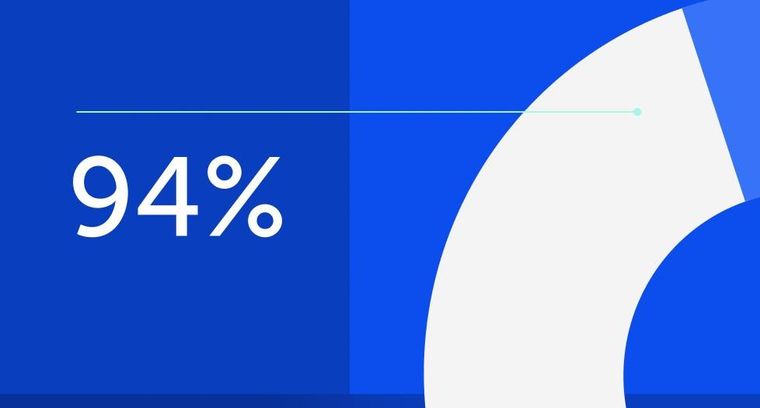
94% of researchers rate our articles as excellent or good
Learn more about the work of our research integrity team to safeguard the quality of each article we publish.
Find out more
ORIGINAL RESEARCH article
Front. Microbiol., 12 May 2022
Sec. Microbial Physiology and Metabolism
Volume 13 - 2022 | https://doi.org/10.3389/fmicb.2022.897066
This article is part of the Research TopicHydrogenase: Structure, Function, Maturation, and ApplicationView all 12 articles
Hydrogen (H2) converted to reducing equivalents is used by acetogens to fix and metabolize carbon dioxide (CO2) to acetate. The utilization of H2 enables not only autotrophic growth, but also mixotrophic metabolism in acetogens, enhancing carbon utilization. This feature seems useful, especially when the carbon utilization efficiency of organic carbon sources is lowered by metabolic engineering to produce reduced chemicals, such as ethanol. The potential advantage was tested using engineered strains of Moorella thermoacetica that produce ethanol. By adding H2 to the fructose-supplied culture, the engineered strains produced increased levels of acetate, and a slight increase in ethanol was observed. The utilization of a knockout strain of the major acetate production pathway, aimed at increasing the carbon flux to ethanol, was unexpectedly hindered by H2-mediated growth inhibition in a dose-dependent manner. Metabolomic analysis showed a significant increase in intracellular NADH levels due to H2 in the ethanol-producing strain. Higher NADH level was shown to be the cause of growth inhibition because the decrease in NADH level by dimethyl sulfoxide (DMSO) reduction recovered the growth. When H2 was not supplemented, the intracellular NADH level was balanced by the reversible electron transfer from NADH oxidation to H2 production in the ethanol-producing strain. Therefore, reversible hydrogenase activity confers the ability and flexibility to balance the intracellular redox state of M. thermoacetica. Tuning of the redox balance is required in order to benefit from H2-supplemented mixotrophy, which was confirmed by engineering to produce acetone.
There is a growing interest in chemical production derived from sources other than fossil fuels. Due to increasing levels of carbon dioxide (CO2) in the atmosphere, low-carbon emissions are required to eliminate environmental threats, such as global warming. Technology to capture and utilize CO2 as a resource is in progress worldwide, and bioprocessing of renewable feedstocks is one promising candidate. However, economic cost is a bottleneck in bioprocessing applications of bulk chemicals. A means to reduce the cost is to maximize carbon conversion of feedstock to the product.
Acetogens are a group of microorganisms capable of autotrophic growth on CO2 and hydrogen (H2) and are thus promising chassis for utilizing CO2 by bioprocesses (Ljungdhal, 1986; Wood, 1991; Drake, 1994; Drake et al., 2008). The main product is acetate, but some acetogens produce other valuable chemicals, such as ethanol. These by-products can be utilized for industrial production from waste materials, such as off-gas from steel mills. This process, called gas fermentation, has attracted worldwide attention (Bengelsdorf et al., 2016; Liew et al., 2016; Bengelsdorf and Dürre, 2017; Teixeira et al., 2018; Omar et al., 2019; Jin et al., 2020; Kopke and Simpson, 2020; Bourgade et al., 2021; Fackler et al., 2021). On the other hand, acetogens are also capable of heterotrophic growth on various carbohydrate substrates and are good candidates for bioconversion of biomass to useful chemicals. Utilization of acetogens is especially effective for carbon utilization because processing by acetogens emits much less CO2 due to the nature of their CO2 fixation pathway. When acetogens metabolize hexose to acetate, two molecules of CO2 are produced, then reassimilated into the CO2 fixation pathway by utilizing reducing equivalents from glycolysis. Therefore, acetogens can theoretically convert one hexose molecule to three acetate molecules (Fontaine et al., 1942; Schuchmann and Müller, 2014, 2016).
Autotrophic and heterotrophic metabolism can be combined for mixotrophic growth, which enables the enhancement of carbon utilization and conversion of extra CO2 using H2 as the source of reducing power (Fast et al., 2015; Maru et al., 2018). Mixotrophy is a general trait of acetogens and is effective in fermentation, especially for products that are more reduced than acetate. A previous report succeeded in increasing overall metabolite yields by supplying H2 to sugar-based cultures of Clostridium ljungdahlii (Jones et al., 2016). In this case, a shift in the metabolite profile was observed by providing H2, with ethanol as the primary metabolite, over less-reduced products. H2 supply for the industrial applications of mixotrophic fermentation would be supported by the development of technology to provide CO2-free H2 using renewable energy-based approaches, such as water splitting, biomass gasification, and ammonia reforming (Hosseini and Wahid, 2016; Aryal et al., 2018). Thus, together with this technology development to provide H2, mixotrophic fermentation would contribute to the low-carbon emitting and economically feasible bioprocesses.
In addition to natural by-products, acetogens can also be engineered to produce chemicals other than acetate. Genetic engineering of acetogens is challenging because of their genetic barrier, such as restriction–modification systems and physical barriers by gram-positive cell walls; however, development of engineering tools has substantially improved the efficiency of engineering acetogens (Minton et al., 2016; Jin et al., 2020; Bourgade et al., 2021). It is also possible to apply metabolic engineering for pathway optimization to enhance the production of target metabolites. Metabolic engineering has begun to highlight the potential of acetogens for chemical production from CO2.
