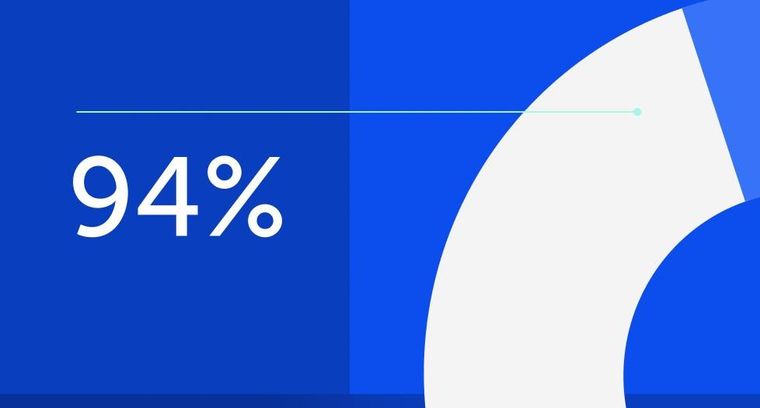
94% of researchers rate our articles as excellent or good
Learn more about the work of our research integrity team to safeguard the quality of each article we publish.
Find out more
ORIGINAL RESEARCH article
Front. Microbiol., 10 June 2022
Sec. Food Microbiology
Volume 13 - 2022 | https://doi.org/10.3389/fmicb.2022.885502
This article is part of the Research TopicMulti-species Biofilms in the Food IndustryView all 5 articles
Various pathogenic and spoilage bacteria frequently coexist in meat processing environments and can form multispecies biofilms, causing significant health and economic issues. Despite the prevalence and coexistence, only less is known about possible interactions between Listeria monocytogenes (LM) and spoilers like Pseudomonas species, and their community-wide resistance against natural preservatives. This study evaluates the interactions between mono- or dual-species biofilms formed by LM and Pseudomonas lundensis (PL), as well as the sensitivity of these bacteria in dual-species biofilms to ε-polylysine hydrochloride (ε-PLH) alone or combined with cinnamon essential oil (CEO). The results showed that the biofilm cell density of P. lundensis in dual species was higher (p < 0.05) than LM, constituting about 85% of the total population. More biofilms and exopolysaccharide both in mono- or dual species of the two psychrotrophic strains were greatly produced at 15°C than at 30°C. The biomass, biovolume, and thickness of dual-species biofilms were significantly lower than single PL biofilm when tested using crystal violet staining, confocal laser scanning microscopy, and scanning electron microscopy, indicating the competitive interactions between them prevail. Additionally, ε-PLH significantly reduced the biofilm development as mono- and dual species in a concentration-dependent manner, especially single LM biofilm, which was consistent with the decrease in autoinducer-2 (AI-2) activity. LM as dual-species biofilms exhibited lower sensitivity to ε-PLH than its mono-biofilm probably due to protective effect conferred by PL. ε-PLH in combination with CEO, at the maximum sublethal concentrations (MSCs), showed enhanced inhibitory activity against dual-species biofilm formation, as evidenced by thin spare spatial structures and reduced AI-2 activity. In addition, the preformed dual biofilms were dramatically eradicated following treatment with ε-PLH combined with CEO at higher than minimum inhibitory concentration in comparison with either of the compounds used alone, indicating the synergistic antibiofilm of the two preservatives. This study reveals the competitive interactions between the two strains in dual-species biofilms, in which the dominant PL significantly contributed toward the tolerance of LM to ε-PLH, and the use of combined preservatives shows it is an effective strategy to control the multispecies biofilms in meat processing.
- LM and PL exhibited a competitive interaction in dual-species biofilms.
- Dominant PL in dual-species biofilms enhanced the resistance of LM to ε-PLH.
- ε-PLH combining with cinnamon EO had synergistic antibiofilm of the dual-species.
- Inhibition of two natural preservatives was correlated with repression of AI-2.
- Combination ε-PLH with cinnamon EO could be a promising approach against multi-biofilms.
A biofilm consists of microbial cells embedded in a matrix of self-produced extracellular polymeric substances (EPS) on biotic or abiotic surfaces. A serious problem in the food industry is the formation of microbial biofilms that can harbor and transmit pathogenic and spoilage bacteria. Approximately more than 60% of foodborne outbreaks are linked to the presence of biofilms in food processing environments (Piercey et al., 2016). Listeria monocytogenes (LM) is a psychrotrophic, Gram-positive, and facultative anaerobic bacterial pathogen that is responsible for listeriosis in humans. Presence of LM on food products may be attributed to its survival and proliferation at low pH, high salinity, and chilling temperatures. Additionally, the ability of LM to form biofilms on food contact surfaces has been demonstrated in previous studies (Gandhi and Chikindas, 2007; Rodriguez-Lopez et al., 2018; Liu et al., 2020). Sessile cells are less susceptible to disinfectants than planktonic cells, which makes it hard to eliminate them and allows them to manage to survive in food processing or storage environments for prolonged periods (Giaouris et al., 2013; Pang et al., 2019). However, the capability of LM to form mono-species biofilm does not seem to be a key factor determining their persistence in food processing (Fagerlund et al., 2021).
The resident microbiota in food processing plants can affect the growth of LM. Psychrotrophic Pseudomonas spp. are the dominant bacteria that cause spoilage in aerobically chilled meat (Stanborough et al., 2018; Wickramasinghe et al., 2019). Pseudomonas lundensis (PL), which is considered one of the most prevalent meat-spoilage bacteria, produces fluorescent and pyocyanin pigments, off-odors, and slime (Wickramasinghe et al., 2019; Fang et al., 2022). Moreover, PL has high capacity to produce biofilms, and a great amount of biofilm was formed at low temperatures (Liu et al., 2015). In a cold chain, the residential psychrotrophs, such as LM and Pseudomonas, attach to the surfaces of fresh meat products and spread through growth, ultimately resulting in the increase of cross-contamination, which leads to shortened shelf-life, and foodborne illnesses (Tenderis et al., 2021).
In food processing environments, biofilms are generally composed of dynamic microbiota, which we refer to as multispecies biofilms. During the formation of multispecies biofilms, the complex interactions within the community significantly affect the structure and biological function of biofilms (Giaouris et al., 2013). More recent biofilm studies have examined LM in co-culture with other food-related commensal bacteria or foodborne pathogens in mixed-species biofilms. Norwood and Gilmour (2000) demonstrated higher LM numbers in mono-cultures compared with the multispecies biofilms formed after its association with Staphylococcus xylosus and Pseudomonas fragi. Pseudomonas fluorescens may enhance the tolerance of LM to antimicrobial in dual-species biofilms by bacterial suspension co-culture (Puga et al., 2016). Thus, once the spoilage and pathogenic bacteria in the food environment form complex multispecies biofilms, they showed enhanced survival and tolerance to chemical disinfectants and UV radiation due to the production of EPS and microbial interactions in co-culture (Bridier et al., 2015; Wang et al., 2020).
