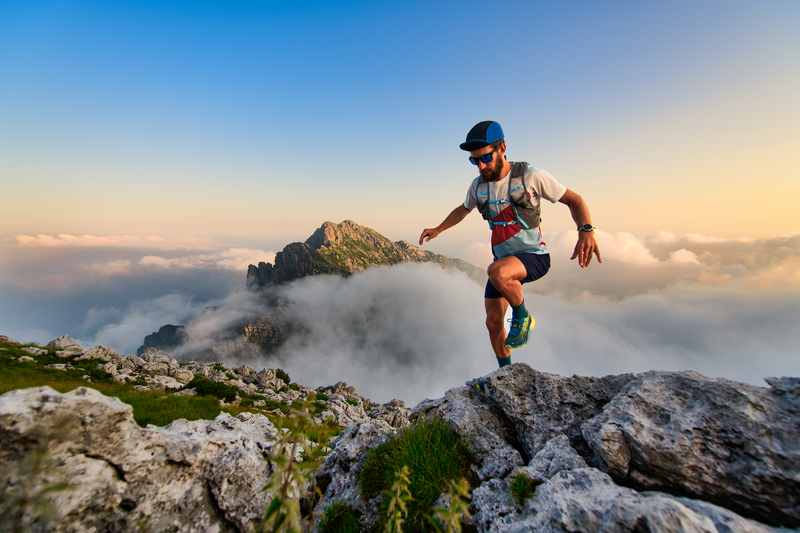
94% of researchers rate our articles as excellent or good
Learn more about the work of our research integrity team to safeguard the quality of each article we publish.
Find out more
REVIEW article
Front. Microbiol. , 31 May 2022
Sec. Terrestrial Microbiology
Volume 13 - 2022 | https://doi.org/10.3389/fmicb.2022.900740
This article is part of the Research Topic Mineral Solubilizing Microorganisms (MSM) and their Applications in Nutrient Availability, Weathering and Bioremediation View all 14 articles
Zinc (Zn) is one of the most abundantly found heavy metals in the Earth’s crust and is reported to be an essential trace metal required for the growth of living beings, with it being a cofactor of major proteins, and mediating the regulation of several immunomodulatory functions. However, its essentiality also runs parallel to its toxicity, which is induced through various anthropogenic sources, constant exposure to polluted sites, and other natural phenomena. The bioavailability of Zn is attributable to various vegetables, beef, and dairy products, which are a good source of Zn for safe consumption by humans. However, conditions of Zn toxicity can also occur through the overdosage of Zn supplements, which is increasing at an alarming rate attributing to lack of awareness. Though Zn toxicity in humans is a treatable and non-life-threatening condition, several symptoms cause distress to human activities and lifestyle, including fever, breathing difficulty, nausea, chest pain, and cough. In the environment, Zn is generally found in soil and water bodies, where it is introduced through the action of weathering, and release of industrial effluents, respectively. Excessive levels of Zn in these sources can alter soil and aquatic microbial diversity, and can thus affect the bioavailability and absorption of other metals as well. Several Gram-positive and -negative species, such as Bacillus sp., Staphylococcus sp., Streptococcus sp., and Escherichia coli, Pseudomonas sp., Klebsiella sp., and Enterobacter sp., respectively, have been reported to be promising agents of Zn bioremediation. This review intends to present an overview of Zn and its properties, uses, bioavailability, toxicity, as well as the major mechanisms involved in its bioremediation from polluted soil and wastewaters.
The surge in anthropogenic activities has exacerbated the problem of heavy metal pollution in the world, which, due to their indefinite persistence in the environment, are non-degradable pollutants and can be detrimental for living beings at high levels (Witkowska et al., 2021). Zinc (Zn) is one of the most profusely abundant transition elements in the Earth’s crust and is also reported to be an essential trace element for all living organisms (Roohani et al., 2013; Jarosz et al., 2017; Lee, 2018). It is a significant component of various proteins, acting as a cofactor or coenzyme of more than 300 enzymes (Rahman and Karim, 2018), and is attributable to the activity and regulation of various enzymes, proteins, DNA and DNA binding proteins, immunity, as well as cell metabolism. It also aids in suppressing the generation of free radicals and reactive oxygen species (ROS), which accentuates protein stability and the function of antioxidative enzymes (Narayanan et al., 2020). In nature, it exists as a divalent cation (Zn2+), thus interacting with various negatively charged ions (CO3−, OH−, C2O42−). Zn was first reported to be essential for the growth of microorganisms in 1869 (Raulin, 1869), much earlier than plants and animals (Todd et al., 1933; Prasad et al., 1963). It was not until the 1960s that Zn was established to be essential for humans as well, prior to which it was not known that its deficiency would cause detrimental effects to human health (Prasad, 2017). In humans, Zn serves the elemental role in the production of hormones and their receptors (Chasapis et al., 2012; Roohani et al., 2013). It is reported that the cumulative concentration of Zn in the human body amounts to approximately 2–3 gm, more than 80% of which is apportioned in the bone and muscles. During transportation within the body, enzymes like superoxide dismutase and carbonic anhydrase in the red blood cells carry the largest quantity of Zn in the bloodstream (Vallee and Falchuk, 1993). However, majority of transported Zn is bound to albumin in plasma, with approximate concentration of 12–16 μm (Rink and Gabriel, 2000). Furthermore, the regulation of Zn in the human body is closely associated with normal levels of cellular proliferation, apoptosis and differentiation (Haase and Rink, 2014a), and the production of cytokines and antibodies, thus serving a significant role in the homeostasis of innate and adaptive immune systems (Haase and Rink, 2014b; Weyh et al., 2022). The supplementation of Zn has also been recommended for the reduction of viral activity, as well as the amelioration of tissue damage and inflammation in many viral infections, including COVID-19 (Asl et al., 2021; Rodelo et al., 2022). The consumption of Zn in humans is typically dependent upon dietary intake, which is varied according to age and gender. According to the German Nutrition Society, the consumption of 1.5 mg/day for infants (0–4 months), 11 and 14 mg/day for adolescent males and females (15–19 years) has been advised, while for adult males and females (ages 19 or older), the average allowance of intake is recommended to be 11–16 and 7–10 mg/day (variable by phytate intake), respectively, by The Food and Nutrition Board, United States (Otten et al., 2006; Rodelo et al., 2022). Thus, deficiency of Zn can be an important factor in creating nutritional imbalance and can adversely impact human health, where it can be a precursor in the development of certain medical ailments, including immune dysregulation, impaired immunity, increased vulnerability to infection, lymphopenia, common cold, epilepsy, acne, Wilson’s disease, and neurological diseases, such as schizophrenia, Parkinson’s, and Alzheimer’s disease (Irving et al., 2003; Walker and Black, 2004; Prasad, 2013). Zn intake and its excess is also reported to be associated with the modulation of gastrointestinal flora and immune systems (Skalny et al., 2021). In this light, it is also important to note that excessive intake of this element can have negative effects, such as disturbances in the levels of other elements, particularly copper, which can subsequently result in copper deficiency anemia, reduction in the copper-dependent enzymes, and cholesterol metabolism dysregulation (Terrin et al., 2020; Brzóska et al., 2021).
In literature, there are various reports which demonstrate the adverse effects caused by excessive micronutrient exposure, including exposure to Zn, causing disturbance to the homeostasis of various biological systems (Hynninen et al., 2010; Potocki et al., 2012). At higher concentrations, Zn can cause toxicity in cells, which can often result in the disruption of essential biological functions triggered by blocking protein thiols through mismetallation with other metals (Marchetti, 2013; Chandrangsu et al., 2017). In the environment, emissions of vast quantities of Zn due to natural and anthropogenic sources has enabled its unmitigated entry into food chains, resulting in biomagnification in living organisms. Bioremediation is feasible and efficient method for the removal of these toxic quantities from contaminated soil and wastewater sources, thus offering a new perspective on the utilization of heavy metal-resistant bacteria through sustainable development (Sharma et al., 2021).
This review aims to compile and present the chemical and physical properties of Zn, its uses, the effects of its toxicity, as well as summarize and highlight the potential mechanism of resistance in bacteria which enable them as promising agents of bioremediation of heavy metals, such as Zn.
Zinc is represented by the symbol “Zn,” with an atomic number and weight of 30 and 65.38 gm, respectively. It was discovered and recognized as a metal for the first time in 1746 by German chemist Andreas Sigismund Marggraf (National Center for Biotechnology Information, 2022). It belongs to group XII (known formerly as II-B) of the periodic table (Period number 4, element group number 12, block “d”; Jensen, 2003), and is a brittle, lustrous, bluish-white metal which is solid at room temperature. When heated at temperatures above 110°C, it readily becomes malleable and ductile and is generally considered to be a moderately reactive metal in terms of its reactivity with oxygen and other metals (Table 1; Wuana and Okieimen, 2011). It acts as a strong reducing agent, and in hydrolytic reactions, acts as a Lewis acid in catalyzing reactions, thereby being associated with various metalloenzymes, DNA binding, and regulatory proteins, as well as transcription factors (Cerasi et al., 2013). Zn naturally occurs as a mineral sphalerite (ZnS) and has five stable isotopes (64Zn, 66Zn, 67Zn, 68Zn, and 70Zn) in nature, from which 64Zn is the major isotope in terms of natural abundance (Audi et al., 2017).
Zn does not generally act as a typical metal, as it does not have an 8-electron octet rather an 18-electron shell upon the loss of its outermost two electrons, rendering it less reactive than other metals. However, since it undergoes neither oxidation nor reduction, it forms a stable metal ion in biological matrices where the redox potential is in constant flux (Graf, 1997). It has an oxidation state of 2+, and readily forms compounds with ammonia, cyanide, and halide ions. When compared to the metal itself, Zn dust is reported to be more reactive and pyro-phoric due to its large surface area. Selective compounds of Zn (chloride and sulfate) are water soluble, whereas other compounds (sulfide, carbonate, phosphate, oxide) are observed to be insoluble or slightly soluble in water (Habashi, 2013). It is capable of displacement of all metals beneath its position in the electrochemical series, and can also displace gold from cyanide solution, the latter of which is a large-scale industrial process (Robinson and Pohl, 2013). The surface of the metal is prone to rapid corrosion, ultimately forming an encapsulant layer of Zn carbonate after reacting with atmospheric carbon dioxide (Holleman et al., 1985), though it can be removed by the corroding action of strong acids (HCl, H2SO4; Porter, 1994).
Sufficient consumption and uptake of Zn is vital for the regulation and optimal function of innate and adaptive immunity in human beings (Bonaventura et al., 2015). In innate immunity, Zn serves an elemental role in maintaining the activity of NADPH oxidase found in neutrophil granulocytes (Hasegawa et al., 2000; DeCoursey et al., 2003). Thus, the lack of Zn uptake and its subsequent deficiency could beget decreased production and killing potential of ROS (Bonaventura et al., 2015). Furthermore, Zn deficiency mediates the induction of decreased adhesion and chemotactic behavior in neutrophil and monocyte granulocytes and impairs the function and maturation phase of macrophages (Shankar and Prasad, 1998). Pertaining to its role in natural killer cells (NK cells), a deficit of Zn in the body could reduce overall NK cell count in blood, where the decreased chemotactic and lytic behavior of infected or cancer cells has also been observed (Rajagopalan et al., 1995; Rajagopalan and Long, 1998).
