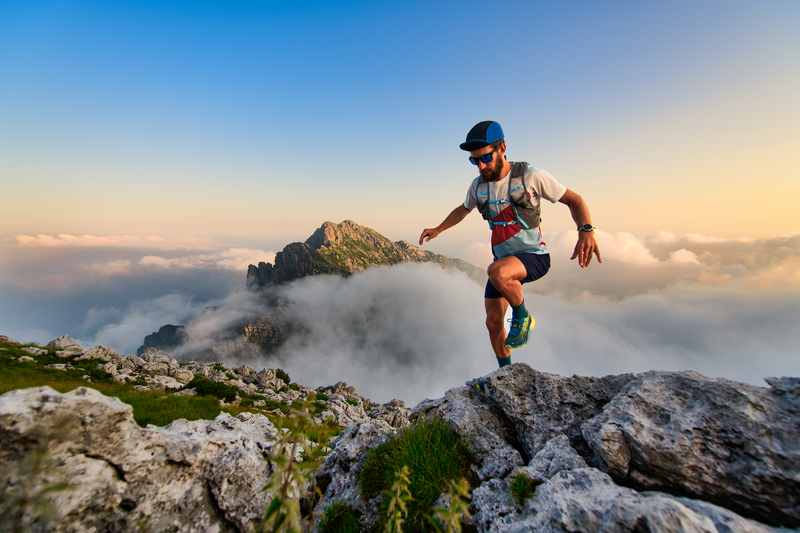
95% of researchers rate our articles as excellent or good
Learn more about the work of our research integrity team to safeguard the quality of each article we publish.
Find out more
ORIGINAL RESEARCH article
Front. Microbiol. , 09 August 2022
Sec. Virology
Volume 13 - 2022 | https://doi.org/10.3389/fmicb.2022.912147
Rotavirus is a major cause of gastroenteritis among infants and children. In this study, nested PCR assays were developed to amplify partial regions of the VP7, VP4, and VP6 genes of Rotavirus A (RVA) for amplicon-based Illumina MiSeq sequencing to investigate RVA genotypes in environmental water samples. Eight sets of inner primers were first designed and screened for use in the nested PCR assays, and four sets of them could produce amplicons. Six sets of outer primers were then designed and combined with the four sets of inner primers that worked. The assays were evaluated for sensitivity using raw water samples collected from one drinking water treatment plant between April 2019 and March 2020 (Sample Set 1; N = 12) and seven DWTPs between 2018 and 2020 (Sample Set 2; N = 18). In total, 43 amplicons from Set 1 were sequenced and diverse sequences from human, porcine, bovine, equine, and feline RVA were observed. Human G8, G3, and G2 genotypes were obtained, with G8 predominant (relative abundance, 36–87%) in samples taken during the rotavirus epidemic season between April and June. Porcine G5, G11, and G4, and bovine G10 and G6 genotypes were also detected. VP4 sequence analysis revealed that the human P[8] genotype was present throughout the year, whereas P[4] and P[9] were present only in the epidemic season. The vaccine strains P[5] and P[8] (RotaTeq®) were also detected. Our approach enables the identification of prevalent human and animal RVA genotypes and their host species that potentially caused fecal contamination in water sources.
Environmental water is often contaminated with wastewater from cities or farms and may contain both human and animal pathogens. Rotaviruses are viral agents that cause severe diarrheal disease and death in infants, children, and young animals (Dhama et al., 2009) and have been detected in both surface and groundwater sources (Miura et al., 2019, 2021; Sylvestre et al., 2021). Rotaviruses have been identified as potential reference pathogens in the WHO Guidelines for Drinking-Water Quality (WHO, 2017). To evaluate the risk from rotaviruses and maintain the safety of drinking water, a better understanding of the rotavirus genotypes that are present in water sources is required.
Rotaviruses belong to the genus Rotavirus in the family Reoviridae and have a non-enveloped structure with triple-layered icosahedral capsids that are approximately 100 nm in diameter including spikes. Rotavirus is currently divided into nine groups (Rotavirus A − D, F – J; ICTV, 2020). Rotavirus A − C has been found in both humans and animals (Estes and Greenberg, 2013) and Rotavirus A (RVA) is one of the important etiological agents of diarrhea in humans. The rotavirus genome consists of 11 double-stranded (ds)RNA segments that encode six structural proteins (VP1–4, VP6, and VP7) and six nonstructural proteins (NSP1–6; Estes and Greenberg, 2013). Based on the RNA sequences that encode VP7 (G: glycoprotein) and VP4 (P: proteinase-sensitive protein), RVA has been classified into the G and P genotypes (Estes and Greenberg, 2013). Recently, a whole-genome classification system was established based on 11 segments: Gx-P[x]-Ix-Rx-Cx-Mx-Ax-Nx-Tx-Ex-Hx, representing the VP7-VP4-VP6-VP1-VP2-VP3-NSP1-NSP2-NSP3-NSP4-NSP5/6 genotypes, respectively (“x” indicates the number of corresponding genotypes; Matthijnssens et al., 2011).
To detect and identify RVA strains in clinical samples, reverse transcription PCR (RT-PCR) assays have been designed for VP7 [e.g., Beg9/End9 primers (Gouvea et al., 1990)], VP4 [e.g., Con3/Con2 primers (Gentsch et al., 1992); Con1/Con4 primers (Fischer et al., 2003)], and VP6 [e.g., VP6-F/VP6-R primers (Iturriza Gómara et al., 2002)]. Nested PCR assays can be applied to sewage and river water samples using inner primers combined with outer primers that were developed for clinical sample analysis (Villena et al., 2003; Fumian et al., 2011; El-Senousy et al., 2020). The prevalence of G and P genotypes in water samples can be determined using nested PCR assays and amplicon-based Sanger sequencing. However, RVA concentrations in drinking water sources are generally too low to obtain amplicons from water using nested PCR assays.
Rotavirus A contamination in surface waters has previously been investigated using real-time PCR targeting the NSP3 gene (Prevost et al., 2015; Miura et al., 2019; Brooks et al., 2020) or the VP6 gene (Mackowiak et al., 2018), and median or mean concentrations ranging from 102 to 105 copies/L have been reported. A high prevalence (86%) and high concentrations (up to 5.5 log10 copies/L) of RVA were observed in surface water samples from 21 drinking water treatment plants (DWTPs) in Japan, suggesting RVA contamination with strains from livestock and vaccinated humans (Miura et al., 2019). Two rotavirus vaccines, Rotarix® (GlaxoSmithKline Biologicals, Rixensart, Belgium) and RotaTeq® (Merck & Co., Inc., Kenilworth, NJ, United States) introduced in Japan in November 2011 and July 2012, respectively, have been routinely given since October 2020 (Tsugawa et al., 2021). The VP6 gene that is specific to RotaTeq® was detected in sewage samples using real-time PCR (Gautam et al., 2014; Ito et al., 2021), raising questions about the impact of vaccine strains as well as wild-type strains on water contamination with RVA. Amplicons from previously reported nested PCR assays for VP7 and VP4 were commonly in the range 663–881 bp (Tort et al., 2015; Imagawa et al., 2020); however, shorter amplicons (200–300 bp) are preferable for next-generation sequencing (NGS) of RVA strains in water samples.
In this study, inner and outer primer sets for use in the nested PCR assays for amplifying partial regions of the VP7, VP4, and VP6 genes were newly designed to be suitable for NGS. The primer sets were tested for sensitivity using viral RNA samples from water samples collected at DWTPs. The amplicons obtained were subjected to NGS and the diversity and seasonal variations of the RVA genotypes were investigated.
