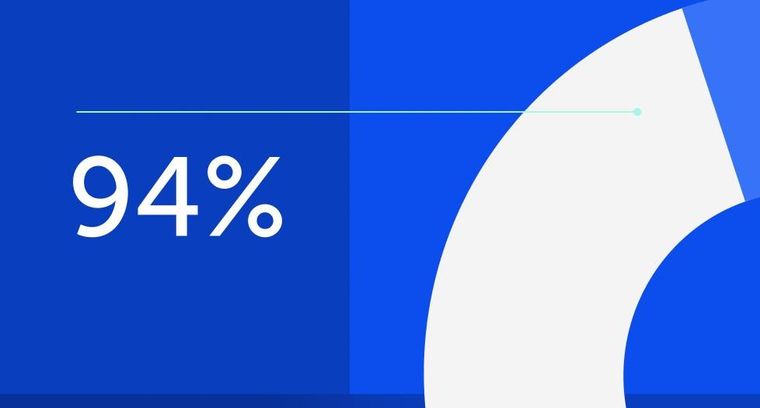
94% of researchers rate our articles as excellent or good
Learn more about the work of our research integrity team to safeguard the quality of each article we publish.
Find out more
ORIGINAL RESEARCH article
Front. Microbiol., 17 June 2022
Sec. Microbiological Chemistry and Geomicrobiology
Volume 13 - 2022 | https://doi.org/10.3389/fmicb.2022.909109
This article is part of the Research TopicElectromicrobiology – From Electrons To Ecosystems, Volume IIView all 12 articles
Geobacter sulfurreducens is a widely applied microorganism for the reduction of toxic metal salts, as an electron source for bioelectrochemical devices, and as a reagent for the synthesis of nanoparticles. In order to understand the influence of metal salts, and of electron transporting, multiheme c-cytochromes on the electron flux during respiration of G. sulfurreducens, the reduction kinetic of Fe3+, Co3+, V5+, Cr6+, and Mn7+ containing complexes were measured. Starting from the resting phase, each G. sulfurreducens cell produced an electron flux of 3.7 × 105 electrons per second during the respiration process. Reduction rates were within ± 30% the same for the 6 different metal salts, and reaction kinetics were of zero order. Decrease of c-cytochrome concentrations by downregulation and mutation demonstrated that c-cytochromes stabilized respiration rates by variation of their redox states. Increasing Fe2+/heme levels increased electron flux rates, and induced respiration flexibility. The kinetic effects parallel electrochemical results of G. sulfurreducens biofilms on electrodes, and might help to optimize bioelectrochemical devices.
Geobacter sulfurreducens has found important applications in remediation of oxidizing and toxic metal salts (Lovley et al., 2011; Pushkar et al., 2021), as an electron source of microbial fuel cells (Bond and Lovley, 2003; Slate et al., 2019), as well as a reagent for the synthesis of nanoparticles (Lloyd et al., 2011; Khan et al., 2020; Egan-Morriss et al., 2022). This microorganism, first discovered and isolated by Lovley et al. (1987) and Caccavo et al. (1994) relies on different extracellular minerals (Lovley and Phillips, 1988; Shi et al., 2016) and metal salts as electron acceptors (Ding et al., 2008; Lloyd et al., 2011; Lovley et al., 2011; Levar et al., 2014). Respiration experiments on insoluble minerals, and electrochemical studies on solid electrodes have shown that the electron donor NADH in the cytoplasm and the extracellular electron acceptors are separated from each other by the periplasm, delimited, respectively, by the inner and by the outer cell membranes. Electron transport occurs via multi-heme-bearing cytochromes (Ueki, 2021), some of which are soluble in the periplasm (Ppc), while others are attached to the inner (Imc) or the outer cell membrane (Omc). Cytochromes of G. sulfurreducens, which are involved in the respiration process, exist in many varieties and contain on average 7.5 iron-hemes (Ueki, 2021), and the total number of iron-hemes per cell is about 107 (Esteve-Núñez et al., 2008). Electron transfer through the periplasm is mainly based on triheme cytochromes (Ppc), some of them as protein clusters (Santos et al., 2015). During the stationary phase of the bacteria, all iron/hemes are in the Fe2+ state. They become rapidly oxidized to Fe3+/hemes (Chabert et al., 2020) upon addition of metal salts with appropriate redox potentials (Santos et al., 2015; Levar et al., 2017). Fe3+/hemes then oxidize NADH via the menaquinol/menaquinone pool, and the resulting proton gradient catalyzes ATP synthesis. In a recent study on the formation of Ag nanoparticles (AgNPs) by G. sulfurreducens respiration with water soluble AgNO3, we have demonstrated that Ag+ ions are bound by outer membrane cytochromes with high complexation constants (Chabert et al., 2020). Subsequent electron transfer in the Ag+/Omc complexes triggered a fast electron flux through G. sulfurreducens, leading to AgNPs at the outer cell membrane (Figure 1). The constant electron flux rate of 3⋅105 e–⋅s–1 per cell was independent of the Ag+ ion concentration, and agreed well with electrochemical measurements on single cells of G. sulfurreducens (Jiang et al., 2013), as well as Shewanella oneidensis (Gross and El-Naggar, 2015). We have now measured reduction rates, and kinetic orders of additional water-soluble metal salts by G. sulfurreducens. [Fe(edta)]–, [Fe(CN)6]3–, Co[(bpy)2CO3]+, [VO2(edta)]3–, CrO42–, and MnO4– ions were chosen as oxidants, because their concentrations, and the redox changes of c-cytochromes could be exactly determined during the fast respiration processes by time resolved experiments. G. sulfurreducens cells in the resting and the exponential growth phases were used. Their c-cytochrome concentrations were changed by downregulation and mutation.
