- 1Guangdong Engineering Laboratory of Biomass High-Value Utilization, Guangdong Plant Fiber Comprehensive Utilization Engineering Technology Research and Development Center, Guangzhou Key Laboratory of Biomass Comprehensive Utilization, Institute of Biological and Medical Engineering, Guangdong Academy of Sciences, Guangzhou, China
- 2Key Laboratory of Quality Evaluation of Chinese Medicine of the Guangdong Provincial Medical Products Administration, the Second Clinical College, Guangzhou University of Chinese Medicine, Guangzhou, China
Ganoderma lucidum has a wide carbon spectrum, while the expression profile of key genes relevant to carbon metabolism on different carbon sources has been seldom studied. Here, the transcriptomes of G. lucidum mycelia cultured on each of 19 carbon sources were conducted. In comparison with glucose, 16 to 1,006 genes were upregulated and 7 to 1,865 genes were downregulated. Significant gene expression dynamics and induced activity were observed in laccase genes when using agricultural and forestry residues (AFRs) as solo carbon sources. Furthermore, study of laccase gene family in two haploids of G. lucidum GL0102 was conducted. Totally, 15 and 16 laccase genes were identified in GL0102_53 and GL0102_8, respectively, among which 15 pairs were allelic genes. Gene structures were conserved between allelic laccase genes, while sequence variations (most were SNPs) existed. Nine laccase genes rarely expressed on all the tested carbon sources, while the other seven genes showed high expression level on AFRs, especially Gllac2 and Gllac7, which showed 5- to 1,149-fold and 4- to 94-fold upregulation in mycelia cultured for 5 days, respectively. The expression of H53lac7 was consistently higher than that of H8lac7_1 on all the carbon sources except XM, exhibiting a case of allelic expression bias. A total of 47 SNPs and 3 insertions/deletions were observed between promoters of H53lac7 and H8lac7_1, which lead to differences in predicted binding sites of zinc fingers. These results provide scientific data for understanding the gene expression profile and regulatory role on different carbon sources and may support further functional research of laccase.
Introduction
Ganoderma lucidum is a typical white-rot fungus and has been suggested as a potential model system for medicinal mushroom study (Chen et al., 2012). G. lucidum has rich pharmacological ingredients, and has been used for 1000 years (Bishop et al., 2015). Traditional cultivation of G. lucidum on basswood, results in a large demand for forestry resources. Thus, new alternatives of biomass resources need to be discovered to improve the current situation and promote the sustainable development of G. lucidum industry. To date, sawdust cultivation and substituted cultivation using AFRs (lignocellulosic compounds, such as cotton seed husk, corn cobs, wheat-straw, poplar wood chip, wheat bran, and date palm leaf; Hapuarachchi et al., 2018; Kurd-Anjaraki et al., 2022) and even food or diaper waste-based cultivations have been achieved (Khoo et al., 2022).
To colony on those substrates, several secreted extracellular enzymes such as laccase, participate in degradation of lignocellulose (especially lignin) to nutrients which suitable for mycelial growth of G. lucidum (Zhou et al., 2018; Yuliana et al., 2020). Besides, laccases are involved in primordium formation and fruiting body development of fungi (Das et al., 1997; Lu et al., 2015; Jiao et al., 2018; Jin et al., 2018). Laccase (EC 1.10.3.2) is a group of phenol oxidases that belongs to the family of multi-copper oxidases. It usually contains three cupredoxin-like domains and is widely distributed in fungi, bacteria, and insects (Giardina et al., 2010; Hakulinen and Rouvinen, 2015). Laccases are multipurpose biocatalysts widely applied in industrial process (Virk et al., 2012; Rodríguez-Delgado et al., 2015; Teng et al., 2019; Backes et al., 2021). The majority of the characterized laccases so far are derived from white-rot fungi. Laccase derived from G. lucidum shows strong temperature and pH tolerance, as well as excellent performance on dye decolorization (Palazzolo et al., 2019), bioethanol production (Fang et al., 2015), lignocellulose degradation (Sitarz et al., 2013), and phenols degradation etc. (Brugnari et al., 2021). Additionally, heterologous expression of G. lucidum laccase in Pichia pastoris has been used for large-scale commercial production (You et al., 2013).
Evidences have shown that the production and activity of laccase are closely related to substrate constitutes (nutrient levels), and lignocellulosic compounds such as corncob, rice/wheat-straw, and even municipal food waste (Wang et al., 2015a; Gupta and Jana, 2019; Yuliana et al., 2020) can promote the production of G. lucidum laccase. Besides, the production and activity of G. lucidum laccase can be induced by exogenous additives (such as Cu2+, kraft lignin, ferulic acid, phenolic, glycerol and ethanol) and co-cultivation with other fungi (Li et al., 2011; Manavalan et al., 2013; Kuhar and Papinutti, 2014; Kuhar et al., 2015; Rodrigues et al., 2019). According to previous researches, the G. lucidum genome encoded 13 or 16 laccase genes in different strains (Chen et al., 2012; Liu et al., 2012), and different isozymes may have different expression patterns or differentially contribute to total laccase activity in Ganoderma species (Zhou et al., 2018; Qin et al., 2019; Ho et al., 2020). Studies have indicated that the expression of laccase is regulated by TFs (Polanco et al., 2006; Qi et al., 2017; Zhang et al., 2021). Moreover, allelic expression bias was reported in fungi (Ha et al., 2017; Gehrmann et al., 2018; Wang et al., 2021). To date, few studies on the regulation of laccase have been conducted and whether there is allelic expression bias in Ganoderma laccase has not been revealed.
AFRs, rich in lignocellulose consisting of lignin, cellulose, and hemicellulose, are misplaced resources. In this study, to investigate the utilization of G. lucidum mycelia of these carbon sources and to discover the key genes involved in the process, the mycelia of G. lucidum strain GL0102 was cultured on various carbon sources (19 kinds) and transcriptome sequencing were conducted. And the laccase gene family showing impressive performance in the transcriptome analysis was systematically studied based on two haploid genomes derived from two mating-compatible monokaryon strains. The findings here shed light on the gene expression and regulation of laccase that play important role in carbon utilization in G. lucidum.
Materials and Methods
Strains and Cultivation
The dikaryotic G. lucidum strain GL0102 (the same strain with “Zhi 102” of Mycological Research Center, Fujian Agriculture and Forestry University) was maintained on Difco™ Potato Dextrose Agar (PDA) at 4°C and stored at the Institute of Biological and Medical Engineering, Guangdong Academy of Sciences. Minimal medium plates containing glucose (20 g/l, MM), (NH4)2SO4 (1.5 g/l), K2HPO4 (1.0 g/l), MgSO4 (0.3 g/l), vitamin B1 (0.5 mg/l), and agar (18 g/l) were prepared. Then, the glucose was replaced with an equal amount of 17 kinds of other carbon sources (20 g/l, Table 1) to make media with different carbon sources. Specifically, the AFRs (such as rice-straw and wheat-straw) were dried and crushed using a pulverizer, and were added to 500 ml triangular flasks together with other components of the media. One rotor was put in each triangular flask before sterilization. Then the triangular flasks were stirred on a magnetic stirrer for 20 s. The media (<50°C) were poured rapidly into the plates (9 cm in diameter), and stirred for 3 s after each pouring to ensure the homogeneity of the medium. Besides, to speed up the solidification and avoid settling of insoluble components, the plate cover was open and the media was blown by sterile air. The medium preparation ensured maximal contact of the mycelia with the carbon sources. GL0102 was then inoculated into the above culture media and PDA plates and cultured at 28°C for 5 days. For media with MM, lignin (LM) and cellulose (CM), 4 mycelial blocks were inoculated in each plate, and 60 plates in total; for media with other carbon sources, one mycelial block was inoculated in each plate, and 30 plates in total. For each sample, the mycelia (approximately 10–20 plates) were quickly scraped and mixed to produce a biological replicate, which was then frozen in liquid nitrogen and stored at −80°C. Three replicates were prepared for each of the treatments. Besides, GL0102 mycelia cultured on the following six carbon sources for 3 days and 7 days were also collected in the same way: glucose, lignin, cottonseed hull, rice-straw, bagasse, and PDA. The growth rates of GL0102 on different carbon sources were determined at 28°C based on the hyphal radical length.
RNA-Sequencing and Expression Profile Analysis
RNA-Seq was performed from mycelia cultured on the above 19 different conditions for 5 days, each in triplicate. Extraction and quality control of the total RNA from each sample were conducted using methods previously reported (Wang et al., 2018), and RNA with RNA Integrity Number ≥ 7.5 was kept (Supplementary Table 1). cDNA library construction and sequencing were carried out following protocols of MGI sequencing platform and paired-end 150-bp reads were generated. Raw read quality was assessed using FastQC1, and low-quality bases or reads were filtered out using Skewer (Jiang et al., 2014) with parameters: “-q 20 -Q 30 -l 50” (Supplementary Table 2). Expression levels (transcript per million, TPM) of genes in mycelia cultured on different carbon sources were calculated using HISAT2 and StringTie (Pertea et al., 2016). Two reference haploid genomes, GL0102_8 and GL0102_53 (two mating-compatible monokaryons from GL0102)2 were downloaded from GPGD (Liao et al., 2021). Hierarchical clustering analysis of TPM values was performed using the “pheatmap” package3 in R. Differentially expressed genes (DEGs) were identified using the “DESeq2” package (Wang et al., 2010), using genome of GL0102_8 as the reference. DEGs were classified as genes that had log2FoldChange ≥2 and value of p ≤1e-6.
