo et al., 2018; Cook et al., 2019).
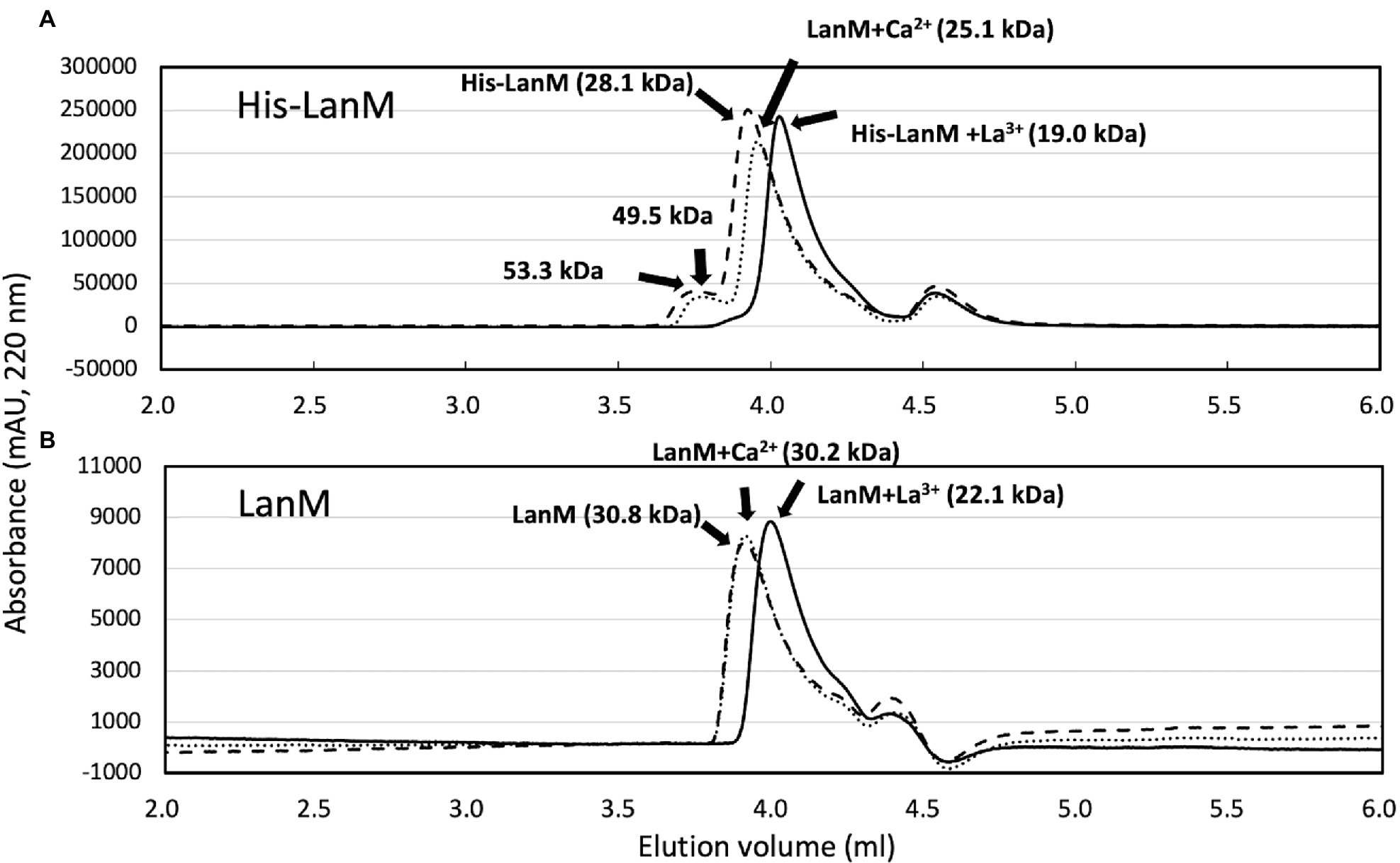
Figure 2. (A) Gel filtration analysis of His-LanM. The purified His-LanM (86 μM, 100 μl) was subjected to gel filtration analysis. Lines, absorbance at 220 nm; dashed line, His-LanM; solid line, His-LanM mixed with 3.4 mM LaCl3; and dotted line, His-LanM mixed with 3.4 mM CaCl2. (B) Gel filtration analysis of LanM. The purified LanM (43 μM, 80 μl) obtained by GST-cleavage of GST-LanM was mixed with 1.7 mM CaCl2 or 1.7 mM LaCl3 and subjected to gel filtration. Lines, absorbance at 220 nm; dashed line, His-LanM; solid line, His-LanM mixed with La3+; and dotted line, His-LanM mixed with Ca2+. Protein molecular weight analysis was carried out with separately analyzed standard proteins of chicken egg albumin, 45 kDa; bovine milk α-lactalbumin, 14.2 kDa; cytochrome c 12.4 kDa; and aprotinin 6,511 Da.
LanM Is Not Essential for Methanol Growth
ΔlanM did not show any growth deficiency on methanol in the presence of varied concentrations of La3+, but showed a higher cell yield than the wild type grown with relatively high La3+ concentrations (10–100 μM; Figure 3). These results suggested that LanM is not required for methanol growth. Next, Ln3+- and XoxF-dependent methanol growth of ΔmxaF was examined in the presence of limited La3+ (Figure 4). ΔmxaFΔlanM showed no difference in La3+ concentration–dependent growth compared to ΔmxaF, suggesting that LanM is not essential for XoxF expression and XoxF metalation. When lanM was expressed under the fae1 promoter, the strain exhibited slightly faster (with 0.02 μM La3+) and slightly slower (with 0.1 μM La3+) growth. These results suggested that overexpression of LanM causes some unknown dysregulation of metabolism.
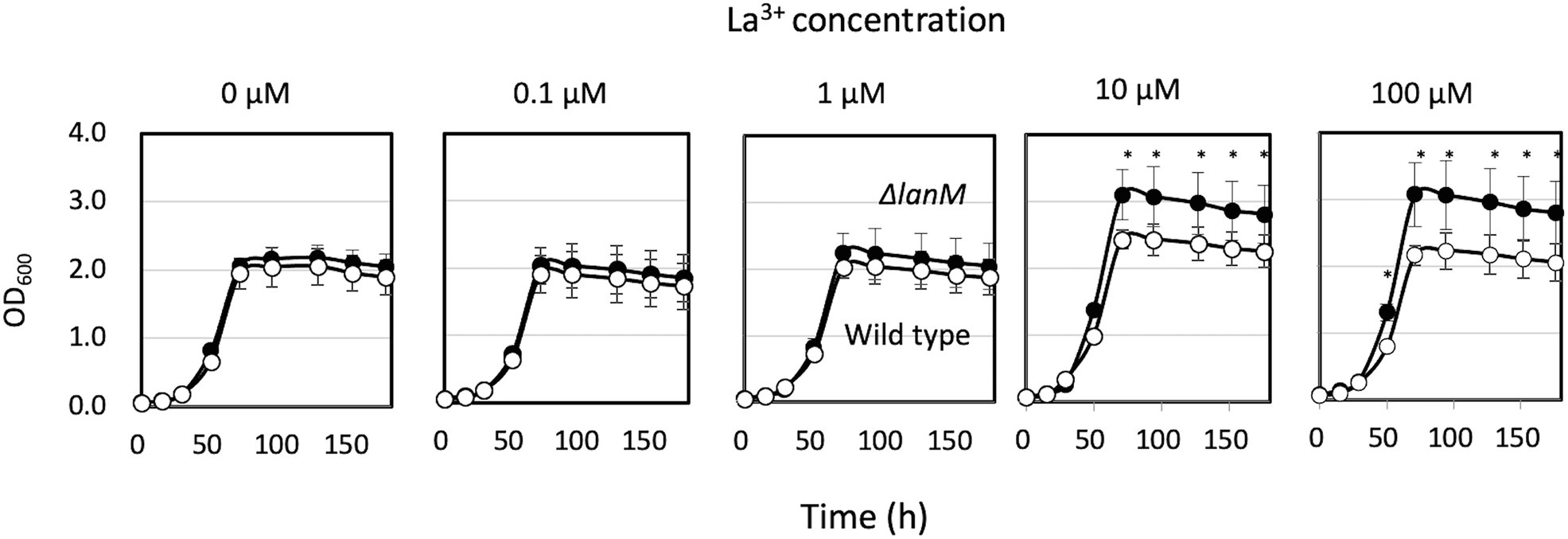
Figure 3. Growth of strain 22A wild type and ΔlanM on methanol under varied concentrations of LaCl3. Filled symbols, wild type; open symbols, ΔlanM. Data are presented as the mean value ± standard deviation (SD; n = 3). The data were analyzed with two-way ANOVA followed by Sidak’s multiple comparisons test. *p < 0.05.