Figure 1. Synthesis of Ag nanoparticles (AgNPs) during respiration of G. sulfurreducens with water soluble Ag+ ions. The c-type cytochromes at the inner cell membrane (Imc), in the periplasm (Ppc), and at the outer cell membrane (Omc) transport electrons from intracellular electron donors like NADH to Ag+/Omc complexes, which leads to AgNPs, attached to the outer cell membrane (Chabert et al., 2020). In recent cell growth experiments with CrO42– (Gong et al., 2018), Cr3+ reduction products could be detected within the cells, if the bacteria reacted for several hours with the chromium salts. Therefore, our reduction experiments of CrO42– might also occur partly inside of the cells, although the reaction conditions are different.
G. sulfurreducens (DSM-12127) was received from the Leibniz Institute DSMZ. Preparation of standard G. sulfurreducens solutions in growth medium A: 5 ml of the purchased bacteria solution were solved in 50 ml of growth medium A (Supplementary Figure 1), which contained in the first growth round 10 mM KCl and 100 μM FeSO4. After 5 days of growing, 5 ml of this bacterial solution was added into 50 ml of a growth medium A that contained 2 μM KCI and 25 μM FeSO4. This growing procedure with 25 μM FeSO4 took about 3–5 days until the fumarate was consumed, and was repeated 4 times. Inductively coupled plasma optical emission spectroscopy (ICP-OES) showed that after this growth process, the bacterial solution of G. sulfurreducens contained ≤ 10 μM iron ion concentrations. These standard solutions in medium A without fumarate, which contained G. sulfurreducens in the resting state (lag phase) and acetate as carbon source (Estevez-Canales et al., 2015), were directly used for the reduction of the water-soluble metal salts. Preparation of G. sulfurreducens solutions in growth medium B: 5 ml of a standard G. sulfurreducens solution, which was prepared in growth medium A, and contained 10 μM Fe2+, was added to 50 ml of growth medium B lacking FeSO4 (Estevez-Canales et al., 2015; Supplementary Figure 1). After 5 days, these solutions in growth medium B, which contained G. sulfurreducens in the resting state and acetate as a carbon source (Estevez-Canales et al., 2015), were directly used for the reduction of the water soluble metal salts. The decrease of c-cytochrome amounts in G. sulfurreducens by growth in medium B compared to medium A was determined by mass spectrometric proteome analysis: disruption of cells and protein extraction were done in a sample homogenizer after adding a lysis buffer (8 M urea, 50 mM Tris-Cl, pH 8) and glass beads (0.18 mm). The same protein amount for each sample was further processed as described in Stekovic et al. (2020). MS raw files were analyzed using the Spectronaut software version 15.7 (Bruderer et al., 2015) with standard settings (without data imputation) in direct DIA mode using reference proteome of G. sulfurreducens (UniProt, UP000000577) and common contaminants. Further data processing and statistical analysis used the Perseus software version 1.6. The results are shown in Supplementary Figure 2 and Table 1. The mutant lacking OmcBEST of G. sulfurreducens (PCA) was provided by Derek R. Lovley (University of Massachusetts, Amherst, United States). One ml was cultured in 10 ml of NBAF medium under anaerobic conditions as described in Coppi et al. (2001). After 5 days of growing, when G. sulfurreducens was again in the lag phase, 5 ml were solved in 50 ml of growth medium A, and reacted for 5 days until G. sulfurreducens was again in the lag phase. This growing procedure was repeated 4 times, and the solutions were used directly for the kinetic experiments with water-soluble metal salts.
Table 1. Functions and remaining percentages of c-cytochromes that were downregulated by at least 50% during fumarate-respiring growth of G. sulfurreducens in medium B compared to growth in medium A (100%).
Na[Fe(edta)], K3[Fe(CN)6], K2CrO4, and KMnO4 were purchased from Sigma-Aldrich. Na3[VO2(edta)] was synthesized according to Komarova et al. (1991). [Co(bpy)2CO3]Cl was generated from [Co(bpy)3]Cl3, which was solved in growth medium A without fumarate and acetate. O2 was exchanged by N2/CO2 (80/20) and the solution was heated to 125°C for 20 min at 1.25 bar. The structure of [Co(bpy)2CO3]Cl was confirmed by single crystal X-ray diffraction (SC-XRD) and electrospray ionization mass spectrometry (ESI-MS). Concentration decrease of the Fe3+, Co3+, V5+, Cr6+, and Mn7+ salts, which were reduced by G. sulfurreducens to Fe2+, Co2+, V4+, Cr3+, and Mn4+ salts, respectively, was analyzed by UV/Vis spectroscopy at wavelengths shown in Figure 2A and Supplementary Figure 3. In order to determine the location of the oxidizing metal salts after G. sulfurreducens respiration, solutions of 4.65 mM Na[Fe(edta)], K3[Fe(CN)6], [Co(bpy)2CO3]Cl, Na3[VO2(edta)], as well as 1.55 mM K2CrO4 and KMnO4, respectively, were treated for 20 min with N2/CO2 (80/20) at ambient temperature. Then, 0.2 ml of them were added to 6 ml of a standard G. sulfurreducens solution. After 1 h reaction time (30°C) 0.35 ml of a 37% HCl solution were added, mixed with a vortex for about 1 min, and centrifuged at 10,000 rpm at 20°C for 10 min. Inductively coupled plasma optical emission spectroscopy (ICP-OES) demonstrated that about 90% of the metal salts were observed outside of the cells, and analysis of the Cr3+ distribution (Gong et al., 2018) showed that up to 93% of the chromium ions were found in the supernatant, about 6% at the cell membrane, and less than 1% was detected inside of the cells (Figure 2B).
Figure 2. Analytical tools for the analysis of extracellular metal ion, Fe2+/heme, and cell concentrations. (A) Reduction of oxidizing metals salts by G. sulfurreducens by time dependent UV/Vis spectroscopy at wavelengths where product absorptions are negligible. CrO42– respiration is shown as an example. (B) ICP analysis of Cr3+ ions outside of the cell (blue) and at the cell membrane (orange) after reduction of CrO42–. Percentage data for reduced metal salts in the supernatant are given. (C) Changes of Fe2+/heme concentration during respiration using the areas of Q-bands. (D) Increase of UV/Vis absorptions during bacteria growth with fumarate as intracellular oxidant. The insert shows scattering increases at 600 nm (OD600) during 2 days of cell growth. (E) UV/Vis absorptions during cell growth using low chromate concentrations for G. sulfurreducens respiration, starting from the resting state.