Moorella thermoacetica is a thermophilic acetogen (Drake and Daniel, 2004; Pierce et al., 2008). Due to its thermophilic nature, M. thermoacetica can be used to establish an advantageous bioprocess for the recovery of products, especially volatile chemicals (Taylor et al., 2009; Abdel-Banat et al., 2010; Basen and Müller, 2017; Redl et al., 2017). However, M. thermoacetica is categorized as a homoacetogen that produces acetate exclusively. Therefore, the metabolic pathway must be modified to produce other chemicals for industrial applications (Iwasaki et al., 2013, 2017; Kita et al., 2013). We previously succeeded in engineering M. thermoacetica to produce ethanol and acetone from sugars and syngas, as well as to enhance yields, by adjusting the carbon flux (Rahayu et al., 2017; Kato et al., 2021; Takemura et al., 2021a). Disruption of the major acetate production pathway enables near-exclusive ethanol production from sugars.
In this study, we attempted to apply H2-supplemented mixotrophy to enhance ethanol yield. Unexpectedly, we found that H2 supplementation inhibited the growth of a high-ethanol-producing strain. Metabolomic analysis revealed that the engineered strain balanced the intracellular redox status by producing H2 to oxidize NADH during heterotrophic growth. Reversible hydrogenase activity, which oxidizes H2 in the wild-type strain under standard conditions, plays a vital role in the redox maintenance of metabolically engineered strains. It is necessary to avoid this reverse reaction to fulfill H2-supplemented-mixotrophic bioproduction.
Moorella thermoacetica ATCC 39073 and its derivatives were used in this study (Table 1). Modified ATCC1754 PETC medium comprising 1.0 g of NH4Cl, 0.1 g of KCl, 0.2 g of MgSO4·7H2O, 0.8 g of NaCl, 0.1 g of KH2PO4, 0.02 g of CaCl2·2H2O, 2.0 g of NaHCO3, 10 ml of trace elements, 10 ml of Wolfe’s vitamin solution (Tanner, 1989), and 1.0 mg of resazurin/L of deionized water was used as the basal medium (Tanner et al., 1993). The pH of the solution was adjusted to 6.9. The medium was prepared anaerobically by boiling and cooling under an N2–CO2 (80:20) mixed-gas atmosphere. After cooling, the medium was dispensed into 125 ml glass culture vials (serum bottles) under an N2–CO2 mixed-gas atmosphere. The vials were crimp-sealed and autoclaved.
Before starting the culture, fructose, yeast extract, and L-cysteine·HCl·H2O were added to reach final concentrations of 2.0, 1.0, and 1.2 g/l, respectively. The final volume was adjusted to 50 ml with water. To provide H2, the headspace pressure in the vials was adjusted to 0.12 MPa by using N2–CO2 (80:20) mixed-gas. H2 gas was then injected at the desired pressure. For example, when 0.01 MPa of H2 was tested, the total pressure was adjusted to 0.13 MPa by the H2 gas injection. Cells were grown at 55°C with shaking at 180 rpm.
We sampled and analyzed 1 ml of the culture medium at each time point and calculated the dry cell weight using the optical density (OD) at 600 nm [OD600; 1 g (dry cell weight)/L = 0.383 OD600; Iwasaki et al., 2017]. The culture supernatant was analyzed for the amount of fructose, formate, acetate, ethanol, and acetone using high-performance liquid chromatography (HPLC; LC-2000 Plus HPLC; Jasco, Tokyo, Japan) equipped with a refractive index detector (RI-2031 Plus; Jasco), Shodex RSpak KC-811 column (Showa Denko, Kanagawa, Japan), and Shodex RSpak KC-G guard column (Showa Denko) at 60°C. Ultrapure water containing 0.1% (v/v) phosphoric acid was used as the mobile phase at a flow rate of 0.7 ml/min, and crotonate was used as the internal standard (Miura et al., 2014). The gas composition in the headspace of the culture vials was analyzed using GC-8A gas chromatograph (Shimadzu, Kyoto, Japan) equipped with a thermal conductivity detector and a stainless steel column packed with activated carbon at 70°C. Argon was used as the carrier gas (Miura et al., 2014). The total gas pressure in the headspace was measured using a differential pressure gauge (DMC-104 N11; Okano Works, Tokyo, Japan).
Strains were grown to reach the exponential phase between 0.5 and 0.7 OD600. The culture was immediately filtered to collect cells equivalent to a total count of 20 OD600 (volume [mL] × OD600 ≈ 20). Filtration was performed using hydrophilic PTFE, 1 μm pore size, and a 90-mm-diameter filter disk (Omnipore; Merck KGaA, Darmstadt, Germany). Harvested cells were immediately immersed in pre-chilled methanol containing 100 μM ribitol and 100 μM (+)-10-camphorsulfonate to quench the metabolic activity. This procedure was quickly performed, within 45 s after opening culture vials, to avoid metabolites from artifacts, such as those caused by oxygenation, degradation, and other modifications. Subsequently, intracellular metabolites were extracted using the chloroform–water–methanol method (Bolten et al., 2007). The supernatant was then concentrated using a centrifugal concentrator (CC-105; Tomy, Tokyo, Japan). According to a previous study, pre-treatment and analysis of the dried samples were performed (Wada et al., 2022).
Moorella thermoacetica can convert one hexose molecule to three acetate molecules in theory (Figure 1A; Fontaine et al., 1942; Schuchmann and Müller, 2014, 2016). The engineered strains were designed to produce ethanol from acetyl-CoA in two steps (reducing reactions; Rahayu et al., 2017; Table 1). The reducing equivalents provided by glycolysis were assumed to be properly consumed (Figures 1A,B). In a model, the Ech complex, HydABC complex, and NfnAB complex would convert the reducing equivalents to NADH and NADPH. These NADH and NADPH would be consumed by the Wood–Ljungdahl pathway to convert CO2 to acetate in the wild-type strain, whereas reduction of acetyl-CoA would consume the NADH and NADPH in the ethanol-producing strains. Therefore, the redox conditions in the engineered strains producing ethanol should be balanced. In fact, we previously observed that all engineered strains (Table 1) grew and produced ethanol on hexose sugars. However, one molecule of CO2 is released to produce one molecule of ethanol because of the requirement for extra reducing equivalents (approximately 33% of the carbon is released from hexose sugars in theory). Therefore we supplied H2 to increase carbon utilization by mixotrophy.
Figure 1. Redox-balanced pathways for acetogenesis (A), ethanol production (B), and acetone production (C) from hexose in wild-type and engineered strains for ethanol production. NADH (shown in blue) and the reduced forms of ferredoxin are produced during glycolysis and the conversion of pyruvate to acetyl-CoA. The reduced ferredoxin is converted to NADPH (shown in green) via hydrogenases and electron-bifurcating enzymes, that is, the Ech complex, HydABC, and NfnAB complexes (shown in orange).