In the case of adaptive immunity, the presence of Zn is fundamental in the generation, maturation, and activity of T cells (Fraker and King, 2004). This is due to its significance in the elemental composition of thymulin, a hormone produced in the thymus which promotes the development of pre-T lymphocytes into mature lymphocytes (Dardenne and Pleau, 1994; Saha et al., 1995), which can be inhibited in the case of Zn deficiency, resulting in thymus atrophy and decreased T-cell count (King et al., 2005). Moreover, Zn deficiency can negatively affect T cell and cytokines (such as IL-2 and IFN-γ) production (Bădulici et al., 1994; Prasad et al., 1997). Apart from T-cell maturation, Zn also aids in the cell differentiation process, where it was observed that a deficiency of Zn demonstrated a decrease in CD4+ T cells, which ultimately results in the disruption of CD4+/CD8+ cells ratio, which is a characteristic sign of immune dysfunction (Beck et al., 1997; Sheikh et al., 2010).
Apart from its effect on the functions of selective immune cells, the uptake of Zn is affiliated with the general regulation of the entire immune system, where various studies have demonstrated that elevated levels of oxidative stress and inflammation are all associated with a deficiency of Zn in the body (Wong et al., 2015, 2019; Gammoh and Rink, 2017). In in vivo models, the supplementation of Zn was observed to induce the production, maturation, and stability of function in regulatory T cells (Rosenkranz et al., 2016, 2017). Moreover, lack of Zn in the body can aggravate cases of chronic inflammation, which can elevate the expression of pro-inflammatory markers, such as IL-1α, IL-1β, and IL-6 in several inflammatory disease (Yazar et al., 2005). This aspect of Zn significance as a micronutrient is therefore essential for the positive downregulation of disease pathogenesis and molecular pathways, such as NF-κB signaling pathway, which significantly regulates apoptosis, immune and inflammatory responses, as well as the expression of pro-inflammatory cytokines (Jarosz et al., 2017). In addition, Zn also importantly affects the expression of Zn finger proteins which are known to suppress the expression of TNFR- and TLR-initiated NF-κB signaling pathways (Gammoh and Rink, 2017).
Over the years, studies have reported the importance of Zn in the alleviation of viral infections, which is mainly associated with the entry of virus particles into the host cells, and their subsequent fusion and replication, as well as translation and emission of virus proteins in the host (Ishida, 2019; Read et al., 2019). In a study, it was reported that supplementing Zn at the recommended dose (>75 mg/day) remarkably aided in the reduction of severity of colds (Maares and Haase, 2020). In elder adults, the supplementation of Zn (45 mg/day of Zn gluconate) was observed to reduce the number of infections, as well as strengthen their immune system via the marked increase in the concentration of Zn in the plasma, along with the lowered production of oxidative stress markers and TNF-α (Prasad et al., 2007). More recent research findings demonstrate the ability of Zn to aid in the inhibition of RNA polymerase of SARS virus, via the reduction of viral replication (Te Velthuis et al., 2010), which are suggestive of Zn to be potentially effective against SARS-CoV-2, and COVID-19 (Skalny et al., 2020; Weyh et al., 2022).
Plants tend to uptake Zn from soil because of which its deficiency is reported to be a significant abiotic stress factor affecting more than 40% of agricultural lands worldwide. Several plant species are reported to be Zn-efficient (rich in Zn), such as carrot, rye, cashew nuts, wheat, sunflower, pea, oats, and alfalfa (Hambidge et al., 2010). Other good sources of Zn include beef, and dairy products, such as milk, eggs, and cheese (Hacisalihoglu, 2020). Though it is well established that the presence of high phytate ions in the diet can ultimately hinder the absorption of several essential minerals like Ca, Fe, and Zn (Castro-Alba et al., 2019), improved absorption of Zn was observed when dairy products were consumed in combination with other foods with high phytate (e.g., bread, tortillas, and rice), which seemed to be associated with the presence and beneficial effects of citrate and phosphopeptides in dairy products (Shkembi and Huppertz, 2021). Zn absorption occurs within the small intestine in its ionized form which is formed when Zn is released from consumed foods. However, this ionized form may then be able to form inhibiting complexes with ligands of organic acids, such as phytates, amino acids, and phosphates, inadvertently affecting its solubility and absorption (Krebs, 2000). The presence of citrate, a low molecular weight ligand found in human milk which binds with Zn, and casein phosphopeptides, which are phosphorylated peptides released upon the digestion of casein which can also bind to Zn, in dairy products has thus been hypothesized to improve Zn absorption by preventing it from binding and forming complexes with phytate ions (Miquel and Farré, 2007). However, there have been some reports which state otherwise (Pécoud et al., 1975; Sandström and Cederblad, 1980; Flanagan et al., 1985; Wood and Zheng, 1990). The availability of Zn from mixed- or vegetable-based diets is reported to be more than 20% (Hacisalihoglu and Blair, 2020). The use of Zn supplements is widespread, though not necessarily mandatory for fulfilling dietary Zn requirements. In a normal and healthy host, the choice of Zn salt used is insignificant (Tran et al., 2004), though the amount of Zn absorbed from supplements is more greater than absorption from diet. Furthermore, despite the beneficial effect of Zn consumption on the immune system, excessive intake can result in its toxicity (Maares and Haase, 2020).
Heavy metals are known to persist in the human body as well as the environment, with various environmental sources being attributable for their wide availability (Haiyan and Stuanes, 2003; Nadal et al., 2005). They can usually be exposed to humans through inhalation, ingestion, and dermal contact (of contaminated soil and water), as well as consumption of contaminated produce (Hough et al., 2004; Baastrup et al., 2008; Mari et al., 2009; Man et al., 2020). In order to ensure public safety and health, it is essential to identify and mitigate these sources of exposure (Hu and Dong, 2011). Zn is one of the most readily available heavy metals found in soil, where its excessive levels may exert a phytotoxic effect which can drastically affect crop quality and yield, respectively, and even pose a health risk to humans upon consumption due to the accumulation of Zn through absorption or deposition (Wang et al., 2005; Baran, 2012, 2013; Bolan et al., 2014). Furthermore, excess levels of Zn in soil also contribute to the inhibition and alteration of soil microorganisms (Olaniran et al., 2013), due to its bioavailability in the form of ionic Zn and as part of organo-metallic complexes, which have been previously determined (Pueyo et al., 2004; Anju and Banerjee, 2011; Fedotov et al., 2012; Agnieszka et al., 2014; Kim et al., 2015; Baran et al., 2018). Therefore, efficient methods of heavy metal remediation are crucial for the alleviation of Zn toxicity in order to preserve soil microorganisms and their biodiversity, as well as plant and human health (Guarino et al., 2020).
Zn is a biologically essential element for the human body, which is significantly required for the normal function and regulation of several enzymes and other proteins. However, excess levels of Zn can act as toxic, which can induce conditions of acute toxicity for living beings (Lindholmer, 1974; Wu et al., 2011; Wadige et al., 2014). According to the Environmental Protection Agency, United States, the Zn toxicity concentration in the case of freshwater aquatic life is 120 μg/l (for short- and long-term hazardous concentration), while the ranges for human health amount to 7,400 and 26,000 μg/l, respectively (for drinking water + consumable aquatic living beings, and consumable living beings only; US EPA, 2009; Li et al., 2019). In humans, Zn toxicity mainly occurs in either of three ways; oral, dermal, and inadvertent inhalation (Toxicological Profile for Zinc, 2005). As most of Zn toxicity cases are reported to be acute, prognosis are often good with complete recovery by treatment, such as chelation therapy or medication (Qu et al., 2012). One of the most common causes of acute Zn toxicity is the over-consumption of dietary Zn supplements. This also creates an imbalance for copper bioavailability, which can subsequently lead to its deficiency. This mechanism can likely be attributable to Zn-induced formation of copper-binding metallothioneins (Sandstead, 2013).
Other reasons include the unpremeditated consumption of dental products rich in Zn, as well as Zn contaminated food and drinks, which can result in acute gastrointestinal illness, with vomiting, nausea, and epigastric pain as the major symptoms. Inhalation of smoke and fumes containing Zn usually occurs in the case of exposure to industrial processes, such as galvanization. Furthermore, Zn-containing smoke bombs are the major sources of inhalation toxicity, primarily in soldiers and armed forces (Freitag and Caduff, 1996). However, in two reports, there was no scientific evidence that the respiratory distress was mainly attributable to Zn (Johnson and Stonehill, 1961; Zerahn et al., 1999). Inhalation of Zn fumes can induce metal fume fever, which is one of its most widely caused and reported effects (Figure 1). It is an acute condition originating in cases of industrial exposure (Zn smelting, welding, and galvanization) in the presence of metal fumes (particular size <1 μm; Vogelmeier et al., 1987). Although a non-life-threatening condition, it can manifest several symptoms in the case of acute exposure which include nausea, muscle fatigue, chest pain, cough, breathing distress, and fever (Rohrs, 1957; Putila and Guo, 2011). With accurate treatment, these symptoms can be cured completely within a matter of days (Martin et al., 1999; Plum et al., 2010). Excessive consumption of Zn can also result in gastrointestinal distress, with several symptoms, such as vomiting, nausea, cramps, diarrhea, and epigastric pain. This can also arise from ingesting Zn from food and drinks stored in galvanized containers, where the acidic nature of the food or drinks enables the liberation of Zn from the galvanized coating layer (Brown et al., 1964). Ingestion of Zn sulphate tablets was also reported to induce gastrointestinal stress symptoms in healthy subjects (Haase et al., 2008). Additionally, other compounds, such as Zn gluconate and oxide, are also observed to induce similar effects on human gastrointestinal health (Callender and Gentzkow, 1937; Lewis and Kokan, 1998; Liu et al., 2006). In human health, though Zn is well-reported to be essential for the regulation of most biological functions, its excessive levels are implicated in the causation and development of prostate cancer (Zaichick et al., 1997; Costello and Franklin, 1998), which is associated with regulating Zip1, a Zn transporter attributed to its accumulation and acquisition in prostate cells (Costello et al., 1999; Franklin et al., 2005). Though men having a moderate to high level of Zn intake may be reported to face a lower risk of prostate cancer, exceedingly high concentrations or perpetual Zn intake can result otherwise (Jarrard, 2005; Plum et al., 2010).
Figure 1. Signs and symptoms of Zn toxicity affecting various organs and sites of the human body (Created with BioRender). Zn toxicity can occur via three primary routes; oral, dermal, and inadvertent inhalation. Though reversible, its toxicity can affect the respiratory and gastrointestinal tracts as well as the brain with various side effects. Inhalation of Zn fumes can induce metal fume fever arising from fume inhalation in industries, manifesting several symptoms in the case of acute exposure which include nausea, muscle fatigue, chest pain, cough, breathing distress, and fever.
In the environment, the persistence of Zn is the culmination of both natural and anthropogenic sources, the latter of which can arise from various sources (Hanahan and Weinberg, 2000). These sources can significantly contaminate water sources when industrial waste is discharged into river streams and water lines, where microbial and marine life get exposed. Industrial wastewaters can also be released into soils and irrigative lands where this contaminated water can enable Zn to leach into soils and affect soil microbial diversity and pH (Gautam et al., 2016).