Recently, effective natural antimicrobial compounds have received interest as an alternative strategy to inhibit the biofilm formation compared with some chemical sanitizers. ε-Poly-lysine hydrochloride (ε-PLH) as a homopolymer of ε-poly-lysine has been found to exhibit good antimicrobial activity with low price and higher stability than ε-poly-lysine (Ding et al., 2019; Li et al., 2021). At present, the effective antimicrobial properties of ε-poly-lysine against wide microorganisms are well documented (Hyldgaard et al., 2014); however, there are few studies on the inhibitory activity of ε-PLH and ε-poly-lysine on the planktonic and biofilm cells of foodborne pathogens. In addition, cinnamon essential oil (CEO) has a wide variety of secondary metabolites that exhibits antibacterial properties. Its main active components include cinnamaldehyde, cinnamate, and cinnamic acid, demonstrating a high performance to control biofilm formation of various pathogenic bacteria and spoilage-related bacteria (Vasconcelos et al., 2018; Liu et al., 2020). The combination of essential oils with other antimicrobial agents to inhibit biofilm-forming bacteria is now a hot research topic, to enhance efficacy, decrease adverse sensory effects, and reduce the required dose, such as binary EOs (Vázquez-Sánchez et al., 2018), combining with nisin (Abdollahzadeh et al., 2014), and ε-PLH (Li et al., 2021).
The objective of this study was to investigate the dual-species biofilms of foodborne pathogen LM and spoilage bacterium PL, to explore the interactions between the two strains under co-cultivation conditions, and their sensitivity to ε-PLH. Furthermore, the synergistic effects of ε-PLH in combination with CEO on the formation and eradication of dual-species biofilms were further evaluated. The results not only shed new light on the significance of properties and interactions in multispecies biofilms from meat products, but also uncovered the impacts of combining natural antimicrobial compounds on multispecies biofilms.
Listeria monocytogenes ATCC19112 used in this study was purchased from the China Microbial Preservation Center (Beijing, China). The strain of PL 28 was previously isolated from chilled beef in our laboratory (Fang et al., 2022). These strains stored at −80°C were revitalized on tryptic soy broth (TSB, Bio-Tech, Qingdao, China) at 30°C with consecutive transfers after 24 h shaking incubation (180 rpm).
Briefly, overnight activated cultures of the two strains were diluted at a ratio of 1:1,000, with 5.0 log cfu/ml as the final cell concentration for each species. The diluted LM and PL were inoculated separately or mixed in tube or polystyrene plates with TSB, and the inoculated samples were cultivated statically for 24 and 48 h at 30 and 15°C. For enumeration of planktonic cells, bacterial suspension as mono- or dual species from each tube was serially diluted with sterile peptone water (0.85% NaCl and 0.1% peptone), and then aliquots of 0.1 ml were spread on Pseudomonas CFC Selective Agar (Bio-Tech, Qingdao, China) for PL and PALCAM Agar (Bio-Tech, Qingdao, China) for LM. For enumeration of the biofilm cells, mono- and dual-species biofilm cells were cultivated on the surface of stainless steel coupons (SS, AISI304, 1 cm × 1 cm × 0.2 cm) in six-well polystyrene plates (one coupon in each well) (Wang et al., 2020). The coupons were soaked in 70% ethanol for 5 min and autoclaved at 121°C for 15 min prior to the experiment. Wells containing TSB alone were considered as control. After incubation at 30 and 15°C, SS coupons were taken out and washed three times with phosphate buffered saline (PBS, pH 7.0) to release loosely attached cells. Subsequently, the biofilm cells on the SS surfaces were obtained by vigorously vortexing in 0.1% peptone water for 5 min. The homogenized suspension was then diluted and enumerated on the above two selective agar plates, and the total number of colonies was counted after incubation at 30°C for 24 h.
The activated LM and PL as mono- or dual-species biofilms were quantified using crystal violet (CV) assay. Briefly, the above diluted cultures were inoculated in 96-well polystyrene microtiter plates as mono- or co-culture, and uninoculated TSB was used as negative control. Following incubation, the biofilm in each well of microtiter plates was carefully washed thrice with sterile PBS to remove unattached cells. Biofilm cells on the bottom and side of each well were stained with 0.2% (w/v) CV for 15 min. After a second washing step, biofilm cell-associated CV in each well was resolubilized with 95.0% ethanol (v/v) for 5 min. The optical absorbance of the samples was measured at 590 nm using a microplate reader (Infinite 200, Tecan, Switzerland).
Extracellular polysaccharides in biofilm were extracted according to Wang et al. (2020). The above diluted LM and PL containing about 5 log cfu/ml of the two strains as mono- or dual species were transferred into six-well polystyrene microtiter plate and incubated at 30 and 15°C for 24 or 48 h. After the cultures in each well were carefully aspirated, the biofilm cells were washed with sterile PBS, redissolved with PBS, and sonicated at 50 kHz for 5 min. Bacterial pellets were then removed by centrifugation (8,000 × g for 30 min) and filtering by a 0.22 μm membrane, and the extracting supernatant was added with 5% phenol and sulfuric acid and bathed at 100°C for 10 min. The absorbance value was determined at 490 nm to quantitatively measure exopolysaccharides using glucose as the standard.
The biofilms formed by PL and LM as mono- or dual species were further observed by optical microscopy (OM), confocal laser scanning microscopy (CLSM), and scanning electron microscopy (SEM). After incubation for 24 h at 30°C, the glass slips (8 mm × 8 mm) in six-well polystyrene microtiter plates were taken out and rinsed with PBS three times to remove the planktonic bacteria. The biofilm cells on the surface were then stained with 0.2% (w/v) CV and directly observed using an OM (Olympus CX21, Tokyo, Japan). Meanwhile, the biofilm structures of mono- or dual species were examined using CLSM with objective lens (Carl Zeiss LSM710, Jena, Germany) after stained with 0.3% SYTO-9 and 0.3% PI (Sigma-Aldrich, Shanghai, China) in the dark at 30°C for 15 min. The maximum excitation/emission used for these stains was approximately 505/525 for SYTO-9 (green channel) and 493/635 for PI (red channel). Representative CLSM images from each coupon were acquired by scanning z-stacks at a scanning step size of 1 μm and were processed in IMARIS 7.6 software (Bitplane AG, Zurich, Switzerland). Three representative areas were selected by scanning the total surface area with a 20× objective. The Zeiss confocal software was used to analyze the biofilm images, allowing for collection of z-stacks. Quantitative structural parameters of the biofilms, including bacterial biovolume and thickness, were calculated using PHLIP (Almeida et al., 2003).
In addition, the biofilms formed by PL and LM as mono- or dual species were further observed by SEM. After the removal of culture media and washing, the glass slips incubated for 24 h at 30°C were collected and fixed with ice-cold 2.5% glutaraldehyde overnight at 4°C. After washing, biofilm cells on glass slip were post-fixed with 1% osmium tetroxide for 1 h. The samples were washed in PBS (pH 7.0) and dehydrated with a graded series of ethanol solutions from 30 to 100% for about 15 min at each step. The dehydrated samples were coated with gold-palladium and observed using SEM (Hitachi SU8010, Japan).