We used a culture containing fructose as the carbohydrate substrate. H2 was added to the headspace of the culture vial at a partial pressure of 0.08 MPa (equivalent to 40% of the gas phase). The gas phase also contained CO2, and therefore extra CO2 could be incorporated in addition to the released CO2. Of the injected gas, CO2 was 12% of the total, and the medium contained NaHCO3 to supply CO2. First, we tested the effect of H2 on the wild-type strain. Acetate was produced as the end product and the carbon molar yield improved from 0.74 to 0.82, as expected (Figures 2A,B). The optical density increased similarly while fructose was consumed, and decreased after the complete consumption of fructose in both conditions, indicating no significant effect on the growth (Figure 2C). We then tested an ethanol-producing strain, Mt-aldh, in which the aldh gene encoding aldehyde dehydrogenase was expressed by a constitutive promoter. The main product was acetate, accompanied by a small amount of ethanol. This trend was similar in the H2-supplied culture, and yield improvement was only observed for acetate production from 0.78 to 0.88 (Figure 2A). The change in ethanol production was not significant (0.02 and 0.03; Figure 2B). No significant effect on the growth was observed (Figure 2D). Although we expected to enhance the yield of reduced products, we reasoned that the abundant activity of the acetate production pathway in the Mt-aldh strain decreased the effect of H2. We then tested another ethanol-producing strain, Mt-ΔpduL2::aldh, which showed less acetate production due to deletion of one of the two genes (pduL2) encoding phosphoacetyl transferase in the acetate production pathway. Despite the dominant production of ethanol over acetate, product yield enhancement was observed with only acetate from 0.19 to 0.25 (Figures 2A,B). The carbon molar yield for ethanol did not change (0.37). The rest was released as CO2. The strain grew similarly in both conditions (Figure 2E). The effect of H2 was in contrast to the results of a previous study, in which the supplementation of reducing power with H2 was reflected in the production of more-reduced chemicals (Jones et al., 2016).
Figure 2. Effect of H2 supplementation on the wild-type strain and the engineered strains for ethanol production in mixotrophic conditions. (A,B) Product profiles shown as carbon molar yields (CM CFructose−1; CMetabolite CFructose−1). Fructose was used as the carbohydrate substrate. The amount of acetate and ethanol production was compared with and without supplementation of H2 (0.08 MPa partial pressure, 40% in the headspace) after 60 h when all the supplied fructose was consumed in the no H2-supplied condition. t-test was performed to evaluate the significance. N.S., not significant; value of *p<0.05; value of **p<0.01. (C- F) Profiles for cell growth and fructose consumption. The culture was same as in (A,B). Cell density was shown as OD600 (optical density at 600 nm) by solid lines. Fructose concentration was shown by dotted lines. Black, no H2-supplied condition; red, H2-supplied condition. (G) Growth profiles of the Mt-ΔpduL1ΔpduL2::aldh strain with various H2 partial pressures. The profiles of the exponential phase were compared. The standard deviation (SD) of three biological replicates is shown by error bars. Some error bars are smaller than the symbols. (H) Specific growth rate plotted against H2 partial pressure. All data shown were obtained in the culture for (G).
We tested the Mt-ΔpduL1ΔpduL2::aldh strain, which produces almost exclusively ethanol, because of the complete knockout of both two genes (pduL1 and pduL2) encoding phosphoacetyl transferase. In this case, we observed an enhancement in the ethanol yield. The carbon molar yield for ethanol was increased from 0.47 to 0.54 (Figure 2B). Approximately 50% of the carbon from fructose was released as CO2 in the absence of H2, and H2 supplementation supported capture and conversion of CO2. However, the supplemented fructose was not completely consumed in the H2-supplied condition after 60 h of cultivation, whereas the same strain in the H2-unsupplied condition, or the other strains in both conditions, consumed fructose completely (Figures 2C–F). Only 40.2% of the supplemented fructose was consumed in the H2-supplied condition, and the growth was significantly reduced in a correlated manner (Figure 2F). The volumetric amount of ethanol was also significantly less in the H2-supplied condition, showing only 45.5% against the no-H2 condition (17.6 mM in the absence of H2 and 8.0 mM in the presence of H2), reflecting the amount of consumed fructose. Therefore, although the Mt-ΔpduL1ΔpduL2::aldh strain showed increased carbon molar yield for ethanol under H2-supplemented mixotrophic conditions, growth inhibition emerged as an unexpected bottleneck.
We also tested the addition of different amounts of H2 to the Mt-ΔpduL1ΔpduL2::aldh strain. In addition to the condition of partial pressure 0.08 MPa, we tested 0.04, 0.02, and 0.01 MPa, because the pressure of H2 is correlated with dissolved H2 in the culture medium. All cases with H2 at any concentration showed growth inhibition effects. Interestingly, the effect of growth inhibition was dose-dependent, showing more potent inhibition by a higher concentration of H2 in the culture medium, rather than by a certain threshold. This tendency was clear when the growth rate was plotted against the H2 pressure, showing a linear correlation (Figure 2H).
To investigate the H2-dependent growth inhibition mechanism of the ethanol-producing strain, we assessed intracellular metabolism by metabolome analysis. We used H2 at 0.02 MPa, because a high dose of H2 (such as 0.08 MPa) inhibited the growth almost completely, and hence might have significant effects on multiple metabolic pathways. We sampled the cells from the exponential phase and analyzed intracellular metabolites using GC–MS and LC–MS following the sample preparation method we developed. We succeeded in quantifying 19 intracellular metabolites, including seven cofactors (Figures 3A,B).