To design primers specific to partial regions of the VP7, VP4, and VP6 genes in human or animal RVA, complete genome sequences were obtained from GenBank.1 The keywords “rotavirus,” “A,” “VP7, VP4, or VP6,” and “complete” were used to obtain 33 and 16 VP7, 25 and 23 VP4, and 24 and 14 VP6 sequences for human and animal RVA, respectively. The sequences were aligned using ClustalW in the MEGA X software (Kumar et al., 2018) and conserved regions were selected visually. Consensus sequences were generated for human or animal VP7, VP4, and VP6 using the “Extract Consensus Sequence” function in CLC Genomics Workbench ver. 11 (Qiagen, Hilden, Germany), and primer sequences were selected from the sequences with Primer3Plus (Untergasser et al., 2012). Eight sets of inner primers (expected amplicon sizes, 229 to 272) were designed and evaluated for their potential to amplify a target gene from viral RNA (screening of designed primers). Based on the result of screening that four inner primer sets could produce amplicons, six sets of outer primers (expected amplicon sizes, 299–462 bp) were then designed for use with the four inner primer sets.
Murine norovirus (MNV) strain S7-PP3 was provided by Dr. Yukinobu Tohya (Nihon University, Japan) for use as a process control to evaluate the virus recovery efficiencies from surface water samples. MNV was cultured using RAW 264.7 cells (ATCC TIB-71) following the methods described by Kitajima et al. (2010) and viruses were recovered and purified using a centrifugal ultrafiltration device (Miura et al., 2021). The purified MNV stock solution was maintained at −80°C until further use.
Viral RNA from two sets of surface water samples was used to select and evaluate the designed primer sets. Grab samples for Set 1 (N = 12) were collected monthly between April 2019 and March 2020 at DWTP E in the Kanto region. The grab samples were collected from a sampling tap connected to the raw water transmission main to the receiving tank in the plant. The plant, which treats river water, is downstream of several highly-populated cities and livestock farms. The pH, electrical conductivity (EC), and turbidity of the collected samples were in the ranges 7.1–7.5, 11–26 mS/m, and 1.3–34 turbidity units of polystyrene latex (TU-PSL), respectively.
To test the sensitivity of the nested PCR assays, Set 2 (N = 18) was used to ascertain the RVA levels (range, 3.4–6.3 log10 copies/L, determined by quantifying the NSP3 gene). The grab samples for Set 2 were collected from seven DWTPs (DWTP A, C, D, G, O, R, and U), which obtained raw water from rivers or reservoirs between 2018 and 2020. The DWTPs are located downstream of at least one wastewater treatment facility. Raw waters of these plants were also thought to contain effluents from wastewater treatment facilities, livestock farms, and the feces of wild animals (i.e., deer and boar). The pH, EC, and turbidity values of the collected samples were in the ranges 6.8–8.0, 9.1–34 mS/m, and 0.3–219 TU-PSL, respectively.
Water samples (5–6 l) were transported to the laboratory at the National Institute of Public Health within 2 days under cool conditions (0–10°C) and processed within 24 h of arrival.
Water samples were filtered (Miura et al., 2019) and concentrated via the adsorption-elution method (Katayama et al., 2002). Further details are given in the Supplementary material. Briefly, 1 l of the sample was inoculated with MNV (106–107 copies) and solids were removed by filtration through a 10-μm hydrophilic polytetrafluoroethylene membrane and a 0.45-μm hydrophilic mixed cellulose ester (MCE) membrane. The virus concentrate (10 ml) was obtained using the adsorption-elution method. DWTP E was affected by heavy rain in August 2019 and DWTP G by a typhoon in October 2019 (turbidity of 33.7 and 219 TU-PSL, respectively). Samples measuring 0.5 l and 0.2 l from these plants, respectively, were therefore processed.
Viral RNA was extracted using the NucliSENS kit (bioMérieux, Lyon, France), following the manufacturer’s instructions. Briefly, 1 ml of viral concentrate was processed using reagents from the kit with the NucliSENS miniMAG to obtain 100 μl of RNA extract. To denature dsRNA of RVA and appropriately measure RVA concentration using real-time RT-PCR, viral RNA extracts were heated at 95°C for 5 min and immediately incubated on ice for 2 min.
Viral RNA was amplified using previously developed primers and probes for the MNV (Kitajima et al., 2010) and RVA NSP3 genes (Pang et al., 2004, 2011; Miura et al., 2018; Supplementary Table S1) using the RNA UltraSense One-Step Quantitative RT-PCR System (Thermo Fisher Scientific, Tokyo, Japan) and the LightCycler 480 System II (Roche Diagnostics, Tokyo, Japan). The reaction conditions are described in the Supplementary material. The cycle threshold (CT) was defined as the cycle in which the fluorescence intensity increased significantly. Undiluted and 10-fold-diluted RNA extracts were analyzed, and the absence of RT-PCR inhibition was evaluated for each sample by comparing the ΔCT values against the slope of a standard curve (Miura et al., 2021). The number of genomic copies in each reaction was calculated by comparing the CT value with the standard curve generated from a dilution series (106–101 copies/well) of plasmids containing each target region. The genome concentration in the sample was calculated based on the volume of RNA extracts analyzed and expressed as the number of copies per liter. CT values < 40 were used for quantification. The limit for quantification was approximately 103 copies/L of RVA in surface water samples.
Murine norovirus was added to all the samples as the whole process control virus (Haramoto et al., 2018), and the percentage of virus recovery was calculated by dividing the amount of MNV detected in the virus concentrate by the amount of MNV added to the sample. Only samples with MNV recovery efficiencies greater than 1% were considered for quantification following the criteria described in ISO 15216-1:2017 for quantification of hepatitis A virus and norovirus in food and bottled water samples (ISO, 2017). The MNV recovery efficiency was used as a quality assurance parameter and was not used for the adjustment (Kazama et al., 2017).
To screen the potential inner primers, 40 cycles of PCR were conducted twice using selected water samples (N = 6) from Sample Set 1. The six samples were collected between April and September, which includes both epidemic and non-epidemic seasons, and RVA concentrations were in the range of 4.1–5.5 log10 copies/L. Complementary DNA (cDNA) was obtained through RT using the PrimeScript RT Master Mix (Perfect Real Time, Takara Bio Inc. Kusatsu, Japan). Briefly, 100 μl of reaction mixture contained 20 μl of 5 × PrimeScript RT Master Mix, 50 μl of viral RNA extracts, and 30 μl of deionized distilled water. RT was conducted at 37°C for 15 min and 42°C for 5 min, and enzyme inactivation was conducted at 85°C for 5 s.
PCR was performed in a 25-μl reaction mixture containing 12.5 μl of the Premix Ex Taq Hot Start Version (Takara Bio Inc.), 400 nM each of forward and reverse primers, and 3 μl of cDNA. The PCR conditions were: 40 cycles at 98°C for 10 s, 55°C for 30 s, and 72°C for 30 s. PCR products were purified using the QIAquick PCR Purification Kit (Qiagen). Then, 3 μl of the purified PCR products was subjected to a second PCR cycle under the same amplification conditions with the same inner primer sets. The PCR products were visualized using 1.5% agarose gel electrophoresis.
The cDNA obtained from sample sets 1 and 2 was subjected to nested PCR assays. Briefly, the first PCR was performed in a 50-μL reaction mixture containing 25 μl of the Premix Ex Taq Hot Start Version with 500 nM each of the outer primers (Table 1) and 10 μl of cDNA, followed by a second PCR using a 50 μl of the reaction mixture consisting of 25 μl of Premix Ex Taq Hot Start Version, 500 nM each of the inner primer (Table 1), and 2 μl of the first PCR products. PCR was performed for 35 cycles at 98°C for 10 s, 50°C for 30 s, and 72°C for 30 s. The second PCR products were visualized using 1.5% agarose gel electrophoresis, and those of the required length were purified using the QIAquick PCR Purification Kit.
