- 1College of Biology & Hunan Provincial Key Laboratory of Medical Virology, Hunan University, Changsha, China
- 2Yunnan Province Key Laboratory of Anti-pathogenic Plant Resources Screening (Cultivation), Yunnan Province Key University Laboratory of Zoonoses Cross-Border Prevention and Quarantine, Institute of Preventive Medicine, School of Public Health, Dali University, Dali, China
Deltacoronavirus (DCoV) is a genus of coronavirus (CoV) commonly found in avian and swine, but some DCoVs are capable of infecting humans, which causes the concern about interspecies transmission of DCoVs. Thus, monitoring the existence of DCoVs in animals near communities is of great importance for epidemic prevention. Black-headed gulls (Chroicocephalus ridibundus) are common migratory birds inhabiting in most urban and rural wetlands of Yunnan Province, China, which is a typical habitat for black-headed gulls to overwinter. Whether Yunnan black-headed gulls carry CoV has never been determined. In this study, we identified three strains of DCoVs in fecal samples of Yunnan black-headed gulls by reverse-transcriptional PCR and sequenced their whole genomes. Genomic analysis revealed that these three strains shared genomic identity of more than 99%, thus named DCoV HNU4-1, HNU4-2, and HNU4-3; their NSP12 showed high similarity of amino acid sequence to the homologs of falcon coronavirus UAE-HKU27 (HKU27), houbara coronavirus UAE-HKU28 (HKU28), and pigeon coronavirus UAE-HKU29 (HKU29). Since both HKU28 and HKU29 were found in Dubai, there might be cross-border transmission of these avian DCoVs through specific routes. Further coevolutionary analysis supported this speculation that HNU4 (or its ancestors) in black-headed gulls originated from HKU28 (or its homologous strain) in houbara, which was interspecies transmission between two different avian orders. In addition, interspecies transmission of DCoV, from houbara to falcon, pigeon and white-eye, from sparrow to common-magpie, and quail and mammal including porcine and Asian leopard cat, from munia to magpie-robin, was predicted. This is the first report of black-headed gull DCoV in Asia which was highly homolog to other avian DCoVs, and the very “active” host-switching events in DCoV were predicted, which provides important reference for the study of spread and transmission of DCoVs.
Introduction
Coronavirus (CoV) usually refers to the viruses of the sub-family of Orthocoronavirinae in the family of Coronaviridae. In the recent two decades, CoVs have caused three intercontinental epidemics, including the ongoing worldwide pandemic of COVID-19 with more than 500 million infections and almost 6 million deaths. It has been reported that human epidemic CoVs were originated from zoonotic CoVs which were reserved in wild animals and infected humans via occasional interspecies transmission (Ge et al., 2013; Corman et al., 2015; Sabir et al., 2016; Tao et al., 2017; Cui et al., 2019). A variety of animals can serve as the reservoir of CoVs, including bats, birds, mice, and even domestic animals (Chen et al., 2020; Zhou et al., 2021). So far, more than 40 species of CoVs have been reported all over the world by the International Committee on Taxonomy of Viruses (ICTV) (Zhou et al., 2021). The Coronaviridae Study Group (CSG) of ICTV classified coronaviruses into four genera: Alphacoronavirus, Betacoronavirus, Gammacoronavirus, and Deltacoronavirus, by analyzing five essential protein domains (3CL-pro, NiRAN, RdRp, ZBD, and HEL1) combined with phylogeny and genomic structure (Coronaviridae Study Group of the International Committee on Taxonomy of Viruses et al., 2020). Alphacoronavirus and betacoronavirus mainly infect mammals (Hamre and Procknow, 1966; McIntosh et al., 1967; van der Hoek et al., 2004; Vlasova et al., 2011; Lin et al., 2017; Saldanha et al., 2019). Gammacoronavirus and deltacoronavirus (DCoV) mainly infect birds (Mihindukulasuriya et al., 2008; Woo et al., 2009, 2012; Hepojoki et al., 2017).
Although birds are major hosts for DCoV, this virus also infects swine, indicating potential interspecies transmission from birds to mammals which is supported by the evolutionary relationship between sparrow DCoV and porcine DCoV (PDCoV) (Woo et al., 2012; Chen et al., 2018). Notably, PDCoV was reported to infect humans (Lednicky et al., 2021). Since birds and swine are common animals inhabiting in or near human communities, some birds are frequently migrating for a long distance, leading to a concern about potential epidemics caused by DCoV. Therefore, it is important to monitor DCoV in these host animals.
Black-headed gull (Chroicocephalus ridibundus) is a migratory bird widely distributed in Eurasia and on the East Coast of North America (Ushine et al., 2017). Xinjiang, Inner Mongolia, and Heilongjiang in China are their major breeding grounds. In winter, flocks of black-headed gulls migrate from Siberia to Southern China to overwinter, and Yunnan province is a typical habitat for black-headed gulls during winters. Since the 1980s, every year from November to March, tens of thousands of black-headed gulls have been inhabiting in Yunnan where they closely contact with local animals, raising the concern about epidemic viruses such as CoV transmitted from black-headed gulls to others (Liao et al., 2019). According to previous studies, gammacoronavirus has been detected in gulls (Domanska-Blicharz et al., 2014; Wille et al., 2016). As far as we know, only a 461 bp fragment in rdrp gene of black-headed gull DCoV (BHG-DCoV) has been reported in Finland (Hepojoki et al., 2017). However, there is no study on the whole genome of black-headed gull CoV.
In this study, we collected the fecal samples of black-headed gulls in Kunming and Dali, two major cities in Yunnan, for DCoV detection. As a result, three strains of BHG-DCoV strains (HNU4-1, HNU4-2, and HNU4-3) were identified with whole-genome identity of more than 99%. Further genome analysis revealed that the genome of HNU4 showed high identity of more than 96% to those of HKU27, HKU28, and HKU29 found in United Arab Emirates, indicating a potential transboundary transmission. The differentiation time analysis and coevolution analysis support that HNU4 (or its ancestors) in black-headed gull (from Charadriiformes) may spread from HKU28 (or its homologous strain) in houbara (from Gruiformesor). According to the sequence of the five essential domains for CoV taxonomy, HNU4 could be classified into the same species of White-eye CoV-HKU16, and further analysis showed that White-eye CoV-HKU16 like coronavirus had been found in six orders of birds, suggesting the activity of host jumping ability of this coronavirus. Our finding reveals the widespread of DCoV as well as its potential transboundary and interspecies transmission. Therefore, more attention should be paid on DCoV in wide birds to prevent potential epidemics.
Materials and Methods
Sample Collection
From January to March 2021, 460 feces samples of black-headed gulls were collected at seven sampling points near Erhai Lake in Dali, Yunnan Province, and Dianchi Lake in Kunming, Yunnan Province. All samples were placed in virus transport medium (VTM) and kept in dry ice for transportation to the laboratory and stored at −80°C until use.
RNA Extraction and Coronavirus Reverse-Transcriptional PCR Screening
Viral RNA was extracted from the fecal samples using MagaBio plus Virus DNA/RNA Purification Kit II (BioFlux, China) following the manufacturer’s instructions. RNA extracted from fecal was washed off with 70-μl RNase-free water and stored in −80°C as a template for reverse-transcriptional PCR (RT-PCR). Initial CoV screening was performed by amplifying a 440-bp fragment of the rdrp gene of CoVs using primers DCoV-F (5′-GTGGVTGTMTTAATGCACAGTC-3′) and -R (5′-TACTGYCTGTTRGTCATRGTG-3′) (Lau et al., 2018). Primescript™ one-step RT-PCR kit ver. 2 (Takara) was used for PCR amplification. About 20 μl PCR mixture included 0.8 μl Primescript 1 step enzyme, 10 μl 2 × 1 step buffer, 5.2 μl ddH2O, and 2 μl extracted RNA. The mixtures were amplified for 40 cycles as 94°C for 30 s, 48°C for 30s, and 72°C for 40 s and a final extension at 72°C for 10 min in an automated thermal cycler (Applied Biosystems). To confirm the bird species, the mitochondrial cytochrome b (cytb) gene was amplified and sequenced of the samples.
The PCR products were gel-purified and sequenced with an ABI Prism 3,700 DNA analyzer (Applied Biosystems), using the PCR primers. The sequences of the PCR products were compared with known sequences of the rdrp genes of CoVs in the GenBank database.
Complete Genome Sequencing
Three complete genomes of BHG-DCoVs were amplified and sequenced using RNA extracted from black-headed gull feces as templates. RNA was amplified with degenerate primers, which were designed by multiple alignments of other coronavirus genomes with the complete genome, using Primescript one-step RT-PCR kit version 2. Additional primers were designed according to the results of the first and subsequent rounds of sequencing. The 5′ and 3′ genome end sequences were obtained by 5′ and 3′ race (Roche), respectively. The expected size of PCR products was purified by gel and directly sequenced. The sequence was assembled to obtain the full-length genome sequence.