Concentrations of Fe2+/hemes were analyzed by their Q-band areas between 540 and 570 nm (Figure 2C), and in some cases also by their Soret band at 420 nm (Figure 2A). Ultrasound treatment, which destroyed the cell membranes of G. sulfurreducens, did not increase the total UV/Vis absorption of iron-hemes. This demonstrates that G. sulfurreducens cells are transparent enough to detect all iron-hemes of the bacteria. Filtration of G. sulfurreducens solutions gave a tiny peak (≤5%) of iron hemes in the supernatant (Supplementary Figure 4). With about 107 iron hemes per cell (Esteve-Núñez et al., 2008) and 10–12 M cell concentrations of our experiments, these 5% lead to less than 1 μM Fe2+/heme solutions outside of the cell, which could at best reduce less than 1% of 0.1 mM extracellular metal ion salt solutions.
Cell growth was analyzed by spectroscopy at 600 nm (Muhamadali et al., 2015). G. sulfurreducens cells are about 1–2 μm large, thus a concentration increase raised the light scattering effect on the UV/Vis spectra (Figure 2D). Test experiments with 40 mM fumarate as an internal oxidant for the cell growth proved that OD600 data followed the same exponential increase as experiments, where cell growth in solution was determined by increase of the cell weight (Engel et al., 2020). Dilution of clear cell solutions changed the OD600 values in a linear way, and an OD600 value of 0.54 corresponds to 0.7 pM G. sulfurreducens (Vasylevskyi et al., 2017). The change of OD600 values during respiration was detected with high accuracy, so that it could also be used to follow cell growth in experiments with low oxidant concentrations (Figure 2E). Rates of metal salt induced cell growth were measured at 30°C under anaerobic conditions: 0.1 ml of a K3[Fe(CN)6] solution was added to 3 ml of a standard G. sulfurreducens solution. The initial concentration of the oxidant in the reaction mixture was 0.15 mM, and the cell growth was analyzed at OD600. Analogous experiments with K2CrO4 were carried out with reaction mixture concentrations of 0.03 and 0.05 mM.
Kinetic measurements were carried out in standard G. sulfurreducens solutions in the lag phase (see above) with 0.15 mM Na[Fe(edta)], K3[Fe(CN)6], Co[(bpy)2CO3]Cl, Na3[VO2(edta)], and 0.05 mM K2CrO4 and KMnO4 solutions, respectively. Under these conditions, the oxidizing metal salts did not kill the bacterial cells, and the reaction mixtures remained homogeneous. All experiments were repeated 3 times at 30°C under N2/CO2 (80/20). Addition of 0.05 or 0.1 ml metal salt solutions to 3 ml standard G. sulfurreducens in the lag phase occurred by injection through a sealing plug with needles that had been sterilized with a Bunsen burner. Oxidants were used in such amounts that their initial concentrations in the reaction mixtures were 0.05 mM for Cr6+ or Mn7+, and 0.15 mM for Fe3+, Co3+, and V5+, respectively. The concentrations of G. sulfurreducens were calculated from the OD600 data. UV/Vis spectra were recorded between 610 and 320 nm. Each run took 25.3 s. Concentration changes of metal salts were analyzed at wavelengths that are listed in Figure 2A and Supplementary Figure 3. Concentration changes of Fe2+/hemes were determined by the areas of the Q-bands (540, 570 nm) if Na[Fe(edta)], K3[Fe(CN)6], KMnO4, and K2CrO4 were used as oxidants (Figure 2C). Because Co2+ and V4+ salts absorb at the Q-bands wavelengths, the differences of Soret bands at 420 nm (Fe2+/heme) and 410 nm (Fe3+/heme) were used. The determined concentrations of oxidizing metal salts and Fe2+/hemes were plotted against reaction times. The linear time dependences of the metal salt concentrations (Figure 3A) are the electron flux rates, and division by G. sulfurreducens concentrations led to the electron flux rate per cell (Figure 3D).
Figure 3. Kinetic experiments of G. sulfurreducens with extracellular metal salts. (A) Reduction of 6 different oxidizing metal salt solutions (blue, 0.05–0.15 mM) with G. sulfurreducens solutions in the resting state (8.9 ± 0.3 pM). Their linear time dependences of the metal salt concentrations demonstrate zero kinetic order of the reduction process. At the start of the experiments, all iron ions of the bacterial multiheme cytochromes were in the Fe2+ state (red). They were rapidly oxidized upon addition of extracellular metal salts, their levels remained low during reduction of the metal salts, and increased again after major consumption of the oxidants. (B) Experiments could be repeated several times. Shown are Fe2+/heme levels upon three consecutive additions of CrO42–. (C) A closer look at the Fe2+/heme (red) and CrO42– (blue) concentrations. The Fe2+ /heme levels increased after major part of CrO42– was reduced, which obviously stabilized EET rates at low concentrations of the extracellular metal salts. (D) Zero order reduction rates per cell for the 6 different metal salts at 30°C and pH = 7.4.
All experiments were carried out under strictly anaerobic conditions at 30°C. To start the growth process with fumarate as an oxidant, 5 ml of the standard G. sulfurreducens solution were added to 50 ml of growth medium A. The growing process was analyzed by taking probes every 3 h and measuring the OD600 values (Figure 4A). After about 30 h, 3 ml of a G. sulfurreducens solution, which was then in the exponential growth (log) phase, was injected with a sterilized needle through a sealing plug into an UV cuvette. The OD600 values of G. sulfurreducens were about 0.50. To this G. sulfurreducens solution, which contained all iron-hemes in the Fe2+ oxidation state, 0.05 ml of a K2CrO4 solution was added, so that the initial concentration of CrO42– was 0.05 mM (experiment 1, Figures 4B,C). Another experiment with G. sulfurreducens (OD600 = 0.65), which had continued its growth with fumarate as oxidant, was carried out about 6 h later: 3 ml of G. sulfurreducens in the exponential growth (log) phase were injected into an UV cuvette, and 0.1 ml of a CrO42– solution was added, so that the reaction mixture was at the start 0.1 mM in CrO42– (experiment 2, Figures 4B,D). Concentration changes of CrO42– and Fe2+/hemes were analyzed from the UV/Vis spectra at 345 nm and the Q-band, respectively.