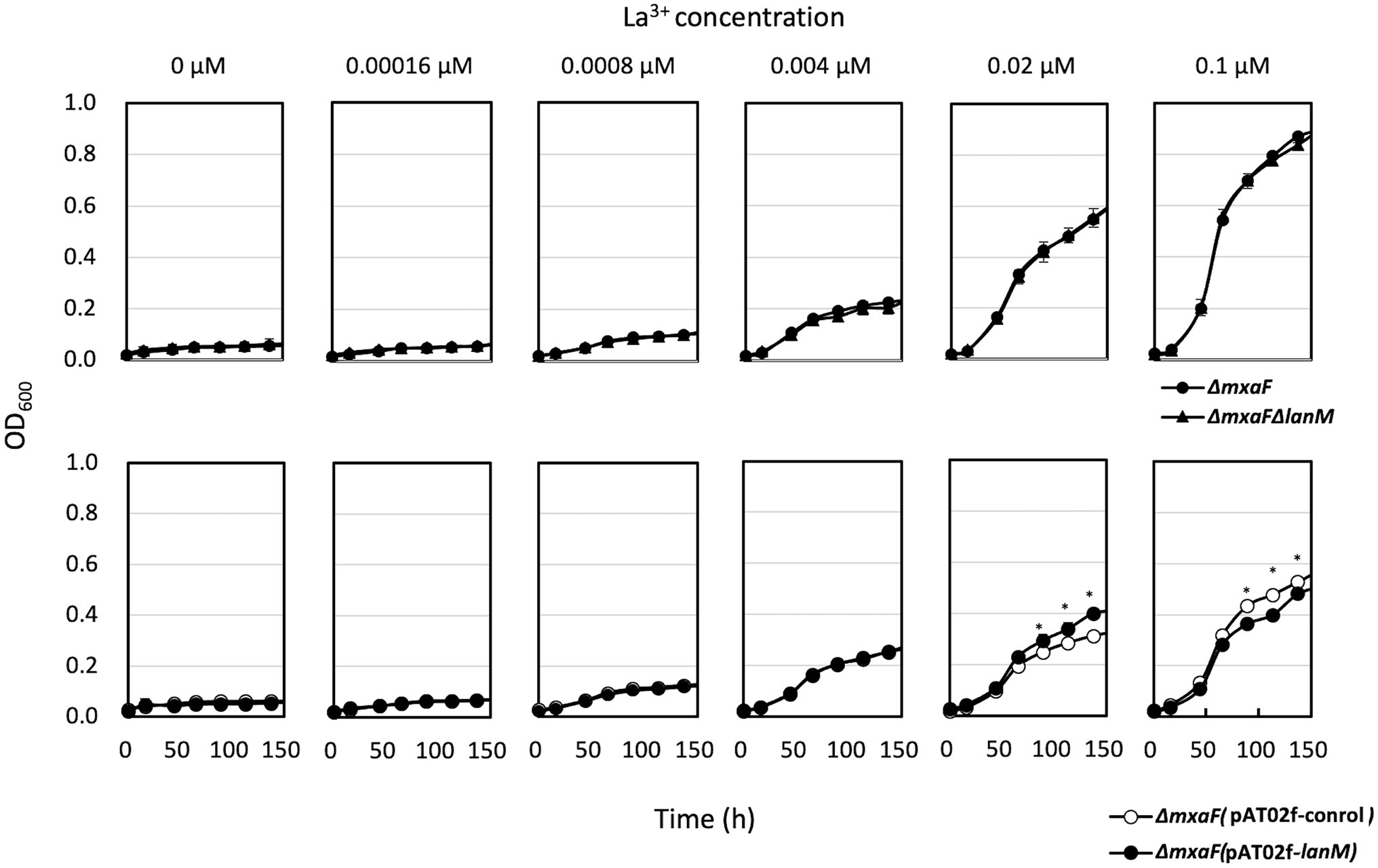
Figure 4. Upper panel: Growth of ΔmxaF (filled circles) and ΔmxaFΔlanM (triangles) on methanol in the presence of limited concentrations of La3+. Lower panel: Growth of ΔmxaF (pAT02f; open circles) and ΔmxaF (pAT02f-lanM; filled circles) on methanol in the presence of limited concentrations of La3+. Data are presented as the mean value ± standard deviation (SD; n = 3). The data were analyzed with two-way ANOVA followed by Sidak’s multiple comparisons test. *p < 0.05.
Identification of an Ln3+-Transporting TBDR
Among 12 TBDR genes encoded in the genome of strain 22A, Maq22A_c14845 showed the highest identity to lutH in the lut gene cluster of strain AM1 (MexAM1_META1p1785, 35.7% identity, EMBOSS water pairwise alignment). The expression of Maq22A_c14845 was induced 1.3-fold by La3+ (Masuda et al., 2018). Th gene is encoded in the upstream of mxcQE in the same orientation, but there are no other apparent methylotrophy- or lanthanide uptake-related genes nearby. Knockout strains of Maq22A_c14845 were successfully generated under wild-type and ΔmxaF backgrounds, and subjected to growth experiments on methanol and succinate. The gene was named tonB_Ln.
ΔtonB_Ln showed no growth deficiency on methanol irrespective of the presence of La3+, because the mutant could use MxaF to grow even in the presence of La3+ (Supplementary Figure S4). Whereas ΔmxaF could still grow in the presence of La3+ but not in the absence of La3+, ΔmxaFΔtonB_Ln could not grow in the presence of La3+. These results suggested that TonB_Ln is involved in Ln3+ uptake that leads to the activation of XoxF. Their growth on succinate was not affected by La3+.
lanM Expression Is Regulated by mxcQE and tonB_Ln, and mxcQE Expression Is Regulated by lanM
To examine genes involved in the regulation of lanM, the lanM promoter reporter vector (pAT06-Lux-PlanM) was introduced into several mutants of strain 22A (Figure 5A). The transformants were grown on succinate and methanol. In the wild type and ∆mxbD, PlanM responded to the presence of La3+. In ΔxoxF, PlanM responded to La3+ even higher than the wild type. In ΔmxcQE and ΔtonB_Ln, PlanM activity was lower and higher than that in the wild type, respectively, and no La3+-induction was observed. In ΔlanM, interestingly, PlanM was highly active even in the absence of La3+. These results suggested that PlanM is under regulation of mxcQE and tonB_Ln, and that PlanM shows self-regulation, as seen in the case of PxoxF.
