- 1Guangxi Key Laboratory for Polysaccharide Materials and Modifications, School of Marine Sciences and Biotechnology, Guangxi Minzu University, Nanning, China
- 2Department of Environmental Science, Veer Bahadur Singh Purvanchal University, Jaunpur, India
- 3Guangxi Key Laboratory of Sugarcane Genetic Improvement, Sugarcane Research Institute, Guangxi Academy of Agricultural Sciences, Nanning, China
- 4Department of Plant Physiology, Institute of Agricultural Sciences, Banaras Hindu University, Varanasi, India
- 5Department of Molecular Biology and Biotechnology, Maharana Pratap University of Agriculture and Technology, Udaipur, India
Plant growth performance under a stressful environment, notably in the agriculture field, is directly correlated with the rapid growth of the human population, which triggers the pressure on crop productivity. Plants perceived many stresses owing to degraded land, which induces low plant productivity and, therefore, becomes a foremost concern for the future to face a situation of food scarcity. Land degradation is a very notable environmental issue at the local, regional, and global levels for agriculture. Land degradation generates global problems such as drought desertification, heavy metal contamination, and soil salinity, which pose challenges to achieving many UN Sustainable Development goals. The plant itself has a varied algorithm for the mitigation of stresses arising due to degraded land; the rhizospheric system of the plant has diverse modes and efficient mechanisms to cope with stress by numerous root-associated microbes. The suitable root-associated microbes and components of root exudate interplay against stress and build adaptation against stress-mediated mechanisms. The problem of iron-deficient soil is rising owing to increasing degraded land across the globe, which hampers plant growth productivity. Therefore, in the context to tackle these issues, the present review aims to identify plant-stress status owing to iron-deficient soil and its probable eco-friendly solution. Siderophores are well-recognized iron-chelating agents produced by numerous microbes and are associated with the rhizosphere. These siderophore-producing microbes are eco-friendly and sustainable agents, which may be managing plant stresses in the degraded land. The review also focuses on the molecular mechanisms of siderophores and their chemistry, cross-talk between plant root and siderophores-producing microbes to combat plant stress, and the utilization of siderophores in plant growth on degraded land.
Introduction
The adequacy of the agricultural soil deteriorates owing to exposures to adverse environmental conditions such as salinity, drought, heavy metal stress, etc., which induces plant stress and reduces plant growth productivity which will trigger food scarcity in the future. The nutrient discrepancy in the plant is a common occurrence found in degraded land, and among different plant nutrients, iron is the essential ingredient for plant growth (Connorton et al., 2017). Rapid industrialization, urbanization, unsuitable land use (IPBES, 2018), fast agricultural practices (Keesstra et al., 2018), soil salinization (Edrisi et al., 2021), soil erosion (Paul et al., 2020a,b), invasion of alien species (Rai, 2021), poor governance management strategy (Gerber et al., 2014), overexploitation of natural resources, excessive mining (Upadhyay and Edrisi, 2021; Shakeel et al., 2022), etc., degrade more than 33% of global land resources through direct and indirect approaches (IPBES, 2018; Srinivasarao et al., 2021).
Iron deficiency is a communally observed phenomenon in CaCO3 (calcium carbonate)-rich desert soil at high pH (Alhendawi et al., 1997). The availability of iron in soil mostly depends on the range of pH, and the character trait of saline (pH 7.2–8.5) and alkaline (pH > 8.5) soil (Upadhyay and Chauhan, 2022) showed iron deficiency due to less solubility of iron at high pH (Mann et al., 2017). Flood and raised concentration of nitrates and phosphates (exogenous use of synthetic fertilizers) in soil reduces iron solubility, alters iron translocation, and induces iron deficiency in the plant (Becker and Asch, 2005).
The plant growth reduction mediated stress due to nutrient imbalance in degraded soil is a common phenomenon; the increase of degraded soil due to salinity, drought, heavy metal, etc. are reported by several workers (Ma et al., 2020; Upadhyay et al., 2021). Out of numerous detrimental factors, the lack of available iron for the plant is one of the major factors (Liliane and Charles, 2020). Several pieces of research demonstrated that plant growth-promoting rhizobacteria (PGPR) may be a promising tool for mitigating the adverse effect of degraded lands; for instance in saline soil (Upadhyay et al., 2009, 2011; Upadhyay and Singh, 2015) drought conditions (Igiehon et al., 2019), and heavy metal conditions (Bhojiya et al., 2022). Positive association between rhizosphere and microbes play a crucial role under iron-stressed degraded land owing to the secretion of iron-chelating compounds i.e., siderophore (Dertz et al., 2006). Plant root secretes siderophore to maintain the iron level for their metabolic and physiological activities in iron-stressed degraded soil, but is not attained at the perfect level (Herlihy et al., 2020). On other hand, siderophore-producing microbes (SPM) produce numerous iron-chelating compounds, which can cut short plant stress under iron-stressed soil. Siderophore-producing microbes produce siderophore and have activities of biofertilizers and bio-control for the plant; thus SPM acts as a signature for sustainable agriculture and is eco-friendly for crop production in degraded land Table 1 (Alam, 2014). Siderophore-producing microbes reduce Fe deficiency and enhance all physiological and biochemical processes of the plant under saline soil (Sultana et al., 2021), drought conditions (Kumar et al., 2016), and heavy metal-stressed soil (Hofmann et al., 2021). Siderophore also changes the oxidation states of heavy metals such as Cd, Cu, Ni, Pb, Zn, Th, U, and Pu and makes them less toxic (Schalk et al., 2011). Siderophore has a strong affinity for iron-chelating compounds, induces a bioremediation process, and enhances nutrient uptake and plant growth (Rajkumar et al., 2010). A bacterial strain like Pseudomonas fluorescence produces pyoverdines siderophore that increases mobility and reduces the toxicity of heavy metals under uranium mines (Edberg et al., 2010). Sharma and Johri (2003) isolated Pseudomonas from rhizospheric soil of Zea mays L., which produces a siderophore that showed a high affinity to chelate of Fe3+ ions. Ahmed and Holmstrom (2014) and Huo et al. (2021) reported that the use of SPM is a suitable approach for reducing plant stress on degraded soil. Bioavailability of iron reduces the saline soil condition which leads to iron deficiency in a plant, and thus the plant faces both salinity stress and iron deficiency (Sultana et al., 2021). To combat iron deficiency under saline conditions, Sultana et al. (2021) isolated four salt-tolerant plant-growth promoting bacteria from rice rhizosphere, Bacillus aryabhattai MS3, which showed maximum siderophore producing ability at 200 mM NaCl concentration than the control. The siderophore-producing ability of B. aryabhattai MS3 increased due to the activation of entD gene by salinity, and entD gene has to be responsible for siderophore biosynthesis (Sultana et al., 2021). Streptomyces tendae F4 reduces cadmium translocation from rhizosphere to plant in heavy metal polluted soil (Dimkpa et al., 2009). Similarly, Sadeghi et al. (2012) observed that the isolate C (Streptomyces) increased siderophore production in the presence of a high concentration of NaCl (300 mM), and also produced auxin, solubilized tricalcium phosphate. Inoculation of isolate C (Streptomyces) increased iron content in the shoot of wheat plants in saline soil (Sadeghi et al., 2012). Therefore, in this context, the present article aims to provide recent updates on plant mechanisms under iron-stressed degraded soil, nexus between plants siderophores and siderophore producing bacteria, and developing sustainable use of siderophore-producing bacteria for plant growth under degraded soil.
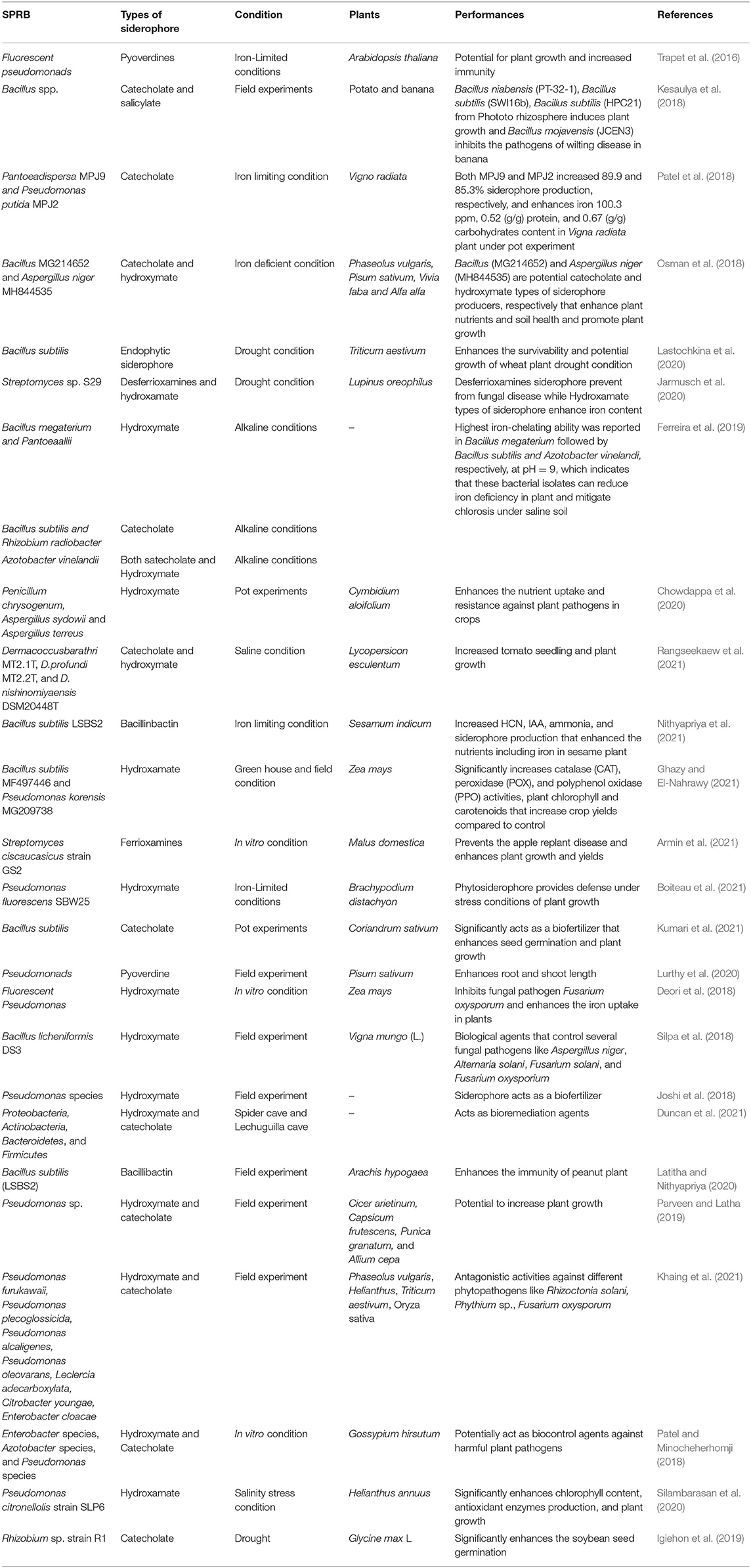
Table 1. Recent studies (2016–2021) showing the main effects on plants exerted by siderophore-producing rhizobacteria alone or in combination in degraded soil conditions.
Plant Stress Under Iron Deficient Degraded Land
Soil degradation is a natural and anthropogenic phenomenon that reduces soil nutrients (Abiala et al., 2018; Upadhyay and Chauhan, 2019; Bhojiya et al., 2022; Shakeel et al., 2022), mediated by soil salinization (Qadir et al., 2014; Machado and Serralheiro, 2017; Abiala et al., 2018; Upadhyay and Chauhan, 2022), drought (Bartels and Sunkar, 2005), and heavy metals contamination (Paul et al., 2020b,c). The occurrence of an available form of Fe lacks in almost all types of soil (neutral, acidic, and alkaline) due to several factors such as soil pH, deposition of CaCO3, saline and desert conditions, etc. (Alhendawi et al., 1997). Degraded soil adversely impacts the growth and output of plants through an imbalance of many nutrients and metabolic pathways (Figure 1) and induces the unfavorable fitness of soil for plant growth. Despite several detrimental factors of degraded soil, the present review discusses iron homeostasis and its possible ability to meet plant sustainability. Iron deficiency hinders several metabolic and physiological aspects in plants and human beings. The crucial role of iron has been well-acknowledged for several redox reactions of different physiological mechanisms of plants like respiration- and photosynthesis-mediated electron transport systems. Iron also participates in several enzymatic activities such as peroxidase, catalase, cytochrome, oxidase, etc. (Tripathi et al., 2018). Also, Fe plays the role of a co-factor in the synthesis of many plant hormones like ethylene and ACC deaminase (Siedow, 1991). Iron plays a crucial role in chlorophyll biosynthesis by maintaining electron flow in CO2 fixation through (PS)-II-b6f/Rieske (PS)-I complex (Ermakova et al., 2019). Iron plays a remarkable co-factor in the electron transport chain of plant photo-system. In photosystem (PS)-I , iron is required to form three 4Fe-4S in clusters, Cytochrome-b6f (Cyt-b6f) requires iron for Rieske subunits as a cluster of 2Fe-2S (Fukuyama et al., 1980; Hurt and Hauska, 1981), and photosystem (PS)-II requires iron as a cofactor for cytochrome (Ben-Shem et al., 2003). Iron is essential for leghemoglobin and nitrogen-fixing machinery in the leguminous plant (Brear et al., 2013). The deficiency of Fe leads to several disorders in the plant by altering the redox and enzymatic reactions and shows primarily a symptom of wilting and chlorosis (Bashir et al., 2016), which leads to a lowering of plant growth and productivity (Figure 1). Several researchers reported that root growth of the plant is hindered under Fe deficient soil (Satbhai et al., 2017), altering the function of the gene responsible for iron uptake (Colangelo and Guerinot, 2004). Iron stress also triggers a plant's reactive oxygen species-mediated Fenton reaction (Tewari et al., 2013; Dumanovic et al., 2021). The Fenton reaction elaborates the interplay of Fe2+ and H2O2 (hydrogen peroxide) to generate hydroxyl radical (OH*), which is one of the reactive oxygen species (Kar and Chattopadhyaya, 2017).