Figure 4. CrO42– induce respiration of G. sulfurreducens, starting from the log phase. (A) Fumarate- respiring growth of G. sulfurreducens. Bacteria after 30 and 36 h growth, respectively, were used for metal induced respiration of G. sulfurreducens in the log phase. (B) Fe2+/heme levels during CrO42– induced respiration of bacteria starting from log phases 1 and 2, as well as the resting (lag) phase. (C) Reduction of CrO42– (0.05 mM) by bacteria (0.63 ± 0.2 pM) in the log phase 1 and the lag phase. (D) Reduction of CrO42– (0.1 mM) by bacteria (0.83 ± 0.2 pM) in the log phase 2 and the lag phase.
Reactions of 0.15 mM Na[Fe(edta)], K3[Fe(CN)6], Co[(bpy)2CO3]Cl, Na3[VO2(edta)], and 0.05 mM K2CrO4 as well as KMnO4 solutions with 0.89 pM G. sulfurreducens in the resting phase, solved in media as described above, oxidized the Fe2+/hemes of cytochromes to Fe3+/hemes within a few seconds, and a steady metal salt reduction occurred over 10 min (Figure 3A). The concentration of the electron transporting Fe2+/hemes remained nearly constant at a low level until up to 80% of the metal salt reductions were completed (Figures 3A,C). After about 7–9 min, when most of metal salts had been reduced, Fe2+/hemes were regenerated by cellular processes, and the bacteria are ready for a second round of the metal ion reduction process (Figure 3B). The electron flux did not change although concentrations of metal salts decreased. Their linear time dependences are the overall reduction rates, and division by G. sulfurreducens concentrations yielded electron flux rates per single cell. Data for CrO42– and MnO4– were furthermore multiplied by the stoichiometric factor of 3, considering a three electron transfer. All six metal salts led to the fast electron flux rate of 3.7⋅105 e–⋅s–1 per cell with a reproducibility of ± 1.2⋅105 e–⋅s–1 (Figure 3D). Thus, reduction rates were not only independent of metal salt concentrations, but also of the metal salt types. The variation of bacteria or initial metal salt concentrations by a factor of 2 did not change reduction rates per cell (Supplementary Figure 5). A fivefold increase of extracellular metal salts started to deactivate cells, which led to a slowdown of the reduction, indicated by a curvature of the time dependent metal salt reduction, and a lower regeneration of the Fe2+/hemes. Additional experiments showed that reduction of metal salts could neither be detected with dead G. sulfurreducens cells nor with the supernatant of living cells, and Cr2(SO4)3 or K3[Co(CN)6], which cannot oxidize Fe2+/hemes of cytochromes, did not drive the respiration.
Experiments were carried out with bacteria, which contained either lower c-cytochrome concentrations, or reacted faster with the metal salts. A downregulation of c-cytochromes was carried out by preparation of G. sulfurreducens cells in a growth medium of low Fe2+ concentration (medium B). Mass spectrometric proteome analysis detected 2,579 proteins after growth in medium A as well as in medium B, from which 64 are c-cytochromes (Supplementary Figure 2). Two thirds of them were downregulated by cell growth in medium B, 18 by more than 50% (Table 1). Comparison with literature data demonstrated that 17 of these 18 cytochromes are involved in the EET process. Most of them are located in the periplasm, from which three are predicted to be bound to the outer membrane, two to the inner membrane, and two cytochromes are outer membrane complexes. As a consequence, the total iron heme concentrations decreased by 50% in the downregulated cells (Figure 5A). Reduction experiments of these bacteria with metal salts showed that electron flux rates remained constant, whereas the Fe2+/heme levels increased considerably (Figure 5B). Obviously, constant rates could be maintained in cells of lower c-cytochrome concentrations by rising the Fe2+/heme levels. A similar effect was observed with a mutant, where outer membrane cytochromes OmcB, OmcE, OmcS, and OmcT were deleted (Figure 5C).
Figure 5. Changes of Fe2+/heme levels during metal induced respiration of G. sulfurreducens. (A) Linear correlation (red line) between Fe2+/heme concentrations (Q-bands) and G. sulfurreducens concentrations (OD600 data). The data (red points) were gained by dilution and different cell growth experiments in medium A. Measurement (magenta point) with G. sulfurreducens, grown in medium B, where c-cytochromes are downregulated. (B) Fe2+/heme levels during CrO42– induced respiration (0.05 mM) of cells grown in media A (0.63 pM) and B (0.73 pM). (C) Fe2+/heme levels (red) and CrO42– concentrations (blue) during CrO42– induced respiration (0.05 mM) of mutants lacking OmcBEST (0.76 pM) grown in medium A. (D) Fe2+/heme levels of experiments with G. sulfurreducens (0.74 pM), grown in medium B, with [Fe(edta)] – (0.15 mM) and CrO42– (0.05 mM), respectively.
An increase of electron flux rates was achieved by experiments starting with G. sulfurreducens in the exponential growth phase (log phase). In order to carry out these measurements, cells were prepared under fumarate-respiring conditions (Butler et al., 2006). The log phase started after several hours, reached a rate maximum at about 30 h, then slowed down, and stopped during the third day (Figure 4A). In the first hours of this growth process, Fe2+/hemes were partly oxidized but became reduced again during the log phase (Supplementary Figure 6). Once all iron hemes of G. sulfurreducens were in the Fe2+ state, K2CrO4 was added to the growing G. sulfurreducens solution, and the CrO42– reduction rate was measured (Figures 4C,D). Experiments at different growth times showed that electron flux was 4–6 times faster in the log phase than with cells starting from the lag phase. Cells in the exponential growth demands faster ATP production, which can be achieved by an increase of the electron flux rate. This was made possible by an increase of the Fe2+/heme levels in the c-cytochromes from 5% (lag phase) to up to 75% (log phase) during the metal salt reduction (Figure 4B).