Genomic and Phylogenetic Analysis
Multiple sequence alignment was performed by MAFFT v7.149 in BioAider v1.334 (Katoh and Standley, 2013; Zhou et al., 2020). The maximum likelihood phylogenetic tree of CoVs’ RdRp was constructed by IQ-tree v1.6.10 in PhyloSuite program with 10,000 ultrafast bootstraps, and the most appropriate substitution model of aa was calculated using ModelFinder according to the Bayesian information criterion (BIC) method (Nguyen et al., 2015; Zhang et al., 2019). The phylogenetic tree of ORF1ab, NIRAN, 3CL-pro, HEL1, and S protein was performed using MEGA7 with 1,000 bootstraps in neighbor-joining method (Kumar et al., 2016).
Estimation of Divergence Dates
The rdrp gene was aligned using MAFFT program with codon method in BioAider v1.334. Then, we detected the temporal structure in these rdrp gene sequences by TreeTime program (Sagulenko et al., 2018). The correlation coefficient of R2 was 0.01, and there was very weak signal between sampling time and genetic distance in the data (Supplementary Figure 1). Therefore, we used a uniform distribution priori value (from 8 × 10–5 to 2 × 10–4 subs/site/year) according to the latest report of evolution rate of rdrp gene in DCoV (Wang et al., 2021). Then, we ran a Markov chain of 10 million steps with sampling every 1,000 steps in BEAST v1.10.4 (Drummond et al., 2012). The mean evolution rate and time of the most recent common ancestor (tMRCA) were calculated under uncorrelated lognormal relaxed clock. The most appropriate substitute model of nucleotide was GTR + F + G4 calculated by ModelFinder according to BIC method. We checked the effective sample size (ESS) of parameters in Tracer v1.7 program and ensured that they all reached convergence (ESS > 200). Finally, the maximum clade credibility (MCC) tree was obtained by discarding the first 10% of states in Tree Annotator package.
Coevolutionary Analyses of Virus and Host
Paired evolutionary trees of DCoVs and their corresponding hosts were used to examine the evolutionary relationship. Some DCoV strains without host records were excluded in this analysis. In addition to our samples, only one or two strains (from different countries) were reserved as the representative for these highly similar sequences with the same host. The host evolutionary tree and topologies were obtained from the TimeTree website1 by entering the Latin text of all species to be analyzed (Kumar et al., 2017). Then, we constructed the viral phylogenetic tree based on the rdrp gene using Mrbayes v3.2.6 program (Ronquist et al., 2012). We run three hot and one cold Markov Chain Monte Carlo (MCMC) chains in Mrbayes, and the appropriate substitution and site-variation model of nucleotides was set to GTR + F + I + G4 which was tested and estimated by ModelFinder software according to BIC method. We performed two independent runs with total generations of 2,000,000 for each run and sampled every 100 generations. The first 10% burned samples were discarded, and the 50% majority rule tree was generated using the remaining samples. We checked the ESS value of each parameter in Tracer v1.6 program and ensured that they all reach convergence (ESS > 200).
According to previous research on the evolutionary history of RNA virus (Shi et al., 2018), we examined the co-divergence (coevolution) and cross-host transfer events among DCoVs and their hosts using eMPRess program (Santichaivekin et al., 2021). The consistency test of paired host–virus tree in eMPRess was conducted based on the duplication-transfer-loss (DTL) model, using a maximum parsimony approach (MPR) to find a “best” mapping of the virus tree onto the host tree which minimized the total “cost.” The null hypothesis is that the reconciliation score of the original dataset was due to change in eMPRess. The “cost” of every event was set as follows: co-divergence = 0 (fixed), duplication = 1, transfer (aka host switch) = 1.2, and loss = 1. The statistical test of co-divergence was conducted by comparing the estimated cost to null distributions calculated from 100 random permutations of the host tip mappings.
Results
Detection Results of Black-Headed Gull Coronavirus
A total of 460 fecal samples were collected. Reverse-transcriptional PCR detection showed that three samples were positive of DCoV, with a total positive rate of 0.65%. These positive samples were collected in Cuihu Park, Kunming (Table 1). The three PCR products were sequenced, and based on the sequences, we constructed a phylogenetic tree together with other coronaviruses, preliminarily confirming that the sequences we detected could be clustered into the genus of deltacoronavirus (Figure 1). Especially, the RdRp sequences were highly similar to those of DCoVs HKU27, HKU28, and HKU29 detected in Dubai in 2018 (Lau et al., 2018), with identities of 99.290–99.645%.
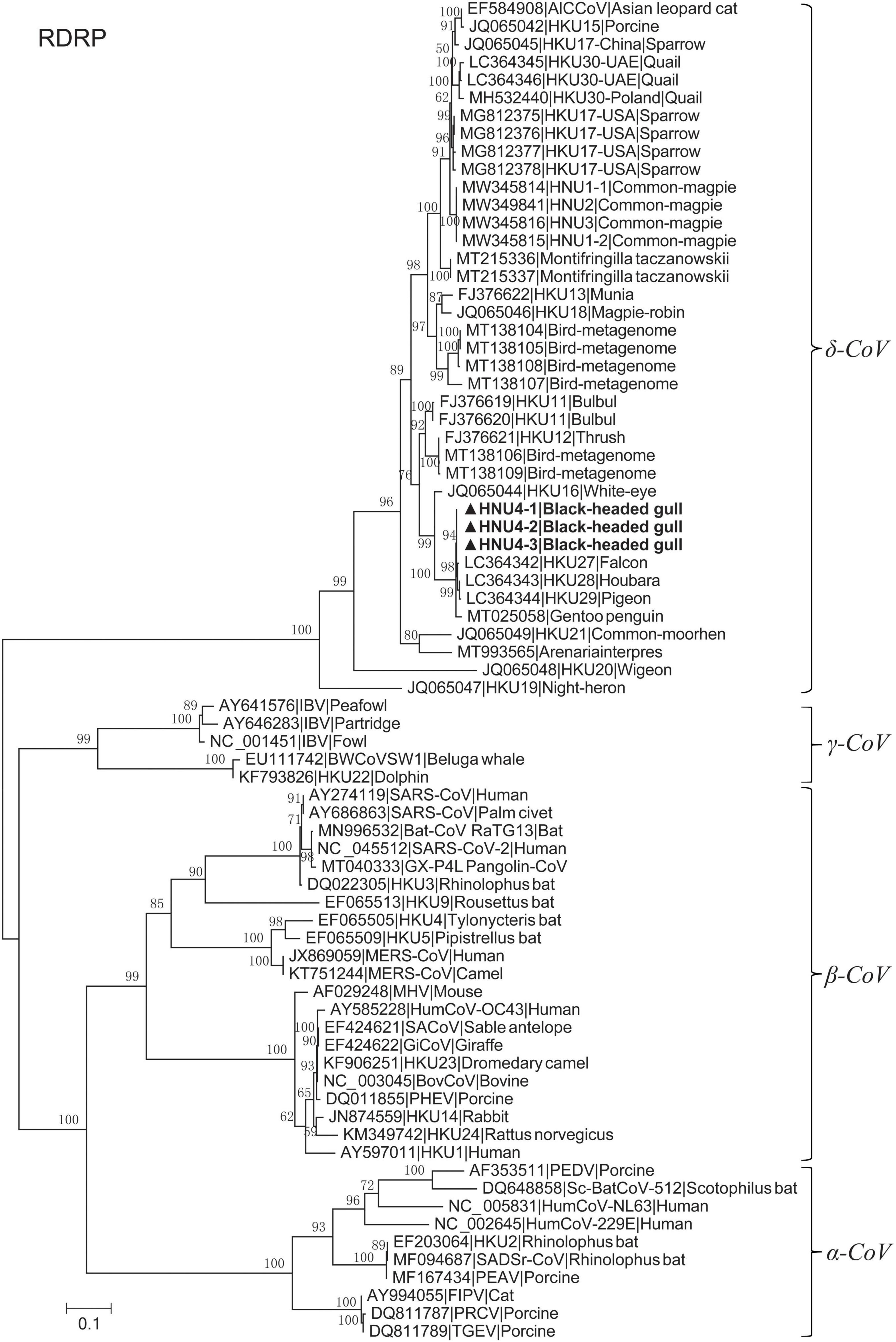
Figure 1. Maximum likelihood tree of RdRp fragment with LG + I + G4 substitution model based on amino acids. The bold ones are BHG-DCoV-HNU4-1, BHG-DCoV-HNU4-2, and BHG-DCoV-HNU4-3 found in this study.
Genome Analysis of the Black-Headed Gull Deltacoronavirus
In order to further reveal the genetic and evolutionary characteristics of the potential DCoVs detected in black-headed gulls, we amplified and sequenced the whole genome of the three DCoVs positives. As a result, three whole-genome sequences of 26,159 nt were obtained. Then, we annotated the genomes by referring to HKU29 genome (LC364344.1) and identified 10 typical open reading frames (ORFs) of coronavirus which were aligned as 5′ untranslated region (5′ UTR)-replicase ORF1ab-spike (S)-envelope (E)-membrane (M)-Nucleocapsid (N)-3′ UTR. Moreover, additional ORFs coding non-structural (NS) proteins (NS6, NS7a, NS7b, NS7c, and NS7d) were identified (Figure 2). The identities among the three genomes ranged from 99.382 to 99.957%, and they showed similarity of 96.606–96.911% to DCoVs HKU27, HKU28, and HKU29 (Table 2). Hence, we named the three DCoV strains in black-headed gulls as BHG-DCoV HNU4-1, HNU4-2, and HNU4-3.