ε-Polylysine hydrochloride (>90% pure, w/w, Silver-Elephant Bio-engineering Co., Ltd., Zhejiang, China) was prepared as a stock solution (20 mg/ml) in distilled sterile water and diluted to work solution (5 mg/ml). First, minimal inhibitory concentration (MIC) and maximum sublethal concentrations (MSC) values of ε-PLH against PL and LM in TSB were obtained using the microwell dilution assay (Wang et al., 2020). MIC was defined as the lowest concentration of the antimicrobial that inhibited >90% of the growth of the tested microorganism (Oo et al., 2010). MSC was considered as the last tested concentration that allowed bacterial growth compared with control (Di Pasqua et al., 2006). Then, the diluted cultures of LM and PL were inoculated separately or mixed in TSB, which were supplemented with ε-PLH at the different concentrations of 0, 2, 4, 8, 16, 32, 64, and 128 μg/ml at the initial time. After incubation at 30°C for 24 h, the populations of biofilm cells as mono- or dual species were counted as mentioned in the “Counting of Planktonic and Biofilm Cell Populations” section, and biofilm biomass and extracellular polysaccharides were determined using the above CV assay and phenol-sulfuric acid method as described in the “Crystal Violet Biofilm and Extracellular Polysaccharides Assay” section, respectively.
The inhibitory activity of ε-PLH in combination with CEO on the formation of dual biofilms of LM and PL was further analyzed. CEO was obtained from Borui Spice Oil Co., Ltd. (>95% pure, v/v, Jiangxi, China). The diluted LM and PL cultures were mixed in equal volumes for dual-species inoculation in fresh TSB, with 5.0 log cfu/ml as the final cell concentration for each species. The two preservatives at the MSCs using alone or combination, including ε-PLH at 8 (P8) or 16 μg/ml (P16), CEO at 25 (C25) or 50 μg/ml (C50), and four combined groups (P8 + C25, P8 + C50, P16 + C25, P16 + C50), were supplemented to wells of the microtiter plate. After incubation at 30°C for 24 h, biofilm biomass and extracellular polysaccharides of the multispecies biofilms were determined. Then, CLSM and SEM were utilized to observe the effect of ε-PLH combined with CEO on the spatial structure of the dual biofilms.
In addition, ε-PLH and CEO were further tested against 24 h performed dual biofilms formed by LM and PL in microtiter plates for their ability to reduce the CV biomass (biofilm removal, %) and metabolic activity (biofilm inactivation, %). Briefly, the 24 h preformed biofilms in each well of 24-microtiter plates were carefully removed and washed with PBS (pH 7.0) to remove unattached cells. ε-PLH and CEO either alone or combination at high than MICs were supplemented to the preformed biofilm in the microtiter plates, including ε-PLH at 64 (P64) or 128 μg/ml (P128), CEO at 50 (C50) and 100 μg/ml (C100), four combined groups (P64 + C50, P64 + C100, P128 + C50, P128 + C100), and sterile peptone water as control. After treatment at 30°C for 30 min, the biomass of inhibitors exposed and non-exposed biofilms was quantified by CV staining. The percentage of biomass removal (% BR) was determined by Equation 1, where is the OD value for preservative non-exposed biofilms (control, C) and T is the OD value for inhibitor exposed biofilms.
The metabolic activity of preservative exposed and non-exposed biofilms was evaluated using XTT [2,3-bis (2-methoxy-4-nitro-5-sulfophenyl)-2H-tetrazolium-5-carbox-anilide] assay kit (Jiangsu Kaiji Biotechnology Co., Ltd., Nanjing, China). After incubation at 37°C for 4 h in the dark, the absorbance in each well was measured at 450 nm. The percentage of biofilm inactivation (% BI) was determined according to Equation 2, where C is the absorbance for control and T is the absorbance for inhibitor exposed biofilms.
Additionally, the effects of ε-PLH combined with CEO on the spatial structure of the 24 h performed dual biofilms were further examined by CLSM.
According to Bodor et al. (2008), autoinducer-2 (AI-2) activities of PL and LM as mono- or dual species were assayed using Vibrio harveyi BB170. Overnight culture of V. harveyi BB170 was diluted (1:5,000) in a fresh AB medium, and the diluted cells were dispensed into 96-well microplates, fresh AB medium as blank control. After incubation for 24 h at 30°C, the supernatant of mono and co-culture treated with ε-PLH and CEO at the MSCs was centrifuged (10,000 × g, 3 min), and then filtered through a 0.22 μm membrane filter. Each cell-free filter was added to each well of microplates containing the diluting BB170 strain. After incubated at 30°C with shaking, the bioluminescence of V. harveyi BB170 was monitored every 30 min using a luminometer (PerkinElmer Victor X, PerkinElmer Inc., Waltham, MA, United States), continuous for 6 h.
All the experiments were performed in three biological replicates, and the mean and standard deviation of experimental values were calculated. The figures were processed using Prism 8.0 software (GraphPad Software Inc., La Jolla, CA, United States), and statistical significance was assessed by one-way ANOVA method using SPSS 17.0 software package (SPSS Inc., Chicago, IL, United States). Significant differences among experimental groups of mono- and dual cultures treated with or without ε-PLH and CEO are expressed as p values of <0.05* and <0.01**.
The planktonic and biofilm cell population of LM and PL as mono- and dual-species cultures incubated at 30°C or 15°C are described in Table 1. The planktonic cell number of single LM reached 9.0 log cfu/ml during the stationary phase, which was 0.2 log cfu/ml higher than in the co-culture (p > 0.05). The planktonic population of PL in both mono- and dual-species cultures reached about 9.0 log cfu/ml in TSB. In addition, LM and PL strains were able to form mono-specie biofilm on the surface of SS, but their biofilm cell count of each strain was significantly lower than the corresponding planktonic cell (p < 0.05). In dual-species biofilms, PL attained approximately 0.6 log cfu/cm2 higher cell density compared to LM, thus constituting approximately 85% of the total population both at 30 and 15°C. Moreover, there was significant difference of LM or PL biofilm cell count between mono- and dual-species biofilms (p < 0.05). Biofilm cell densities of LM in dual-species biofilms reached 6.47–6.58 log cfu/cm2 at 30 and 15°C, in contrast to about 7.0 log cfu/cm2 in mono-species biofilm (p < 0.05). The dual-biofilm cell populations of PL were 7.26–7.40 log cfu/cm2 during mature phase, which were slightly lower than mono-species-attained numbers of biofilm cells (p < 0.05).
Table 1. Planktonic cell and biofilm cell of L. monocytogenes and P. lundensis in mono-culture and co-culture at 30 and 15°C on stainless steel coupons.
Moreover, PL showed significantly higher quantities of biofilm biomass (Figure 1A) and exopolysaccharides (Figure 1B) than LM in mono species (p < 0.01) using microtiter plates, indicating the strong biofilm-forming ability of PL. In spite of no difference of viable cell number, biofilm biomass and exopolysaccharide both in mono- or dual-species LM and PL were greatly higher at 15°C than those at 30°C, indicating that the low temperature could stimulate the secretion of biofilm extracellular matrix of the two strains. Unexpectedly, the levels of biofilm formation and polysaccharide production in the co-culture were significantly lower than that of mono-species PL both at two culture temperatures (p < 0.05).