Respiration induces the formation of ATP, which leads to bacterial growth in ATP dependent processes (Brown, 1992; Velten et al., 2007; Bochdansky et al., 2021). To elucidate whether ATP formation and ATP consumption occur with the same rates, the decrease of the metal salts, and the increase of cell growth were measured. Reactions started with G. sulfurreducens in the resting state and medium A as solvent, which lacks fumarate as oxidant. Respiration was induced by addition of 0.03–0.05 mM CrO42– or 0.15 mM [Fe(CN)6]3– solutions. Figures 6A–C demonstrate that cell growth increased linearly, and required the same reaction times as the respiration process. The OD600 increase during respiration revealed that a 0.15 mM electron flux generated 4.5 ± 0.5% cell increase from 0.81 pM G. sulfurreducens solutions. Hence, a flow of 0.15 mM electrons produced 0.036 pM cell growth, so that 4⋅109 electrons were needed to synthesize enough ATP for one cell division, which agrees with the analysis of electrochemical experiments (Levar et al., 2013).
Figure 6. Increase of cell concentration and decrease of oxidants [Fe(CN)6]3– and CrO42– during G. sulfurreducens respiration in grow medium A, lacking fumarate, and stating from the lag phase. (A) Linear increase of the bacterial growth with 0.15 mM [Fe(CN)6]3–, R2 = 0.98. (B) Linear increase of the bacterial growth with 0.05 and 0.03 mM CrO42– (insert), R2 = 0.98. (C) Linear CrO42– decrease (blue, R2 = 0.99), and linear bacterial growth (brown, R2 = 0.98) of the same respiration experiment.
Respiration of lag phase G. sulfurreducens cells in a growth medium, which lacks the oxidant fumarate, reduced Fe3+, Co3+, V5+, Cr6+, and Mn7+ ions of the 6 different metal salts with electron flux rates of 3.7⋅105 e–⋅s–1 per cell at 30°C, a pH of 7.4 and a reproducibility of ± 30% (Figures 3A,D). The nearly constant Fe2+/heme levels demonstrated that the oxidation of Fe2+/hemes by the metal salts, and the reduction of Fe3+/hemes by the menaquinole pool occurred with the same rates, leading to a constant electron flux. Rates and kinetic orders agree well with our earlier reduction experiments of Ag+ ions by G. sulfurreducens (Chabert et al., 2020), which led to Ag nanoparticles (AgNPs), as reaction products (Figure 1). We had measured high binding constants of Ag+ ions to Met and His of outer membrane cytochromes, and observed the formation of AgNPs at the outer cell membrane. In recent cell growth experiments with CrO42– (Gong et al., 2018), Cr3+ reduction products could also be detected within the cells, if the bacteria were treated for several hours with metal salts. Our experiments were finished within 10–20 min, and we never observed Cr3+ within the cells (Figure 2B), but to avoid overinterpretation, we don’t exclude the possibility that some of the CrO42– was reduced inside of the cells even under our different reaction conditions. This obviously did not change the electron flux rate (Figure 3A), which is driven by the need for a constant ATP production (Velten et al., 2007; Bochdansky et al., 2021; Wilson and Matschinsky, 2021) during G. sulfurreducens respiration (Figures 6A–C). Interestingly, the same electron flux rates were also measured in electrochemical experiments on single cells of G. sulfurreducens (Jiang et al., 2013) as well as Shewanella oneidensis (Gross and El-Naggar, 2015).
The influence of Fe2+/hemes on electron flux rates was studied (a) with G. sulfurreducens cells of downregulated c-cytochromes, (b) with cells in the exponential growth phase, and (c) with G. sulfurreducens mutants. Decrease of c-cytochrome concentrations (Figure 5A and Table 1) was observed in microorganisms that were prepared under fumarate-respiring conditions at very low FeSO4 concentrations (medium B). Addition of CrO42– ions to these downregulated cells induced nearly the same reaction rates as experiments with G. sulfurreducens, grown in medium A (Figure 5B). This was surprising, as c-cytochromes are the electron transporting carriers and their decrease should slow down the electron flux. Obviously, the observed rise of Fe2+/heme levels from ≤ 5 to 30% compensated the downregulation of c-cytochromes. Electron flux rates of metal salt induced respiration, which used G. sulfurreducens cells in the exponential growth phase, were 4–6 times faster compared to experiments starting from G. sulfurreducens in the resting phase (Figures 4C,D). Such an acceleration is reasonable as the ATP demand increases in the exponential growth phase, requiring at the same time a faster electron flux, which was achieved by an increase of Fe2+:/heme levels in the cytochromes from 5% via 40 to 75% (Figure 4B). Such a rise of the Fe2+/heme level augments the reductive power of multiheme cytochromes (Quian et al., 2011; Liu and Bond, 2012; Santos et al., 2015), and increases electron transfer rates by the Marcus theory (Marcus, 1993). It demonstrates the importance of the electron storing capacities of multiheme cytochromes (Esteve-Núñez et al., 2008), which can regulate respiration by their redox states. Our observations are again in accord with electrochemical experiments, where the redox status of c-cytochromes in G. sulfurreducens biofilms changed with the applied potential (Liu et al., 2011). Thus, the Fe2+/heme level is an important parameter for the optimization of G. sulfurreducens as an electron-producing source.