To perform NGS on the RVA amplicons from Sample Set 1, unique adaptors were ligated at both the 5′ and 3′ ends of the amplicons by PCR for preparation of the DNA libraries. Further details are given in the Supplementary material. Briefly, an adapter sequence (SeqCap Adapter Kit, Roche Diagnostics, Basel, Switzerland) was ligated to the DNA fragments in the purified nested PCR products using KAPA Hyper Prep Kit (KAPA Biosystems, Inc., Wilmington, MA, United States). The ligation products were purified, and PCR (12 cycles) was performed to adjust the concentrations in the DNA libraries using KAPA HiFi Hot Start Ready Mix (KAPA Biosystems, Inc., Wilmington, MA, USA), followed by additional purification. The concentrations were measured and the quality and the expected peaks were checked using the Agilent 2,100 Bioanalyzer (Agilent Technologies, Inc., Santa Clara, CA, USA). The prepared DNA libraries were then sent to FASMAC (Atsugi, Japan), and the 229–272 bp paired-end RVA sequences were determined using the Illumina MiSeq platform (600 cycles; Illumina Inc., San Diego, CA, United States).
Sequencing data processing was performed using CLC Genomics Workbench ver.11. Paired-end reads were subjected to adapter clipping and quality trimming using “Trim sequences.” Reads with Phred quality scores of less than 30 were eliminated. The OTU sequences were the nucleotide sequences that represented units for classification for sequencing reads and were determined using “de novo OTU clustering” with default parameters (a minimum occurrence of 2, a chimera crossover cost of 3, and a k-mer size of 6). The sequence similarity was set to a minimum of 97%, which is generally used for OTU clustering in viruses (Moniruzzaman et al., 2016; Bisseux et al., 2020). The number of OTU sequences, reads that were unmapped to OTU sequences, and the total number of reads were then extracted. The OTU sequences in each water sample, into which more than 500 reads were classified, were analyzed further if their relative abundances were greater than 1%.
Sequences similar to the determined OTU sequences were identified using megaBLAST in BLASTN (Morgulis et al., 2008). The OTU sequence the similarity of which to RVA sequences recorded in GenBank was more than 80% was regarded as an RVA sequence. When the genotype of closely-related RVA sequences (> 80% similarity) was not reported, the genotype of OTU sequence was not determined. The selected OTU sequences were indexed with the RVA genotypes and the associated hosts. To evaluate the diversity of the RVA genotypes in each water sample, the relative abundance was calculated by dividing the number of OTU sequences assigned to each genotype by the number of OTU sequences identified as RVA. The genetic diversity of RVA in DWTP E was estimated by rarefaction analysis with Analytic Rarefaction ver 2.32 to check whether the genetic diversity was successfully investigated by NGS and to identify the diverse human VP7, VP4, VP6, and animal VP6 genotypes in each group.
To compare the OTU nucleotide sequences from the water samples with the closely-related reference sequences from the BLASTN search, phylogenetic analysis was performed for the G, P, and I genotypes. FASTA files for each group (human VP7, VP4, VP6, and animal VP6), which included both the determined OTU and the closely-related nucleotide sequences from GenBank, were prepared for analysis. The nucleotide sequences in the FASTA files were aligned using ClustalW in MEGA X. An appropriate nucleotide substitution model was selected using the command “Find best DNA/Protein Models (ML).” The Tamura 3 parameter model (Tamura, 1992) with gamma distribution was identified for all genotypes and applied to calculate the genetic distance. Bootstrapped phylogenetic trees were constructed based on the maximum likelihood estimation with 1,000 bootstrap replications using MEGA X. The estimated phylogenetic trees were output as a Newick file, which included information about the branch length and bootstrap values and were visualized using the “ggtree” package in the R software version 3.5.0.3
Nucleotide sequence data were deposited in the NCBI under accession number DRA012665.
The number of rotavirus gastroenteritis cases reported in the catchment upstream of DWTP E was obtained from the Infectious Diseases Weekly Report from the National Institute of Infectious Diseases, Japan (NIID, 2020). Core sentinel hospitals (approximately 500 in Japan) report the number of gastroenteritis cases caused by rotaviruses separately from those by other pathogens (e.g., noroviruses).
Based on the complete sequences of human and animal RVA VP7, VP4, and VP6 genes, eight sets of inner primers were designed and screened using selected samples (N = 6) from Sample Set 1. Amplicons were obtained for four sets of primers: H7F373/H7R611 for human VP7, H4F865/H4R1093 for human VP4, H6F745/H6R994 for human VP6, and A6F303/A6R574 for animal VP6 (Table 1). Amplicons of 229–272 bp for the screened inner primer sets were considered applicable for NGS.
To obtain amplicons with high sensitivity and specificity, outer primers were designed for the four successful inner primer sets. Six sets of outer primers that could be used for the first PCR were designed: H7F292_N/H7R638_N or H7F340_N/H7R638_N for human VP7, H4F793_N/H4R1149_N or H4F793_N/H4R1208_N for human VP4, H6F648_N/H6R1109_N for human VP6, and A6F192_N/A6R648_N for animal VP6 (Table 1). The amplicons comprising the outer primer sets were designed to be as small as possible (299–462 bp) to achieve high sensitivity.
MNV was inoculated into the water samples from DWTP E (Sample Set 1, N = 12), with recovery rates ranging from 13 to 77% [mean ± standard deviation (SD), 41 ± 21%]. Real-time RT-PCR was inhibited in 5 of the 12 samples, and the CT values of diluted RNA extracts were used for quantification of these five samples. The water samples from seven DWTPs (Sample Set 2, N = 18) resulted in MNV recovery rates ranging from 10 to 80% (mean ± SD, 36 ± 15%). Real-time RT-PCR was inhibited in 15 of the 18 samples (79%). The MNV recovery rates were therefore > 1% for both sample sets 1 and 2, indicating that no significant loss of viruses or RNA occurred during the concentration or extraction of viral RNA.
Rotavirus A concentrations were determined by quantifying the NSP3 gene, which ranged from 4.1 to 5.5 log10 copies/L in Sample Set 1 (Table 2, Figure 1). The concentration was high between April and August (4.9 to 5.5 log10 copies/L) and low between September and January, except for October (4.1 to 4.3 log10 copies/L). The RVA concentrations in Sample Set 2 ranged from 3.4 to 6.3 log10 copies/L (Table 3), which corresponds to the CT values of 26.90–39.81 for undiluted RNA extracts.
Figure 1. Concentration and genetic diversity of Rotavirus A (RVA) in water samples from DWTP E. The RVA NSP3 gene was quantitatively detected by real-time RT-PCR and plotted together with cases of rotavirus gastroenteritis reported for the week of sample collection in the prefecture upstream (A). Relative abundance (%) was calculated for RVA G genotypes determined by the VP7 gene (B), P genotypes determined by the VP4 gene (C), and I genotypes determined by the VP6 gene amplified in human (D) and animal (E) nested PCR assays. The value above each column of relative abundance represents the number of OTUs with > 500 reads.
Six sets of nested PCR assays were tested for sensitivity using Sample Set 1, with amplicons obtained from five (Table 2). Hereafter, each assay is specified by the names of outer primer sets. No amplicons for the human VP7 gene were obtained using the outer primer sets H7F292_N/H7R638_N; however, the amplicons were found using H7F340_N/H7R638_N in samples collected between April and July when RVA concentrations were high. Amplicons for the human VP4 gene were obtained only from high-concentration samples collected between April and June using the outer primers H4F793_N/H4R1149_N, whereas amplicons for the VP4 genes were obtained from all samples using H4F793_N/H4R1208_N. The two assays for human and animal VP6 genes could produce amplicons in all samples. The sensitivity of the five nested PCR assays was therefore considered sufficient for water samples affected by both human and animal feces throughout the year.