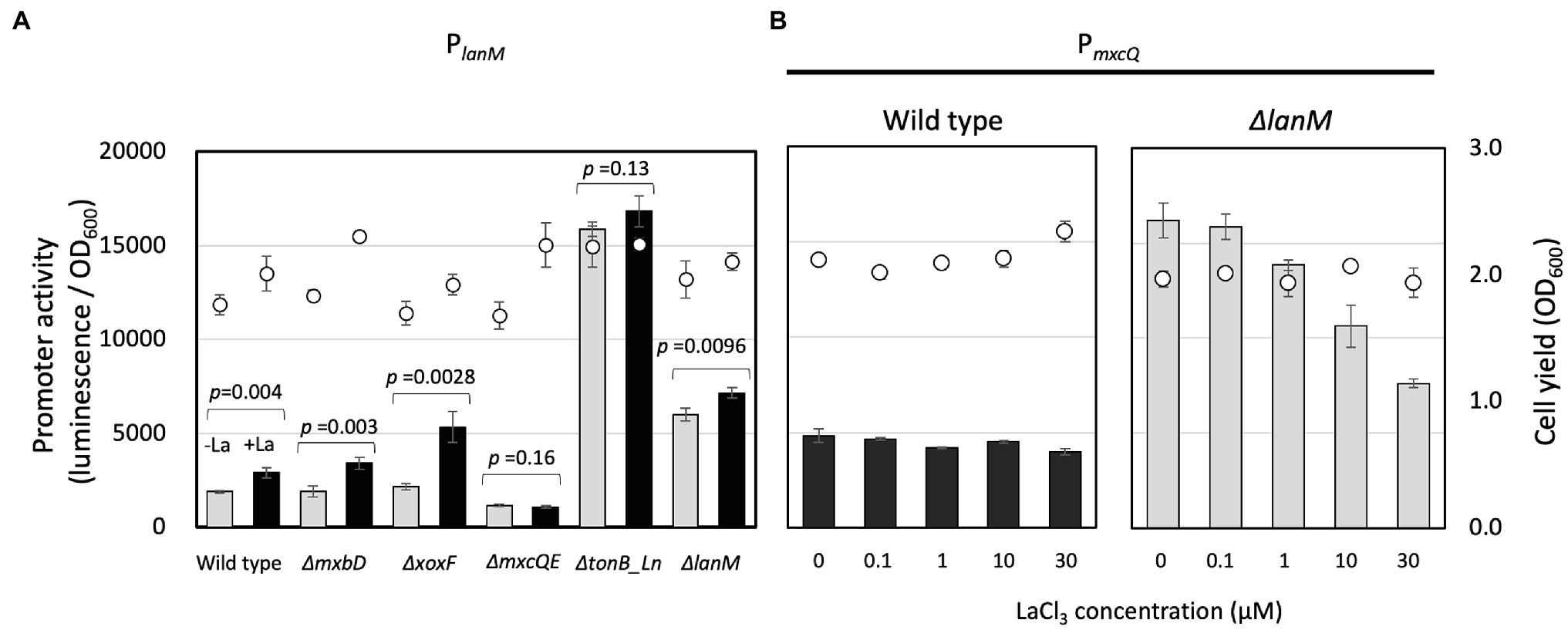
Figure 5. (A) PlanM activity in the mutants grown on methanol plus succinate. Promoter activity was assessed as maximum luminescence per OD600 of the culture at the time. Gray bars, without LaCl3; filled bars, with 30 μM LaCl3; circles, cell yield (OD600). The promoter activity was analyzed by Student’s t-test and p values are indicated. (B) PmxcQ activity in the wild type and ΔlanM grown on methanol in the presence of varied concentrations of LaCl3. Bars, PmxcQ activity; and circles, cell yield (OD600). Data are presented as the mean value ± standard deviation (SD; n = 3).
PmxcQ showed little response to La3+ in the wild-type background, however, it showed higher activity than in the wild type, and it responded negatively to La3+ concentration in the ΔlanM background (Figure 5B), suggesting that LanM is involved in the Ln3+ concentration–dependent regulation of mxcQ.
Cell Membrane Integrity Was Impaired in ΔlanM
We noticed that ΔlanM cells tend to aggregate upon the addition of La3+ in a 10 mM HEPES buffer (pH 7.0). We therefore assessed the viability of ΔlanM upon exposure to La3+. We found that the CFUs of ΔlanM decreased upon the addition of La3+ in 10 min (Figure 6A). The ΔlanM cells aggregated in the presence of La3+ and showed high permeability for ethidium homodimer III (EthD-III), which is used to assess cell death (Figure 6B). In physiological saline, this phenomenon did not occur (data not shown); therefore, this effect was dependent on HEPES. Since HEPES is known to be cytotoxic (Zigler et al., 1985), these results suggested that the cell membrane integrity is impaired in ΔlanM upon exposure to La3+.
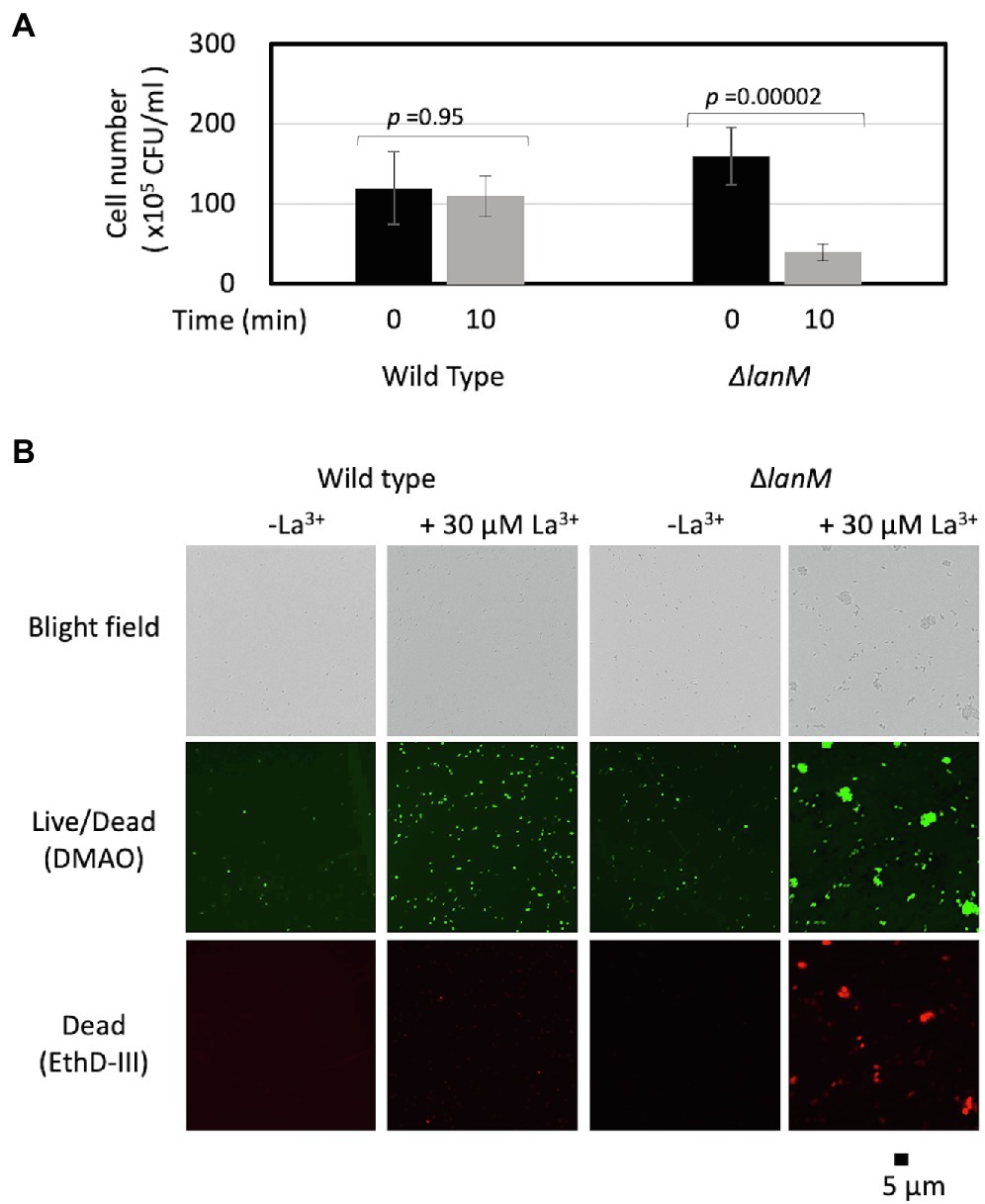
Figure 6. Cell aggregation of ΔlanM upon exposure to La3+. (A) Determination of CFUs of the wild type and ΔlanM before and after exposure to 30 μM La3+. Data are presented as the mean value ± standard deviation (SD; n = 3) and analyzed with Student’s t-test. (B) Fluorescent microscopic analysis of the Live/Dead-stained wild type and ΔlanM exposed to 30 μM La3+.
