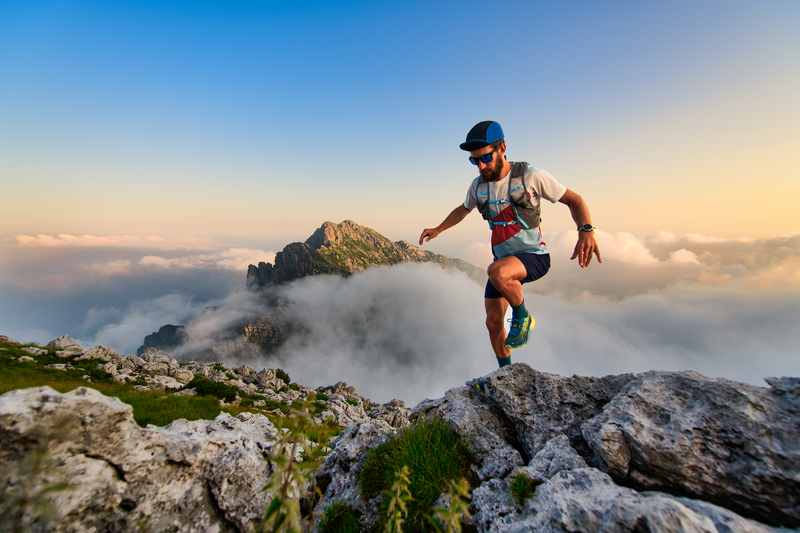
95% of researchers rate our articles as excellent or good
Learn more about the work of our research integrity team to safeguard the quality of each article we publish.
Find out more
ORIGINAL RESEARCH article
Front. Microbiol. , 21 April 2022
Sec. Aquatic Microbiology
Volume 13 - 2022 | https://doi.org/10.3389/fmicb.2022.885585
Fish disease surveillance methods can be complicated and time consuming, which limits their value for timely intervention strategies on aquaculture farms. Novel molecular-based assays using droplet digital Polymerase Chain Reaction (ddPCR) can produce immediate results and enable high sample throughput with the ability to multiplex several targets using different fluorescent dyes. A ddPCR tetraplex assay was developed for priority salmon diseases for farmers in New Zealand including New Zealand Rickettsia-like organism 1 (NZ-RLO1), NZ-RLO2, Tenacibaculum maritimum, and Yersinia ruckeri. The limit of detection in singleplex and tetraplex assays was reached for most targets at 10−9 ng/μl with, respectively, NZ-RLO1 = 0.931 and 0.14 copies/μl, NZ-RLO2 = 0.162 and 0.21 copies/μl, T. maritimum = 0.345 and 0.93 copies/μl, while the limit of detection for Y. ruckeri was 10−8 with 1.0 copies/μl and 0.7 copies/μl. While specificity of primers was demonstrated in previous studies, we detected cross-reactivity of T. maritimum with some strains of Tenacibaculum dicentrarchi and Y. ruckeri with Serratia liquefaciens, respectively. The tetraplex assay was applied as part of a commercial fish disease surveillance program in New Zealand for 1 year to demonstrate the applicability of tetraplex tools for the salmonid aquaculture industry.
Aquaculture production has grown 5-fold over the last three decades with a total of 82.1 million tons of aquatic animals produced in 2018, making it the fastest growing food sector in the world (Boyd and McNevin, 2015; FAO, 2020). Measuring by resource requirements of production, aquaculture can achieve a significantly reduced carbon footprint and is therefore considered a sustainable food source for the growing world population (Hai et al., 2018; Boyd et al., 2020; MacLeod et al., 2020). Atlantic salmon and rainbow trout are ranked first and second for global production volume for farmed marine finfish species, respectively (FAO, 2020), making salmonids one of the most successfully farmed fishes (Iversen et al., 2020). In New Zealand, Chinook or king salmon, Oncorhynchus tshawytscha, is one of the highest valued aquaculture products with a total annual revenue of NZ$ 254 million and growing (Aquaculture New Zealand, 2021a,b).
Aquaculture growth and rapid domestication of aquatic organisms come with an increased risk of diverse diseases triggered through elevated stress levels due to stocking densities and potential suboptimal environmental conditions (e.g., due to sea temperature rise or anomalies; Bateman et al., 2021; Feidantsis et al., 2021). For example, more than 20 potentially pathogenic taxa (viruses, bacteria, and parasites) have been recorded from farmed O. tshawytscha in New Zealand among which NZ Rickettsia-like organisms, Tenacibaculum maritimum, and Yersinia ruckeri (serogroup O1b) are actively managed through preventative measures such as controlled area notices and vaccines (Lane et al., 2020).
Fish disease surveillance usually involves fish, water, or sediment samples sent to commercial laboratories for testing using bacteriology, virology, histology, and molecular assays (Khor et al., 2021). A variety of molecular tools have been developed for disease surveillance from fish samples. For example, fluorescence in situ and other hybridization techniques have been used to screen for proliferative kidney disease (PKX) in salmonids (Morris et al., 2000); DNA microarrays coupled with conventional PCR have been used for herpesvirus and pathogenic Flavobacterium species in fish (Lievens et al., 2011), RNA viruses were targeted with reverse transcriptase PCR in shrimp (Poulos and Lightner, 2006) and many-related technologies such as real-time PCR, loop mediated isothermal amplification (LAMP), random amplified polymorphic DNA (RAPD), restriction/amplified fragment length polymorphism (R/AFLP), and genotyping have been used for pathogen detection in aquaculture (Kim et al., 2017). Overall, efficient, sensitive, and cost-effective methods guarantee competitiveness on the national and international market (Bozzi et al., 2021; Mordecai et al., 2021) but need to overcome complicated and timely protocols leading to inefficient real-life applicability (Law et al., 2014).