To further evaluate the sensitivity of the five assays where the amplicons were obtained, Sample Set 2 (N = 18) from seven DWTPs with wider RVA concentrations was employed (Table 3). Amplicons were obtained from 17 of the 18 samples by at least one of the assays. No amplicons were obtained from the sample with the second lowest concentration (3.5 log10 copies/L), which was affected by a typhoon (turbidity, 219 TU-PSL) before collection from DWTP G in October 2019, inhibiting real-time RT-PCR [based on the CT of undiluted and 10-fold diluted RNA extracts were 31.37 and 32.16, respectively (ΔCT = 0.79) for MNV detection; CT of undiluted RNA extract was 39.81 for NSP3 gene detection]. However, amplicons of human VP7, VP4, and VP6, and animal VP6 genes were successfully obtained from the sample with the lowest concentration (3.4 log10 copies/L). The nine samples in which the VP7 gene was amplified were also positive for the VP4 and VP6 genes. In contrast to Sample Set 1, the outer primers H4F793_N/H4R1149_N were found to obtain more amplicons than H4F793_N/H4R1208_N. The nested PCR assays for the animal VP6 gene could obtain amplicons from all samples, except the one that was affected by the typhoon. These results suggested that the five nested PCR assays have a potential to obtain amplicons from surface water samples as long as the NSP3 gene is detected by using real-time RT-PCR.
A total of 43 amplicon samples were obtained for human VP7, VP4, and VP6, and animal VP6 genes from Sample Set 1 and subjected to NGS. After filtering the nucleotide sequences (denoising and chimera removal), a total of 2,635,931 sequence reads were obtained as RVA reads, with a mean of 61,300 per amplicon sample. The mean number of OTUs with more than 500 reads per amplicon sample was 17.8 (range, 2–37, Supplementary Table S2). G, P, or I genotype was determined for each OTU sequence, and the relative abundance was calculated for each sample (Figure 1). Based on the megaBLAST analysis, we confirmed that there was no cross-reaction with non-rotavirus sequences. Rarefaction curves for each amplicon sample (Supplementary Figure S1) and prevalent genotype (Supplementary Figure S2) plateaued, indicating that the genetic diversity was comprehensively ascertained in the amplicon-based NGS.
Nine G genotypes (maximum seven genotypes per sample) were detected in water samples collected between April and July 2019 (Figure 1B). The human G8 genotype was predominant (relative abundance, 36–87%) in samples from April and June, the rotavirus season in Japan. The relative abundance of the G8 genotype decreased as reports of rotavirus infection decreased. The human G3 genotype was detected with a relative abundance of 2.4–5.8% during the epidemic season, while small amounts (0.7%) of the G2 genotype were found only in June. Although the assay was based on human VP7 sequences, porcine G5, G11, and G4, and bovine G10 and G6 genotypes were also detected. Porcine G5 was detected each month between April and July, and its relative abundance increased as human G8 decreased. Bovine G10 and G6 genotypes were detected sporadically in June.
Phylogenetic analysis of the VP7 sequences revealed that human G8 and G3, porcine G5, and bovine G10 (including giraffe G10) OTU sequences are clustered in two sub-clusters (Figure 2A). One G8 OTU sub-cluster contained sequences that were isolated from the stool samples of gastroenteritis patients in Japan (accession No., LC477362.1; isolated in 2017), the Czech Republic (accession No., MN401299.1; isolated in 2017), and South Korea (accession No., MN058762.1; isolated in 2017) (maximum and minimum sequence similarity within the sub-cluster: 0.966 and 0.996), while the other sub-cluster comprised only OTU sequences (maximum and minimum sequence similarity: 0.979 and 0.967). Similarly, the G3 OTU sequences in one sub-cluster were closely related to those isolated from gastroenteritis patients in Japan (accession No., LC340021.1 and AB919147.1; isolated in 2014 and 2011), Russia (accession No., KF018794.2; isolated in 2007), and Vietnam (accession No., LC433666.1; isolated in 2016), while the remaining OTU sequences formed another sub-cluster (maximum and minimum sequence similarity: 0.979 and 0.870).
Figure 2. Phylogenetic trees of Rotavirus A strains detected in surface water samples collected at DWTP E. Trees were built for partial regions of the VP7 (A), VP4 (B), and VP6 genes (C,D) obtained using human and animal nested PCR assays with maximum likelihood and bootstrapped with 1,000 repetitions. The sequence lengths of VP7, VP4, and human and animal VP6 were 242, 230, 239, and 274 bp, respectively. Circles with branch colors represent reference sequences that are closely related to OTU sequences. White and gray circles represent reference sequences for RotaTeq® and closely-related OTU sequences. The information of reference sequences is provided in Supplementary Table S3 in Supplementary material. Triangles at the nodes represent bootstrap rates of >0.8. The name of each sub-cluster consists of the genotype, followed by host or RotaTeq®, 1 (cluster with reference sequences) or 2 (cluster with only OTU sequences), and a or b for more than two sub-clusters. The values represent the minimum/maximum sequence similarity within each sub-cluster.
A total of eight P genotypes (a maximum of five genotypes per sample) were identified in the amplicons obtained by the two nested PCR assays for VP4 genes. The human P[8] genotype was detected throughout the year, except for December 2019 and March 2020, and was the predominant genotype (relative abundance, 22–100%) in most months (Figure 1C). Human P[4] and P[9] genotypes were detected during the epidemic season, amplified by the outer primers H4F793_N/H4R1149_N, and P[5] and P[8] RotaTeq® sequences were observed sporadically in June, July, and September 2019, and January and March 2020. Rotarix® sequences were not detected in any sample. Porcine and bovine genotypes were also detected. The porcine P[6] genotype was detected between June and March, with a relative abundance of 0.6–67%. The bovine P[5] genotype was found only in June, with a small abundance (3.7%), at the same time as bovine G10 and G6 genotypes were detected.
Operational taxonomic unit sequences belonging to the human P[8] genotype formed two sub-clusters in the phylogenetic tree, with some sequences closely related to those isolated from gastroenteritis patients in Singapore, Thailand, South Korea, Vietnam, Dominican Republic, United States, and Japan (MG996086.1, LC514473.1, MN058759.1, LC491560.1, MG652355.1, MF168111.1, and LC172539.1; Figure 2B). The sequence similarities within the human P[8] sub-cluster, which included reference sequences, were 0.996 and 0.889 at maximum and minimum, respectively. Another human P[8] sub-cluster composed of only OTU sequences also showed high sequence similarity (0.916 and 0.902 at maximum and minimum). The P[8] RotaTeq®-like OTU sequences formed another cluster (maximum and minimum sequence similarity: 0.978 and 0.964), and P[5] RotaTeq®-like OTU sequences formed two sub-clusters, one of which included a reference sequence (MF469343.1). The maximum/minimum sequence similarities of two P[5] RotaTeq sub-clusters were 0.978/0.827 (a sub-cluster including reference sequences) and 0.978/0.920 (another composed of only OTU sequences). Porcine P[6] OTU sequences in one of these sub-clusters were closely related to sequences isolated from pigs in the USA, Taiwan, Japan, and China (KR052749.1, MK227950.1, AB924098.1, and MG570048.1), human stool in Thailand (KY748311.1), and wild boar in Japan (AB573872.1; maximum and minimum sequence similarity: 0.991 and 0.818), while the other OTU sequences formed another sub-cluster (maximum and minimum sequence similarity: 0.987 and 0.840).