Figure 1. Biofilm formation of L. monocytogenes and P. lundensis as mono- and dual species at 30 and 15°C. (A) Biofilm biomass by CV, (B) polysaccharides production, and (C) OM images (1,000×), CLSM staining living cells by SYTO9 (20×) and SEM images (4,000×). Data were expressed as mean ± standard deviations (n = 3). *p < 0.05; **p < 0.01: significant interspecies difference.
Meanwhile, OM, CLSM, and SEM were employed to visualize the adhesion and structure of mono- and dual-species biofilms at micro level (Figure 1C). Massive adhesive cells were distributed in the mono-species biofilm of LM and PL after 24 h by OM observation. Compared to LM, PL formed the denser and thicker maturing biofilm with multiple layers followed by CLSM and SEM analysis, while the dual-species biofilms developed sparer and higher heterogeneity than PL. In addition, LM and PL as mono-culture, and their co-culture formed the biofilms with thickness of 20.3, 100.5, and 90.4 μm, respectively. Furthermore, PL as mono- or dual species produced the large EPS wrapping cell aggregation forming spatial structure observed by SEM, especially mono-species PL. Generally, the results of these microscopies were consistent with those as determined by CV staining and exopolysaccharides.
The MICs of ε-PLH against LM and PL were 16 and 100 μg/ml in TSB, respectively (Supplementary Table 1). ε-PLH at the concentration lower than 8 and 50 μg/ml had no significant effect on the planktonic cell densities of LM and PL during the stationary phase, respectively, and these latter concentrations of 8 and 50 μg/ml were thus as MSCs of ε-PLH against the two strains. Effects of ε-PLH treatment on the biofilm formation of the two strains, cultivated under either mono- or dual-species conditions on SS coupons, were evaluated (Figure 2A). The treatment of ε-PLH resulted in a significant reduction of mono- or dual-species biofilm biomass of LM and PL compared with untreated control (p < 0.05). The biofilm cell counts of dual-species LM were apparently decreased after 32 μg/ml ε-PLH treatment compared with mono-species LM treated with 4 μg/ml ε-PLH. In contrast, the viable cell population of PL both in mono- and dual-culture biofilms treated with 64 μg/ml ε-PLH was significantly decreased (p < 0.05) and did not differ between two biofilm status (p > 0.05). More specifically, the ε-PLH treatment at 64 μg/ml achieved 4.32 and 0.85 log decrease of dual-species biofilms of LM and PL compared with 5.87 and 0.96 log reduction of individual mono-culture, respectively.
Figure 2. Effects of ε-PLH on the biofilm formation of L. monocytogenes and P. lundensis as mono- and dual species at 30°C for 24 h. (A) Reducing biofilm cells, (B) biofilm biomass by CV, and (C) polysaccharides production. Data were expressed as mean ± standard deviations (n = 3). *p < 0.05; **p < 0.01: significant interspecies difference.
The effects of ε-PLH at the different concentrations on the biofilm biomass and exopolysaccharide production of LM and PL as mono- or dual-species biofilms are illustrated in Figures 2B,C. When treated with ε-PLH ranging from 2 to 128 μg/ml, biofilm biomass of LM and PL as mono-culture was significantly reduced at 2 and 16 μg/ml of ε-PLH (p < 0.05), respectively, whereas the dual-species biofilms were apparently dropped at 32 μg/ml of ε-PLH. When treated with ε-PLH at 32 μg/ml, biofilm biomass for LM and PL as mono-species reduced by 83.6 and 36.9%, respectively, which were higher than that for the dual-species biofilms (31.0%). LM failed to form the biofilm in supplement with 64 μg/ml ε-PLH. Likewise, the exopolysaccharide production of LM and PL as mono-culture was decreased in the presence of ε-PLH at 2 and 16 μg/ml, respectively, compared with LM and PL as dual-species biofilms at 32 μg/ml ε-PLH (p < 0.05). When treated with ε-PLH at 32 μg/ml, exopolysaccharide of LM and PL as mono- and dual-species biofilms reduced by 79.22, 27.66, and 25.84%, respectively. These findings indicated that ε-PLH effectively inhibited the biofilm development of LM as mono species in a concentration-dependent manner; however, multispecies biofilms of the two strains exhibited their community-wide resistance, resulting in higher tolerance to ε-PLH than their counterparts in mono-species biofilm.
Due to the high tolerance of multispecies biofilms to ε-PLH, the impacts of ε-PLH combined with CEO at the MSCs against biofilm formation as dual-species LM and PL were further assayed (Figure 3A). Compared with no effect of alone ε-PLH and CEO at two MSCs, the inhibitory effect of four combinations of ε-PLH and CEO significantly enhanced against the dual biofilms (p < 0.05). After incubation for 24 h, the combined treatments of P8 + C25, P8 + C50, P16 + C25, and P16 + C50 exhibited 19.4, 43.2, 37.17, and 55.27% (p < 0.05) decrease in CV biomass, respectively. The inhibitory rate of the three combined groups except P8 + C25 was apparently higher compared with either of the compounds used alone (p < 0.05). Similarly, the four combined treatments showed 24.13, 46.87, 45.03, and 54.03% reduction in exopolysaccharide levels of dual-species biofilms, respectively, in contrast to treatment of P16 (7.4%) or C50 (25.87%) used alone (Figure 3B). It was revealed that ε-PLH in combination with CEO constituents at the MSCs induced a good synergistic effect to inhibit multispecies biofilm formation of LM and PL.
Figure 3. Effects of ε-PLH combined with CEOs on the biofilm formation, polysaccharides, and spatial structure of L. monocytogenes and P. lundensis as co-culture. (A) Biofilm biomass by CV, (B) polysaccharides production, (C) CLSM images, and (D) SEM images. Data were expressed as mean ± standard deviations (n = 3). *p < 0.05; **p < 0.01: significant interspecies difference.
We further observed the adhesion of dual-species biofilms formed by LM and PL treated with ε-PLH and CEO compounds by CLSM and SEM to assess the changes in biofilm spatial structures (Figure 3C). The two strains formed the dual biofilms with dense matrices, while the biofilm homogeneity gradually increased with the treatment of ε-PLH and CEO, as well as the decrease in viable cells. The spatial structure of dual biofilms was impaired by CEO at two MSCs in a concentration-dependent manner, in contrast to weak effect of ε-PLH at 8 and 16 μg/ml. Compared to the two compounds used alone, the four combined treatments apparently decreased the biovolume of viable cells with green signals and increased the dead cells with red signals in the dual-species biofilms. When treated with the combined groups of P8 + C25, P8 + C50, P16 + C25, and P16 + C50, the multispecies biofilm thickness was decreased to 71, 36, 42, and 23.7 μm, respectively, in contrast to 89.7 μm of thickness for dual-species biofilms control. Observations by SEM showed that many small amorphous aggregates (LM) appeared around the bacilli (PL) in the spare biofilms with less EPS matrix treated by P16 + C25 compared to control of dual-species biofilms (Figure 3D). As the concentration of combined CEO increased to 50 μg/ml (P16 + C50), few monolayers of PL adhered to surface and formed the biofilms without EPS matrix. The observations by CLSM and SEM were in line with the strong inhibition of biofilm biomass and exopolysaccharides secretion when combining ε-PLH with CEO. Thus, the combined treatments of ε-PLH and CEO at the MSCs repressed effectively adhesion and destroyed the multispecies biofilm spatial structures, indicating their synergistic inhibitory activity.