Gene Functional Enrichment Analysis
Gene ontology (GO) enrichment analysis was carried out using clusterProfiler (Yu et al., 2012), and enrichment results with a value of p <1 × 10−3 were retained.
Measurement of Laccase Activity
Ground powder (0.2 g) from mycelia in the presence of liquid nitrogen was extracted using 800 μl of 0.9% normal saline at 4°C for 10 min. After centrifuging (10,000 × g, 15 min, 4°C), the supernatant was used as the crude enzyme. The enzymatic activity of laccases was assayed by the ABTS (2, 2′-azino-bis-3-ethylbenzthiazoline-6-sulphonate) method (Johannes and Majcherczyk, 2000) using the following steps: 250 μl enzyme solution was added to the mixture of 500 μl acetate buffer (pH 4.5) and 250 μl ABTS (0.5 mm), then the absorbance at 420 nm was recorded within 3 min. One unit of enzyme activity was defined as the amount of laccase that oxidized 1 μm ABTS per minute.
Identification of Laccase Genes
Firstly, all the annotated proteins in GL0102_8 and GL0102_53 genomes were searched against the PFAM database (Pfam 32.0) using PfamScan (E value ≤1e-5).4 Genes with hits to PFAM ID PF00394.24, PF07731.16, and PF07732.17 were considered as the candidate laccase genes. Secondly, the genes were viewed and corrected using the Apollo browser (Dunn et al., 2019) as previously described (Wang et al., 2020). Thirdly, to rule out the false-positive results, all the laccase genes were reconfirmed using PfamScan. Finally, the whole set of laccase genes of GL0102_8 was searched against that of GL0102_53 using BlastP (Camacho et al., 2009). The allelic (one-to-one) relationship among these laccase genes was confirmed according to the amino acid (aa) similarity (identity ≥95%). For the other three individuals of G. lucidum, namely CGMCC5.0026 (Chen et al., 2012), P9 (single basidiospore; Liu et al., 2012), and Lingjian-2 (Tian et al., 2021), the laccase genes was identified following the same process above.
Sequence Information Analysis and Duplication Events Identification
The number of aa, isoelectric point (pI), and molecular weight (MW) were computed using ProtParam.5 The presence of signal peptide cleavage sites was obtained using SignalP 5.0.6 The domains of laccases were identified using PfamScan.7 The duplication of laccase genes was identified by the two criteria: (1) the protein length of the shorter sequence covered ≥75% of the longer sequence and (2) the similarity of the two aligned sequences was ≥90%.
Phylogenetic Analysis of Laccase Genes
A multiple sequence alignment of laccase genes in GL0102_8 and GL0102_53 was performed on full-length protein sequences using clustal (Sievers et al., 2011). A maximum likelihood (ML) phylogenetic tree was constructed using RAxML (Stamatakis, 2014) with 1,000 bootstrap replicates. In addition, a ML phylogenetic tree containing laccase protein sequences in three additional G. lucidum individuals (CGMCC5.0026, P9, and Lingjian-2) was built following the same process.
Genetic Variation Detection and SNP Validation Between Paired Allelic Laccase Genes
Genetic variations were detected using MEGA7 (Kumar et al., 2016) after alignment using clustal, and the exhibition of SNPs were plotted using trackViewer (Ou and Zhu, 2019). To validate the SNP loci, the cDNA derived from mycelia cultured on RSM was used as PCR amplification template. The synthesizing of primers (Supplementary Table 3), cloning and sequencing of amplification products were conducted by Tsingke Biotechnology Co., Ltd.
Expression Analysis by Quantitative PCR
RNA samples for sequencing were further synthesized into cDNA. Glyceraldehyde 3-phosphate dehydrogenase (gapdh) was used as a reference. Primers for the laccase genes (Supplementary Table 3) were designed and synthesized by Tsingke Biotechnology Co., Ltd. The qPCR reaction was performed using the Applied Biosystems ABI 7500 PCR System (ABI, United States) according to previously reported methods (Wang et al., 2017). The PCR amplification mixture contained 1 μl of cDNA, 10 μl of ChamQ Universal SYBR qPCR Master Mix (Vazyme Biotech Co., Ltd), 0.4 μl of 10 μm forward and reverse primers, and 8.2 μl ddH2O. The PCR reaction was performed with the initial denaturation step for 3 min at 95°C; 40 cycles of 3 s at 95°C and annealing at 60°C for 32 s, and a holding step for 30 s at 72°C. The melting curve (60–95°C) and agarose gel electrophoresis of qPCR products were used to check the specificity of each qPCR reaction. The standard curves were generated using a twofold dilution gradient of the cDNA. Amplification efficiencies (E = 10-1/slope–1) and correlation coefficient (R2) values were calculated by standard curves. The relative gene expression was calculated with the 2–ΔΔCT method (Livak and Schmittgen, 2001). Parametric one-way analysis of variance (ANOVA) followed by Duncan’s test was used to calculate significant differences among different groups (p < 0.05).
Detection and Validation of Allelic Expression Bias
The allelic expression bias was identified based on RNA-Seq read depths of SNP loci between alleles. The validation of allelic expression bias was conducted as follows: (1) primers were designed at flanking regions of SNP locus (Supplementary Table 3); (2) PCR amplification was conducted using cDNA derived from mycelia cultured on RSM; and (3) PCR products was cloned and then randomly selected and sequenced at Tsingke Biotechnology Co., Ltd.
Co-expression Analysis of Laccase Genes
Co-expression analysis was conducted by Multiscale Embedded Gene Co-expression Network Analysis (MEGENA) pipeline (Song and Zhang, 2015) as follows: (1) pairwise Pearson correlation coefficients of all the DEGs were calculated based on expression TPM values; (2) planar filtered network was set up by taking significant correlation pairs; and (3) multiple correspondence analysis was employed to cluster genes into modules.
Promoter Sequence Analysis and Prediction of Binding Sites
The 3 kb upstream of the start codon of laccase was extracted as the promoter region. Promoter sequences were aligned and analyzed by MEGA7. The binding sites matrix of fungi were downloaded from JASPAR8, and CiiiDER9 were used to predict the possible binding sites.
Results
Mycelia Cultured on Different Carbon Sources and Gene Expression Pattern
The G. lucidum strain GL0102 was cultured on a total of 19 kinds of media with different carbon sources as solo carbon source (Table 1), respectively, and the growth rate was calculated accordingly. Mycelia cultured on media with glucose (MM), lignin (LM) or cellulose (CM) as carbon sources were sparse and had much lower growth rate (0.17 ± 0.004 to 0.37 ± 0.008 cm/d; Figure 1A; Supplementary Figure 1), while mycelia cultured on media with AFRs as carbon sources were lush and had higher growth rate, especially on wheat-straw (WSM), corncob (COM), pteridophyte (PTM), and chestnut leaf (CLM; 0.76 ± 0.015 to 0.80 ± 0.012 cm/d; Figure 1A; Supplementary Figure 1). This indicates that single-component carbon sources are possibly not comparable to complex carbon sources (AFRs) for mycelia production of G. lucidum.