Analysis of the VP6 sequences obtained with the human VP6 nested PCR assay resulted in identification of the human I2 and I1 genotypes (Figure 1D). The human I2 genotype was predominant during the epidemic season, when it was detected every month with a relative abundance of 13–100%. The human I1 genotype was sporadic and abundant in October and December 2019 (66 and 38%, respectively) compared to the I2 genotype. Feline I2 and equine I2 genotypes were detected with relative abundances of 3.2 and 21%, respectively, in July 2019. The genotypes of some OTU sequences were not specified because no closely-related sequences were recorded in GenBank.
Of the VP6 sequences that were obtained by the animal VP6 nested PCR assay, the porcine I5 genotype detected between August 2019 and March 2020 was predominant (Figure 1E). The equine I3 genotype showed a relative abundance of 38% in July when the equine I2 genotype was present. Bovine I2 genotype was detected in November and December with relative abundances of 45 and 1.5%, respectively, whereas no bovine genotypes were detected in June when G10, G6, and P[5] were found. All of the OTU sequences obtained in April, May, June, and September were undetermined. Relatively low abundances of human I2 and I3 genotypes were detected between December and March (3.1–12%).
Phylogenetic analysis of the human VP6 nested PCR amplicons revealed diverse human I2 OTU sequences (Figure 2C). RotaTeq® is a pentavalent rotavirus vaccine that contains five human-bovine reassortant strains, in which the VP6 gene is classified as the bovine I2 genotype (Matthijnssens et al., 2010). I2 OTU sequences were closely related to a reference sequence of the reassortant vaccine strain G4 (KC215501.1), clustered into a sub-cluster of human and animal I2 genotypes (maximum and minimum sequence similarities: 1.000 and 0.958).
Porcine I5 OTU sequences formed three sub-clusters in the phylogenetic tree of the animal VP6 sequences (Figure 2D). One sub-cluster contained reference sequences from pigs in Spain, China, Slovakia, Taiwan, Japan, South Africa, Belgium, and the Czech Republic (MH238295.1, MH238299.1, MK936420.1, MK936421.1, EU372799.1, MK410285.1, MN203570.1, MK597975.1, MK597964.1, KF303566.1, MF139477.1, KU739976.1, AB924088.1, AB924099.1, KP753126.1, KM820727.1, and KU887650.1; maximum and minimum sequence similarities: 0.974 and 0.871), while the other sub-clusters comprised only OTU sequences (maximum and minimum sequence similarities: 0.974 and 0.897). Equine I3 OTU sequences were closely related to a reference sequence from a horse in Argentina (JX036369.1; maximum and minimum sequence similarities: 0.978 and 0.974) and the bovine I2 OTU sequences were closely related to reference sequences from cows in Japan (LC553621.1, AB853894.1, and AB573082.1; maximum and minimum sequence similarities: 0.967 and 0.949).
Surface water contains both human and animal RVA, and a method for analyzing low concentrations of the diverse RVA genotypes is required to evaluate the potential health risks associated with water usage. In this study, five nested PCR assays for human RVA VP7, VP4, and VP6 genes and the animal RVA VP6 gene were successfully developed. The amplicons derived from water samples obtained each month (Sample Set 1) were subjected to NGS and the diverse RVA genotypes and OTU sequences were obtained. The results indicated that the abundance of the identified RVA genotypes varied over the study period.
An amplicon-based NGS approach was applied to water samples collected at DWTPs to analyze the diverse RVA genotypes present. RVA concentrations are generally lower in drinking water sources than sewage, rendering it difficult to obtain appropriate amplicons for genotyping. The five nested PCR assays developed succeeded in amplifying parts of the human VP7, VP4, and VP6 genes and animal VP6 genes from water samples with concentrations as low as 3.4 log10 copies/L (CT, 37.67). Nine G (human G8, G3, G2, porcine G5, G11, G4, bovine G10, G6, and giraffe G10), eight P (human P[8], P[6], P[4], P[9], RotaTeq P[5], P[8], porcine P[6], bovine P[5]), and eight I (human I1, I2, I3, porcine I5, bovine I2, feline I2, equine I2, I3) genotypes were identified. Although obtaining a whole sequence of an RNA segment is informative, as reference sequences of the same genotype formed a distinct cluster in the phylogenetic trees, the sequence lengths of 200–300 bp can be applicable to determine RVA G, P, and I genotypes. The lengths were suitable for our purposes of obtaining amplicons from environmental water samples and performing NGS. Although both human and animal sequences were unexpectedly amplified partly because we designed degenerate primers, this was advantageous for our purpose to understand diverse RVA genotypes detected in environmental water. An amplicon-based NGS approach can also be applicable to other RVA genome segments; however, the number of recorded sequences would be smaller compared to VP7, VP4, and VP6 genes which have been used for conventional genotyping. As for the sewage samples where RVA concentrations were generally higher, the full length of VP7 (1,063 bp) and VP4 (2,359 bp) genes was obtained and 9 G and 13 P genotypes were detected from eight sewage samples in China by NGS (Du et al., 2021). Adriaenssens et al. (2018) used a viromic (viral metagenomics) approach to investigate human pathogenic RNA viruses in sewage and detected RVA genome fragments from the G10, G8, P[1], P[14] P[41], and I2 genotypes. To our knowledge, RVA genome fragments have not been detected in drinking water sources by using viromic approaches. Viromic approaches have a potential to detect novel viruses or genotypes (Nieuwenhuijse and Koopmans, 2017; Adriaenssens et al., 2018), while the amplicon-based approach can detect viruses in low concentrations and observe the genetic diversity in depth, as demonstrated in this study.
Human G8, P[8], and I2 genotypes, which were predominant in water samples from April 2019, decreased in relative abundance over the next few months, alongside a decrease in the number of cases reported (Figure 1). The human G3 genotype detected between April and June was at lower relative abundance than the G8 genotype. The G8P[8] genotype, which was first reported in Hokkaido in 2014 (Kondo et al., 2017), and the G8 genotype were predominant in patients with gastroenteritis during the epidemic season between 2018 and 2019 in Japan (Tsugawa et al., 2021). An equine-like G3P[4] strain was first isolated in Sendai in 2013 (Malasao et al., 2015) and equine-like G3P[8] strains have been identified in gastroenteritis patients since 2015 (Kikuchi et al., 2018; Komoto et al., 2018). The G8P[8], equine-like G3P[4], and equine-like G3P[8] strains have the I2 genotype (Malasao et al., 2015; Tsugawa et al., 2021), which is consistent with our observation that I2 was the predominant I genotype together with G8 and P[8] in the water samples. In 2018 and 2019, on average 1–3 cases of rotavirus-associated gastroenteritis were weekly reported in February or March in the catchment area (Supplementary Table S4; NIID, 2020), and the concentration of RVA in the surface water increased in 2019 (Supplementary Figure S3). However, no cases were reported in February and March 2020, and RVA concentrations remained stable (Figure 1). This may be due to the closure of schools from March to May 2020, when most schools and nurseries were closed in the coronavirus infectious disease 2019 pandemic. This may explain why the RotaTeq® P[5] genotype predominated water samples in March 2020. Thus, the RVA genotypes in the river water samples can be assumed to reflect the genotypes circulating in human populations, as demonstrated for noroviruses in sewage (Kazama et al., 2017).
In phylogenetic trees, some OTU sequences of the same genotype were classified into different sub-clusters (Figure 2). The sequence similarities between the sub-clusters which were classified as the same genotype were less than 0.7, which suggests that there are not a small number of non-synonymous mutations in one sub-cluster and such nontrivial mutations lead to the formation of multiple sub-clusters. Most OTU sequences of the VP4 gene were classified as P[8], which is composed of several clades, but the bootstrap probability did not exceed 0.8. These results indicate that the human P[8] genotype has high genetic diversity due to a high evolutionary rate, and many variants exist in drinking water sources. In addition to the VP4 gene, OTU sequences of the human I2 genotype within the same sub-clusters were closely related. As high genetic diversity is advantageous for viral spread (Bordería et al., 2015; Kadoya et al., 2020), it is important that the genetic diversity of RVA is appropriately decreased during the water treatment processes.
