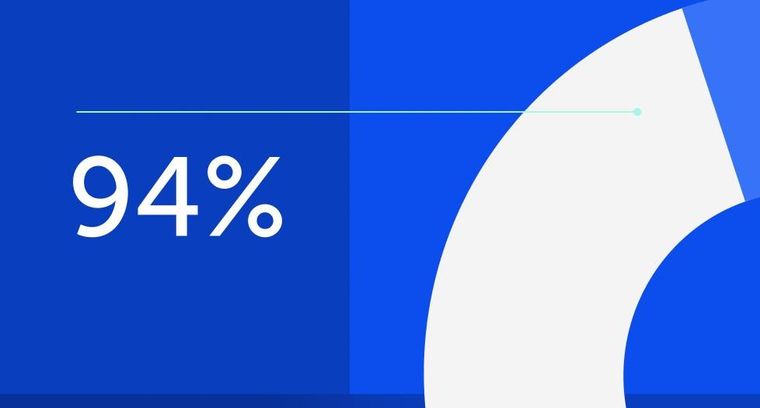
94% of researchers rate our articles as excellent or good
Learn more about the work of our research integrity team to safeguard the quality of each article we publish.
Find out more
REVIEW article
Front. Microbiol., 06 May 2022
Sec. Microorganisms in Vertebrate Digestive Systems
Volume 13 - 2022 | https://doi.org/10.3389/fmicb.2022.877776
Gut microbiota is key to human health and disease. Convincing studies have demonstrated that dysbiosis in the commensal gut microbiota is associated with intestinal and extra-intestinal diseases. Recent explorations have significantly contributed to the understanding of the relationship between gut microbiota and bone diseases (osteoporosis, osteoarthritis, rheumatoid arthritis, and bone cancer). Gut microbiota and its metabolites may become associated with the development and progression of bone disorders owing to their critical role in nutrient absorption, immunomodulation, and the gut–brain–bone axis (regulation hormones). In this work, we review the recent developments addressing the effect of gut microbiota modulation on skeletal diseases and explore a feasible preventive approach and therapy for bone diseases.
The microorganisms in the human gastrointestinal tract, henceforth referred to as gut microbiota (gut microbiota; most of them bacteria but also viruses, fungi, archaea, and protozoa), have a symbiotic relationship with the human body (Bäckhed et al., 2005). Bacteria are an abundant component of the microbiome, particularly in the gut, where the ratio of gut bacteria to the host cells is much closer to 1:1 (Sender et al., 2016). However, gut microbiota encodes more than 3 million genes, approximately 150 times the number of genes in the host genome; it regulates various functions of the host and consequently influences host health, phenotype, and diseases. Thus, the gut microbiome is now considered as a virtual metabolic organ in the host body (Eckburg et al., 2005; Rath and Dorrestein, 2012; Vyas and Ranganathan, 2012). In recent years, different levels of evidence have demonstrated the pivotal role of gut microbiota in both human health and diseases. Gut microbiota participates in the regulation of various physiological processes in the human body, including modulation of host physiological development and functions, such as nutrient absorption and production, immune function, metabolic balance, and resistance to pathogens at different period of ages (Dominguez-Bello et al., 2019). It is also associated with many complex human intestinal and extra-intestinal diseases, such as inflammatory bowel disease, obesity, diabetes, cardiovascular disease, cancer, and depression (Guarner and Malagelada, 2003; Wang et al., 2011; Valdes et al., 2018; Sepich-Poore et al., 2021). Furthermore, gut microbiota strongly interacts with certain drugs to influence their response and efficacy (Vich Vila et al., 2020).
Bone disorders, such as osteoporosis (OP), osteoarthritis (OA), and rheumatoid arthritis (RA), present one of the major threats to human health with the aging of society (Briggs et al., 2016). The most common characteristics of these diseases are pain, reduced physical function, significant disability, and increased mortality (Xia et al., 2019). The prevention and treatment of most skeletal disorders include pharmacological interventions (e.g., non-disease-specific drugs that ameliorate pain or more specific ones targeting the pathophysiology of skeletal diseases) and non-pharmacological interventions (e.g., an active lifestyle; an adequate intake of dietary protein, calcium, and vitamin D; and weight management; Papageorgiou and Biver, 2021). Despite the continuous development of these treatments, which indeed relieve the primary pain and improve the quality of life of patients, there is still a considerable proportion of patients gain little or no benefit and even experience adverse effects (Tu et al., 2018). For example, Opioids, as the potent analgesics for their efficacy in ameliorating pain in chronic bone disorders, are concerning due to associated addiction, gastrointestinal symptoms, and respiratory inhibition in a substantial proportion of patients (Wang and Roy, 2017). Therefore, this global challenge requires urgent and feasible solutions to improve the outcomes of these diseases.
There has been growing interest in the role of gut microbiota as an inducer or modulator of bone health and diseases, which is predominantly driven by an improved understanding of the crucial role of gut microbiota and its metabolites in maintaining structural and functional homeostasis (Zmora et al., 2019). The studies with different levels of evidence supporting these associations range from those in animal models to human studies. In the present review, we summarize the communication between gut microbiota and the host that contributes to the development and progression of some bone diseases and discuss potential mechanisms underlying these diseases. We have classified the potential mechanisms that link gut microbiota to bone health into three categories: (1) nutrient absorption, (2) immunomodulation, and (3) gut–brain–bone axis (Figure 1). Furthermore, we describe in detail the relationship of gut microbiota with skeletal outcomes, such as OP, OA, and RA and its role in bone tumor.
Figure 1. Schematic representation for the role of gut microbiota in bone health and disease. The potential mechanisms include (1) changes in nutrition absorption (i.e., increase in microbial metabolites with health benefits, such as SCFAs); (2) changes in immunomodulation (i.e., regulation of immune cells and cytokines); (3) regulation of the gut–brain–bone axis (i.e., 5-HT). FMT, fecal microbiota transplantation; SCFAs, short-chain fatty acids; Ca, Calcium; VitD, vitamin D; VitB, vitamin B; VitK, vitamin K; and 5-HT, 5-hydroxytryptamine.
Gut microbiota provides the host with essential capacities for the fermentation of non-digestible substrates, such as dietary fiber and endogenous intestinal mucus, production of various vitamins, biotransformation of bile acids, and synthesis of essential and non-essential amino acids. Notably, these functionalities have beneficial or detrimental effects on bone through alterations in gut microbiota composition and function.
Calcium is a key nutrient necessary for human bone health, and its deficiency may precipitate or exacerbate osteopenia and OP (Holick, 2007). It has been found that increased dietary calcium intake improves bone mineral density in a short time. Calcium intake is a significant predictor of the total body bone mass (Zhu et al., 2008). It was shown that probiotic supplement (Lactobacillus strains) is beneficial for calcium transport and uptake in mice (Raveschot et al., 2020). Probiotics, which are defined as “live microorganisms” confer health benefits to the host when administered in adequate amounts (Morelli and Capurso, 2012). In line with this, a human trial demonstrated that supplementation with Lactobacillus helveticus fermented milk in postmenopausal women increased serum calcium levels and reduced serum parathyroid hormone (PTH) levels (Narva et al., 2004). The changes in calcium and PTH suggest a benefit to bone health.
In addition, studies on prebiotics provide several indications for the regulation of calcium absorption by the manipulation of gut microbiota. Prebiotics cannot be hydrolyzed and absorbed by the gut; however, they are defined as substrates that can be selectively used by host gastrointestinal micro-organisms resulting in a health benefit (Bindels et al., 2015). All compounds considered prebiotics are microbiota accessible carbohydrates or fermentable dietary fiber, however, the reverse is not true (Deehan et al., 2017). Prebiotics, especially non-digestible oligosaccharides are the most promising and the most investigated substances in association with calcium intake and metabolism (Scholz-Ahrens et al., 2007). In an experiment with male Sprague Dawley rats, the consumption of galactooligosaccharides (GOS) led to increased calcium and magnesium absorption and bone density (Weaver et al., 2011). Moreover, in growing Sprague Dawley rats, calcium and magnesium absorption increased in a dose–response manner with GOS supplementation (Weaver et al., 2011). These benefits to mineral utilization were associated with decreased cecal pH, increased cecal wall and content weight, and increased proportion of Bifidobacterium (Weaver et al., 2011). Another study found that calcium absorption in postmenopausal women was higher in the 20 g GOS treatment group than in the control group (van den Heuvel et al., 2000).
Probiotics and prebiotics improve calcium bioavailability through several potential mechanisms. First, the ingestion of probiotics and prebiotics is associated with an increase in cell density, intestinal crypt depth, and blood flow, which may increase intestinal surface area and enhance calcium absorption (Narva et al., 2004; Raschka and Daniel, 2005). Second, the transformation of prebiotics to short-chain fatty acids (SCFAs) under metabolism by gut microbiota lower the pH in the intestinal lumen; such changes may hinder the formation of calcium phytate/oxalate complexes and increase calcium solubility, thereby increasing the amount of available calcium for absorption (Weaver, 2015). Third, probiotics and prebiotics may upregulate the expression of calcium transporters, which increases calcium absorption, reduces PTH, and has downstream effects on bone by reduction in bone resorption (Ohta et al., 1998; Raveschot et al., 2020). The potential beneficial effect of calcium ingestion on skeletal health may be associated with the modifications of intestinal microbiota and integrity. Calcium supplementation or high calcium diet in animal models has been demonstrated to increase the number of potentially beneficial bacteria (e.g., Lactobacillus, Ruminococcaceae, and Akkermansia) and SCFA production, maintain intestinal integrity, prevent endotoxemia, and regulate tight junction proteins (Hwang et al., 2013; Gomes et al., 2015; Chaplin et al., 2016; Nadeem Aslam et al., 2016; Cha et al., 2020).