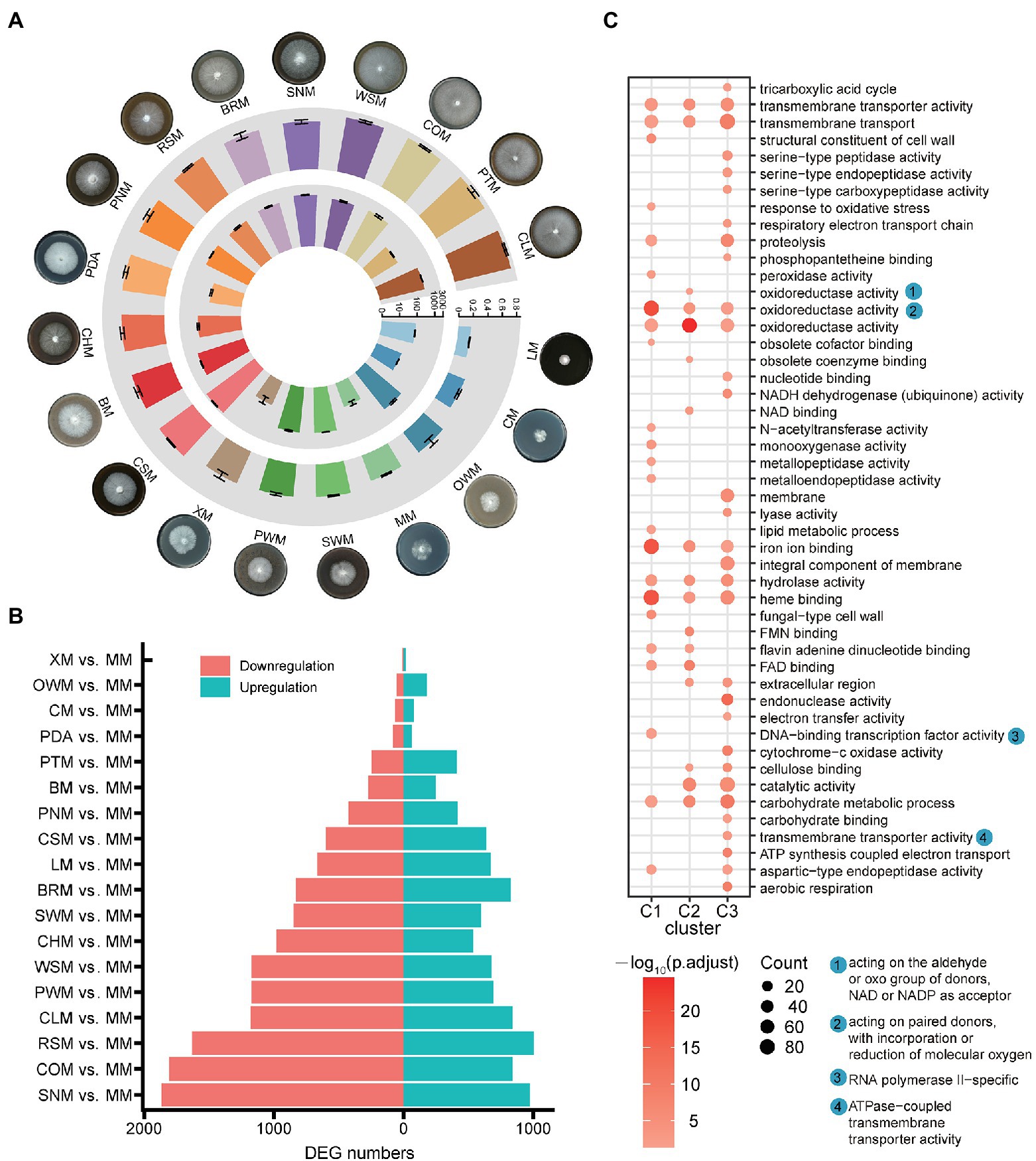
Figure 1. Ganoderma lucidum mycelia cultured on different carbon sources and differentially expressed genes. (A) Growth status of mycelia (outer circle), growth rate of mycelia (middle circle, cm/d), and laccase activity of mycelia (inner circle, U/mg). (B) DEGs in the comparison of MM with all the other carbon sources. (C) Functional enrichment of DEGs. Glucose (MM), lignin (LM), cellulose (CM), xylan (XM), corncob (COM), cottonseed hull (CHM), wheat-straw (WSM), rice-straw (RSM), bran (BRM), pine wood sawdust (PWM), pine needle (PNM), spruce wood sawdust (SWM), spruce needle (SNM), pteridophyte (PTM), chestnut shell (CSM), chestnut leaf (CLM), bagasse (BM), oak wood sawdust (OWM), and potato dextrose agar (PDA).
To uncover the key genes involved in mycelia growth on different carbon sources, we compared the gene expression data obtained from the mycelia cultured on 18 carbon sources with that on glucose (MM), respectively. Among all the pairwise comparisons, 7 (XM vs. MM) to 1,865 (SNM vs. MM) genes were downregulated, while 16 (XM vs. MM) to 1,006 (RSM vs. MM) were upregulated (Figure 1B). According to their expression profile, all the DEGs could be divided into three main clusters (Supplementary Figure 2). Genes in cluster 1 showed relatively high expression on MM, XM, CM, and PDA; genes in cluster 2 showed relatively high expression on LM; and genes in cluster 3 showed relatively high expression on carbon sources derived from AFRs. Besides, the genes of these three clusters all showed enrichment in carbohydrate degradation-related functions, such as oxidoreductase activity, hydrolase activity, and carbohydrate metabolic process (Figure 1C).
Notably, the laccase gene family, which is closely related with lignin degradation, showed significant expression dynamics when the mycelia was cultured on different carbon sources. Thus, the laccase activity was tested. The mycelia cultured on single-component carbon source showed quite low laccase activity, such as 26 ± 7.3 U/mg on MM, 29 ± 13.1 U/mg on XM, and 32 ± 0.78 U/mg on CM (Figure 1A), while laccase activity of mycelia cultured on AFRs (except PTM) was significantly induced, showing values greater than 281 ± 35.4 U/mg (Figure 1A; Supplementary Figure 1). Specially, mycelia cultured on CSM showed laccase activity of 2,410 ± 122.4 U/mg which was much higher than those on other carbon sources (Figure 1A). Given that there are multiple laccase members in the G. lucidum genome, and the characteristics of each laccase member and their contribution to laccase activity may be different (Wang et al., 2015b; Ha et al., 2017; Kumar et al., 2017), comprehensive analysis regarding G. lucidum laccase was then performed.
Genome-Wide Identification and Characterization of Ganoderma lucidum Laccase Genes
Totally, 15 and 16 laccase genes were identified in GL0102_53 and GL0102_8, respectively. According to the sequence similarity, two laccase genes in GL0102_8 were speculated to derived from duplication event. Hence, laccase genes in the two genomes were divided into 15 categories (Gllac1-Gllac15), and the two duplicated laccase genes in GL0102_8 were separately named as H8lac7_1 and H8lac7_2 (Supplementary Table 4). The laccase genes located on seven chromosomes of GL0102_8 and GL0102_53 and emerged the most frequently on chromosome 6 (Supplementary Table 4). Paired allelic laccase genes showed highly similar sequence features in protein length, pI, and MW. For example, H8lac14 and H53lac14 had minimal protein length of 460 aa, and H8lac7_1 and H53lac7 had maximal protein length of 667 aa (Supplementary Table 4). All the laccase genes were predicted to encode secreted proteins.
A ML phylogenetic tree of laccase genes of GL0102 was constructed, and all paired allelic laccase genes clustered with each other (Figure 2A). It was reported that G. lucidum strain CGMCC5.0026 and P9 had 13 (Chen et al., 2012) and 16 (Liu et al., 2012) laccase genes, while 15 and 17 laccase genes were identified, respectively, after the manual correction in this study (Gllac5 and Gllac6 each had one more copy in P9; Supplementary Table 5). Besides, 15 laccase genes were identified in G. lucidum strain Lingjian-2 (Supplementary Table 5). In the phylogenetic tree constructed with all the above laccase genes, each of the 15 laccase categories from different strains/haploids were clustered together (Supplementary Figure 3), indicating that laccase genes were relatively conserved in G. lucidum.
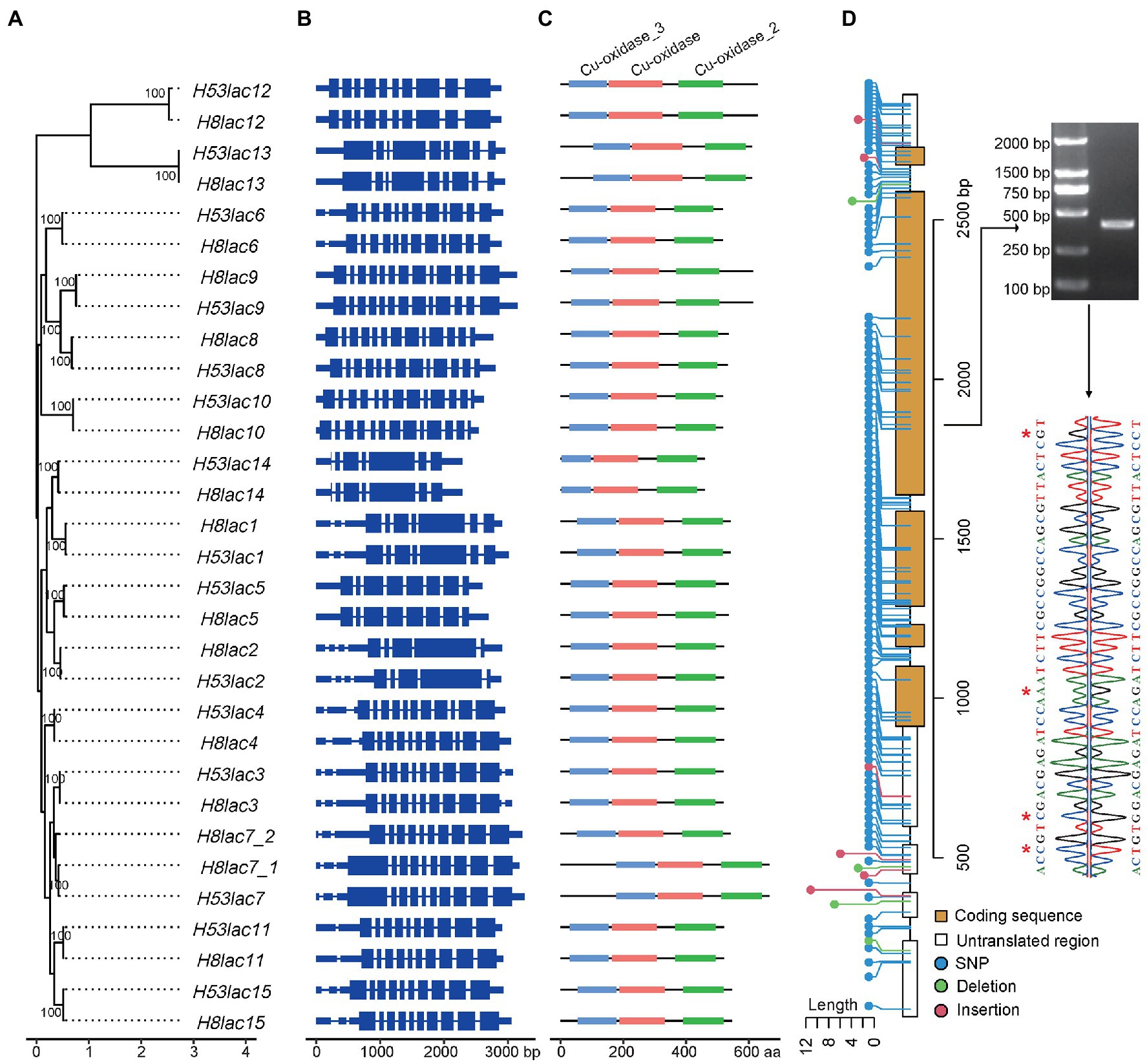
Figure 2. Characteristics of allelic laccase genes in G. lucidum. (A) Phylogenetic relationships (numbers on the nodes represent supporting values). (B) Gene structures. Blue rectangles represent the coding sequences, thin blue lines connecting two exons represent introns, and thick blue lines represent 5′-UTR or 3′-UTR. (C) Cu-domain. (D) Genetic variations, agarose gel electrophoresis, and Sanger sequencing of Gllac2. Red stars represent SNP loci.