Autoinducer-2 activities of LM and PL were performed in co-culture treated by ε-PLH or CEO, as presented in Figure 4. The supernatants of the LM and PL as mono- or co-culture induced bioluminescence in V. harveyi BB170, especially PL. The AI-2 activity in the co-culture of the two strains was significantly lower than that of single PL, which might be consistent with the biofilm-forming ability. Moreover, AI-2 activity of co-culture was reduced by 3.42–14.92% and 5.54–17.81% after exposure to ε-PLH or CEO used alone, while the inhibitory rates of P8 + C50, P16 + C25, and P16 + C50 were as high as 35.55–46.89%, respectively, indicating the inhibitory efficiency of combined compounds in a concentration-dependent manner (p < 0.05).
Figure 4. Effects of ε-PLH combined with CEO on the AI-2 activity of L. monocytogenes and P. lundensis as co-culture. Data were expressed as mean ± standard deviations (n = 3). *p < 0.05; **p < 0.01: significant interspecies difference.
The combination of ε-PLH with CEO at higher than MICs was further applied to eradicate the preformed biofilms of LM and PL as dual species after incubation for 24 h. In Figures 5A,B, biofilm removal and inactivation of combined treatments significantly increased compared to the two compounds used alone. Approximately 47.08–60.36% of dual biofilms were removed after treatment with four combined groups of P64 + C50, P64 + C100, P128 + C50, and P128 + C100 compared with 43.82% with P128 and 36.38% with C100, respectively. As expected, the combined treatments of ε-PLH and CEO resulted in the great inactivation of cellular viability in performed biofilms as dual species. The four combined treatments showed 72.46–80.5% decrease in the cell viability of 24 h performed biofilms as dual species, which were higher than P128 (36.99%) and C100 (72.05%) used alone (p < 0.05). Additionally, ε-PLH was effective in the biofilm removal of dual-species biofilms, while CEO exhibited the strong inactivation of biofilm cell, which could be associated with their synergistic eradication of performed biofilms. Furthermore, CLSM images also confirmed that ε-PLH in combination with CEO significantly enhanced eradication of the multispecies biofilms, in contrast to either of the compounds used alone (Figure 5C). Following the combined treatment, viable cells with green disappeared and dead cells in red increased throughout the biofilms, indicating that the dual biofilms were susceptible to combined treatments, and the combined treatment of P128 + C100 showed the highest biofilm-eradicating efficacy. In addition, the spatial structure of LM and PL as dual-species biofilms was effectively destroyed by the combined compounds, as well as the significant decrease in biofilm thickness (p < 0.05). More specifically, the biofilm thickness treated with P128 + C100 was decreased by nearly 50% compared to the dual-biofilms control.
Figure 5. Eradication of ε-PLH combined with CEO on the performed dual-biofilms of L. monocytogenes and P. lundensis. (A) Biofilm removal measured by CV biomass, (B) biofilm inactivation measured by metabolic activity, and (C) CLSM images. Data were expressed as mean ± standard deviations (n = 3). *p < 0.05; **p < 0.01: significant interspecies difference.
Listeria monocytogenes has been isolated from various raw products and surfaces found in food processing environments following regular sanitation treatments, together with other microorganisms, mainly of spoilage and commensal microflora (Heir et al., 2019). This study evaluated the interactions of LM and PL as multispecies biofilms, as well as their sensitivity to ε-PLH in combination with CEO. It was shown that PL as mono- and dual species exhibited significantly higher biofilm formation than LM. Similarly, various Pseudomonas spp. readily form biofilms under the chilled conditions, such as P. fragi in chilled meat (Wickramasinghe et al., 2019), P. lundensis in pork meat (Liu et al., 2015), and P. fluorescens in fish (Zhu et al., 2019). Moreover, PL were here found to clearly dominate in the co-culture biofilm communities during the incubation period, rather than planktonic state. This was in agreement with the results of Papaioannou et al. (2018), who reported that Pseudomonas was the dominant species in mixed-species biofilm of LM. In addition, the incubation temperature showed a great increase on biofilm-forming ability of the two investigated psychrotrophs, similarly observed by Zhu et al. (2019) and Quintieri et al. (2020). For psychrotrophic species, the expression and properties of several bacterial structures, such as flagella, fimbrae, pilli, and curli, may be affected by cold temperature environment, subsequently stimulating bacterial attachment and biofilm formation (Quintieri et al., 2020).
Furthermore, the co-culture of LM with PL resulted in a significant decrease in biofilm cell and biomass, and formed the thin spare biofilm structure, which indicated that LM and PL displayed the competitive effects in the formation of dual-species biofilms. This is consistent with the findings of Puga et al. (2016, 2018), who observed a significant inhibitory effect of LM attachment when co-culture with Pseudomonas spp., Escherichia coli, and indigenous microorganisms compared with biofilms formed in individual pure cultures. The advantage of dominant PL over LM in dual-species biofilms could mainly be attributed to available space and nutrients to colonize between the two strains (Jahid and Ha, 2014; Wang et al., 2020). Recent works showed that E. coli O157:H7 was mainly distributed in the low layer of the dual-species biofilm, in contrast to a spoiler P. fragi in the top layer (Cheng et al., 2022). As obligatory aerobic and motile bacteria, Pseudomonas had high attachment rates and could quickly attach irreversibly to a surface and embed themselves in EPS (Mann and Wozniak, 2012). In whole multiple microbial communities, bacteria survived in top layers of multispecies were generally considered to present competitive advantages toward their counterparts occupying low layer due to easily obtained essential resources, including oxygen and nutrients (Jahid and Ha, 2014). Additionally, siderophore secreting from Pseudomonas could acquire iron from the environment, resulting in antagonism in the growth of neighboring microorganisms (Saha et al., 2013).