Novel molecular-based assays using droplet digital Polymerase Chain Reaction (ddPCR) can produce immediate results and high sample throughput without compromising detection sensitivity (Miotke et al., 2014; Cao et al., 2020). While traditional PCR technologies are currently applied by New Zealand’s Ministry for Primary Industries (MPI) for salmon aquaculture surveillance (Brosnahan, 2020), the advantages of ddPCR are now fully acknowledged and targeted assays are being used for routine monitoring and commercial applications across medicine to biosecurity (Rowlands et al., 2019; Wood et al., 2019; Kiefer et al., 2020; Lewin et al., 2020; Netzer et al., 2021; Orioles et al., 2022). The digital droplet PCR system QX100/QX200 (Bio-Rad, California, United States) is based on partitioning each sample (e.g., extracted DNA from fish tissue) into approximately 20,000 individual droplets, with each small reaction volume containing a single target DNA fragment, which minimizes inhibition (Mazaika and Homsy, 2014; Nathan et al., 2014). Additionally, partitioning into droplets enables absolute quantification of the targeted gene fragments to be conducted through direct measurement of DNA copy numbers, removing the need for replicates and standard curve extrapolation (Hindson et al., 2011). Finally, the possibility to multiplex several target genes into a single ddPCR reaction through two optical channels and adjusting the fluorescence signal of the different targets allows for significant time and cost savings when large datasets are being processed (Hughesman et al., 2016; Lewin et al., 2020).
Endemic pathogens have been identified as immediate and emerging concerns for New Zealand’s salmon aquaculture industry, given its freedom from exotic and notifiable disease agents (Aquaculture New Zealand, 2021a,b). For example, in 2015, up to 70% of salmon summer mortalities in the Marlborough Sounds of New Zealand were associated with bacterial pathogens including New Zealand Rickettsia-like organism (NZ-RLO) and T. maritimum (see Brosnahan et al., 2016). At least two strains of NZ-RLO have been associated with clinically diseased fishes; NZ RLO1, which shares 100% homology with Tasmanian RLO, and NZ-RLO 2 which is suggested to be the more virulent of the two strains (Brosnahan et al., 2019). Tenacibaculum maritimum is a Gram-negative filamentous bacterium that causes ulcerative skin disease, tenacibaculosis, and has been associated with high mortality in marine fishes (Avendaño-Herrera et al., 2006; Chapela et al., 2017). Virulence is likely associated with extreme environmental conditions such as high water temperatures and/or co-infections (Diggles, 2016). Another bacterial pathogen of concern in New Zealand O. tshawytscha farms is an endemic strain of Y. ruckeri (serotype O1b), which causes enteric red-mouth disease. This bacterium originates in freshwater hatcheries but can persist in fish following transfer to marine farms (Tobback et al., 2007; Chapela et al., 2018; Lewin et al., 2020). Collectively, these four bacteria cause disease responsible for multi-billion dollar losses globally (Assefa and Abunna, 2018); therefore, cost-effective diagnostics tools are required to enable early detection and appropriate management responses to outbreaks (Brosnahan, 2020).
The aim of this study was to design and validate a novel ddPCR tetraplex assay for priority salmon diseases for use in commercial applications. The exemplar species used for the assay included New Zealand Rickettsia-like organism 1 (NZ-RLO1), NZ-RLO2, T. maritimum, and Y. ruckeri. Following validation, the tetraplex assay was applied as part of a commercial fish disease surveillance program in New Zealand for 1 year to demonstrate the applicability of tetraplex tools for the salmonid aquaculture industry.
A ddPCR assay was developed for four pathogens that are known to infect farmed O. tshawytscha in New Zealand: New Zealand Rickettsia-like organism 1 (NZ-RLO1), New Zealand Rickettsia-like organism 2 (NZ-RLO2), T. maritimum, and Y. ruckeri serotype O1b. For ddPCR assay validation, synthetic gene fragments (gBlocks™) of each targeted gene region with specific primer and probe binding sites for the four pathogens were designed (see Supplementary Table 1) and purchased from Integrated DNA Technologies (IDT™, Singapore). Specifically, sequences from in-house cultured NZ-RLO1 and NZ-RLO2 were used as well as sequences deposited in GenBank including LC475109.1 T. maritimum CF3 gene for 16S ribosomal RNA, partial sequence, and NR_119063.1 Y. ruckeri strain ATCC 29473 16S ribosomal RNA, partial sequence (See Supplementary Table 1). Diluted gBlocks™ served further as positive controls for consistency of the assays’ performance, together with negative controls that were included in all individual runs.
Further positive testing occurred on extracted DNA from pure bacterial cultures of NZ-RLO1, NZ-RLO2, T. maritimum, and Y. ruckeri. The reference isolate of T. maritimum for this study was isolated in-house from the skin of New Zealand farmed O. tshawytscha exhibiting clinical ulcerative disease. The bacterial isolate was then tested and confirmed by PCR using the primers described by Fringuelli et al. (2012). Reference cultures for Y. ruckeri [W11_2108 #14b (serotype O1b)], NZ-RLO1 (IDC W15_494 10Sp), and NZ-RLO2 (IDC W16_237) were obtained from the Ministry for Primary Industries (MPI). Single colonies of T. maritimum and Y. ruckeri were used for genomic DNA extraction. The frozen culture of NZ-RLO1 and NZ-RLO2 in Epithelioma papulosum cyprini (EPC) derived from a skin tumor of carp cells was centrifuged at 6,000 rpm for 30 min to collect a pellet. The collected pellet was subsequently used for the genomic DNA extraction.
All colonies were then extracted by adding 180 μl Qiagen lysis buffer (ATL) and 20 μl of Proteinase K and incubated at 56°C for a minimum of 3 h and further processed following the AllPrep DNA/RNA mini kit instructions (Qiagen, Hilden, Germany). The quantity and quality of extracted DNA were measured using a NanoPhotometer (Implen, Munich, Germany).