As shown in Figure 2B, paired allelic laccases presented highly conserved gene structures and possessed relatively large number of introns, for example, each of Gllac8, Gllac9, and Gllac10 had 12 introns. Each laccase contained three conserved domains: Cu-oxidase (PF00394.24), Cu-oxidase_2 (PF07731.16), and Cu-oxidase_3 (PF07732.17; Figure 2C).
Genetic variations were detected between paired allelic laccase genes, and SNPs were identified as the most abundant variations (Supplementary Table 6). Among all the allelic laccase pairs, 1 to 48 SNPs causing 0 to 16 amino acid changes were observed in coding regions, while none of the amino acid changes occurred in laccase signature sequence regions (Supplementary Table 6; Supplementary Figure 4). In general, the SNP rate was higher in untranslated regions (UTR) and introns. For example, a total of 113 SNPs and 10 insertions/deletions were identified in genic regions between Gllac2 alleles, of which 42 SNPs existed in coding regions (Figure 2D). Randomly selected SNP loci between H53lac2 and H8lac2 were confirmed by Sanger sequence (Figure 2D).
Expression Patterns of Laccase Genes on Different Carbon Sources
The expression pattern of laccase genes on different carbon sources was further analyzed based on the RNA-Seq data using GL0102_8 genome as reference. Nine laccase genes (Gllac1, Gllac4, Gllac6, Gllac8, Gllac9, Gllac10, Gllac11, Gllac15, and H8lac7_2) showed quite low expression level (barely detectable, TPM value <10 under most conditions) under all the testing conditions (except that H8lac7_2 showed relatively high expression level on LM), while the other seven laccase genes (Gllac2, Gllac3, Gllac5, Gllac7, Gllac12, Gllac13 and Gllac14) showed high expression level especially on complex carbon sources (TPM value >100 under certain conditions; Figure 3A). Notably, Gllac2 and Gllac7 were the top two expressed laccase genes on complex carbon sources. Differential expression analysis was conducted on all carbon sources pairwisely. In all comparisons, 1 to 10 laccases were found differentially expressed. And the maximal number of differentially expressed laccase genes were found in the comparisons of XM vs. SNM and PDA vs. SNM (Supplementary Figure 5).
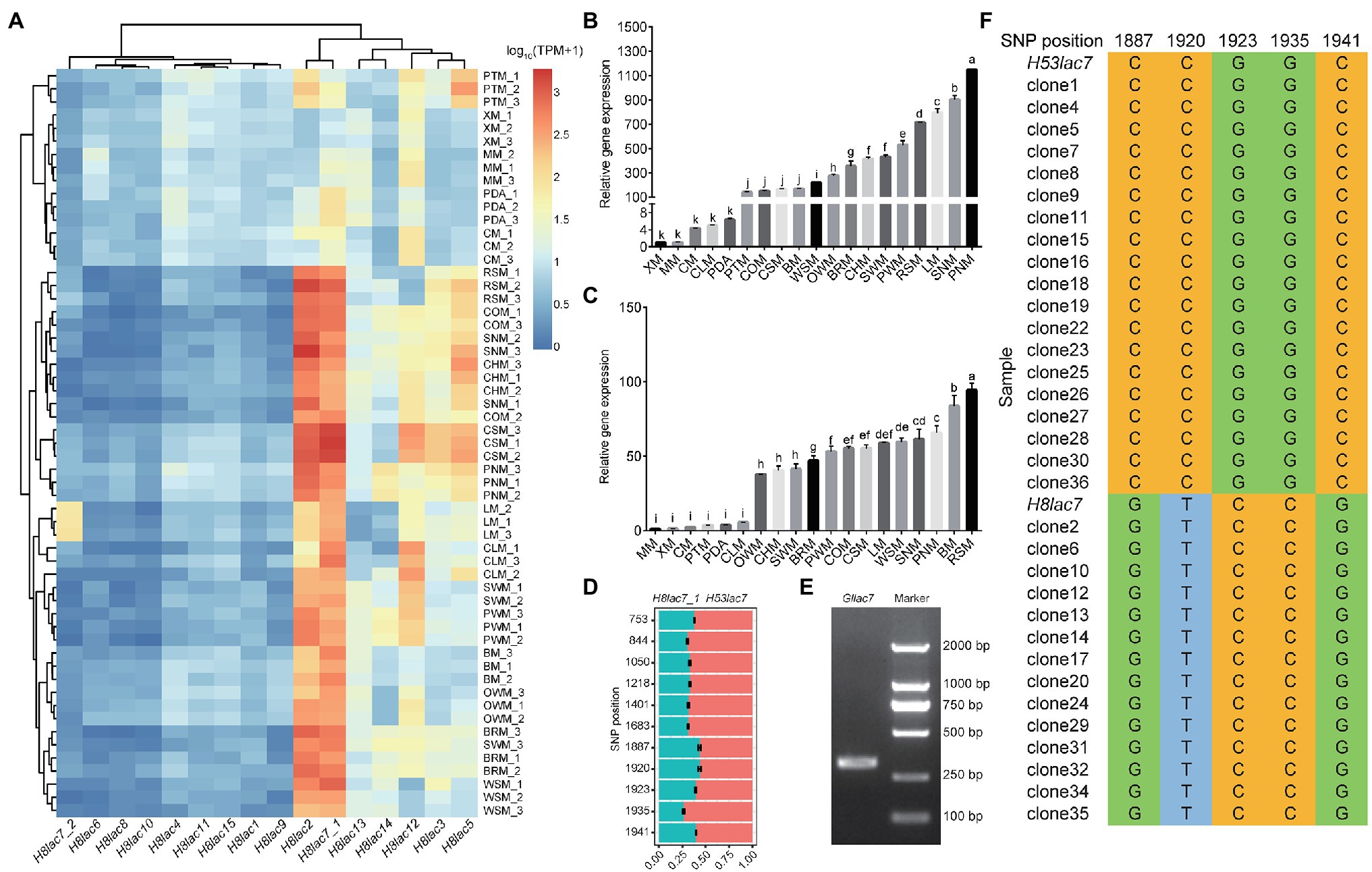
Figure 3. Expression patterns of laccase genes in G. lucidum. (A) Expression levels of 16 laccase genes on different carbon sources. (B) Relative expression of Gllac2 detected by qPCR. (C) Relative expression of Gllac7 detected by qPCR. (D) Read count of allelic Gllac7 based on SNP loci in the mycelia cultured on RSM. (E) Agarose gel electrophoresis of PCR amplification products of partial Gllac7 (311 bp). (F) SNP loci validation by clone sequencing. Glucose (MM), lignin (LM), cellulose (CM), xylan (XM), corncob (COM), cottonseed hull (CHM), wheat-straw (WSM), rice-straw (RSM), bran (BRM), pine wood sawdust (PWM), pine needle (PNM), spruce wood sawdust (SWM), spruce needle (SNM), pteridophyte (PTM), chestnut shell (CSM), chestnut leaf (CLM), bagasse (BM), oak wood sawdust (OWM), and potato dextrose agar (PDA). The error bars with different letters over the columns denote significant differences (p < 0.05).