In this study, ε-PLH exhibited stronger antimicrobial and antibiofilm activity against LM than PL. Similar results were reported in Staphylococcus aureus (Tan et al., 2019). Like ε-polylysine, ε-PLH, as a water-soluble substance, induces structural changes in the peptidoglycan of cell wall in Gram-positive strains, causing a fragile structure of cell wall (Hyldgaard et al., 2014). Conversely, ε-PLH had a weak antibiofilm activity against PL, which could be related to strong biofilm-forming ability and high MIC of ε-PLH (Supplementary Table 1). Moreover, LM cells in the dual-species biofilm exhibited higher tolerance to ε-PLH than that in mono-species biofilm, indicating that the presence of Pseudomonas enhanced the survival of LM to ε-PLH. Single LM failed to form thick biofilms in the presence of shear forces, while in co-culture, the strong biofilm former Pseudomonas provided a protected biofilm with large EPS matrix in which LM could thrive (Fagerlund et al., 2021). Likewise, the presence of P. aeruginosa was showed to protect significantly Salmonella cells in biofilms from disinfection treatment (Pang et al., 2017). In contrast, Pseudomonas viable cells both in mono- or dual biofilms decreased significantly treated by 64 μg/ml ε-PLH, showing the similar resistance of two PL biofilm cells to ε-PLH. The competitive interactions of LM and PL decreased the cell adhesion and cell population of multispecies biofilms, but did not significantly influence the production of biofilm exopolysaccharide between co-culture and single PL, suggesting that the predominant species in coexistence with LM could mainly determine the structure and characteristics of the dual-species biofilms. As a shelter for bacterial community in biofilms, EPS in the multispecies biofilms could act as a diffusion-limiting barrier against the preservatives, resulting in limited compounds access to LM in the deeper layer of the biofilms (Karygianni et al., 2020). Previous studies demonstrated a competitive interaction and increased sanitizer resistance of LM when co-cultured with Pseudomonas putida (Saa Ibusquiza et al., 2012). The dominating competitive interactions among multispecies brewery biofilm, community members confer tolerance against the antimicrobial sulphathiazole, due to a reduction in competition upon antimicrobial treatment (Parijs and Steenackers, 2018). Conversely, Vibrio parahaemolyticus and LM were more susceptible to antibiotics when grown together in a dual-species biofilm due to the competitive interaction (Chen et al., 2019). These differences may be associated with several factors, such as specific strains, complex interactions, and different stresses (Chen et al., 2015). The specific mechanism of this cross-protection by the dominant species PL to LM in the multispecies biofilm community would be further explored.
Considering the high tolerance of dual-biofilms of LM and PL to ε-PLH used alone, the combination of ε-PLH and CEO was applied to inhibit biofilm formation and eradicate the performed biofilms of dual species. Interestingly, the adherent cell population and EPS produced by LM and PL as dual-species biofilms were remarkably repressed when treated with combined treatment at the MSCs, demonstrating a synergistic effect. When combined treatment of P16 + C25, many small amorphous aggregates appeared around the bacilli PL, speculating that LM biofilm cells transfer to growth state to survive in the adverse environment, such as viable-but-non-culturable state cells (VBNC). Truchado et al. (2021) reported that peroxyacetic acid and chlorine dioxide induced VBNC stage of LM and E. coli O157:H7 in wash water. Meanwhile, QS-mediated signaling is involved in promoting multispecies biofilms by entangling species, which are responsive toward AI-2 (Laganenka and Sourjik, 2018). Compared to single PL, quantity of AI-2 activity in the co-culture of LM and PL significantly decreased, which was positively correlated with the competition of biofilm formation as the dual-species cultures. Wang et al. (2020) indicated that DPD as precursor of AI-2 greatly promoted the development of dual-species biofilms of P. fluorescens and S. aureus. Furthermore, ε-PLH and CEO at the MSCs significantly repressed the reduction of AI-2 activity, as well as the biofilm formation as dual species, suggesting the antibiofilm activity of these compounds might be related to interference with AI-2. Indeed, cinnamon and main compound cinnamaldehyde also inhibit autoinducers of the QS system and biofilm formation without influencing bacterial growth (Vasconcelos et al., 2018).
The combined treatments of ε-PLH and CEO showed the apparent removal and inactivation of the performed biofilms of dual species, especially P128 combined with C100. The strong removal of ε-PLH indicated that the compound could effectively degrade the EPS matrix in structuring multi-biofilms, accelerating the entry of CEO into the biofilm cells. The high hydrophilicity of CEO probably enhanced the diffusion of cinnamon oil into the extracellular matrix, and targeted the inner LM and PL as dual-species biofilms by damaging cell membrane, altering the lipid profile, inhibiting ATPase (Vasconcelos et al., 2018), and causing the strong biofilm inactivation of CEO. Due to diverse modes of action affecting bacterial cells and cell targets, the synergistic combinations of ε-PLH and CEO had stronger antibiofilm activities than the compound used alone, resulting in a significant reduction in the viability of biofilm cell. Otherwise, EO effective concentration needs to be reduced to minimize consumer rejection or possible toxicological effects (Abdollahzadeh et al., 2014). The combination technology offers various advantages of using lower concentration, diminishing the formation of resistance capacity of microorganisms and achieving acceptable levels of pathogen inactivation not seen when the antimicrobial agents were applied singly (Soni et al., 2014). Combinations of thyme oil and nisin produced a high decrease in the population of LM present in minced fish meat (Abdollahzadeh et al., 2014) and minced beef (Solomakos et al., 2008) under refrigerated conditions.
The finding of this study provides evidence of a competitive interaction between LM and PL in the dual-species biofilms, rather than no difference in planktonic cells. The biomass, viable cells, and thickness of dual-species biofilms were much less than of each mono-species biofilm, which could be involved in the regulation of AI-2 activity. Compared to the dominant PL, LM biofilm cells as mono-culture were sensitive to ε-PLH, but in dual-species biofilms increased its tolerance due to the protective effect of Pseudomonas EPS matrix. Moreover, ε-PLH and CEO displayed synergistic antibiofilm effects against the dual-species biofilms of LM and PL. Overall, the combined preservatives of ε-PLH and CEO have the potential to be developed as multi-biofilm inhibitors used in the food industry.
The original contributions presented in this study are included in the article/Supplementary Material, further inquiries can be directed to the corresponding author.
JZ contributed to the conception, funding acquisition, supervision, resources, and writing – review and editing. JL contributed to the data curation, investigation, methodology, software, and writing – original draft. XH contributed to the data curation, investigation, methodology, and software. YS contributed to the writing –review and editing. All authors contributed to the article and approved the submitted version.
This study was supported by grants from the Public Projects of Zhejiang Province (LGN20C200007) and the Fundamental Research Funds for the Zhejiang Province Universities (XRK21001).
The authors declare that the research was conducted in the absence of any commercial or financial relationships that could be construed as a potential conflict of interest.
All claims expressed in this article are solely those of the authors and do not necessarily represent those of their affiliated organizations, or those of the publisher, the editors and the reviewers. Any product that may be evaluated in this article, or claim that may be made by its manufacturer, is not guaranteed or endorsed by the publisher.