The 3CL-pro, NiRAN, RdRp, ZBD, and HEL1 are the hallmark domains for classification of coronaviruses (International Committee on Taxonomy of Viruses Executive Committee et al., 2020). In order to confirm the taxonomic status of HNU4-1, HNU4-2, and HNU4-3, we compared the aa sequences of these domains with the known DCoVs White-eye coronavirus HKU16 (WECoV-HKU16), HKU27, HKU28, and HKU29. The result showed that all the eight viruses could be classified into the same species under the genus of deltacoronavirus (Table 2). Although the eight DCoVs were classified into the same species, the structural proteins of the BHG-DCoV, HKU27, HKU28, and HKU29 shared high similarity while WECoV-HKU16’s were quite distinct from the others (Table 2).
Amino Acid Variants in ORF1ab and Other Main Proteins
In order to further compare the differences among BHG-DCoV and HKU27, HKU28, and HKU29, we compared the amino acids encoded in their coding regions. The results showed that there were amino acid changes in ORF1ab. We used the cleavage sites of 16 NSPs of ORF1ab analyzed previously to predict the cleavage sites of ORF1ab of BHG-DCoV (Lau et al., 2018). The results showed that the mutations of ORF1ab mainly occurred in NSP3 and NSP6 (Figure 3). BHG-DCoV and HKU27, HKU28, and HKU29 also had different degrees of amino acid mutations in M, N, NS7a, NS7b, and NS7c. Among them, the most amino acid mutation was N protein, with a total of five amino acids mutated, and the least was NS7c, with only one amino acid mutation (Figure 3).

Figure 3. Amino acid variations on NSP3, NSP6, M, N, NS7a, NS7b, and NS7c proteins of six DCoV strains.
Amino Acid Variants in Spike Protein
The difference of S protein among BHG-DCoV, HKU27, HKU28, HKU29, mammalian DCoV, and sparrow coronavirus HKU17-USA (close to PDCoV) was compared (Figure 4 and Supplementary Figure 2). The S protein was quite different between avian DCoV (except HKU17-USA) and mammalian DCoV, and the BHG-DCoV only shared about 45% aa identity with mammalian DCoV (Supplementary Table 1). Besides, there were multiple deletions/insertions and mutations in the NTD domain of S1 region, which may be a possible region for host receptor utilization restriction (Figure 4). However, the difference in the S2 region was relatively small (Supplementary Figure 2). We further analyzed the variation in BHG-DCoV compared with HKU27, HKU28, and HKU29 in S1 region. The result showed that there were nine sites with completely different amino acids between BHG-DCoV, HKU27, HKU28, and HKU29 in S1-NTD (D59A, N/S65Q, Q162L, G/S163I, S179E, T180K, L190F, Q207K, and A253R), and two sites in S1-CTD (Y355H and N419T). Besides, HKU28 was the closest BHG-DCoV on S protein and showed about 95.7% aa identity.
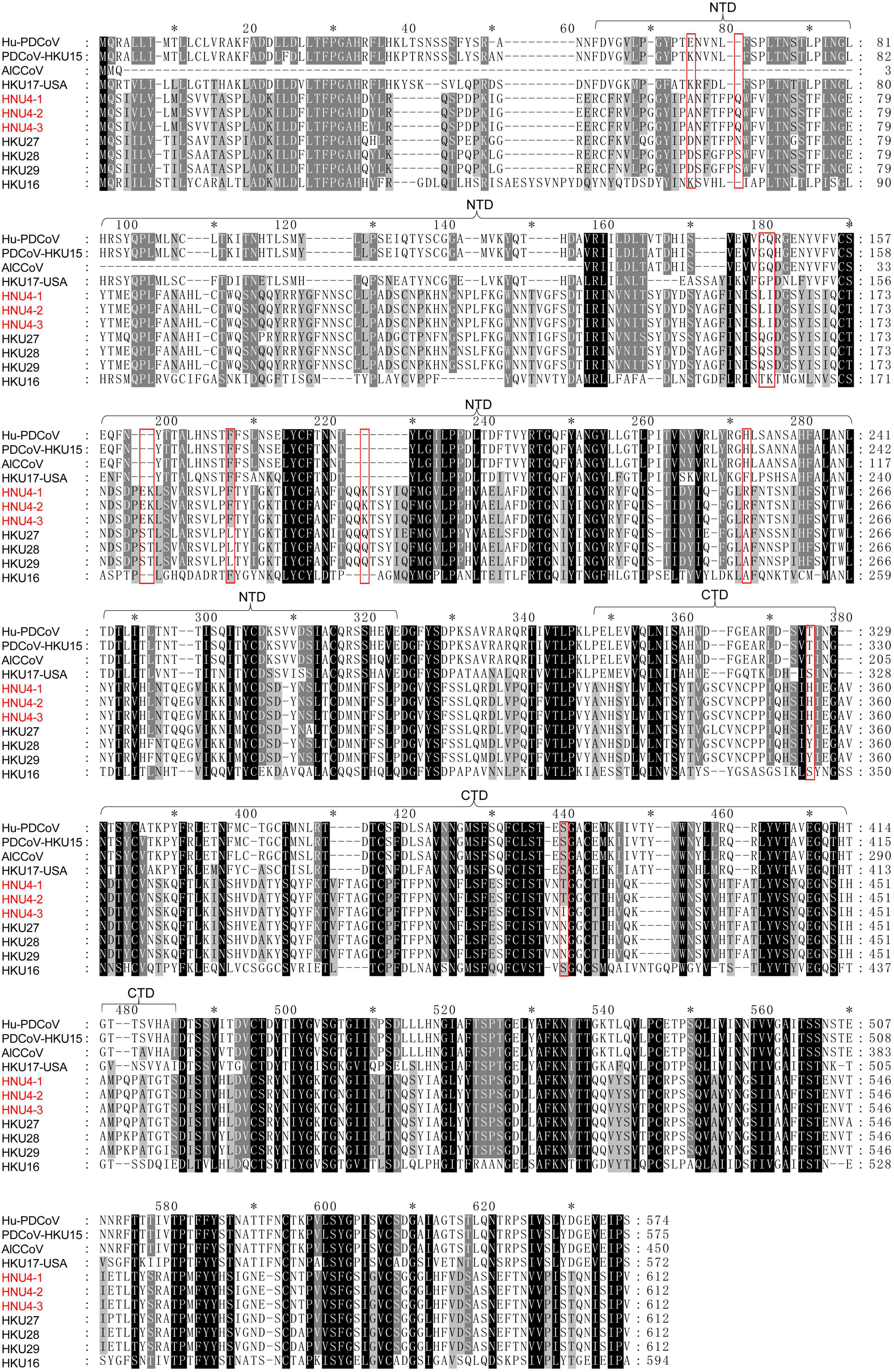
Figure 4. Multiple comparison of S1 fragment among BHG-DCoVs, HKU27, HKU28, HKU29, HKU16, Hu-PDCoV (MW685622.1), PDCoV-HKU15 (JQ065042.2), HKU17-USA (MG812377.1), and AlCCoV (EF584908.1). The annotation of NTD and CTD domains in S1 was with reference to PDCoV (Shang et al., 2018). The red boxes indicate unique sites of BHG-DCoVs compared to HKU27, HKU28, and HKU29.
To assess the impact of mutations on S protein structure and function, we compared the differences in primary and tertiary structures of HNU4-1 and HKU28. In primary sequence, there were a total of 50 aa mutations and one aa insertion in S protein of HNU4-1 compared to HKU28 (Supplementary Table 2). Of these, 16 mutations were in S1-NTD and five mutations were in S1-CTD. We subsequently predicted the tertiary structures of S protein of HNU4-1 and HKU28 by homology modeling method, respectively (Figure 5). The tertiary structure of HKU28 covers the region from aa 51 to 1061, and the tertiary structure of HNU4-1 covers the region from aa 46 to 1062, the chain A as shown in Figure 5A. In general, the tertiary structure of the S protein of HNU4-1 and HKU28 was very similar. However, some mutation sites caused significant changes in residues conception, such as D59A, Q162L, S163I, S179E, T180K, Q207K, N256T, and P258N in S1-NTD, and Y355H, N419T, and K455Q in S1-CTD (Figure 5B). Notably, Q162L, S163I, S179E, T180K, and Q207K are at the top of the NTD, and Y355H, N419T, and K455Q are also at the top of the CTD; we speculate that it may be related to host receptor utilization. However, avian receptor utilization for DCoV is currently unclear, which greatly limits the in-depth exploration of the impact of mutations on function.