To test if the laccase expression was related with different growth stages, GL0102 mycelia cultured for 3, 5, and 7 days on MM, LM, CHM, RSM, BM, and PDA were collected, respectively. And the transcription levels of Gllac2, Gllac4, Gllac7, Gllac9, Gllac10, and Gllac12 were analyzed by qPCR after we evaluated multiple parameters of the qPCR reaction and confirmed their acceptability (Supplementary Figure 6). Overall, the expression levels of Gllac2 and Gllac7 were highest in mycelia cultured for 3 days (on LM, RSM, BM and CHM) and decreased gradually with the increase of culture time (Supplementary Figure 7). Specifically, compared to 5d-mycelia cultured on MM, Gllac2 showed 5-fold (on CLM) to 1,149-fold (on PNM) upregulation and Gllac7 showed 4-fold (on PTM) to 94-fold (on RSM) upregulation on complex carbon sources (Figures 3B,C). Gllac4, Gllac9 and Gllac10 maintained low expression levels from 3 to 7 days (Supplementary Figure 7).
Allelic Expression Bias Analysis of Gllac7
Further analysis showed that the two nuclei in G. lucidum contributed unevenly to the total expression of laccase, in other words, allelic expression bias was observed in laccase. On all the 19 carbon sources except XM, the expression of H53lac7 was consistently higher than that of H8lac7_1 (value of p < 0.05), and the read count ratio of H53lac7 ranged from 51.71 to 76.54% with an average of 62.6% (Figure 3D; Supplementary Figure 8). To confirm this allelic bias case, partial sequences of Gllac7 (311 bp), which contains SNP loci, were amplified using cDNA derived from RSM-cultured mycelia (Figure 3E). After cloning and sequencing, 19 out of 33 clones were consistent with H53lac7, while 14 clones were consistent with H8lac7_1 (Figure 3F), confirming the existing of allelic expression bias. To analyze the possible causes of allelic expression bias, we analyzed the promoter regions of H8lac7_1 and H53lac7. Totally, 47 SNPs and three insertions/deletions with length of 5, 8 and 9 bp were identified. These variants may cause differences in binding sites of transcription factors. For example, zinc fingers, a type of important regulators, have many binding sites in the promoter of Gllac7, while differences of binding sites were observed between promoters of H53lac7 and H8lac7_1 (Figure 4A).
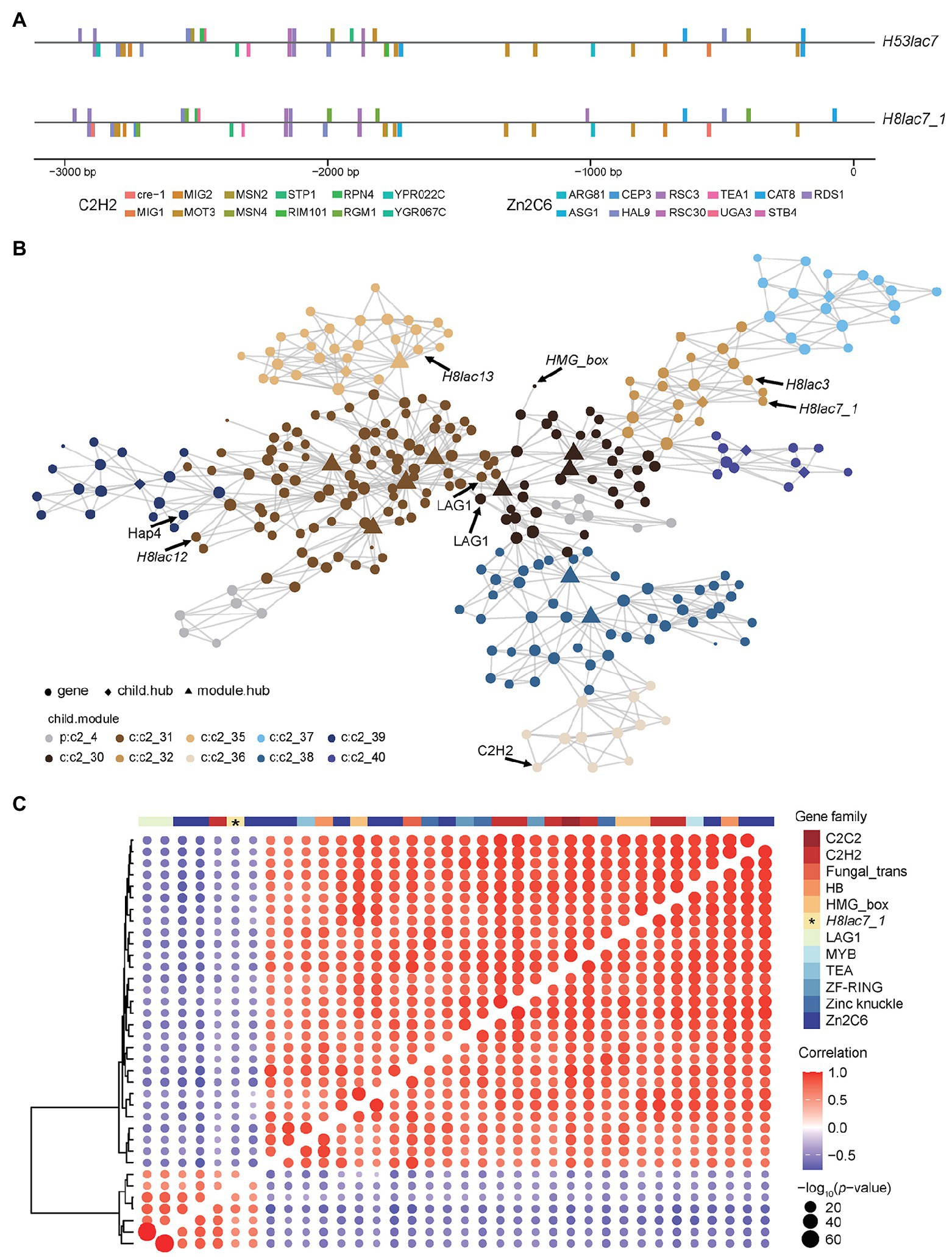
Figure 4. TF binding site analysis and co-expression network of Gllac7. (A) Predicted binding sites of zinc finger proteins in promoters of H53lac7 and H8lac7_1. (B) The co-expression network of genes in the module containing Gllac7. (C) Correlation of Gllac7 and TFs.
A co-expression network was built based on expression levels of DEGs (in GL0102_8) using MEGENA pipeline (Song and Zhang, 2015). In the co-expression network, Gllac7 (H8lac7_1), H8lac3, H8lac12, and H8lac13 were clustered into a shared module (Figure 4B). This module contained 299 genes, among which five were TFs. Overall, there were 68 TFs in the DEGs and most TFs were downregulated on complex carbon sources. Moreover, Pearson correlation analysis between Gllac7 and these TFs indicated that 35 TFs were significantly correlated with Gllac7, of which six TFs showed positive correlation, while the other 29 TFs showed negative correlation with Gllac7 (Figure 4C). Most of these TFs belong to zinc finger family such as C2H2 (7/35) and Zn2C6 (12/35).
Discussion
Carbon source affects the growth and metabolism of fungi (Wu et al., 2019; Lok et al., 2021; Zhou et al., 2021). G. lucidum has a relative wide carbon spectrum (Hapuarachchi et al., 2018; Kurd-Anjaraki et al., 2022), enabling it to be an ideal model fungus in research on carbon source utilization. AFRs, numerous in variety, are high quality biomass for G. lucidum cultivation. Thus, in this study, a total of 13 byproducts of agricultural (cottonseed hull, corncob, wheat-straw, rice-straw, bran, bagasse) and forestry (sawdust and leaf), and a widely distributed pteridophyte (Dicranopteris pedata) were used in mycelial cultivation of G. lucidum. Transcriptome analysis allows us to understand the overall impact of changes in carbon sources on gene expression network as a whole. In this study, up to 1,006 upregulation genes and 1,865 downregulation genes showed differential expression levels on divergent carbon sources (glucose as the control). Genes with function of oxidoreductase activity were significantly enriched, indicating their universal and indispensable roles in carbon utilization.
Due to its complex heterostructure, lignin becomes a natural antidegradation barrier of lignocellulose (Kumar and Chandra, 2020). Considering the vital function of laccase in lignin degradation, we conducted a comprehensive analysis toward laccase gene family of G. lucidum. Macro-fungi species usually contain more than 10 laccase genes, e.g., 14 in Lentinula edodes (Yan et al., 2019), 12 in Pleurotus ostreatus (Jiao et al., 2018), and 11 in Flammulina velutipes (Wang et al., 2015b). And it is assumed that the presence of large gene families of laccases in fungal genomes could be explained by broad spectrum of enzyme’s physiological functions required during the life cycle of a fungus (Kües and Rühl, 2011). In this study, 15 allelic laccase genes were identified in two haploid genomes (GL0102_8 and GL0102_53) of a G. lucidum strain GL0102, and H8lac7_2 was possibly formed by gene duplication of H8lac7_1 in GL0102_8. In addition, 15 laccase categories were confirmed in each of G. lucidum strain CGMCC5.0026, P9, and Lingjian-2 after a rigorously manual correction. The aforementioned results indicated that the 15 laccase categories in G. lucidum species are relatively conserved. Allelic laccase genes showed similar features (sequence characteristics/gene structures) and clustered together in the phylogenetic tree. Yet a certain number of genetic variations, especially SNPs, were identified in paired allelic laccase genes, indicating genetic diversity of allelic laccase genes in G. lucidum. Although SNPs caused several amino acid changes between allelic laccases of G. lucidum, none of the changes were located in conserved domain. More SNPs are located in non-coding regions which may have substantial impact on gene regulation, thereby contributing to phenotypic diversity (Albert and Kruglyak, 2015; van Arensbergen et al., 2019).