Species-specific primers and TaqMan® probes used in the present study were designed and validated in previous publications (see Table 1). Primer and probe sequences were synthesized at IDT™ (Singapore) and applied in a singleplex ddPCR assay on a QX200 AutoDG Droplet Digital PCR System (Bio-Rad, California, United States). Different genetic regions were targeted for each pathogen; specifically, the internal transcript spacer region (ITS) for NZ-RLO1, the β-subunit of the bacterial RNA polymerase (rpoB) for NZ-RLO2, and the bacterial 16S ribosomal RNA (16S rRNA) gene for T. maritimum and Y. ruckeri (Table 1).
Table 1. Primers and probes used in this study for specific detection of New Zealand Rickettsia-like organism strain 1 (NZ-RLO1), strain 2 (NZ-RLO2), Tenacibaculum maritimum, and Yersinia ruckeri.
The singleplex ddPCR assays were optimized using the gBlocks™ (Supplementary Table 1). The bacterial isolates were amplified under the same reagent concentrations and thermocycling conditions for all four pathogens (slightly differing from the most optimal singleplex conditions, see Supplementary Table 2). Reaction mixtures were performed in 22 μl volumes containing 10 μl ddPCR SuperMix for Probes (No dUTP; Bio-Rad, California, United States), 450 nM of each primer and FAM or HEX labelled probes, and 1 μl of template diluted to 10−7 ng/μl (gBlock™). The thermocycling conditions were initiated at 94°C for 3 min followed by 35 cycles of 94°C for 30 s, 52°C for 30 s, and 72°C for 1 min, with a final extension step at 72°C for 7 min. Droplet generation was carried out according to the manufacturer’s protocol.
QuantaSoft™ Analysis Pro software (version 1.0.596) was used to assign positive and negative droplets and to convert droplet counts to copies/μl. Thresholds were manually set for each run using the amplitude between negative and positive control samples.
The Bio-Rad QX200 ddPCR reader contains two optical fluorescence channels, in this instance for detecting FAM and HEX labeled probes. Multiplexing of more than two targets, individually labeled with either one of the two probes with different dye labels, requires segregating the droplets according to the templates. One strategy is to mix different concentrations of FAM and HEX for the third and fourth target (Hughesman et al., 2016). For example, the assay would have 100% FAM for target 1, 100% HEX for target 2, a mix of 70% FAM and 30% HEX for target 3, and a mix of 70% HEX and 30% FAM for target 4. Using these proportions, positive droplets will align orthogonally in a 2-D amplitude display (Supplementary Figure 1). The second strategy explores a staggered layout in the 2-dimensional display that can be reached by adjusting amplitude fluorescence using different primer and probe concentrations and additionally profiting from the different length of the targeted amplicons (Dobnik et al., 2016), ranging in this study from 79 to 247 bp.
The optimized 22 μl reaction volume for the tetraplex assay therefore consisted of 5 μl ddPCR Multiplex SuperMix for probes (Bio-Rad), 450 nM of all four primer combinations (see Table 1), and varying probe concentrations: 450 nM of the NZ-RLO1 FAM labeled probe, 900 nM of the NZ-RLO2 FAM labeled probe, 220 nM of the T. maritimum HEX labeled probe, and 450 nM of the Y. ruckeri HEX labeled probe. The thermocycling conditions were then adjusted to 95°C for 10 min, 40 cycles of 95°C for 30 s, 54°C for 1 min, and a final enzyme deactivation step at 98°C for 10 min.
To define the limit of detection, the tetraplex ddPCR assay was then evaluated for sensitivity running the assay on 10-fold dilution series of each gBlock™ starting from 10 to 10−9 ng/μl (see Table 2). Additionally, the assay was run individually and for all targets combined on undiluted bacterial isolates that were extracted from cultures but were of insufficient DNA quality for further dilutions and inhibition experiments. Copies/μl between individual and combined targets were compared to detect sensitivity loss between the singleplex and tetraplex assays.
Table 2. Droplet digital PCR results on singleplex and tetraplex assay in copies/μl on a 10-fold dilution series of NZ-RLO1, NZ-RLO2, Tenacibaculum maritimum, and Yersinia ruckeri gBlocks™ starting from 10 ng/μl.
A spiking experiment to check inhibition through fish tissue was performed on gBlocks™. New Zealand farmed O. tshawytscha were sourced from a freshwater salmon farm, where three of the marine pathogens, NZ-RLO1, NZ-RLO2, and T. maritimum have not been detected. Approximately 30 mg of fish skin with muscle was dissected under sterile conditions for DNA extraction (as described in section “gBlock™ Development and Bacterial Isolates”) and tested negative for all four pathogens using the developed tetraplex assay prior to the experiment. Triplicate samples of 30 mg clean O. tshawytscha tissue were spiked with gBlocks™ from NZ-RLO1, NZ-RLO2, T. maritimum, and Y. ruckeri individually and with all four in combination (see Figure 1). Negative tissue controls were included and the ddPCR tetraplex assay was performed as described under section “gBlock™ Development and Bacterial Isolates”. Droplet digital PCR copies/μl were then assessed for each of the samples.
Figure 1. Tetraplex assay sensitivity testing on triplicate samples of DNA from Oncorhynchus tshawytscha tissue spiked with gBlocks™ representing either New Zealand Rickettsia-like organism 1 (NZ-RLO1), NZ-RLO2, Tenacibaculum maritimum, and Yersinia ruckeri, and triplicate samples of gBlocks™ with no and just Oncorhynchus tshawytscha DNA were included for each pathogen individually and all together. The tetraplex assay was then run for the extracted DNA of all samples and copies/μl compared between samples. Schematic created with BioRender.com.