The analogous effects of our metal salt induced electron flux measurements with electrochemical current production of biofilms at the anode inititated us to measure the reduction rate of CrO42– by G. sulfurreducens mutants, in which OmcBEST was deleted. Electrochemical measurements on biofilms had shown (Nevin et al., 2009) that “deletion of OmcS, OmcB and OmcE had nearly no impact on maximum current production.” This is in strong contrast to Fe3+ oxide and Fe3+ citrate induced cell growth experiments. G. sulfurreducens mutants, in which OmcB, OmcE, or OmcS were mutated out (Leang et al., 2003; Richter et al., 2011) reduced the cell growth rates dramatically. Our rate measurements with water-soluble CrO42– ions are again in accord with electrochemical results. Figure 5C demonstrates that the mutant, in which OmcBEST was deleted, hardly changed the CrO42– reduction time, but the missing outer membrane cytochromes induced a drastic increase of the Fe2+/hemes level during the metal salt reduction. It demonstrates that the effect of decreasing outer membrane cytochromes on the respiration rate was compensated by an increase of the Fe2+/heme level. Thus, Fe2+/hemes play a central role in the regulation of electron flux rates. These measurements stimulate studies to elucidate the effects of G. sulfurreducens mutations on electron flux rates in bioelectrochemical measurements compared to cell growth experiments. An obvious difference between these two techniques is that in cell growth experiments the cells are several hours in contact to the oxidizing minerals and the reduced metal ions. In contrast, electrochemical experiments with G. sulfurreducens biofilms on electrodes, as well as the metal salt induced electron flux measurements, presented in this publication, take only some minutes. We will check in future work, whether the sensitivity of mutated cells against strong oxidants is one of the reasons for the differences between long time cell growth and short time bioelectrochemical experiments.
The datasets presented in this study can be found in online repositories. The names of the repository/repositories and accession number(s) can be found below: ProteomeXchange, PXD032892.
MK designed and carried out the kinetic experiments. MK and BG analyzed the data and calculated reaction kinetics. MS carried out the proteomic experiments. MS, JD, and CS discussed the proteomic experiments. MK, BG, and KF discussed all data and suggested the reaction mechanism. All authors contributed to the article and approved the submitted version.
We thank the Swiss National Science Foundation for support via the grant 178827, as well as the University of Fribourg and the NCCR Bioinspired Materials.
The authors declare that the research was conducted in the absence of any commercial or financial relationships that could be construed as a potential conflict of interest.
All claims expressed in this article are solely those of the authors and do not necessarily represent those of their affiliated organizations, or those of the publisher, the editors and the reviewers. Any product that may be evaluated in this article, or claim that may be made by its manufacturer, is not guaranteed or endorsed by the publisher.
We thank D. R. Lovley and T. L. Woodward for the G. sulfurreducens mutant lacking OmcBEST.
The Supplementary Material for this article can be found online at: https://www.frontiersin.org/articles/10.3389/fmicb.2022.909109/full#supplementary-material
Aklujkar, M., Coppi, M. V., Leang, C., Kim, B. C., Chavan, M. A., Perpetua, L. A., et al. (2013). Proteins involved in electron transfer to Fe(III) and Mn(IV) oxides by Geobacter sulfurreducens and Geobacter uraniireducens. Microbiology 159, 515–535. doi: 10.1099/mic.0.064089-0
Bochdansky, A. B., Stouffer, A. N., and Washington, N. N. (2021). Adenosine triphosphate (ATP) as a metric of microbial biomass in aquatic systems: new simplified protocols, laboratory validation, and a reflection on data from the literature. Limnol. Oceanogr. Methods 19, 115–131. doi: 10.1002/lom3.10409
Bond, D. R., and Lovley, D. R. (2003). Electricity production by geobacter sulfurreducens attached to electrodes. Appl. Environ. Microbiol. 69, 1548–1555. doi: 10.1128/AEM.69.3.1548-1555.2003
Brown, G. C. (1992). Control of respiration and ATP synthesis in mammalian mitochondria and cells. Biochem. J. 284, 1–13. doi: 10.1042/bj2840001
Bruderer, R., Bernhardt, O. M., Gandhi, T., Miladinović, S. M., and Cheng, L. Y. (2015). Extending the limits of quantitative proteome profiling with data-independent acquisition and application to acetaminophen-treated three-dimensional liver microtissues. Mol. Cell. Proteomics 14, 1400–1410. doi: 10.1074/mcp.M114.044305
Butler, J. E., Glaven, R. H., Esteve-Nuìnϸez, A., Nuìnϸez, C., Shelobolina, E. S., Bond, D. R., et al. (2006). Genetic characterization of a single bifunctional enzyme for fumarate reduction and succinate oxidation in Geobacter sulfurreducens and engineering of fumarate reduction in Geobacter metallireducens. J. Bacteriol. 188, 450–455. doi: 10.1128/JB.188.2.450-455.2006
Butler, J. E., Young, N. D., and Lovley, D. R. (2010). Evolution of electron transfer out of the cell: comparative genomics of six Geobacter genomes. BMC Genom. 11:40. doi: 10.1186/1471-2164-11-40
Caccavo, F., Lonergan, D. J., Lovley, D. R., Davis, M., Stoiz, J. F., and McInerney, M. J. (1994). Geobacter sulfurreducens sp. nov., a hydrogen- and acetate-oxidizing dissimilatory metal-reducing microorganism. Appl. Environ. Microbiol. 60, 3752–3759. doi: 10.1128/aem.60.10.3752-3759.1994