Although a relatively large number of laccase genes were existed in macro-fungi, only one or a few laccase isozymes showed high expression abundance (Ha et al., 2017), and a few laccases showed activity in the Native-PAGE under one or some given conditions (Wang et al., 2015a; Kumar et al., 2017). In this study, laccase activity of mycelia cultured on LM was medium, less than that on AFRs. Compared to AFRs, the ingredients of commercial lignin (LM) are more homogeneous. The lignin with complex structures and categories in AFRs was considered to have stronger induction for laccase activity. Laccases may have functional differentiation and different members under fine regulation. Thus, the relatively large laccase gene members may be a result of function redundancy, or the appropriate expression conditions for some laccase genes have not been revealed. Enhanced understanding of genetic mechanisms of laccase expression and regulation would benefit for optimal laccase production in G. lucidum using biotechnologies. In this study, more than half (9) of the G. lucidum laccase genes showed quite low expression level under all the testing conditions, while the other seven laccase genes showed obviously higher expression level in complex carbon sources than in single-component carbon sources. The induced gene expression may contribute to the fast growth and high laccase activity of G. lucidum mycelia cultured on complex carbon sources. Among all the laccase genes, Gllac2 and Gllac7 showed maximal expression abundance on complex carbon sources especially in the early stage of mycelial culture, indicating their inducible transcription by exogenous polysaccharides.
In diploid basidiomycetes, two nuclei co-exist in the common cytoplasm but divide independently (Clark and Anderson, 2004). Thus, whether one of the nuclei is dominant in allelic gene expression is worthy exploring. To date, studies on allele-specific gene expression have mainly focused on humans or animals (Tian et al., 2018; Tangwancharoen et al., 2020), with only a few cases having been reported in fungi. According to Ha et al, expression bias in the allelic laccase genes of two nuclei of L. edodes was detected (Ha et al., 2017). In this study, allelic expression bias was found in Gllac7, and the expression of H53lac7 was consistently higher than that of H8lac7_1 under the testing conditions (except on XM). The coordination of allelic genes in the expression may be a result of environmental adaptation, and allelic expression bias may contribute to formation of physiological characteristics of a nucleus, which will be useful in elite strain selection.
In carbon metabolism, zinc finger is a vital regulator, and the C2H2 zinc finger CreA has been widely identified as a universal carbon catabolism repressor (Kunitake et al., 2019; Hu et al., 2020). And in G. lucidum, PacC, a C2H2 zinc finger was proved to negatively regulate laccase activity (Zhu et al., 2022). In this study, zinc finger TFs showed significant correlation with expression of Gllac7, and many binding sites of zinc finger proteins were detected in the Gllac7 promoter, indicating their potential regulation roles of Gllac7.
Data Availability Statement
The datasets presented in this study can be found in online repositories. The names of the repository/repositories and accession number(s) can be found at: http://www.gpgenome.com/species/408.
Author Contributions
LW, LL, and QW conceived the project. QH, BH, XD, and QW performed the experiments. LW and QW analyzed the data. LW drafted the manuscript. LW and XD revised the manuscript. All authors contributed to the article and approved the submitted version.
Funding
This research was funded by GDAS’ Project of Science and Technology Development (2020GDASYL-20200103071 and 2022GDASZH-2022010110), Guangzhou Science and Technology Program Project (202103000080), Special Support Program of Guangdong Province (2019TQ05N232), and Heyuan Science and Technology Program Project (HYZP201909007).
Conflict of Interest
The authors declare that the research was conducted in the absence of any commercial or financial relationships that could be construed as a potential conflict of interest.
Publisher’s Note
All claims expressed in this article are solely those of the authors and do not necessarily represent those of their affiliated organizations, or those of the publisher, the editors and the reviewers. Any product that may be evaluated in this article, or claim that may be made by its manufacturer, is not guaranteed or endorsed by the publisher.
Supplementary Material
The Supplementary Material for this article can be found online at: https://www.frontiersin.org/articles/10.3389/fmicb.2022.890686/full#supplementary-material
Footnotes
1. ^http://www.bioinformatics.babraham.ac.uk/projects/fastqc/
2. ^http://www.gpgenome.com/species/408
3. ^https://cran.rproject.org/web/packages/pheatmap/
4. ^http://www.ebi.ac.uk/Tools/pfa/pfamscan
5. ^https://web.expasy.org/protparam/
6. ^http://www.cbs.dtu.dk/services/SignalP/
References
Albert, F. W., and Kruglyak, L. (2015). The role of regulatory variation in complex traits and disease. Nat. Rev. Genet. 16, 197–212. doi: 10.1038/nrg3891
Backes, E., Kato, C. G., Corrêa, R. C. G., Peralta Muniz Moreira, R. D. F., Peralta, R. A., Barros, L., et al. (2021). Laccases in food processing: current status, bottlenecks and perspectives. Trends Food Sci. Technol. 115, 445–460. doi: 10.1016/j.tifs.2021.06.052
Bishop, K. S., Kao, C. H. J., Xu, Y., Glucina, M. P., Paterson, R. R. M., and Ferguson, L. R. (2015). From 2000 years of Ganoderma lucidum to recent developments in nutraceuticals. Phytochemistry 114, 56–65. doi: 10.1016/j.phytochem.2015.02.015
Brugnari, T., Contato, A. G., Pereira, M. G., Freitas, E. N. D., Bubna, G. A., Aranha, G. M., et al. (2021). Characterisation of free and immobilised laccases from Ganoderma lucidum: application on bisphenol a degradation. Biocatal. Biotransform. 39, 71–80. doi: 10.1080/10242422.2020.1792448
Camacho, C., Coulouris, G., Avagyan, V., Ma, N., Papadopoulos, J., Bealer, K., et al. (2009). BLAST+: architecture and applications. BMC Bioinformat. 10:421. doi: 10.1186/1471-2105-10-421
Chen, S. L., Xu, J., Liu, C., Zhu, Y. J., Nelson, D. R., Zhou, S. G., et al. (2012). Genome sequence of the model medicinal mushroom Ganoderma lucidum. Nat. Commun. 3:913. doi: 10.1038/ncomms1923
Clark, T. A., and Anderson, J. B. (2004). Dikaryons of the basidiomycete fungus Schizophyllum commune: evolution in long-term culture. Genetics 167, 1663–1675. doi: 10.1534/genetics.104.027235
Das, N., Sengupta, S., and Mukherjee, M. (1997). Importance of laccase in vegetative growth of Pleurotus florida. Appl. Environ. Microbiol. 63, 4120–4122. doi: 10.1128/aem.63.10.4120-4122.1997
Dunn, N. A., Unni, D. R., Diesh, C., Munoz-Torres, M., Harris, N. L., Yao, E., et al. (2019). Apollo: democratizing genome annotation. PLoS Comput. Biol. 15:e1006790. doi: 10.1371/journal.pcbi.1006790
Fang, Z. M., Liu, X. M., Chen, L. Y., Shen, Y., Zhang, X. C., Fang, W., et al. (2015). Identification of a laccase Glac15 from Ganoderma lucidum 77002 and its application in bioethanol production. Biotechnol. Biofuels 8, 54–12. doi: 10.1186/s13068-015-0235-x
Gehrmann, T., Pelkmans, J. F., Ohm, R. A., Vos, A. M., Sonnenberg, A. S. M., Baars, J. J. P., et al. (2018). Nucleus-specific expression in the multinuclear mushroom-forming fungus Agaricus bisporus reveals different nuclear regulatory programs. P. Natl. Acad. Sci. USA 115, 4429–4434. doi: 10.1073/pnas.1721381115
Giardina, P., Faraco, V., Pezzella, C., Piscitelli, A., Vanhulle, S., and Sannia, G. (2010). Laccases: a never-ending story. Cell. Mol. Life Sci. 67, 369–385. doi: 10.1007/s00018-009-0169-1