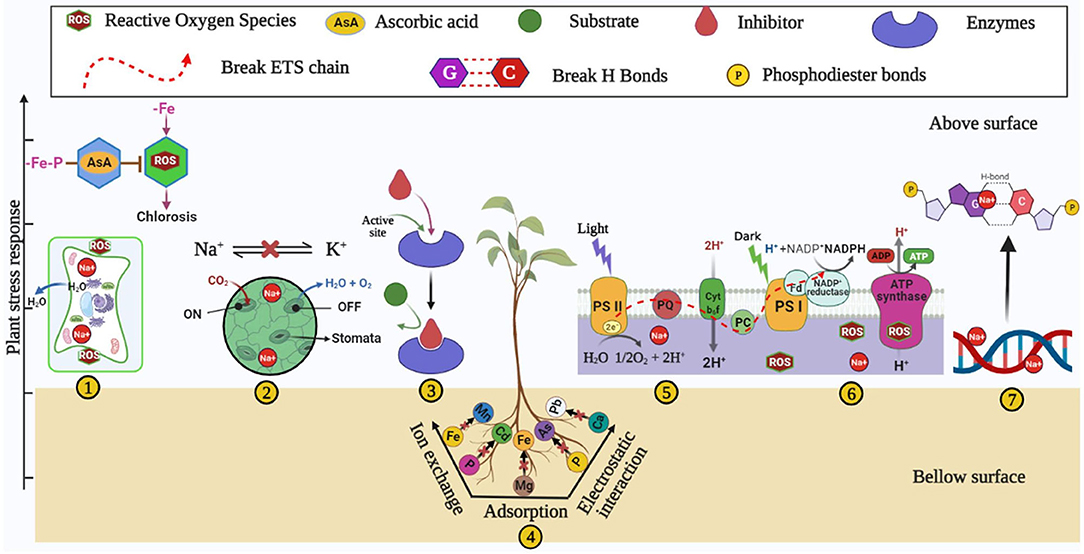
Figure 1. Plant stress responses such as (1) wilting and chlorosis, (2) altered stomatal activities, (3) inhibition of enzymatic activities (4) nutrient imbalance (5) increased ROS, (6) altered electron transport system, and (7) DNA damage under iron-deficient/degraded soil.
Iron stress also leads to necrosis in tissue, blackening of roots, and an overall decrease in plant growth (Rai et al., 2021). Degraded soil due to salinity increases ionic and osmotic stress and reduces plant growth and productivity (Upadhyay et al., 2012; Orozco-Mosqueda et al., 2020). Ionic stress induces the influx of Na+ ions, resulting in the efflux of K+ ions in soil (Yang et al., 2009; Orozco-Mosqueda et al., 2020), while osmotic stress accumulates the NaCl concentration in the rhizospheric soil (Egamberdieva et al., 2019). Soil salinity induces nutrient imbalance (Upadhyay and Chauhan, 2022) and iron deficiency (Rabhi et al., 2007; Sultana et al., 2021). Both iron and NaCl stresses induce reactive oxygen species (ROS), which directly causes injury to the plant tissue (Rabhi et al., 2007; Jha and Subramanian, 2020; Kamran et al., 2020), and salinity damages the base and cross-correlation of double-stranded DNA (Santoyo and Strathern, 2008; Orozco-Mosqueda et al., 2020). More salinity and iron deficiency affect the morphological traits such as a decrease in root length, plant size, variety of leaves, flowering of plants (Rabhi et al., 2007; Kapoor and Srivastava, 2010; Mallahi et al., 2018), decrease in the plant's pigment chlorophyll content, resulting in reduced photosynthesis (Ashraf et al., 2017); hence poor plant growth reduces the crop productivity (Palaniyandi et al., 2014; Machado and Serralheiro, 2017).
Rapid changes in climatic conditions alter the cycle of atmospheric rain, precipitation, and biogeochemical cycle, leading to an increase in Fe deficient soil and degraded land, developing water-deficit soil environment, etc. (Morrissey and Guerinot, 2009; Lal, 2012; Fuentes et al., 2018; Sileshi et al., 2020). Therefore, drought stress is noticed at a global level (Takahashi et al., 2020), and a substantial decrease in plant growth and productivity has been observed under drought stress-mediated iron-deficient soil (Tripathi et al., 2018). Heavy metals are found in degraded land, which poses hazardous environmental stress that arises both naturally and anthropogenically (Wasi et al., 2012; Bernard et al., 2018). An increase in the concentration of heavy metals in the soil creates various problems for flora and fauna (Alengebawy et al., 2021). Leskova et al. (2017) reported that Fe deficiency is a common phenomenon in soil contaminated with heavy metals. In the purview to tackle these issues, it is, therefore, necessary to develop a sustainable approach that improves plant growth and productivity under iron-stressed/degraded soil. The following section of this review discusses the possible application of siderophores-producing bacteria for plant growth under iron-deficient soil.
Siderophore-Producing Bacteria
Siderophore-producing rhizobacteria that promote plant growth were demonstrated by several researchers, for example, Bacillus subtilis, B. licheniformis, B. coagulanse, B. circulance, Pseudomonas koreensis, P. fluroscence (Ghazy and El-Nahrawy, 2021), P. aeruginosa (Subramanium and Sundaram, 2020; Singh et al., 2021a), Pseudoalteromonas tetraodonis, Bacillus cereus, Psychrobacter pocilloporae, Micrococcus, aloeverae, Pseudomonas weihenstephanensis (Sinha et al., 2019), Pseudomonas sp. (Singh et al., 2022), Enterobacter genera, Bacillus, and Rhodococcus (Sah and Singh, 2015), Bacillus megaterium (Singh et al., 2020a), Pantoae cypripedii (Singh et al., 2021b), Kosakonia radicincitans (Singh et al., 2020b), and Pantoae dispersa (Singh et al., 2021c).
The environmental conditions such as pH, temperature, nutrient sources, aerobic/anaerobic, etc. influence the production of bacterial siderephores. Sinha et al. (2019) isolated Enterococcus casseliflavus and Psychrobacter piscatorii from Kerguelen Islands, and P. astetraodonis, B. cereus, P. pocilloporae, Micrococcus aloeverae, and P. weihenstephanesis were isolated from Prydz Bay. Isolates from Prydz Bay-produced either hydroxamate or catecholate types of siderophores at 15–25°C and 8.5 pH. Pseudomonas fluorescens synthesizes pyoverdine type of siderophores that enrich the ferric iron as nutrients in Solanum lycopersicum plants enhancing photosynthetic pigments and biomass of the plant (Nagata et al., 2013); B. subtilis produces hexadentate triscatecholamide bacillibactin which has an affinity to chelates the iron (Dertz et al., 2006); P. aeruginosa and P. fluorescens are capable of producing siderophore that increases the rate of phytoextraction and phytoremediation of heavy metals (Braud et al., 2009a). Essen et al. (2007) reported that Pseudomonas strtzeri 36,651 produces ferrioxamine type of siderophore under both aerobic and anaerobic conditions, and non-sulfur bacterium Rhodopseudomonas palustris str. CGA009 produces two types of siderophore rhodopertobactin under both aerobic and anaerobic conditions (Baars et al., 2018).
Siderophores: Chemistry and Mechanism
Siderophores facilitate several functions of plants such as respiration (Aznar and Dellagi, 2015), photosynthesis (Nagata et al., 2013), bioremediation (Saha et al., 2016), plant growth promotion (Yadav et al., 2011; Ghazy and El-Nahrawy, 2021), and phytoremediation of heavy metals (Kong and Glick, 2017; Leguizamo et al., 2017; Ustiatik et al., 2021). Siderophores are also produced by non-ribosomal peptides bonds (Hu and Xu, 2011) and multidentate iron-chelating compounds that solubilize and chelate organic and inorganic forms of compounds in soil (Singh et al., 2017). The term is derived from the Greek words sidero meaning “iron” and phore meaning “carriers” or iron-bearing compounds that uptake insoluble iron from different environmental sources (Nagoba and Vedpathak, 2011). Primarily siderophore-producing bacteria release iron-binding proteins, such as permeases and ATPases, that chelate the ferric iron (Fe3+) and transport Fe3+ ions in the cell membrane in gram-positive bacteria (Ahmed and Holmstrom, 2014). Gram-negative bacteria have a complex mechanism for the transportation of ferric iron (Fe3+) mediated by many enzymes, periplasmic binding proteins, outer membrane receptors, and cytoplasmic membrane proteins which make Fe3+ available for plant cells (Ahmed and Holmstrom, 2014; Schutze et al., 2015).
Siderophores are classified based on many criteria such as the source of siderophore, cyclic and linear structure of siderophore, and the chemical nature of functional groups of the siderophore, as shown in Figure 2. On the basis of functional groups, siderophores are classified as hydroxamate-type siderophore, catecholate-type siderophore, carboxyalate-type siderophore, and mixed ligand siderophore (Ito and Butler, 2005; Zawadzka et al., 2006; Butler and Theisen, 2010). Hydroxamate siderophores are a group of C(=O) N-(OH)R, where R is either amino acid or a derivative of amino acids, which contains two oxygen molecules to form bidentate ligand with iron ions; therefore, each siderophore is able to form hexadentate ligands, octahedral complex compounds with Fe3+ ions at a different range between 1,022 and 1,032 M−1 (Winkelmann, 2007). During the combination of hydroxamate with Fe3+ ions, hydroxamate functional group loses a proton from the hydroxylamine (-NOH) group to form a bidentate ligand (Fiestner et al., 1993).
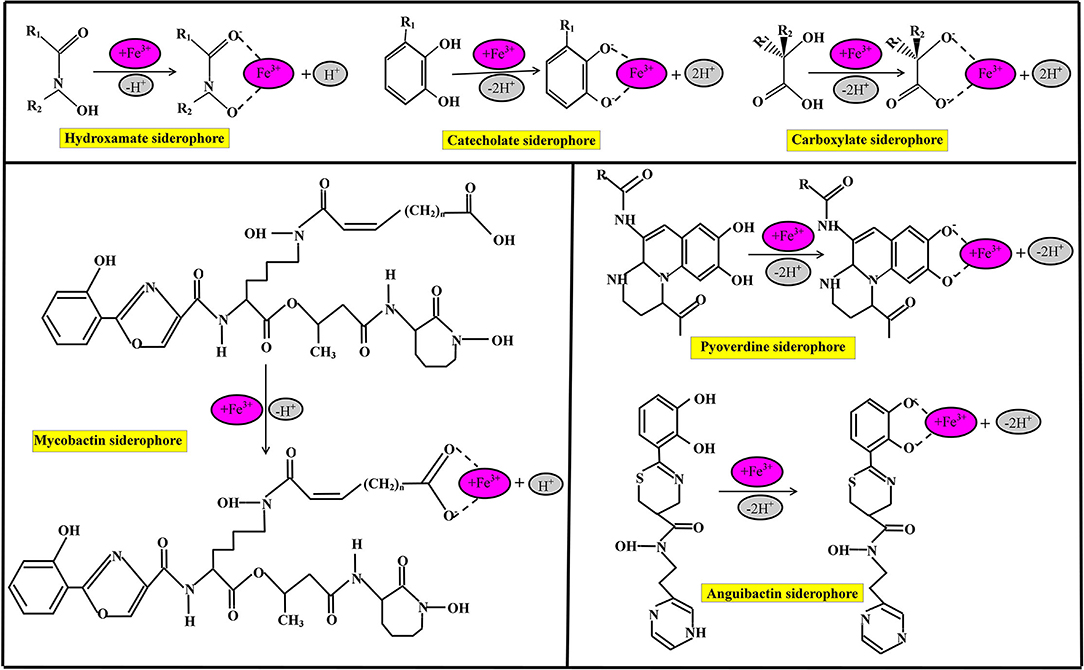
Figure 2. Chemical nature of commonly identified siderophores and their iron (Fe3+) chelating binding sites.