Zn is the one of the most abundant elements (23rd in rank) found in the Earth’s crust, with an average of about 78 mg/kg (Alloway, 2008). It is deemed requisite for modern times, as it is one of most utilized metals alongside iron (Fe), aluminum (Al), and copper (Cu) in the world in terms of tonnage. Sphalerite, Zn’s primary ore, is among the most principal ores in the world. Zn mine global production was reported to increase in 2021 from that of its predecessor year, where production was observed to be halted due to lockdown restrictions (International Lead and Zinc Study Group, 2020). Therefore, in 2021, production reached approximately 14.13 million tons which resulted in a production surplus (Guo and Feng, 2013). The identified Zn resources are reported to amount to more than 1.8 billion tons in the world, with China being the second largest country in the world in terms of extracting, importing, and consumption of Zn (Li et al., 2019; U.S. Geological Survey, 2022). The natural introduction of Zn in soils is attributable to natural phenomenon of parent rock erosion and weathering. The exchangeability and bioavailability of Zn (in Zn2+ form) is dependent upon the pH of the soil, where adsorption and desorption into soil organic matter takes place (Mertens and Smolders, 2013). However, at high concentration of Zn, non-specific sorption to clay as well as mineral precipitation are primary and there is little or no correlation to pH. Typical concentrations of Zn in the soil range from 10–300 mg kg−1 (White, 1993). Zn concentration is reported to be richer in soils formed from basic rocks (e.g., basalt) as compared to acid rocks (e.g., granite; Vinogradov, 1959). Furthermore, Zn content is found to be comparatively greater in heavier soils than lighter ones (Frank et al., 1976). Other sources may likely include atmospheric deposition through natural sources like volcanic ash, forest fires, and dust (Nriagu, 1989), along with man-made sources, such as combustion of fossil fuels, galvanization, tire and railing rust, motor oil, cement, tar production, and hydraulic fluid (Imseng et al., 2019). In soil, Zn can occur in either of these five forms; water soluble, complexed, chelated, adsorbed, or exchangeable; where these forms can vary in terms of uptake, dissemination, and strength and are regulated by several factors, such as pH, and the concentration of other ions, such as Fe and manganese (Mn; Deb, 1992). Moreover, the availability of Zn to plants in the soil is contingent on clay, carbonate, and total Zn content, redox, and moisture conditions, microbial activity in soil, as well as the concentration of micro- and macro-nutrients (Mandal et al., 1993; Alloway, 2008). In soils formed from limestone, the chemisorption of Zn onto calcium carbonate aids the formation of Zn hydroxycarbonate which ultimately inhibits its availability to surrounding plants. Moreover, Zn readily adsorbs to kaolinite and illite types of clay in the presence of high pH conditions (Farrah and Pickering, 1977). The formation of poorly soluble Zn sulfide is facilitated by the oxidation of organic matter in the case of Zn sedimentation, which also decreases the availability of Zn to plants (Sandstead, 2013). In the atmosphere, Zn is usually found in its oxidized form, and particles containing Zn can range up to 5 mm in size. In freshwaters, the pH range most often favors the adsorption of Zn, where its binding to organic matter is facilitated by pH above 6. Normal concentration of Zn in surface and groundwater may be <10 μg/l and about 10–40 μg/l (Elinder, 1986; Sandstead, 2013). Industrial uses of Zn span a wide range of applications, including galvanization, production of brass, bronze, Zn-based alloys, metal-coating, paint, dyes, and pigments (Incharoensakdi and Kitjaharn, 2002; Wu et al., 2017).
The worldwide dilemma of environmental pollution has been aggravated by the incessant usage of heavy metals in various industries. According to the US EPA, more than 40% of industrial wastewaters are reported to be contaminated with heavy metals and organic pollutants (Sharma et al., 2018). To tackle this worsening situation, many physical, chemical, and biological methods have been introduced over the years to successfully remove these pollutants from wastewater. Physical and chemical methods have since been proven to be exorbitant and unfeasible at large-scale applications (Wang and Chen, 2009). In this regard, developing and promoting biological methods to alleviate toxic levels of heavy metals was imperative. Therefore, the introduction of novel approaches has unlocked the usage of biological sources (microorganisms, plants as well as algae) to combat this problem (Ahemad and Malik, 2012). Plants have evolved to adapt numerous defense mechanisms against the exposure of excess heavy metals, including complexation, chelation with metallothioneins, and migration with ligands via plasma membrane channels (Cunningham et al., 1995; Wei et al., 2021). This mechanism is known as phytoremediation. Furthermore, bacteria, yeast, fungi, protozoa, and algae that grow despite the harsh environment of industrial wastewaters have evolved to harbor various resistance mechanisms that can aid in their survival (Zahoor and Rehman, 2009). Bioremediation is the process which employs the use of biological agents for the removal of heavy metals from various environments, particularly effluents and wastewaters, which has since been hailed as a cheaper, more efficient method than chemical and physical ones (Timková et al., 2018). As the problem of pollution is getting more pronounced all over the world, more and more importance is being given to detoxification methods of effluents prior to their release (Shamim and Rehman, 2012). In soil, excessive levels of heavy metals may induce alteration to soil microbial diversity and selective survival in soil microbes (Berendonk et al., 2015; Epelde et al., 2015). This can be mitigated by adapting an efficient strategy for the evolution of microbes, based on encoded genetic mechanisms, a phenomenon found in several bacterial strains which survive in heavy metal-polluted areas (Aka and Babalola, 2017). Therefore, bacteria can be utilized for remediating heavy metals from polluted areas (Chen et al., 2018). Though many efforts regarding heavy metal resistance mechanisms in bacteria are majorly focusing upon single isolated strains, a comprehensive elucidation of resistance mechanisms on the whole, contaminated areas, and the feasibility of such strategies are crucial for the optimization of bioremediation (Chen et al., 2019).
Intracellular concentrations of Zn are regulated through its homeostasis, where the use of a Zn regulator enables cells to regulate the transcriptional expression of Zn transporters which facilitate its import and export to and from the cell. This mechanism employed by cells is driven by conditions of Zn toxicity (efflux) and starvation (uptake) across the bacterial cell membrane (Capdevila et al., 2016). In bacteria, general resistance mechanisms pertain to both Gram-negative and -positive bacteria, with many agents overlapping in many species for a particular metal. These general mechanisms can aid in metal resistance through various ways, such as efflux, sequestration, chelation, and uptake. For Zn, several of these mechanisms are widely reported in many bacterial species. The intracellular regulation (uptake and efflux) of Zn2+ ions across the cell membrane is mediated by Zur/SlyA/MarR family and the MerR (ZntR) and ArsR/SmtB families, respectively (Mikhaylina et al., 2018). Zur is a member of the Fur family, which represses znuABC (an ABC transporter which facilitates Zn2+ ions uptake) under normal concentrations of Zn2+ in the cell (Hantke, 2001). However, in conditions of Zn2+ scarcity, Zur reverses its functions and induces the transporter to uptake Zn2+ from the outer environment. Moreover, it activates the transcription of a P-type ATPase which mediates the efflux of Zn2+ out of the cell under conditions of its toxicity (Capdevila et al., 2016). In Streptococcus pneumoniae, CzcD, a member of the CDF family, acts as major resistance determinant for Zn (Figure 2; Suryawati, 2018).
Figure 2. Overview of the general resistance mechanisms for Zn found in Gram-negative and -positive bacteria (Created with BioRender). In Gram negative and positive bacteria, general resistance mechanisms aid in efflux, sequestration, chelation, and metal uptake for their survival in toxic or scarce conditions. For the metal Zn, uptake in Gram positive bacteria from the outer environment is facilitated by P-type ATPase (ZosA), and Zn membrane transporters. For efflux of Zn ions out of the cell, CDF and RND efflux pumps (Czc determinants found in both Gram positive and negative bacteria), as well as CPx-type (CadA in Gram positive bacteria) and P-type ATPases (ZntA in both Gram positive and negative bacteria) are majorly involved. Moreover, intracellular or periplasmic proteins can bind to Zn inside the cell.
In order to control Zn homeostasis, bacterial cells are able to enable the import and export of Zn ions via Zn uptake systems. In most bacterial species, two highly specified uptake systems are found to act in conditions of extreme toxicity (low-affinity uptake system) and extreme scarcity (high-affinity uptake systems), respectively, both of which are used in different conditions. In moderate conditions inside the cell, low-affinity uptake transporters are used to maintain levels of Zn (Hantke, 2001). The ABC family, comprised of three regulatory proteins (ZnuA, ZnuB, and ZnuC) is attributable to high-affinity uptake of Zn in the cell (Patzer and Hantke, 1999) and is a member of the ATP-binding cassette transporter family of the ZnuABC and AdcABC/ZitSPQ type. ZnuA is a soluble protein which resides in the outer membrane of the bacterial cell, whereas ZnuB is the protein which is found in the inner membrane and interacts with ZnuA. The third protein, ZnuC, regulates the breakdown of ATP, thus contributing to the uptake and efflux of the metal. Reports suggest that the deletion of ZnuA, ZnuB, and/or ZnuC or znuABC in different bacterial species can result in the decreased uptake of Zn (Porcheron et al., 2013). Though much is known about this high-affinity uptake system, very little is reported about the other uptake system (Chandra et al., 2007). In Bacillus subtilis, the uptake of Zn2+ is regulated majorly by Zur family, but another uptake system known as ZosA (which is a P-type ATPase) was also reported to be active in conditions of oxidative stress (Suryawati, 2018; Figure 2; Table 2).
In conditions of Zn toxicity, the expression of efflux systems in bacterial cells aids in the prevention of overabundance of Zn (Guilhen et al., 2013). In bacteria, three families of exporters; cation diffusion facilitator (CDF), Resistance nodulation division (RND) efflux pumps, and P-type ATPases are majorly reported to be involved in metal export out of bacterial cells (Kolaj-Robin et al., 2015). Among these families, the CDF family is one of the most commonly found protein families in living beings. In Escherichia coli, ZitB and YiiP proteins, members of the CDF family, are transporters which regulate the efflux of Zn2+ ions via using the energy generated through the uptake of H+ ions (Porcheron et al., 2013). Another member of the CDF family, ZntA, reported to mediate resistance against Zn2+ ions in Staphylococcus aureus, is a well-known Zn transporter (Singh et al., 1999). In Caulobacter crescentus, the czrCBA system is the major efflux facilitator for Cd2+ and Zn2+ ions (Valencia et al., 2013). The Czc determinant confers resistance via efflux against various metals, such as Co2+, Zn2+, and Cd2+ ions in Alcaligenes eutrophus (Nies et al., 1989). This efflux system is a cation/proton anti-porter system which enables the efflux of cations from the cell, and consists of three structural genes (czcABC) which in turn encode three regulatory proteins of the efflux pump CzcA, CzcB, and CzcD (Nies, 1992). Moreover, efflux of Zn2+ ions in B. subtilis is mediated by CadA, which is a CPx-type ATPase efflux system (Gaballa et al., 2002). P-type ATPase family is reported to be found in both eukaryotes and bacteria, though they are generally well-defined in the latter. These transporters are regulated either by MerR/ZntR or ArsR/SmtB family members, which are often found to be working in close association with other metal transport proteins (Hantke, 2001). P-type ATPases regulate the transportation of metal ions across the bacterial cell membrane by utilizing the energy of ATP hydrolysis (Apell, 2004). A good example of P-type ATPase is ZntA in E. coli, which is regulated by MerR-like regulator via the attachment of apo-ZntR dimer to the promotor region of zntA, repressing its transcription (Porcheron et al., 2013). This phenomenon takes place when intracellular Zn concentration exceed sub-toxicity levels (Khan et al., 2002). The RND family is majorly found in Gram-negative bacteria which is involved in the active efflux of antibiotics and other therapeutic agents. These systems have large periplasmic domains and in many bacterial species are known to form complexes with extracellular channels and adaptor proteins (Nikaido and Takatsuka, 2009). In C. crescentus, CzrCBA is known to export cadmium and Zn ions from cytoplasm of cells (Valencia et al., 2013). The export of Zn and other metal ions in Ralstonia metallidurans is facilitated by CzcABC system, which is regulated by CzcS/CzcR two-component regulatory system (Anton et al., 1999; Hantke, 2001; Blencowe and Morby, 2003; Blindauer, 2015; Suryawati, 2018; Figure 2).