Gupta, A., and Jana, A. K. (2019). Production of laccase by repeated batch semi-solid fermentation using wheat straw as substrate and support for fungal growth. Bioprocess Biosyst. Eng. 42, 499–512. doi: 10.1007/s00449-018-2053-6
Ha, B., Lee, S., Kim, S., Kim, M., Moon, Y. J., Song, Y., et al. (2017). Nucleus-selective expression of laccase genes in the dikaryotic strain of Lentinula edodes. Mycobiology 45, 379–384. doi: 10.5941/MYCO.2017.45.4.379
Hakulinen, N., and Rouvinen, J. (2015). Three-dimensional structures of laccases. Cell. Mol. Life Sci. 72, 857–868. doi: 10.1007/s00018-014-1827-5
Hapuarachchi, K. K., Elkhateeb, W. A., Karunarathna, S. C., Cheng, C. R., Bandara, A. R., Kakumyan, P., et al. (2018). Current status of global Ganoderma cultivation, products, industry and market. Mycosphere 9, 1025–1052. doi: 10.5943/mycosphere/9/5/6
Ho, P. Y., Namasivayam, P., Sundram, S., and Ho, C. L. (2020). Expression of genes encoding manganese peroxidase and laccase of Ganoderma boninense in response to nitrogen sources, hydrogen peroxide and phytohormones. Gen. Dent. 11:1263. doi: 10.3390/genes11111263
Hu, Y. R., Xu, W. Z., Hu, S. S., Lian, L. D., Zhu, J., Shi, L., et al. (2020). In Ganoderma lucidum, Glsnf1 regulates cellulose degradation by inhibiting GlCreA during the utilization of cellulose. Environ. Microbiol. 22, 107–121. doi: 10.1111/1462-2920.14826
Jiang, H. S., Lei, R., Ding, S. W., and Zhu, S. F. (2014). Skewer: a fast and accurate adapter trimmer for next-generation sequencing paired-end reads. BMC Bioinformat. 15:182. doi: 10.1186/1471-2105-15-182
Jiao, X. Y., Li, G. Q., Wang, Y., Nie, F., Cheng, X., Abdullah, M., et al. (2018). Systematic analysis of the Pleurotus ostreatus laccase gene (PoLac) family and functional characterization of PoLac2 involved in the degradation of cotton-straw lignin. Molecules 23:880. doi: 10.3390/molecules23040880
Jin, W. S., Li, J. H., Feng, H. C., You, S., Zhang, L. Y., Norvienyeku, J., et al. (2018). Importance of a laccase gene (Lcc1) in the development of Ganoderma tsugae. Int. J. Mol. Sci. 19:471. doi: 10.3390/ijms19020471
Johannes, C., and Majcherczyk, A. (2000). Laccase activity tests and laccase inhibitors. J. Biotechol. 78, 193–199. doi: 10.1016/S0168-1656(00)00208-X
Khoo, S. C., Ma, N. L., Peng, W. X., Ng, K. K., Goh, M. S., Chen, H. L., et al. (2022). Valorisation of biomass and diaper waste into a sustainable production of the medical mushroom Lingzhi Ganoderma lucidum. Chemosphere 286:131477. doi: 10.1016/j.chemosphere.2021.131477
Kües, U., and Rühl, M. (2011). Multiple multi-copper oxidase gene families in basidiomycetes - what for? Curr. Genomics 12, 72–94. doi: 10.2174/138920211795564377
Kuhar, F., Castiglia, V., and Levin, L. (2015). Enhancement of laccase production and malachite green decolorization by co-culturing Ganoderma lucidum and Trametes versicolor in solid-state fermentation. Int. Biodeterior. Biodegradation 104, 238–243. doi: 10.1016/j.ibiod.2015.06.017
Kuhar, F., and Papinutti, L. (2014). Optimization of laccase production by two strains of Ganoderma lucidum using phenolic and metallic inducers. Rev. Argent. Microbiol. 46, 144–149. doi: 10.1016/s0325-7541(14)70063-x
Kumar, A., and Chandra, R. (2020). Ligninolytic enzymes and its mechanisms for degradation of lignocellulosic waste in environment. Heliyon 6:e03170. doi: 10.1016/j.heliyon.2020.e03170
Kumar, A., Singh, D., Sharma, K. K., Arora, S., Singh, A. K., Gill, S. S., et al. (2017). Gel-based purification and biochemical study of laccase isozymes from Ganoderma sp. and its role in enhanced cotton callogenesis. Front. Microbiol. 8:674. doi: 10.3389/fmicb.2017.00674
Kumar, S., Stecher, G., and Tamura, K. (2016). MEGA7: molecular evolutionary genetics analysis version 7.0 for bigger datasets. Mol. Biol. Evol. 33, 1870–1874. doi: 10.1093/molbev/msw054
Kunitake, E., Li, Y., Uchida, R., Nohara, T., Asano, K., Hattori, A., et al. (2019). CreA-independent carbon catabolite repression of cellulase genes by trimeric G-protein and protein kinase A in Aspergillus nidulans. Curr. Genet. 65, 941–952. doi: 10.1007/s00294-019-00944-4
Kurd-Anjaraki, S., Ramezan, D., Ramezani, S., Samzadeh-Kermani, A., Pirnia, M., and Shahi, B. Y. (2022). Potential of waste reduction of agro-biomasses through Reishi medicinal mushroom (Ganoderma lucidum) production using different substrates and techniques. Acta Ecol. Sin. 42, 90–101. doi: 10.1016/j.chnaes.2021.04.010
Li, P., Wang, H. L., Liu, G. S., Li, X., and Yao, J. M. (2011). The effect of carbon source succession on laccase activity in the co-culture process of Ganoderma lucidum and a yeast. Enzym. Microb. Technol. 48, 1–6. doi: 10.1016/j.enzmictec.2010.07.005
Liao, B. S., Hu, H. Y., Xiao, S. M., Zhou, G. R., Sun, W., Chu, Y., et al. (2021). Global pharmacopoeia genome database is an integrated and mineable genomic database for traditional medicines derived from eight international pharmacopoeias. Sci. China Life Sci. 65, 809–817. doi: 10.1007/s11427-021-1968-7
Liu, D. B., Gong, J., Dai, W. K., Kang, X. C., Huang, Z., Zhang, H. M., et al. (2012). The genome of Ganoderma lucidum provides insights into triterpenes biosynthesis and wood degradation. PLoS One 7:e36146. doi: 10.1371/journal.pone.0036146
Livak, K. J., and Schmittgen, T. D. (2001). Analysis of relative gene expression data using real-time quantitative PCR and the 2−ΔΔCT method. Methods 25, 402–408. doi: 10.1006/meth.2001.1262
Lok, B., Adam, M. A. A., Kamal, L. Z. M., Chukwudi, N. A., Sandai, R., and Sandai, D. (2021). The assimilation of different carbon sources in Candida albicans: fitness and pathogenicity. Med. Mycol. 59, 115–125. doi: 10.1093/mmy/myaa080
Lu, Y. P., Wu, G. M., Lian, L. D., Guo, L. X., Wang, W., Yang, Z. Y., et al. (2015). Cloning and expression analysis of Vvlcc3, a novel and functional laccase gene possibly involved in stipe elongation. Int. J. Mol. Sci. 16, 28498–28509. doi: 10.3390/ijms161226111
Manavalan, T., Manavalan, A., Thangavelu, K. P., and Heese, K. (2013). Characterization of optimized production, purification and application of laccase from Ganoderma lucidum. Biochem. Eng. J. 70, 106–114. doi: 10.1016/j.bej.2012.10.007
Ou, J. H., and Zhu, L. H. J. (2019). trackViewer: a bioconductor package for interactive and integrative visualization of multi-omics data. Nat. Methods 16, 453–454. doi: 10.1038/s41592-019-0430-y
Palazzolo, M. A., Postemsky, P. D., and Kurina-Sanz, M. (2019). From agro-waste to tool: biotechnological characterization and application of Ganoderma lucidum E47 laccase in dye decolorization. 3. Biotech 9:213. doi: 10.1007/s13205-019-1744-2
Pertea, M., Kim, D., Pertea, G. M., Leek, J. T., and Salzberg, S. L. (2016). Transcript-level expression analysis of RNA-seq experiments with HISAT, StringTie and Ballgown. Nat. Protoc. 11, 1650–1667. doi: 10.1038/nprot.2016.095
Polanco, R., Canessa, P., Rivas, A., Larrondo, L. F., Lobos, S., and Vicuña, R. (2006). Cloning and functional characterization of the gene encoding the transcription factor Acel in the basidiomycete Phanerochaete chrysosporium. Biol. Res. 39, 641–648. doi: 10.4067/S0716-97602006000500007
Qi, Y. C., Liu, C., Sun, X. K., Qiu, L. Y., and Shen, J. W. (2017). The identification of transcriptional regulation related gene of laccase poxc through yeast one-hybrid screening from Pleurotus ostreatus. Fungal Biol. 121, 905–910. doi: 10.1016/j.funbio.2017.06.005
Qin, P., Wu, Y. T., Adil, B., Wang, J., Gu, Y. F., Yu, X. M., et al. (2019). Optimization of laccase from Ganoderma lucidum decolorizing remazol brilliant blue R and Glac1 as main laccase-contributing gene. Molecules 24:3914. doi: 10.3390/molecules24213914
Rodrigues, E. M., Karp, S. G., Malucelli, L. C., Helm, C. V., and Alvarez, T. M. (2019). Evaluation of laccase production by Ganoderma lucidum in submerged and solid-state fermentation using different inducers. J. Basic Microbiol. 59, 784–791. doi: 10.1002/jobm.201900084