Some bacterial species have the potential to hydroxamate siderophore production, including P. aeruginosa, which is able to produce pyoverdin hydroxamate type of siderophore under limited iron conditions (Meneely and Lamb, 2007). Catecholate, commonly known as phenolate (2, 3–dihydroxy benzoate) siderophore is an orthoisomer of three molecules of isomeric benzenediols (Sah and Singh, 2015). The functional group of catecholate siderophore loses two protons and forms a five-member ring structure with Fe (Kraemer, 2004). Bacterial species are the most dominant species for catecholate types of siderophore production (Dave et al., 2006). The common bacterial species are Escherichia coli, Salmonella typhimurium, and Klebsiella pnemoniae which dominantly produce enterochelin subtypes of catecholate types of siderophore production (Dertz et al., 2006). The bacterial species Azotobacter vinelandii is the source of various types of catecholate siderophores such as monocatecholate aminochelin, dicatecholate azotochelin, and tricatecholate protochelin under iron-limiting conditions (Wittmann et al., 2001).
Carboxyalate-type siderophore is a unique class of siderophore, which bears hydroxyl and carboxyl compounds (Dave and Dube, 2000); carboxyalate-type siderophore is neither related to hydroxamate nor phenolate ligands. Bacterial species such as Staphylococci, Rhizobium meliloti, and Mucorals are the sources of Staphyloferrin A and B, rhizobactin, and rhizoferrin carboxylate siderophores, respectively. Many siderophoral species such as lysine derivative, ornithine derivative, and histidine derivative contain mixed ligands with Fe3+ ions. Mycobactins are lysine derivative siderophores that bear 2-hydroxy phenyl oxazoline compounds which recover iron. Mycobactin siderophore is produced by Mycobacteria bacterial species, therefore, called mycobactin, which consists of two hydroxamate, one phenolate, and another oxazoline nitrogen. Pyoverdine is a dihydroxyquinoline compound, and structurally every pyoverdine siderophore differs from each other, while chromophore (1S)-5-amino-2,3-hydro-8,9-dihydro-8,9-dihydroxy-1H-pyrimido[1,2-a] quinoline-1 carboxylic acid shows similarities with azobactin that secretes by A. vinelandii. Pyoverdines and pseudobactins are isolated by pseudomonas bacterial species and are applicable in agriculture sectors and as human pathogens (Kloepper et al., 1980). The anguibactin siderophore is a histamine derivative mixed ligands siderophore; structurally anguibactin siderophore is a ω-N-hydroxy-ω-[[2′-(2",3"-dihydroxyphenyl) thiazolin-4′-yl]-carboxy] histamine. The anguibactin siderophore is applicable in living cells as an inducer for iron uptake.
Action and Strategies of Siderophores
Several microbes such as bacteria, fungi, algae, and dicotyledonous plants (Das et al., 2007) are involved in siderophoral activities that solubilize Fe3+ ions in the simple form and which are transported through specific receptos proteins in cells (Diaz de Villegas, 2007). This mechanism involves the reduction of a complex form of iron (Fe3+) to a simple form of iron (Fe2+) (Butler and Martin, 2005; Hopkinson and Morel, 2009). The transport systems of Fe-siderophore in Gram-negative and Gram-positive bacteria are different, the outer membrane transporters are broadly absent in Gram-positive bacteria, while they are found in Gram-negative bacteria and play an impressive role in the transport of Fe-siderophore. In Gram-negative bacteria, the Fe-siderophore passes on to the periplasmic binding protein-mediated TonB-ExbBD complex (Ferguson and Deisenhofer, 2002; Koebnik, 2005), and the bound Fe-siderophore with surface-binding-proteins are then imported into the cytoplasm via the possible siderophore-permease-ATPase system. The role of the surface periplasmic binding protein, ATPase, and permeases in Gram-positive bacteria is similar as in Gram-negative bacteria mediated by periplasmic surface binding protein permease with the ATP system (Fukushima et al., 2013). The movement of siderophore across the bacterial cell membrane owing to chemiosmotic potential is mediated by a complex of three membrane-spanning proteins (TonB, ExbD, and ExbB; Ferguson and Deisenhofer, 2002). TonB-dependent outer membrane receptors are involved in the adhesion of Fe3+ siderophore complexes on the bacterial cell surface (Schalk et al., 2012). Then Fe3+ siderophore complex is transported from outside of the outer membrane to the cell through the outer membrane of a bacterial cell by energy-dependent system and reaches the periplasm (Schalk et al., 2012). Afterward, Fe3+ siderophore complex ions bind with periplasmic binding protein (PBP) (Noinaj et al., 2010; Ribeiro and Simoe, 2019; Figure 3). Iron (Fe3+) siderophore complex is transported from the periplasm to the cytoplasm across the inner membrane by ATP binding cassette system and reaches the cytoplasm due to the reduction in Fe3+ ions to form Fe2+ ions. With this process being repeated in the bacterial cell, Fe+2 ions are directly absorbed by the rhizosphere of plants that promote the growth of plants (Ahmed and Holmstrom, 2014). In the case of gram-positive bacteria, due to the lack of an outer membrane all the processes occur in the periplasm and cytoplasm, Fe3+ siderophore complex ions adhere to the surface of periplasm, and the establishment of Fe2+ ions occurs in the cytoplasm (Faraldo-Gomez and Sansom, 2003; Fukushima et al., 2013; Schalk and Guillon, 2013; Ribeiro and Simoe, 2019; Figure 3).
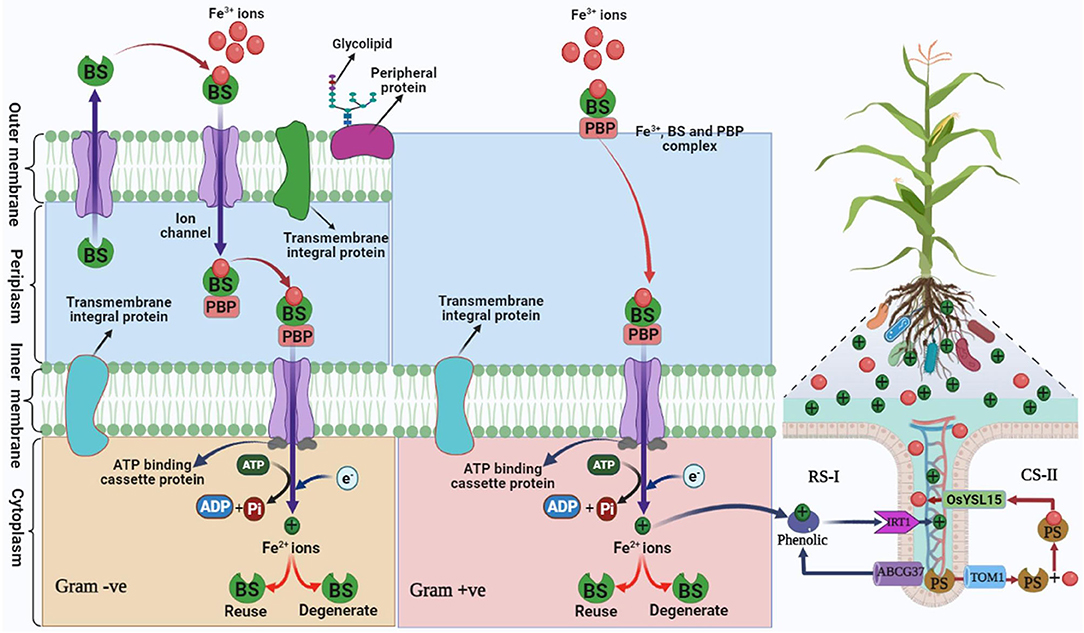
Figure 3. Mechanism of siderophore in plant growth-promoting gram-positive and gram-negative bacteria mediating iron uptake in plants under iron-deficient/degraded soil. Bacterial siderophore (BS), periplasmic binding protein (PBP), reduction strategy (RS-I), chelation strategy (CS-II), and plant siderophore (PS). Iron regulated transporter 1 (IRT), Yellow Stripe-Like Transporter of Oryza sativa (OsYSL15), ATP-binding cassette transporter (ABC) G37, translocase of outer membrane 1 (TOM) (Modified as sources of Fukushima et al., 2013; Seyoum et al., 2021).
Two strategies have been reported in the rhizospheric region to maintain iron uptake mediated by siderophore under iron-deficient soil. The first is a reduction strategy (RS-I), and second, a chelation strategy (CS-II) (Rai et al., 2021; Figure 3). Among both strategies, chelation strategies are common under stress conditions and tolerate a change in pH as compared to reduction strategy (Ahmed and Holmstrom, 2014), while in rice plants, both the strategies are reported (Krohling et al., 2016). RS-I is common in non-grass plants under low iron conditions in the rhizosphere where H+-ATPase AHA2 releases H+ and reduces the pH of the soil and induces the solubility of Fe3+. Iron once in apoplast gets chelated by phenolic compounds of the coumarin family and is transported by transporter ABCG37 (Mladenka et al., 2010). Ferric chelate reductase (Ferric Reduction Oxidase-2) reduces Fe3+-Fe2+ in the plasma membrane (Ahmed and Holmstrom, 2014). IRT1 (Iron Regulator Transporter-1) transports Fe2+ in the epidermal cell of plant root (Barberon et al., 2014).
Microorganisms such as bacteria/fungi and grasses follow the mechanism of CS-II. This strategy is commonly found in alkaline soil where acidification of rhizosphere is too difficult, thus bacteria are remarkable agents for their application in alkaline soil as well as stressed soil. This strategy (CS-II) is based on biosynthesis, secretion of siderophore such as phytosiderophore (PS)/bacterial siderophore (BS) that chelates Fe3+ and form Fe3+-BS/Fe3+-PS complex and transported through YS/YSL (Yellow Stripe/Yellow Stripe-Like) and TOM1 transporter family to the root (Dai et al., 2018).
Genetic Mechanisms and Regulation of Siderophores
The key enzyme “non-ribosomal cytoplasmic synthase” produces siderophore by utilizing the precursors such as citrate, amino acids, dihydroxybenzoate, and N5-acyl-N5-hydroxyornithine, and their genes have been identified in several microorganisms (Paul et al., 2014; Paul and Dubey, 2015). In microbes such as bacteria, Aspergillus fumigates, yeast siderophore operon consists of several genes namely sidA, sidD, sidG, sidF, sidC and sidL which are located on different chromosomes (Blatzer et al., 2011; Khan et al., 2018; El-Maraghy et al., 2020).
The sidC gene, highly conserved among fungi, is characterized as non-ribosomal peptide synthetase (NRPS) and required for the biosynthesis of both ferricrocin (FC) and hydroxyferricrocin (HFC) (Schrettl et al., 2007). The sidF and sidG genes are characterized as acetyl transferase having the role of TAFC biosynthesis. The sidA gene encodes an L-ornithine N5-monooxygenase which initiates siderophore production (Seifert et al., 2008) whereas sidL gene which is located in cytoplasm and is a constitutively active N5-hydroxyornithine-acetylase required for FC biosynthesis (Blatzer et al., 2011). The siderophores uptake is facilitated by siderophore uptake genes i.e., sit1, mirB and mirC (Silva-Bailao et al., 2014). In contrast to fungi, the biosynthesis of different siderophores in bacteria has been governed by different genes such as entB gene (enterobactin biosynthesis), iroB gene (salmochelin biosynthesis), entS gene (enterobactin synthesis) (Watts et al., 2011; Paul and Dubey, 2015). In E. coli, the enterobactin synthesis operon consists of entCDEBAH genes whereas, for enterobactin uptake and utilization, fepA, fepB, fepC, fepD, fepE, fepG, fes, and entS genes are responsible (Peralta et al., 2016). In the gram-negative Yersinia pestis bacterium, the siderophore type yersiniabactin is synthesized by irp1 and irp2 genes (Guilvout et al., 1993). Etchegaray et al. (2004) reported that siderophores in Xanthomonas species are synthesized by non-ribosomal peptide synthetase from a precursor such as polyamine derivatives. Najimi et al. (2008) identified asbG, asbF, asbD, asbC, asbB, and asbI genes encoding proteins similar to components of the siderophore biosynthetic machinery in Aeromonas salmonicida bacteria. In P. aeruginosa, the siderophore pyochelin is synthesized by the genes pchDCBA and pchEF, and pyochelin precursors such as salicylate and dihydroaeruginoate (Dha), are clustered with the pyochelin regulatory genes pchR on its genome (Reimmann et al., 2001). Searle et al. (2015) developed multiple primers to screen environment samples for the presence of different microbial siderophores such as Enterobactin (entA, entB, entC, entE, fepA genes), Salmochelin (iroB, iroC, iroD, iroE, iron genes), Yersiniabactin (irp1, irp2, irp3, irp4, & 5, fyuA genes) and Aerobactin (iucA, iucB, iucC, iucD, iutA genes). Hofmann et al. (2020) reported that the gene grdesA from Gordoniarub ripertincta CWB2 and psdes A from Pimelobacter simplex VkMAC-2033D encodes lysine decarboxylases presumed to be involved in the synthesis of desferrioxamine siderophores. Wang et al. (2021) identified a novel Non-ribosomal Peptide Synthetase (NRPS) cluster in the bacteria Burkholderia seminalis strain R456 which is responsible for the production of a novel undescribed siderophore, along with previously reported ornibactin and pyochelin type siderophores, and also it is a crucial component in regulator protein Fur which regulates siderophore production.