The Supplementary Material for this article can be found online at: https://www.frontiersin.org/articles/10.3389/fmicb.2022.885502/full#supplementary-material
Abdollahzadeh, E., Rezaei, M., and Hosseini, H. (2014). Antibacterial activity of plant essential oils and extracts: the role of thyme essential oil, nisin, and their combination to control Listeria monocytogenes inoculated in minced fish meat. Food Control 35, 177–183. doi: 10.1016/j.foodcont.2013.07.004
Almeida, J. S., White, D. C., and Xavier, J. B. (2003). Automated biofilm morphology quantification from confocal laser scanning microscopy imaging. Water Sci. Technol. 47, 31–37. doi: 10.2166/wst.2003.0273
Bodor, A., Elxnat, B., Thiel, V., Schulz, S., and Wagner-Dobler, I. (2008). Potential for luxS related signalling in marine bacteria and production of autoinducer-2 in the genus Shewanella. BMC Microbiol. 8:13. doi: 10.1186/1471-2180-8-13
Bridier, A., Sanchez-Vizuete, P., Guilbaud, M., Piard, J. C., Naitali, M., and Briandet, R. (2015). Biofilm-associated persistence of food-borne pathogens. Food Microbiol. 45, 167–178. doi: 10.1016/j.fm.2014.04.015
Chen, D., Zhao, T., and Doyle, M. P. (2015). Single- and mixed-species biofilm formation by Escherichia coli O157:H7 and Salmonella, and their sensitivity to levulinic acid plus sodium dodecyl sulfate. Food Cont. 57, 48–53. doi: 10.1016/j.foodcont.2015.04.006
Chen, P., Wang, J. J., Hong, B., Tan, L., Yan, J., Zhang, Z., et al. (2019). Characterization of mixed-species biofilm formed by Vibrio parahaemolyticus and Listeria monocytogenes. Front. Microbiol. 10:2543. doi: 10.3389/fmicb.2019.02543
Cheng, Y., Zhang, S., Zhang, C., Mi, X., Zhang, W., Wang, L., et al. (2022). Escherichia coli O157:H7 is challenged by the presence of Pseudomonas, but successfully co-existed in dual-species microbial communities. Food Microbiol. 106:104034. doi: 10.1016/j.fm.2022.104034
Di Pasqua, R., Hoskins, N., Betts, G., and Mauriello, G. (2006). Changes in membrane fatty acids composition of microbial cells induced by addiction of thymol, carvacrol, limonene, cinnamaldehyde, and eugenol in the growing media. J. Agric. Food Chem. 54, 2745–2749. doi: 10.1021/jf052722l
Ding, T., Li, T., and Li, J. (2019). Discovery of quorum sensing inhibitors of Pseudomonas fluorescens P07 by using a receptor-based pharmacophore model and virtual screening. LWT 109, 171–178. doi: 10.1016/j.lwt.2019.04.030
Fagerlund, A., Langsrud, S., and Møretrø, T. (2021). Microbial diversity and ecology of biofilms in food industry environments associated with Listeria monocytogenes persistence. Curr. Opin. Food Sci. 37, 171–178. doi: 10.1016/j.cofs.2020.10.015
Fang, J., Feng, L., Lu, H., and Zhu, J. (2022). Metabolomics reveals spoilage characteristics and interaction of Pseudomonas lundensis and Brochothrix thermosphacta in refrigerated beef. Food Res. Inter. 156:111139. doi: 10.1016/j.foodres.2022.111139
Gandhi, M., and Chikindas, M. L. (2007). Listeria: A foodborne pathogen that knows how to survive. Int. J. Food Microbiol. 113, 1–15. doi: 10.1016/j.ijfoodmicro.2006.07.008
Giaouris, E., Chorianopoulos, N., Doulgeraki, A., and Nychas, G. J. (2013). Co-culture with Listeria monocytogenes within a dual-species biofilm community strongly increases resistance of Pseudomonas putida to benzalkonium chloride. PLoS One 8:e77276. doi: 10.1371/journal.pone.0077276
Heir, E., Liland, K. H., Carlehog, M., and Holck, A. L. (2019). Reduction and inhibition of Listeria monocytogenes in cold-smoked salmon by Verdad N6, a buffered vinegar fermentate, and UV-C treatments. Int. J. Food Microbiol. 291, 48–58. doi: 10.1016/j.ijfoodmicro.2018.10.026
Hyldgaard, M., Mygind, T., Vad, B. S., Stenvang, M., Otzen, D. E., and Meyer, R. L. (2014). The antimicrobial mechanism of action of epsilon-poly-l-lysine. Appl. Environ. Microbiol. 80, 7758–7770. doi: 10.1128/AEM.02204-14
Jahid, I. K., and Ha, S.-D. (2014). The paradox of mixed-species biofilms in the context of food safety. Compr. Rev. Food Sci. Food Saf. 13, 990–1011. doi: 10.1111/1541-4337.12087
Karygianni, L., Ren, Z., Koo, H., and Thurnheer, T. (2020). Biofilm matrixome: extracellular components in structured microbial communities. Trends Microbiol. 28, 668–681. doi: 10.1016/j.tim.2020.03.016
Laganenka, L., and Sourjik, V. (2018). Autoinducer 2-dependent Escherichia coli biofilm formation is enhanced in a dual-species coculture. Appl. Environ. Microbiol. 84, e02638–e02617. doi: 10.1128/AEM.02638-17
Li, Y., Cui, L., Du, F., Han, X., and Li, J. (2021). Impacts of ε−polylysine hydrochloride with thymol on biogenic amines formation and biochemical changes of squid (Illex argentinus). J. Food Process. Pres. 45:e15505. doi: 10.1111/jfpp.15505
Liu, Y. G., Dong, P. C., Zhu, L. X., Zhang, Y. M., and Luo, X. (2020). Effect of four kinds of natural antimicrobial compounds on the biofilm formation ability of Listeria monocytogenes isolated from beef processing plants in China. LWT 133:110020. doi: 10.1016/j.lwt.2020.110020
Liu, Y. J., Xie, J., Zhao, L. J., Qian, Y. F., Zhao, Y., and Liu, X. (2015). Biofilm formation characteristics of Pseudomonas lundensis isolated from meat. J. Food Sci. 80, M2904–M2910. doi: 10.1111/1750-3841.13142
Mann, E. E., and Wozniak, D. J. (2012). Pseudomonas biofilm matrix composition and niche biology. FEMS Microbiol. Rev. 36, 893–916. doi: 10.1111/j.1574-6976.2011.00322.x
Norwood, D. E., and Gilmour, A. (2000). The growth and resistance to sodium hypochlorite of Listeria monocytogenes in a steady-state multispecies biofilm. J. Appl. Microbiol. 88, 512–520. doi: 10.1046/j.1365-2672.2000.00990.x
Oo, T. Z., Cole, N., Garthwaite, L., Willcox, M. D., and Zhu, H. (2010). Evaluation of synergistic activity of bovine lactoferricin with antibiotics in corneal infection. J. Antimicrob. Chemother. 65, 1243–1251. doi: 10.1093/jac/dkq106
Pang, X., Wong, C., Chung, H.-J., and Yuk, H.-G. (2019). Biofilm formation of Listeria monocytogenes and its resistance to quaternary ammonium compounds in a simulated salmon processing environment. Food Control 98, 200–208. doi: 10.1016/j.foodcont.2018.11.029
Pang, X. Y., Yang, Y. S., and Yuk, H. G. (2017). Biofilm formation and disinfectant resistance of Salmonella spp. in mono- and dual-species with Pseudomonas aeruginosa. J. Appl. Microbiol. 123, 651–660. doi: 10.1111/jam.13521