It is well established that vitamin D has an immense influence on promoting gut calcium and phosphorus absorption and bone metabolism (Holick, 2007). The gut microbiome regulates vitamin D metabolism and function, as evidenced by the finding that germ-free (GF) mice have defective vitamin D metabolism (low 1, 25-dihydroxvitamin D levels; hypocalcemia), whereas colonization of GF mice with microbiota showed recovered levels of 1, 25-dihydroxvitamin D and calcium (Bora et al., 2018). Furthermore, a clinical trial showed that the oral probiotic supplementation of Lactobacillus reuteri NCIMB 30242 increases the level of mean circulating 25-hydroxyvitamin D (Jones et al., 2013). The recent discovery that the vitamin D receptor (VDR) is highly expressed in the gastrointestinal tract suggests that the effects of vitamin D are exerted via the endocrine and immune systems. Vitamin D activates the VDR and plays a role in maintaining the intestinal epithelial barrier function and gut microbiota eubiosis (Barbáchano et al., 2017). Dysbiotic gut microbiota profiles with an increased abundance of the phyla Bacteroidetes and Proteobacteria and decreased abundance of the phyla Firmicutes and Deferribacteres in the feces were reported in VDR knockout mice vs. wild-type mice, and the disorder was more likely to induce colitis in VDR knockout mice, whereas after vitamin D supplementation, inflammation was controlled and the proportion of Firmicutes and Deferribacteres was restored (Ooi et al., 2013). Additional investigations provided evidence that vitamin D deficiency induces gut inflammation by lower expression of E-cadherin at epithelial surfaces and immune cells, and fewer tolerogenic dendritic cells in mouse models (Ooi et al., 2013). Such changes (increased host inflammation) may allow pathogens to outcompete commensal bacteria, resulting in the upregulation or downregulation of immune responses (Ooi et al., 2013). The finding is consistent with observations in human studies, which showed that the differences in gut microbiota composition and inflammatory markers are in accord with vitamin D intake or vitamin D levels (Charoenngam et al., 2020). Moreover, multiple epidemiological studies have shown that humans with low serum vitamin D levels are at increased risk of multiple adverse health outcomes including OP, cancer, autoimmune diseases, and cardiovascular disease (Bikle, 2016). Therefore, vitamin D supplementation may reinstate a healthier gut microbiome profile and weaken inflammation and it is important to ensure sufficient vitamin intake in daily life.
Dietary fiber (defined as edible carbohydrate polymers or complex carbohydrates) is resistant to the endogenous digestive enzymes and thus is neither hydrolyzed nor absorbed in the small intestine (Jones, 2014) because the human genome encodes a limited number of glycoside hydrolases and no polysaccharide lyases (collectively referred to carbohydrate-active enzymes or CAZymes; Cantarel et al., 2012). In contrast, the gut microbiome is estimated to encode thousands of CAZymes (Cantarel et al., 2012). Thus, the degradation and fermentation of dietary fiber are one of the dominant functions of gut microbiota (multiple common genera including Phascolarcto bacterium, Roseburia, Bacteroides, Clostridium, Ruminococcus; Labbé et al., 2014). Carbohydrate metabolism is a major source of energy for gut epithelial cells and a minor supply of nutrients and energy source for gut microbe growth and proliferation (such as Desulfotomaculum spp.; Guarner and Malagelada, 2003). Moreover, it produces SCFAs and hydrogen gas (Wong et al., 2006).
Studies have investigated the association between dietary fiber and skeletal outcomes involving SCFAs, such as signaling molecules regulating bone homeostasis (bone formation and resorption) either by inhibiting histone deacetylases (HDACs) or by acting as ligands for several G protein-coupled receptors. They thus affect various physiological processes and may contribute to bone health and disease. Butyrate, the most abundant SCFA, promotes osteoblast differentiation by inhibiting HDACs (Lee et al., 2006) and stimulates mineralized nodule formation and osteoprotegerin (OPG) expression (Katono et al., 2008) to promote bone formation. It also directly stimulates osteoblast activation and bone formation via T regulatory cell-mediated regulation of WNT10b expression in CD8+ T cells by producing TGFβ (Tyagi et al., 2018). SCFAs other than butyrate, also have direct effects on osteoclasts (Rahman et al., 2003; Katono et al., 2008). They directly induce metabolic reprogramming of osteoclast precursors, resulting in enhanced glycolysis at the expense of oxidative phosphorylation, thereby downregulating essential osteoclast genes, such as TRAF6 and NFATc1, and this metabolic process does not require the participation of G protein-coupled receptors (Lucas et al., 2018). Moreover, the indirect effects of SCFAs on osteoclasts may contribute to their ability to induce T regulatory (Treg) cells, which have been shown to suppress osteoclast differentiation (Zaiss et al., 2007, 2010). In summary, these findings suggest that SCFAs are potent regulators of bone metabolism and homeostasis. However, excessive SCFA intake has adverse effects on the host. A high-fiber diet in mice, which causes a significant increase in butyrate in the gut, is associated with a markedly increased risk of susceptibility to pathogenic Escherichia coli O157:H7; it is likely that the intestinal tissue of the mice with a high-fiber diet bound to more Shiga toxin 1 and expressed more globotriaosylceramide (Zumbrun et al., 2013).
Gut microbial enzymes also have been directly implicated in the conversion of other specific products; for example, insulin-like growth factor 1 (IGF-1), produced predominantly in the liver in response to food intake and regulated by microbes and microbial products, was the first metabolite identified to be a mediator in the gut–bone axis (Yan et al., 2016; Novince et al., 2017).
Nutrient absorption is affected by host diet, and the dietary ingredients have an important effect on host health (Valdes et al., 2018). Adequate consumption of dietary protein is important for maintaining bone health because proper dietary intake causes positive changes in gut microbiota and promotes the absorption of nutrients by the intestinal epithelial mucosal barrier, which is beneficial for skeletal metabolism (Rizzoli et al., 2018). In turn, the gut microbiome is implicated in protein metabolism and utilization. For example, the intestinal bacteria hydrolyze undigested proteins into amino acids and synthesize essential amino acids from nitrogen sources (Davila et al., 2013; Neis et al., 2015). Intestinal microbes also play a key role in the synthesis of vitamin B and K and bile acid metabolism (LeBlanc et al., 2013; Guzior and Quinn, 2021). Vitamin B and K are essential for bone health (Boskey et al., 1998; Hoang et al., 2003; Poundarik et al., 2012; Stone et al., 2017), and bile acids may play a key role in the regulation of calcium absorption (Long et al., 2017). Therefore, in addition to gut microbiota homeostasis, a balanced diet and an appropriate ratio of dietary fiber, proteins, and minerals positively affect the bone health. Otherwise, it may negatively affect the bone health, leading to skeletal diseases.
Interactions between gut microbiota and the host immune system begin at birth. In the gastrointestinal tract, numerous microbial communities present on the mucosal surface or in the intestinal lumen. The host intestinal epithelium, which ensures the anatomical separation of gut microbiota from the host, plays a key role in regulating immune responses and supporting mutualistic associations between the host and gut microbiota (Schroeder and Bäckhed, 2016; Kundu et al., 2017; Levy et al., 2017). The intestinal epithelial cells are connected to each other by intercellular junctions (tight junctions, adhesion junctions, and desmosomes). These junctions work together with selective permeability, which allows the passage of nutrients across the epithelial layer and helps beneficial microorganisms to interact with the intestinal mucosal immune cells to influence their maturation and differentiation. They also protect the integrity of the epithelial layer. Mucosal barriers contain not only a single layer of epithelial cells but are also shielded by a thick mucus layer and various other physical (such as peristalsis) and biochemical (such as secretory immunoglobulin A) means (Turner, 2009). Below the epithelium is a host of immune cells, which act as a third line of defense to prevent the gut microbiota and pathogens from entering the systemic circulation. These gut immune cells, including macrophages, dendritic cells, T cells, and B cells, are housed within gut-associated lymphoid tissues, such as Peyer’s Patches or scattered throughout the lamina propria (Perez-Lopez et al., 2016; Kobayashi et al., 2019; Figure 2).
Figure 2. Gut microbial balance (BLUE) vs. gut microbial dysbiosis (RED). When in intestinal homeostasis, gut barrier contributes to surveillance the gut for the entrance of any pathogens. Disruption of the homeostatic relationship between the gut microbiota and gut barrier, the increasing intestinal permeability is involved in further increasing inflammation via gut mucosal immune system, leading to disease.
Gut microbiota and its specific molecules promote intestinal homeostasis, barrier integrity, and immune maturation by signaling to the innate and adaptive immune systems, leading to the host’s immune response and the release of protective peptides and cytokines. The result can be a protective response to commensal bacteria, an inflammatory response to pathogenic organisms, or a trigger for host cell apoptosis. For example, effects of Escherichia coli Nissle1917 through the Toll-like receptor (TLR) signaling pathway are necessary to inhibit intestinal inflammatory and maintain gut barrier homeostasis in inflammatory bowel disease (Damoogh et al., 2021).
Disrupted barrier function is linked to an increased risk of inflammation and immune-mediated diseases (Gensollen et al., 2016; von Mutius and Vercelli, 2019). Diabetes and obesity are well-known examples of impaired barrier function, which further increases inflammation (Nagpal et al., 2018; Thaiss et al., 2018). Another study suggested an association between arthritis and dysfunction of crucial barrier proteins, such as tight junction proteins (Tajik et al., 2020). However, to communicate with distant organs, gut microbial signals first need to be transmitted across the intestinal epithelium. These signals (active molecular) can be either structural components of the bacteria (such as LPS) or metabolites produced from the microbiota (SCFAs, bile acid) that affect distal organs either directly or by signaling through nerves or hormones from the gut. The microbe–host communication is essential to maintain vital functions in a healthy host, which enables the gut bacteria to connect to the immune and hormonal systems, to the brain (the gut–brain axis), and to the host.