Chabert, V., Babel, L., Füeg, M. P., Karamash, M., Madivoli, E. S., Herault, N., et al. (2020). Kinetics and mechanism of mineral respiration: how iron hemes synchronize electron transfer rates. Angew. Chem. Int. Ed. 59, 12331–12336. doi: 10.1002/anie.201914873
Coppi, M. V., Leang, C., Sandler, S. J., and Lovley, D. R. (2001). Development of a genetic system for Geobacter sulfurreducens. Appl. Environ. Microbiol. 67, 3180–3187. doi: 10.1128/AEM.67.7.3180-3187.2001
Ding, Y.-H. R., Hixson, K. K., Aklujkar, M. A., Lipton, M. S., Smith, R. D., Lovley, D. R., et al. (2008). Proteome of Geobacter sulfurreducens grown with Fe(III) oxide or Fe(III) citrate as the electron acceptor. Biochim. Biophys. Acta 1784, 1935–1941. doi: 10.1016/j.bbapap.2008.06.011
Egan-Morriss, C., Kimber, R. L., Powell, N. A., and Lloyd, J. R. (2022). Biotechnological syntheses of Pd-based nanoparticle catalysis. Nanoscale Adv. 4, 654–679. doi: 10.1039/D1NA00686J
Embree, M., Qiu, Y., Shieu, W., Nagarajan, H., O’Neil, R., Lovley, D., et al. (2014). The iron stimulon and fur regulon of Geobacter sulfurreducens and their role in energy metabolism. Appl. Environ. Microbiol. 80, 2918–2927. doi: 10.1128/AEM.03916-13
Engel, C. E. A., Vorländer, D., Biedendieck, R., Krull, R., and Dohnt, K. (2020). Quantification of microaerobic growth of Geobacter sulfurreducens. PLoS One 15:e0215341. doi: 10.1371/journal.pone.0215341
Esteve-Núñez, A., Sosnik, J., Visconti, P., and Lovley, D. R. (2008). Fluorescent properties of c-type cytochromes reveal their potential role as an extracytoplasmic electron sink in Geobacter sulfurreducens. Environ. Microbiol. 10, 497–505. doi: 10.1111/j.1462-2920.2007.01470.x
Estevez-Canales, M., Kuzume, A., Borjas, Z., Füeg, M., Lovley, D., Wandlowski, T., et al. (2015). A severe reduction in the cytochrome c content of Geobacter sulfurreducens eliminates its capacity for extracellular electron transfer. Environ. Microbiol. Rep. 7, 219–226. doi: 10.1111/1758-2229.12230
Gong, Y., Werth, C. J., He, Y., Su, Y., Zhang, Y., and Zhou, X. (2018). Intracellular versus extracellular accumulation of hexavalent chromium reduction products by Geobacter sulfurreducens PCA. Environ. Pollut. 240, 485–492. doi: 10.1016/j.envpol.2018.04.046
Gross, B. J., and El-Naggar, M. Y. (2015). A combined electrochemical and optical trapping platform for measuring single cell respiration rates at electrode interfaces. Rev. Sci. Instrum. 86:064301.
Jiang, X., Hu, J., Petersen, E. R., Fritzgerald, L. A., Jackan, C. S., Lieber, A. M., et al. (2013). Probing single- to multi-cell level charge transport in Geobacter sulfurreducens DL-1. Nat. Commun. 4:2751. doi: 10.1038/ncomms3751
Kato, S., Hashimoto, K., and Watanabe, K. (2013). Iron-oxide minerals affect extracellular electron-transfer paths of geobacter spp. Microbes Environ. 28, 141–148. doi: 10.1264/jsme2.ME12161
Khan, M. R., Fromm, K. M., Rizvi, T. F., Giese, B., Ahamad, F., Turner, R. J., et al. (2020). Metal nanoparticle–microbe interactions: synthesis and antimicrobial effects. Part. Syst. Charact. 37:1900419. doi: 10.1002/ppsc.201900419
Komarova, T. V., Obrezkov, O. N., and Shpigun, O. A. (1991). Ion chromatographic behaviour of anionic EDTA complexes of vanadium(IV) and vanadium(V). Anal. Chim. Acta 254:63. doi: 10.1016/0003-2670(91)90009-T
Leang, C., Coppi, M. V., and Lovley, D. R. (2003). OmcB, a c-type polyheme cytochrome, involved in fe(III) reduction in Geobacter sulfurreducens. J. Bacteriol. 185, 2096–2103. doi: 10.1128/JB.185.7.2096-2103.2003
Levar, C. E., Chan, C. H., Mehta-Kolte, M. G., and Bond, D. R. (2014). An inner membrane cytochrome required only for reduction of high redox potential extracellular electron acceptors. mBio 5:e2034. doi: 10.1128/mBio.02034-14
Levar, C. E., Hoffman, C. L., Dunshee, A. J., Toner, B. M., and Bond, D. R. (2017). Redox potential as a master variable controlling pathways of metal reduction by Geobacter sulfurreducens. ISME J. 11, 741–752. doi: 10.1038/ismej.2016.146
Levar, C. E., Rollefson, J. B., and Bond, D. R. (2013). “Energetic and molecular constraints on the mechanism of environmental fe(III) reduction by geobacter,” in Microbial Metal Respiration, eds J. Gescher and A. Kappler (Berlin, Heidelberg: Springer), 29–48. doi: 10.1007/978-3-642-32867-1_2
Liu, Y., and Bond, D. R. (2012). Long-distance electron transfer by g. sulfurreducens biofilms results in accumulation of reduced c-type cytochromes. ChemSusChem 5, 1047–1053. doi: 10.1002/cssc.201100734
Liu, Y., Kim, H., Franklin, R. R., and Bond, D. R. (2011). Linking spectral and electrochemical analysis to monitor c-type cytochrome redox states in living Geobacter sulfurreducens biofilms. ChemPhysChem 12, 2235–2241. doi: 10.1002/cphc.201100246
Lloyd, J. R., Byrne, J. M., and Coker, V. S. (2011). Biotechnological synthesis of functional nanomaterials. Curr. Opin. Biotechnol. 22, 509–515. doi: 10.1016/j.copbio.2011.06.008
Lovley, D. R., and Phillips, E. J. (1988). Novel mode of microbial energy metabolism: organic carbon oxidation coupled to dissimilatory reduction of iron or manganese. Appl. Environ. Microbiol. 54:1480. doi: 10.1128/aem.54.6.1472-1480.1988
Lovley, D. R., Stolz, J. F., Nord, G. L., and Phillips, E. J. P. (1987). Anaerobic production of magnetite by a dissimilatory iron-reducing microorganism. Nature 330, 252–254. doi: 10.1038/330252a0