The bioremediation of Zn from various environments has been reported in various studies over the years. Brevibacterium sp. was effective in removing Zn from polluted environments, even in concentrations as low as 0.1 mm (Taniguchi et al., 2000). The biosorption potential of Delftia tsuruhatensis for Zn and lead (Pb) was also reported in a study (Bautista-Hernandez et al., 2012). Bacterial consortia of several species, such as Alcaligenes faecalis, Staphylococcus aureus, Streptococcus lactis, Micrococcus luteus, and Enterobacter aerogenes, was reported to remove various heavy metals, such as copper (Cu), cadmium (Cd), Pb, and Zn from wastewaters (Silambarasan and Abraham, 2013). Pseudomonas putida, another Gram-negative bacterium, was reported to demonstrate highest ability of Zn removal among other isolates in a study, whereas Bacillus subtilis was the most effective among Gram-positive bacteria, respectively (Kamika and Momba, 2013). Rhodobacter capsulatus was reported to efficiently remove Zn from polluted environments with the help of its wild-type strain and an enclosed plasmid which conferred resistance (Magnin et al., 2014). Bacillus licheniformis and Salmonella typhi were reported to remove more than 90% of Zn from contaminated samples, whereas Pseudomonas fluorescens and E. coli were effective in removing more than 96 and 93% of Zn, respectively (Basha and Rajaganesh, 2014). P. fluorescens and P. putida were also observed to be good bioremediation agents for many heavy metals, including Zn (Upadhyay and Srivastava, 2014; Ahmady-Asbchin et al., 2015; Naz et al., 2016). In a similar study, several bacterial species, such as P. aeruginosa, S. aureus, E. coli, Proteus vulgaris, and Klebsiella pneumoniae, were evaluated for their heavy metal removal ability, where their mixed consortium was able to remediate more than 90% of Zn. Among pure isolates, P. aeruginosa was effective in removing the largest quantity of Zn from contaminated medium (53.9%), as well as other heavy metals (Oaikhena et al., 2016). Among Gram-positive bacteria, Staphylococcus epidermidis was also reported to be effective in removing Zn (<80%) as well as other heavy metals from contaminated water (Nwagwu et al., 2017). Streptomyces sp. also demonstrated to remove Zn under controlled conditions (Sedlakova-Kadukova et al., 2019). Another study revealed Serratia sp. to be effective in tolerating high concentrations of Zn. High values of biosorption (more than 90%) were observed under Zn stress, which also aided in growth augmentation of plant roots, shoots, and chlorophyll content (Kour et al., 2019). Furthermore, the bioremediation of Zn by various Bacillus sp. from soil and wastewaters has been well-reported in various studies over the years (Joo et al., 2010; Singh and Chopra, 2014; Wierzba, 2015; Huang et al., 2020; Table 3). A recent study also sought out to investigate Zn removal efficacy of Sporosarcina pasteurii from contaminated soils, where urease-producing isolates and S. pasteurii had removal ability of more than 70%, and L-asparaginase-producing isolate had the ability to remove more than 90% Zn in solution (Ghorbanzadeh et al., 2022).
Table 3. Zn biosorption and metal uptake ability of various Gram-positive and Gram-negative bacterial species, as reported in different studies.
Zn is the one of the major elements (23rd) found in the Earth’s crust, with an average concentration of approximately 78 mg/kg. After Fe, Al, and Cu, it is the major metal used in the world, in terms of tonnage. Zn is regarded as a biologically essential element for living beings. It is reported to be imperatively required for the normal functioning of major enzymes and proteins. It is also reported to be effective in combating viral infections and strengthening the immune system against them, which involves the inhibition of the entry, fusion, replication, translation, and emission of virus particles into and out of the host cells. However, excess levels of Zn can act as toxic, which can induce conditions of acute toxicity for living beings. For its mitigation from the environment, bioremediation is a comparatively cheaper, more efficient, and effective method employed for the removal of heavy metals from various environments, like effluents and wastewaters. In bacteria, general resistance mechanisms (such as efflux, uptake, sequestration, and chelation) are found in Gram-negative and -positive bacteria which aid in the removal of heavy metals, such as Zn, from polluted environments. However, these resistance mechanisms need to be investigated on a molecular level so that these species could be manipulated for industrial applications, as one of the drawbacks of bioremediation is its inability to be reproduced from lab scale to large scale. This review focuses on the uses, toxicity, and bioremediation of Zn with special insight into its chemical and physical properties as well as its role in nutrition and immunity. Further innovative aspects of Zn remediation and toxicity can aid in the elucidation of resistance mechanisms, as well as Zn bioavailability.
All authors contributed equally in this manuscript. All authors read and approved the submitted version.
The authors declare that the research was conducted in the absence of any commercial or financial relationships that could be construed as a potential conflict of interest.
All claims expressed in this article are solely those of the authors and do not necessarily represent those of their affiliated organizations, or those of the publisher, the editors and the reviewers. Any product that may be evaluated in this article, or claim that may be made by its manufacturer, is not guaranteed or endorsed by the publisher.
This study was financially supported by the Science and Technology Major Project of Inner Mongolia (No. ZDZX2018054), China, the National Natural Science Funds of China (31370474), and the Jiangsu Province Postdoctoral Excellence Program (2022ZB155). Special thanks also go to the three reviewers for their very useful comments and suggestions.
ATP, Adenosine 5′-triphosphate; CDF, Cation diffusion facilitator; DNA, Deoxyribonucleic acid; IFN-γ, Interferon gamma; IL-1α, Interleukin 1 alpha; IL-1β, Interleukin 1 beta; IL-2, Interleukin-2; IL-6, Interleukin-6; NADPH, Reduced nicotinamide adenine dinucleotide phosphate; NF-κB, Nuclear factor kappa B; NK, Natural killer; RNA, Ribonucleic acid; RND, Resistance nodulation division; ROS, Reactive oxygen species; SARS, Severe acute respiratory syndrome; TLR, Toll-like receptor; TNFR, Tumor necrosis factor receptors; TNF-α, Tumor necrosis factor α.
Agnieszka, B., Tomasz, C., and Jerzy, W. (2014). Chemical properties and toxicity of soils contaminated by mining activity. Ecotoxicology 23, 1234–1244. doi: 10.1007/s10646-014-1266-y
Ahemad, M., and Malik, A. (2012). Bioaccumulation of heavy metals by zinc resistant bacteria isolated from agricultural soils irrigated with wastewater. J. Bacteriol. 2, 12–21. doi: 10.3923/bj.2012.12.21
Ahmady-Asbchin, S., Safari, M., and Tabaraki, R. (2015). Biosorption of Zn(II) by Pseudomonas aeruginosa isolated from a site contaminated with petroleum. Desalin. Water Treat. 54, 3372–3379. doi: 10.1080/19443994.2014.913202
Aka, R. J. N., and Babalola, O. O. (2017). Identification and characterization of Cr-, cd-, and Ni-tolerant bacteria isolated from mine tailings. Biorem. J. 21, 1–19. doi: 10.1080/10889868.2017.1282933
Alam, M. Z., and Ahmad, S. (2013). Multi-metal biosorption and bioaccumulation by Exiguobacterium sp. ZM-2. Ann. Microbiol. 63, 1137–1146. doi: 10.1007/s13213-012-0571-z
Alloway, B. J. (2008). Zinc in Soils and crop Nutrition. Brussels, Belgium and Paris: International fertilizer Industry Association and International Zinc Association, 135.
Anju, M., and Banerjee, D. K. (2011). Associations of cadmium, zinc, and lead in soils form lead and zinc mining area as studied by single and sequential extractions. Environ. Monit. Assess. 176, 67–85. doi: 10.1007/s10661-010-1567-4
Anton, A., Grosse, C., Reissmann, J., Pribyl, T., and Nies, D. H. (1999). CzcD is a heavy metal ion transporter involved in regulation of heavy metal resistance in Ralstonia sp. strain CH34. J. Bacteriol. 181, 6876–6881. doi: 10.1128/JB.181.22.6876-6881.1999
Asl, S. H., Nikfarjam, S., Majidi Zolbanin, N., Nassiri, R., and Jafari, R. (2021). Immunopharmacological perspective on zinc in SARS-CoV-2 infection. Int. Immunopharmacol. 96, 107630–107617. doi: 10.1016/j.intimp.2021.107630
Audi, G., Kondev, F. G., Wang, M., Huang, W. J., and Naimi, S. (2017). The NUBASE2016 evaluation of nuclear properties. Chin. Phys. C. 41:30001. doi: 10.1088/1674-1137/41/3/030001
Apell, H. J. (2004). How do P-type ATPases transport ions?. Bioelectrochemistry (Amsterdam, Netherlands), 63, 149–156. doi: 10.1016/j.bioelechem.2003.09.021
Baastrup, R., Sørensen, M., Balstrøm, T., Frederiksen, K., Larsen, C. L., and Tjønneland, A. (2008). Arsenic in drinking-water and risk for cancer in Denmark. Environ. Health Persp. 116, 231–237. doi: 10.1289/ehp.10623
Babák, L., Šupinová, P., Zichová, M., Burdychová, R., and Vítová, E. (2012). Biosorption of cu, Zn and Pb by thermophilic bacteria - effect of biomass concentration on biosorption capacity. Acta Univ. Agric. Silvic. Mendelianae Brun. 60, 9–18. doi: 10.11118/actaun201260050009
Bădulici, S., Chirulescu, Z., Chirilă, P., Chirilă, M., and Roşca, A. (1994). Treatment with zincum metallicum CH5 in patients with liver cirrhosis—preliminary study. Rom. J. Intern. Med. 32, 215–219.
Baran, A. (2012). Assessment of zinc content and mobility in maize. Ecol. Chem. Eng. A. 19, 699–706. doi: 10.2428/ecea.2012.19(07)069
Baran, A. (2013). Assessment of Zea mays sensitivity to toxic content of zinc in soil. Pol. J. Environ. Stud. 22, 77–83.
Baran, A., Wieczorek, J., Mazurek, R., Urbański, K., and Klimkowicz-Pawlas, A. (2018). Potential ecological risk assessment and predicting zinc accumulation in soils. Environ. Geochem. Health 40, 435–450. doi: 10.1007/s10653-017-9924-7
Basha, S. A., and Rajaganesh, K. (2014). Microbial bioremediation of heavy metals from textile industry dye effluents using isolated bacterial strains. Int. J. Curr. Microbiol. App. Sci. 3, 785–794.