The gut–brain axis refers to the network of connections that integrates neural, hormonal, and immunological signaling between the gut and the brain (Johnson and Foster, 2018). The communication system is bidirectional. Signaling from the brain via the autonomic nervous system and the hypothalamic–pituitary–adrenal axis influences processes associated with gastrointestinal homeostasis, such as peristalsis and transit, mucin production, immune functions, and intestinal permeability, relative gut microbial abundance, and gene expression patterns in certain gut microorganisms (Mayer, 2011; Moreira et al., 2016; Yu et al., 2017). Conversely, gut microbiota communicates with the brain via hundreds of metabolites, which are sensed by specialized cells in the gut, including enteroendocrine cells (EECs), enterochromaffin cells (ECCs), and primary or secondary afferent nerve endings. Sensing of bacterial metabolites by these cells results in neural signals to the brain and interactions with gut-based immune cells leading to local and systemic immune activation, or circulating metabolites enter the central nervous system by crossing the blood–brain barrier and directly affect neuroactivity (Rothhammer et al., 2016). Based on the gut–brain axis as a multidirectional interactive communication highway, these signaling processes influence multiple organs (the gut and non-gut organs, such as liver, brain, and bone).
In recent years, serotonin [5-hydroxytryptamine (5-HT)], an important and widely studied neurotransmitter, has been shown to regulate bone metabolism via the gut microbiota (Sjögren et al., 2012). 5-HT, a critical regulator of bone health, has two types depending on its synthesis site: brain-derived serotonin (BDS) and gut-derived serotonin (GDS). Interestingly, the two types of 5-HT fulfill distinct functions; gut-derived 5-HT has a negative effect on bone formation, whereas brain-derived 5-HT has the opposite effect (Pawlak et al., 2017). The enterochromaffin cells of the duodenum are responsible for GDS synthesis, which is partially regulated by gut microbiota (Reigstad et al., 2015). Both osteoblasts and osteoclasts synthesize 5-HT, express serotonin receptors, and regulate the uptake of 5-HT. In rats, an increased level of 5-HT induced bone loss (Bliziotes et al., 2006; Park et al., 2018). Another study showed that gut microbiota not only induced T cells and cytokines to regulate bone metabolism but also regulated the level of GDS by promoting a decrease in the rate-limiting enzyme of 5-HT biosynthesis, tryptophan hydroxylase-1, and an increase in serotonin transporter (Sjögren et al., 2012). However, recolonization of germ-free mice (GF mice) with a normal gut microbiota caused only a small change in the level of 5-HT after normalization of bone mass. It cannot be neglected that the intestinal microbiome regulates bone mass through 5-HT in the gut–brain axis. Therefore, the established links that have been made among microbes, the brain, and bones (the gut–brain–bone axis) support the feasibility of a novel approach in the treatment of bone disorders.
Previous studies have established associations between gut microbiota and a seemingly ever-increasing number of diseases, syndromes, and functional aberrations. Bone is an essential organ of the human body, which relies on the dynamic balance between osteoblasts and osteoclasts to maintain its normal function, and imbalance between osteoblasts and osteoclasts may lead to bone diseases that are also closely associated with gut microbiota. In the following paragraphs, we review the communication between gut microbiota and the host that contributes to the development and progression of some bone diseases and discuss how misconfigured signaling may contribute to these diseases.
OP, the most prevalent metabolic bone disease affecting society and families, is characterized by the deterioration of bone microarchitecture and low bone mass and leads to bone fragility and even bone fractures (Estell and Rosen, 2021). With the general aging of the population, as many as 200 million people worldwide have OP, and approximately 9 million fractures occur annually (Pisani et al., 2016). This disease is likely the result of several complicating factors including nutrition, hormones, sex, heredity, and lifestyle. The most recognizable regulators of bone metabolism are estrogen, calcium, vitamin D, PTH, and inflammatory factors; however, the pathogenesis of OP remains under explored. Despite a range of effective compounds to reduce fracture risk, the treatment rates are still low among high-risk individuals (Pisani et al., 2016).
OP is most common in postmenopausal women; however, it occurs in individuals of any sex and at any age (Horowitz, 1993). Declining estrogen levels stimulate excessive osteoclast formation and bone resorption, driving rapid bone loss (Horowitz, 1993). Gut microbiota dysbiosis, such as lower diversity of gut microbiota, may result in a reduction in circulating estrogens because gut microbiota regulates estrogens through the secretion of β-glucuronidase, an enzyme that deconjugates estrogens into their active forms (Baker et al., 2017). These results can be verified in ovariectomy (OVX) mice, a model of postmenopausal OP; OVX induces bone loss through microbial-dependent trafficking of intestinal TNF+ T cells and Th17 cells owing to increased levels of IL-17a, TNF, and RANKL (Yu et al., 2021). However, probiotic treatment based on different species (mainly including Lactobacillus) prevents the bone loss induced by OVX (Chiang and Pan, 2011; Britton et al., 2014; Ohlsson et al., 2014; Li et al., 2016). For instance, Lactobacillus paracasei and Lactobacillus plantarum enhance bone volume/tissue volume, trabecular number, trabecular thickness, and cortical bone loss by reducing the expression of osteoclastogenic cytokines (TNF-α and IL-1β) and increased OPG expression in osteoporotic mice (Ohlsson et al., 2014). Moreover, Bifidobacterium longum also increases bone mass density in OVX rats with bone loss (Parvaneh et al., 2015). Furthermore, studies have demonstrated that supplementation with L. reuteri is an effective treatment for trabecular bone loss in mice with OP owing to glucocorticoid-induced or post-antibiotic-induced intestinal microbial dysbiosis and barrier dysfunction (Schepper et al., 2019, 2020).
As described above, sex steroid depletion increases the production of pro-inflammatory and pro-osteoclastogenic cytokines resulting in OP. Moreover, sex steroid deficiency augments inflammation in the gut by increasing intestinal permeability, allowing the translocation of bacteria, and increasing the number of bacterial antigens entering the epithelial mucosa (Li et al., 2016). In mice, the effect of gut microbiota in increasing these cytokines and reducing bone mass is dependent on NOD1 and NOD2 signaling, which elicits an inflammatory response (Ohlsson et al., 2017). Toll-like receptor 5 (TLR5) is the innate immune receptor for flagellin, and knockout mice for this receptor show changes in gut microbiota, which leads to an immune system deficiency. Studies have demonstrated that activation of TLR5 prompts osteoclast formation and bone loss in mice. Moreover, TLR5 knockout mice present with increasing periosteal expansion, which is normalized when there is a disruption in gut microbiota. This is consistent with the mediation role of gut microbiota (low microbial diversity; Guss et al., 2017).
Recently, studies have indicated a potential role of gut microbiota in the pathogenesis of OP. Compared to conventionally raised (CONV-R) mice, GF mice were protected against OP and the increase in bone turnover induced by sex steroid deprivation because of the lack of increase in TNF, RANKL, and IL-17 (Li et al., 2016). At the same time, the number of osteoclasts per bone surface were decreased. The frequency of the CD4+ T cells and CD11b+/Gr1− osteoclast precursor cells in the bone marrow reduced, whereas bone formation was not affected (Sjögren et al., 2012). Consistent with this finding, GF mice colonized with conventional microbiota after sexual maturity had lower trabecular bone mass and significantly higher bone resorption marker CTX-I 1 month after colonization (Li et al., 2016; Yan et al., 2016). Similarly, a role of gut microbiota in the pathogenesis of OP was indicated by studies mainly on the development of lymphoid cells and production of circulating cytokines.
Taken together, the gut microbiota-dependent expansion of bone marrow Th17 cells and TNF-α-producing T cells, which increases their production of pro-inflammatory and pro-osteoclastogenic cytokines, such as TNF-α, IL-17, and RANKL, and decreases the secretion of the RANKL antagonist OPG (D’Amelio et al., 2008; Pacifici, 2012), may be the potential immunomodulatory mechanism in the development of OP. RANKL induces osteoclast formation, TNF potentiates RANKL activity and induces the expansion of Th17 cells, and IL-17 reduces bone formation (Cenci et al., 2000; Lam et al., 2000; Chen et al., 2011; Sugita et al., 2012; Li et al., 2015; Uluçkan et al., 2016). In addition, newly produced osteoclasts activate TNF-α-producing CD4+ T cells and induce the production of Treg cells (Ibáñez et al., 2016). Th17 cells, an osteoclastogenic population of CD4+ T cells, are defined by their ability to produce IL-17 and also secrete RANKL and TNF (Miossec et al., 2009; Basu et al., 2013), whereas Treg cells (differentiation from native CD4+ T cells through the intrinsic epigenetic upregulation of the transcription factor gene FOXP3 in CD4+ T cells) suppress immune responses, which can induce and maintain the immune tolerance of the host, and decrease chronic inflammation via various mechanisms including the production of the immunosuppressive cytokines IL-10 and TGF-β (Bollrath and Powrie, 2013; Tyagi et al., 2018). As described above, the relationship between Th17 cells and Treg cells is complex; it can either inhibit or promote bone health. However, intestinal bacteria may control this balance (Figure 3).
Figure 3. Potential gut microbiota-related mechanisms in the development of osteoporosis related to the bone remodeling balance including: 1 influencing absorption of nutrition, decreasing in estrogen biotransformation and the level of IGF-1, then regulating bone formation; 2 The GM-dependent expansion of lymphoid cells and cytokines could result in the formation and differentiation of osteoclasts thereby promoting bone destruction; and 3 changing the level of serotonin via the gut–brain–bone axis.
IGF-1, an important hormone mainly produced in the liver and regulated by microbes and microbial products, plays a vital role in modulating skeletal development and postnatal growth. For example, colonization of sexually mature germ-free (GF) mice with conventional specific pathogen-free (SPF) gut microbiota increased both bone formation and resorption and increased circulating IGF-1. SCFA supplementation in antibiotic-treated mice restores serum IGF-1 and bone mass to the levels equivalent to those in non-antibiotic-treated mice. The study indicates that SCFAs produced by gut microbiota via the fermentation of non-digestible polysaccharides positively promote the production of serum IGF-1, and gut microbiota may provide an anabolic stimulus to the skeleton through IGF-1 (Yan et al., 2016; Shen et al., 2021).