Figure 3. Metabolome analysis of the Mt-ΔpduL1ΔpduL2::aldh strain and the wild-type strain with and without H2 supplementation. (A) Metabolic pathways for the Moorella thermoacetica strains based on KEGG database (https://www.genome.jp/kegg-bin/show_organism?org=mta) and a previous study on a metabolic model (Islam et al., 2015). Metabolites quantified except for cofactors are shown by red squares. EMP, Embden–Meyerhof–Parnas pathway; PPP, pentose phosphate pathway; Fru, fructose; F1P, fructose 1-phosphate; G6P, glucose-6-phosphate; F6P, fructose 6-phosphate; FBP, fructose-1,6-bisphosphate; GAP, glyceraldehyde 3-phosphate; DHAP, dihydroxyacetone phosphate; 1,3BPG, 1,3-bisphosphoglyceric acid; 3PG, 3-phosphoglyceric acid; PEP, phosphoenolpyruvic acid; PYR, pyruvate; X5P, xylulose 5-phosphate; RU5P, ribulose 5-phosphate; R5P, ribose 5-phosphate; S7P, sedoheptulose 7-phosphate; E4P, erythrose 4-phosphate; Ac-CoA, Acetyl-CoA; Ac-P, acetyl phosphate; Acal, acetaldehyde; CIT, citrate; ICIT, isocitrate; αKG, alpha-ketoglutarate; SUC-CoA, succinyl-CoA; SUC, succinate; Glu, glutamate; OAA, oxaloacetic acid; MAL, malate; FUM, fumarate; ASP, aspartate; GAP, glyceraldehyde 3-phosphate. (B) Relative concentrations of intracellular metabolites for the Mt-ΔpduL1ΔpduL2::aldh strain and the wild-type strain in the absence (black) and presence of H2 (0.02 MPa, red) on fructose as the carbohydrate substrate. The experiment with DMSO supplementation in addition to H2 is shown in orange. All the cell samples were collected at around 0.6 of OD600. DMSO was added at 0.4 of OD600. For each sample, values were normalized to the Mt-ΔpduL1ΔpduL2::aldh strain without H2 supplementation. The vertical axis represents a unitless ratio of metabolite concentrations. Error bars represent the SD of at least two biological replicates. Red. Equiv. represents reducing equivalents. t-test was performed to evaluate the significance between data sets 1 and 2, data sets 3 and 4, and data sets 1 and 3. Significant differences are shown. value of *p<0.05; value of **p<0.01.
We then compared the Mt-ΔpduL1ΔpduL2::aldh strain and the wild-type strain, with or without H2 supplementation. Among all analyzed metabolites, NADH levels were striking in the Mt-ΔpduL1ΔpduL2::aldh strain under H2-supplied conditions. The NADH level increased by approximately four times compared to that in the no H2 condition, whereas H2 supplementation did not affect the NADH level in the wild-type strain. There was no such significant difference specific to H2 supplementation in the other metabolites in the Mt-ΔpduL1ΔpduL2::aldh strain or metabolites in the wild-type strain (Figure 3B).
When the overall metabolite profiles were compared in the Mt-ΔpduL1ΔpduL2::aldh and wild-type strains in the no H2 condition, the levels of glucose-6-phosphate, fructose-6-phosphate, fructose-1,6-bisphosphate, 3-phosphoglyceric acid, glutamate, and ATP were lower, and the levels of acetyl-CoA, AMP, and NADPH were higher in the Mt-ΔpduL1ΔpduL2::aldh strain. The ATP level was lowered in the Mt-ΔpduL1ΔpduL2::aldh due to the knockout of acetate production coupled with substrate-level phosphorylation, but the ATP level was enough to maintain the growth (Figure 2F). In contrast, the AMP level was higher in the Mt-ΔpduL1ΔpduL2::aldh, and this may be related with the change of ATP level. The higher level of acetyl-CoA probably reflects a difference of conversion rate of acetyl-CoA to ethanol and acetate. The higher level of NADPH in the Mt-ΔpduL1ΔpduL2::aldh strain suggests that the redox balance in this strain was altered by metabolic engineering. Metabolome analysis indicated that the Mt-ΔpduL1ΔpduL2::aldh strain suffered redox imbalances due to both metabolic engineering and H2 supplementation.
Metabolomic analysis suggested a strong relationship between growth inhibition by H2 and increased levels of intracellular NADH. In M. thermoacetica, NAD+ is reduced to NADH by the electron-bifurcating hydrogenase HydABC complex (Wang et al., 2013). The HydABC complex reduces NAD+ and ferredoxin using electrons from H2. This reaction is reversible and produces H2 from NADH and reduced ferredoxin in vitro. Therefore, we measured the amount of H2 in the headspace of culture vials (Figure 4). The amount of H2 in the headspace was traced in conditions with or without H2 supplementation, and the wild-type strain and the Mt-ΔpduL1ΔpduL2::aldh strain were compared. H2 was supplied at 0.02 MPa of a partial pressure, in addition to fructose as a carbohydrate substrate, which was the same condition for our metabolome analysis. There was almost no H2 production by the wild-type strain, and the supplied H2 was consumed over time (Figure 4A). In contrast, the Mt-ΔpduL1ΔpduL2::aldh strain apparently did not consume H2 under the same conditions (Figure 4B). Moreover, when H2 was not supplied, the H2 level increased in the culture vial of the Mt-ΔpduL1ΔpduL2::aldh strain, in contrast to that in the wild-type strain. H2 production is usually attributed to the disposal of excess electrons from metabolism. In this case, this was most likely due to the increased level of NADH. However, H2 formation would require a sufficiently low level of H2 as the product. Therefore, the Mt-ΔpduL1ΔpduL2::aldh strain would have produced H2 for the clearance of the excess electrons from catabolizing fructose, and H2 supplementation would inhibit the H2 production, causing the growth inhibition due to the redox imbalance.
Figure 4. Monitoring the consumption and evolution of H2 by the wild-type strain (A) and the Mt-ΔpduL1ΔpduL2::aldh strain (B). The total amount of H2 was divided by the volume of the culture medium. Black, no H2-supplied condition; red, H2-supplied condition (0.02 MPa). Error bars, which are smaller than symbols, are the SD of three biological replicates.
The results of the H2 measurement strongly indicated that the Mt-ΔpduL1ΔpduL2::aldh strain produced H2 using excess reducing equivalents and balanced the intracellular redox. Our metabolome analysis showed imbalanced redox in the Mt-ΔpduL1ΔpduL2::aldh strain, manifested as an increased level of intracellular NADH. If a high level of intracellular NADH is the direct cause of growth inhibition, the Mt-ΔpduL1ΔpduL2::aldh strain should recover its growth in the presence of H2 by lowering the intracellular NADH level. M. thermoacetica uses dimethyl sulfoxide (DMSO) as an electron acceptor, and the reported cases of bacterial DMSO reduction are NADH-dependent reactions (Zinder and Brock, 1978; De Bont et al., 1981; Drake and Daniel, 2004; Takemura et al., 2021b; Rosenbaum et al., 2022). We attempted to oxidize intracellular NADH via DMSO reduction by supplementing the culture medium with DMSO. We set up a culture of the Mt-ΔpduL1ΔpduL2::aldh strain with H2 supplied at 0.08 MPa of partial pressure in addition to fructose, which showed strong growth inhibition (Figures 2F–H). At 24 h, the culture was supplied with 10 mM DMSO. The Mt-ΔpduL1ΔpduL2::aldh strain showed very slow growth with H2 supplementation, but the growth rate dramatically increased upon DMSO supplementation (Figure 5A). The fructose supplied was completely consumed after 55 h (Figure 5B). The supplied DMSO was readily consumed within 20 h after the DMSO addition, consistent with the recovery of growth and fructose consumption (Figure 5C). The addition of DMSO also improved total ethanol production, whereas acetate production remained minor (Figures 5D,E).