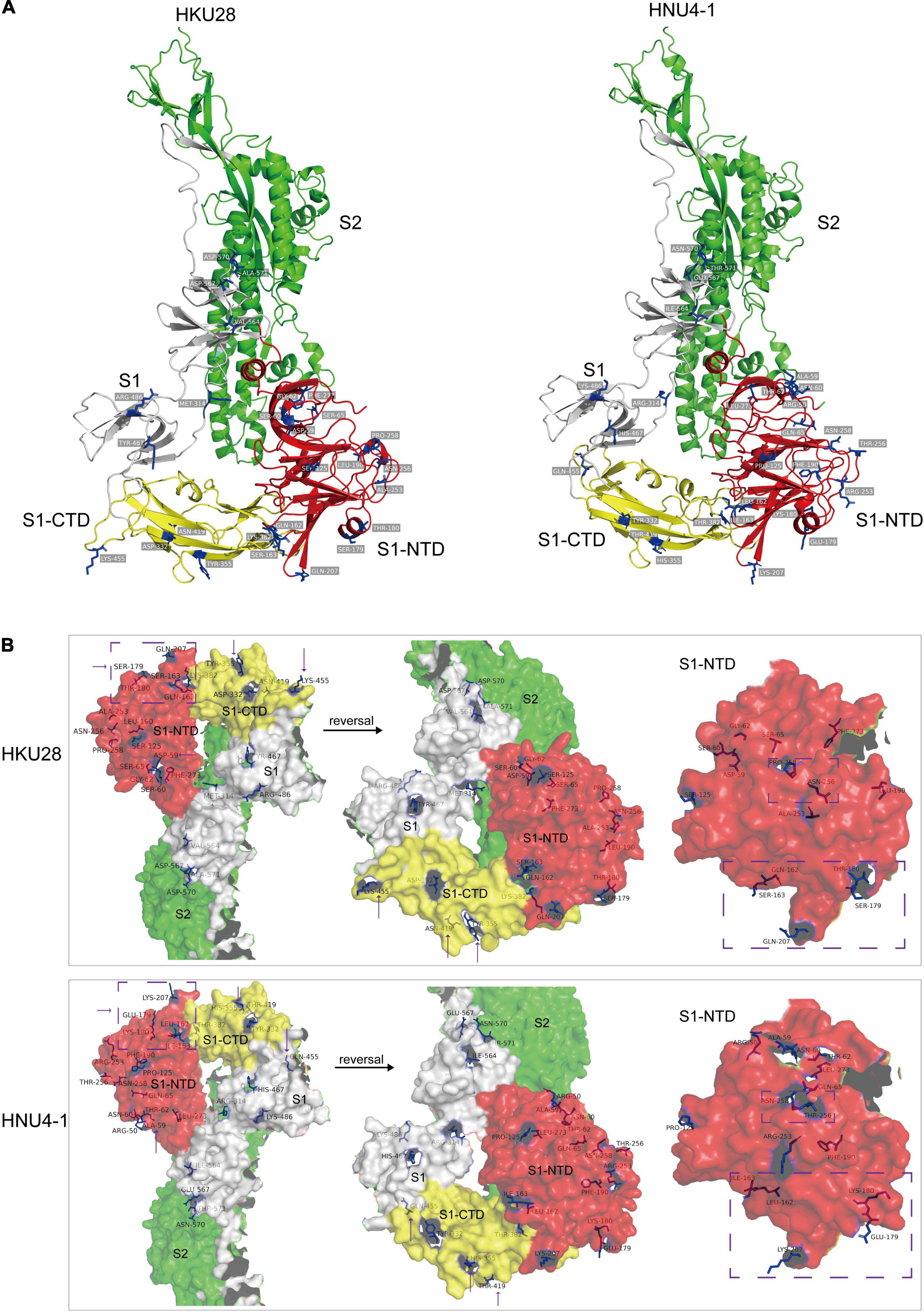
Figure 5. Tertiary structure (chain A) of S protein of HKU28 and HNU4-1. These structures were predicted by SWISS-MODEL server (https://swissmodel.expasy.org/interactive) using S protein of PDCoV as template (PDB ID: 6bfu.1). (A) Different colors indicate different regions of the S protein, red (S1-NTD), yellow (S1-CTD), gray (fragment in S1), and green (S2). (B) Surface of the S protein, differential residue sites between HKU28 and HNU4-1 were marked in blue.
Phylogenetic Analyses of Black-Headed Gull Deltacoronavirus
Based on the amino acid sequences of ORF1ab, S, HEL1, 3CL-pro, and NIRAN, we constructed the phylogenetic trees of BHG-DCoVs and the other 30 DCoVs. The results showed that HNU4-1, HNU4-2, HNU4-3, HKU27, HKU28, and HKU29 were clustered as a sub-branch within a branch including WECoV-HKU16 (Figure 6). Compared with HKU27, HKU28, and HKU29, the three BHG-DCoV are more closely related. It is worth noting that in the phylogenetic tree of ORF1ab, HEL1, 3CL-pro, and NIRAN, they were also clustered with GPCoV found in Antarctica. In the phylogenetic tree constructed by S fragment, except HKU27, HKU28, and HKU29, they also clustered with a Arenaria interpres DCoV (ARDCoV) found in Canada (Figure 6; Wille et al., 2021).
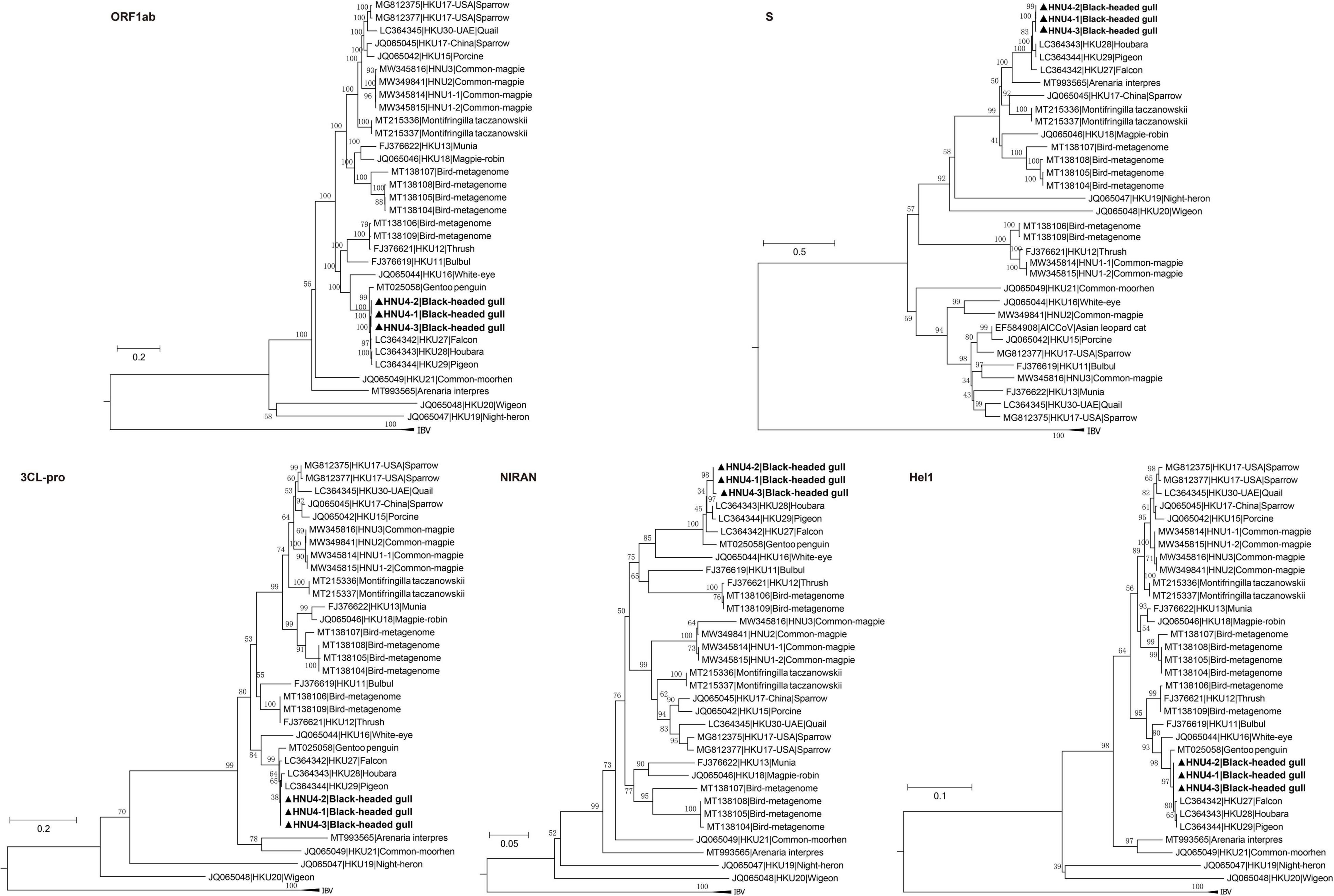
Figure 6. Phylogenetic trees of amino acid sequences of polyprotein 1ab (ORF1ab), spike (S) protein, chymotrypsin-like protease (3CL-pro), superfamily 1 helicase (HEL1), and nidovirus RdRp-associated nucleotidyltransferase (NIRAN). The bold ones are BHG-DCoV-HNU4-1, BHG-DCoV-HNU4-2, and BHG-DCoV-HNU4-3 found in this study.