The majority of these findings demonstrate that GF mice have increased bone mass. In contrast to these observations, a study by Schwarzer and colleagues demonstrated that femur length is longer, and trabecular and cortical bone mass is significantly higher in CONV-R mice than in GF mice, with no difference in the bone mineral density (Schwarzer et al., 2016). Several studies on mice treated with antibiotics to alter the composition of gut microbiota present different conclusions regarding the effect on bone density (Cho et al., 2012; Cox et al., 2014). To sum up, these discrepancies may be explained by the differences in the bone mass phenotypes of GF mice and bone density measurement and antibiotic treatment protocols used (Cho et al., 2012; Sjögren et al., 2012; Cox et al., 2014; Li et al., 2016; Schwarzer et al., 2016; Yan et al., 2016).
However, data on the association between the human gut microbiota and OP is very limited. Recently, it was shown that gut microbiota structure and diversity, especially the abundance of Dialister and Faecalibacterium, are significantly enriched in patients with OP compared with those in healthy controls. However, the findings link the differences in gut microbiota components neither with inflammation and immune system nor with bone turnover (Xu et al., 2020). Moreover, it has been shown that probiotics also affect bone health in humans. Intervention with Lactobacillus casei Shirota in elderly men and women improved distal radius fracture healing (Lei et al., 2016), and another study showed that in healthy postmenopausal Japanese women, the total hip bone mineral density was increased in the group treated by the probiotic Bacillus subtilis C-3102 over 6 months compared with the control group treated by the placebo (Takimoto et al., 2018; Table 1).
OA, the most common type of arthritis, is a degenerative disease with low-grade inflammation, a leading cause of joint pain and disability worldwide, especially among the elderly (2018). Pathological changes in OA affect all joint tissues, leading to degradation of cartilage and bone, abnormal bone formation (osteophytes), and inflammation of the synovial membrane (synovitis). Previous studies have shown that obesity and metabolic syndrome are well-known risk factors for OA through overweight load on joint and low-grade systemic inflammation, and gut microbiota is implicated in its pathogenesis (Yoshimura et al., 2012; Johnson and Hunter, 2014; Huang and Kraus, 2016). In general, gut microbiota alleviates obesity through various mechanisms, which would have a positive influence on modifying the risk of OA. Accordingly, some animal studies with ingenious designs demonstrated that mice following a long-term high-fat diet or high-sucrose diet are prone to develop obesity-mediated knee OA (Griffin et al., 2012; Collins et al., 2015). However, this risk and the symptoms are reduced by intervention with L. casei or the prebiotic oligofructose (So et al., 2011; Schott et al., 2018). Moreover, other studies recently demonstrated that changes in the abundance of Fusobacterium, Faecalibacterium, and Ruminococcus play a key role in exacerbating OA in mice. In addition, studies show that high levels of lipopolysaccharide (LPS) in the serum and synovial fluid are associated with knee OA severity, macrophage-associated inflammation, and worsening of OA pathology (Huang and Kraus, 2016; Huang et al., 2016). Moreover, a large population study in adults recently validated that the abundance of Streptococcus species is associated with increased knee pain driven by local inflammation in the knee joint (Boer et al., 2019). Notably, randomized, double-blind, placebo-controlled clinical trials in patients with knee OA have also shown that probiotics L. casei Shirota and Streptococcus thermophilus (TCI633) have a positive effect of improvement in knee OA (Lei et al., 2017; Lyu et al., 2020; Table 1).
RA is a systemic chronic inflammatory disease mediated by immune system that leads to progressive joint destruction and impairs the quality of life. To date, many studies have focused on RA to study the role microbiota in autoimmunity. Modified gut microbiota structure has been observed in RA in both animal and human studies (Lyu et al., 2020). In RA cases, Prevotella and various Lactobacillus species have been shown to be more abundant at the species level (Liu et al., 2013). Therefore, the increased abundance of Prevotella and imbalance in the gastrointestinal microbiota are potential sources of the initiation or progression of RA. However, the mechanism of action of Prevotella-induced RA remains unknown. Probiotics containing Lactobacillus spp. (mainly L. casei and Lactobacillus acidophilus) are commonly used as alleviating agents or food supplements to manage RA and maintain general health (Vaghef-Mehrabany et al., 2014; Paul et al., 2021; Table 1).
Recently, emerging scientific advances have significantly contributed to the understanding of the potential associations between gut microbiota and the initiation, progression, and prognosis of multiple cancer types (Sepich-Poore et al., 2021). The International Agency for Research on Cancer has designated specific pathogens as procarcinogens and carcinogens in gastrointestinal and non-gastrointestinal cancers in humans (de Martel et al., 2020). Among them, Helicobacter pylori, which colonizes the gastric mucosa, accounts for approximately 50% of individuals worldwide; it is identified as the main cause of chronic gastric inflammation (Suerbaum and Michetti, 2002), and its role in the etiology of human gastric cancer has also been identified (Alfarouk et al., 2019). The potential mechanism through which H. pylori mediates the carcinogenesis of gastric cancer is associated with the presence of urease, Lewis antigens, cytotoxin-associated gene A, vacuolating cytotoxin, and BabA2 and induces chronic inflammation and promotes carcinogenesis (Alfarouk et al., 2019). The gram-negative anaerobic commensal Bacteroides fragilis has been identified to be potentially involved in colorectal tumorigenesis (Allen et al., 2019; Maiuri et al., 2019; DeStefano Shields et al., 2021), and there is emerging evidence for the involvement of Acidovorax (phylum Proteobacteria) in the development of lung cancer (Greathouse et al., 2018). Advances in 16S rRNA gene sequencing technology have opened up a new frontier in human gut microbiota analysis during cancer onset or progression. Metabolomics or metatranscriptomics have further advanced microbiome analyses, allowing researchers to characterize both the microbiome and its function in the development of human cancers. This knowledge of the gut microbiome in a multitude of human cancers offers an insight into the development of microbial-based diagnosis and treatments with the aim of enhancing patient care and outcomes.
Bone cancer, especially osteosarcoma, usually occurs in children and adolescents. Although the clinical treatment (including surgical techniques, chemotherapy, and radiotherapy) has advanced significantly in the last few decades, the outcome is still poor in patients with metastatic disease at present or in relapse, resulting in a high mortality rate. Thus, bone cancer treatment remains a major challenge worldwide. To date, the association between gut microbiota and bone tumor has not been reported. Herein, we focus on studying the characteristics of gut microbiota in patients with bone tumor and how specific bacteria and its structure affect tumor development and progression. Our study will explore a field to combine bone tumor and gut microbiota and may provide feasible prevention and therapy for bone cancer-related disease. It is of great significance of improve the prognosis and survival rate of patients.
Over the past decades, experiments using different approaches and studies in humans have provided remarkable novel insights into the complex interplay between gut microbiota and bone diseases. We analyzed recent studies that address the association between gut microbiota and certain skeletal disorders and propose potential mechanisms. Thus, gut microbiota has emerged as an important regulator of bone homeostasis. Nevertheless, current evidence is limited and often indirect. For example, an understanding of the interaction between gut microbiota and OP pathology and treatment (probiotics, prebiotics, fecal microbiota transplantation) is mostly derived from animal model studies that explored the contribution of gut microbiota in bone metabolic pathways (related to calcium and hormones, for example) rather than the effect of specific gut microbiota and its characteristics in these processes. However, the interactions have only rarely been assessed in humans. Therefore, well-controlled clinical trials are needed to explore how differences in microbiota composition affect different bone disorders including bone cancer. Further understanding of these aspects may aid the development of tools that take into consideration patients’ gut microbiomes and guide advances in targeted therapeutics based on gut microbiota, which may have the potential to improve the efficacy of these therapeutics.
YC and ZR designed the study. YC, XW, CZ, and CL retrieve references and analyze data. YC wrote the manuscript. ZR revised the manuscript. All authors contributed to the article and approved the submitted version.
This study was sponsored by grants from National Key Research and Development Program of China (2018YFC2000501), National Natural Science Foundation of China (U2004121), and China Postdoctoral Science Foundation (2020T130609).
The authors declare that the research was conducted in the absence of any commercial or financial relationships that could be construed as a potential conflict of interest.
All claims expressed in this article are solely those of the authors and do not necessarily represent those of their affiliated organizations, or those of the publisher, the editors and the reviewers. Any product that may be evaluated in this article, or claim that may be made by its manufacturer, is not guaranteed or endorsed by the publisher.
We thank ZR from Department of Infectious Diseases, The First Affiliated Hospital of Zhengzhou University for the help of the study design.
OP, Osteoporosis; OA, Osteoarthritis; RA, Rheumatoid arthritis; PTH, Parathyroid hormone; SCFAs, Short-chain fatty acids; HDACs, Histone deacetylases; OPG, Osteoprotegerin; Treg cells, T Regulatory cells; IGF-1, Insulin-like growth factor 1; TLR, Toll-like receptor; 5-HT, 5-Hydroxytryptamine; GDS, Gut-derived serotonin; GF mice, Germ-free mice; OVX, Ovariectomy; CONV-R mice, Conventionally raised mice; SPF, Specific pathogen-free.