Bautista-Hernandez, D. A., Ramirez-Burgos, I. L., Duran-Paramo, E., and Fernandez-Linares, L. (2012). Zinc and lead biosorption by Delftia tsuruhatensis: A bacterial strain resistant to metals isolated from mine tailings. J. Water Resour. Protect. 4, 207–216. doi: 10.4236/jwarp.2012.44023
Bautista-Hernández, D., Ramírez-Burgos, L., Duran-Páramo, E., and Fernández-Linares, L. (2012). Zinc and lead biosorption by Delftia tsuruhatensis: A bacterial strain resistant to metals isolated from mine tailings. J. Water Resour. Protect. 04, 207–216. doi: 10.4236/jwarp.2012.44023
Beck, F. W., Prasad, A. S., Kaplan, J., Fitzgerald, J. T., and Brewer, G. J. (1997). Changes in cytokine production and T cell subpopulations in experimentally induced zinc-deficient humans. Am. J. Phys. 272, E1002–E1007. doi: 10.1152/ajpendo.1997.272.6.E1002
Berendonk, T. U., Manaia, C. M., Merlin, C., Fatta-Kassinos, D., Cytryn, E., Walsh, F., et al. (2015). Tackling antibiotic resistance: The environmental framework. Nat. Rev. Microbiol. 13, 310–317. doi: 10.1038/nrmicro3439
Blencowe, D. K., and Morby, A. P. (2003). Zn(II) metabolism in prokaryotes. FEMS Microbiol. Rev. 27, 291–311. doi: 10.1016/S0168-6445(03)00041-X
Blindauer, C. A. (2015). Advances in the molecular understanding of biological zinc transport. Chem. Commun. 51, 4544–4563. doi: 10.1039/C4CC10174J
Bolan, N., Kunhikrishnan, A., Thangarajan, R., Kumpiene, J., Park, J., Makino, T., et al. (2014). Remediation of heavy metal(loid)s contaminated soils - to mobilize or to immobilize? J. Hazard. Mater. 266, 141–166. doi: 10.1016/j.jhazmat.2013.12.018
Bonaventura, P., Benedetti, G., Albarède, F., and Miossec, P. (2015). Zinc and its role in immunity and inflammation. Autoimmun. Rev. 14, 277–285. doi: 10.1016/j.autrev.2014.11.008
Brown, M. A., Thom, J. V., Orth, G. L., Cova, P., and Juarez, J. (1964). Food poisoning involving zinc contamination. Arch. Environ. Health 8, 657–660. doi: 10.1080/00039896.1964.10663736
Brzóska, M. M., Kozłowska, M., Rogalska, J., Gałażyn-Sidorczuk, M., Roszczenko, A., and Smereczański, N. M. (2021). Enhanced zinc intake protects against oxidative stress and its consequences in the brain: A study in an in vivo rat model of cadmium exposure. Nutrients 13, 478–504. doi: 10.3390/nu13020478
Callender, G. R., and Gentzkow, C. J. (1937). Acute poisoning by the zinc and antimony content of limeade prepared in a galvanized iron can. Mil. Surg. 80, 67–71.
Capdevila, D. A., Wang, J. F., and Giedroc, D. P. (2016). Bacterial strategies to maintain zinc metallostasis at the host-pathogen interface. J. Biol. Chem. 291, 20858–20868. doi: 10.1074/jbc.R116.742023
Castro-Alba, V., Lazarte, C. E., Bergenståhl, B., and Granfeldt, Y. (2019). Phytate, iron, zinc, and calcium content of common Bolivian foods and their estimated mineral bioavailability. Food Sci. Nutr. 7, 2854–2865. doi: 10.1002/fsn3.1127
Cerasi, M., Ammendola, S., and Battistoni, A. (2013). Competition for zinc binding in the host-pathogen interaction. Front. Cell. Infect. Microbiol. 3: 108. doi: 10.3389/fcimb.2013.00108
Chandra, B. R., Yogavel, M., and Sharma, A. (2007). Structural analysis of ABC-family periplasmic zinc binding protein provides new insights into mechanism of ligand uptake and release. J. Mol. Biol. 367, 970–982. doi: 10.1016/j.jmb.2007.01.041
Chandrangsu, P., Rensing, C., and Helmann, J. D. (2017). Metal homeostasis and resistance in bacteria. Nat. Rev. Microbiol. 15, 338–350. doi: 10.1038/nrmicro.2017.15
Chasapis, C. T., Loutsidou, A. C., Spiliopoulou, C. A., and Stefanidou, M. E. (2012). Zinc and human health: An update. Arch. Toxicol. 86, 521–534. doi: 10.1007/s00204-011-0775-1
Chen, Y., Jiang, Y., Huang, H., Mou, L., Ru, J., Zhao, J., et al. (2018). Long-term and high-concentration heavy-metal contamination strongly influences the microbiome and functional genes in Yellow River sediments. Sci. Total Environ. 637, 1400–1412. doi: 10.1016/j.scitotenv.2018.05.109
Chen, J., Li, J., Zhang, H., Shi, W., and Liu, Y. (2019). Bacterial heavy-metal and antibiotic resistance genes in a copper tailing dam area in northern China. Front. Microbiol. 10:1916. doi: 10.3389/fmicb.2019.01916
Costello, L. C., and Franklin, R. B. (1998). Novel role of zinc in the regulation of prostate citrate metabolism and its implications in prostate cancer. Prostate 35, 285–296. doi: 10.1002/(sici)1097-0045(19980601)35:4<285::aid-pros8>3.0.co;2-f
Costello, L. C., Liu, Y., Zou, J., and Franklin, R. B. (1999). Evidence for a zinc uptake transporter in human prostate cancer cells which is regulated by prolactin and testosterone. J. Biol. Chem. 274, 17499–17504. doi: 10.1074/jbc.274.25.17499
Cunningham, S. D., Berti, W. R., and Huang, J. W. W. (1995). Phytoremediation of contaminated soils. Trends Biotechnol. 13, 393–397.
Dardenne, M., and Pleau, J. M. (1994). Interactions between zinc and thymulin. Metal. Bas. Drugs. 1, 233–239. doi: 10.1155/MBD.1994.233
Deb, D. L. (1992). Development of seoil and plant analytical methods for micronutrients and sulphur in Sri Lanka. GCPF/SRI/047/NET field document No. 11. 16.
DeCoursey, T. E., Morgan, D., and Cherny, V. V. (2003). The voltage dependence of NADPH oxidase reveals why phagocytes need proton channels. Nature 422, 531–534. doi: 10.1038/nature01523
El-barbary, T. A. A., and El-Badry, M. A. (2018). Bioremediation potential of Zn(II) by different bacterial species. Saudi J. Biomed. Res. 3, 144–150. doi: 10.21276/sjbr.2018.3.4.3
Elinder, C. G. (1986). Handbook on the Toxicology of Metals. Amsterdam: Elsevier Science Publishers.
Epelde, L., Lanzén, A., Blanco, F., Urich, T., and Garbisu, C. (2015). Adaptation of soil microbial community structure and function to chronic metal contamination at an abandoned Pb-Zn mine. FEMS Microbiol. Ecol. 91, 1–11. doi: 10.1038/nature01523
Farrah, H., and Pickering, W. F. (1977). Influence of clay-solute interactions on aqueous heavy metals ion levels. Water Air Soil Pollut. 8, 189–197. doi: 10.1007/BF00294042
Fedotov, P. S., Koerdel, W., Miro, M., Peijnenburg, W. J. G. M., Wennrich, R., and Huang, P. M. (2012). Extraction and fraction methods for exposure assessment of trace metals, metalloids and hazardous organic compounds in terrestrial environments. Crit. Rev. Environ. Sci. Technol. 42, 1117–1171. doi: 10.1080/10643389.2011.556544
Flanagan, P. R., Cluett, J., Chamberlain, M. J., and Valberg, L. S. (1985). Dual-isotope method for determination of human zinc absorption: The use of a test meal of Turkey meat. J. Nutr. 115, 111–122. doi: 10.1093/jn/115.1.111
Fraker, P. J., and King, L. E. (2004). Reprogramming of the immune system during zinc deficiency. Annu. Rev. Nutr. 24, 277–298. doi: 10.1146/annurev.nutr.24.012003.132454
Frank, R., Ishida, K., and Suda, P. (1976). Metals in agricultural soils of Ontario. Can. J. Soil Sci. 56, 181–196. doi: 10.4141/cjss76-027
Franklin, R. B., Feng, P., Milon, B., Desouki, M. M., Singh, K. K., Kajdacsy-Balla, A., et al. (2005). hZIP1 zinc uptake transporter down regulation and zinc depletion in prostate cancer. Mol. Cancer 4, 32–45. doi: 10.1186/1476-4598-4-32
Freitag, A., and Caduff, B. (1996). ARDS caused by military zinc fumes exposure. Schweiz. Med. Wochenschr. 126, 1006–1010.
Gaballa, A., Wang, T., Ye, R. W., and Helmann, J. D. (2002). Functional analysis of the Bacillus subtilis Zur regulon. J. Bacteriol. 184, 6508–6514. doi: 10.1128/JB.184.23.6508-6514.2002
Gammoh, N. Z., and Rink, L. (2017). Zinc in infection and inflammation. Nutrients 9, 624–649. doi: 10.3390/nu9060624
Gautam, P. K., Gautam, R. K., Banerjee, S., Chattopadhyaya, M. C., and Pandey, J. D. (2016). “Heavy metals in the environment: fate, transport, toxicity and remediation technologies,” in Heavy Metals. ed. D. Pathania (New York: Nava Science Publishers, Inc.), 101–130.
Ghorbanzadeh, N., Ghanbari, Z., Farhangi, M. B., and Rad, M. K. (2022). Zinc bioremediation in soil by two isolated L-asparaginase and urease producing bacteria strains. Appl. Geochem. 140:105271. doi: 10.1016/j.apgeochem.2022.105271
Graf, G. G. (1997). “Zinc,” in Handbook of Extractive Metallurgy. ed. F. Habashi (Weinheim: Wiley-VCH), 641–688.
Green-Ruiz, C., Rodriguez-Tirado, V., and Gomez-Gil, B. (2008). Cadmium and zinc removal from aqueous solutions by Bacillus jeotgali: pH, salinity and temperature effects. Bioresour. Technol. 99, 3864–3870. doi: 10.1016/j.biortech.2007.06.047
Guarino, F., Improta, G., Triassi, M., Cicatelli, A., and Castiglione, S. (2020). Effects of zinc pollution and compost amendment on the root microbiome of a metal tolerant poplar clone. Front. Microbiol. 11:1677. doi: 10.3389/fmicb.2020.01677
Guilhen, C., Taha, M. K., and Veyrier, F. J. (2013). Role of transition metal exporters in virulence: the example of Neisseria meningitidis. Front. Cell. Infect. Microbiol. 3:102. doi: 10.3389/fcimb.2013.00102
Guo, C. Q., and Feng, J. C. (2013). Zinc market analysis and outlook. China Nonferr. Metals. 2, 44–45.
Haase, H., Overbeck, S., and Rink, L. (2008). Zinc supplementation for the treatment or prevention of disease: current status and future perspectives. Exp. Gerontol. 43, 394–408. doi: 10.1016/j.exger.2007.12.002
Haase, H., and Rink, L. (2014a). Multiple impacts of zinc on immune function. Metallomics 6, 1175–1180. doi: 10.1039/c3mt00353a
Haase, H., and Rink, L. (2014b). Zinc signals and immune function. Biofactors 40, 27–40. doi: 10.1002/biof.1114
Habashi, F. (2013). “Zinc, physical and chemical properties”, in Encyclopedia of Metalloproteins, ed. Kretsinger, R. H., Uversky, V. N., and Permyakov, E. A. (New York, NY, Springer).