Uptake of La3+
Next, we examined the time-dependent Ln3+ uptake in the wild type, ΔlanM, and lanM-OX in the presence of 30 μM La3+ (Figure 7). The cell concentration of the suspensions was 39.3 ± 2.5 × 106 CFUs (wild type, data presented as mean ± standard deviation [SD], technical triplicates), 42.7 ± 2.3 × 106 CFUs (ΔlanM), and 52.3 ± 3.1 × 106 CFUs (lanM-OX). The La3+ content in three fractions, the supernatant of the cell suspension, the cells, and the remnants on the tube wall, were measured. The control experiment without the cells showed almost constant La3+ concentration in the supernatant and the precipitate, and La3+ absorption to the tube wall was not observed. The La3+ concentration in the supernatant of the wild-type cell suspension decreased over time, down to almost zero in 96 h, and the cell-associated La3+ content became greater. A substantial amount of La3+ was also detected in the tube wall fraction. ΔlanM showed a similar trend but the decrease in supernatant La3+ was faster, and the La3+ content in the tube wall fractions was greater than the wild type, whereas it showed comparative La3+ content in the cell-associated fraction. lanM-OX showed an even faster decrease in the supernatant within 24 h, but later the supernatant La3+ content became higher than the other two. Whereas the La3+ content in the cell-associated fraction was higher in the earlier timing (4–24 h), the tube wall fraction contained the least La in the end. These results suggested that the LanM facilitates Ln3+ uptake, and that the lack of LanM causes aggregation Ln3+-bound cells.
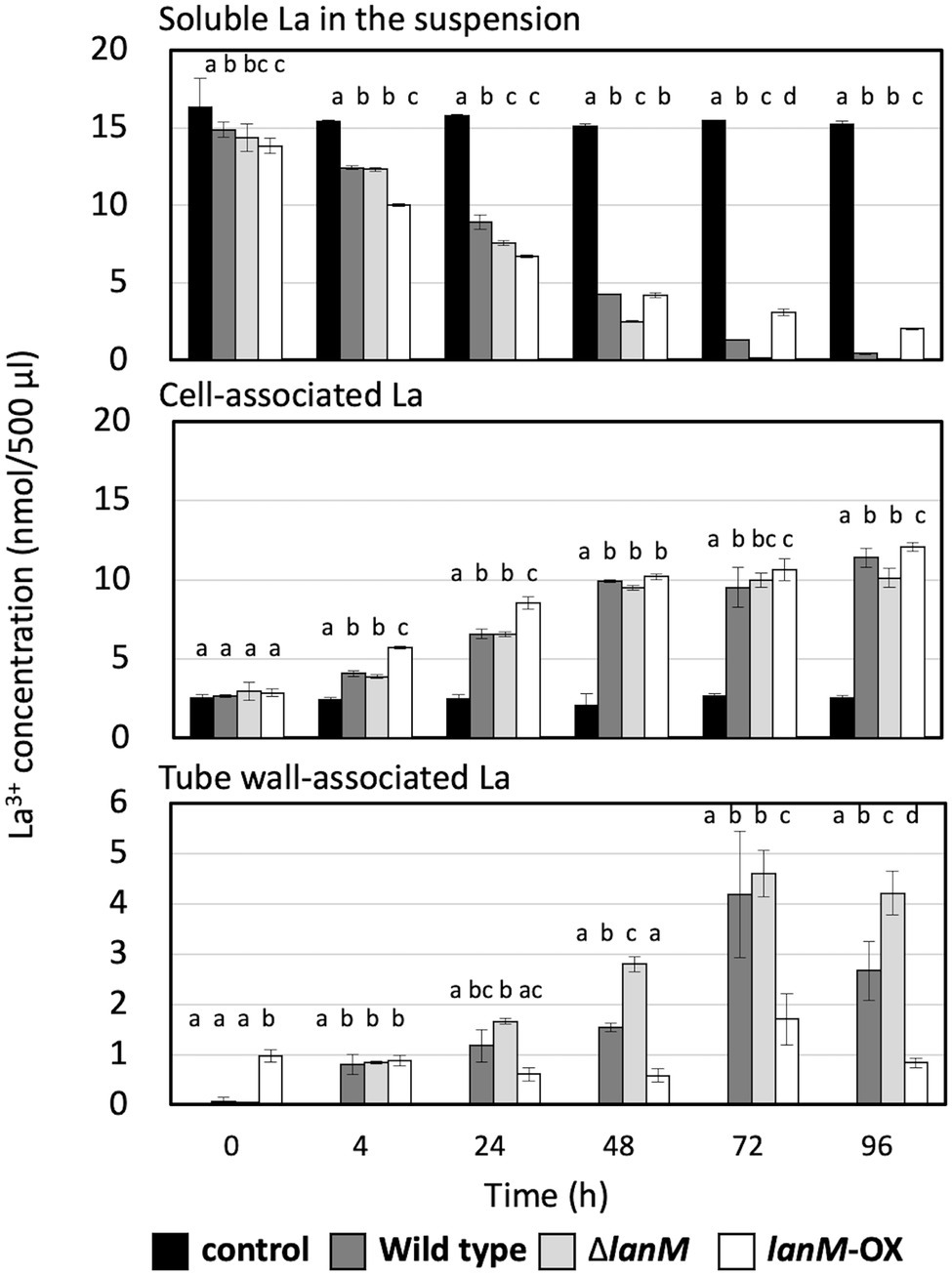
Figure 7. Time-dependent changes of the soluble La in the suspension, cell-associated La, and tube wall–associated La in the cell suspensions of the wild type, ΔlanM, and ΔlanM (pAT01-Pfae1-lanM). The details of the experiment are included in the “Materials and Methods”. Data are presented as the mean value ± standard deviation (SD), two-way ANOVA with a Tukey’s multiple comparisons test and compact letter display (p < 0.05, n = 3).
We were interested in the La3+ found in the tube wall fraction. Another independent experiment in the same setting was conducted, and the 96 h samples of tube wall fractions were subjected to tryptic digestion and proteomics analysis. As shown in Supplementary Table S2, the only protein detected in the samples of the wild-type and lanM-overexpressing strains was flagellin. Various major proteins, including Fae1, MtdA, and PhaA, in addition to the flagellin were detected in the ΔlanM sample. Due to the intracellular nature of some of these proteins, these results suggested that the ΔlanM cells adhered to the tube wall in the presence of La3+, possibly due to damage or disintegration of the cell outer membrane, which did not happen in the wild-type and lanM-overexpressing strain. We also could not rule out possible cell lysis and resultant adhesion of proteins.
Subcellular Localization of LanM and La Deposition in the Cells
We next examined the subcellular localization of GFP-tagged LanM in ΔlanM. The GFP signal was found mainly in the periphery of the cells, and it was brighter in the presence of La3+ (Figure 8). The cells expressing only GFP showed intracellular GFP signals irrespective of La3+. With the presence of the signal peptide (Supplementary Figure S1B), this result suggested that LanM is a periplasmic protein.
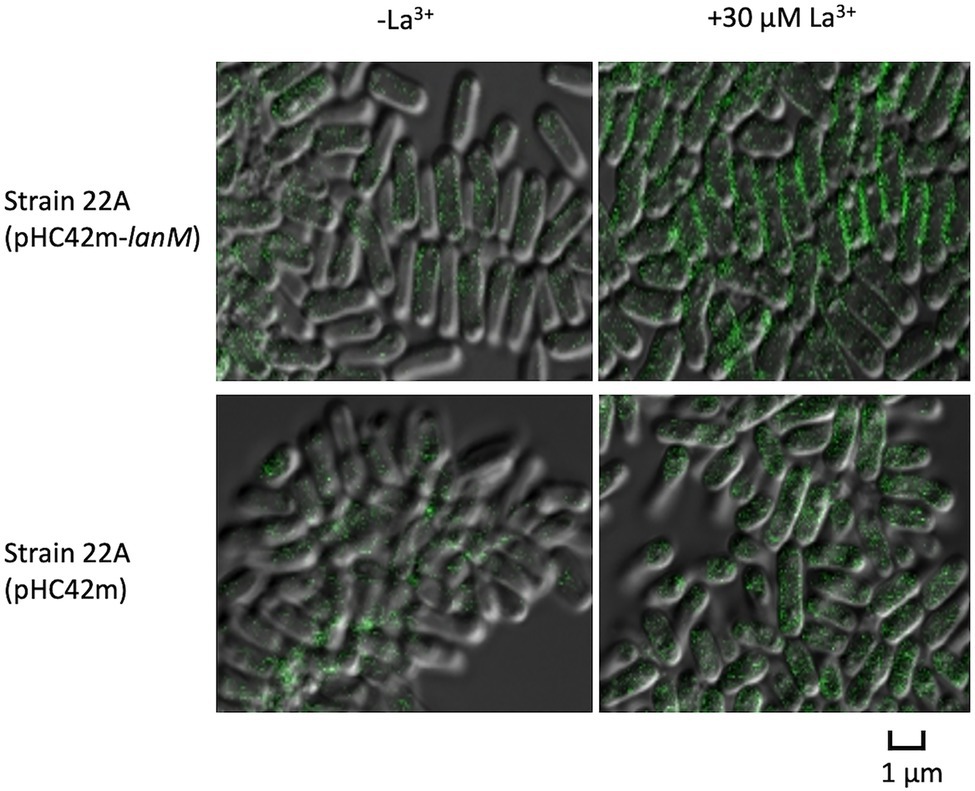
Figure 8. Cellular localization of GFP-tagged LanM in strain 22A cells. Strain 22A wild type containing pHC42m-lanM and pHC42m was grown on methanol in the absence/presence of 30 μM La3+ for 3 days and observed with confocal fluorescence microscopy. Bar, 1 μm.