Evolutionary Rate and tMRCA
The estimated mean evolutionary rate of DCoV rdrp gene was about 1.49 × 10–4 subs/site/year (9.10 × 10–5–2.0 × 10–4, 95% HPD). The time of the most recent common ancestor (tMRCA) differentiation time of DCoV was about 2977 B.C (6617 B.C–1035 B.C, 95% HPD). The tMRCA of White-eye coronavirus HKU16 species was about 1200 A.D (594 A.D–1508 A.D, 95% HPD). The tMRCA of HNU4-1, HNU4-2, and HNU4-3 was about 1964 A.D (1914 A.D–1993 A.D, 95% HPD). However, the tMRCA of HKU27, HKU28, and HKU29 was about 1952 A.D (1896 A.D–1983 A.D), and it suggests that they may differentiate earlier than BHG-DCoV (Figure 7).
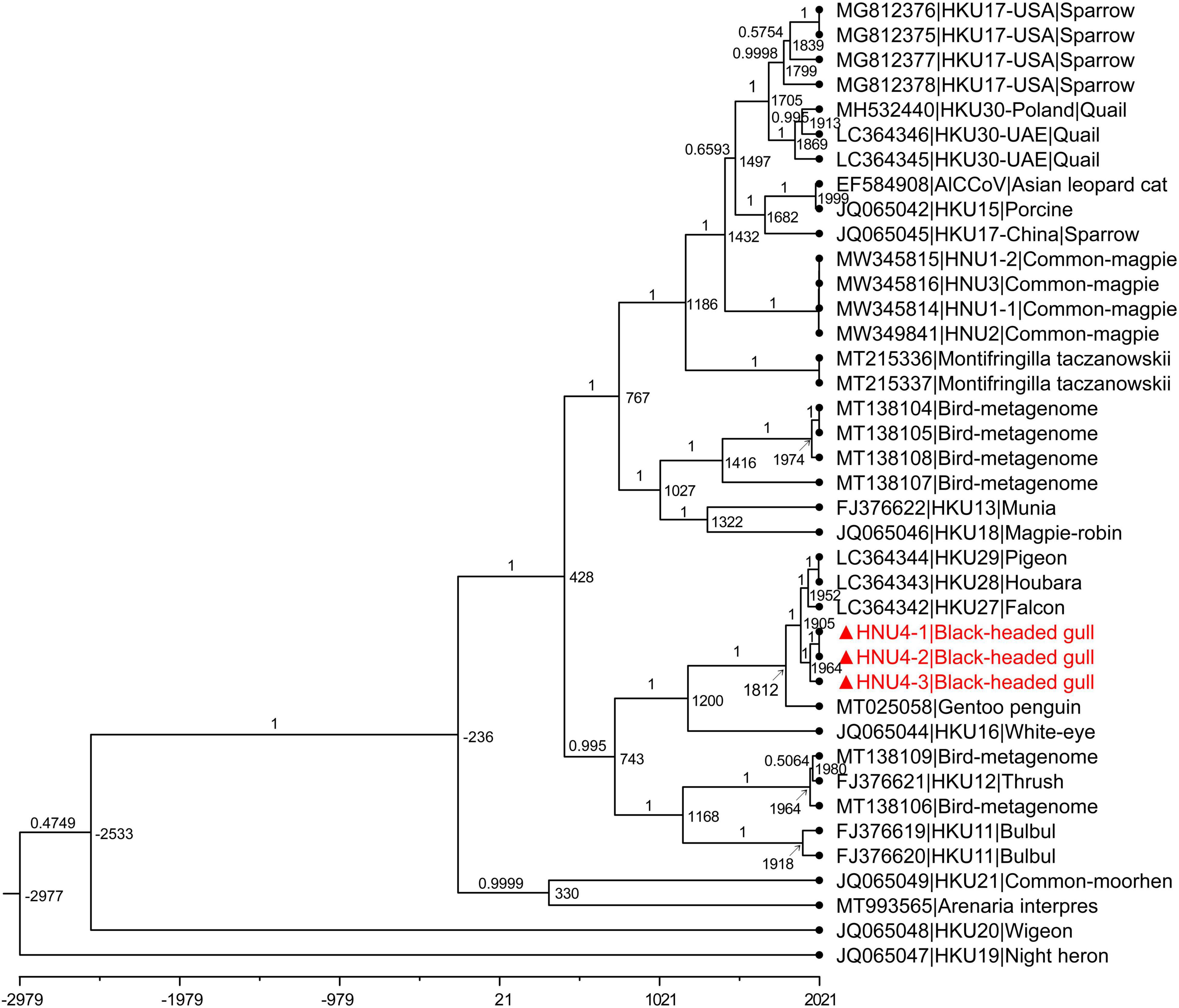
Figure 7. MCC tree with divergence time based on rdrp gene. The value near the node indicates the age of the node, and the label on the branch represents the Bayesian posterior probability. The red bold ones are BHG-DCoV-HNU4-1, BHG-DCoV-HNU4-2, and BHG-DCoV-HNU4-3 found in this study.
Frequent Host-Switching Events of Deltacoronavirus in Birds
The distribution of virus taxa on the host tree showed the host range of DCoV, including 18 species of the Aves class and two species of the Mammalia class (Figure 8 and Supplementary Table 3). The analysis using eMPRess of virus–host mapping detected a total of 23 events, including five co-divergence events, four duplication events, and 14 host-switching events (Figure 8). The global co-divergence test indicated that the co-divergence signal was weak in the host–virus tree, because the “cost” of the original host–virus tree was not significantly less than the randomness (p-value > 0.01) (Supplementary Figure 3). Frequent host-switching events indicate that DCoV was actively jumping among different hosts. Especially, during the history of evolution, HKU28 underwent several reliable host-switching events (with supporting values of 100) among which one led to the generation of HNU4 (Figure 8). This indicated that HNU4 might originate from HKU28 via cross-species transmission among different avian orders.
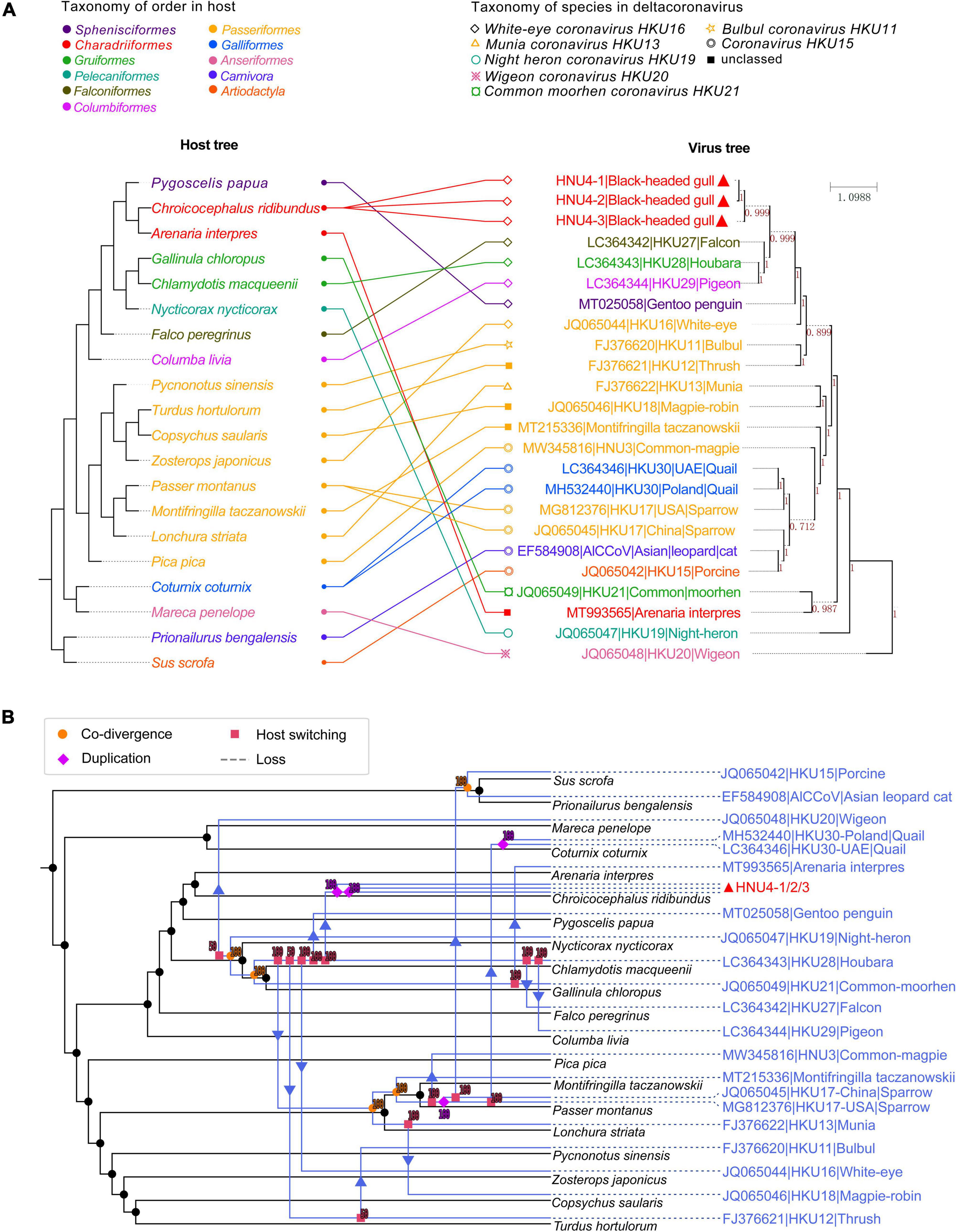
Figure 8. Coevolutionary analysis of virus and host. (A) The tanglegram of host and virus tree. The left is the host evolutionary tree, and the same color represents the same order. The right is phylogenetic tree of viral rdrp gene, and the same shape represents the same virus species. The value on branch in viral tree represents Bayesian posterior probability. The tanglegram of host–virus was drawn by PhyloSuite software. (B) Co-divergence and host switch of DCoV and their hosts. The host tree was marked in black with DCoV tree in blue, and different events were marked with different symbols. Co-divergence, duplication, host switching, and loss events were marked with filled circles, diamond, squares and arrow, and dotted lines, respectively.