RotaTeq® P[5] and P[8] genotypes were detected regardless of season, and OTU sequences similar to the RotaTeq® reference sequences, which had not been detected in surface water, were identified in this study. Less RotaTeq® (7.8–11%) vaccinations were given in the catchment area between April 2019 and March 2020 compared to Rotarix® (66–94%), as estimated by the number of each vaccine provided by GlaxoSmithKline K.K. and MSD K.K. and the number of live births. Our observation that only RotaTeq® OTU sequences were identified in the surface water samples could be explained by the different use of Rotarix® and RotaTeq®, as pointed by Ito et al. (2021). Inoculation with Rotarix® is conducted twice before 24 weeks and RotaTeq® three times before 32 weeks. Infants generally begin weaning at 20 to 24 weeks in Japan, and their feces are incorporated into the sewage. Therefore, more RotaTeq® is likely to reach the environmental waters.
The porcine G5 genotype was more frequently detected than the G4 and G11 genotypes, with P[6] the only porcine P genotype identified. Pigs can become infected with several different strains of RVA and the G5 genotype was detected most frequently, followed by G11, G2, G9, and G4, at a farm in Japan (Miyazaki et al., 2012). The predominant RVA genotypes in each developmental stage change annually, with the G4P[13], G5P[6], and G9P[6] genotypes prevalent from 2006 to 2008 (Miyazaki et al., 2013). Although the predominant RVA genotypes in swine populations inhabiting the catchment area are unknown, the G5 and P[6] genotypes are likely to have dominated during the study period. Imagawa et al. (2020) reported that G5 was the most frequently detected genotype in river water samples from the Philippines during 2012–2013 and G5P[6] and G4P[6] were identified in clinical samples, which may point to reassortment between human and porcine RVA. Porcine G4 and G5 serotypes have been found to be closely related to RVA strains circulating in humans, suggesting that swine play a crucial role as reservoir and generator of newly adapted and emerging strains in humans (Dhama et al., 2009).
Although human G8, P[8], and I2 genotypes were predominant in the water samples collected between April and June, other host species identified by the detected genotypes did not always match within a water sample. This was because animal genotypes were detected as well as human genotypes and the amplification efficiencies may be different from strain to strain. As the nested PCR assays developed in this study may detect both human and animal RVA strains, the amplicons need to be sequenced. The two outer primer sets for VP4 (H4F793_N/H4R1149_N; H4F793_N/H4R1208_N) presented different results for sample sets 1 and 2, and human P[4] and P[9] genotypes were detected only by H4F793_N/H4R1149_N. As it has no known cause, the detection sensitivities and genotypes detected by the two outer primer sets require further evaluation. To increase the chance of obtaining amplicons, we recommend to use the two outer primer sets for the VP4 gene. These are the limitations in use of developed assays.
In this study, the OTU sequence, the similarity of which to RVA sequences recorded in GenBank was more than 80%, was regarded as an RVA sequence. We confirmed there were no non-rotavirus sequences based on the megaBLAST analysis of obtained OTU sequences. As for the undetermined RVA sequences, these sequences were certainly rotavirus ones but the genotype and associated host were not reported for the closely-related RVA sequences found in GenBank. If new animal source of rotavirus, new genotype, or variants are found and their sequences are registered properly, the genotype and host of currently undetermined RVA sequences can be revealed.
The genotype distribution of RVA in the epidemic season varies in different areas (Tsugawa et al., 2021). Therefore, continuous investigation of the RVA strains that are in circulation is necessary to elucidate the predominant genotype and detect the emergence of novel genotypes. The established primer sets successfully captured various RVA genotypes in the water and could be used to monitor changes in the RVA genotypes present. Genotypes with higher relative abundance are likely associated with RVA cases at the time of monitoring, meaning that monitoring is useful for alerting RVA outbreaks. Changes in the genotype distribution after mass vaccination in developing countries with limited clinical surveillance systems can also be evaluated using our approach. The reassortment of human RVA and RotaTeq® strains or human and animal RVA strains has been reported previously (Bucardo et al., 2012). As our primer sets detect both human and animal RVA, our approach may also be applicable to the investigation of animal RVA genotypes in the environment. However, it remains unknown which genotypes our primer sets can detect and whether specific genotypes are more prone to be captured by the primer sets.
In conclusion, nested PCR assays were newly developed to amplify partial regions of the VP7, VP4, and VP6 genes of RVA for amplicon-based NGS to investigate the RVA genotypes in water samples. The assays demonstrated sufficient sensitivity to obtain amplicons from drinking water sources with concentrations as low as 3.4 log10 copies/L, and a large diversity of RVA genotypes was identified. More observations from around the world would be useful to better understand the genetic diversity of both human and animal RVA in drinking water sources, which will be considered in risk assessment.
The datasets presented in this study can be found in online repositories. The names of the repository/repositories and accession number(s) can be found in the article/Supplementary material.
TM developed the concept and drafted the first manuscript. TM, S-sK, and HT performed the primer design, water sample processing, RT-PCR, and bioinformatics analyses. S-sK, HT, DS, and MA reviewed and edited the manuscript. All authors contributed to the article and approved the submitted version.
This work was supported by the Ministry of Health, Labour, and Welfare, Japan, through a Health and Labour Sciences Research Grant (19LA1005 and 22LA1007) and by the Japan Society for the Promotion of Science through KAKENHI (JP19K04680 and JP20H04359).
We are grateful to the waterworks personnel for collecting water samples. We also thank Nobuko Maeda, Nobue Yoshida, and Marina Tokuyasu (National Institute of Public Health) for their technical assistance.
The authors declare that the research was conducted in the absence of any commercial or financial relationships that could be construed as a potential conflict of interest.
All claims expressed in this article are solely those of the authors and do not necessarily represent those of their affiliated organizations, or those of the publisher, the editors and the reviewers. Any product that may be evaluated in this article, or claim that may be made by its manufacturer, is not guaranteed or endorsed by the publisher.
The Supplementary material for this article can be found online at: https://www.frontiersin.org/articles/10.3389/fmicb.2022.912147/full#supplementary-material
Adriaenssens, E. M., Farkas, K., Harrison, C., Jones, D. L., Allison, H. E., and McCarthy, A. J. (2018). Viromic analysis of wastewater input to a river catchment reveals a diverse assemblage of RNA viruses. mSystems 3, e00025–e00018. doi: 10.1128/mSystems.00025-18
Bisseux, M., Debroas, D., Mirand, A., Archimbaud, C., Peigue-Lafeuille, H., Bailly, J.-L., et al. (2020). Monitoring of enterovirus diversity in wastewater by ultra-deep sequencing: an effective complementary tool for clinical enterovirus surveillance. Water Res. 169:115246. doi: 10.1016/j.watres.2019.115246
Bordería, A. V., Isakov, O., Moratorio, G., Henningsson, R., Agüera-González, S., Organtini, L., et al. (2015). Group selection and contribution of minority variants during virus adaptation determines virus fitness and phenotype. PLoS Pathog. 11:e1004838. doi: 10.1371/journal.ppat.1004838
Brooks, Y. M., Spirito, C. M., Bae, J. S., Hong, A., Mosier, E. M., Sausele, D. J., et al. (2020). Fecal indicator bacteria, fecal source tracking markers, and pathogens detected in two Hudson River tributaries. Water Res. 171:115342. doi: 10.1016/j.watres.2019.115342
Bucardo, F., Rippinger, C. M., Svensson, L., and Patton, J. T. (2012). Vaccine-derived NSP2 segment in rotaviruses from vaccinated children with gastroenteritis in Nicaragua. Infect. Genet. Evol. 12, 1282–1294. doi: 10.1016/j.meegid.2012.03.007
Dhama, K., Chauhan, R. S., Mahendran, M., and Malik, S. V. S. (2009). Rotavirus diarrhea in bovines and other domestic animals. Vet. Res. Commun. 33, 1–23. doi: 10.1007/s11259-008-9070-x
Du, H., Xiong, P., Ji, F., Lin, X., Wang, S., Zhang, L., et al. (2021). Genetic diversity and molecular epidemiological characterization of group A rotaviruses in raw sewage in Jinan by next generation sequencing. Infect. Genet. Evol. 91:104814. doi: 10.1016/j.meegid.2021.104814
El-Senousy, W. M., Abu Senna, A. S. M., Mohsen, N. A., Hasan, S. F., and Sidkey, N. M. (2020). Clinical and environmental surveillance of rotavirus common genotypes showed high prevalence of common P genotypes in Egypt. Food Environ. Virol. 12, 99–117. doi: 10.1007/s12560-020-09426-0
Estes, M. K., and Greenberg, H. B. (2013). “Rotaviruses,” in Fields Virology. 6th Edn. eds. D. M. Knipe and P. M. Howley (Philadelphia, PA: Lippincott Williams & Wilkins), 1347–1401.
