- 1State Key Laboratory of Microbial Resources, Institute of Microbiology, Chinese Academy of Sciences, Beijing, China
- 2College of Life Science, University of Chinese Academy of Sciences, Beijing, China
- 3CAS Key Laboratory of Bio-based Materials, Qingdao Institute of Bioenergy and Bioprocess Technology, Chinese Academy of Sciences, Qingdao, China
- 4College of Environment, Hohai University, Nanjing, China
- 5School of Life Sciences, Hebei University, Baoding, China
Thioalkalivibrio versutus D301 has been widely used in the biodesulfurization process, as it is capable of oxidizing hydrogen sulfide to elemental sulfur under strongly halo-alkaline conditions. Glycine betaine contributes to the increased tolerance to extreme environments in some of Thioalkalivibrio species. However, the biosynthetic pathway of glycine betaine in Thioalkalivibrio remained unknown. Here, we found that genes associated with nitrogen metabolism of T. versutus D301 were significantly upregulated under high-salt conditions, causing the enhanced production of glycine betaine that functions as a main compatible solute in response to the salinity stress. Glycine betaine was synthesized by glycine methylation pathway in T. versutus D301, with glycine N-methyltransferase (GMT) and sarcosine dimethylglycine N-methyltransferase (SDMT) as key enzymes in this pathway. Moreover, substrate specificities of GMT and SDMT were quite different from the well characterized enzymes for glycine methylation in halophilic Halorhodospira halochloris. Our results illustrate the glycine betaine biosynthetic pathway in the genus of Thioalkalivibrio for the first time, providing us with a better understanding of the biosynthesis of glycine betaine in haloalkaliphilic Thioalkalivibrio.
Introduction
Microbes face many challenges in halo-alkaline environments, which impose high extracellular osmotic pressures on microbial cells. To avoid the outflow of intracellular water and maintain the functions of biomacromolecules, microbes mainly adopt “salt-in” and “compatible solute” strategies to balance the intra- and extracellular osmotic pressures (Gunde-Cimerman et al., 2018). The “salt-in” strategy used by many halophilic archaea and a few halophilic bacteria is to increase the intracellular osmotic pressure by accumulating high concentrations of inorganic salts (mainly KCl) (Christian and Waltho, 1962; Ginzburg et al., 1970; Eisenberg and Wachtel, 1987; Gunde-Cimerman et al., 2018). Microorganisms that use salt-in strategy usually have an acidic proteome to adapt to the intracellular high-salt content, which is necessary for acidic proteins to maintain their structural stabilities and functional activities (Reistad, 1970; Lanyi, 1974; Dennis and Shimmin, 1997). Therefore, microorganisms utilizing “salt-in” strategy are highly dependent on high-salt environments and generally unable to survive under low-salt conditions. Microorganisms that use “compatible solute” strategy can synthesize or import small organic molecules called compatible solutes to maintain the osmotic balance of cells (Kempf and Bremer, 1998). The compatible solutes mainly include sugars, polyols, amino acids, and their derivatives (Galinski and Truper, 1994; Roberts, 2005). The accumulation of compatible solutes will increase the intracellular osmolarity without interfering with the normal cellular activities (Brown, 1976). Given microorganisms using compatible solutes can better adapt to environmental fluctuations than those using “salt-in” strategy, “compatible solute” strategy is more widely adopted by microbes inhabiting halo-alkaline environments.
Haloalkaliphilic Thioalkalivibrio species are a class of obligate chemoautotrophs using reduced sulfur compound as energy source and carbon dioxide (CO2) as carbon source (Sorokin et al., 2001). They live in environments of different pHs and salinities, ranging from 7.5 to 10.5 and 0.3 to 4.0 M Na+, respectively (Sorokin et al., 2001). Members of Thioalkalivibrio genus mainly employ “compatible solute” strategy to balance the intra- and extracellular osmotic pressures and then survive in high-salt environments (Banciu et al., 2004a,b, 2005). N-containing glycine betaine was detected in Thioalkalivibrio halophilus when grown in 4 M NaCl and 4 M soda media, with osmotic pressures of 9.3 and 5 osm/kg, respectively (Banciu et al., 2004a). The extracellular osmotic pressure but not just the concentration of Na+ determines the biosynthesis of glycine betaine, given that a higher osmotic pressure contributes to a higher level of glycine betaine. Besides, a osmolarity-dependent production of glycine betaine was also observed in Thioalkalivibrio versutus ALJ 15 (Banciu et al., 2005). In addition, glycine betaine confers resistance to the low-temperature pressure in two moderately halophilic Thioalkalivibrio strains (Ahn et al., 2021). Though glycine betaine is of great importance for Thioalkalivibrio to keep them alive in extreme environments, the biosynthetic pathway of glycine betaine is still unclear in Thioalkalivibrio species.
The choline oxidation pathway and glycine methylation pathway are two pathways that have already been known for the biosynthesis of glycine betaine (Figure 1). In the choline oxidation pathway, choline is converted to glycine betaine by choline dehydrogenase and betaine-aldehyde dehydrogenase, with betaine-aldehyde as the intermediate (Landfald and Strom, 1986; Boyd et al., 1991). In addition, choline oxidase alone is also able to convert choline to glycine betaine (Fan et al., 2004). In the glycine methylation pathway, S-adenosyl-L-methionine (SAM)-dependent N-methyltransferases catalyze the conversion of glycine to glycine betaine by the addition of three methyl groups to its amino group, with sarcosine and dimethylglycine as the intermediates. The substrate specificities of SAM-dependent N-methyltransferases that catalyze the conversion of glycine to glycine betaine vary from organism to organism. A single glycine sarcosine dimethylglycine N-methyltransferase (TpGSDMT) can complete the whole conversion process in Thalassiosira pseudonana (Kageyama et al., 2018). More commonly, two enzymes are involved in the three methylation reactions in halophiles, such as glycine sarcosine N-methyltransferase (GSMT)/sarcosine dimethylglycine N-methyltransferase (SDMT) in Halorhodospira halochloris (formerly Ectothiorhodospira halochloris) and Methanohalophilus portucalensis (Nyyssola et al., 2000, 2001; Lai and Lai, 2011), and glycine sarcosine N-methyltransferase (GSMT)/dimethylglycine N-methyltransferase (DMT) in Aphanothece halophytica and Synechococcus sp. (Waditee et al., 2003; Lu et al., 2006).