Besides, the DCoV in houbara (Gruiformesor) looks “restless” and spread to other hosts, such as falcon (Falco peregrinus), pigeon (Columba livia), and white-eye (Zosterops japonicus). Consistent with the previous speculation, the host transfer between houbara and falcons may be caused by the food chain (Lau et al., 2018). It is worth noting that the DCoV in white-rumped snowfinch (Montifringilla taczanowskii), sparrow, and munia shared co-divergence with three hosts. Then, the sparrow DCoV spread to common-magpie (Pica pica), quail (Coturnix coturnix), and mammal (porcine and Asian leopard cat). The munia DCoV may spread to magpie-robin (Copsychus saularis). In general, many host-switching events were predicted in DCoV, which imply that DCoV was very “active” in their hosts. However, the current sample of bird DCoV is still limited, and only a single host species has been detected in some orders. Therefore, more convincing evolutionary relationships require more samples to support in future. The large number of potential host-switching events indicates that the prevalence of DCoV in birds is wide and complex, and it is necessary to strengthen monitoring.
Discussion
Increasing studies have shown that the outbreak of human epidemic is closely related to pathogens reserved in wildlife (Daszak et al., 2000). Especially, the three pathogenic CoVs (SARS-CoV, MERS-CoV, and SARS-CoV-2) causing worldwide epidemics in the recent two decades were all believed to originate from wild animals, revealing a great importance of monitoring CoVs in their natural reservoirs. In this study, we detected three DCoV strains (HNU4-1, HNU4-2, and HNU4-3) in black-headed gulls in Yunnan Province and sequenced their whole genomes. These were the first BHG-DCoVs detected in China and the first whole-genome sequences of BHG-DCoV ever reported. The rdrp gene (461bp) identity between BHG-DCoV in Yunnan and BHG-CoV found in Finland is 94.794–95.662%. In view of the high conservation of rdrp gene, BHG-DCoV in black-headed gulls may also be diverse. According to the hallmark domain sequences for CoV classification proposed by ICTV, these newly found BHG-DCoV strains could be classified into the species White-eye coronavirus HKU16, but the overall genome sequences of the BHG-DCoV showed low identity with White-eye coronavirus HKU16, the prototypical virus of the species. In comparison, these BHG-DCoVs genomes were highly similar (>96%) to the genomes of HKU27, HKU28, and HKU29 found in Dubai, indicating the close evolutionary relationship of these avian DCoVs and potential interspecies and transboundary transmission of them.
Despite the high similarity in the whole-genome level, HNU4, HKU27, HKU28, and HKU29 showed a few amino acid variations in several structural and non-structural proteins, including M, N, NSP3, NSP6, NS7a, NS7b, and NS7c. These proteins are critical for CoV life cycle. For instance, NSP3 is a scaffold protein with activity of deubiquitination (DUB) and deISGylation which is necessary for immune evasion (Neuman, 2016; Alfuwaires et al., 2017); NSP6 is capable of activating autophagy which is required for CoV replication (Cottam et al., 2011, 2014; Angelini et al., 2013; Benvenuto et al., 2020); M and N are structural proteins essential for viral assembly (Neuman et al., 2011; McBride et al., 2014; Lee and Lee, 2015). The variance of functional proteins in these closely related DCoVs potentially reveals the adaption of the virus to different hosts, whereas the detailed mechanisms need to be further studied.
Spike protein is the key factor determining the receptor recognition of coronavirus (Gallagher and Buchmeier, 2001; Holmes, 2003). Coronavirus spike has two subunits, S1 and S2. S1 binds to cellular receptor cells for virus attachment, and S2 mediates membrane fusion and virus internalization (Li, 2016). S1 contains two domains related to receptor recognition, S1-NTD binding to polysaccharide and S1-CTD binding to protein (Krempl et al., 1997; Mou et al., 2013; Li, 2016; Shang et al., 2018). In this study, we found that the S protein of BHG-DCoVs, HNU4-1, HNU4-2, and HNU4-3 is highly similar to that of HKU27, HKU28, and HKU29; more detailed studies show that the S1-NTD and S1-CTD domains of BHG-CoV and HKU27, HKU28, and HKU29 are highly similar, but still a few amino acids have changed, and whether this change will affect their selection and utilization of receptors needs further research.
The estimation of differentiation date showed that tMRCA of HKU27, HKU28, and HKU29 was earlier than BHG-DCoV, which supports the possibility of cross-species transmission of them together with the host-switching event. In addition, the host–virus mapping model constructed in this study also supported that the origin of mammalian DCoVs was bird DCoVs (Figure 8). However, when the host transfer event appears at the node of the virus tree, its descendant node is transferred to a branch of the host tree which is not ancestrally related (neither an ancestor nor a descendant of the host) to the host (Santichaivekin et al., 2021). For the host transfer event, we could only explain the possible source through the tip (or the node near tip) of the virus tree. Therefore, whether the DCoV in houbara directly spreading to white-rumped snowfinch (Montifringilla taczanowskii), sparrow (Passer montanus), and munia (Lonchura striata), or their common ancestor, was not clear. Of note, the evolutionary relationship between the ancestors of Night heron coronavirus HKU19, Wigeon coronavirus HKU20, and their hosts was not clear due to the lower support value, and the host transfer from houbara to thrush (Turdus hortulorum) also lacked sufficient support.
It was believed previously that the host of DCoVs was limited to avian and swine only, but three child cases of acute undifferentiated febrile illness positive for porcine DCoV were reported in Haiti recently (Lednicky et al., 2021), raising the concern about transmission and pathogenicity of DCoV to humans, especially during long-term and close contact. Since White-eye coronavirus HKU16 like coronavirus has been found in six orders of birds, suggesting the active interspecies transmission ability of White-eye coronavirus HKU16 like coronavirus, ornithological studies have shown that the black-headed gulls in Yunan usually migrate for long distance from Russia, Mongolia, and Xinjiang Uygur Autonomous Region (Gan et al., 2019). In the large areas for migration, black-headed gulls contact a lot of people and animals, and the BHG-DCoVs carried by them may transmit to other birds including poultry fed in human communities, causing poultry epidemics and even human infections. Therefore, it is necessary to continue the monitoring of this DCoV.
Alphacoronavirus and betacoronavirus mainly infect mammals and have been reported to cause human diseases and even worldwide pandemics. Thus, the existence of alphacoronavirus and betacoronavirus in wild and domestic animals has been widely studied. In comparison, less attention has been paid to DCoV which mainly infects avian. However, increasing evidence indicates that DCoV is capable of causing diseases in poultry, swine, and even humans. Considering the widespread birds and rapid mutation of DCoV, interspecies transmission of DCoV may probably cause epidemics of humans and domestic animals. Thus, people should not omit the potential threat of DCoV to public health and animal husbandry industry. Our identification of DCoVs in black-headed gulls, the common birds with frequently long-distance and transboundary migration, further substantiates the importance of monitoring DCoVs in the nature.
Data Availability Statement
The datasets presented in this study can be found in online repositories. The names of the repository/repositories and accession number(s) can be found below: https://www.ncbi.nlm.nih.gov/genbank/, OL311150, OL311151, and OL311152.
Author Contributions
K-KC, Z-JZ, Y-ZZ, and X-YG contributed to the conception and design of the study. K-KC and LG collected the samples. Z-JZ organized the database. K-KC and Z-JZ performed the data analysis. K-KC and YQ wrote the first draft of the manuscript. All authors contributed to manuscript revision, read, and approved the submitted version.
Funding
This study was funded by the National Natural Science Foundation of China (U2002218, 32041001, 81874274, and 81902070); Yunnan high-level health and family planning technical personnel training project (l-2017027); Doctoral research start-up fee project of Dali University (KYBS2018004); and Cross-border prevention, control, and quarantine innovation group of zoonosis in Dali University (ZKPY2019302).