Next, we observed the cells of the wild type, ΔlanM, and lanM-OX with a TEM (Figure 9A). The medium contained insoluble deposits in all samples, and the amount became smaller in 3 days than in 1 day, suggesting that the deposits were solubilized according to the cell growth. They were mixtures of at least two different substances (shown in the wild type, 3 day culture, and lanM-OX, 1 day culture); the denser particles were found to contain La, P, and O, and less dense particles were found to contain Fe, P, and O. Therefore, the La and Fe added in the medium was assumed to form insoluble particles with phosphates in our medium. Occasionally, electron-dense particles sized 0.1 to 0.2 μm could be observed in the cells of all samples, especially in the 3 day samples. We found that ΔlanM cells showed electron-dense deposits in the periplasm (Figure 9B). In the non-stained samples, we found electron-dense deposits in all cell samples; a typical one in the wild type (1 day) is shown in Figure 9C. EDX analysis showed that they contain La, P and O, whereas a part of cells that apparently not containing the deposit did not show any of these elements (data not shown). Occasionally, cells exhibited specific La-containing relatively large deposits at the periplasm. Typical ones in ΔlanM and lanM-OX are shown. Similar deposits have been also observed in M. extorquens strain AM1 lut gene mutants (Roszczenko-Jasińska et al., 2020) and Beijerinckiaceae bacterium RH AL1 (Wegner et al., 2021).
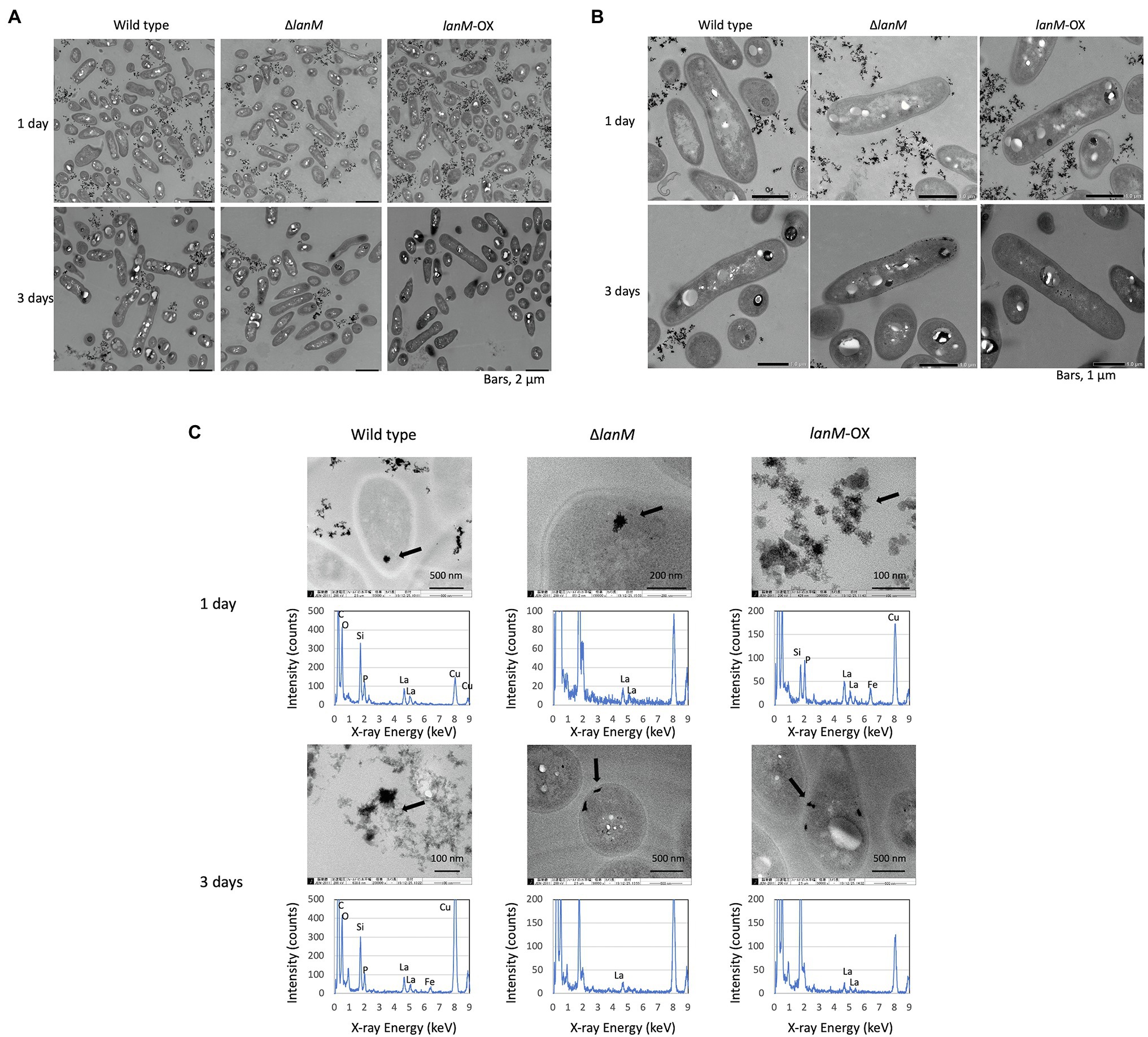
Figure 9. TEM images of wild type, ΔlanM, and ΔlanM (pAT01-Pfae1-lanM) grown in liquid mineral medium containing 0.5% methanol and 30 μM La3+. (A,B) Cells stained with uranyl acetate and lead citrate. (C) EDX analysis for electron-dense particles observed in each sample. Arrows indicate the EDX-analyzed positions. The detected Si and Cu are derived from TEM lens tube and grids, respectively.
Proteome Analysis for ΔlanM
Through SWATH-MS proteome analysis of the wild type and ΔlanM, we could quantify 1,263 proteins and 3,597 peptides in total (Supplementary Table S3). The differentially expressed proteins were extracted and are shown as a heatmap in Supplementary Figure S5. There was no protein involved directly in methylotrophy, which is understandable because ΔlanM did not show any defects in methanol growth. Maq22A_c16420 encoding an ABC transporter is most homologous to LutE (MexAM1_META1p1782), recently identified in M. extorquens strain AM1 as a component for an Ln3+ transporter across the inner membrane. The expression of the protein is upregulated in the wild type but repressed in ΔlanM in response to La3+, suggesting that lanM deletion resulted in restricted Ln3+ uptake into the cell. Transmembrane glycoprotein N-acetylglucosamine-1-phosphodiester-alpha-N-acetylglucosaminidase (Maq22A_c12225), UDP-diphospho-muramoylpentapeptide-beta-N-acetylglucosaminyltransferase (Maq22A_c12405), cell wall metabolism sensor kinase (Maq22A_1p36015), and D-alanyl-D-alanine carboxypeptidase (Maq22A_c17650) were differentially expressed together with fatty acid biosynthesis genes such as biotin carboxyl carrier protein (Maq22A_c14865) and 3-hydroxyacyl-CoA dehydrogenase (Maq22A_2p41960), suggesting that cell wall biogenesis is differentially regulated in ΔlanM. In addition, the differentially expressed inositol monophosphatase (Maq22A_c12285) and phosphate starvation protein PhoH (Maq22A_1p32125) suggested altered phosphate metabolism in the cell, as Ln3+ is known to bind phosphate. MxaK protein is involved in Ca2+ insertion into MxaF (Richardson and Anthony, 1992), which was also differentially regulated in ΔlanM.