Additionally, the tetraplex assay was run against DNA extracted from pure bacterial isolates relevant to New Zealand marine aquaculture, to ensure no cross-reactivity occurred (see Supplementary Table 3).
The ddPCR tetraplex assay was applied as part of a commercial disease surveillance program for a New Zealand salmon farming company for 12 months. Tests were conducted for approximately 30 fish samples per month (i.e., more than 360 fish in total) from up to 10 different locations including fish of freshwater and marine origin. The salmon company determined which locations were sampled each month. Tissue received for testing (pooled anterior kidney, spleen, and liver) was preserved in DNA/RNA-Shield™ isolation buffer (Zymo Research, United States). Once received by the laboratory, samples were stored at −20°C. For DNA extraction, the pooled tissue of each individual fish was subsampled to 30 mg and placed into 180 ATL buffer and 20 μl of Proteinase K and incubated at 56°C for 3 h and further processed as previously described. DNA was eluted into 100 μl and stored at −20°C until the ddPCR tetraplex assay was run, maximizing sample numbers to increase economic efficiency. The commercial testing included bacteriological plating techniques as in (Kumanan et al., 2020, in prep.) and conventional PCR techniques from MPI for cross-validation of representative samples.
Droplet digital PCR singleplexing and tetraplexing detected all four salmonid pathogens; New Zealand Rickettsia-like organism 1 (NZ-RLO1), New Zealand Rickettsia-like organism 2 (NZ-RLO2), T. maritimum, and Y. ruckeri in a reproducible and quantitative manner. The limit of detection in the singleplex and tetraplex assay was reached for most targets at 10−9 ng/μl with, respectively, NZ-RLO1 = 0.931 and 0.14 copies/μl, NZ-RLO2 = 0.162 and 0.21 copies/μl, T. maritimum = 0.345 and 0.93 copies/μl, while the limit of detection for Y. ruckeri was 10−8 with 1.0 copies/μl and 0.7 copies/μl (Table 2, Figure 2). Optimal droplet separation obtained a strong signal for each pathogen derived from the gBlocks™ dilution series at 10−6 ng/μl in singleplex and at 10−7 ng/μl in tetraplex and was used further for assay optimization and as positive controls (see Table 2).
Figure 2. Droplet digital PCR results on singleplex (left) and tetraplex (right) assays visualized using the QuantaSoft™ Analysis Pro software (version 1.0.596) on a 10-fold gBlocks™ dilution series for NZ-RLO1, NZ-RLO2, Tenacibaculum maritimum, and Yersinia ruckeri, starting from 10 ng/μl. Tetraplex outputs are only displayed for concentration of 10−6 ng/μl. Blue and green dots are positive droplets on the FAM and HEX channel, respectively. Gray dots are counted as negative droplets.
When the assay was tested as single- and tetraplex on bacterial isolates, signals resulted in 7.29 (SE = 1.19) and 7.20 (SE = 1.23) copies/μl for NZ-RLO1, 2.77 (SE = 0.3) and 2.87 (SE = 0.05) copies/μl for NZ-RLO2, 1.46 (SE = 0.29) and 1.43 (SE = 0.11) copies/μl for T. maritimum and 2.87 (SE = 0.37) and 2.79 (SE = 0.21) copies/μl for Y. ruckeri O1b, with no significant differences between the assays (Figure 3).
Figure 3. Droplet digital PCR singleplex and tetraplex assays run for bacterial isolates for NZ-RLO1, NZ-RLO2, Tenacibaculum maritimum, and Yersinia ruckeri in copies/μl. Error bars describe the standard error (SE) of the triplicate runs.
Oncorhynchus tshawytscha DNA that was spiked with the gBlock™ individually (singleplex) did not reveal any inhibitory effects. Mean signals of unspiked and spiked samples resulted in 696 (SE = 32.2) and 636 (SE = 43.5) copies/μl for NZ-RLO1, 676 (SE = 4.5) and 709 (SE = 49.1) copies/μl for NZ-RLO2, 271 (SE = 135.0) and 186 (SE = 21.6) copies/μl for T. maritimum and 112 (SE = 30.6) and 48 (SE = 6.5) copies/μl for Y. ruckeri, respectively. Tetraplexing the four pathogens showed no sign of inhibition between unspiked and spiked samples. Values ranged between 545 (SE = 67.1) and 793 (SE = 96.5) copies/μl for NZ-RLO1, 623 (SE = 30.0) and 646 (SE = 11.4) copies/μl for NZ-RLO2, 217 (SE = 42.7) and 166 (SE = 10.2) copies/μl for T. maritimum and 30 (SE = 1.46) and 31 (SE = 6.59) copies/μl for Y. ruckeri, respectively (Figure 4).
Figure 4. Droplet digital PCR results on singleplex and tetraplex assays run for gBlocks™ of NZ-RLO1, NZ-RLO2, Tenacibaculum maritimum, and Yersinia ruckeri individually and in combination as well as on unspiked clean (red) and spiked (blue) Oncorhynchus tshawytscha samples. Error bars describe the standard error (SE) of the triplicate runs.
Running the ddPCR tetraplex assay on extracted DNA of aquaculture relevant in-house pathogens revealed some cross-reactivity of the assay with Serratia liquefaciens for the Y. ruckeri primer and probe set. Further investigation showed that one base at the 5′-end of the Y. ruckeri forward primer should be A instead of G and was designed on a potentially incorrectly deployed sequence in GenBank (i.e., NR_119063.1 Y. ruckeri strain ATCC 29473 16S ribosomal RNA and partial sequence) and could be corrected to increase specificity. The ddPCR tetraplex assay also cross-reacted between T. maritimum and 3 out of 14 tested T. dicentrarchi strains (copy numbers = 0.13–35, Supplementary Table 3).