Figure 5. Effect of DMSO supplementation on the culture profile in the H2-supplemented mixotrophic condition of the Mt-ΔpduL1ΔpduL2::aldh strain. H2 (0.08 MPa) was supplied to the fructose-supplemented culture, and DMSO (10 mM) was added to one group of the culture medium after 24 h (shown by arrows). The DMSO-supplied condition is shown in blue, whereas the no-DMSO condition is shown in red. Each graph shows the culture profiles for (A), OD600; (B), Fructose concentration; (C), DMSO concentration; (D), Ethanol production; (E), Acetate production. Error bars represent the SD of three biological replicates.
We analyzed the effect of DMSO on the intracellular metabolome in the presence of H2 (Figure 3B). We used the same culture conditions as in the metabolome analysis (H2 partial pressure = 0.02 MPa), except for DMSO supplementation, which was provided when the cells entered the exponential phase. As expected, the intracellular level of NADH was lower than that in the H2 condition. Therefore, growth recovery correlated with intracellular NADH levels.
We found that the ethanol production pathway designed to balance the redox reaction requires tuning the imbalanced redox by producing H2. This means that our ethanol-producing strains need to be re-engineered to balance the redox reaction to benefit from H2-supplemented mixotrophy. However, it is possible that artificial modifications in the genome can affect metabolic activities in an unpredictable manner. We previously succeeded in engineering M. thermoacetica to produce acetone (Kato et al., 2021; Table 1). The acetone synthesis pathway does not require any oxidoreductases to convert acetyl-CoA to acetone, which has the same redox balance as that of the native acetate pathway (Figure 1C). Therefore, acetone production has completely the same redox balance as acetate production, and the redox balance should not be affected. On the other hand, the pduL2::acetone strain has the same elements of genetic modification as an ethanol-producing strain, Mt-ΔpduL2::aldh, using a pyrF marker for selection and a constitutive G3PD (glyceraldehyde 3-phosphate dehydrogenase) promoter to express aldh, disrupting the acetate pathway. Therefore, we examined whether the introduction of an oxidoreductase affected the redox balance by testing the H2-supplemented mixotrophic acetone production of the pduL2::acetone strain.
We set up cultures of the pduL2::acetone strain with fructose as the carbohydrate substrate and tested the effect of H2 supplementation, as was performed for ethanol-producing strains. H2 was supplied at 0.08 MPa of partial pressure, the highest dose used for the ethanol-producing strains. The pduL2::acetone strain grew in the presence of H2 in the same manner as in its absence (Figure 6A). The optical density increased while fructose was consumed, and decreased after the complete consumption of fructose in both cases. Furthermore, the produced acetone and acetate increased with H2 supplementation (Figure 6B), in contrast to ethanol production. Acetone production was enhanced by 13%, and the total carbon molar yield (the sum of acetate and acetone) was greater than one, indicating that extra CO2 was converted to these metabolites. CO2 was externally provided in the headspace gas and from NaHCO3 in the medium, in addition to CO2 released from metabolism. Engineering to introduce a non-reductive pathway did not erase the effect of mixotrophy. Therefore, we concluded that introducing oxidoreductase reactions to convert acetyl-CoA to ethanol caused H2 production by extra electrons and H2 inhibition.
Figure 6. Culture profiles of the metabolically engineered strain of Moorella thermoacetica for acetone production in the H2-supplemented mixotrophic condition. (A) shows cell growth (solid lines) and fructose consumption (dotted lines) in the absence (black) and presence (red) of H2. (B) shows carbon molar yields for acetate and acetone production. Error bars represent the SD of three biological replicates, some of which are smaller than symbols.
Metabolic engineering to divert acetate to more reduced chemicals lowers carbon utilization and releases more CO2 in acetogens due to the loss of the reducing power to fix and convert CO2 for oxidoreductase reactions. One strategy for overcoming this issue is H2-supplemented mixotrophy. However, our engineered strains, Mt-aldh and Mt-ΔpduL2::aldh, produced an increased amount of acetate instead of ethanol. Growth inhibition was observed in the Mt-ΔpduL1ΔpduL2::aldh strain. Therefore, the strategy for enhancing carbon utilization by H2 is not effective for metabolically engineered strains in terms of ethanol production.
Growth inhibition due to H2 supplementation has been reported in several studies. For example, one classical case involves Clostridium cellobioparum, an H2 producer. When H2 was added to the culture, C. cellobioparum growth was inhibited in an H2 dose-dependent manner (Chung, 1976). This trend was similar to that of our ethanol-producing strain, Mt-ΔpduL1ΔpduL2::aldh. The study also reported that the removal of H2 recovered the growth using a catalyst, gassing out, or co-culture with methanogenic microorganisms. C. cellobioparum is a resident of the bovine rumen, living together with methanogens; therefore, the H2 level remains low and does not affect their growth in situ (Hungate, 1967; Hungate et al., 1970). Another example was observed in the case of a thermophilic microorganism for H2 bioproduction, Caldicellulosiruptor saccharolyticus (Willquist et al., 2011). A high level of H2 produced by C. saccharolyticus inhibits its own growth, demanding continuous stripping of the produced H2 from fermentation. Interestingly, the desired by-product for high H2 yields is acetate, because more-reduced products, such as ethanol, drain electrons from H2 production. When H2 levels increase, C. saccharolyticus produces lactate and ethanol instead of H2 to oxidize NADH and maintain the NADH/NAD ratio. In contrast, our metabolically engineered ethanol-producing strain of M. thermoacetica produced H2 instead of ethanol to oxidize NADH. M. thermoacetica has been reported to evolve H2 under certain conditions, such as in a CO-supplemented culture with glucose (Martin et al., 1983). The intracellular activity level of hydrogenases is significantly enhanced by CO, but not by other gas phases, including H2 (Kellum and Drake, 1984). M. thermoacetica does not evolve H2 in a standard culture under heterotrophic conditions, as seen in our experiment.