Fischer, T. K., Page, N. A., Griffin, D. D., Eugen-Olsen, J., Pedersen, A. G., Valentiner-Branth, P., et al. (2003). Characterization of incompletely typed rotavirus strains from Guinea-Bissau: identification of G8 and G9 types and a high frequency of mixed infections. Virology 311, 125–133. doi: 10.1016/S0042-6822(03)00153-3
Fumian, T. M., Gagliardi Leite, J. P., Rose, T. L., Prado, T., and Miagostovich, M. P. (2011). One year environmental surveillance of rotavirus specie A (RVA) genotypes in circulation after the introduction of the Rotarix® vaccine in Rio de Janeiro, Brazil. Water Res. 45, 5755–5763. doi: 10.1016/j.watres.2011.08.039
Gautam, R., Esona, M. D., Mijatovic-Rustempasic, S., Ian Tam, K., Gentsch, J. R., and Bowen, M. D. (2014). Real-time RT-PCR assays to differentiate wild-type group A rotavirus strains from Rotarix® and RotaTeq® vaccine strains in stool samples. Hum. Vaccin. Immunother. 10, 767–777. doi: 10.4161/hv.27388
Gentsch, J. R., Glass, R. I., Woods, P., Gouvea, V., Gorziglia, M., Flores, J., et al. (1992). Identification of group A rotavirus gene 4 types by polymerase chain reaction. J. Clin. Microbiol. 30, 1365–1373. doi: 10.1128/jcm.30.6.1365-1373.1992
Gouvea, V., Glass, R. I., Woods, P., Taniguchi, K., Clark, H. F., Forrester, B., et al. (1990). Polymerase chain reaction amplification and typing of rotavirus nucleic acid from stool specimens. J. Clin. Microbiol. 28, 276–282. doi: 10.1128/jcm.28.2.276-282.1990
Haramoto, E., Kitajima, M., Hata, A., Torrey, J. R., Masago, Y., Sano, D., et al. (2018). A review on recent progress in the detection methods and prevalence of human enteric viruses in water. Water Res. 135, 168–186. doi: 10.1016/j.watres.2018.02.004
Imagawa, T., Saito, M., Yamamoto, D., Saito-Obata, M., Masago, Y., Ablola, A. C., et al. (2020). Genetic diversity of species A rotaviruses detected in clinical and environmental samples, including porcine-like rotaviruses from hospitalized children in the Philippines. Infect. Genet. Evol. 85:104465. doi: 10.1016/j.meegid.2020.104465
ISO (2017). Microbiology of the Food chain—Horizontal Method for Determination of Hepatitis A Virus and Norovirus using real-time RT-PCR — Part 1: Method for Quantification. Geneva: ISO. 15216-1.
Ito, E., Pu, J., Miura, T., Kazama, S., Nishiyama, M., Ito, H., et al. (2021). Detection of rotavirus vaccine strains in oysters and sewage and their relationship with the gastroenteritis epidemic. Appl. Environ. Microbiol. 87:e02547–20. doi: 10.1128/AEM.02547-20
Iturriza Gómara, M., Wong, C., Blome, S., Desselberger, U., and Gray, J. (2002). Molecular characterization of VP6 genes of human rotavirus isolates: correlation of genogroups with subgroups and evidence of independent segregation. J. Virol. 76, 6596–6601. doi: 10.1128/jvi.76.13.6596-6601.2002
Kadoya, S.-S., Urayama, S.-I., Nunoura, T., Hirai, M., Takaki, Y., Kitajima, M., et al. (2020). Bottleneck size-dependent changes in the genetic diversity and specific growth rate of a rotavirus A strain. J. Virol. 94, e02083–e02019. doi: 10.1128/JVI.02083-19
Katayama, H., Shimasaki, A., and Ohgaki, S. (2002). Development of a virus concentration method and its application to detection of enterovirus and Norwalk virus from coastal seawater. Appl. Environ. Microbiol. 68, 1033–1039. doi: 10.1128/aem.68.3.1033-1039.2002
Kazama, S., Miura, T., Masago, Y., Konta, Y., Tohma, K., Manaka, T., et al. (2017). Environmental surveillance of norovirus genogroups I and II for sensitive detection of epidemic variants. Appl. Environ. Microbiol. 83:e03406-16. doi: 10.1128/AEM.03406-16
Kikuchi, W., Nakagomi, T., Gauchan, P., Agbemabiese, C. A., Noguchi, A., Nakagomi, O., et al. (2018). Detection in Japan of an equine-like G3P[8] reassortant rotavirus A strain that is highly homologous to European strains across all genome segments. Arch. Virol. 163, 791–794. doi: 10.1007/s00705-017-3668-7
Kitajima, M., Oka, T., Takagi, H., Tohya, Y., Katayama, H., Takeda, N., et al. (2010). Development and application of a broadly reactive real-time reverse transcription-PCR assay for detection of murine noroviruses. J. Virol. Methods 169, 269–273. doi: 10.1016/j.jviromet.2010.07.018
Komoto, S., Ide, T., Negoro, M., Tanaka, T., Asada, K., Umemoto, M., et al. (2018). Characterization of unusual DS-1-like G3P[8] rotavirus strains in children with diarrhea in Japan. J. Med. Virol. 90, 890–898. doi: 10.1002/jmv.25016
Kondo, K., Tsugawa, T., Ono, M., Ohara, T., Fujibayashi, S., Tahara, Y., et al. (2017). Clinical and molecular characteristics of human rotavirus G8P[8] outbreak strain, Japan, 2014. Emerging Infectious Disease journal 23, 968–972. doi: 10.3201/eid2306.160038
Kumar, S., Stecher, G., Li, M., Knyaz, C., and Tamura, K. (2018). MEGA X: molecular evolutionary genetics analysis across computing platforms. Mol. Biol. Evol. 35, 1547–1549. doi: 10.1093/molbev/msy096
Mackowiak, M., Leifels, M., Hamza, I. A., Jurzik, L., and Wingender, J. (2018). Distribution of Escherichia coli, coliphages and enteric viruses in water, epilithic biofilms and sediments of an urban river in Germany. Sci. Total Environ. 626, 650–659. doi: 10.1016/j.scitotenv.2018.01.114
Malasao, R., Saito, M., Suzuki, A., Imagawa, T., Nukiwa-Soma, N., Tohma, K., et al. (2015). Human G3P[4] rotavirus obtained in Japan, 2013, possibly emerged through a human–equine rotavirus reassortment event. Virus Genes 50, 129–133. doi: 10.1007/s11262-014-1135-z
Matthijnssens, J., Ciarlet, M., McDonald, S. M., Attoui, H., Bányai, K., Brister, J. R., et al. (2011). Uniformity of rotavirus strain nomenclature proposed by the rotavirus classification working group (RCWG). Arch. Virol. 156, 1397–1413. doi: 10.1007/s00705-011-1006-z
Matthijnssens, J., Joelsson, D. B., Warakomski, D. J., Zhou, T., Mathis, P. K., van Maanen, M.-H., et al. (2010). Molecular and biological characterization of the 5 human-bovine rotavirus (WC3)-based reassortant strains of the pentavalent rotavirus vaccine, RotaTeq®. Virology 403, 111–127. doi: 10.1016/j.virol.2010.04.004