All pathogens were detected from commercial samples undergoing routine disease surveillance screening and were confirmed as true positives using alternative techniques including media plating, biochemical tests, PCR, and sequencing (see Supplementary Table 3). Of the 360 fish tested, nine tested positive for NZ-RLO1, five for NZ-RLO2, 146 for T. maritimum, and 5 for Y. ruckeri.
The ddPCR tetraplex assay developed in this study provides a rapid, cost-effective, and reliable screening tool for four primary aquaculture pathogens with commercial relevance and application. Other studies have proven multiple pathogens to be screened in single real-time PCR assays and their implementation as a cost-efficient disease monitoring tool (Chapela et al., 2018; Peters et al., 2018).
For this molecular diagnostic tool to become an accredited test, it needs to undergo appropriate validation (Thalinger et al., 2020). The primers and probes used in this study have been previously published and checked for sensitivity and specificity (see Table 1 and references therein) and were then optimized to work in combination with a ddPCR instrument. While the approach with different probe concentrations (i.e., the assay having 100% FAM for target 1, 100% HEX for target 2, a mix of 70% FAM and 30% HEX for target 3, and a mix of 70% HEX and 30% FAM for target 4) revealed good separation between the target organisms (Supplementary Figure 1), we found an amplitude assay was easier for pipetting purposes and clearer result output especially when dealing with unclean signals (“rain”) from a degraded sample. In vitro testing on reference tissue was achieved by successfully amplifying reference cultures of the pathogens individually and in combination. Additionally, gBlocks™ were spiked into uncontaminated, presumably healthy, fish tissue DNA to check for any inhibitory effects. No inhibition was detected. This test was applied commercially for salmon farm surveillance for 12 months in conjunction with additional validation via bacterial culture techniques and standard and qPCR confirmatory analysis by MPI. Successful and true positive detections were achieved using our tetraplex assay on field samples that also aligned with other studies, e.g., T. maritimum detections in Bateman et al. (2021).
Diagnostic tools can never achieve 100% sensitivity and specificity (Assefa and Abunna, 2018). For example, cultivation-based methods will only detect bacteria that are able to replicate under the provided conditions and most aquatic microorganisms (>99%) are unable to be cultivated using standard methods (Netzer et al., 2021). NZ-RLO1 and NZ-RLO2 are fastidious, and good-quality DNA extracts from cultures could not be achieved for molecular purposes; thus, artificially constructed DNA oligos (gBlocks™) were used for assay optimization. By combining these screening technologies, cross-validating outcomes and interpreting them in the context of their designed application, developed tools need to be continuously optimized.
During this study, three important observations gave us novel insights for New Zealand aquaculture surveillance. First, the T. maritimum specific primers and probe used in this study revealed low positive signal for a non-typical T. maritimum-like bacterial colony on agar plates, which was then confirmed as T. dicentrarchi through 16S rRNA gene sequencing. Fringuelli et al. (2012) did not include this strain for specificity testing as it is simply not possible to validate primers on all existing bacterial strains (Collins et al., 2006). We tested the assay on several T. dicentrarchi strains and received three positive signals out of 19 tested strains. Further strain confirmation by sequencing and intraspecific variability are needed to confirm cross-reactivity or wrongly identified strains/cross-contamination with T. maritimum.
Our second observation of cross-reactivity of Y. ruckeri with closely related Serratia species (both Yersiniaceae) was also not included as part of the specificity testing by Carson and Wilson (2009) and Ghosh et al. (2016). We discovered through a standard GenBank BLAST that the Y. ruckeri forward primer might have been developed on an incorrectly deposited reference sequence (NR_119063.1) that included a single base-pair mistake at the 5′-end of the sequence, dating back to 1993 when sequencing technology was in its beginnings and prone to erroneous nucleotide outputs. This theory is supported by the fact that none of the recent deposited sequences for Y. ruckeri identified that particular nucleotide which is located at the 3′-end of our forward primer. DNA polymerase requires the 3′ base of a primer to form appropriate hydrogen bonds to initiate polymerization and might experience loss in sensitivity through nucleotide ambiguities (van Pelt-Verkuil et al., 2008).
Finally, a high number of positive detections of NZ-RLO 1 following inactivated (DNA) vaccination on the fish farm revealed that testing DNA with the ddPCR specific assays would not be able to differentiate between a vaccinated fish or a true infection, reported previously (Laurin et al., 2020). Investigating RNA or other viability tests such as PEMAX that focus on live pathogens could help circumvent this problem at least for inactivated vaccines (Brosnahan et al., 2020).
Optimizing the tetraplex assay further will involve designing more specific primers to avoid cross-reactions between strains and correcting other primer issues, including the design of new primers for emerging agents, such as T. dicentrarchi and Serratia strains. Novel ddPCR technologies such as the QX600 AutoDG Flex from Bio-Rad are already on the market that work on four optical channels for multiplexing up to eight samples which could be used to test eight pathogens simultaneously. Overall, there is substantial interest in adopting approaches that allow for point-of-need and in-field surveillance (Peters et al., 2018). Future technologies such as the portable Oxford Nanopore Sequencing or the NS2 Nucleic Sensing System1 which allows for in-field (water) multi-probe ddPCR analyses are promising for these purposes.
The baseline ddPCR tetraplex assay presented here can be repurposed and adjusted to advanced technologies, including new pathogens of interest or analytical methods, which will foster scientific research output and expand the molecular surveillance toolbox. For example, applying occupancy and co-occurrence modeling approaches on the detection signals of different pathogens enables detection probabilities even if all samples return negative (Willoughby et al., 2016; Laurin et al., 2020) and provides a cost-efficient tool for aquaculture surveillance (Farrell et al., 2021).
The original contributions presented in the study are included in the article/Supplementary Material, further inquiries can be directed to the corresponding author/s.