Lovley, D. R., Ueki, T., Zhang, T., Malvankar, N. S., Shrestha, P. M., Flanagan, K. A., et al. (2011). Geobacter: the microbe electric’s physiology, ecology, and practical applications. Adv. Microb. Physiol. 59, 1–100. doi: 10.1016/B978-0-12-387661-4.00004-5
Marcus, R. A. (1993). Electron transfer reactions in chemistry: theory and experiment. Angew. Chem. Int. Ed. 32, 1111–1222. doi: 10.1002/anie.199311113
Muhamadali, H., Xu, Y., Ellis, D. I., Allwood, J. W., Rattray, N. J. W., Correa, E., et al. (2015). Metabolic profiling of Geobacter sulfurreducens during industrial bioprocess scale-up. Appl. Environ. Microbiol. 81, 3288–3298. doi: 10.1128/AEM.00294-15
Nevin, K. P., Kim, B.-C., Glaven, R. H., Johnson, J. P., Woodward, T. L., Methé, B. A., et al. (2009). Anode biofilm transcriptomics reveals outer surface components essential for high density current production in Geobacter sulfurreducens fuel cells. PLoS One 4:e5628. doi: 10.1371/journal.phone.0005628
Otero, F. J., Chan, C. H., and Bond, D. R. (2018). Identification of different putative outer membrane electron conduits necessary for Fe(III) citrate, Fe(III) oxide, Mn(IV) oxide, or electrode reduction by Geobacter sulfurreducens. J. Bacteriol. 200:e347. doi: 10.1128/JB.00347-18
Pushkar, B., Sevak, P., Parab, J., and Nikanth, N. (2021). Chromium pollution and its bioremediation mechanisms in bacteria: a review. Environ. Polution 240, 483–492. doi: 10.1016/j.envpol.2018.04.046
Quian, X., Mester, T., Morgado, L., Arakawa, T., Sharma, M. L., Inoue, K., et al. (2011). Biochemical characterization of purified OmcS, a c-type cytochrome required for insoluble Fe(III) reduction in Geobacter sulfurreducens. Biochim. Biophys. Acta 1807, 404–412. doi: 10.1016/j.bbabio.2011.01.003
Richter, K., Schickberger, M., and Gescher, J. (2011). Dissimilatory Reduction of Extracellular Electron Acceptors in Anaerobic Respiration. Appl. Environ. Microbiol. 78, 913–921. doi: 10.1128/AEM.06803-11
Santos, T. C., Silva, M. A., Morgado, L., Dantas, J. M., and Salgueiro, C. A. (2015). Diving into the redox properties of Geobacter sulfurreducens cytochromes: a model for extracellular electron transfer. Dalton Trans. 44, 9335–9344. doi: 10.1039/C5DT00556F
Shelobolina, E. S., Coppi, M. V., Korenevsky, A. A., DiDonato, L. N., Sullivan, S. A., Konishi, H., et al. (2007). Importance of c-type cytochromes for U(VI) reduction by Geobacter sulfurreducens. BMC Microbiol. 7:16. doi: 10.1186/1471-2180-7-16
Shi, L., Dong, H., Reguera, G., Beyenal, H., Lu, A., Liu, J., et al. (2016). Extracellular electron transfer mechanisms between microorganisms and minerals. Nat. Rev. Microbiol. 14, 651–662. doi: 10.1038/nrmicro.2016.93
Slate, A. J., Whitehead, K. A., Brownson, D. A. C., and Banks, C. E. (2019). Microbial fuel cells: an overview of current technology. Renew. Sustain. Energy Rev. 101, 60–81. doi: 10.1016/j.rser.2018.09.044
Stekovic, S., Hofer, S. J., Tripolt, N., Aon, M. A., Royer, P., Pein, L., et al. (2020). Alternate day fasting improves physiological and molecular markers of aging in healthy, non-obese humans. Cell Metab 30, 462–476.e6. doi: 10.1016/j.cmet.2019.07.016
Ueki, T. (2021). Cytochromes in extracellular electron transfer in geobacter. Appl. Environ. Microbiol. 87, e3109–e3120. doi: 10.1128/AEM.03109-20
Vasylevskyi, S. I., Kracht, S., Corcosa, P., Fromm, K. M., Giese, B., and Füeg, M. (2017). Formation of silver nanoparticles by electron transfer in peptides and c-cytochromes. Angew. Chem. Int. Ed. 56, 5926–5930. doi: 10.1002/anie.201702621
Velten, S., Hammes, F., Boller, M., and Egli, T. (2007). Rapid and direct estimation of active biomass on granular activated carbon through adenosine tri-phosphate (ATP) determination. Water Res. 41, 1973–1983. doi: 10.1016/j.watres.2007.01.021
Keywords: reaction kinetic, c-cytochrome, Geobacter sulfurreducens, remediation, bioelectrochemistry
Citation: Karamash M, Stumpe M, Dengjel J, Salgueiro CA, Giese B and Fromm KM (2022) Reduction Kinetic of Water Soluble Metal Salts by Geobacter sulfurreducens: Fe2+/Hemes Stabilize and Regulate Electron Flux Rates. Front. Microbiol. 13:909109. doi: 10.3389/fmicb.2022.909109
Received: 31 March 2022; Accepted: 20 May 2022;
Published: 17 June 2022.
Edited by:
Amelia-Elena Rotaru, University of Southern Denmark, DenmarkReviewed by:
Yonggang Yang, Guangdong Academy of Science, ChinaCopyright © 2022 Karamash, Stumpe, Dengjel, Salgueiro, Giese and Fromm. This is an open-access article distributed under the terms of the Creative Commons Attribution License (CC BY). The use, distribution or reproduction in other forums is permitted, provided the original author(s) and the copyright owner(s) are credited and that the original publication in this journal is cited, in accordance with accepted academic practice. No use, distribution or reproduction is permitted which does not comply with these terms.
*Correspondence: Bernd Giese, YmVybmQuZ2llc2VAdW5pZnIuY2g=; Katharina M. Fromm, a2F0aGFyaW5hLmZyb21tQHVuaWZyLmNo
Disclaimer: All claims expressed in this article are solely those of the authors and do not necessarily represent those of their affiliated organizations, or those of the publisher, the editors and the reviewers. Any product that may be evaluated in this article or claim that may be made by its manufacturer is not guaranteed or endorsed by the publisher.
Research integrity at Frontiers
Learn more about the work of our research integrity team to safeguard the quality of each article we publish.