Sustainable Application of SPM For Plant Growth in Iron Deficient Degraded Land
Siderophore-producing microbes reduce the Fe deficiency and enhance all physiological and biochemical processes of crops in saline soil (Table 1). Siderophore-producing microbes B. aryabhattai MS3 are the most applicable in rice plants that enhance 60 and 43% of crop production under non-saline and saline (200 mM NaCl) conditions, respectively (Sultana et al., 2021). Siderophore-producing microbe B. subtilis DR2 act as a biofertilizer and promotes seed germination and plant growth in Coriandrum sativum (Kumari et al., 2021). Rangseekaew et al. (2021) reported that a specific bacterial strain of deep-sea Dermacoccus barathri MT2.1T and D. profundi MT2.2T strain have the ability to promote seedling in tomato plants under 150 mM concentration of NaCl as compared to the terrestrial strain D. nishinomiyaensis DSM20448T, due to the production of many plant-growth promoting attributes such as siderophore production, indole-3-acetic acid, and phosphate solubilization. Nadeem et al. (2012) reported that rhizospheric bacterial species Variovorax paradoxus (JN858091), P. fluorescens (JN858088), and B. megeterium (JN858098) have potential PGP attributes such as siderophore production, phosphate solubilization, exopolysaccharides production, indole acetic acid production, and ACC deaminase activity under both saline and normal conditions that alleviate the negative impacts of salinity and enhance the nutrients uptake for plant growth in cucumber plants.
Siderophore-producing microbes can produce plant growth-promoting attributes such as plant hormones, phosphate solubilization, secondary metabolites, etc., and provide suitable environments in stressed soil that enhances plant growth such as drought (Vivas et al., 2003; Breitkreuz et al., 2021). B. subtilis produce iron-chelating compounds that enhance the nutrient level in soil resulting in the growth of wheat plants under drought conditions (Lastochkina et al., 2020). Two siderophore-producing rhizobacterial species such as P. putida and B. amyloliquefaciens have the tolerance ability under drought stress due to the secretion of PGP attributes like siderophore production, hormone production, mineral solubilization, biofilm formation, and ACC deaminase activity, ameliorating the negative effects of drought and ensuring potential growth of Cicer arietinum L. under drought stress (Kumar et al., 2016). Several plant growth microbes survive under drought stress enhancing plant growth and yields; the inoculation of Bacillus sp. in lettuce increases the nitrogen, phosphorous, and potassium nutrients under drought stress conditions (Vivas et al., 2003). Siderophore-producing microbe Pseudomonas strains enhance the soil nutrients and other activities, including phosphate solubilization, potassium solubilization, and siderophore production under drought conditions (Breitkreuz et al., 2021).
Siderophore changes the oxidation states of heavy metals including Cd, Cu, Ni, Pb, Zn and Th4+, U4+, and Pu4+ to make them less toxic in nature (Schalk et al., 2011). Siderophores also bind different toxic metals such as Cr3+, Cu3+, Pb2+, Cu2+, V4+, and Al3+, while the binding capability of siderophores to Fe is more as compared to toxic heavy metals (Baysse et al., 2000; Braud et al., 2009b). Siderophores bind to toxic heavy metals, and thus toxic heavy metals do not hinder the efficiency of plant cells (Braud et al., 2009b). Therefore, the toxic heavy metal detoxifying and binding capability of siderophore plays a remarkable role in plant growth under heavy metal polluted land.
Siderophore has a strong affinity for the formation of iron-chelating compounds that help in the bioremediation process enhancing nutrient uptake and plant growth (Rajkumar et al., 2010). Bacterial strain P. fluorescence produces pyoverdine-type of siderophore that enhances mobility and reduces the toxicity of heavy metals in uranium mines (Edberg et al., 2010). Sharma and Johri (2003) reported that plant growth-promoting rhizobacterial genus Pseudomonas isolated from Zea mays L. secretes siderophores that have the potential to mobilize iron and have a high affinity to chelate Fe3+ ions resulting in heavy metals uptake. Pseudomonas strain GRP3 producing siderophore enhances the chlorophyll level in siderophore-treated mung bean plants (Sharma and Johri, 2003), and phytosiderophore enhances the iron efficiency of barley and wheat. Vishnupradeep et al. (2022) reported that two bacterial species Providencia sp. (TCR05) and Proteus mirabilis (TCR20) reduce the Cr toxicity from Cr(VI) to Cr(III) and enhanced plant pigments, protein, phenolics, and relative water content, while proline, lipid peroxidation, and superoxide dismutase decreased in Zea mays under heavy metal contaminated and drought conditions. Siderophore-producing microbes have the potential for phytoremediation of heavy metals and can overcome iron deficiency (Rajkumar et al., 2010). Dimkpa et al. (2009) reported that PGP rhizobacterial species Streptomyces tendae F4 phytoremediated cadmium (Cd) and enhanced the uptake of metals in heavy metals polluted lands. Enterobacter cloacae rhizospheric bacteria isolated from Spilanthes acmella Murr (toothache plant) of Shivalik hills region secretes PGP attributes including, exopolysaccharides (EPS) and 1-aminocyclopropane-a-carboxylic acid (ACC), acts as a biocontrol and biofertilizer under drought stress conditions (Thakur et al., 2021). Symbiotic association among plant and SPM is potentially involved in heavy metal uptake, SPM Rhizobium strains promoted Cu uptake while Pseudomonas strain promoted Cu and Fe uptake by Phaseolus vulgaris plants (Carrillo-Castaneda et al., 2007), and S. acidiscabies SPM secretes hydroxamate types of siderophores responsible for the solubilization and uptake of nickel and iron by Vigna unguiculata plants under nickel stress condition (Dimkpa et al., 2008). Symbiotic association of SPM Kluyvera ascorbata and plants decreased the toxicity of heavy metals (Burd et al., 2000) and suppressed the phytopathogens (Glick, 2012).
Siderophores maintain iron starvation in plants (Sayyed et al., 2019) and suppress the phytopathogens (Shaikh et al., 2014; Saha et al., 2016; Sayyed et al., 2019) like Phytophthora parasitica (Seuk et al., 1988), Phythium Ultimum (Hamdan et al., 1991). Ghazy and El-Nahrawy (2021) reported that bacterial strains such as B. subtilis MF497446 and P. koreensis MG209738 produce siderophores and induce disease resistance against Cephalosporium maydis in maize crops. Brevibacillus brevis GZDF3 (PGPR strain) isolated from the rhizosphere of Pinellia ternate plants play an important role in antagonistic activity against Candida albicans fungal disease by siderophore production (Mohammed et al., 2020; Sheng et al., 2020); P. flurescens and P. aeruginosa bacterial strain act as a biocontrol agent against Ralstonia solanacerum of tomato wilt. Siderophore-producing microbes, namely gram-negative bacteria Escherichia coli, secretes secondary metabolites such as siderophores that enhance iron uptake and plant growth performances under iron stress conditions (Neilands, 1995), and gram-negative bacterial genus Streptomyces acts as a biofertilizer that enhances the plant nutrients (Fe, P, and N), significantly increasing the germination rate, shoot length, and dry weight of wheat plant under saline stress condition (Sadeghi et al., 2012; Upadhyay et al., 2019).
Conclusion and Way Forwards
A proportional relation exists between growth performance and yield of plants; however, a big challenge arises in this proportional relationship due to the rapid rise in degraded land across the globe. The utilization of degraded land for agricultural practices becomes an issue for researchers to meet global food production for the future with eco-friendly and sustainable technology. Degraded land poses several detrimental impacts on plant growth and induces plant stress by less cycling of available nutrients and disruption in the metabolic function of the plant. The review discussed the influence of iron-deficient soil on plant and their management through eco-friendly products i.e., siderophores. The diverse chemical nature of siderophores can chelate Fe3+, which is produced by siderophore-producing rhizobacteria, and plant roots commonly known as bacterial siderophore (BS) and plant siderophore (PS).
In the rhizospheric microenvironment, both BS and PS synergistically facilitate iron uptake in the plant from iron-deficient soil mediated by reduction and chelation strategies. The utilization of siderophore-producing rhizobacteria can effectively maintain the iron level in plants and induce plant growth performances under degraded soil effectively when their selections meet compatibly with plant roots specifically. Future research requires the selection of the perfect candidate for siderophore-producing rhizobacteria, for a specific plant in degraded soil that would be useful for plant stress management and plant productivity at the field level.
Author Contributions
SU, PC, DJ, PS, and RS: conceptualization and visualization of the present review and writing the original draft. SU and PC: prepared the figures and tables. DJ, PD, and RS: contributed to re-structuring the review. PD, JW, and MJ contributed special remarks and edited the review for final submission. All authors contributed to the article and approved the submitted version.
Funding
The authors acknowledge the funding for the Science and Technology Major Project of Guangxi (AA18242026) and National Natural Science Foundation of China (81960164).
Conflict of Interest
The authors declare that the research was conducted in the absence of any commercial or financial relationships that could be construed as a potential conflict of interest.
Publisher's Note
All claims expressed in this article are solely those of the authors and do not necessarily represent those of their affiliated organizations, or those of the publisher, the editors and the reviewers. Any product that may be evaluated in this article, or claim that may be made by its manufacturer, is not guaranteed or endorsed by the publisher.
Acknowledgments
This review work was supported by the Department of Environmental Science, Veer Bahadur Singh Purvanchal University, Jaunpur, India.