All fish sampling was done with approval from the Nelson Marlborough Institute of Technology Animal Ethics Committee (AEC 2018 CAW01). Written informed consent was obtained from the owners for the participation of their animals in this study.
UA, JS, and XP designed the research. UA, TA, and KK performed the research. UA, CB, JS, and KH contributed the reagents and analytical tools. UA analyzed the data. UA, JS, KH, and XP wrote the paper. All authors contributed to the article and approved the submitted version.
This work was funded by the New Zealand Ministry of Business, Innovation and Employment, under the program: Aquaculture Health to Maximise Productivity and Security (CAWX1707).
The authors declare that the research was conducted in the absence of any commercial or financial relationships that could be construed as a potential conflict of interest.
All claims expressed in this article are solely those of the authors and do not necessarily represent those of their affiliated organizations, or those of the publisher, the editors and the reviewers. Any product that may be evaluated in this article, or claim that may be made by its manufacturer, is not guaranteed or endorsed by the publisher.
We thank Oliver Quinn of MPI for reference cultures; High Country Salmon Farms for fish supply; Sol Green from Bio-Rad for advice on the droplet digital PCR; and Seumas Walker, Mark Englefield, Ryan Hunter, Catherine Moisan, Jonathan Banks, and Andrew Fidler of the Cawthron Institute for provision of materials and management of commercial testing services and Jeremy Carson, from Carson BioConsulting for his support during the commercial surveillance. Finally, Zac Waddington from New Zealand King Salmon for reviewing the manuscript from an industry perspective.
The Supplementary Material for this article can be found online at: https://www.frontiersin.org/articles/10.3389/fmicb.2022.885585/full#supplementary-material
Aquaculture New Zealand (2021a). Aquaculture for New Zealand – a sector overview with key facts and statistics for 2020. (Accessed September 07, 2021).
Aquaculture New Zealand (2021b). “Salmon Biosecurity Standards May 2019.” New Zealand Sustainable Aquaculture.
Assefa, A., and Abunna, F. (2018). Maintenance of fish health in aquaculture: review of epidemiological approaches for prevention and control of infectious disease of fish. Vet. Med. Int. 2018:5432497. doi: 10.1155/2018/5432497
Avendaño-Herrera, R., Toranzo, A. E., and Magariños, B. (2006). Tenacibaculosis infection in marine fish caused by Tenacibaculum maritimum: a review. Dis. Aquat. Org. 71, 255–266. doi: 10.3354/dao071255
Bateman, A. W., Schulze, A. D., Kaukinen, K. H., Tabata, A., Mordecai, G., Flynn, K., et al. (2021). Descriptive multi-agent epidemiology via molecular screening on Atlantic salmon farms in the Northeast Pacific Ocean. Sci. Rep. 11:3466. doi: 10.1038/s41598-020-78978-9
Boyd, C. E., D’Abramo, L. R., Glencross, B. D., Huyben, D. C., Juarez, L. M., Lockwood, G. S., et al. (2020). Achieving sustainable aquaculture: historical and current perspectives and future needs and challenges. J. World Aquacult. Soc. 51, 578–633. doi: 10.1111/jwas.12714
Bozzi, D., Rasmussen, J. A., Carøe, C., Sveier, H., Nordøy, K., Gilbert, M. T. P., et al. (2021). Salmon gut microbiota correlates with disease infection status: potential for monitoring health in farmed animals. Anim. Microbiome 3, 1–17. doi: 10.1186/s42523-021-00096-2
Brosnahan, C. L. (2020). Diagnostic investigation into summer mortality events of farmed chinook salmon (Oncorhynchus tshawytscha) in New Zealand. Doctor of Philosophy, Massey University.
Brosnahan, C., Georgiades, E., McDonald, C., and Jones, B. (2020). Viability PCR for aquatic animal pathogens Biosecurity New Zealand Technical Paper No: 2020/01 Prepared for Operational Research Science and Risk Directorate. B. N. Zealand.
Brosnahan, C. L., Ha, H. J., Booth, K., McFadden, A. M. J., and Jones, J. B. (2016). First report of a rickettsia-like organism from farmed Chinook salmon, Oncorhynchus tshawytscha (Walbaum), in New Zealand. N. Z. J. Mar. Freshw. Res. 51, 356–369. doi: 10.1080/00288330.2016.1242081
Brosnahan, C. L., Munday, J. S., Ha, H. J., Preece, M., and Jones, J. B. (2019). New Zealand rickettsia-like organism (NZ-RLO) and Tenacibaculum maritimum: distribution and phylogeny in farmed Chinook salmon (Oncorhynchus tshawytscha). J. Fish Dis. 42, 85–95. doi: 10.1111/jfd.12909
Cao, Y., Yu, M., Dong, G., Chen, B., and Zhang, B. (2020). Digital PCR as an emerging tool for monitoring of microbial biodegradation. Molecules 25:706. doi: 10.3390/molecules25030706
Chapela, M.-J., Ferreira, M., Ruiz-Cruz, A., Martin-Varela, I., Fernández-Casal, J., and Garrido-Maestu, A. (2017). Application of real-time PCR for early diagnosis of diseases caused by Aeromonas salmonicida, Vibrio anguillarum, and Tenacibaculum maritimum in turbot: a field study. J. Appl. Aquac. 30, 76–89. doi: 10.1080/10454438.2017.1406419
Chapela, M. J., Ferreira, M., Varela, C., Arregui, L., and Garrido-Maestu, A. (2018). Development of a multiplex real-time PCR method for early diagnosis of three bacterial diseases in fish: a real-case study in trout aquaculture. Aquaculture 496, 255–261. doi: 10.1016/j.aquaculture.2018.07.003
Collins, G., Kavanagh, S., McHugh, S., Connaughton, S., Kearney, A., Rice, O., et al. (2006). Accessing the black box of microbial diversity and ecophysiology: recent advances through polyphasic experiments. J. Environ. Sci. Health A Tox. Hazard. Subst. Environ. Eng. 41, 897–922. doi: 10.1080/10934520600614546
Diggles, B. (2016). Updated disease risk assessment report relocation of salmon farms in Marlborough sounds. DigsFish services report: DF 16-01 7. D. Services.