Hacisalihoglu, G. (2020). Zinc (Zn): The last nutrient in the alphabet and shedding light on Zn efficiency for the future of crop production under suboptimal Zn. Plants 9, 1471–1480. doi: 10.3390/plants9111471
Hacisalihoglu, G., and Blair, M. (2020). Current advances in zinc in soils and plants: implications for zinc efficiency and biofortification studies. Achiev. Sustain. Crop Nutr. 76, 337–353. doi: 10.19103/AS.2019.0062.16
Haiyan, W., and Stuanes, A. O. (2003). Heavy metal pollution in air-water-soil-plant system of Zhuzhou City, Hunan Province. China. Water Air Soil Pollut. 147, 79–107. doi: 10.1023/A:1024522111341
Hambidge, K. M., Miller, L. V., Westcott, J. E., Sheng, X., and Krebs, N. F. (2010). Zinc bioavailability and homeostasis. Am. J. Clin. Nutr. 91, 1478S–1483S. doi: 10.3945/ajcn.2010.28674I
Hanahan, D., and Weinberg, R. A. (2000). The hallmarks of cancer. Cell 100, 57–70. doi: 10.1016/s0092-8674(00)81683-9
Hantke, K. (2001). Bacterial zinc transporters and regulators. Biometals 14, 239–249. doi: 10.1023/a:1012984713391
Hasegawa, H., Suzuki, K., Suzuki, K., Nakaji, S., and Sugawara, K. (2000). Effects of zinc on the reactive oxygen species generating capacity of human neutrophils and on the serum opsonic activity in vitro. Luminescence 15, 321–327. doi: 10.1002/1522-7243(200009/10)15:5<321::AID-BIO605>3.0.CO;2-O
Holleman, A. F., Wiberg, E., and Wiberg, N. (1985). “Zink,” in Lehrbuch der Anorganischen Chemie. ed. A. F. Holleman (Germany: Walter de Gruyter), 91–100.
Hough, R. L., Breward, N., Young, S. D., Crout, N. M. J., Tye, A. M., Moir, A. M., et al. (2004). Assessing potential risk of heavy metal exposure from consumption of home-produced vegetables by urban populations. Environ. Health Persp. 112, 215–221. doi: 10.1289/ehp.5589
Hu, J., and Dong, Z. (2011). Development of lead source-specific exposure standards based on aggregate exposure assessment: Bayesian inversion from biomonitoring information to multipathway exposure. Environ. Sci. Technol. 46, 1144–1152. doi: 10.1021/es202800z
Huang, J., Wang, J., and Jia, L. (2020). Removal of zinc (II) from livestock and poultry sewage by a zinc (II) resistant bacteria. Sci. Rep. 10:21027. doi: 10.1038/s41598-020-78138-z
Hynninen, A., Tönismann, K., and Virta, M. (2010). Improving the sensitivity of bacterial bioreporters for heavy metals. Bioeng Bugs. 1, 132–138. doi: 10.4161/bbug.1.2.10902
Imseng, M., Wiggenhauser, M., Müller, M., Keller, A., Frossard, E., Wilcke, W., et al. (2019). The fate of Zn in agricultural soils: A stable isotope approach to anthropogenic impact, soil formation and soil-plant cycling. Environ. Sci. Technol. 53, 4140–4149. doi: 10.1021/acs.est.8b03675
Incharoensakdi, A., and Kitjaharn, P. (2002). Zinc biosorption from aqueous solution by a halotolerant cyanobacterium Aphanothece halophytica. Curr. Microbiol. 45, 261–264. doi: 10.1007/s00284-002-3747-0
International Lead and Zinc Study Group. (2020) ILZSG Session/Forecasts: Lisbon, Portugal: International Lead and Zinc Study Group press release.
Irving, J. A., Mattman, A., Lockitch, G., Farrell, K., and Wadsworth, L. D. (2003). Element of caution: A case of reversible cytopenias associated with excessive zinc supplementation. CMAJ 169, 129–131.
Ishida, T. (2019). Review on the role of Zn2+ ions in viral pathogenesis and the effect of Zn2+ ions for host cell-virus growth inhibition. Am. J. Biomed. Sci. Res. 2, 28–37. doi: 10.34297/AJBSR.2019.02.000566
Jarosz, M., Olbert, M., Wyszogrodzka, G., Młyniec, K., and Librowski, T. (2017). Antioxidant and anti-inflammatory effects of zinc. Zinc-dependent NF-κB signaling. Inflammopharmacology 25, 11–24. doi: 10.1007/s10787-017-0309-4
Jarrard, D. F. (2005). Does zinc supplementation increase the risk of prostate cancer? Arch. Ophthalmol. 123, 102–103. doi: 10.1001/archopht.123.1.102
Jensen, W. B. (2003). The place of zinc, cadmium, and mercury in the periodic table. J. Chem. Ed. 80, 952–961. doi: 10.1021/ed080p952
Johnson, F. A., and Stonehill, R. B. (1961). Chemical pneumonitis from inhalation of zinc chloride. Dis. Chest 40, 619–624. doi: 10.1378/chest.40.6.619
Joo, J. H., Hassan, S. H., and Oh, S. E. (2010). Comparative study of biosorption of Zn2+ by Pseudomonas aeruginosa and Bacillus cereus. Int. Biodeter. Biodegrad. 64, 734–741. doi: 10.1016/j.ibiod.2010.08.007
Kamika, I., and Momba, M. N. (2013). Assessing the resistance and bioremediation ability of selected bacterial and protozoan species to heavy metals in metal-rich industrial wastewater. BMC Microbiol. 13, 28–42. doi: 10.1186/1471-2180-13-28
Khan, S., Brocklehurst, K. R., Jones, G. W., and Morby, A. P. (2002). The functional analysis of directed amino-acid alterations in ZntR from Escherichia coli. Biochem. Biophys. Res. Commun. 299, 438–445. doi: 10.1016/s0006-291x(02)02660-8
Kim, R. Y., Yoon, J. K., Kim, T. S., Yang, J. E., Owens, G., and Kim, K. R. (2015). Bioavailability of heavy metals in soils: definitions and practical implementation: a critical review. Environ. Geochem. Health 37, 1041–1061. doi: 10.1007/s10653-015-9695-y
King, L. E., Frentzel, J. W., Mann, J. J., and Fraker, P. J. (2005). Chronic zinc deficiency in mice disrupted T cell lymphopoiesis and erythropoiesis while B cell lymphopoiesis and myelopoiesis were maintained. J. Am. Coll. Nutr. 24, 494–502. doi: 10.1080/07315724.2005.10719495
Kolaj-Robin, O., Russell, D., Hayes, K. A., Pembroke, J. T., and Soulimane, T. (2015). Cation diffusion facilitator family: structure and function. FEBS Lett. 589, 1283–1295. doi: 10.1016/j.febslet.2015.04.007
Kour, R., Jain, D., Bhojiya, A. A., Sukhwal, A., Sanadhya, S., Saheewala, H., et al. (2019). Zinc biosorption, biochemical and molecular characterization of plant growth-promoting zinc-tolerant bacteria. 3. Biotech 9, 421–438. doi: 10.1007/s13205-019-1959-2
Krebs, N. F. (2000). Overview of zinc absorption and excretion in the human gastrointestinal tract. J. Nutr. 130, 1374S–1377S. doi: 10.1093/jn/130.5.1374S
Kumaran, N. S., Sundaramanicam, A., and Bragadeeswaran, S. (2011). Adsorption studies on heavy metals by isolated cyanobacterial strain (nostoc sp.) from uppanar estuarine water, southeast coast of India. J. Appl. Sci. Res. 7, 1609–1615.
Lee, S. R. (2018). Critical role of zinc as either an antioxidant or a prooxidant in cellular systems. Oxidative Med. Cell. Longev. 2018, 1–11. doi: 10.1155/2018/9156285
Lewis, M. R., and Kokan, L. (1998). Zinc gluconate: acute ingestion. J. Toxicol. Clin. Toxicol. 36, 99–101. doi: 10.3109/15563659809162595
Li, X. F., Wang, P. F., Feng, C. L., Liu, D. Q., Chen, J. K., and Wu, F. C. (2019). Acute toxicity and hazardous concentrations of zinc to native freshwater organisms under different pH values in China. Bull. Environ. Contam. Toxicol. 103, 120–126. doi: 10.1007/s00128-018-2441-2
Lindholmer, C. (1974). Toxicity of zinc ions to human spermatozoa and the influence of albumin. Andrologia 6, 7–16. doi: 10.1111/j.1439-0272.1974.tb01583.x
Liu, C. H., Lee, C. T., Tsai, F. C., Hsu, S. J., and Yang, P. M. (2006). Gastroduodenal corrosive injury after oral zinc oxide. Ann. Emerg. Med. 47:296. doi: 10.1016/j.annemergmed.2005.09.020
Maares, M., and Haase, H. (2020). A guide to human zinc absorption: general overview and recent advances of in vitro intestinal models. Nutrients 12, 762–805. doi: 10.3390/nu12030762
Magnin, J. P., Gondrexon, N., and Willison, J. C. (2014). Zinc biosorption by the purple non-sulfur bacterium Rhodobacter capsulatus. Can. J. Microbiol. 60, 829–837. doi: 10.1139/cjm-2014-0231
Man, Y. B., Sun, X. L., Zhao, Y. G., Lopez, B. N., Chung, S. S., Wu, S. C., et al. (2020). Health risk assessment of abandoned agricultural soils based on heavy metal contents in Hong Kong, the world’s most populated city. Environ. Int. 36, 570–576. doi: 10.1016/j.envint.2010.04.014
Mandal, B., Mandal, L. N., and Ali, M. H. (1993). “Chemistry of zinc Availability in Submerged Soils in Relation to zinc Nutrition of rice crop,” in Proceedings of the Workshop on Micronutrients, Bhubaneswar, India, 240–253.
Marchetti, C. (2013). Role of calcium channels in heavy metal toxicity. ISRN Toxicol. 2013, 1–9. doi: 10.1155/2013/184360
Mari, M., Nadal, M., Schuhmacher, M., and Domingo, J. L. (2009). Exposure to heavy metals and PCDD/fs by the population living in the vicinity of a hazardous waste landfill in Catalonia, Spain: health risk assessment. Environ. Int. 35, 1034–1039. doi: 10.1016/j.envint.2009.05.004
Martin, C. J., Le, X. C., Guidotti, T. L., Yalcin, S., Chum, E., Audette, R. J., et al. (1999). Zinc exposure in Chinese foundry workers. Am. J. Ind. Med. 35, 574–580. doi: 10.1016/j.envint.2009.05.004
Mertens, J., and Smolders, E. (2013). “Zinc”, in Heavy Metals in Soils: Trace Metals and Metalloids in Soils and their Bioavailability (Dordrecht;, Springer) Brian J. Alloway 465–493.
Mikhaylina, A., Ksibe, A. Z., Scanlan, D. J., and Blindauer, C. A. (2018). Bacterial zinc uptake regulator proteins and their regulons. Biochem. Soc. Trans. 46, 983–1001. doi: 10.1042/BST20170228
Miquel, E., and Farré, R. (2007). Effects and future trends of casein phosphopeptides on zinc bioavailability. Trends Food Sci. Technol. 18, 139–143. doi: 10.1016/j.tifs.2006.11.004
Mwandira, W., Nakashima, K., Kawasaki, S., Arabelo, A., Banda, K., Nyambe, I., et al. (2020). Biosorption of Pb (II) and Zn(II) from aqueous solution by Oceanobacillus profundus isolated from an abandoned mine. Sci. Rep. 10:21189. doi: 10.1038/s41598-020-78187-4
Nadal, M., Bocio, A., Schuhmacher, M., and Domingo, J. (2005). Trends in the levels of metals in soils and vegetation samples collected near a hazardous waste incinerator. Arch. Environ. Contam. Tox. 49, 290–298. doi: 10.1007/s00244-004-0262-2
Narayanan, S. E., Rehuman, N. A., Harilal, S., Vincent, A., Rajamma, R. G., Behl, T., et al. (2020). Molecular mechanism of zinc neurotoxicity in Alzheimer’s disease. Environ. Sci. Pollut. Res. 27, 43542–43552. doi: 10.1007/s11356-020-10477-w
National Center for Biotechnology Information. (2022) PubChem element summary for atomic number 30, zinc. https://pubchem.ncbi.nlm.nih.gov/element/Zinc (Accessed on February 18, 2022).