Alfarouk, K., Bashir, A., Aljarbou, A., Ramadan, A., Muddathir, A., AlHoufie, S., et al. (2019). Helicobacter pylori the possible role of in gastric cancer and its management. Front. Oncol. 9:75. doi: 10.3389/fonc.2019.00075
Allen, J., Hao, S., Sears, C., and Timp, W. J. I. (2019). Epigenetic changes induced by Bacteroides fragilis toxin. Infect. Immun. 87, e00447–e00518. doi: 10.1128/iai.00447-18
Bäckhed, F., Ley, R. E., Sonnenburg, J. L., Peterson, D. A., and Gordon, J. I. (2005). Host-bacterial mutualism in the human intestine. Science 307, 1915–1920. doi: 10.1126/science.1104816
Baker, J., Al-Nakkash, L., and Herbst-Kralovetz, M. J. M. (2017). Estrogen-gut microbiome axis: physiological and clinical implications. Maturitas 103, 45–53. doi: 10.1016/j.maturitas.2017.06.025
Barbáchano, A., Fernández-Barral, A., Ferrer-Mayorga, G., Costales-Carrera, A., Larriba, M., Muñoz, A. J. M., et al. (2017). The endocrine vitamin D system in the gut. Mol. Cell. Endocrinol. 453, 79–87. doi: 10.1016/j.mce.2016.11.028
Basu, R., Hatton, R. D., and Weaver, C. T. (2013). The Th17 family: flexibility follows function. Immunol. Rev. 252, 89–103. doi: 10.1111/imr.12035
Bikle, D. D. (2016). Extraskeletal actions of vitamin D. Ann. N. Y. Acad. Sci. 1376, 29–52. doi: 10.1111/nyas.13219
Bindels, L., Delzenne, N., Cani, P., and Walter, J. (2015). Towards a more comprehensive concept for prebiotics. Nat. Rev. Gastroenterol. Hepatol. 12, 303–310. doi: 10.1038/nrgastro.2015.47
Bliziotes, M., Eshleman, A., Burt-Pichat, B., Zhang, X., Hashimoto, J., Wiren, K., et al. (2006). Serotonin transporter and receptor expression in osteocytic MLO-Y4 cells. Bone 39, 1313–1321. doi: 10.1016/j.bone.2006.06.009
Boer, C., Radjabzadeh, D., Medina-Gomez, C., Garmaeva, S., Schiphof, D., Arp, P., et al. (2019). Intestinal microbiome composition and its relation to joint pain and inflammation. Nat. Commun. 10:4881. doi: 10.1038/s41467-019-12873-4
Bollrath, J., and Powrie, F. J. S. (2013). Immunology. Feed your Tregs more fiber. Science 341, 463–464. doi: 10.1126/science.1242674
Bora, S. A., Kennett, M. J., Smith, P. B., Patterson, A. D., and Cantorna, M. T. (2018). The gut microbiota regulates endocrine vitamin D metabolism through fibroblast growth factor 23. Front. Immunol. 9:408. doi: 10.3389/fimmu.2018.00408
Boskey, A., Gadaleta, S., Gundberg, C., Doty, S., Ducy, P., and Karsenty, G. J. B. (1998). Fourier transform infrared microspectroscopic analysis of bones of osteocalcin-deficient mice provides insight into the function of osteocalcin. Bone 23, 187–196. doi: 10.1016/S8756-3282(98)00092-1
Briggs, A. M., Cross, M. J., Hoy, D. G., Sànchez-Riera, L., Blyth, F. M., Woolf, A. D., et al. (2016). Musculoskeletal health conditions represent a global threat to healthy aging: a report for the 2015 World Health Organization world report on ageing and health. Gerontologist 56(Suppl. 2), S243–S255. doi: 10.1093/geront/gnw002
Britton, R. A., Irwin, R., Quach, D., Schaefer, L., Zhang, J., Lee, T., et al. (2014). Probiotic L. reuteri treatment prevents bone loss in a menopausal ovariectomized mouse model. J. Cell. Physiol. 229, 1822–1830. doi: 10.1002/jcp.24636
Cantarel, B., Lombard, V., and Henrissat, B. (2012). Complex carbohydrate utilization by the healthy human microbiome. PLoS One 7:e28742. doi: 10.1371/journal.pone.0028742
Cenci, S., Weitzmann, M. N., Roggia, C., Namba, N., Novack, D., Woodring, J., et al. (2000). Estrogen deficiency induces bone loss by enhancing T-cell production of TNF-alpha. J. Clin. Invest. 106, 1229–1237. doi: 10.1172/JCI11066
Cha, K. H., Yang, J. S., Kim, K. A., Yoon, K. Y., Song, D. G., Erdene-Ochir, E., et al. (2020). Improvement in host metabolic homeostasis and alteration in gut microbiota in mice on the high-fat diet: a comparison of calcium supplements. Food Res. Int. 136:109495. doi: 10.1016/j.foodres.2020.109495
Chaplin, A., Parra, P., Laraichi, S., Serra, F., and Palou, A. (2016). Calcium supplementation modulates gut microbiota in a prebiotic manner in dietary obese mice. Mol. Nutr. Food Res. 60, 468–480. doi: 10.1002/mnfr.201500480
Charoenngam, N., Shirvani, A., Kalajian, T. A., Song, A., and Holick, M. F. (2020). The effect of various doses of oral vitamin D(3) supplementation on gut microbiota in healthy adults: a randomized, double-blinded, dose-response study. Anticancer Res. 40, 551–556. doi: 10.21873/anticanres.13984
Chen, D. Y., Chen, Y. M., Chen, H. H., Hsieh, C. W., Lin, C. C., and Lan, J. L. (2011). Increasing levels of circulating Th17 cells and interleukin-17 in rheumatoid arthritis patients with an inadequate response to anti-TNF-α therapy. Arthritis Res. Ther. 13:R126. doi: 10.1186/ar3431
Chiang, S. S., and Pan, T. M. (2011). Antiosteoporotic effects of lactobacillus -fermented soy skim milk on bone mineral density and the microstructure of femoral bone in ovariectomized mice. J. Agric. Food Chem. 59, 7734–7742. doi: 10.1021/jf2013716
Cho, I., Yamanishi, S., Cox, L., Methé, B., Zavadil, J., Li, K., et al. (2012). Antibiotics in early life alter the murine colonic microbiome and adiposity. Nature 488, 621–626. doi: 10.1038/nature11400
Collins, K., Reimer, R., Seerattan, R., Leonard, T., and Herzog, W. (2015). Using diet-induced obesity to understand a metabolic subtype of osteoarthritis in rats. Osteoarthr. Cartil. 23, 957–965. doi: 10.1016/j.joca.2015.01.015
Cox, L., Yamanishi, S., Sohn, J., Alekseyenko, A., Leung, J., Cho, I., et al. (2014). Altering the intestinal microbiota during a critical developmental window has lasting metabolic consequences. Cell 158, 705–721. doi: 10.1016/j.cell.2014.05.052
D’Amelio, P., Grimaldi, A., Di Bella, S., Brianza, S., Cristofaro, M., Tamone, C., et al. (2008). Estrogen deficiency increases osteoclastogenesis up-regulating T cells activity: a key mechanism in osteoporosis. Bone 43, 92–100. doi: 10.1016/j.bone.2008.02.017
Damoogh, S., Vosough, M., Hadifar, S., Rasoli, M., Gorjipour, A., Falsafi, S., et al. (2021). Evaluation of E. coli Nissle1917 derived metabolites in modulating key mediator genes of the TLR signaling pathway. BMC Res. Notes 14:156. doi: 10.1186/s13104-021-05568-x
Davila, A. M., Blachier, F., Gotteland, M., Andriamihaja, M., Benetti, P. H., Sanz, Y., et al. (2013). Intestinal luminal nitrogen metabolism: role of the gut microbiota and consequences for the host. Pharmacol. Res. 68, 95–107. doi: 10.1016/j.phrs.2012.11.005
de Martel, C., Georges, D., Bray, F., Ferlay, J., and Clifford, G. M. (2020). Global burden of cancer attributable to infections in 2018: a worldwide incidence analysis. Lancet Glob. Health 8, e180–e190. doi: 10.1016/S2214-109X(19)30488-7
Deehan, E., Duar, R., Armet, A., Perez-Muñoz, M., Jin, M., and Walter, J. J. M. S. (2017). Modulation of the gastrointestinal microbiome with nondigestible fermentable carbohydrates to improve human health. Microbiol. Spectr. 5. doi: 10.1128/microbiolspec.BAD-0019-2017
DeStefano Shields, C., White, J., Chung, L., Wenzel, A., Hicks, J., Tam, A., et al. (2021). BRAF bacterial-driven inflammation and mutant expression combine to promote murine colon tumorigenesis that is sensitive to immune checkpoint therapy. Cancer Discov. 11, 1792–1807. doi: 10.1158/2159-8290.CD-20-0770
Dominguez-Bello, M. G., Godoy-Vitorino, F., Knight, R., and Blaser, M. J. (2019). Role of the microbiome in human development. Gut 68, 1108–1114. doi: 10.1136/gutjnl-2018-317503
Eckburg, P. B., Bik, E. M., Bernstein, C. N., Purdom, E., Dethlefsen, L., Sargent, M., et al. (2005). Diversity of the human intestinal microbial flora. Science 308, 1635–1638. doi: 10.1126/science.1110591
Estell, E., and Rosen, C. J. (2021). Emerging insights into the comparative effectiveness of anabolic therapies for osteoporosis. Nat. Rev. Endocrinol. 17, 31–46. doi: 10.1038/s41574-020-00426-5
Gensollen, T., Iyer, S., Kasper, D., and Blumberg, R. J. (2016). How colonization by microbiota in early life shapes the immune system. Science 352, 539–544. doi: 10.1126/science.aad9378
Gomes, J. M., Costa, J. A., and Alfenas, R. C. (2015). Could the beneficial effects of dietary calcium on obesity and diabetes control be mediated by changes in intestinal microbiota and integrity? Br. J. Nutr. 114, 1756–1765. doi: 10.1017/S0007114515003608
Greathouse, K., White, J., Vargas, A., Bliskovsky, V., Beck, J., von Muhlinen, N., et al. (2018). Interaction between the microbiome and TP53 in human lung cancer. Genome Biol. 19:123. doi: 10.1186/s13059-018-1501-6
Griffin, T., Huebner, J., Kraus, V., Yan, Z., and Guilak, F. (2012). Induction of osteoarthritis and metabolic inflammation by a very high-fat diet in mice: effects of short-term exercise. Arthritis Rheum. 64, 443–453. doi: 10.1002/art.33332