Dobnik, D., Stebih, D., Blejec, A., Morisset, D., and Zel, J. (2016). Multiplex quantification of four DNA targets in one reaction with bio-rad droplet digital PCR system for GMO detection. Sci. Rep. 6:35451. doi: 10.1038/srep35451
Farrell, J. A., Whitmore, L., and Duffy, D. J. (2021). The promise and pitfalls of environmental DNA and RNA approaches for the monitoring of human and animal pathogens from aquatic sources. Bioscience 71, 609–625. doi: 10.1093/biosci/biab027
Feidantsis, K., Portner, H. O., Giantsis, I. A., and Michaelidis, B. (2021). Advances in understanding the impacts of global warming on marine fishes farmed offshore: Sparus aurata as a case study. J. Fish Biol. 98, 1509–1523. doi: 10.1111/jfb.14611
Fringuelli, E., Savage, P. D., Gordon, A., Baxter, E. J., Rodger, H. D., and Graham, D. A. (2012). Development of a quantitative real-time PCR for the detection of Tenacibaculum maritimum and its application to field samples. J. Fish Dis. 35, 579–590. doi: 10.1111/j.1365-2761.2012.01377.x
Ghosh, B., Nguyen, T. D., Crosbie, P. B. B., Nowak, B. F., and Bridle, A. R. (2016). Oral vaccination of first-feeding Atlantic salmon, Salmo salar L., confers greater protection against yersiniosis than immersion vaccination. Vaccine 34, 599–608. doi: 10.1016/j.vaccine.2015.12.044
Gias, E., Brosnahan, C. L., Orr, D., Binney, B., Ha, H. J., Preece, M. A., et al. (2018). In vivo growth and genomic characterization of rickettsia-like organisms isolated from farmed Chinook salmon (Oncorhynchus tshawytscha) in New Zealand. J. Fish Dis. 41, 1235–1245. doi: 10.1111/jfd.12817
Hindson, B. J., Ness, K. D., Masquelier, D. A., Belgrader, P., Heredia, N. J., Makarewicz, A. J., et al. (2011). High-throughput droplet digital PCR system for absolute quantitation of DNA copy number. Anal. Chem. 83, 8604–8610. doi: 10.1021/ac202028g
Hughesman, C. B., Lu, X. J., Liu, K. Y., Zhu, Y., Poh, C. F., and Haynes, C. (2016). A robust protocol for using multiplexed droplet digital PCR to quantify somatic copy number alterations in clinical tissue specimens. PLoS One 11:e0161274. doi: 10.1371/journal.pone.0161274
Iversen, A., Asche, F., Hermansen, Ø., and Nystøyl, R. (2020). Production cost and competitiveness in major salmon farming countries 2003–2018. Aquaculture 522:735089. doi: 10.1016/j.aquaculture.2020.735089
Khor, L., Delamare-Deboutteville, J., and Chadag, V. (2021). Sampling materials for fish disease diagnostics.
Kiefer, A., Tang, P., Arndt, S., Fallico, V., and Wong, C. (2020). Optimization of viability treatment essential for accurate droplet digital PCR enumeration of probiotics. Front. Microbiol. 11:1811. doi: 10.3389/fmicb.2020.01811
Kim, A., Thanh, L. N., and Do-Hyung, K. (2017). Modern methods of diagnosis. Diagnosis and control of diseases of fish and shellfish: 109–147.
Lane, H. S., Brosnahan, C. L., and Poulin, R. (2020). Aquatic disease in New Zealand: synthesis and future directions. N. Z. J. Mar. Freshw. Res. 56, 1–42. doi: 10.1080/00288330.2020.1848887
Laurin, E., Gardner, I. A., Pena, A., Rozas-Serri, M., Gayosa, J., Neumann Heise, J., et al. (2020). Bayesian estimation of diagnostic sensitivity and specificity of a qPCR and a bacteriological culture method for Piscirickettsia salmonis in farmed Atlantic salmon (Salmo salar L.) in Chile. J. Fish Dis. 43, 1167–1175. doi: 10.1111/jfd.13226
Law, J. W., Ab Mutalib, N. S., Chan, K. G., and Lee, L. H. (2014). Rapid methods for the detection of foodborne bacterial pathogens: principles, applications, advantages and limitations. Front. Microbiol. 5:770. doi: 10.3389/fmicb.2014.00770
Lewin, A. S., Haugen, T., Netzer, R., Tondervik, A., Dahle, S. W., and Hageskal, G. (2020). Multiplex droplet digital PCR assay for detection of Flavobacterium psychrophilum and Yersinia ruckeri in Norwegian aquaculture. J. Microbiol. Methods 177:106044. doi: 10.1016/j.mimet.2020.106044
Lievens, B., Frans, I., Heusdens, C., Juste, A., Jonstrup, S. P., Lieffrig, F., et al. (2011). Rapid detection and identification of viral and bacterial fish pathogens using a DNA array-based multiplex assay. J. Fish Dis. 34, 861–875. doi: 10.1111/j.1365-2761.2011.01304.x
MacLeod, M. J., Hasan, M. R., Robb, D. H. F., and Mamun-Ur-Rashid, M. (2020). Quantifying greenhouse gas emissions from global aquaculture. Sci. Rep. 10:11679. doi: 10.1038/s41598-020-68231-8
Mazaika, E., and Homsy, J. (2014). Digital droplet PCR: CNV analysis and other applications. Curr. Protoc. Hum. Genet. 82, 7.24.1–7.24.13. doi: 10.1002/0471142905.hg0724s82
Miotke, L., Lau, B. T., Rumma, R. T., and Ji, H. P. (2014). High sensitivity detection and quantitation of DNA copy number and single nucleotide variants with single color droplet digital PCR. Anal. Chem. 86, 2618–2624. doi: 10.1021/ac403843j