Naz, T., Khan, M. D., Ahmed, I., Rehman, S. U., Rha, E. S., Malook, I., et al. (2016). Biosorption of heavy metals by Pseudomonas species isolated from sugar industry. Toxicol. Ind. Health 32, 1619–1627. doi: 10.1177/0748233715569900
Nies, D. H. (1992). Resistance to cadmium, cobalt, zinc, and nickel in microbes. Plasmid 27, 17–28. doi: 10.1016/0147-619x(92)90003-s
Nies, D. H., Nies, A., Chu, L., and Silver, S. (1989). Expression and nucleotide sequence of a plasmid determined divalent cation efflux system from Alcaligenes eutrophus. Proc. Natl. Acad. Sci. U. S. A. 86, 7351–7355. doi: 10.1073/pnas.86.19.7351
Nikaido, H., and Takatsuka, Y. (2009). Mechanisms of RND multidrug efflux pumps. Biochim. Biophys. Acta 1794, 769–781. doi: 10.1016/j.bbapap.2008.10.004
Nriagu, J. O. (1989). A global assessment of natural sources of atmospheric trace metals. Nature 338, 47–49. doi: 10.1038/338047a0
Nwagwu, E. C., Yilwa, V. M., Egbe, N. E., and Onwumere, G. B. (2017). Isolation and characterization of heavy metal tolerant bacteria from Panteka stream, Kaduna, Nigeria and their potential for bioremediation. Afr. J. Biotech. 16, 32–40. doi: 10.5897/AJB2016.15676
Oaikhena, E. E., Makaije, D. B., Denwe, S. D., Namadi, M. M., and Haroun, A. A. (2016). Bioremediation potentials of heavy metal tolerant bacteria isolated from petroleum refinery effluent. Am. J. Environ. Protect. 5, 29–34. doi: 10.11648/j.ajep.20160502.12
Olaniran, A. O., Balgobind, A., and Pillay, B. (2013). Bioavailability of heavy metals in soil: impact on microbial biodegradation of organic compounds and possible improvement strategies. Int. J. Mol. Sci. 14, 10197–10228. doi: 10.3390/ijms140510197
Otten, J. J., Pitzi Hellwig, J., and Meyers, L. D. (2006). Dietary Reference Intakes. Washington, DC, USA: National Academies Press.
Patzer, S. I., and Hantke, K. (1999). SufS is a NifS-like protein, and SufD is necessary for stability of the [2Fe-2S] FhuF protein in Escherichia coli. J. Bacteriol. 181, 3307–3309. doi: 10.1128/JB.181.10.3307-3309.1999
Pécoud, A., Donzel, P., and Schelling, J. L. (1975). Effect of foodstuffs on the absorption of zinc sulfate. Clin. Pharmacol. Ther. 17, 469–474. doi: 10.1002/cpt1975174469
Plum, L. M., Rink, L., and Haase, H. (2010). The essential toxin: impact of zinc on human health. Int. J. Environ. Res. Public Health 7, 1342–1365. doi: 10.3390/ijerph7041342
Porcheron, G., Garenaux, A., Proulx, J., Sabri, M., and Dozois, C. M. (2013). Iron, copper, zinc, and manganese transport and regulation in pathogenic Enterobacteria: correlations between strains, site of infection and the relative importance of the different metal transport systems for virulence. Front. Cell. Infect. Microbiol. 3:90. doi: 10.3389/fcimb.2013.00090
Potocki, S., Rowinska-Zyrek, M., Witkowska, D., Pyrkosz, M., Szebesczyk, A., Krzywoszynska, K., et al. (2012). Metal transport and homeostasis within the human body: toxicity associated with transport abnormalities. Curr. Med. Chem. 19, 2738–2759. doi: 10.2174/092986712800609698
Prasad, A. S. (2013). Discovery of human zinc deficiency: its impact on human health and disease. Adv. Nutr. 4, 176–190. doi: 10.3945/an.112.003210
Prasad, A. S. (2017). Discovery of zinc for human health and biomarkers of zinc deficiency. Molecular, genetic, and nutritional aspects of major and trace minerals. Adv. Nutr. 4, 176–190. doi: 10.3945/an.112.003210
Prasad, A. S., Beck, F. W. J., Bao, B., Fitzgerald, J. T., Snell, D. C., Steinberg, J. D., et al. (2007). Zinc supplementation decreases incidence of infections in the elderly: effect of zinc on generation of cytokines and oxidative stress. Am. J. Clin. Nutr. 85, 837–844. doi: 10.1093/ajcn/85.3.837
Prasad, A. S., Beck, F. W., Grabowski, S. M., Kaplan, J., and Mathog, R. H. (1997). Zinc deficiency: changes in cytokine production and T-cell subpopulations in patients with head and neck cancer and in noncancer subjects. Proc. Assoc. Am. Phys. 109, 68–77.
Prasad, A. S., Miale, A., Farid, Z., Schulert, A., and Sandstead, H. H. (1963). Zinc metabolism in patients with the syndrome of iron deficiency anemia, hypogonadism and dwarfism. J. Lab. Clin. Med. 61, 537–549.
Pueyo, M., Lopez-Sanchez, J. F., and Rauret, G. (2004). Assessment of CaCl2, NaNO3, and NH4NO3 extraction procedures for the study of cd, cu, Pb and Zn extractability in contaminated soils. Anal. Chim. Acta 504, 217–226. doi: 10.1016/j.aca.2003.10.047
Putila, J. J., and Guo, N. L. (2011). Association of arsenic exposure with lung cancer incidence rates in the United States. PLoS One 6, 1–7. doi: 10.1371/journal.pone.0025886
Qu, C. S., Ma, Z. W., Yang, J., Liu, Y., Bi, J., and Huang, L. (2012). Human exposure pathways of heavy metals in a lead-zinc mining area, Jiangsu province, China. PLOS One 7, 1–11. doi: 10.1371/journal.pone.0046793
Rahman, M. T., and Karim, M. M. (2018). Metallothionein: A potential link in the regulation of zinc in nutritional immunity. Biol. Trace Elem. Res. 182, 1–13. doi: 10.1007/s12011-017-1061-8
Rajagopalan, S., and Long, E. O. (1998). Zinc bound to the killer cell-inhibitory receptor modulates the negative signal in human NK cells. J. Immunol. 161, 1299–1305.
Rajagopalan, S., Winter, C. C., Wagtmann, N., and Long, E. O. (1995). The Ig-related killer cell inhibitory receptor binds zinc and requires zinc for recognition of HLA-C on target cells. J. Immunol. 155, 4143–4146.
Read, S. A., Obeid, S., Ahlenstiel, C., and Ahlenstiel, G. (2019). The role of zinc in antiviral immunity. Adv. Nutr. 10, 696–710. doi: 10.1093/advances/nmz013
Rink, L., and Gabriel, P. (2000). Zinc and the immune system. Proc. Nutr. Soc. 59, 541–552. doi: 10.1017/s0029665100000781
Robinson, N. J., and Pohl, E. (2013). “Zinc sensors in Bacteria,” in Encyclopedia of Metalloproteins. eds. R. H. Kretsinger, V. N. Uversky, and E. A. Permyakov (NY, Springer: New York).
Rodelo, C. G., Salinas, R. A., Armenta JaimeArmenta, E., Armenta, S., Galdámez-Martínez, A., Castillo-Blum, S. E., et al. (2022). Zinc associated nanomaterials and their intervention in emerging respiratory viruses: journey to the field of biomedicine and biomaterials. Coord. Chem. Rev. 457, 214402–214431. doi: 10.1016/j.ccr.2021.214402
Rohrs, L. C. (1957). Metal-fume fever from inhaling zinc oxide. A.M.A. Arch. Ind. Health 16, 42–47. doi: 10.1001/archinte.1957.00260070058005
Roohani, N., Hurrell, R., Kelishadi, R., and Schulin, R. (2013). Zinc and its importance for human health: An integrative review. J. Res. Med. Sci. 18, 144–157.
Rosenkranz, E., Hilgers, R.-D., Uciechowski, P., Petersen, A., Plümäkers, B., and Rink, L. (2017). Zinc enhances the number of regulatory T cells in allergen-stimulated cells from atopic subjects. Eur. J. Nutr. 56, 557–567. doi: 10.1007/s00394-015-1100-1
Rosenkranz, E., Maywald, M., Hilgers, R.-D., Brieger, A., Clarner, T., Kipp, M., et al. (2016). Induction of regulatory T cells in Th1-/Th17-driven experimental autoimmune encephalomyelitis by zinc administration. J. Nutr. Biochem. 29, 116–123. doi: 10.1016/j.jnutbio.2015.11.010
Sabae, S. Z., Hazaa, M., Hallim, S. A., Awny, N. M., and Daboor, S. M. (2006). Bioremediation of Zn+2, cu+2 and Fe+2 using Bacillus subtilis D215 and Pseudomonas putida biovar A D225. Biosci. Res. 3, 189–204.
Saha, A. R., Hadden, E. M., and Hadden, J. W. (1995). Zinc induces thymulin secretion from human thymic epithelial cells in vitro and augments splenocyte and thymocyte responses in vivo. Int. J. Immunopharmacol. 17, 729–733. doi: 10.1016/0192-0561(95)00061-6
Salehizadeh, H., and Shojaosadati, S. A. (2003). Removal of metal ions from aqueous solution by polysaccharide produced from Bacillus firmus. Water Res. 37, 4231–4235. doi: 10.1016/S0043-1354(03)00418-4
Sandstead, H. H. (2013). Human zinc deficiency: discovery to initial translation. Adv. Nutr. 4, 76–81. doi: 10.3945/an.112.003186
Sandström, B., and Cederblad, A. (1980). Zinc absorption from composite meals II. Influence of the main protein source. Am. J. Clin. Nutr. 33, 1778–1783. doi: 10.1093/ajcn/33.8.1778
Sedlakova-Kadukova, J., Kopcakova, A., Gresakova, L., Godany, A., and Pristas, P. (2019). Bioaccumulation and biosorption of zinc by a novel Streptomyces K11 strain isolated from highly alkaline aluminium brown mud disposal site. Ecotoxicol. Environ. Saf. 167, 204–211. doi: 10.1016/j.ecoenv.2018.09.123
Shamim, S., and Rehman, A. (2012). Cadmium resistance and accumulation potential of Klebsiella pneumoniae strain CBL-1 isolated from industrial wastewater. Pak. J. Zool. 44, 203–208.