References
Abiala, M. A., Abdelrahman, M., Burritt, D. J., Tran, L., and Son, P. (2018). Salt stress tolerance mechanisms and potential applications of legumes for sustainable reclamation of salt degraded soils. Land Degrad. Dev. 29, 3812–2822. doi: 10.1002/ldr.3095
Ahmed, E., and Holmstrom, S. J. (2014). Siderophores in environmental research: roles and applications. Micro. Biotech. 7, 196–208. doi: 10.1111/1751-7915.12117
Alam, A. (2014). Soil degradation: a challenge to sustainable agriculture. Int. J. Sci. Res. Agri. Sci. 1, 50–55. doi: 10.12983/ijsras-2014-p0050-0055
Alengebawy, A., Abdelkhalek, S. T., Qureshi, S. R., and Wang, M. Q. (2021). Heavy metals and pesticides toxicity in agricultural soil and plants: ecological risks and human health implications. Toxics. 9, 42. doi: 10.3390/toxics9030042
Alhendawi, R. A., Römheld, V., Kirkby, E. A., and Marschner, H. (1997). Influence of increasing bicarbonate concentrations on plant growth, organic acid accumulation in roots and iron uptake by barley, sorghum, and maize. J. Plan. Nut. 20, 1731–1753. doi: 10.1080/01904169709365371
Armin, R., Zuhlke, S., Grunewaldt-Stocker, G., Mahnkopp-Dirks, F., and Kusari, S. (2021). Production of siderophores by an apple root-associated Streptomyces ciscaucasicus strain GS2 using chemical and biological OSMAC approaches. Molecules 26, 3517. doi: 10.3390/molecules26123517
Ashraf, M., Shahzad, M., Akhtar, N., Imtiaz, M., and Ali, A. (2017). Salinization/sodification of soil and physiological dynamics of sunflower irrigated with saline–sodic water amending by potassium and farm yard manure. J. Water Reuse Desalin. 7, 476–487. doi: 10.2166/wrd.2016.053
Aznar, A., and Dellagi, A. (2015). New insights into the role of siderophores as triggers of plant immunity: what can we learn from animals? J. Exp. Bot. 66, 3001–3010. doi: 10.1093/jxb/erv155
Baars, O., Morel, F. M. M., and Zhang, X. (2018). The purple non-sulfur bacterium Rhodopseudomonas palustris produces novel petrobactin-related siderophores under aerobic and anaerobic conditions. Env. Microbiol. 20, 1667–1676. doi: 10.1111/1462-2920.14078
Barberon, M., Dubeaux, G., Kolb, C., Isono, E., Zelazny, E., and Vert, G. (2014). Polarization of iron-regulated transporter 1(IRT1) to the plant-soil interface plays crucial role in metal homeostasis. PNAS 111, 8293–8298. doi: 10.1073/pnas.1402262111
Bartels, D., and Sunkar, R. (2005). Drought and salt tolerance in plants. Crit. Rev. Plant Sci. 24, 23–58. doi: 10.1080/07352680590910410
Bashir, K., Rasheed, S., Kobayashi, T., Seki, M., and Nishizawa, N. K. (2016). Regulating subcellular metal homeostasis: the key to crop improvement. Front. Plant. Sci. 5, 1192. doi: 10.3389/fpls.2016.01192
Baysse, C., Baysse, C., De Vos, D., Naudet, Y., Vandermonde, A., Ochsner, U., et al. (2000). Vanadium interferes with siderophore-mediated iron uptake in Pseudomonas aeruginosa. Microbiology 146, 2425–2434. doi: 10.1099/00221287-146-10-2425
Becker, M., and Asch, F. (2005). Iron toxicity in rice—conditions and management concepts. J. Plant Nutr. Soil Sci. 168, 558–573. doi: 10.1002/jpln.200520504
Ben-Shem, A., Frolow, F., and Nelson, N. (2003). Crystal structure of plant photosystem I. Nature 426, 630–635. doi: 10.1038/nature02200
Bernard, E. I., Okoduwa, S. I. R., Idoko, G. O., Akabuogu, E. P., Adeyi, A. O., and Ejiogu, I. K. (2018). Toxicity and bioremediation of heavy metals contaminated ecosystem from tannery wastewater: a review. J. Toxicol. 2018, 2568038. doi: 10.1155/2018/2568038
Bhojiya, A. A., Joshi, H., Upadhyay, S. K., Srivastava, A. K., Pathak, V. V., Pandey, V. C., et al. (2022). Screening and optimization of zinc removal potential in Pseudomonas aeruginosa-HMR1 and its plant growth-promoting attributes. Bull. Env. Cont. Toxicol. 108, 468–477. doi: 10.1007/s00128-021-03232-5
Blatzer, M., Schrettl, M., Sarg, B., Lindner, H., Pfaller, K., and Haas, H. (2011). SidL, an Aspergillus fumigatus transacetylase involved in biosynthesis of the siderophores ferricrocin and hydroxyferricrocin. App. Env. Microbiol. 77, 4959–4966. doi: 10.1128/AEM.00182-11
Boiteau, R. M., Markillie, L. M., Hoyt, D. W., Hu, D., Chu, R. K., Mitchell, H. D., et al. (2021). Metabolic interactions between Brachypodiumand Pseudomonas fluorescens under controlled iron-limited conditions. mSystem 6, e00580–20. doi: 10.1128/mSystems.00580-20
Braud, A., Hoegy, F., Jezequel, K., Lebeau, T., and Schalk, I. J. (2009a). New insights into the metal specificity of the Pseudomonas aeruginosa pyoverdine-iron uptake pathway. Env. Microbiol. 11, 1079–1091. doi: 10.1111/j.1462-2920.2008.01838.x
Braud, A., Jezequel, K., Bazot, S., and Lebeau, T. (2009b). Enhanced phytoextraction of an agricultural Cr- and Pb-contaminated soil by bioaugmentation with siderophore-producing bacteria. Chemosphere 74, 280–286. doi: 10.1016/j.chemosphere.2008.09.013
Brear, E. M., Day, D. A., and Smith, P. M. C. (2013). Iron: an essential micronutrient for the legume-rhizobium symbiosis. Front. Plant Sci. 4, 359. doi: 10.3389/fpls.2013.00359
Breitkreuz, C., Reitz, T., Schulz, E., and Tarkka, M. T. (2021). Drought and plant community composition affect the metabolic and genotypic diversity of pseudomonas strains in grassland soils. Micro. 9, 1677. doi: 10.3390/microorganisms9081677
Burd, G. I., Dixon, D. G., and Glick, B. R. (2000). Plant growth promoting bacteria that decrease heavy metal toxicity in plants. Can. J. Microbiol. 46, 237–245. doi: 10.1139/w99-143
Butler, A., and Martin, J. (2005). “The marine biogeochemistry of iron,” in Metal Ions in Biological Systems, eds H. Sigel and M. Dekker (New York, NY: CRC Press), 21–46. doi: 10.1201/9780849346071-2
Butler, A., and Theisen, R. M. (2010). Iron(III)–siderophore coordination chemistry: reactivity of marine siderophores. Coordin. Chem. Rev. 254, 288–296. doi: 10.1016/j.ccr.2009.09.010
Carrillo-Castaneda, G., Munoz, J. J., Peralta-Videa, J. R., Gomez, E., and Gardea-Torresdey, J. L. (2007). Modulation of uptake and translocation of iron and copper from root to shoot in common bean by siderophore-producing microorganisms. J. Plant Nut. 28, 1853–1865. doi: 10.1080/01904160500251340
Chowdappa, S., Jagannath, S., Konappa, N., Udayashankar, A. C., and Jogaiah, S. (2020). Detection and characterization of antibacterial siderophores secreted by endophytic fungi from Cymbidium aloifolium. Biomolecules 10, 1412. doi: 10.3390/biom10101412
Colangelo, E. P., and Guerinot, M. L. (2004). The essential basic helix-loop-helix protein FIT1 is required for the iron deficiency response. Plant Cell 16, 3400–3412. doi: 10.1105/tpc.104.024315
Connorton, J. M., Balk, J., and Rodriguez-Celma, J. (2017). Iron homeostasis in plants – a brief overview. Metallomics 9, 813–823. doi: 10.1039/C7MT00136C
Dai, J., Wang, N., Xiong, H., Qiu, W., Nakanishi, H., Kobayashi, T., et al. (2018). The yellow stripe-like (YSL) gene functions in internal copper transport in peanut. Genes 9, 635. doi: 10.3390/genes9120635
Das, A., Prasad, R., Srivastava, A., Giang, P. H., Bhatnagar, K., and Varma, A. (2007). “Fungal siderophores: structure, functions and regulation,” in Soil Biology, eds A. Varma, and S. Chincholkar (Heidelberg: Springer), 1–42. doi: 10.1007/978-3-540-71160-5_1
Dave, B. P., Anshuman, K., and Hajela, P. (2006). Siderophores of halophilic archaea and their chemical characterization. Ind. J. Exp. Biol. 44, 340–344
Dave, B. P., and Dube, H. C. (2000). Chemical characterization of fungal siderophores. Ind. J. Exp. Biol. 38, 56–62.
Deori, M., Jayamohan, N. S., and Kumudini, B. S. (2018). Production, characterization and iron binding affinity of hydroxamate siderophores from rhizosphere associated fluorescent Pseudomonas. J. Plant Product. Res. 58, 36–43. doi: 10.24425/119116
Dertz, E. A., Xu, J., Stintzi, A., and Raymond, K. N. (2006). Bacillibactin-mediated iron transport in Bacillus subtilis. J. Am. Chem. Soc.128, 22–23. doi: 10.1021/ja055898c
Diaz de Villegas, M. E. (2007). “Biotechnological production of siderophores,” in Soil Biology, eds A. Varma, and S. Chincholkar (Heidelberg: Springer), 219–231. doi: 10.1007/978-3-540-71160-5_11
Dimkpa, C., Svatos, A., Merten, D., Buchel, G., and Kothe, E. (2008). Hydroxamate siderophores produced by Streptomyces acidiscabies E13 bind nickel and promote growth in cowpea (Vigna unguiculata L.) under nickel stress. Can. J. Microbiol. 54, 163–172. doi: 10.1139/w07-130
Dimkpa, C. O., Merten, D., Svatos, A., Buchel, G., and Kothe, E. (2009). Siderophores mediate reduced and increased uptake of cadmium by Streptomyces tendae F4 and sunflower (Helianthus annuus), respectively. J. App. Microbiol. 107, 1687–1696. doi: 10.1111/j.1365-2672.2009.04355.x
Dumanovic, J., Nepovimova, E., Natic, M., Kuca, K., and Jacevic, V. (2021). The significance of reactive oxygen species and antioxidant defense system in plants: a concise overview. Front. Plant Sci. 11, 552969. doi: 10.3389/fpls.2020.552969
Duncan, T. R., Werner-Washburne, M., and Northup, D. E. (2021). Diversity of siderophore-producing bacterial cultures from Carlsbad Caverns National Park caves, Carlsbad, New Mexico. J. Cav. Kart Stud. 83, 29-43. doi: 10.4311/2019ES0118
Edberg, F., Kalinowski, B. E., Holmstrom, S. J., and Holm, K. (2010). Mobilization of metals from uranium mine waste: the role of pyoverdines produced by Pseudomonas fluorescens. Geobiology 8, 278–292. doi: 10.1111/j.1472-4669.2010.00241.x
Edrisi, S. A., Tripathi, V., Chaturvedi, R. K., Dubey, D. K., Patel, G., and Abhilash, P. C. (2021). Saline soil reclamation index as an efficient tool for assessing restoration progress of saline land. Land Degrad. Dev. 32, 123–138. doi: 10.1002/ldr.3641
Egamberdieva, D., Wirth, S., Bellingrath-Kimura, S. D., Mishra, J., and Arora, N. K. (2019). Salt-Tolerant plant growth promoting rhizobacteria for enhancing crop productivity of saline soils. Front. Microbiol. 10, 2791. doi: 10.3389/fmicb.2019.02791
El-Maraghy, S. S., Tohamy, T. A., and Hussein, K. A. (2020). Expression of SidD gene and physiological characterization of the rhizosphere plant growth-promoting yeasts. Heliyon 6, e04384. doi: 10.1016/j.heliyon.2020.e04384
Ermakova, M., Lopez-Calcagno, P. E., Raines, C. A., Furbank, R. T., and von Caemmerer, S. (2019). Overexpression of the rieske FeS protein of the cytochrome b6f complex increases C4 photosynthesis in Setariaviridis. Comm. Biol. 16, 314. doi: 10.1038/s42003-019-0561-9
Essen, S. A., Johnsson, A., Bylund, D., Pedersen, K., and Lundstrom, U. S. (2007). Siderophore production by Pseudomonas stutzeri under aerobic and anaerobic conditions. App. Env. Microbiol. 73, 5857–5864. doi: 10.1128/AEM.00072-07
Etchegaray, A., Silva-Stenico, M., Moon, D., and Tsai, S. (2004). In silico analysis of non-ribosomal peptide synthetases of Xanthomonas axonopodis pv. citri: identification of putative siderophore and lipopeptide biosynthetic genes. Microbiol. Res. 159, 425–437. doi: 10.1016/j.micres.2004.09.009
Faraldo-Gomez, J. D., and Sansom, M. S. (2003). Acquisition of siderophores in gram-negative bacteria. Nat. Rev. Mol. Cell Biol. 4, 105–116. doi: 10.1038/nrm1015
Ferguson, A. D., and Deisenhofer, J. (2002). TonB-dependent receptors-structural perspectives. Biochim. Biophys. Acta 1565, 318–332. doi: 10.1016/S0005-2736(02)00578-3
Ferreira, C. M. H., Vilas, B. A., Sousa, C. A., Soares, H. M. V. M., and Soares, E. V. (2019). Comparison of five bacterial strains producing siderophores with ability to chelate iron under alkaline conditions. AMB Express 9, 78. doi: 10.1186/s13568-019-0796-3
Fiestner, G. J., Stahl, D. C., and Gabrik, A. H. (1993). Proferrioxamine siderophores of erwinia amylovora. A capillary liquid chromatographic/electrospray tandem mass spectrometric analysis. Org. Mass Spectrom. 28, 163–175. doi: 10.1002/oms.1210280307
Fuentes, M., Bacaicoa, E., Rivero, M., Zamarreno, A. M., and Garcia-Mina, J. M. (2018). Complementary evaluation of iron deficiency root responses to assess the effectiveness of different iron foliar applications for chlorosis remediation. Front. Plant Sci. 20, 351. doi: 10.3389/fpls.2018.00351
Fukushima, T., Allred, B. E., Sia, A. K., Nichiporuk, R., Andersen, U. N., and Raymond, K. N. (2013). Gram positive siderophore-shuttle with iron-exchange from Fe-siderophore to apo-siderophore by Bacillus cereus YxeB. Proc. Nat. Acad. Sci. U.S.A. 110, 13821–13826. doi: 10.1073/pnas.1304235110
Fukuyama, K., Hase, T., Matsumoto, S., Tsukihara, T., Katsube, Y., Tanaka, N., et al. (1980). Structure of s-platensis 2Fe-2S ferredoxin and evolution of chloroplast-type ferredoxins. Nature 286, 522–524. doi: 10.1038/286522a0
Gerber, N., Nkonya, E., and von Braun, J. (2014). “Land degradation, poverty and marginality,” in Marginality, eds J. von Braun, and F. Gatzweiler (Dordrecht: Springer). doi: 10.1007/978-94-007-7061-4_12
Ghazy, N., and El-Nahrawy, S. (2021). Siderophore production by Bacillus subtilis MF497446 and Pseudomonas koreensis MG209738 and their efficacy in controlling Cephalosporium maydis in maize plant. Arch. Microbiol. 203, 1195–1209. doi: 10.1007/s00203-020-02113-5
Glick, B. R. (2012). Plant growth-promoting bacteria: mechanisms and applications. Scientifica 2012, 963401. doi: 10.6064/2012/963401
Guilvout, I., Mercereau-Puijalon, O., Bonnefoy, S., Pugsley, A., and Carniel, E. (1993). High molecular-weight protein 2 of Yersinia enterocolitica is homologous to AngR of Vibrio anguillarum and belongs to a family of proteins involved in non-ribosomal peptide synthesis. J. Bacteriol. 175, 5488–5504. doi: 10.1128/jb.175.17.5488-5504.1993
Hamdan, H., Weller, D., and Thomashow, L. (1991). Relative importance of fluorescens siderophores and other factors in biological control of Gaeumannomyces graminis var. tritici by Pseudomonas fluorescens 2-79 and M4-80R. App. Env. Microbiol. 57, 3270–3277. doi: 10.1128/aem.57.11.3270-3277.1991
Herlihy, J. H., Long, T. A., and McDowell, J. M. (2020). Iron homeostasis and plant immune responses: recent insights and translational implications. J. Biolol. Chem. 295, 13444–13457. doi: 10.1074/jbc.REV120.010856
Hofmann, M., Heine, T., Malik, L., Hofmann, S., Joffroy, K., Senges, C. H. R., et al. (2021). Screening for microbial metal-chelating siderophores for the removal of metal ions from solutions. Microorganisms 9, 111. doi: 10.3390/microorganisms9010111
Hofmann, M., Retamal-Morales, G., and Tischler, D. (2020). Metal binding ability of microbial natural metal chelators and potential applications. Nat. Prod. Rep. 37, 1262–1283. doi: 10.1039/C9NP00058E
Hopkinson, B. M., and Morel, F. M. (2009). The role of siderophores in iron acquisition by photosynthethic marine microorganisms. Biometals 22, 659–669. doi: 10.1007/s10534-009-9235-2
Hu, Q. P., and Xu, J. U. (2011). A simple double layered chrome azurol S agar (SD CASA) plate assay to optimize the production of siderophores by a potential biocontrol agent Bacillus. Afr. J. Microbiol. Res. 5, 4321–4327. doi: 10.5897/AJMR11.238
Huo, Y., Kang, J. P., Ahn, J. C., Kim, Y. J., Piao, C. H., Yang, D. U., et al. (2021). Siderophore-producing rhizobacteria reduce heavy metal-induced oxidative stress in Panax ginseng Meyer. J. Gins Res. 45, 218–227. doi: 10.1016/j.jgr.2019.12.008
Hurt, E., and Hauska, G. A. (1981). Cytochrome f/b6 complex of five polypeptides with plastoquinol-plastocyanin-oxidoreductase activity from spinach chloroplasts. Eur. J. Biochem. 117, 591–599. doi: 10.1111/j.1432-1033.1981.tb06379.x
Igiehon, N. O., Babalola, O. O., and Aremu, B. R. (2019). Genomic insights into plant growth promoting rhizobia capable of enhancing soybean germination under drought stress. BMC microbiol. 19, 159. doi: 10.1186/s12866-019-1536-1
IPBES (2018). Summary for Policy makers of the Assessment Reporton Land Degradation and Restoration of IPBES, eds R. Scholes, L. Montanarella, A. Brainich, N. Barger, B. ten Brink, M. Cantele, B. Erasmus, J. Fisher, T. Gardner, T. G. Holland, et al. (Bonn: Intergovernmental Science-Policy Platform on Biodiversity and Ecosystem Services).