Guarner, F., and Malagelada, J. R. (2003). Gut flora in health and disease. Lancet 361, 512–519. doi: 10.1016/S0140-6736(03)12489-0
Guss, J. D., Horsfield, M. W., Fontenele, F. F., Sandoval, T. N., Luna, M., Apoorva, F., et al. (2017). Alterations to the gut microbiome impair bone strength and tissue material properties. J. Bone Miner. Res. 32, 1343–1353. doi: 10.1002/jbmr.3114
Guzior, D., and Quinn, R. J. M. (2021). Review: microbial transformations of human bile acids. Microbiome 9:140. doi: 10.1186/s40168-021-01101-1
Hoang, Q., Sicheri, F., Howard, A., and Yang, D. J. N. (2003). Bone recognition mechanism of porcine osteocalcin from crystal structure. Nature 425, 977–980. doi: 10.1038/nature02079
Holick, M. F. (2007). Vitamin D deficiency. N. Engl. J. Med. 357, 266–281. doi: 10.1056/NEJMra070553
Horowitz, M. J. S. (1993). Cytokines and estrogen in bone: anti-osteoporotic effects. Science 260, 626–627. doi: 10.1126/science.8480174
Huang, Z., and Kraus, V. B. (2016). Does lipopolysaccharide-mediated inflammation have a role in OA? Nat. Rev. Rheumatol. 12, 123–129. doi: 10.1038/nrrheum.2015.158
Huang, Z., Stabler, T., Pei, F., and Kraus, V. J. O. (2016). Both systemic and local lipopolysaccharide (LPS) burden are associated with knee OA severity and inflammation. Osteoarthr. Cartil. 24, 1769–1775. doi: 10.1016/j.joca.2016.05.008
Hwang, I., Yang, H., Kang, H. S., Ahn, C., Hong, E. J., An, B. S., et al. (2013). Alteration of tight junction gene expression by calcium- and vitamin D-deficient diet in the duodenum of calbindin-null mice. Int. J. Mol. Sci. 14, 22997–23010. doi: 10.3390/ijms141122997
Ibáñez, L., Abou-Ezzi, G., Ciucci, T., Amiot, V., Belaïd, N., Obino, D., et al. (2016). Inflammatory osteoclasts prime TNFα-producing CD4 T cells and express CX CR1. J. Bone Miner. Res. 31, 1899–1908. doi: 10.1002/jbmr.2868
Johnson, K., and Foster, K. R. (2018). Why does the microbiome affect behaviour? Nat. Rev. Microbiol. 16, 647–655. doi: 10.1038/s41579-018-0014-3
Johnson, V. L., and Hunter, D. J. (2014). The epidemiology of osteoarthritis. Best Pract. Res. Clin. Rheumatol. 28, 5–15. doi: 10.1016/j.berh.2014.01.004
Jones, J. M. (2014). CODEX-aligned dietary fiber definitions help to bridge the ‘fiber gap’. Nutr. J. 13:34. doi: 10.1186/1475-2891-13-34
Jones, M., Martoni, C., and Prakash, S. (2013). Oral supplementation with probiotic L. reuteri NCIMB 30242 increases mean circulating 25-hydroxyvitamin D: a post hoc analysis of a randomized controlled trial. J. Clin. Endocrinol. Metab. 98, 2944–2951. doi: 10.1210/jc.2012-4262
Katono, T., Kawato, T., Tanabe, N., Suzuki, N., Iida, T., Morozumi, A., et al. (2008). Sodium butyrate stimulates mineralized nodule formation and osteoprotegerin expression by human osteoblasts. Arch. Oral Biol. 53, 903–909. doi: 10.1016/j.archoralbio.2008.02.016
Kobayashi, N., Takahashi, D., Takano, S., Kimura, S., and Hase, K. J. F. I. I. (2019). The roles of peyer's patches and microfold cells in the gut immune system: relevance to autoimmune diseases. Front. Immunol. 10:2345. doi: 10.3389/fimmu.2019.02345
Kundu, P., Blacher, E., Elinav, E., and Pettersson, S. (2017). Our gut microbiome: the evolving inner self. Cell 171, 1481–1493. doi: 10.1016/j.cell.2017.11.024
Labbé, A., Ganopolsky, J., Martoni, C., Prakash, S., and Jones, M. L. (2014). Bacterial bile metabolising gene abundance in Crohn's, ulcerative colitis and type 2 diabetes metagenomes. PLoS One 9:e115175. doi: 10.1371/journal.pone.0115175
Lam, J., Takeshita, S., Barker, J. E., Kanagawa, O., Ross, F. P., and Teitelbaum, S. L. (2000). TNF-alpha induces osteoclastogenesis by direct stimulation of macrophages exposed to permissive levels of RANK ligand. J. Clin. Invest. 106, 1481–1488. doi: 10.1172/JCI11176
LeBlanc, J., Milani, C., de Giori, G., Sesma, F., van Sinderen, D., and Ventura, M. (2013). Bacteria as vitamin suppliers to their host: a gut microbiota perspective. Curr. Opin. Biotechnol. 24, 160–168. doi: 10.1016/j.copbio.2012.08.005
Lee, H., Suh, J., Kim, A., Lee, Y., Park, S., and Kim, J. B. (2006). Histone deacetylase 1-mediated histone modification regulates osteoblast differentiation. Mol. Endocrinol. 20, 2432–2443. doi: 10.1210/me.2006-0061
Lei, M., Guo, C., Wang, D., Zhang, C., and Hua, L. (2017). The effect of probiotic Lactobacillus casei Shirota on knee osteoarthritis: a randomised double-blind, placebo-controlled clinical trial. Benefic. Microbes 8, 697–703. doi: 10.3920/BM2016.0207
Lei, M., Hua, L. M., and Wang, D. W. (2016). The effect of probiotic treatment on elderly patients with distal radius fracture: a prospective double-blind, placebo-controlled randomised clinical trial. Benefic. Microbes 7, 631–637. doi: 10.3920/BM2016.0067
Levy, M., Kolodziejczyk, A., Thaiss, C., and Elinav, E. (2017). Dysbiosis and the immune system. Nat. Rev. Immunol. 17, 219–232. doi: 10.1038/nri.2017.7
Li, J., Chassaing, B., Tyagi, A., Vaccaro, C., Luo, T., Adams, J., et al. (2016). Sex steroid deficiency-associated bone loss is microbiota dependent and prevented by probiotics. J. Clin. Invest. 126, 2049–2063. doi: 10.1172/JCI86062
Li, J. Y., D'Amelio, P., Robinson, J., Walker, L. D., Vaccaro, C., Luo, T., et al. (2015). IL-17A is increased in humans with primary hyperparathyroidism and mediates PTH-induced bone loss in mice. Cell Metab. 22, 799–810. doi: 10.1016/j.cmet.2015.09.012
Liu, X., Zou, Q., Zeng, B., Fang, Y., and Wei, H. (2013). Analysis of fecal lactobacillus community structure in patients with early rheumatoid arthritis. Curr. Microbiol. 67, 170–176. doi: 10.1007/s00284-013-0338-1
Long, S., Gahan, C., and Joyce, S. A. (2017). Interactions between gut bacteria and bile in health and disease. Mol. Asp. Med. 56, 54–65. doi: 10.1016/j.mam.2017.06.002
Lucas, S., Omata, Y., Hofmann, J., Böttcher, M., Iljazovic, A., Sarter, K., et al. (2018). Short-chain fatty acids regulate systemic bone mass and protect from pathological bone loss. Nat. Commun. 9:55. doi: 10.1038/s41467-017-02490-4
Lyu, J. L., Wang, T. M., Chen, Y. H., Chang, S. T., Wu, M. S., Lin, Y. H., et al. (2020). Oral intake of streptococcus thermophil us improves knee osteoarthritis degeneration: a randomized, double-blind, placebo-controlled clinical study. Heliyon 6:e03757. doi: 10.1016/j.heliyon.2020.e03757
Maiuri, A., Savant, S., Podicheti, R., Rusch, D., and O'Hagan, H. (2019). DNA methyltransferase inhibition reduces inflammation-induced colon tumorigenesis. Epigenetics 14, 1209–1223. doi: 10.1080/15592294.2019.1634986
Mayer, E. A. (2011). Gut feelings: the emerging biology of gut-brain communication. Nat. Rev. Neurosci. 12, 453–466. doi: 10.1038/nrn3071
Miossec, P., Korn, T., and Kuchroo, V. K. (2009). Interleukin-17 and type 17 helper T cells. N. Engl. J. Med. 361, 888–898. doi: 10.1056/NEJMra0707449
Moreira, C., Russell, R., Mishra, A., Narayanan, S., Ritchie, J., Waldor, M., et al. (2016). Bacterial adrenergic sensors regulate virulence of enteric pathogens in the gut. MBio 7, e00826–e00916. doi: 10.1128/mBio.00826-16
Morelli, L., and Capurso, L. (2012). FAO/WHO guidelines on probiotics: 10 years later. J. Clin. Gastroenterol. 46, S1–S2. doi: 10.1097/MCG.0b013e318269fdd5
Nadeem Aslam, M., Bassis, C., Zhang, L., Zaidi, S., Varani, J., and Bergin, I. L. (2016). Calcium reduces liver injury in mice on a high-fat diet: alterations in microbial and bile acid profiles. PLoS One 11:e0166178. doi: 10.1371/journal.pone.0166178
Nagpal, R., Newman, T., Wang, S., Jain, S., Lovato, J., and Yadav, H. (2018). Obesity-linked gut microbiome dysbiosis associated with derangements in gut permeability and intestinal cellular homeostasis independent of diet. J. Diabetes Res. 2018:3462092. doi: 10.1155/2018/3462092
Narva, M., Nevala, R., Poussa, T., and Korpela, R. (2004). The effect of Lactobacillus helveticus fermented milk on acute changes in calcium metabolism in postmenopausal women. Eur. J. Nutr. 43, 61–68. doi: 10.1007/s00394-004-0441-y
Neis, E. P., Dejong, C. H., and Rensen, S. S. (2015). The role of microbial amino acid metabolism in host metabolism. Nutrients 7, 2930–2946. doi: 10.3390/nu7042930
Novince, C., Whittow, C., Aartun, J., Hathaway, J., Poulides, N., Chavez, M., et al. (2017). Commensal gut microbiota immunomodulatory actions in bone marrow and liver have catabolic effects on skeletal homeostasis in health. Sci. Rep. 7:5747. doi: 10.1038/s41598-017-06126-x
Ohlsson, C., Engdahl, C., Fåk, F., Andersson, A., Windahl, S. H., Farman, H. H., et al. (2014). Probiotics protect mice from ovariectomy-induced cortical bone loss. PLoS One 9:e92368. doi: 10.1371/journal.pone.0092368
Ohlsson, C., Nigro, G., Boneca, I., Bäckhed, F., Sansonetti, P., and Sjögren, K. (2017). Regulation of bone mass by the gut microbiota is dependent on NOD1 and NOD2 signaling. Cell. Immunol. 317, 55–58. doi: 10.1016/j.cellimm.2017.05.003