Conflict of Interest
The authors declare that the research was conducted in the absence of any commercial or financial relationships that could be construed as a potential conflict of interest.
Publisher’s Note
All claims expressed in this article are solely those of the authors and do not necessarily represent those of their affiliated organizations, or those of the publisher, the editors and the reviewers. Any product that may be evaluated in this article, or claim that may be made by its manufacturer, is not guaranteed or endorsed by the publisher.
Supplementary Material
The Supplementary Material for this article can be found online at: https://www.frontiersin.org/articles/10.3389/fmicb.2022.895741/full#supplementary-material
Footnotes
References
Alfuwaires, M., Altaher, A., and Kandeel, M. (2017). Molecular dynamic studies of interferon and innate immunity resistance in MERS CoV Non-Structural Protein 3. Biol. Pharm. Bull. 40, 345–351. doi: 10.1248/bpb.b16-00870
Angelini, M. M., Akhlaghpour, M., Neuman, B. W., and Buchmeier, M. J. (2013). Severe acute respiratory syndrome coronavirus nonstructural proteins 3, 4, and 6 induce double-membrane vesicles. mBio 4:e00524-13. doi: 10.1128/mBio.00524-13
Benvenuto, D., Angeletti, S., Giovanetti, M., Bianchi, M., Pascarella, S., Cauda, R., et al. (2020). Evolutionary analysis of SARS-CoV-2: How mutation of Non-Structural Protein 6 (NSP6) could affect viral autophagy. J. Infect. 81, e24–e27. doi: 10.1016/j.jinf.2020.03.058
Chen, Q., Wang, L., Yang, C., Zheng, Y., Gauger, P. C., Anderson, T., et al. (2018). The emergence of novel sparrow deltacoronaviruses in the United States more closely related to porcine deltacoronaviruses than sparrow deltacoronavirus HKU17. Emerg. Microbes Infect. 7:105. doi: 10.1038/s41426-018-0108-z
Chen, Y., Liu, Q., and Guo, D. (2020). Emerging coronaviruses: genome structure, replication, and pathogenesis. J. Med. Virol. 92, 418–423.
Corman, V. M., Baldwin, H. J., Tateno, A. F., Zerbinati, R. M., Annan, A., Owusu, M., et al. (2015). Evidence for an ancestral association of human coronavirus 229E with Bats. J. Virol. 89, 11858–11870. doi: 10.1128/JVI.01755-15
Coronaviridae Study Group of the International Committee on Taxonomy of Viruses, Gorbalenya, AE., Baker, SC., Baric, RS., de Groot, RJ., Drosten, C., et al. (2020). The species Severe acute respiratory syndrome-related coronavirus: classifying 2019-nCoV and naming it SARS-CoV-2. Nat Microbiol 5, 536–544. doi: 10.1038/s41564-020-0695-z
Cottam, E. M., Maier, H. J., Manifava, M., Vaux, L. C., Chandra-Schoenfelder, P., Gerner, W., et al. (2011). Coronavirus nsp6 proteins generate autophagosomes from the endoplasmic reticulum via an omegasome intermediate. Autophagy 7, 1335–1347. doi: 10.4161/auto.7.11.16642
Cottam, E. M., Whelband, M. C., and Wileman, T. (2014). Coronavirus NSP6 restricts autophagosome expansion. Autophagy 10, 1426–1441. doi: 10.4161/auto.29309
Cui, J., Li, F., and Shi, Z. L. (2019). Origin and evolution of pathogenic coronaviruses. Nat. Rev. Microbiol. 17, 181–192. doi: 10.1038/s41579-018-0118-9
Daszak, P., Cunningham, A. A., and Hyatt, A. D. (2000). Emerging infectious diseases of wildlife–threats to biodiversity and human health. Science 287, 443–449. doi: 10.1126/science.287.5452.443
Domanska-Blicharz, K., Jacukowicz, A., Lisowska, A., Wyrostek, K., and Minta, Z. (2014). Detection and molecular characterization of infectious bronchitis-like viruses in wild bird populations. Avian Pathol. 43, 406–413. doi: 10.1080/03079457.2014.949619
Drummond, A. J., Suchard, M. A., Xie, D., and Rambaut, A. (2012). Bayesian phylogenetics with BEAUti and the BEAST 1.7. Mol. Biol. Evol. 29, 1969–1973. doi: 10.1093/molbev/mss075
Gallagher, T. M., and Buchmeier, M. J. (2001). Coronavirus spike proteins in viral entry and pathogenesis. Virology 279, 371–374. doi: 10.1006/viro.2000.0757
Gan, L., Cao, X., Wang, Y., Wang, Y., Jiang, H., Lan, R., et al. (2019). Carriage and potential long distance transmission of Listeria monocytogenes by migratory black-headed gulls in Dianchi Lake, Kunming. Emerg. Microbes Infect. 8, 1195–1204. doi: 10.1080/22221751.2019.1647764
Ge, X. Y., Li, J. L., Yang, X. L., Chmura, A. A., Zhu, G., Epstein, J. H., et al. (2013). Isolation and characterization of a bat SARS-like coronavirus that uses the ACE2 receptor. Nature 503, 535–538. doi: 10.1038/nature12711
Hamre, D., and Procknow, J. J. (1966). A new virus isolated from the human respiratory tract. Proc. Soc. Exp. Biol. Med. 121, 190–193. doi: 10.3181/00379727-121-30734
Hepojoki, S., Lindh, E., Vapalahti, O., and Huovilainen, A. (2017). Prevalence and genetic diversity of coronaviruses in wild birds, Finland. Infect. Ecol. Epidemiol. 7:1408360. doi: 10.1080/20008686.2017.1408360
Holmes, K. V. (2003). SARS-associated coronavirus. N. Engl. J. Med. 348, 1948–1951. doi: 10.1056/NEJMp030078
International Committee on Taxonomy of Viruses Executive Committee, Gorbalenya, A. E., Krupovic, M., Mushegian, A., Kropinski, A. M., Siddell, S. G. et al. (2020). The new scope of virus taxonomy: partitioning the virosphere into 15 hierarchical ranks. Nat. Microbiol. 5, 668–674. doi: 10.1038/s41564-020-0709-x
Katoh, K., and Standley, D. M. (2013). MAFFT multiple sequence alignment software version 7: improvements in performance and usability. Mol. Biol. Evol. 30, 772–780. doi: 10.1093/molbev/mst010
Krempl, C., Schultze, B., Laude, H., and Herrler, G. (1997). Point mutations in the S protein connect the sialic acid binding activity with the enteropathogenicity of transmissible gastroenteritis coronavirus. J. Virol. 71, 3285–3287. doi: 10.1128/JVI.71.4.3285-3287.1997
Kumar, S., Stecher, G., Suleski, M., and Hedges, S. B. (2017). Timetree: a resource for timelines, timetrees, and divergence times. Mol. Biol. Evol. 34, 1812–1819. doi: 10.1093/molbev/msx116
Kumar, S., Stecher, G., and Tamura, K. (2016). MEGA7: Molecular Evolutionary Genetics Analysis Version 7.0 for bigger datasets. Mol. Biol. Evol. 33, 1870–1874. doi: 10.1093/molbev/msw054
Lau, S. K. P., Wong, E. Y. M., Tsang, C. C., Ahmed, S. S., Au-Yeung, R. K. H., Yuen, K. Y., et al. (2018). Discovery and sequence analysis of four deltacoronaviruses from birds in the middle east reveal interspecies jumping with recombination as a potential mechanism for avian-to-avian and avian-to-mammalian transmission. J. Virol. 92:e00265-18. doi: 10.1128/JVI.00265-18
Lednicky, J. A., Tagliamonte, M. S., White, S. K., Elbadry, M. A., Alam, M. M., Stephenson, C. J., et al. (2021). Independent infections of porcine deltacoronavirus among Haitian children. Nature 600, 133–137. doi: 10.1038/s41586-021-04111-z
Lee, S., and Lee, C. (2015). Functional characterization and proteomic analysis of the nucleocapsid protein of porcine deltacoronavirus. Virus Res 208, 136–145. doi: 10.1016/j.virusres.2015.06.013
Li, F. (2016). Structure, function, and evolution of coronavirus spike proteins. Annu. Rev. Virol. 3, 237–261. doi: 10.1146/annurev-virology-110615-042301
Liao, F., Gu, W., Li, D., Liang, J., Fu, X., Xu, W., et al. (2019). Characteristics of microbial communities and intestinal pathogenic bacteria for migrated Larus ridibundus in southwest China. Microbiologyopen 8:e00693. doi: 10.1002/mbo3.693
Lin, X. D., Wang, W., Hao, Z. Y., Wang, Z. X., Guo, W. P., Guan, X. Q., et al. (2017). Extensive diversity of coronaviruses in bats from China. Virology 507, 1–10. doi: 10.1016/j.virol.2017.03.019