MV Analysis
Confocal microscopy images of MVs extracted by the OMV Extraction Kit from the cultures of wild-type, ΔlanM, and lanM-OX grown in methanol medium supplemented with 30 μM LaCl3 showed that MVs from lanM-OX appeared darker than those from the others (Supplementary Figure S6). MVs were scarce in the ΔlanM culture. The MV fraction from LanM-OX collected by ultracentrifugation also showed more blackish particles compared to those from wild type and ΔlanM.
The La content of the kit-purified MV fractions was analyzed by ICP-MS. The amount of La normalized to the amount of protein revealed that LanM-OX showed the highest La content, although there were no statistically significant differences due to high variance (Supplementary Figure S7).
Next, the ultracentrifugation-purified MV fractions were subjected to LC–MS analysis. The detected proteins are summarized in Supplementary Table S4. The protein concentrations of the samples for LC–MS analysis were 1.2, 0.37, and 0.45 mg/ml for the wild type, ΔlanM, and LanM-OX, respectively. The presence of a number of outer membrane proteins, including porin and flagella proteins, indicated that the MV fractions indeed contained MVs. The sample from the wild type contained many cytoplasmic proteins, including those involved in methylotrophy, which might suggest some contamination of the cells or abundant proteins in the cells. The fractions from ΔlanM and lanM-OX contained some unique proteins, which are involved in flagella, porin, and an unknown outer membrane protein. Through this qualitative analysis, we did not identify any proteins that are apparently or possibly involved in Ln sequestration, however, it is of note that LanM protein and all other periplasmic proteins, such as MDHs, were not detected in the samples.
Discussion
In this study, we focused on LanM in strain 22A and attempted to determine its biological and physiological function. In our previous study, we predicted that the protein would bind Ln3+ (Masuda et al., 2018). Actually, LanM from M. extorquens strain AM1 was shown to bind Ln3+ (Cotruvo et al., 2018; Cook et al., 2019). However, no phenotype was observed in M. extorquens strain PA1 (Ochsner et al., 2019) and the screen-based on transposon mutagenesis did not identify lanM as a gene encoding a product essential for lanthanide-dependent growth (Roszczenko-Jasińska et al., 2020). It has been suggested that the protein shuttles Ln to Ln3+-dependent enzymes (Cotruvo et al., 2018; Cook et al., 2019). LanM also responds to Ln3+ in non-methylotrophy settings in a methylotrophic Beijerinckiaceae bacterium RH CH11 (Wegner et al., 2021).
lanM is associated with other lut genes in strain AM1 and PA1 genomes, but it is associated with the genes of different functions in strain 22A (Supplementary Figure S2). The amino acid sequence of TlyC (hemolytic element) includes a transmembrane domain (DUF21, pfam01595), a CBS_pair_CorC_HlyC_assoc (cd04590) domain, and a transporter-related domain (CorC_HlyC, smart01091). The latter two domains are related to magnesium and cobalt efflux. A hypothetical protein gene (c02045) adjacent to lanM has no known domains. Next, the gene encodes an adenylate cyclase that is a eukaryotic protein activated by calmodulin, to which LanM shows homology. In the case of pathogenic Bordetella pertussis, adenylate cyclase increases cAMP levels in the human host in response to host calmodulin (Voegele et al., 2018). Transcriptional regulators of the MucR family are involved in extracellular polysaccharide synthesis in Rhizobium species (UniProtKB-P55323). At the moment, it is unknown whether these gene products are associated with LanM function, but similar gene clusters are conserved in phylogenetically related species of Methylobacterium, suggesting their possible functional association.
The increased expression of lanM in response to La3+ (Masuda et al., 2018) was confirmed in this study (Figure 1). The conformational change upon binding of La3+ (Figure 2) is likely important for its interaction with other proteins. Regardless of La3+, LanM is not required for the wild type to grow on methanol, and growth of the lanM mutant is rather better than that for the wild type (Figure 3). LanM is not essential for XoxF expression and XoxF metalation (Figure 4). These data suggested that LanM is not necessary for the methylotrophy in strain 22A, despite its Ln3+-binding ability.
Ln3+ is transported into the periplasm by a TBDR, one of which has been identified in strain AM1. We found the most homologous TBDR gene and generated a knockout mutant, accompanied with mxcQE, which is involved in MDH regulation. We confirmed that tonB_Ln is necessary for XoxF-dependent methanol growth and that mxcQE is necessary for MxaF-dependent methanol growth (Supplementary Figure S4). PlanM is regulated by the mxcQE and tonB_Ln, and PlanM exhibits self-regulation, as observed in the case of PxoxF (Figure 5). In addition, PmxcQ showed a constant expression level independent of LaCl3 concentration in the wild type, whereas it showed an La-dependently decreasing expression level in ∆lanM (Figure 5). These results suggested that there is a system monitoring Ln levels through the lanM function. In the wild type, MxcQE expression is maintained constant regardless of the presence of Ln3+, which is controlled by LanM. Because MxcQE is responsible for MxaF expression, LanM is partly involved in the Ln-switch, although there was little change in the methylotrophic phenotype. The full picture of the Ln-switch mechanism is currently patchy, but here we can add LanM as one of the important players involved in the regulation. To clarify this complex mechanism, it is required to identify the ligands for, and the genes regulated by, the dual two-component signaling systems of MxcQE and MxbDM.
ΔlanM showed an obvious increase in cell aggregation and membrane permeability upon exposure to La3+ (Figure 6). These results suggested that ΔlanM altered the integrity of the cell membrane. Since ΔlanM did not exhibit growth retardation (the cell yield increased in the presence of high La3+, Figure 3), this altered membrane integrity was not detrimental to cells, but was rather temporal. The aggregation might suggest neutralization of the cell-surface negative charge by La3+, which would result in the cell adhesion to plastic tubes (Supplementary Table S2). LanM overexpressing strain showed faster La3+ uptake and subsequently higher amounts of extracellular soluble La (Figure 7). LanM localized to the periplasm (Figure 8), and ΔlanM showed La deposition in the periplasm (Figure 9). These results suggested that LanM simultaneously enhances both La3+ uptake and the efflux of soluble La3+ in the form of MV (Supplementary Figures S5–S7). Since LanM was not detected in the MV proteomics samples (Supplementary Table S4), there may be other proteins or factors that selectively translocate Ln (deposition) into the MV. Regulation of MV synthesis and selection of proteins into MV cargo are now an emerging field of microbiological studies (Toyofuku et al., 2018). MV formation has been suggested as one of the Ln uptake systems in the methylotrophic Beijerinckiaceae bacterium RH AL1 (Wegner et al., 2021). MV is also reported to be involved in the excretion of excess copper (Lima et al., 2022), suggesting that MV formation is one of the cellular strategies to excrete excess metals to maintain metal homeostasis. PQQ can bind Ln3+ (Lumpe and Daumann, 2019) as a possible factor for Ln excretion. PQQ is secreted to medium when strain 22A grows on methanol in the absence of La3+, but the presence of La3+ represses it (Masuda et al., 2018); this was also found in strain AM1 (Good et al., 2016). Thus, PQQ is also suggested to be one of the lanthanophores (Good et al., 2022), however, it is unknown whether PQQ is compartmented with Ln in the MV. The lanthanophore biosynthesis genes found in M. extorquens strain AM1 are similar to those of aerobactin siderophores (Zytnick et al., 2022). Such lanthanophores may work in Ln compartmentation into the MV. Thus, there is much to be investigated to fully understand the roles of these factors in Ln uptake and excretion. Taken together, we conclude that LanM maintains the Ln level in the periplasm by facilitating the uptake as well as excretion into MV, and this Ln-responsive process is regulated by MxcQE and the Ln-transporting TBDR, comprising a part of lanthanome and the Ln-switch.
Data Availability Statement
The original contributions presented in the study are included in the article/Supplementary Material, further inquiries can be directed to the corresponding author.