Papaioannou, E., Giaouris, E. D., Berillis, P., and Boziaris, I. S. (2018). Dynamics of biofilm formation by Listeria monocytogenes on stainless steel under mono-species and mixed-culture simulated fish processing conditions and chemical disinfection challenges. Int. J. Food Microbiol. 267, 9–19. doi: 10.1016/j.ijfoodmicro.2017.12.020
Parijs, I., and Steenackers, H. P. (2018). Competitive inter-species interactions underlie the increased antimicrobial tolerance in multispecies brewery biofilms. ISME J. 12, 2061–2075. doi: 10.1038/s41396-018-0146-5
Piercey, M. J., Hingston, P. A., and Truelstrup Hansen, L. (2016). Genes involved in Listeria monocytogenes biofilm formation at a simulated food processing plant temperature of 15? Int. J. Food Microbiol. 223, 63–74. doi: 10.1016/j.ijfoodmicro.2016.02.009
Puga, C. H., Rodríguez-López, P., Cabo, M. L., SanJose, C., and Orgaz, B. (2018). Enzymatic dispersal of dual-species biofilms carrying Listeria monocytogenes and other associated food industry bacteria. Food Control 94, 222–228. doi: 10.1016/j.foodcont.2018.07.017
Puga, C. H., SanJose, C., and Orgaz, B. (2016). Biofilm development at low temperatures enhances Listeria monocytogenes resistance to chitosan. Food Control 65, 143–151. doi: 10.1016/j.foodcont.2016.01.012
Quintieri, L., Fanelli, F., Zuhlke, D., Caputo, L., Logrieco, A. F., Albrecht, D., et al. (2020). Biofilm and pathogenesis-related proteins in the foodborne P. fluorescens ITEM 17298 with distinctive phenotypes during cold storage. Front. Microbiol. 11:991. doi: 10.3389/fmicb.2020.00991
Rodriguez-Lopez, P., Rodriguez-Herrera, J. J., Vazquez-Sanchez, D., and Lopez Cabo, M. (2018). Current knowledge on Listeria monocytogenes biofilms in food-related environments: incidence, resistance to biocides, ecology and biocontrol. Foods 7:85. doi: 10.3390/foods7060085
Saa Ibusquiza, P., Herrera, J. J., Vazquez-Sanchez, D., Parada, A., and Cabo, M. L. (2012). A new and efficient method to obtain benzalkonium chloride adapted cells of Listeria monocytogenes. J. Microbiol. Methods 91, 57–61. doi: 10.1016/j.mimet.2012.07.009
Saha, R., Saha, N., Donofrio, R. S., and Bestervelt, L. L. (2013). Microbial siderophores: a mini review. J. Basic Microbiol. 53, 303–317. doi: 10.1002/jobm.201100552
Solomakos, N., Govaris, A., Koidis, P., and Botsoglou, N. (2008). The antimicrobial effect of thyme essential oil, nisin, and their combination against Listeria monocytogenes in minced beef during refrigerated storage. Food Microbiol. 25, 120–127. doi: 10.1016/j.fm.2007.07.002
Soni, D. K., Singh, M., Singh, D. V., and Dubey, S. K. (2014). Virulence and genotypic characterization of Listeria monocytogenes isolated from vegetable and soil samples. BMC Microbiol. 14:241. doi: 10.1186/s12866-014-0241-3
Stanborough, T., Fegan, N., Powell, S. M., Singh, T., Tamplin, M., and Chandry, P. S. (2018). Genomic and metabolic characterization of spoilage-associated Pseudomonas species. Int. J. Food Microbiol. 268, 61–72. doi: 10.1016/j.ijfoodmicro.2018.01.005
Tan, Z., Shi, Y., Xing, B., Hou, Y., Cui, J., and Jia, S. (2019). The antimicrobial effects and mechanism of ε-poly-lysine against Staphylococcus aureus. Bioresour. Bioprocess. 6:11. doi: 10.1186/s40643-019-0246-8
Tenderis, B., Kılıç, B., Yalçın, H., and Şimşek, A. (2021). Controlling growth of Listeria monocytogenes and Pseudomonas fluorescens in thermally processed ground beef by sodium lactate, encapsulated or unencapsulated polyphosphates incorporation. LWT 144:111169. doi: 10.1016/j.lwt.2021.111169
Truchado, P., Gil, M. I., and Allende, A. (2021). Peroxyacetic acid and chlorine dioxide unlike chlorine induce viable but non-culturable (VBNC) stage of Listeria monocytogenes and Escherichia coli O157:H7 in wash water. Food Microbiol. 100:103866. doi: 10.1016/j.fm.2021.103866
Vasconcelos, N. G., Croda, J., and Simionatto, S. (2018). Antibacterial mechanisms of cinnamon and its constituents: A review. Microb. Pathog. 120, 198–203. doi: 10.1016/j.micpath.2018.04.036
Vázquez-Sánchez, D., Galvão, J. A., Ambrosio, C. M. S., Gloria, E. M., and Oetterer, M. (2018). Single and binary applications of essential oils effectively control Listeria monocytogenes biofilms. Ind. Crop. Prod. 121, 452–460. doi: 10.1016/j.indcrop.2018.05.045
Wang, Y., Hong, X., Liu, J., Zhu, J., and Chen, J. (2020). Interactions between fish isolates Pseudomonas fluorescens and Staphylococcus aureus in dual-species biofilms and sensitivity to carvacrol. Food Microbiol. 91:103506. doi: 10.1016/j.fm.2020.103506
Wickramasinghe, N. N., Ravensdale, J., Coorey, R., Chandry, S. P., and Dykes, G. A. (2019). The predominance of psychrotrophic Pseudomonads on aerobically stored chilled red meat. Compr. Rev. Food Sci. Food Saf. 18, 1622–1635. doi: 10.1111/1541-4337.12483
Keywords: Listeria monocytogenes, Pseudomonas lundensis, ε-polylysine hydrochloride, cinnamon essential oil, dual-species biofilms
Citation: Zhu J, Liu J, Hong X and Sun Y (2022) Synergism With ε-Polylysine Hydrochloride and Cinnamon Essential Oil Against Dual-Species Biofilms of Listeria monocytogenes and Pseudomonas lundensis. Front. Microbiol. 13:885502. doi: 10.3389/fmicb.2022.885502
Received: 28 February 2022; Accepted: 10 May 2022;
Published: 10 June 2022.
Edited by:
Faizan Ahmed Sadiq, Flanders Research Institute for Agriculture, Fisheries and Food, BelgiumReviewed by:
Koen De Reu, Institute for Agricultural, Fisheries and Food Research (ILVO), BelgiumCopyright © 2022 Zhu, Liu, Hong and Sun. This is an open-access article distributed under the terms of the Creative Commons Attribution License (CC BY). The use, distribution or reproduction in other forums is permitted, provided the original author(s) and the copyright owner(s) are credited and that the original publication in this journal is cited, in accordance with accepted academic practice. No use, distribution or reproduction is permitted which does not comply with these terms.
*Correspondence: Junli Zhu, anVubGl6aHUwMzA1QDE2My5jb20=
Disclaimer: All claims expressed in this article are solely those of the authors and do not necessarily represent those of their affiliated organizations, or those of the publisher, the editors and the reviewers. Any product that may be evaluated in this article or claim that may be made by its manufacturer is not guaranteed or endorsed by the publisher.
Research integrity at Frontiers
Learn more about the work of our research integrity team to safeguard the quality of each article we publish.