Liu, C., Tang, J., Ma, Y., Liang, X., Yang, Y., Peng, G., et al. (2015). Receptor usage and cell entry of porcine epidemic diarrhea coronavirus. J. Virol. 89, 6121–6125. doi: 10.1128/JVI.00430-15
McBride, R., van Zyl, M., and Fielding, B. C. (2014). The coronavirus nucleocapsid is a multifunctional protein. Viruses 6, 2991–3018. doi: 10.3390/v6082991
McIntosh, K., Becker, W. B., and Chanock, R. M. (1967). Growth in suckling-mouse brain of “IBV-like” viruses from patients with upper respiratory tract disease. Proc. Natl. Acad. Sci. U.S.A. 58, 2268–2273. doi: 10.1073/pnas.58.6.2268
Mihindukulasuriya, K. A., Wu, G., St Leger, J., Nordhausen, R. W., and Wang, D. (2008). Identification of a novel coronavirus from a beluga whale by using a panviral microarray. J. Virol. 82, 5084–5088. doi: 10.1128/JVI.02722-07
Mou, H., Raj, V. S., van Kuppeveld, F. J., Rottier, P. J., Haagmans, B. L., and Bosch, B. J. (2013). The receptor binding domain of the new Middle East respiratory syndrome coronavirus maps to a 231-residue region in the spike protein that efficiently elicits neutralizing antibodies. J. Virol. 87, 9379–9383. doi: 10.1128/JVI.01277-13
Neuman, B. W. (2016). Bioinformatics and functional analyses of coronavirus nonstructural proteins involved in the formation of replicative organelles. Antiviral Res. 135, 97–107. doi: 10.1016/j.antiviral.2016.10.005
Neuman, B. W., Kiss, G., Kunding, A. H., Bhella, D., Baksh, M. F., Connelly, S., et al. (2011). A structural analysis of M protein in coronavirus assembly and morphology. J. Struct. Biol. 174, 11–22. doi: 10.1016/j.jsb.2010.11.021
Nguyen, L. T., Schmidt, H. A., von Haeseler, A., and Minh, B. Q. (2015). IQ-TREE: a fast and effective stochastic algorithm for estimating maximum-likelihood phylogenies. Mol. Biol. Evol. 32, 268–274. doi: 10.1093/molbev/msu300
Ronquist, F., Teslenko, M., van der Mark, P., Ayres, D. L., Darling, A., Höhna, S., et al. (2012). MrBayes 3.2: efficient Bayesian phylogenetic inference and model choice across a large model space. Syst. Biol. 61, 539–542. doi: 10.1093/sysbio/sys029
Sabir, J. S., Lam, T. T., Ahmed, M. M., Li, L., Shen, Y., Abo-Aba, S. E., et al. (2016). Co-circulation of three camel coronavirus species and recombination of MERS-CoVs in Saudi Arabia. Science 351, 81–84. doi: 10.1126/science.aac8608
Sagulenko, P., Puller, V., and Neher, R. A. (2018). TreeTime: maximum-likelihood phylodynamic analysis. Virus Evol. 4:vex042. doi: 10.1093/ve/vex042
Saldanha, I. F., Lawson, B., Goharriz, H., Rodriguez-Ramos Fernandez, J., John, S. K., Fooks, A. R., et al. (2019). Extension of the known distribution of a novel clade C betacoronavirus in a wildlife host. Epidemiol. Infect. 147:e169. doi: 10.1017/S0950268819000207
Santichaivekin, S., Yang, Q., Liu, J., Mawhorter, R., Jiang, J., Wesley, T., et al. (2021). eMPRess: a systematic cophylogeny reconciliation tool. Bioinformatics 37, 2481–2482. doi: 10.1093/bioinformatics/btaa978
Shang, J., Zheng, Y., Yang, Y., Liu, C., Geng, Q., Tai, W., et al. (2018). Cryo-electron microscopy structure of porcine deltacoronavirus spike protein in the prefusion state. J. Virol. 92:e01556-17. doi: 10.1128/JVI.01556-17
Shi, M., Lin, X. D., Chen, X., Tian, J. H., Chen, L. J., Li, K., et al. (2018). The evolutionary history of vertebrate RNA viruses. Nature 556, 197–202. doi: 10.1038/s41586-018-0012-7
Tao, Y., Shi, M., Chommanard, C., Queen, K., Zhang, J., Markotter, W., et al. (2017). Surveillance of bat coronaviruses in kenya identifies relatives of human coronaviruses NL63 and 229E and Their Recombination History. J. Virol. 91:e01953-16. doi: 10.1128/jvi.01953-16
Ushine, N., Sato, T., Kato, T., and Hayama, S. I. (2017). Analysis of body mass changes in the Black-Headed Gull (Larus ridibundus) during the winter. J. Vet. Med. Sci. 79, 1627–1632. doi: 10.1292/jvms.17-0099
van der Hoek, L., Pyrc, K., Jebbink, M. F., Vermeulen-Oost, W., Berkhout, R. J., Wolthers, K. C., et al. (2004). Identification of a new human coronavirus. Nat. Med. 10, 368–373. doi: 10.1038/nm1024
Vlasova, A. N., Halpin, R., Wang, S., Ghedin, E., Spiro, D. J., and Saif, L. J. (2011). Molecular characterization of a new species in the genus Alphacoronavirus associated with mink epizootic catarrhal gastroenteritis. J. Gen. Virol. 92(Pt 6), 1369–1379. doi: 10.1099/vir.0.025353-0
Wang, Q., Zhou, Z. J., You, Z., Wu, D. Y., Liu, S. J., Zhang, W. L., et al. (2021). Epidemiology and evolution of novel deltacoronaviruses in birds in central China. Transbound. Emerg. Dis. 69, 632–644. doi: 10.1111/tbed.14029
Wille, M., Muradrasoli, S., Nilsson, A., and Järhult, J. D. (2016). High prevalence and putative lineage maintenance of avian coronaviruses in Scandinavian Waterfowl. PLoS One 11:e0150198. doi: 10.1371/journal.pone.0150198
Wille, M., Shi, M., Hurt, A. C., Klaassen, M., and Holmes, E. C. (2021). RNA virome abundance and diversity is associated with host age in a bird species. Virology 561, 98–106. doi: 10.1016/j.virol.2021.06.007
Woo, P. C., Lau, S. K., Lam, C. S., Lai, K. K., Huang, Y., Lee, P., et al. (2009). Comparative analysis of complete genome sequences of three avian coronaviruses reveals a novel group 3c coronavirus. J. Virol. 83, 908–917. doi: 10.1128/JVI.01977-08
Woo, P. C., Lau, S. K., Lam, C. S., Lau, C. C., Tsang, A. K., Lau, J. H., et al. (2012). Discovery of seven novel Mammalian and avian coronaviruses in the genus deltacoronavirus supports bat coronaviruses as the gene source of alphacoronavirus and betacoronavirus and avian coronaviruses as the gene source of gammacoronavirus and deltacoronavirus. J. Virol. 86, 3995–4008. doi: 10.1128/JVI.06540-11
Zhang, Z., Chuang, Y. H., Huang, N., and Mitch, W. A. (2019). Predicting the contribution of chloramines to contaminant decay during ultraviolet/hydrogen peroxide advanced oxidation process treatment for potable reuse. Environ. Sci. Technol. 53, 4416–4425. doi: 10.1021/acs.est.8b06894
Zhou, Z., Qiu, Y., and Ge, X. (2021). The taxonomy, host range and pathogenicity of coronaviruses and other viruses in the Nidovirales order. Anim. Dis. 1:5. doi: 10.1186/s44149-021-00005-9
Keywords: deltacoronavirus, black-headed gull, phylogeny, interspecies transmission, codivergence
Citation: Chu K-K, Zhou Z-J, Wang Q, Ye S-B, Guo L, Qiu Y, Zhang Y-Z and Ge X-Y (2022) Characterization of Deltacoronavirus in Black-Headed Gulls (Chroicocephalus ridibundus) in South China Indicating Frequent Interspecies Transmission of the Virus in Birds. Front. Microbiol. 13:895741. doi: 10.3389/fmicb.2022.895741
Received: 14 March 2022; Accepted: 19 April 2022;
Published: 12 May 2022.
Edited by:
Susanna K. P. Lau, The University of Hong Kong, Hong Kong SAR, ChinaReviewed by:
Feifei Yin, Hainan Medical University, ChinaPan Qin, Fujian Agriculture and Forestry University, China
Copyright © 2022 Chu, Zhou, Wang, Ye, Guo, Qiu, Zhang and Ge. This is an open-access article distributed under the terms of the Creative Commons Attribution License (CC BY). The use, distribution or reproduction in other forums is permitted, provided the original author(s) and the copyright owner(s) are credited and that the original publication in this journal is cited, in accordance with accepted academic practice. No use, distribution or reproduction is permitted which does not comply with these terms.
*Correspondence: Ye Qiu, cWl1eWVAaG51LmVkdS5jbg==; Yun-Zhi Zhang, emhhbmd5dW56aGkxODE4QDE2My5jb20=; Xing-Yi Ge, eHlnZUBobnUuZWR1LmNu
†These authors have contributed equally to this work