Metabolomic analysis revealed that the Mt-ΔpduL1ΔpduL2::aldh strain could maintain NADH levels in the absence of H2. This was due to H2 production for NADH oxidation, and might also be due to NADPH production using the NfnAB complex. The NfnAB complex transfers electrons from reduced ferredoxin and NADH to NADP+ (Huang et al., 2012). However, because the Mt-ΔpduL1ΔpduL2::aldh strain possesses a high NADPH level, the conversion of reduced ferredoxin and NADH to NADPH would be inhibited or difficult. It is unclear why the basal level of NADPH in the Mt-ΔpduL1ΔpduL2::aldh strain was higher than that in the wild-type strain. One possibility is the slow conversion of acetyl-CoA to ethanol, because metabolome analysis showed that the intracellular level of acetyl-CoA was higher in the Mt-ΔpduL1ΔpduL2::aldh strain (Figure 3B). The reaction speed may have limited the consumption of cofactors NADH and NADPH, and the cofactors of the reduced forms accumulated. Although NADH production can be balanced by H2 formation, NADPH production cannot be balanced. The Mt-ΔpduL1ΔpduL2::aldh strain could grow and produce ethanol in non-H2-supplemented conditions, but NADH could not be balanced upon H2 supplementation due to the blockage of H2 production. Another possibility is unregulated NADPH production by the pentose phosphate pathway caused by unknown mechanisms due to metabolic engineering. Although a high level of NADPH remained, even in the presence of DMSO to consume NADH under H2-supplemented conditions (Figure 3B), the NADPH level did not inhibit growth. Growth inhibition was caused by high NADH levels. We assume that the target reaction influenced by NADH level is glyceraldehyde 3-phosphate dehydrogenase, because this is NADH-dependent (Thauer, 1972; Huang et al., 2012). In studies on ethanol tolerance, glyceraldehyde 3-phosphate dehydrogenase was found to be inhibited by high levels of NADH in Clostridium thermocellum (Tian et al., 2017).
Finally, we confirmed that the increased acetate formation, but not ethanol formation, was due to the redox balance itself in the Mt-ΔpduL2::aldh strain. An engineered strain for acetone production produced higher levels of acetate and acetone with H2 supplementation. Therefore, our strategy for gene manipulation itself did not affect redox balance, and the usefulness of H2-supplemented mixotrophy was confirmed. The introduction of oxidoreductases affected the redox balance in ethanol-producing strains; hence, H2 supplementation only enhanced acetate or inhibited growth. Although the metabolic pathway was designed to be redox-balanced by choosing oxidoreductases with appropriate cofactors (Figure 1), fine-tuning and a different design for properly balanced redox is required to derive benefits from H2-supplemented mixotrophy for ethanol production. In contrast, if H2 production is the aim of engineering, a metabolic design to increase the intracellular NADH level is one strategy to exploit the hydrogenase reaction.
The original contributions presented in the study are included in the article/supplementary material, and further inquiries can be directed to the corresponding author.
JK and YN conceived and designed the experiments. ShK, JK, and KW performed the experiments. ShK, JK, KW, KT, SeK, TF, YI, YA, TM, AM, and KM analyzed the data. ShK, JK, and KW visualized the data. JK prepared the manuscript. YN supervised the project. All authors have contributed to the manuscript and approved the submitted version.
Part of this work was supported by JSPS KAKENHI Grant Number 18 K04853 and JST-Mirai Program Grant Number JPMJMI18E5, Japan.
The authors declare that the research was conducted in the absence of any commercial or financial relationships that could be construed as potential conflicts of interest.
All claims expressed in this article are solely those of the authors and do not necessarily represent those of their affiliated organizations, or those of the publisher, the editors and the reviewers. Any product that may be evaluated in this article, or claim that may be made by its manufacturer, is not guaranteed or endorsed by the publisher.
We thank Hikaru Miyaoka for providing technical assistance.
Abdel-Banat, B. M., Hoshida, H., Ano, A., Nonklang, S., and Akada, R. (2010). High-temperature fermentation: how can processes for ethanol production at high temperatures become superior to the traditional process using mesophilic yeast? Appl. Microbiol. Biotechnol. 85, 861–867. doi: 10.1007/s00253-009-2248-5
Aryal, N., Kvist, T., Ammam, F., Pant, D., and Ottosen, L. D. M. (2018). An overview of microbial biogas enrichment. Bioresour. Technol. 264, 359–369. doi: 10.1016/j.biortech.2018.06.013
Basen, M., and Müller, V. (2017). “Hot” acetogenesis. Extremophiles 21, 15–26. doi: 10.1007/s00792-016-0873-3
Bengelsdorf, F. R., and Dürre, P. (2017). Gas fermentation for commodity chemicals and fuels. Microb. Biotechnol. 10, 1167–1170. doi: 10.1111/1751-7915.12763
Bengelsdorf, F. R., Poehlein, A., Linder, S., Erz, C., Hummel, T., Hoffmeister, S., et al. (2016). Industrial acetogenic biocatalysts: a comparative metabolic and genomic analysis. Front. Microbiol. 7:1036. doi: 10.3389/fmicb.2016.01036
Bolten, C. J., Kiefer, P., Letisse, F., Portais, J. C., and Wittmann, C. (2007). Sampling for metabolome analysis of microorganisms. Anal. Chem. 79, 3843–3849. doi: 10.1021/ac0623888
Bourgade, B., Minton, N. P., and Islam, M. A. (2021). Genetic and metabolic engineering challenges of C1-gas fermenting acetogenic chassis organisms. FEMS Microbiol. Rev. 45:fuab008. doi: 10.1093/femsre/fuab008
Chung, K. T. (1976). Inhibitory effects of H2 on growth of clostridium cellobioparum. Appl. Environ. Microbiol. 31, 342–348. doi: 10.1128/aem.31.3.342-348.1976
De Bont, J. A. M., Van Dijken, J. P., and Harder, W. (1981). Dimethyl sulphoxide and dimethyl sulphide as a carbon, Sulphur and energy source for growth of Hyphomicrobium S. Microbiology 127, 315–323. doi: 10.1099/00221287-127-2-315
Drake, H. L., and Daniel, S. L. (2004). Physiology of the thermophilic acetogen Moorella thermoacetica. Res. Microbiol. 155, 869–883. doi: 10.1016/j.resmic.2004.10.002
Drake, H. L., Gossner, A. S., and Daniel, S. L. (2008). Old acetogens, new light. Ann. N. Y. Acad. Sci. 1125, 100–128. doi: 10.1196/annals.1419.016