Miura, T., Gima, A., and Akiba, M. (2019). Detection of norovirus and rotavirus present in suspended and dissolved forms in drinking water sources. Food Environ. Virol. 11, 9–19. doi: 10.1007/s12560-018-9361-5
Miura, T., Schaeffer, J., Le Saux, J.-C., Le Mehaute, P., and Le Guyader, F. S. (2018). Virus type-specific removal in a full-scale membrane bioreactor treatment process. Food Environ. Virol. 10, 176–186. doi: 10.1007/s12560-017-9330-4
Miura, T., Takino, H., Gima, A., Haramoto, E., and Akiba, M. (2021). Recovery of nucleic acids of enteric viruses and host-specific Bacteroidales from groundwater by using an adsorption-direct extraction method. Appl. Environ. Microbiol. 87:e00710–21. doi: 10.1128/AEM.00710-21
Miyazaki, A., Kuga, K., Suzuki, T., Kohmoto, M., Katsuda, K., and Tsunemitsu, H. (2013). Annual changes in predominant genotypes of rotavirus A detected in the feces of pigs in various developmental stages raised on a conventional farm. Vet. Microbiol. 163, 162–166. doi: 10.1016/j.vetmic.2012.11.044
Miyazaki, A., Kuga, K., Suzuki, T., and Tsunemitsu, H. (2012). Analysis of the excretion dynamics and genotypic characteristics of rotavirus A during the lives of pigs raised on farms for meat production. J. Clin. Microbiol. 50, 2009–2017. doi: 10.1128/JCM.06815-11
Moniruzzaman, M., Gann, E. R., LeCleir, G. R., Kang, Y., Gobler, C. J., and Wilhelm, S. W. (2016). Diversity and dynamics of algal Megaviridae members during a harmful brown tide caused by the pelagophyte, Aureococcus anophagefferens. FEMS Microbiol. Ecol. 92:fiw058. doi: 10.1093/femsec/fiw058
Morgulis, A., Coulouris, G., Raytselis, Y., Madden, T. L., Agarwala, R., and Schäffer, A. A. (2008). Database indexing for production MegaBLAST searches. Bioinformatics 24, 1757–1764. doi: 10.1093/bioinformatics/btn322
Nieuwenhuijse, D. F., and Koopmans, M. P. G. (2017). Metagenomic sequencing for surveillance of food-and waterborne viral diseases. Front. Microbiol. 8:230. doi: 10.3389/fmicb.2017.00230
NIID (2020). Infectious Diseases Weekly Report, Surveillance Data Table [Online]. National Institute of Infectious Diseases. Available at: https://www.niid.go.jp/niid/en/survaillance-data-table-english.html (Accessed December 22, 2021).
Pang, X., Cao, M., Zhang, M., and Lee, B. (2011). Increased sensitivity for various rotavirus genotypes in stool specimens by amending three mismatched nucleotides in the forward primer of a real-time RT-PCR assay. J. Virol. Methods 172, 85–87. doi: 10.1016/j.jviromet.2010.12.013
Pang, X. L., Lee, B., Boroumand, N., Leblanc, B., Preiksaitis, J. K., and Yu Ip, C. C. (2004). Increased detection of rotavirus using a real time reverse transcription-polymerase chain reaction (RT-PCR) assay in stool specimens from children with diarrhea. J. Med. Virol. 72, 496–501. doi: 10.1002/jmv.20009
Prevost, B., Lucas, F. S., Goncalves, A., Richard, F., Moulin, L., and Wurtzer, S. (2015). Large scale survey of enteric viruses in river and waste water underlines the health status of the local population. Environ. Int. 79, 42–50. doi: 10.1016/j.envint.2015.03.004
Sylvestre, É., Prévost, M., Burnet, J.-B., Pang, X., Qiu, Y., Smeets, P., et al. (2021). Demonstrating the reduction of enteric viruses by drinking water treatment during snowmelt episodes in urban areas. Water Res. X 11:100091. doi: 10.1016/j.wroa.2021.100091
Tamura, K. (1992). Estimation of the number of nucleotide substitutions when there are strong transition-transversion and G+C-content biases. Mol. Biol. Evol. 9, 678–687. doi: 10.1093/oxfordjournals.molbev.a040752
Tort, L. F. L., Victoria, M., Lizasoain, A., García, M., Berois, M., Cristina, J., et al. (2015). Detection of common, emerging and uncommon VP4, and VP7 human group A rotavirus genotypes from urban sewage samples in Uruguay. Food Environ. Virol. 7, 342–353. doi: 10.1007/s12560-015-9213-5
Tsugawa, T., Akane, Y., Honjo, S., Kondo, K., and Kawasaki, Y. (2021). Rotavirus vaccination in Japan: efficacy and safety of vaccines, changes in genotype, and surveillance efforts. J. Infect. Chemother. 27, 940–948. doi: 10.1016/j.jiac.2021.04.002
Untergasser, A., Cutcutache, I., Koressaar, T., Ye, J., Faircloth, B. C., Remm, M., et al. (2012). Primer3—new capabilities and interfaces. Nucleic Acids Res. 40, e115. doi: 10.1093/nar/gks596
Villena, C., El-Senousy, W. M., Abad, F. X., Pintó, R. M., and Bosch, A. (2003). Group A rotavirus in sewage samples from Barcelona and Cairo: emergence of unusual genotypes. Appl. Environ. Microbiol. 69, 3919–3923. doi: 10.1128/aem.69.7.3919-3923.2003
Keywords: rotavirus, massive parallel sequencing, molecular epidemiology, surface water, drinking water source
Citation: Miura T, Kadoya S-s, Takino H, Sano D and Akiba M (2022) Temporal variations of human and animal Rotavirus A genotypes in surface water used for drinking water production. Front. Microbiol. 13:912147. doi: 10.3389/fmicb.2022.912147
Received: 04 April 2022; Accepted: 07 July 2022;
Published: 09 August 2022.
Edited by:
Cristina García-Aljaro, University of Barcelona, SpainReviewed by:
Leena Maunula, University of Helsinki, FinlandCopyright © 2022 Miura, Kadoya, Takino, Sano and Akiba. This is an open-access article distributed under the terms of the Creative Commons Attribution License (CC BY). The use, distribution or reproduction in other forums is permitted, provided the original author(s) and the copyright owner(s) are credited and that the original publication in this journal is cited, in accordance with accepted academic practice. No use, distribution or reproduction is permitted which does not comply with these terms.
*Correspondence: Takayuki Miura, bWl1cmEudC5hYUBuaXBoLmdvLmpw
Disclaimer: All claims expressed in this article are solely those of the authors and do not necessarily represent those of their affiliated organizations, or those of the publisher, the editors and the reviewers. Any product that may be evaluated in this article or claim that may be made by its manufacturer is not guaranteed or endorsed by the publisher.
Research integrity at Frontiers
Learn more about the work of our research integrity team to safeguard the quality of each article we publish.