Ohta, A., Motohashi, Y., Sakai, K., Hirayama, M., Adachi, T., and Sakuma, K. (1998). Dietary fructooligosaccharides increase calcium absorption and levels of mucosal calbindin-D9k in the large intestine of gastrectomized rats. Scand. J. Gastroenterol. 33, 1062–1068. doi: 10.1080/003655298750026769
Ooi, J., Li, Y., Rogers, C., and Cantorna, M. T. (2013). Vitamin D regulates the gut microbiome and protects mice from dextran sodium sulfate-induced colitis. J. Nutr. 143, 1679–1686. doi: 10.3945/jn.113.180794
Pacifici, R. (2012). Role of T cells in ovariectomy induced bone loss--revisited. J. Bone Miner. Res. 27, 231–239. doi: 10.1002/jbmr.1500
Papageorgiou, M., and Biver, E. (2021). Interactions of the microbiome with pharmacological and non-pharmacological approaches for the management of ageing-related musculoskeletal diseases. Ther. Adv. Musculoskelet. Dis. 13:1759720x211009018. doi: 10.1177/1759720X211009018
Park, K., Kim, E., Hong, J., and Yun, H. J. T. (2018). Dysregulation of 5-hydroxytryptamine 6 receptor accelerates maturation of bone-resorbing osteoclasts and induces bone loss. Theranostics 8, 3087–3098. doi: 10.7150/thno.24426
Parvaneh, K., Ebrahimi, M., Sabran, M., Karimi, G., Hwei, A., Abdul-Majeed, S., et al. (2015). Probiotics (Bifidobacterium longum) increase bone mass density and upregulate Sparc and Bmp-2 genes in rats with bone loss resulting from ovariectomy. Biomed. Res. Int. 2015:897639. doi: 10.1155/2015/897639
Paul, A. K., Paul, A., Jahan, R., Jannat, K., Bondhon, T. A., Hasan, A., et al. (2021). Probiotics and amelioration of rheumatoid arthritis: significant roles of Lactobacillus casei and lactobacillus acidophilus. Microorganisms 9:1070. doi: 10.3390/microorganisms9051070
Pawlak, D., Domaniewski, T., Znorko, B., Oksztulska-Kolanek, E., Lipowicz, P., Doroszko, M., et al. (2017). The impact of peripheral serotonin on leptin-brain serotonin axis, bone metabolism and strength in growing rats with experimental chronic kidney disease. Bone 105, 1–10. doi: 10.1016/j.bone.2017.08.004
Perez-Lopez, A., Behnsen, J., Nuccio, S., and Raffatellu, M. (2016). Mucosal immunity to pathogenic intestinal bacteria. Nat. Rev. Immunol. 16, 135–148. doi: 10.1038/nri.2015.17
Pisani, P., Renna, M., Conversano, F., Casciaro, E., Di Paola, M., Quarta, E., et al. (2016). Major osteoporotic fragility fractures: risk factor updates and societal impact. World J. Orthop. 7, 171–181. doi: 10.5312/wjo.v7.i3.171
Poundarik, A., Diab, T., Sroga, G., Ural, A., Boskey, A., Gundberg, C., et al. (2012). Dilatational band formation in bone. Proc. Natl. Acad. Sci. U. S. A. 109, 19178–19183. doi: 10.1073/pnas.1201513109
Rahman, M. M., Kukita, A., Kukita, T., Shobuike, T., Nakamura, T., and Kohashi, O. (2003). Two histone deacetylase inhibitors, trichostatin A and sodium butyrate, suppress differentiation into osteoclasts but not into macrophages. Blood 101, 3451–3459. doi: 10.1182/blood-2002-08-2622
Raschka, L., and Daniel, H. (2005). Mechanisms underlying the effects of inulin-type fructans on calcium absorption in the large intestine of rats. Bone 37, 728–735. doi: 10.1016/j.bone.2005.05.015
Rath, C. M., and Dorrestein, P. C. (2012). The bacterial chemical repertoire mediates metabolic exchange within gut microbiomes. Curr. Opin. Microbiol. 15, 147–154. doi: 10.1016/j.mib.2011.12.009
Raveschot, C., Coutte, F., Frémont, M., Vaeremans, M., Dugersuren, J., Demberel, S., et al. (2020). Probiotic lactobacillus strains from Mongolia improve calcium transport and uptake by intestinal cells in vitro. Food Res. Int. 133:109201. doi: 10.1016/j.foodres.2020.109201
Reigstad, C., Salmonson, C., Rainey, J., Szurszewski, J., Linden, D., Sonnenburg, J., et al. (2015). Gut microbes promote colonic serotonin production through an effect of short-chain fatty acids on enterochromaffin cells. FASEB J. 29, 1395–1403. doi: 10.1096/fj.14-259598
Rizzoli, R., Biver, E., Bonjour, J. P., Coxam, V., Goltzman, D., Kanis, J. A., et al. (2018). Benefits and safety of dietary protein for bone health-an expert consensus paper endorsed by the European Society for Clinical and Economical Aspects of Osteopororosis, osteoarthritis, and musculoskeletal diseases and by the international osteoporosis foundation. Osteoporos. Int. 29, 1933–1948. doi: 10.1007/s00198-018-4534-5
Rothhammer, V., Mascanfroni, I., Bunse, L., Takenaka, M., Kenison, J., Mayo, L., et al. (2016). Type I interferons and microbial metabolites of tryptophan modulate astrocyte activity and central nervous system inflammation via the aryl hydrocarbon receptor. Nat. Med. 22, 586–597. doi: 10.1038/nm.4106
Schepper, J., Collins, F., Rios-Arce, N., Kang, H., Schaefer, L., Gardinier, J., et al. (2020). Involvement of the gut microbiota and barrier function in glucocorticoid-induced osteoporosis. J. Bone Miner. Res. 35, 801–820. doi: 10.1002/jbmr.3947
Schepper, J., Collins, F., Rios-Arce, N., Raehtz, S., Schaefer, L., Gardinier, J., et al. (2019). Probiotic Lactobacillus reuteri prevents postantibiotic bone loss by reducing intestinal dysbiosis and preventing barrier disruption. J. Bone Miner. Res. 34, 681–698. doi: 10.1002/jbmr.3635
Scholz-Ahrens, K., Ade, P., Marten, B., Weber, P., Timm, W., Açil, Y., et al. (2007). Prebiotics, probiotics, and synbiotics affect mineral absorption, bone mineral content, and bone structure. J. Nutr. 137, 838S–846S. doi: 10.1093/jn/137.3.838S
Schott, E., Farnsworth, C., Grier, A., Lillis, J., Soniwala, S., Dadourian, G., et al. (2018). Targeting the gut microbiome to treat the osteoarthritis of obesity. JCI Insight 3:e95997. doi: 10.1172/jci.insight.95997
Schroeder, B., and Bäckhed, F. (2016). Signals from the gut microbiota to distant organs in physiology and disease. Nat. Med. 22, 1079–1089. doi: 10.1038/nm.4185
Schwarzer, M., Makki, K., Storelli, G., Machuca-Gayet, I., Srutkova, D., Hermanova, P., et al. (2016). Lactobacillus plantarum strain maintains growth of infant mice during chronic undernutrition. Science 351, 854–857. doi: 10.1126/science.aad8588
Sender, R., Fuchs, S., and Milo, R. J. C. (2016). Are we really vastly outnumbered? Revisiting the ratio of bacterial to host cells in humans. Cell 164, 337–340. doi: 10.1016/j.cell.2016.01.013
Sepich-Poore, G., Zitvogel, L., Straussman, R., Hasty, J., Wargo, J., and Knight, R. (2021). The microbiome and human cancer. Science 371:eabc4552. doi: 10.1126/science.abc4552
Shen, S., Prame Kumar, K., Wen, S., Shim, R., Wanrooy, B., Stanley, D., et al. (2021). Deficiency of dietary fiber modulates gut microbiota composition, neutrophil recruitment and worsens experimental colitis. Front. Immunol. 12:619366. doi: 10.3389/fimmu.2021.619366
Sjögren, K., Engdahl, C., Henning, P., Lerner, U., Tremaroli, V., Lagerquist, M., et al. (2012). The gut microbiota regulates bone mass in mice. J. Bone Miner. Res. 27, 1357–1367. doi: 10.1002/jbmr.1588
So, J., Song, M., Kwon, H., Lee, C., Chae, C., Sahoo, A., et al. (2011). Lactobacillus casei enhances type II collagen/glucosamine-mediated suppression of inflammatory responses in experimental osteoarthritis. Life Sci. 88, 358–366. doi: 10.1016/j.lfs.2010.12.013
Stone, K., Lui, L., Christen, W., Troen, A., Bauer, D., Kado, D., et al. (2017). Effect of combination folic acid, vitamin B, and vitamin B supplementation on fracture risk in women: a randomized, controlled trial. J. Bone Miner. Res. 32, 2331–2338. doi: 10.1002/jbmr.3229
Suerbaum, S., and Michetti, P. (2002). Helicobacter pylori infection. Dtsch. Arztebl. Int. 347, 1175–1186. doi: 10.1056/NEJMra020542
Sugita, S., Kawazoe, Y., Imai, A., Yamada, Y., Horie, S., and Mochizuki, M. (2012). Inhibition of Th17 differentiation by anti-TNF-alpha therapy in uveitis patients with Behçet's disease. Arthritis Res. Ther. 14:R99. doi: 10.1186/ar3824
Tajik, N., Frech, M., Schulz, O., Schälter, F., Lucas, S., Azizov, V., et al. (2020). Targeting zonulin and intestinal epithelial barrier function to prevent onset of arthritis. Nat. Commun. 11:1995. doi: 10.1038/s41467-020-15831-7
Takimoto, T., Hatanaka, M., Hoshino, T., Takara, T., Tanaka, K., Shimizu, A., et al. (2018). Effect of Bacillus subtilis C-3102 on bone mineral density in healthy postmenopausal Japanese women: a randomized, placebo-controlled, double-blind clinical trial. Biosci. Microbiota Food Health 37, 87–96. doi: 10.12938/bmfh.18-006
Thaiss, C., Levy, M., Grosheva, I., Zheng, D., Soffer, E., Blacher, E., et al. (2018). Hyperglycemia drives intestinal barrier dysfunction and risk for enteric infection. Science 359, 1376–1383. doi: 10.1126/science.aar3318
Tu, K. N., Lie, J. D., Wan, C. K. V., Cameron, M., Austel, A. G., Nguyen, J. K., et al. (2018). Osteoporosis: a review of treatment options. P T 43, 92–104.