Author Contributions
YF and AT designed the research, performed the experiments, performed the data analysis, and drafted the manuscript. TS analyzed proteomics samples. All authors reviewed the manuscript. All authors contributed to the article and approved the submitted version.
Funding
This work was supported in part by MEXT KAKENHI (18H02129 and 21H02105 to AT). The funders had no role in study design, data collection and interpretation, or the decision to submit the work for publication.
Conflict of Interest
TS was employed by K.K. AB SCIEX.
The remaining authors declare that the research was conducted in the absence of any commercial or financial relationships that could be construed as a potential conflict of interest.
Publisher’s Note
All claims expressed in this article are solely those of the authors and do not necessarily represent those of their affiliated organizations, or those of the publisher, the editors and the reviewers. Any product that may be evaluated in this article, or claim that may be made by its manufacturer, is not guaranteed or endorsed by the publisher.
Acknowledgments
We are grateful to K. Koike and M. Maeda for TEM and EDX analyses carried out at the Natural Science Center for Basic Research and Development, Hiroshima University, S. Rikiishi (IPSR, Okayama University) for ICP-MS analysis, and T. Shiokawa and H. Tada in the Division of Instrumental Analysis, Okayama University, for CHIP-QTOF LC–MS analysis.
Supplementary Material
The Supplementary Material for this article can be found online at: https://www.frontiersin.org/articles/10.3389/fmicb.2022.921636/full#supplementary-material
Footnotes
1. ^https://www.ebi.ac.uk/Tools/psa/emboss_water/
2. ^https://services.healthtech.dtu.dk/service.php?SignalP-5.0
References
Alamgir, K. M., Masuda, S., Fujitani, Y., Fukuda, F., and Tani, A. (2015). Production of ergothioneine by Methylobacterium species. Front. Microbiol. 6:1185. doi: 10.3389/fmicb.2015.01185
Alessa, O., Ogura, Y., Fujitani, Y., Takami, H., Hayashi, T., Sahin, N., et al. (2021). Comprehensive comparative genomics and phenotyping of Methylobacterium species. Front. Microbiol. 12:2852. doi: 10.3389/fmicb.2021.740610/bibtex
Chistoserdova, L. (2016). Lanthanides: new life metals? World J. Microbiol. Biotechnol. 32:138. doi: 10.1007/s11274-016-2088-2
Chistoserdova, L. (2019). New pieces to the lanthanide puzzle. Mol. Microbiol. 111, 1127–1131. doi: 10.1111/mmi.14210
Chistoserdova, L., Kalyuzhnaya, M. G., and Lidstrom, M. E. (2009). The expanding world of methylotrophic metabolism. Annu. Rev. Microbiol. 63, 477–499. doi: 10.1146/annurev.micro.091208.073600
Chou, H. H., Berthet, J., and Marx, C. J. (2009). Fast growth increases the selective advantage of a mutation arising recurrently during evolution under metal limitation. PLoS Genet. 5:e1000652. doi: 10.1371/journal.pgen.1000652
Cook, E. C., Featherston, E. R., Showalter, S. A., and Cotruvo, J. A. (2019). Structural basis for rare earth element recognition by Methylobacterium extorquens Lanmodulin. Biochemistry 58, 120–125. doi: 10.1021/acs.biochem.8b01019
Cotruvo, J. A., Featherston, E. R., Mattocks, J. A., Ho, J. V., and Laremore, T. N. (2018). Lanmodulin: A highly selective lanthanide-binding protein from a lanthanide-utilizing bacterium. J. Am. Chem. Soc. 140, 15056–15061. doi: 10.1021/jacs.8b09842
Fitriyanto, N. A., Fushimi, M., Matsunaga, M., Pertiwiningrum, A., Iwama, T., and Kawai, K. (2011). Molecular structure and gene analysis of Ce3+-induced methanol dehydrogenase of Bradyrhizobium sp. J. Biosci. Bioeng. 111, 613–617. doi: 10.1016/j.jbiosc.2011.01.015
Gillet, C. L., Navarro, P., Tate, S., Röst, H., Selevsek, N., Reiter, L., et al. (2012). Targeted data extraction of the MS/MS spectra generated by data-independent acquisition: a new concept for consistent and accurate proteome analysis. Mol. Cell. Proteomics 11:016717. doi: 10.1074/mcp.O111.016717
Good, N. M., Lee, H. D., Hawker, E. R., Su, M. Z., Gilad, A. A., and Martinez-Gomez, N. C. (2022). Hyperaccumulation of gadolinium by Methylorubrum extorquens AM1 reveals impacts of lanthanides on cellular processes beyond methylotrophy. Front. Microbiol. 13:820327. doi: 10.3389/fmicb.2022.820327
Good, N. M., Vu, H. N., Suriano, C. J., Subuyuj, G. A., Skovran, E., and Martinez-Gomez, N. C. (2016). Pyrroloquinoline quinone ethanol dehydrogenase in Methylobacterium extorquens AM1 extends lanthanide-dependent metabolism to multicarbon substrates. J. Bacteriol. 198, 3109–3118. doi: 10.1128/JB.00478-16.Editor
Green, P. N., and Ardley, J. K. (2018). Review of the genus Methylobacterium and closely related organisms: A proposal that some Methylobacterium species be reclassified into a new genus, Methylorubrum gen. Nov. Int. J. Syst. Evol. Microbiol. 68, 2727–2748. doi: 10.1099/ijsem.0.002856
Green, P., and Bousfield, I. (1983). Emendation of Methylobacterium Patt, Cole, and Hanson 1976; Methylobacterium rhodinum (Heumann 1962) comb. nov. corrig.; Methylobacterium radiotolerans (Ito and Iizuka 1971) comb. nov. corrig.; and Methylobacterium mesophilicum (Austin and Goodfellow 1979) comb. nov. Int. J. Syst. Evol. Microbiol. 33, 875–877. doi: 10.1099/00207713-33-4-875
Hibi, Y., Asai, K., Arafuka, H., Hamajima, M., Iwama, T., and Kawai, K. (2011). Molecular structure of La3+-induced methanol dehydrogenase-like protein in Methylobacterium radiotolerans. J. Biosci. Bioeng. 111, 547–549. doi: 10.1016/J.JBIOSC.2010.12.017
Keltjens, J. T., Pol, A., Reimann, J., and Op Den Camp, H. J. M. (2014). PQQ-dependent methanol dehydrogenases: rare-earth elements make a difference. Appl. Microbiol. Biotechnol. 98, 6163–6183. doi: 10.1007/S00253-014-5766-8
Lima, S., Matinha-Cardoso, J., Giner-Lamia, J., Couto, N., Pacheco, C. C., Florencio, F. J., et al. (2022). Extracellular vesicles as an alternative copper-secretion mechanism in bacteria. J. Hazard. Mater. 431:128594. doi: 10.1016/j.jhazmat.2022.128594
Lowry, H. O., Rosbrough, J. N., Farr, L. A., and Randall, J. R. (1951). Protein measurement with the folin phenol reagent. J. Biol. Chem. 193, 265–275. doi: 10.1016/S0021-9258(19)52451-6
Lumpe, H., and Daumann, L. J. (2019). Studies of redox cofactor pyrroloquinoline quinone and its interaction with lanthanides(III) and calcium(II). Inorg. Chem. 58, 8432–8441. doi: 10.1021/acs.inorgchem.9b00568
Lv, H., Sahin, N., and Tani, A. (2018). Isolation and genomic characterization of Novimethylophilus kurashikiensis gen. Nov. sp. nov., a new lanthanide-dependent methylotrophic species of Methylophilaceae. Environ. Microbiol. 20, 1204–1223. doi: 10.1111/1462-2920.14062
Lv, H., Sahin, N., and Tani, A. (2020). Methylotenera oryzisoli sp. nov., a lanthanide-dependent methylotrophic bacteria isolated from rice field soil. Int. J. Syst. Evol. Microbiol. 70, 2713–2718. doi: 10.1099/IJSEM.0.004098
Marx, C. J., and Lidstrom, M. E. (2001). Development of improved versatile broad-host-range vectors for use in methylotrophs and other gram-negative bacteria. Microbiology 147, 2065–2075. doi: 10.1099/00221287-147-8-2065