Rodríguez-Delgado, M. M., Alemán-Nava, G. S., Rodríguez-Delgado, J. M., Dieck-Assad, G., Martínez-Chapa, S. O., Barceló, D., et al. (2015). Laccase-based biosensors for detection of phenolic compounds. TrAC-Trend. Anal. Chem. 74, 21–45. doi: 10.1016/j.trac.2015.05.008
Sievers, F., Wilm, A., Dineen, D., Gibson, T. J., Karplus, K., Li, W., et al. (2011). Fast, scalable generation of high-quality protein multiple sequence alignments using Clustal omega. Mol. Syst. Biol. 7:539. doi: 10.1038/msb.2011.75
Sitarz, A. K., Mikkelsen, J. D., Højrup, P., and Meyer, A. S. (2013). Identification of a laccase from Ganoderma lucidum CBS 229.93 having potential for enhancing cellulase catalyzed lignocellulose degradation. Enzym. Microb. Technol. 53, 378–385. doi: 10.1016/j.enzmictec.2013.08.003
Song, W. M., and Zhang, B. (2015). Multiscale embedded gene co-expression network analysis. PLoS Comput. Biol. 11:e1004574. doi: 10.1371/journal.pcbi.1004574
Stamatakis, A. (2014). RAxML version 8: a tool for phylogenetic analysis and post-analysis of large phylogenies. Bioinformatics 30, 1312–1313. doi: 10.1093/bioinformatics/btu033
Tangwancharoen, S., Semmens, B. X., and Burton, R. S. (2020). Allele-specific expression and evolution of gene regulation underlying acute heat stress response and local adaptation in the copepod Tigriopus californicus. J. Hered. 111, 539–547. doi: 10.1093/jhered/esaa044
Teng, C., Wu, S. M., and Gong, G. Y. (2019). Bio-removal of phenanthrene, 9-fluorenone and anthracene-9, 10-dione by laccase from Aspergillus Niger in waste cooking oils. Food Control 105, 219–225. doi: 10.1016/j.foodcont.2019.06.015
Tian, L., Khan, A., Ning, Z., Yuan, K., Zhang, C., Lou, H., et al. (2018). Genome-wide comparison of allele-specific gene expression between African and European populations. Hum. Mol. Genet. 27, 1067–1077. doi: 10.1093/hmg/ddy027
Tian, Y. Z., Wang, Z. F., Liu, Y. D., Zhang, G. Z., and Li, G. (2021). The whole-genome sequencing and analysis of a Ganoderma lucidum strain provide insights into the genetic basis of its high triterpene content. Genomics 113, 840–849. doi: 10.1016/j.ygeno.2020.10.015
van Arensbergen, J., Pagie, L., FitzPatrick, V. D., de Haas, M., Baltissen, M. P., Comoglio, F., et al. (2019). High-throughput identification of human SNPs affecting regulatory element activity. Nat. Genet. 51, 1160–1169. doi: 10.1038/s41588-019-0455-2
Virk, A. P., Sharma, P., and Capalash, N. (2012). Use of laccase in pulp and paper industry. Biotechnol. Prog. 28, 21–32. doi: 10.1002/btpr.727
Wang, L. K., Feng, Z. X., Wang, X., Wang, X. W., and Zhang, X. G. (2010). DEGseq: an R package for identifying differentially expressed genes from RNA-seq data. Bioinformatics 26, 136–138. doi: 10.1093/bioinformatics/btp612
Wang, L. N., Gao, W., Wu, X. L., Zhao, M. R., Qu, J. B., Huang, C. Y., et al. (2018). Genome-wide characterization and expression analyses of Pleurotus ostreatus MYB transcription factors during developmental stages and under heat stress based on de novo sequenced genome. Int. J. Mol. Sci. 19:2052. doi: 10.3390/ijms19072052
Wang, L. N., Huang, Q. H., Zhang, L. L., Wang, Q. F., Liang, L., and Liao, B. S. (2020). Genome-wide characterization and comparative analysis of MYB transcription factors in Ganoderma species. G3 -genes Genom. Gen. Dent. 10, 2653–2660. doi: 10.1534/g3.120.401372
Wang, H. L., Li, P., Yang, Y. H., and Liu, Y. F. (2015a). Overproduction of laccase from a newly isolated Ganoderma lucidum using the municipal food waste as main carbon and nitrogen supplement. Bioprocess Biosyst. Eng. 38, 957–966. doi: 10.1007/s00449-014-1341-z
Wang, L. N., Liao, B. S., Gong, L., Xiao, S. M., and Huang, Z. H. (2021). Haploid genome analysis reveals a tandem cluster of four HSP20 genes involved in the high-temperature adaptation of Coriolopsis trogii. Microbiol. Spectr. 9, e00287–e00221. doi: 10.1128/Spectrum.00287-21
Wang, W., Liu, F., Jiang, Y., Wu, G. M., Guo, L. X., Chen, R. L., et al. (2015b). The multigene family of fungal laccases and their expression in the white rot basidiomycete Flammulina velutipes. Gene 563, 142–149. doi: 10.1016/j.gene.2015.03.020
Wang, L. N., Wu, X. L., Gao, W., Zhao, M. R., Zhang, J. X., and Huang, C. Y. (2017). Differential expression patterns of Pleurotus ostreatus catalase genes during developmental stages and under heat stress. Gen. Dent. 8:335. doi: 10.3390/genes8110335
Wu, Y., Li, T., Gong, L., Wang, Y., and Jiang, Y. (2019). Effects of different carbon sources on fumonisin production and FUM gene expression by Fusarium proliferatum. Toxins 11:289. doi: 10.3390/toxins11050289
Yan, L. L., Xu, R. P., Bian, Y. B., Li, H. X., and Zhou, Y. (2019). Expression profile of laccase gene family in white-rot basidiomycete Lentinula edodes under different environmental stresses. Gen. Dent. 10:1045. doi: 10.3390/genes10121045
You, L. F., Liu, Z. M., Lin, J. F., Guo, L. Q., Huang, X. L., and Yang, H. X. (2013). Molecular cloning of a laccase gene from Ganoderma lucidum and heterologous expression in Pichia pastoris. J. Basic Microbiol. 54, S134–S141. doi: 10.1002/jobm.201200808
Yu, G., Wang, L., Han, Y., and He, Q. (2012). clusterProfiler: an R package for comparing biological themes among gene clusters. OMICS 16, 284–287. doi: 10.1089/omi.2011.0118
Yuliana, T., Putri, N. Z., Komara, D. Z., Mardawati, E., Lanti, I., and Rahimah, S. (2020). Study of Ganoderma lucidum in laccase production using corncob and paddies straw substrates on submerged fermentation system. Pak. J. Biol. Sci. 23, 1060–1065. doi: 10.3923/pjbs.2020.1060.1065
Zhang, Y., Wu, Y. Y., Yang, X. L., Yang, E., Xu, H. N., Chen, Y. H., et al. (2021). Alternative splicing of heat shock transcription factor 2 regulates expression of the laccase gene family in response to copper in Trametes trogii. Appl. Environ. Microbiol. 87, e00055–e00021. doi: 10.1128/AEM.00055-21
Zhou, S., Zhang, J. S., Ma, F. Y., Tang, C. H., Tang, Q. J., and Zhang, X. Y. (2018). Investigation of lignocellulolytic enzymes during different growth phases of Ganoderma lucidum strain G0119 using genomic, transcriptomic and secretomic analyses. PLoS One 13:e0198404. doi: 10.1371/journal.pone.0198404
Zhou, S., Zhang, X. Y., Ma, F. Y., Xie, S. X., Tang, C. H., Tang, Q. J., et al. (2021). Integrative analysis of selected metabolites and the fungal transcriptome during the developmental cycle of Ganoderma lucidum strain G0119 correlates lignocellulose degradation with carbohydrate and triterpenoid metabolism. Appl. Environ. Microbiol. 87, e0053321–e0000521. doi: 10.1128/AEM.00533-21
Keywords: G. lucidum, laccase, agricultural and forestry residues, transcriptome profile, allelic expression bias, zinc finger
Citation: Wang L, Ding X, Huang Q, Hu B, Liang L and Wang Q (2022) Gllac7 Is Induced by Agricultural and Forestry Residues and Exhibits Allelic Expression Bias in Ganoderma lucidum. Front. Microbiol. 13:890686. doi: 10.3389/fmicb.2022.890686
Edited by:
Zhongxiong Lai, Fujian Agriculture and Forestry University, ChinaReviewed by:
Jing Li, Fujian Agriculture and Forestry University, ChinaYongxin Tao, Fujian Agriculture and Forestry University, China Zhongxiong Ding, Jiangnan University, China
Copyright © 2022 Wang, Ding, Huang, Hu, Liang and Wang. This is an open-access article distributed under the terms of the Creative Commons Attribution License (CC BY). The use, distribution or reproduction in other forums is permitted, provided the original author(s) and the copyright owner(s) are credited and that the original publication in this journal is cited, in accordance with accepted academic practice. No use, distribution or reproduction is permitted which does not comply with these terms.
*Correspondence: Lining Wang, d2FuZ2xpbmluZzkwQDEyNi5jb20=; Qingfu Wang, a3Vhbmd3dHlAMTYzLmNvbQ==