Ito, Y., and Butler, A. (2005). Structure of synechobactins, new siderophores of the marine cyanobacterium Synechococcus sp. PCC 7002. Limnol. Oceanogr. 50, 1918–1923. doi: 10.4319/lo.2005.50.6.1918
Jarmusch, S. A., Lagos-Susaeta, D., Diab, E., Salazar, O., Asenjo, J. A., Ebela, R., et al. (2020). Iron-meditated fungal starvation by lupine rhizosphere-associated and extremotolerant Streptomyces sp. S29 desferrioxamine production. Mol. Omics. 17, 95–107. doi: 10.1039/D0MO00084A
Jha, Y., and Subramanian, R. B. (2020). PGPR regulate caspase-like activity, programmed cell death, and antioxidant enzyme activity in paddy under salinity. Physiol. Mol. Biol. Plants 20, 201–207. doi: 10.1007/s12298-014-0224-8
Joshi, R. S., Shaikh, S. H., and Joshi, S. S. (2018). Optimization and partial characterization of siderophore produced by pseudomonas species isolated from agricultural soil. J. Glob. Biosci. 7, 5342–5349.
Kamran, M., Parveen, A., Ahmar, S., Malik, Z., Hussain, S., Chattha, M. S., et al. (2020). An overview of hazardous impacts of soil salinity in crops, tolerance mechanisms, and amelioration through selenium supplementation. Int. J. Mol. Sci. 21, 148. doi: 10.3390/ijms21010148
Kapoor, K., and Srivastava, A. (2010). Assessment of salinity tolerance of Vigna mungo var. Pu-19 using ex vitro and in vitro methods. Asian J. Biotechnol. 2, 73–85. doi: 10.3923/ajbkr.2010.73.85
Kar, I., and Chattopadhyaya, R. (2017). Effect of seven Indian plant extracts on fenton reaction-mediated damage to DNA constituents. J. Biomol. Struct. Dyn. 35, 2997–3011. doi: 10.1080/07391102.2016.1244493
Keesstra, S., Mol, G., De Leeuw, J., Okx, J., Molenaar, C., De Cleen, M., et al. (2018). Soil-Related sustainable development goals: four concepts to make land degradation neutrality and restoration work. Land 7, 133. doi: 10.3390/land7040133
Kesaulya, H., Hasinu, J. V., and Tuhumury, G. N. C. (2018). “Potential of Bacillusspp produces siderophores in suppressing the wilt disease of banana plants,” in International Symposium on Food and Agro-biodiversity (ISFA) (Semarang), Vol. 102 012016. doi: 10.1088/1755-1315/102/1/012016
Khaing, A., Theint, W. T., Thi, O. K., and Fu, P. (2021). Antagonistic activity of indigenous rhizobacteria through biosynthesis of indole-3-acetic acid (IAA), hydrogen cyanide (HCN), and siderophores. Aust. J. Biotechnol. Bioeng. 8, 1110. doi: 10.26420/austinjbiotechnolbioeng.2021.1110
Khan, A., Singh, P., and Srivastava, A. (2018). Synthesis, nature and utility of universal iron chelator – siderophore: a review. Microbiol. Res. 212–213, 103–111. doi: 10.1016/j.micres.2017.10.012
Kloepper, J. W., Leong, J., Teinize, M., and Schroth, M. N. (1980): Enhanced plant growth by siderophores produced by plant growth promoting rhizobacteria. Nature 286, 885–886. doi: 10.1038/286885a0
Koebnik, R. (2005). TonB-dependent trans-envelope signalling: the exception or the rule? Trends Microbiol. 13, 343–347. doi: 10.1016/j.tim.2005.06.005
Kong, Z., and Glick, B. R. (2017). The role of plant growth- promoting bacteria in metal phytoremediation zhaoyu. Adv. Microbiol. Physiol. 71, 327–353. doi: 10.1016/bs.ampbs.2017.04.001
Kraemer, S. (2004). Iron oxide dissolution and solubility in the presence of siderophores. Aquat. Sci. 66, 3–18. doi: 10.1007/s00027-003-0690-5
Krohling, C. A., Eutropio, F. J., Bertolazi, A. A., Dobbss, L. B., Campostrini, E., Dias, T., et al. (2016). Ecophysiology of iron homeostasis in plants. Soil Sci. Plant Nut. 62, 39–47. doi: 10.1080/00380768.2015.1123116
Kumar, M., Mishra, S., Dixit, V., Kumar, M., Agarwal, L., Chauhan, P. S., et al. (2016). Synergistic effect of Pseudomonas putida and Bacillus amyloliquefaciensameliorates drought stress in chickpea (Cicer arietinum L.). Plant Signal Behav. 11, e1071004. doi: 10.1080/15592324.2015.1071004
Kumari, S., Kiran, S., Kumari, S., Kumar, P., and Singh, A. (2021). Optimization of siderophore production by Bacillus Subtilis DR2 and its effect on growth of Coriandrum Sativum. Res. Squ. 1–25. doi: 10.21203/rs.3.rs-567897/v1
Lal, R. (2012). Climate change and soil degradation mitigation by sustainable management of soils and other natural resources. Agric. Res.1, 199–212. doi: 10.1007/s40003-012-0031-9
Lastochkina, O., Garshina, D., and Pusenkova, L. (2020). “Effect of endophytic Bacillus subtilis on drought stress tolerance of Triticum aestivum plants of steppe volga and forest-steppe west siberian agroecological groups,” in 2nd International Conference Plants and Microbes: The Future of Biotechnology (Saratov), 5–9. doi: 10.28983/PLAMIC2020.149
Latitha, S., and Nithyapriya, S. (2020). Production of bacillibactin siderophore from soil bacteria, Bacillus subtilis: a bioinoculant enhances plant growth in Arachis hypogaea L. through elevated uptake of nutrients. Adv. Biol. Sci. Res. 13, 71–81. doi: 10.2991/absr.k.210609.013
Leguizamo, M. A. O., Gomez, W. D. F., and Sarmiento, M. C. G. (2017). Native herbaceous plant species with potential use in phytoremediation of heavy metals, spotlight on wetlands – a review. Chemosphere 168, 1230–1247. doi: 10.1016/j.chemosphere.2016.10.075
Leskova, A., Giehl, R., Hartmann, A., Fargasova, A., and von Wiren, N. (2017). Heavy metals induce iron deficiency responses at different hierarchic and regulatory levels. Plant Physiol. 174, 1648–1668. doi: 10.1104/pp.16.01916
Liliane, T. N., and Charles, M. S. (2020). “Factors affecting yield of crops,” in Agronomy - Climate Change & Food Security (IntechOpen). doi: 10.5772/intechopen.90672
Lurthy, T., Cantat, C., Jeudy, C., Declerck, P., Gallardo, K., Barraud, C., et al. (2020). Impact of bacterial siderophores on iron status and ionome in pea. Front. Plant Sci. 11, 730. doi: 10.3389/fpls.2020.00730
Ma, Y., Dias, M. C., and Freitas, H. (2020). Drought and salinity stress responses and microbe-induced tolerance in plants. Front. Plant Sci. 11, 591911. doi: 10.3389/fpls.2020.591911
Machado, R. M. A., and Serralheiro, R. P. (2017). Soil salinity: effect on vegetable crop growth. Management practices to prevent and mitigate soil salinization. Horticulturae 3, 30. doi: 10.3390/horticulturae3020030
Mallahi, T., Saharkhiz, M. J., and Javanmardi, J. (2018). Salicylic acid changes morpho-physiological attributes of feverfew (Tanacetum parthenium L.) under salinity stress. Acta Ecol. Sin. 38, 351–355. doi: 10.1016/j.chnaes.2018.02.003
Mann, A., Singh, A. L., Oza, S., Goswami, N., Mehta, D., and Chaudhari, V. (2017). Effect of iron source on iron deficiency induced chlorosis in groundnut. Leg. Res. 40, 241–249. doi: 10.18805/lr.v0iOF.6849
Meneely, K. M., and Lamb, A. L. (2007). Biochemical characterization of an FAD-dependent monooxygenase, the ornithine hydroxylase from Pseudomonas aeruginosa, suggests a novel reaction mechanism. Biochemistry 46, 11930–11937. doi: 10.1021/bi700932q
Mladenka, P., Macakova, K., Zatloukalova, L., Rehakova, Z., Singh, B. K., Prasad, A. K., et al. (2010). In vitro interactions of coumarins with iron. Biochimie 92, 1108–1114. doi: 10.1016/j.biochi.2010.03.025
Mohammed, A. F., Oloyede, A. R., and Odeseye, A. O. (2020). Biological control of bacterial wilt of tomato caused by Ralstonia solanacearum using Pseudomonas species isolated from the rhizosphere of tomato plants. Arch. Phytopathol. Plant Protect. 53, 1–16. doi: 10.1080/03235408.2020.1715756
Morrissey, J., and Guerinot, M. L. (2009). Iron uptake and transport in plants: the good, the bad, and the ionome. Chemical reviews 109, 4553–4567. doi: 10.1021/cr900112r
Nadeem, S. M., Shaharoona, B., Arshad, M., and Crowley, D. E. (2012). Population density and functional diversity of plant growth promoting rhizobacteria associated with avocado trees in saline soils. Appl Soil Ecol. 62, 147–154. doi: 10.1016/j.apsoil.2012.08.005
Nagata, T., Oobo, T., and Aozasa, O. (2013). Efficacy of a bacterial siderophore, pyoverdine, to supply iron to Solanum lycopersicum plants. J. Bio Sci. Bioeng. 115, 686–690. doi: 10.1016/j.jbiosc.2012.12.018
Nagoba, B., and Vedpathak, D. V. (2011). Medical applications of siderophores–a review. Eur. J. Gen. Med. 8, 230–233. doi: 10.29333/ejgm/82743
Najimi, M., Lemos, M. L., and Osorio, C. R. (2008). Identification of siderophore biosynthesis genes essential for growth of Aeromonas salmonicida under iron limitation conditions. Appl. Env. Microbiol. 74, 2341–2348. doi: 10.1128/AEM.02728-07
Neilands, J. B. (1995). Siderophores: structure and function of microbial iron transport compounds. J. Biol. Chem. 270, 26723–26726. doi: 10.1074/jbc.270.45.26723
Nithyapriya, S., Lalitha, S., Sayyed, R. Z., Reddy, M. S., Dailin, D. J., El Enshasy, H. A., et al. (2021). Production, purification, and characterization of bacillibactin siderophore of Bacillus subtilis and its application for improvement in plant growth and oil content in sesame. Sustainable 13, 5394. doi: 10.3390/su13105394
Noinaj, N., Guillier, M., Barnard, T. J., and Buchanan, S. K. (2010). TonB-dependent transporters: regulation, structure and function. Annu. Rev. Microbiol. 64, 43–60. doi: 10.1146/annurev.micro.112408.134247
Orozco-Mosqueda, M. D. C., Glick, B. R., and Santoyo, G. (2020). ACC deaminase in plant growth-promoting bacteria (PGPB): An efficient mechanism to counter salt stress in crops. Microbiol. Res. 235, 126439. doi: 10.1016/j.micres.2020.126439
Osman, Y., Mowafy, A. M., Gebreil, A. S., and Hamed, S. M. (2018). Siderophore production by rhizosphere inhabiting bacteria and fungi. J. Plant Prod. 9, 717–721. doi: 10.21608/jpp.2018.36395
Palaniyandi, S. A., Damodharan, K., Yang, S. H., and Suh, J. W. (2014). Streptomyces sp. strain PGPA39 alleviates salt stress and promotes growth of ‘Micro Tom' tomato plants. J. Appl. Microbiol. 117, 766–773. doi: 10.1111/jam.12563
Parveen, S. R., and Latha, D. (2019). Characterization of siderophore producing PseudomonasSp for its plant growth promoting properties. Biosc. Biotech. Res. Comm. 12, 1031–1037. doi: 10.21786/bbrc/12.4/26
Patel, T. M., and Minocheherhomji, F. P. (2018). Isolation and characterization of several siderophore producing bacteria from cotton plant. Inter. J. Inn. Res. Sci. Eng. Tech. 7, 159–166. doi: 10.15680/IJIRSET.2018.0701026
Patel, T. M., and Minocheherhomji, F. P. (2018). Isolation and characterization of several siderophore producing bacteria from cotton plant. Inter. J. Inn. Res. Sci. Eng. Tech. 7, 159–166.