Turner, J. R. (2009). Intestinal mucosal barrier function in health and disease. Nat. Rev. Immunol. 9, 799–809. doi: 10.1038/nri2653
Tyagi, A., Yu, M., Darby, T., Vaccaro, C., Li, J., Owens, J., et al. (2018). The microbial metabolite butyrate stimulates bone formation via T regulatory cell-mediated regulation of WNT10B expression. Immunity 49, 1116.e1117–1131.e1117. doi: 10.1016/j.immuni.2018.10.013
Uluçkan, Ö., Jimenez, M., Karbach, S., Jeschke, A., Graña, O., Keller, J., et al. (2016). Chronic skin inflammation leads to bone loss by IL-17-mediated inhibition of Wnt signaling in osteoblasts. Sci. Transl. Med. 8:330ra337. doi: 10.1126/scitranslmed.aad8996
Vaghef-Mehrabany, E., Alipour, B., Homayouni-Rad, A., Sharif, S., Asghari-Jafarabadi, M., and Zavvari, S. (2014). Probiotic supplementation improves inflammatory status in patients with rheumatoid arthritis. Nutrition 30, 430–435. doi: 10.1016/j.nut.2013.09.007
Valdes, A. M., Walter, J., Segal, E., and Spector, T. D. (2018). Role of the gut microbiota in nutrition and health. BMJ 361:k2179. doi: 10.1136/bmj.k2179
van den Heuvel, E. G., Schoterman, M. H., and Muijs, T. (2000). Transgalactooligosaccharides stimulate calcium absorption in postmenopausal women. J. Nutr. 130, 2938–2942. doi: 10.1093/jn/130.12.2938
Vich Vila, A., Collij, V., Sanna, S., Sinha, T., Imhann, F., Bourgonje, A. R., et al. (2020). Impact of commonly used drugs on the composition and metabolic function of the gut microbiota. Nat. Commun. 11:362. doi: 10.1038/s41467-019-14177-z
von Mutius, E., and Vercelli, D. (2019). Author correction: farm living: effects on childhood asthma and allergy. Nat. Rev. Immunol. 19:594. doi: 10.1038/s41577-019-0199-3
Vyas, U., and Ranganathan, N. (2012). Probiotics, prebiotics, and synbiotics: gut and beyond. Gastroenterol. Res. Pract. 2012:872716. doi: 10.1155/2012/872716
Wang, Z., Klipfell, E., Bennett, B. J., Koeth, R., Levison, B. S., Dugar, B., et al. (2011). Gut flora metabolism of phosphatidylcholine promotes cardiovascular disease. Nature 472, 57–63. doi: 10.1038/nature09922
Wang, F., and Roy, S. (2017). Gut homeostasis, microbial dysbiosis, and opioids. Toxicol. Pathol. 45, 150–156. doi: 10.1177/0192623316679898
Weaver, C. M. (2015). Diet, gut microbiome, and bone health. Curr. Osteoporos. Rep. 13, 125–130. doi: 10.1007/s11914-015-0257-0
Weaver, C., Martin, B., Nakatsu, C., Armstrong, A., Clavijo, A., McCabe, L., et al. (2011). Galactooligosaccharides improve mineral absorption and bone properties in growing rats through gut fermentation. J. Agric. Food Chem. 59, 6501–6510. doi: 10.1021/jf2009777
Wong, J., de Souza, R., Kendall, C., Emam, A., and Jenkins, D. J. (2006). Colonic health: fermentation and short chain fatty acids. J. Clin. Gastroenterol. 40, 235–243. doi: 10.1097/00004836-200603000-00015
Xia, W., Cooper, C., Li, M., Xu, L., Rizzoli, R., Zhu, M., et al. (2019). East meets west: current practices and policies in the management of musculoskeletal aging. Aging Clin. Exp. Res. 31, 1351–1373. doi: 10.1007/s40520-019-01282-8
Xu, Z., Xie, Z., Sun, J., Huang, S., Chen, Y., Li, C., et al. (2020). Gut microbiome reveals specific dysbiosis in primary osteoporosis. Front. Cell. Infect. Microbiol. 10:160. doi: 10.3389/fcimb.2020.00160
Yan, J., Herzog, J., Tsang, K., Brennan, C., Bower, M., Garrett, W., et al. (2016). Gut microbiota induce IGF-1 and promote bone formation and growth. Proc. Natl. Acad. Sci. U. S. A. 113, E7554–E7563. doi: 10.1073/pnas.1607235113
Yoshimura, N., Muraki, S., Oka, H., Tanaka, S., Kawaguchi, H., Nakamura, K., et al. (2012). Accumulation of metabolic risk factors such as overweight, hypertension, dyslipidaemia, and impaired glucose tolerance raises the risk of occurrence and progression of knee osteoarthritis: a 3-year follow-up of the ROAD study. Osteoarthr. Cartil. 20, 1217–1226. doi: 10.1016/j.joca.2012.06.006
Yu, M., Jia, H., Zhou, C., Yang, Y., Zhao, Y., Yang, M., et al. (2017). Variations in gut microbiota and fecal metabolic phenotype associated with depression by 16S rRNA gene sequencing and LC/MS-based metabolomics. J. Pharm. Biomed. Anal. 138, 231–239. doi: 10.1016/j.jpba.2017.02.008
Yu, M., Pal, S., Paterson, C. W., Li, J. Y., Tyagi, A. M., Adams, J., et al. (2021). Ovariectomy induces bone loss via microbial-dependent trafficking of intestinal TNF+ T cells and Th17 cells. J. Clin. Invest. 131:e143137. doi: 10.1172/JCI143137
Zaiss, M. M., Axmann, R., Zwerina, J., Polzer, K., Gückel, E., Skapenko, A., et al. (2007). Treg cells suppress osteoclast formation: a new link between the immune system and bone. Arthritis Rheum. 56, 4104–4112. doi: 10.1002/art.23138
Zaiss, M. M., Sarter, K., Hess, A., Engelke, K., Böhm, C., Nimmerjahn, F., et al. (2010). Increased bone density and resistance to ovariectomy-induced bone loss in FoxP3-transgenic mice based on impaired osteoclast differentiation. Arthritis Rheum. 62, 2328–2338. doi: 10.1002/art.27535
Zhu, K., Greenfield, H., Zhang, Q., Du, X., Ma, G., Foo, L., et al. (2008). Growth and bone mineral accretion during puberty in Chinese girls: a five-year longitudinal study. J. Bone Miner. Res. 23, 167–172. doi: 10.1359/jbmr.071006
Zmora, N., Suez, J., and Elinav, E. (2019). You are what you eat: diet, health and the gut microbiota. Nat. Rev. Gastroenterol. Hepatol. 16, 35–56. doi: 10.1038/s41575-018-0061-2
Keywords: gut microbiota, bone diseases, osteoporosis, osteoarthritis, rheumatoid arthritis, bone tumor
Citation: Chen Y, Wang X, Zhang C, Liu Z, Li C and Ren Z (2022) Gut Microbiota and Bone Diseases: A Growing Partnership. Front. Microbiol. 13:877776. doi: 10.3389/fmicb.2022.877776
Received: 04 March 2022; Accepted: 19 April 2022;
Published: 06 May 2022.
Edited by:
Eugenia Bezirtzoglou, Democritus University of Thrace, GreeceReviewed by:
Zan Huang, Nanjing Agricultural University, ChinaCopyright © 2022 Chen, Wang, Zhang, Liu, Li and Ren. This is an open-access article distributed under the terms of the Creative Commons Attribution License (CC BY). The use, distribution or reproduction in other forums is permitted, provided the original author(s) and the copyright owner(s) are credited and that the original publication in this journal is cited, in accordance with accepted academic practice. No use, distribution or reproduction is permitted which does not comply with these terms.
*Correspondence: Zhigang Ren, ZmNjcmVuemdAenp1LmVkdS5jbg==
Disclaimer: All claims expressed in this article are solely those of the authors and do not necessarily represent those of their affiliated organizations, or those of the publisher, the editors and the reviewers. Any product that may be evaluated in this article or claim that may be made by its manufacturer is not guaranteed or endorsed by the publisher.
Research integrity at Frontiers
Learn more about the work of our research integrity team to safeguard the quality of each article we publish.