Mordecai, G. J., Di Cicco, E., Gunther, O. P., Schulze, A. D., Kaukinen, K. H., Li, S., et al. (2021). Discovery and surveillance of viruses from salmon in British Columbia using viral immune-response biomarkers, metatranscriptomics, and high-throughput RT-PCR. Virus Evol. 7:veaa069. doi: 10.1093/ve/veaa069
Morris, D. J., Adams, A., and Richards, R. H. (2000). In situ hybridisation identifies the gill as a portal of entry for PKX (Phylum Myxozoa), the causative agent of proliferative kidney disease in salmonids. Parasitol. Res. 86, 950–956. doi: 10.1007/PL00008525
Nathan, L. M., Simmons, M., Wegleitner, B. J., Jerde, C. L., and Mahon, A. R. (2014). Quantifying environmental DNA signals for aquatic invasive species across multiple detection platforms. Environ. Sci. Technol. 48, 12800–12806. doi: 10.1021/es5034052
Netzer, R., Ribicic, D., Aas, M., Cave, L., and Dhawan, T. (2021). Absolute quantification of priority bacteria in aquaculture using digital PCR. J. Microbiol. Methods 183:106171. doi: 10.1016/j.mimet.2021.106171
Orioles, M., Bulfoni, M., Saccà, E., Cesselli, D., Schmidt, J. G., and Galeotti, M. (2022). Development and application of a sensitive droplet digital PCR for the detection of red mark syndrome infection in rainbow trout (Oncorhynchus mykiss). Aquaculture 551:737910. doi: 10.1016/j.aquaculture.2022.737910
Peters, L., Spatharis, S., Dario, M. A., Dwyer, T., Roca, I. J. T., Kintner, A., et al. (2018). Environmental DNA: A new low-cost monitoring tool for pathogens in salmonid aquaculture. Front. Microbiol. 9:3009. doi: 10.3389/fmicb.2018.03009
Poulos, B. T., and Lightner, D. V. (2006). Detection of infectious myonecrosis virus (IMNV) of penaeid shrimp by reverse-transcriptase polymerase chain reaction (RT-PCR). Dis. Aquat. Org. 73, 69–72. doi: 10.3354/dao073069
Rowlands, V., Rutkowski, A. J., Meuser, E., Carr, T. H., Harrington, E. A., and Barrett, J. C. (2019). Optimisation of robust singleplex and multiplex droplet digital PCR assays for high confidence mutation detection in circulating tumour DNA. Sci. Rep. 9:12620. doi: 10.1038/s41598-019-49043-x
Thalinger, B., Deiner, K., Harper, L. R., Rees, H. C., Blackman, R. C., Sint, D., et al. (2020). A validation scale to determine the readiness of environmental DNA assays for routine species monitoring. Environmental DNA.
Tobback, E., Decostere, A., Hermans, K., Haesebrouck, F., and Chiers, K. (2007). Yersinia ruckeri infections in salmonid fish. J. Fish Dis. 30, 257–268. doi: 10.1111/j.1365-2761.2007.00816.x
van Pelt-Verkuil, E., Van Belkum, A., and Hays, J. P. (2008). Principles and Technical Aspects of PCR Amplification. The Netherlands: Springer Science & Business Media.
Willoughby, J. R., Wijayawardena, B. K., Sundaram, M., Swihart, R. K., and DeWoody, J. A. (2016). The importance of including imperfect detection models in eDNA experimental design. Mol. Ecol. Resour. 16, 837–844. doi: 10.1111/1755-0998.12531
Wood, S. A., Pochon, X., Laroche, O., von Ammon, U., Adamson, J., and Zaiko, A. (2019). A comparison of droplet digital polymerase chain reaction (PCR), quantitative PCR and metabarcoding for species-specific detection in environmental DNA. Mol. Ecol. Resour. 19, 1407–1419. doi: 10.1111/1755-0998.13055
Keywords: droplet digital PCR, multiplex assay, Oncorhynchus tshawytscha, Chinook salmon, aquatic animal health, fish disease
Citation: von Ammon U, Averink T, Kumanan K, Brosnahan CL, Pochon X, Hutson KS and Symonds JE (2022) An Efficient Tetraplex Surveillance Tool for Salmonid Pathogens. Front. Microbiol. 13:885585. doi: 10.3389/fmicb.2022.885585
Received: 28 February 2022; Accepted: 01 April 2022;
Published: 21 April 2022.
Edited by:
Jin Zhou, Tsinghua University, ChinaReviewed by:
Spencer Russell, Vancouver Island University, CanadaCopyright © 2022 von Ammon, Averink, Kumanan, Brosnahan, Pochon, Hutson and Symonds. This is an open-access article distributed under the terms of the Creative Commons Attribution License (CC BY). The use, distribution or reproduction in other forums is permitted, provided the original author(s) and the copyright owner(s) are credited and that the original publication in this journal is cited, in accordance with accepted academic practice. No use, distribution or reproduction is permitted which does not comply with these terms.
*Correspondence: Ulla von Ammon, dWxsYS52b25hbW1vbkBjYXd0aHJvbi5vcmcubno=
Disclaimer: All claims expressed in this article are solely those of the authors and do not necessarily represent those of their affiliated organizations, or those of the publisher, the editors and the reviewers. Any product that may be evaluated in this article or claim that may be made by its manufacturer is not guaranteed or endorsed by the publisher.
Research integrity at Frontiers
Learn more about the work of our research integrity team to safeguard the quality of each article we publish.