Fackler, N., Heijstra, B. D., Rasor, B. J., Brown, H., Martin, J., Ni, Z., et al. (2021). Stepping on the gas to a circular economy: accelerating development of carbon-negative chemical production from gas fermentation. Annu. Rev. Chem. Biomol. Eng 12, 439–470. doi: 10.1146/annurev-chembioeng-120120-021122
Fast, A. G., Schmidt, E. D., Jones, S. W., and Tracy, B. P. (2015). Acetogenic mixotrophy: novel options for yield improvement in biofuels and biochemicals production. Curr. Opin. Biotechnol. 33, 60–72. doi: 10.1016/j.copbio.2014.11.014
Fontaine, F. E., Peterson, W. H., Mccoy, E., Johnson, M. J., and Ritter, G. J. (1942). A new type of glucose fermentation by Clostridium thermoaceticum. J. Bacteriol. 43, 701–715. doi: 10.1128/JB.43.6.701-715.1942
Hosseini, S. E., and Wahid, M. A. (2016). Hydrogen production from renewable and sustainable energy resources: promising green energy carrier for clean development. Renew. Sustain. Energy Rev. 57, 850–866. doi: 10.1016/j.rser.2015.12.112
Huang, H., Wang, S., Moll, J., and Thauer, R. K. (2012). Electron bifurcation involved in the energy metabolism of the acetogenic bacterium Moorella thermoacetica growing on glucose or H2 plus CO2. J. Bacteriol. 194, 3689–3699. doi: 10.1128/JB.00385-12
Hungate, R. E. (1967). Hydrogen as an intermediate in the rumen fermentation. Arch. Mikrobiol. 59, 158–164. doi: 10.1007/BF00406327
Hungate, R. E., Smith, W., Bauchop, T., Yu, I., and Rabinowitz, J. C. (1970). Formate as an intermediate in the bovine rumen fermentation. J. Bacteriol. 102, 389–397. doi: 10.1128/jb.102.2.389-397.1970
Islam, M. A., Zengler, K., Edwards, E. A., Mahadevan, R., and Stephanopoulos, G. (2015). Investigating Moorella thermoacetica metabolism with a genome-scale constraint-based metabolic model. Integr. Biol. 7, 869–882. doi: 10.1039/c5ib00095e
Iwasaki, Y., Kita, A., Sakai, S., Takaoka, K., Yano, S., Tajima, T., et al. (2013). Engineering of a functional thermostable kanamycin resistance marker for use in Moorella thermoacetica ATCC39073. FEMS Microbiol. Lett. 343, 8–12. doi: 10.1111/1574-6968.12113
Iwasaki, Y., Kita, A., Yoshida, K., Tajima, T., Yano, S., Shou, T., et al. (2017). Homolactic acid fermentation by the genetically engineered thermophilic homoacetogen Moorella thermoacetica ATCC 39073. Appl. Environ. Microbiol. 83, e00247–e00217. doi: 10.1128/AEM.00247-17
Jin, S., Bae, J., Song, Y., Pearcy, N., Shin, J., Kang, S., et al. (2020). Synthetic biology on acetogenic bacteria for highly efficient conversion of C1 gases to biochemicals. Int. J. Mol. Sci. 21:7639. doi: 10.3390/ijms21207639
Jones, S. W., Fast, A. G., Carlson, E. D., Wiedel, C. A., Au, J., Antoniewicz, M. R., et al. (2016). CO2 fixation by anaerobic non-photosynthetic mixotrophy for improved carbon conversion. Nat. Commun. 7:12800. doi: 10.1038/ncomms12800
Kato, J., Takemura, K., Kato, S., Fujii, T., Wada, K., Iwasaki, Y., et al. (2021). Metabolic engineering of Moorella thermoacetica for thermophilic bioconversion of gaseous substrates to a volatile chemical. AMB Express 11:59. doi: 10.1186/s13568-021-01220-w
Kellum, R., and Drake, H. L. (1984). Effects of cultivation gas phase on hydrogenase of the acetogen clostridium thermoaceticum. J. Bacteriol. 160, 466–469. doi: 10.1128/jb.160.1.466-469.1984
Kita, A., Iwasaki, Y., Sakai, S., Okuto, S., Takaoka, K., Suzuki, T., et al. (2013). Development of genetic transformation and heterologous expression system in carboxydotrophic thermophilic acetogen Moorella thermoacetica. J. Biosci. Bioeng. 115, 347–352. doi: 10.1016/j.jbiosc.2012.10.013
Kopke, M., and Simpson, S. D. (2020). Pollution to products: recycling of ‘above ground’ carbon by gas fermentation. Curr. Opin. Biotechnol. 65, 180–189. doi: 10.1016/j.copbio.2020.02.017
Liew, F., Martin, M. E., Tappel, R. C., Heijstra, B. D., Mihalcea, C., and Kopke, M. (2016). Gas fermentation-a flexible platform for commercial scale production of low-carbon-fuels and chemicals from waste and renewable feedstocks. Front. Microbiol. 7:694. doi: 10.3389/fmicb.2016.00694
Ljungdhal, L. G. (1986). The autotrophic pathway of acetate synthesis in acetogenic bacteria. Annu. Rev. Microbiol. 40, 415–450. doi: 10.1146/annurev.mi.40.100186.002215
Martin, D. R., Lundie, L. L., Kellum, R., and Drake, H. L. (1983). Carbon monoxide-dependent evolution of hydrogen by the homoacetate-fermenting bacterium Clostridium thermoaceticum. Curr. Microbiol. 8, 337–340. doi: 10.1007/bf01573705
Maru, B. T., Munasinghe, P. C., Gilary, H., Jones, S. W., and Tracy, B. P. (2018). Fixation of CO2 and CO on a diverse range of carbohydrates using anaerobic, non-photosynthetic mixotrophy. FEMS Microbiol. Lett. 365:fny039. doi: 10.1093/femsle/fny039
Minton, N. P., Ehsaan, M., Humphreys, C. M., Little, G. T., Baker, J., Henstra, A. M., et al. (2016). A roadmap for gene system development in Clostridium. Anaerobe 41, 104–112. doi: 10.1016/j.anaerobe.2016.05.011
Miura, T., Kita, A., Okamura, Y., Aki, T., Matsumura, Y., Tajima, T., et al. (2014). Evaluation of marine sediments as microbial sources for methane production from brown algae under high salinity. Bioresour. Technol. 169, 362–366. doi: 10.1016/j.biortech.2014.07.013
Omar, B., El-Gammal, M., Abou-Shanab, R., Fotidis, I. A., Angelidaki, I., and Zhang, Y. (2019). Biogas upgrading and biochemical production from gas fermentation: impact of microbial community and gas composition. Bioresour. Technol. 286:121413. doi: 10.1016/j.biortech.2019.121413
Pierce, E., Xie, G., Barabote, R. D., Saunders, E., Han, C. S., Detter, J. C., et al. (2008). The complete genome sequence of Moorella thermoacetica (f. Clostridium thermoaceticum). Environ. Microbiol. 10, 2550–2573. doi: 10.1111/j.1462-2920.2008.01679.x
Rahayu, F., Kawai, Y., Iwasaki, Y., Yoshida, K., Kita, A., Tajima, T., et al. (2017). Thermophilic ethanol fermentation from lignocellulose hydrolysate by genetically engineered Moorella thermoacetica. Bioresour. Technol. 245, 1393–1399. doi: 10.1016/j.biortech.2017.05.146
Redl, S., Sukumara, S., Ploeger, T., Wu, L., Olshoj Jensen, T., Nielsen, A. T., et al. (2017). Thermodynamics and economic feasibility of acetone production from syngas using the thermophilic production host Moorella thermoacetica. Biotechnol. Biofuels 10:150. doi: 10.1186/s13068-017-0827-8
Rosenbaum, F. P., Poehlein, A., Daniel, R., and Müller, V. (2022). Energy-conserving dimethyl sulfoxide reduction in the acetogenic bacterium Moorella thermoacetica. Environ Microbiol. doi: 10.1111/1462-2920.15971 [Epub ahead of print].