Paul S., Upadhyay S. K., and Lal E. P. (2014). Accumulation of arsenic in radish (Raphanus sativus L.), and their effects on growth and antioxidant activities. IJPSR. 5, 3536–3543. doi: 10.13040/IJPSR.0975-8232.5(8).3536-43
Paul, A., and Dubey, R. (2015). Characterization of protein involved in nitrogen fixation and estimation of co-factor. Appl. J. Curr. Res. Biosci. Plant Biol. 2, 89–97.
Paul, R. A. I., Dhivyadharsini, D., and Mathivadhana, K. S. (2020b). Rehabilitation of heavy metal contamination and soil erosion through integrated management. Agri. Rev. 1, 1–8, doi: 10.18805/ag.R-2052
Paul, S., Singh, V., Chauhan, P. K., Srivastava, A. K., and Upadhyay, S. K. (2020c). Assessment of carrot growth performance with inoculation of AsT-PGPR under arsenic infested zone. G J. Env. Sci. Technol. 7, 78–84. Available online at: https://gjestenv.com/index.php/gjest/article/view/131
Paul, S., Upadhyay, S. K., and Singh, N. (2020a). Geogenic source of arsenic and their effect on vegetable seed germination. Trop. Plant Res. 7, 110–116. doi: 10.22271/tpr.2020.v7.i1.015
Peralta, D., Adler, C., Corbalan, N., Paz Garcia, E., Pomares, M., and Vincent, P. (2016). Enterobactin as part of the oxidative stress response repertoire. PLoS ONE 11, e0157799. doi: 10.1371/journal.pone.0157799
Qadir, M., Quillerou, E., Nangia, V., Murtaza, G., Singh, M., Thomas, R. J., et al. (2014). Economics of salt induced land degradation and restoration. Nat. Resourc. For. 38, 282–295. doi: 10.1111/1477-8947.12054
Rabhi, M., Barhoumi, Z., Ksouri, R., Abdelly, C., and Gharsalli, M. (2007). Interactive effects of salinity and iron deficiency in Medicago ciliaris. C. R. Biol. 330, 779–788. doi: 10.1016/j.crvi.2007.08.007
Rai, P. K. (2021). Environmental degradation by invasive alien plants in the anthropocene: challenges and prospects for sustainable restoration. Anthr. Sci. 1, 5–28. doi: 10.1007/s44177-021-00004-y
Rai, S., Singh, P. K., Mankotia, S., Swain, J., and Satbhai, S. B. (2021). Iron homeostasis in plants and its crosstalk with copper, zinc, and manganese. Plant Stress 1, 100008. doi: 10.1016/j.stress.2021.100008
Rajkumar, M., Ae, N., Prasad, M., N., V., and Freitas H (2010). Potential of siderophore-producing bacteria for improving heavy metal phytoextraction. Trends Biotech. 28, 142–149. doi: 10.1016/j.tibtech.2009.12.002
Rangseekaew, P., Barros-Rodriguez, A., Pathom-aree, W., and Manzanera, M. (2021). Deep-Sea actinobacteria mitigate salinity stress in tomato seedlings and their biosafety testing. Plants 10, 1687. doi: 10.3390/plants10081687
Reimmann, C., Patel, H. M., Serino, L., Barone, M., Walsh, C. T., and Haas, D. (2001). Essential PchG-dependent reduction in pyochelin biosynthesis of Pseudomonas aeruginosa. J. Bacteriol. 183, 813–820. doi: 10.1128/JB.183.3.813-820.2001
Ribeiro, M., and Simoe, M. (2019). Advances in the antimicrobial and therapeutic potential of siderophores. Environ. Chem. Lett. 17, 1485–1494. doi: 10.1007/s10311-019-00887-9
Sadeghi, A., Karimi, E., Dahaji, P. A., Javid, M. G., Dalvand., Y., and Askar, H. (2012). Plant growth promoting activity of an auxin and siderophore producing isolate of Streptomyces under saline soil conditions. World J. Microbiol. Biotechnol. 28, 1503–1509. doi: 10.1007/s11274-011-0952-7
Sah, S., and Singh, R. (2015). Siderophore: structural and functional characterization—a comprehensive review. Agriculture 6, 97–114. doi: 10.1515/agri-2015-0015
Saha, M., Sarkar, S., Sarkar, B., Sharma, B. K., Bhattacharjee, S., and Tribedi, P. (2016). Microbial siderophores and their potential applications: a review. Env. Sci. Pollut. Res. Int. 23, 3984–3999. doi: 10.1007/s11356-015-4294-0
Santoyo, G., and Strathern, J. N. (2008). Non-homologous end joining is important for repair of Cr(VI)-induced DNA damage in Saccharomyces cerevisiae. Microbiol. Res. 163, 113–119. doi: 10.1016/j.micres.2007.09.001
Satbhai, S. B., Setzer, C., Freynschlag, F., Slovak, R., Kerdaffrec, E., and Busch, W. (2017). Natural allelic variation of FRO2 modulates Arabidopsis root growth under iron deficiency. Nat. Comm. 24, 8,15603. doi: 10.1038/ncomms15603
Sayyed, R. Z., Ilyas, N., Tabassum, B., Hashem, A., AbdAllah, E. F., and Jadhav, H. P. (2019). Plausible role of plant growth-promoting rhizobacteria in future climatic scenario. Environ. Biotech. 175–197. doi: 10.1007/978-981-10-7284-0_7
Schalk, I. J., and Guillon, L. (2013). Fate of ferrisiderophores after import across bacterial outer membranes: different iron release strategies are observed in the cytoplasm or periplasm depending on the siderophore pathways. Amino Acids 44, 1267–1277. doi: 10.1007/s00726-013-1468-2
Schalk, I. J., Hannauer, M., and Braud, A. (2011). Mini review new roles for bacterial. Enviorn. Microbiol. 13, 2844–2854. doi: 10.1111/j.1462-2920.2011.02556.x
Schalk, I. J., Mislin, G. L. A., and Brillet, K. (2012). Structure, function and binding selectivity and stereo selectivity of siderophore-iron outer membrane transporters. Curr. Top. Membr. 69, 37–66. doi: 10.1016/B978-0-12-394390-3.00002-1
Schrettl, M., Bignell, E., Kragl, C., Sabiha, Y., Loss, O., Eisendle, M., et al. (2007). Distinct roles for intra- and extracellular siderophores during Aspergillus fumigatus infection. PLoS Pathog. 3, e128. doi: 10.1371/journal.ppat.0030128
Schutze, E., Ahmed, E., Voit, A., et al. (2015). Siderophore production by streptomycetes—stability and alteration of ferrihydroxamates in heavy metal-contaminated soil. Environ. Sci. Pollut. Res. 22,19376–19383, doi: 10.1007/s11356-014-3842-3
Searle, L. J., Meric, G., Porcelli, I., Sheppard, S. K., and Lucchini, S. (2015). Variation in siderophore biosynthetic gene distribution and production across environmental and faecal populations of Escherichia coli. PLoS ONE 10, e0117906. doi: 10.1371/journal.pone.0117906
Seifert, M., Nairz, M., Schroll, A., Schrettl, M., Haas, H., and Weiss, G. (2008). Effects of the Aspergillus fumigatus siderophore systems on the regulation of macrophage immune effector pathways and iron homeostasis? Immunobiology 213, 767–778. doi: 10.1016/j.imbio.2008.07.010
Seuk, C., Paulita, T., and Baker, R. (1988). Attributes associate with increased bio control activity of fluorescent Pseudomonads. J. Plant Pathol. 4, 218–225.
Seyoum, Y., Baye, K., and Humblot, C. (2021). Iron homeostasis in host and gut bacteria - a complex interrelationship. Gut Microbes. 13, 1–19. doi: 10.1080/19490976.2021.1874855
Shaikh, S. S., Patel, P. R., Patel, S. S., Nikam, S. D., Rane, T. U., and Sayyed, R. Z. (2014). Production of biocontrol traits by banana field Fluorescent pseudomonads and their comparison with chemical fungicides. Ind. J. Exp. Biol. 52, 917-920.