Masuda, T., and Ishihara, Y. (2016). Sample preparation for shotgun proteomics by using phase transfer surfactants. Proteome Lett. 1, 95–100. doi: 10.14889/jpros.1.2_95
Masuda, S., Suzuki, Y., Fujitani, Y., Mitsui, R., Nakagawa, T., Shintani, M., et al. (2018). Lanthanide-dependent regulation of methylotrophy in Methylobacterium aquaticum strain 22A. mSphere 3, 1–16. doi: 10.1128/msphere.00462-17
Mattocks, J. A., Ho, J. V., and Cotruvo, J. A. (2019). A selective, protein-based fluorescent sensor with picomolar affinity for rare earth elements. J. Am. Chem. Soc. 141, 2857–2861. doi: 10.1021/jacs.8b12155
Nakagawa, T., Mitsui, R., Tani, A., Sasa, K., and Tashiro, S. (2012). A catalytic role of XoxF1 as La 3+-dependent methanol dehydrogenase in Methylobacterium extorquens strain AM1. PLoS One 7:50480. doi: 10.1371/journal.pone.0050480
Ochsner, A. M., Hemmerle, L., Vonderach, T., Nüssli, R., Bortfeld-Miller, M., Hattendorf, B., et al. (2019). Use of rare-earth elements in the phyllosphere colonizer Methylobacterium extorquens PA1. Mol. Microbiol. 111, 1152–1166. doi: 10.1111/mmi.14208
Pol, A., Barends, T. R. M., Dietl, A., Khadem, A. F., Eygensteyn, J., Jetten, M. S. M., et al. (2014). Rare earth metals are essential for methanotrophic life in volcanic mudpots. Environ. Microbiol. 16, 255–264. doi: 10.1111/1462-2920.12249
Richardson, I. W., and Anthony, C. (1992). Characterization of mutant forms of the quinoprotein methanol dehydrogenase lacking an essential calcium ion. Biochem. J. 287, 709–715. doi: 10.1042/bj2870709
Roszczenko-Jasińska, P., Vu, H. N., Subuyuj, G. A., Crisostomo, R. V., Cai, J., Lien, N. F., et al. (2020). Gene products and processes contributing to lanthanide homeostasis and methanol metabolism in Methylorubrum extorquens AM1. Sci. Rep. 10, 12663–12615. doi: 10.1038/s41598-020-69401-4
Schäfer, A., Tauch, A., Jäger, W., Kalinowski, J., Thierbach, G., and Pühler, A. (1994). Small mobilizable multi-purpose cloning vectors derived from the Escherichia coli plasmids pK18 and pK19: selection of defined deletions in the chromosome of Corynebacterium glutamicum. Gene 145, 69–73. doi: 10.1016/0378-1119(94)90324-7
Shilov, V. I., Seymour, L. S., Patel, A. A., Loboda, A., Tang, H. W., Keating, P. S., et al. (2007). The paragon algorithm, a next generation search engine that uses sequence temperature values and feature probabilities to identify peptides from tandem mass spectra. Mol. Cell. Proteomics 6, 1638–1655. doi: 10.1074/mcp.T600050-MCP200
Tani, A., Akita, M., Murase, H., and Kimbara, K. (2011). Culturable bacteria in hydroponic cultures of moss Racomitrium japonicum and their potential as biofertilizers for moss production. J. Biosci. Bioeng. 112, 32–39. doi: 10.1016/J.JBIOSC.2011.03.012
Tani, A., Takai, Y., Suzukawa, I., Akita, M., Murase, H., and Kimbara, K. (2012). Practical application of methanol-mediated mutualistic symbiosis between Methylobacterium species and a roof greening moss, Racomitrium japonicum. PLoS ONE 7:e33800. doi: 10.1371/JOURNAL.PONE.0033800
Toyofuku, M., Nomura, N., and Eberl, L. (2018). Types and origins of bacterial membrane vesicles. Nature Rev. Microbiol. 17, 13–24. doi: 10.1038/s41579-018-0112-2
Voegele, A., O’Brien, D. P., Subrini, O., Sapay, N., Cannella, S. E., Enguéné, V. Y. N., et al. (2018). Translocation and calmodulin-activation of the adenylate cyclase toxin (CyaA) of Bordetella pertussis. Pathog. Dis. 76, 1–10. doi: 10.1093/femspd/fty085
Vorholt, J. A. (2012). Microbial life in the phyllosphere. Nat. Rev. Microbiol. 10, 828–840. doi: 10.1038/nrmicro2910
Vu, H. N., Subuyuj, G. A., Vijayakumar, S., Good, N. M., Martinez-Gomez, N. C., and Skovran, E. (2016). Lanthanide-dependent regulation of methanol oxidation systems in Methylobacterium extorquens AM1 and their contribution to methanol growth. J. Bacteriol. 198, 1250–1259. doi: 10.1128/JB.00937-15
Wang, L., Suganuma, S., Hibino, A., Mitsui, R., Tani, A., Matsumoto, T., et al. (2019). Lanthanide-dependent methanol dehydrogenase from the legume symbiotic nitrogen-fixing bacterium Bradyrhizobium diazoefficiens strain USDA110. Enz. Microb. Technol. 130:109371. doi: 10.1016/j.enzmictec.2019.109371
Wegner, C. E., Gorniak, L., Riedel, S., Westermann, M., and Küsel, K. (2019). Lanthanide-dependent methylotrophs of the family Beijerinckiaceae: physiological and genomic insights. Appl. Environ. Microbiol. 86, e01830–e01819. doi: 10.1128/AEM.01830-19
Wegner, C. E., Westermann, M., Steiniger, F., Gorniak, L., Budharaja, R., Adrian, L., et al. (2021). Extracellular and intracellular lanthanide accumulation in the methylotrophic Beijerinckiaceae bacterium RH AL1. Appl. Environ. Microbiol. 87:e0314420. doi: 10.1128/AEM.03144-20
Wehrmann, M., Billard, P., Martin-Meriadec, A., Zegeye, A., and Klebensberger, J. (2017). Functional role of lanthanides in enzymatic activity and transcriptional regulation of pyrroloquinoline quinone-dependent alcohol dehydrogenases in Pseudomonas putida KT2440. mBio 8:e00570-17. doi: 10.1128/MBIO.00570-17
Whittenbury, R., and Dalton, H. (1981). “Chapter 71: The methylotrophic bacteria,” in The Prokaryotes. ed. Starr, P. M., 894–902.
Yanpirat, P., Nakatsuji, Y., Hiraga, S., Fujitani, Y., Izumi, T., Masuda, S., et al. (2020). Lanthanide-dependent methanol and formaldehyde oxidation in Methylobacterium aquaticum strain 22A. Microorganisms 8, 1–17. doi: 10.3390/microorganisms8060822
Ye, Y., Lee, H. W., Yang, W., Shealy, S., and Yang, J. J. (2005). Probing site-specific calmodulin calcium and lanthanide affinity by grafting. J. Am. Chem. Soc. 127, 3743–3750. doi: 10.1021/ja042786x
Zigler, J. S., Lepe-Zuniga, J. L., Vistica, B., and Gery, I. (1985). Analysis of the cytotoxic effects of light-exposed hepes-containing culture medium. In Vitro Cell. Dev. Biol. 21, 282–287. doi: 10.1007/BF02620943.s
Keywords: lanmodulin, lanthanide, methanol dehydrogenase, Methylobacterium species, membrane vesicles
Citation: Fujitani Y, Shibata T and Tani A (2022) A Periplasmic Lanthanide Mediator, Lanmodulin, in Methylobacterium aquaticum Strain 22A. Front. Microbiol. 13:921636. doi: 10.3389/fmicb.2022.921636
Edited by:
Masahiro Ito, Toyo University, JapanReviewed by:
Carl-Eric Wegner, Friedrich Schiller University Jena, GermanyNorma Cecilia Martinez-Gomez, University of California, Berkeley, United States
Copyright © 2022 Fujitani, Shibata and Tani. This is an open-access article distributed under the terms of the Creative Commons Attribution License (CC BY). The use, distribution or reproduction in other forums is permitted, provided the original author(s) and the copyright owner(s) are credited and that the original publication in this journal is cited, in accordance with accepted academic practice. No use, distribution or reproduction is permitted which does not comply with these terms.
*Correspondence: Akio Tani, YXRhbmlAb2theWFtYS11LmFjLmpw