Schuchmann, K., and Müller, V. (2014). Autotrophy at the thermodynamic limit of life: a model for energy conservation in acetogenic bacteria. Nat. Rev. Microbiol. 12, 809–821. doi: 10.1038/nrmicro3365
Schuchmann, K., and Müller, V. (2016). Energetics and application of heterotrophy in acetogenic bacteria. Appl. Environ. Microbiol. 82, 4056–4069. doi: 10.1128/AEM.00882-16
Takemura, K., Kato, J., Kato, S., Fujii, T., Wada, K., Iwasaki, Y., et al. (2021b). Enhancing hydrogen-dependent autotrophic growth of the thermophilic acetogen Moorella thermoacetica by supplementation of dimethyl sulfoxide as an electron acceptor. SSRN [preprint] Available at: https://papers.ssrn.com/sol3/papers.cfm?abstract_id=3995298. (Accessed April 14, 2022)
Takemura, K., Kato, J., Kato, S., Fujii, T., Wada, K., Iwasaki, Y., et al. (2021a). Autotrophic growth and ethanol production enabled by diverting acetate flux in the metabolically engineered Moorella thermoacetica. J. Biosci. Bioeng. 132, 569–574. doi: 10.1016/j.jbiosc.2021.08.005
Tanner, R. S. (1989). Monitoring sulfate-reducing bacteria: comparison of enumeration media. J. Microbiol. Methods 10, 83–90. doi: 10.1016/0167-7012(89)90004-3
Tanner, R. S., Miller, L. M., and Yang, D. (1993). Clostridium ljungdahlii sp. nov., an acetogenic species in clostridial rRNA homology group I. Int. J. Syst. Bacteriol. 43, 232–236. doi: 10.1099/00207713-43-2-232
Taylor, M. P., Eley, K. L., Martin, S., Tuffin, M. I., Burton, S. G., and Cowan, D. A. (2009). Thermophilic ethanologenesis: future prospects for second-generation bioethanol production. Trends Biotechnol. 27, 398–405. doi: 10.1016/j.tibtech.2009.03.006
Teixeira, L. V., Moutinho, L. F., and Romão-Dumaresq, A. S. (2018). Gas fermentation of C1 feedstocks: commercialization status and future prospects. Biofuels Bioprod. Biorefin. 12, 1103–1117. doi: 10.1002/bbb.1912
Thauer, R. K. (1972). CO2-reduction to formate by NADPH. The initial step in the total synthesis of acetate from CO2 in clostridium thermoaceticum. FEBS Lett. 27, 111–115. doi: 10.1016/0014-5793(72)80421-6
Tian, L., Perot, S. J., Stevenson, D., Jacobson, T., Lanahan, A. A., Amador-Noguez, D., et al. (2017). Metabolome analysis reveals a role for glyceraldehyde 3-phosphate dehydrogenase in the inhibition of C. thermocellum by ethanol. Biotechnol. Biofuels 10:276. doi: 10.1186/s13068-017-0961-3
Wada, K., Saika, A., Ushimaru, K., Sato, S., Fukuoka, T., and Morita, T. (2022). Metabolomic evaluation of the central metabolic pathways of mannosylerythritol lipid biosynthesis in Moesziomyces antarcticus T-34. J. Oleo Sci. 71, 119–125. doi: 10.5650/jos.ess21229
Wang, S., Huang, H., Kahnt, J., and Thauer, R. K. (2013). A reversible electron-bifurcating ferredoxin- and NAD-dependent [FeFe]-hydrogenase (HydABC) in Moorella thermoacetica. J. Bacteriol. 195, 1267–1275. doi: 10.1128/JB.02158-12
Willquist, K., Pawar, S. S., and Van Niel, E. W. (2011). Reassessment of hydrogen tolerance in Caldicellulosiruptor saccharolyticus. Microb. Cell Factories 10:111. doi: 10.1186/1475-2859-10-111
Wood, H. G. (1991). Life with CO or CO2 and H2 as a source of carbon and energy. FASEB J. 5, 156–163. doi: 10.1096/fasebj.5.2.1900793
Keywords: acetogen, metabolic engineering, ethanol production, hydrogen inhibition, hydrogen production, redox balance, mixotrophy
Citation: Kobayashi S, Kato J, Wada K, Takemura K, Kato S, Fujii T, Iwasaki Y, Aoi Y, Morita T, Matsushika A, Murakami K and Nakashimada Y (2022) Reversible Hydrogenase Activity Confers Flexibility to Balance Intracellular Redox in Moorella thermoacetica. Front. Microbiol. 13:897066. doi: 10.3389/fmicb.2022.897066
Received: 15 March 2022; Accepted: 19 April 2022;
Published: 12 May 2022.
Edited by:
Constanze Pinske, Martin Luther University of Halle-Wittenberg, GermanyReviewed by:
Gary Sawers, Martin Luther University of Halle-Wittenberg, GermanyCopyright © 2022 Kobayashi, Kato, Wada, Takemura, Kato, Fujii, Iwasaki, Aoi, Morita, Matsushika, Murakami and Nakashimada. This is an open-access article distributed under the terms of the Creative Commons Attribution License (CC BY). The use, distribution or reproduction in other forums is permitted, provided the original author(s) and the copyright owner(s) are credited and that the original publication in this journal is cited, in accordance with accepted academic practice. No use, distribution or reproduction is permitted which does not comply with these terms.
*Correspondence: Yutaka Nakashimada, bnl1dGFrYUBoaXJvc2hpbWEtdS5hYy5qcA==
†These authors have contributed equally to this work and share first authorship
Disclaimer: All claims expressed in this article are solely those of the authors and do not necessarily represent those of their affiliated organizations, or those of the publisher, the editors and the reviewers. Any product that may be evaluated in this article or claim that may be made by its manufacturer is not guaranteed or endorsed by the publisher.
Research integrity at Frontiers
Learn more about the work of our research integrity team to safeguard the quality of each article we publish.