Shakeel, A., Khan, A. A., and Upadhyay, S. K. (2022). Eco-friendly dual-edged management of fly ash and its antagonistic interplay with Meloidogyne incognita on beetroot (Beta vulgaris L.). Environ. Res. 209,112767, doi: 10.1016/j.envres.2022.112767
Sharma, A., and Johri, B. N. (2003). Growth promoting influence of siderophore-producing pseudomonas strains GRP3A and PRS9 in maize (Zea mays L.) under iron limiting conditions. Microbiol. Res.158, 243–248. doi: 10.1078/0944-5013-00197
Sheng, M., Jia, H., Zhang, G., Zeng, L., Zhang, T., Long, Y., et al. (2020). Siderophore production by rhizosphere biological control bacteria Brevibacillus brevis GZDF3 of Pinelliaternata and its antifungal effects on Candida albicans. J. Micro. Biotech. 30, 689–699. doi: 10.4014/jmb.1910.10066
Siedow, J. N. (1991). Plant lipoxygenase: structure and function. Ann. Rev. Plant Physiol. Plant Mol. Biol. 42, 145–188. doi: 10.1146/annurev.pp.42.060191.001045
Silambarasan, S., Logeswari, P., Valentine, A., Cornejo, P., and Kannan, V. R. (2020). Pseudomonas citronellolis strain SLP6 enhances the phytoremediation efficiency of Helianthus annuus in copper contaminated soils under salinity stress. Plant Soil 457, 241–253. doi: 10.1007/s11104-020-04734-7
Sileshi, G. W., Mafongoya, P. L., and Nath, A. J. (2020). “Agroforestry systems for improving nutrient recycling and soil fertility on degraded lands,” in Agroforestry for Degraded Landscapes, eds J. C. Dagar, S. R. Gupta, and D. Teketay (Singapore: Springer). doi: 10.1007/978-981-15-4136-0_8
Silpa, D., Brahmaji R. P., and Kranthi K. G. (2018). Biocontrol activity of Siderophores producing Bacillus licheniformis DS3 against several pathogenic fungi in Black gram [Vigna mungo (L.) hepper]. Int. J. Cur. Res. 10, 71590–71594. doi: 10.24941/ijcr.31092.07.2018
Silva-Bailao, M. G., Bailao, E. F., Lechner, B. E., Gauthier, G. M., Lindner, H., Bailao, A. M., et al. (2014). Hydroxamate production as a high affinity iron acquisition mechanism in Paracoccidioides spp. PLoS ONE 9, e105805. doi: 10.1371/journal.pone.0105805
Singh, P., Singh, R. K., Guo, D. J., Sharma, A., Singh, R. N., Li, D. P., et al. (2021a). Whole genome analysis of sugarcane root-associated endophyte Pseudomonas aeruginosa B18—a plant growth-promoting bacterium with antagonistic potential against Sporisorium scitamineum. Front. Microbiol. 1, 628376. doi: 10.3389/fmicb.2021.628376
Singh, P., Singh, R. K., Li, H. B., Guo, D. J., Sharma, A., Lakshmanan, P., et al. (2021b). Diazotrophic bacteria Pantoea dispersa and Enterobacter asburiae promote sugarcane growth by inducing nitrogen uptake and defense-related gene expression. Front. Microbiol. 11, 600417. doi: 10.3389/fmicb.2020.600417
Singh, P., Singh, R. K., Zhou, Y., Wang, J., Jiang, Y., Shen, N., et al. (2022). Unlocking the strength of plant growth promoting Pseudomonas in improving crop productivity in normal and challenging environments: a review. J. Plant Interact. 17, 220–238. doi: 10.1080/17429145.2022.2029963
Singh, R., Pandey, D. K., and Kumar, A. (2017). PGPR isolates from the rhizosphere of vegetable crop Momordica charantia: characterization and application as biofertilizer. Int. J. Curr. Microbiol. Appl Sci. 6, 1789–1802. doi: 10.20546/ijcmas.2017.603.205
Singh, R. K., Singh, P., Guo, D. J., Sharma, A., Li, D. P., Li, X., et al. (2021c). Root-Derived endophytic diazotrophic bacteria Pantoea cypripedii AF1 and Kosakonia arachidis EF1 promote nitrogen assimilation and growth in sugarcane. Front. Microbiol. 12, 774707. doi: 10.3389/fmicb.2021.774707
Singh, R. K., Singh, P., Li, H. B., Guo, D. J., Song, Q. Q., Yang, L. T., et al. (2020b). Plant-PGPR interaction study of plant growth-promoting diazotrophs Kosakonia radicincitans BA1 and Stenotrophomonas maltophilia COA2 to enhance growth and stress-related gene expression in Saccharum spp. J. Plant Interact. 15, 427–445. doi: 10.1080/17429145.2020.1857857
Singh, R. K., Singh, P., Li, H. B., Song, Q. Q., Guo, D. J., Solanki, M. K., et al. (2020a). Diversity of nitrogen-fixing rhizobacteria associated with sugarcane, a comprehensive study of plant-microbe interactions for growth enhancement in Saccharum spp. BMC Plant Biol. 20, 220. doi: 10.1186/s12870-020-02400-9
Sinha, A. K., Parli, V. B., Tripathy, S. C., Sarkar, A., and Prabhakaran, S. (2019). Effects of growth conditions on siderophore producing bacteria and siderophore production from Indian Ocean sector of Southern Ocean. J. Basic Microbiol. 59, 412–424. doi: 10.1002/jobm.201800537
Srinivasarao, C., Rakesh, S., Kumar, G. R., Manasa, R., Somashekar, G., Lakshmi, C. S., et al. (2021). Soil degradation challenges for sustainable agriculture in tropical India. Curr. Sci. 120, 492–500. Available online at: https://www.currentscience.ac.in/Volumes/120/03/0492.pdf
Subramanium, N., and Sundaram, L. (2020). Siderophore producing Pseudomonas spp. isolated from rhizospheric soil and enhancing iron content in Arachis hypogaea L. plant. Int. J. Agri. Technol. 16, 429–442.
Sultana, S., Alamb, S., and Karimc, M. M. (2021). Screening of siderophore-producing salt-tolerant rhizobacteria suitable for supporting plant growth in saline soils with iron limitation. J. Agri. Food Res. 4, 100150. doi: 10.1016/j.jafr.2021.100150
Takahashi, F., Kuromori, T., Urano, K., Yamaguchi-Shinozaki, K., and Shinozaki, K. (2020). Drought stress responses and resistance in plants: from cellular responses to long-distance intercellular communication. Front. Plant Sci. 11, 556972. doi: 10.3389/fpls.2020.556972
Tewari, R. K., Hadacek, F., Sassmann, S., and Lang, I. (2013). Iron deprivation-induced reactive oxygen species generation leads to non-autolytic PCD in Brassica napus leaves. Environ. Exp. Bot. 91, 74–83. doi: 10.1016/j.envexpbot.2013.03.006
Thakur, M., Mittal, D., Khosla, P. K., Saini, V., Saini, R. V., and Saini, A. K. (2021). Rhizobacteria associated with Spilanthes acmella Murr. confer drought-tolerance and plant growth promotion. Biointer. Res. Appl. Chem. 11, 13155–13170. doi: 10.33263/BRIAC115.1315513170
Trapet, P., Avoscan, L., Klinguer, A., Pateyron, S., Citerne, S., Chervin, C., et al. (2016). The Pseudomonas fluorescens siderophore pyoverdine weakens Arabidopsis thaliana defense in favor of growth in iron-deficient conditions. Plant Physiol. 171, 675–693. doi: 10.1104/pp.15.01537
Tripathi, D. K., Singh, S., Gaur, S., Singh, S., Yadav, V., Liu, S., et al. (2018). Acquisition and homeostasis of iron in higher plants and their probable role in abiotic stress tolerance. Front. Environ. Sci. 5, 86. doi: 10.3389/fenvs.2017.00086
Upadhyay, S. K., Ahmad, M., Srivastava, A. K., Abhilash, P. C., and Sharma, B. (2021). Optimization of eco-friendly novel amendments for sustainable utilization of fly ash based on growth performance, hormones, antioxidant, and heavy metal translocation in chickpea (Cicer arietinum L.) plant. Chemosphere 267, 129216. doi: 10.1016/j.chemosphere.2020.129216
Upadhyay, S. K., and Chauhan, P. K. (2019). Study of land use and land cover of ravine area using geospatial satellite data. J. Environ. Sci. Pollut. Res. 5, 383–386. doi: 10.30799/jespr.181.19050401
Upadhyay, S. K., and Chauhan, P. K. (2022). Optimization of Eco-friendly amendments as sustainable asset for salt-tolerant plant growth-promoting bacteria mediated maize (Zea Mays L.) plant growth, Na uptake reduction and saline soil restoration. Environ. Res. 211, 113081. doi: 10.1016/j.envres.2022.113081
Upadhyay, S. K., and Edrisi, S. A. (2021). Developing sustainable measures to restore fly ash contaminated lands: current challenges and future prospects. Land Degrad. Dev. 32, 4817–4831. doi: 10.1002/ldr.4090
Upadhyay, S. K., Saxena, A. K., Singh, J. S., and Singh, D. P. (2019). Impact of native ST-PGPR (Bacillus pumilus; EU927414) on PGP traits, antioxidants activities, wheat Plant growth and yield under salinity. Climat. Chan. Env. Sust. 7, 157–168. doi: 10.5958/2320-642X.2019.00021.8
Upadhyay, S. K., and Singh, D. P. (2015). Effect of salt-tolerant plant growth-promoting rhizobacteria on wheat plants and soil health in a saline environment. Plant Biol. 17, 288–293. doi: 10.1111/plb.12173
Upadhyay, S. K., Singh, D. P., and Saikia, R. (2009). Genetic diversity of plant growth promoting rhizobacteria isolated from rhizospheric soil of wheat under saline condition. Cur. Microbiol. 59, 489–496. doi: 10.1007/s00284-009-9464-1
Upadhyay, S. K., Singh, J. S., Saxena, A. K., and Singh, D. P. (2012). Impact of PGPR inoculation on growth and antioxidant status of wheat under saline conditions. Plant Biol. 14, 605–611. doi: 10.1111/j.1438-8677.2011.00533.x
Upadhyay, S. K., Singh, J. S., and Singh, D. P. (2011). Exopolysaccharide-producing plant growth-promoting rhizobacteria under salinity condition. Pedosphere 21, 214–222. doi: 10.1016/S1002-0160(11)60120-3
Ustiatik, R., Nuraini, Y., and Suharjono, H. E. (2021). Siderophore production of the Hg-resistant endophytic bacteria isolated from local grass in the Hg-contaminated soil. J. Ecol. Eng. 22, 129–138. doi: 10.12911/22998993/135861
Vishnupradeep, R., Bruno, L. B., Taj, Z., Karthik, C., Challabathula, D., Triptid, K. umar, A., et al. (2022). Plant growth promoting bacteria improve growth and phytostabilization potential of Zea mays under chromium and drought stress by altering photosynthetic and antioxidant responses. Env. Technol. Inno. 25, 102154. doi: 10.1016/j.eti.2021.102154
Vivas, A., Marulanda, A., Ruiz-Lozano, J. M., Barea, J. M., and Azcon, R. (2003). Influence of a Bacillus sp. on physiological activities of two arbuscular mycorrhizal fungi and on plant responses to PEG induced drought stress. Mycorrhiza 13, 249–256. doi: 10.1007/s00572-003-0223-z
Wang, X., Zhang, M., Loh, B., Leptihn, S., Ahmed, T., and Li, B. (2021). A novel NRPS cluster, acquired by horizontal gene transfer from algae, regulates siderophore iron metabolism in Burkholderiaseminalis R456. Int. J. Biol. Macromol. 182, 838–848. doi: 10.1016/j.ijbiomac.2021.04.051
Wasi, S., Tabrez, S., and Ahmad, M. (2012). Toxicological effects of major environmental pollutants: an overview. Environ. Monit. Assess. 185, 2585–2593. doi: 10.1007/s10661-012-2732-8
Watts, R., Totsika, M., Challinor, V., Mabbett, A., Ulett, G., De Voss, J., et al. (2011). Contribution of siderophore systems to growth and urinary tract colonization of asymptomatic bacteriuria Escherichia coli. Infect. Immun. 80, 333–344. doi: 10.1128/IAI.05594-11
Winkelmann, G. (2007): Ecology of siderophores with special reference to the fungi. Biometals 20, 379–392. doi: 10.1007/s10534-006-9076-1.
Wittmann, S., Heinisch, L., Scherlitz-Hofmann, I. N. A., Stoiber, T., Dorothe, A. F., and Mollmann, U. (2001): Catecholates and ixed catecholate hydroxamates as artificial siderophores for mycobacteria. Biometals 17, 53–64. doi: 10.1023/A:1024409517626
Yadav, S., Kaushik, R., Saxena, A. K., and Arora, D. K. (2011). Diversity and phylogeny of plant growth-promoting bacilli from moderately acidic soil. J. Basic Microbiol. 51, 98–106. doi: 10.1002/jobm.201000098
Yang, J., Kloepper, J. W., and Ryu, C. M. (2009). Rhizosphere bacteria help plants tolerate abiotic stress. Trend. Plant. Sci. 14, 1–4. doi: 10.1016/j.tplants.2008.10.004
Keywords: plant stress, siderophores, molecular mechanism, rhizospheric microbes, degraded land
Citation: Singh P, Chauhan PK, Upadhyay SK, Singh RK, Dwivedi P, Wang J, Jain D and Jiang M (2022) Mechanistic Insights and Potential Use of Siderophores Producing Microbes in Rhizosphere for Mitigation of Stress in Plants Grown in Degraded Land. Front. Microbiol. 13:898979. doi: 10.3389/fmicb.2022.898979
Received: 18 March 2022; Accepted: 09 June 2022;
Published: 11 July 2022.
Edited by:
Shekhar Jain, Mandsaur University, IndiaReviewed by:
Pramod Kumar Sahu, Indian Council of Agricultural Research (ICAR), IndiaAsma Imran, National Institute for Biotechnology and Genetic Engineering, Pakistan
Copyright © 2022 Singh, Chauhan, Upadhyay, Singh, Dwivedi, Wang, Jain and Jiang. This is an open-access article distributed under the terms of the Creative Commons Attribution License (CC BY). The use, distribution or reproduction in other forums is permitted, provided the original author(s) and the copyright owner(s) are credited and that the original publication in this journal is cited, in accordance with accepted academic practice. No use, distribution or reproduction is permitted which does not comply with these terms.
*Correspondence: Mingguo Jiang, bXp4eWppYW5nJiN4MDAwNDA7MTYzLmNvbQ==; Sudhir K. Upadhyay, c2t1LmVudi5sa28mI3gwMDA0MDtnbWFpbC5jb20=
†These authors have contributed equally to this work and share first authorship