Shankar, A. H., and Prasad, A. S. (1998). Zinc and immune function: The biological basis of altered resistance to infection. Am. J. Clin. Nutr. 68, 447S–463S. doi: 10.1093/ajcn/68.2.447S
Sharma, P., Kumar, S., and Pandey, A. (2021). Bioremediated techniques for remediation of metal pollutants using metagenomics approaches: A review. J. Environ. Chem. Eng. 9:105684. doi: 10.1016/j.jece.2021.105684
Sharma, S., Tiwari, S., Hasan, A., Saxena, V., and Pandey, L. M. (2018). Recent advances in conventional and contemporary methods for remediation of heavy metal contaminated soils. 3. Biotech 8, 216–234. doi: 10.1007/s13205-018-1237-8
Sheikh, A., Shamsuzzaman, S., Ahmad, S. M., Nasrin, D., Nahar, S., Alam, M. M., et al. (2010). Zinc influences innate immune responses in children with enterotoxigenic Escherichia coli-induced diarrhea. J. Nutr. 140, 1049–1056. doi: 10.3945/jn.109.111492
Shkembi, B., and Huppertz, T. (2021). Influence of dairy products on bioavailability of zinc from other food products: A review of complementarity at a meal level. Nutrients 13, 4253–4263. doi: 10.3390/nu13124253
Silambarasan, S., and Abraham, J. (2013). Biosorption and characterization of metal tolerant bacteria isolated from Palar river basin Vellore. J. Sci. Res. 6, 125–131. doi: 10.3329/jsr.v6i1.14678
Singh, P. P., and Chopra, A. K. (2014). Removal of Zn2+ and Pb2+ using new isolates of Bacillus spp. PPS03 and Bacillus subtilis PPS04 from paper mill effluents using indigenously designed bench-top bioreactor. J. Appl. Nat. Sci. 6, 47–56. doi: 10.31018/jans.v6i1.374
Singh, V. K., Xiong, A., Usgaard, T. R., Chakrabarti, S., Deora, R., Misra, T. K., et al. (1999). ZntR is an autoregulatory protein and negatively regulates the chromosomal zinc resistance operon znt of Staphylococcus aureus. Mol. Microbiol. 33, 200–207. doi: 10.1046/j.1365-2958.1999.01466.x
Skalny, A. V., Aschner, M., Lei, X. G., Gritsenko, V. A., Santamaria, A., Alekseenko, S. I., et al. (2021). Gut microbiota as a mediator of essential and toxic effects of zinc in the intestines and other tissues. Int. J. Mol. Sci. 22, 13074–13090. doi: 10.3390/ijms222313074
Skalny, A. V., Rink, L., Ajsuvakova, O. P., Aschner, M., Gritsenko, V. A., Alekseenko, S. I., et al. (2020). Zinc and respiratory tract infections: perspectives for COVID-19. Int. J. Mol. Med. 46, 17–26. doi: 10.3892/ijmm.2020.4575
Suryawati, B. (2018). Zinc homeostasis mechanism and its role in bacterial virulence capacity. AIP Conf. Proceed. 2021:070021. doi: 10.1063/1.5062819
Taniguchi, J., Hemmi, H., Tanahashi, K., Amano, N., Nakayama, T., and Nishino, T. (2000). Zinc biosorption by a zinc resistant bacterium, Brevibacterium sp. strain HZM-1. Appl. Microbiol. Biotechnol. 54, 581–588. doi: 10.1007/s002530000415
Te Velthuis, A. J. W., van den Worm, S. H. E., Sims, A. C., Baric, R. S., Snijder, E. J., and van Hemert, M. J. (2010). Zn2+ inhibits coronavirus and arterivirus RNA polymerase activity in vitro and zinc ionophores block the replication of these viruses in cell culture. PLoS Pathog. 6, e1001176–e1001110. doi: 10.1371/journal.ppat.1001176
Terrin, G., Boscarino, G., Chiara, M. D., Iacobelli, S., Faccioli, F., Greco, C., et al. (2020). Nutritional intake influences zinc levels in preterm newborns: An observational study. Nutrients 12, 529–538. doi: 10.3390/nu12020529
Timková, I., Sedláková-Kaduková, J., and Pristaš, P. (2018). Biosorption and bioaccumulation abilities of Actinomycetes/Streptomycetes isolated from metal contaminated sites. Separations 5, 54–68. doi: 10.3390/separations5040054
Todd, W. R., Elvehjem, C. A., and Hart, E. B. (1933). Zinc in the nutrition of the rat. Am. J. Phys. 107, 146–156. doi: 10.1152/ajplegacy.1933.107.1.146
Toxicological profile for zinc. (2005). Agency for Toxic Substances and Disease Registry Division of Toxicology and Environmental Medicine GA, USA: Atlanta.
Tran, C. D., Miller, L. V., Krebs, N. F., Lei, S., and Hambidge, K. M. (2004). Zinc absorption as a function of the dose of zinc sulfate in aqueous solution. Am. J. Clin. Nutr. 80, 1570–1573. doi: 10.1093/ajcn/80.6.1570
Upadhyay, A., and Srivastava, S. (2014). Mechanism of zinc resistance in a plant growth promoting Pseudomonas fluorescens strain. World J. Microbiol. Biotechnol. 30, 2273–2282. doi: 10.1007/s11274-014-1648-6
US EPA (2009). National Recommended water Quality Criteria. Washington DC: Office of Water, Office of Science and Technology.
Valencia, E. Y., Braz, V. S., Guzzo, C., and Marques, M. V. (2013). Two RND proteins involved in heavy metal efflux in Caulobacter crescentus belong to separate clusters within Proteobacteria. BMC Microbiol. 13, 79–91. doi: 10.1186/1471-2180-13-79
Vallee, B. L., and Falchuk, K. H. (1993). The biochemical basis of zinc physiology. Physiol. Rev. 73, 79–118. doi: 10.1152/physrev.1993.73.1.79
Vinogradov, A. P. (1959). The Geochemistry of rare and Dispersed Chemical Elements in Soils. New York: Consultants Bureau.
Vogelmeier, C., Konig, G., Bencze, K., and Fruhmann, G. (1987). Pulmonary involvement in zinc fume fever. Chest 92, 946–948. doi: 10.1378/chest.92.5.946
Wadige, C. P. M., Taylor, A. M., Maher, W. A., and Krikowa, F. (2014). Bioavailability and toxicity of zinc from contaminated freshwater sediments: linking exposure-dose-response relationships of the freshwater bivalve Hyridella australis to zinc-spiked sediments. Aquat. Toxicol. 156, 179–190. doi: 10.1016/j.aquatox.2014.08.012
Walker, C. F., and Black, R. E. (2004). Zinc and the risk for infectious disease. Annu. Rev. Nutr. 24, 255–275. doi: 10.1146/annurev.nutr.23.011702.073054
Wang, J., and Chen, C. (2009). Biosorbents for heavy metals removal and their future. Biotechnol. Adv. 27, 195–226.
Wang, X., Sato, T., Xing, B., and Tao, S. (2005). Health risks of heavy metals to the general public in Tianjin, China via consumption of vegetables and fish. Sci. Total Environ. 350, 28–37. doi: 10.1016/j.scitotenv.2004.09.044
Wei, Z., Gu, H., Le, Q. V., Peng, W., Lam, S. S., Yang, Y., et al. (2021). Perspectives on phytoremediation of zinc pollution in air, water and soil. Sustain. Chem. and Pharm. 24:100550. doi: 10.1016/j.scp.2021.100550
Weyh, C., Krüger, K., Peeling, P., and Castell, L. (2022). The role of minerals in the optimal functioning of the immune system. Nutrients 14:4. doi: 10.3390/nu14030644
White, C. L. (1993). Zn in Soils and Plants. Dordrecht, The Netherlands, Kulwer Academic Publishers.
Wierzba, S. (2015). Biosorption of lead(II), zinc (II) and nickel(II) from industrial wastewater by Stenotrophomonas maltophilia and Bacillus subtilis. Pol. J. Chem. Technol. 17, 79–87. doi: 10.1515/pjct-2015-0012
Witkowska, D., Słowik, J., and Chilicka, K. (2021). Heavy metals and human health: possible exposure pathways and the competition for protein binding sites. Molecules 26, 6060–6076. doi: 10.3390/molecules26196060
Wong, C. P., Dashner-Titus, E. J., Alvarez, S. C., Chase, T. T., Hudson, L. G., and Ho, E. (2019). Zinc deficiency and arsenic exposure can act both independently or cooperatively to affect zinc status, oxidative stress, and inflammatory response. Biol. Trace Elem. Res. 191, 370–381. doi: 10.1007/s12011-019-1631-z
Wong, C. P., Rinaldi, N. A., and Ho, E. (2015). Zinc deficiency enhanced inflammatory response by increasing immune cell activation and inducing IL6 promoter demethylation. Mol. Nutr. Food Res. 59, 991–999. doi: 10.1007/s12011-019-1631-z
Wood, R. J., and Zheng, J. J. (1990). Milk consumption and zinc retention in postmenopausal women. J. Nutr. 120, 398–403. doi: 10.1093/jn/120.4.398
Wu, T., Bi, X., Li, Z., Sun, G., Feng, X., Shang, L., et al. (2017). Contaminations, sources, and health risks of trace metal(loid)s in street dust of a small city impacted by artisanal Zn smelting activities. Int. J. Environ. Res. Public Health 14, 961–980. doi: 10.3390/ijerph14090961
Wu, F. C., Feng, C. L., Cao, Y. J., Zhang, R. Q., Li, H. X., and Zhao, X. L. (2011). Study on the toxicity characteristics and water quality of fresh water by zinc. Asian J. Ecotoxicol. 6, 367–382.
Wuana, R. A., and Okieimen, F. E. (2011). Heavy metals in contaminated soils: A review of sources, chemistry, risks and best available strategies for remediation. Int. Sch. Res. Not. 2011, 1–20. doi: 10.5402/2011/402647
Yazar, M., Sarban, S., Kocyigit, A., and Isikan, U. E. (2005). Synovial fluid and plasma selenium, copper, zinc, and iron concentrations in patients with rheumatoid arthritis and osteoarthritis. Biol. Trace Elem. Res. 106, 123–132. doi: 10.1385/BTER:106:2:123
Zahoor, A., and Rehman, A. (2009). Isolation of Cr (VI) reducing bacteria from industrial effluents and their potential use in bioremediation of chromium containing wastewater. J. Environ. Sci. 21, 814–820. doi: 10.1016/S1001-0742(08)62346-3
Zaichick, V., Sviridova, T. V., and Zaichick, S. V. (1997). Zinc in the human prostate gland: normal, hyperplastic and cancerous. Int. Urol. Nephrol. 29, 565–574. doi: 10.1007/BF02552202
Keywords: zinc, bioremediation, pollution, wastewater, heavy metal, toxicity
Citation: Hussain S, Khan M, Sheikh TMM, Mumtaz MZ, Chohan TA, Shamim S and Liu Y (2022) Zinc Essentiality, Toxicity, and Its Bacterial Bioremediation: A Comprehensive Insight. Front. Microbiol. 13:900740. doi: 10.3389/fmicb.2022.900740
Received: 21 March 2022; Accepted: 09 May 2022;
Published: 31 May 2022.
Edited by:
Maqshoof Ahmad, The Islamia University of Bahawalpur, PakistanReviewed by:
Ajay Kumar, Agricultural Research Organization (ARO), IsraelCopyright © 2022 Hussain, Khan, Sheikh, Mumtaz, Chohan, Shamim and Liu. This is an open-access article distributed under the terms of the Creative Commons Attribution License (CC BY). The use, distribution or reproduction in other forums is permitted, provided the original author(s) and the copyright owner(s) are credited and that the original publication in this journal is cited, in accordance with accepted academic practice. No use, distribution or reproduction is permitted which does not comply with these terms.
*Correspondence: Taha Majid Mahmood Sheikh, dGFoYW1hamlkMTcwNUB5YWhvby5jb20=; Saba Shamim, c2FiYXNoYW1pbWdlbmV0aWNzQGdtYWlsLmNvbQ==; Yuhong Liu, eWhsaXVAaGh1LmVkdS5jbg==
†These authors have contributed equally to this work
Disclaimer: All claims expressed in this article are solely those of the authors and do not necessarily represent those of their affiliated organizations, or those of the publisher, the editors and the reviewers. Any product that may be evaluated in this article or claim that may be made by its manufacturer is not guaranteed or endorsed by the publisher.
Research integrity at Frontiers
Learn more about the work of our research integrity team to safeguard the quality of each article we publish.