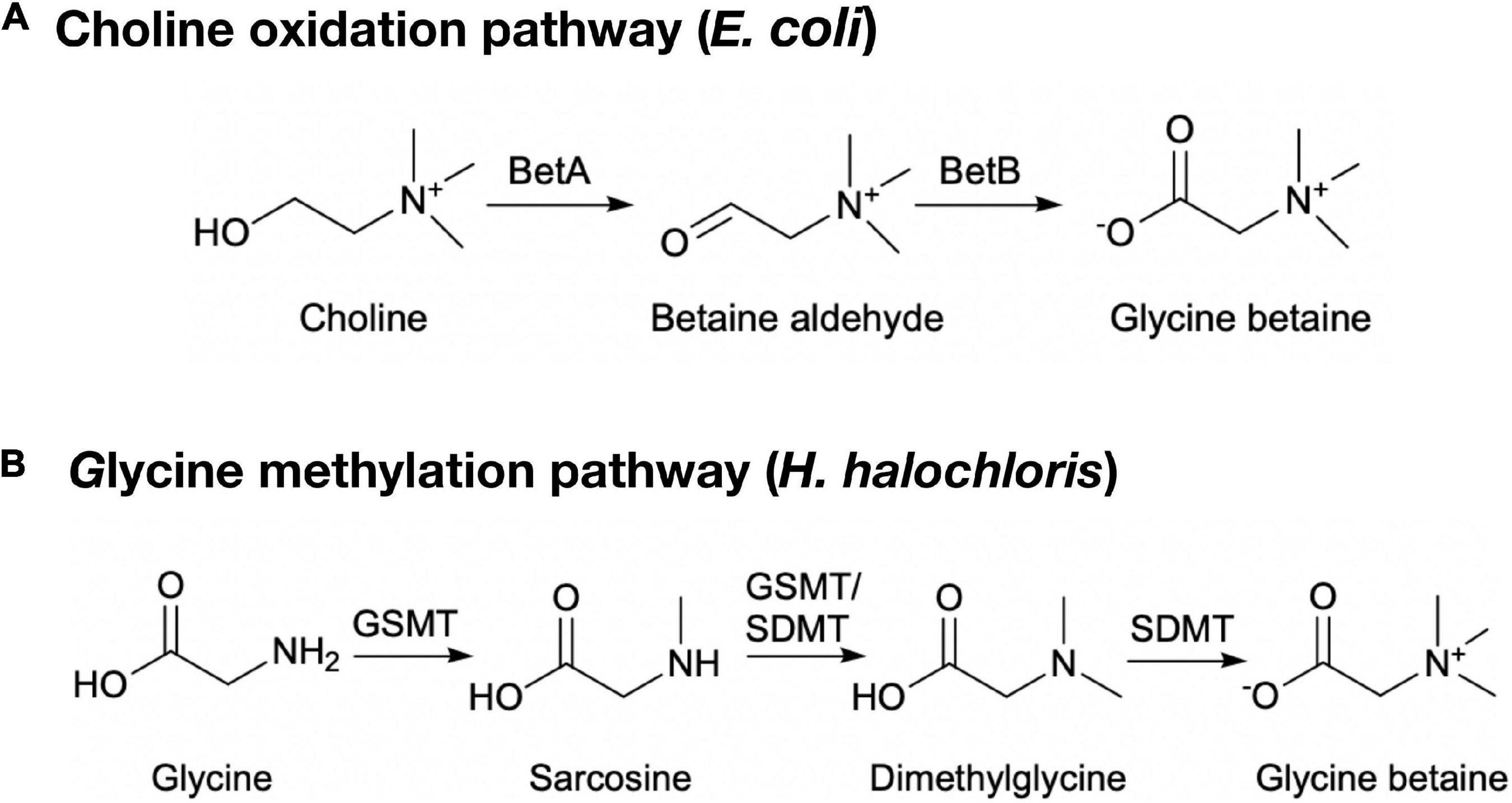
Figure 1. Glycine betaine biosynthetic pathways in microorganisms. (A) The choline oxidation pathway in E. coli. BetA, choline dehydrogenase; BetB, betaine-aldehyde dehydrogenase. (B) The glycine methylation pathway in H. halochloris. GSMT, glycine sarcosine N-methyltransferase; SDMT, sarcosine dimethylglycine N-methyltransferase.
Thioalkalivibrio versutus D301, a strain widely used in biodesulfurization industry, contains a 2,969,361-bp circular chromosome (Mu et al., 2016, 2021). The genes coding for choline oxidation pathway are absent in the genome of T. versutus D301, but a set of chromosomal genes (TVD_RS00875 and TVD_RS00880) homologous to the GSMT and SDMT genes from H. halochloris are available. Though Thioalkalivibrio has a close phylogenetic relationship to Halorhodospira, it is still unclear whether SAM-dependent N-methyltransferases from Thioalkalivibrio species also catalyze the conversion of glycine to glycine betaine (Nyyssola et al., 2001; Sorokin et al., 2001). So far, no study has been carried out to characterize the glycine methylation pathway of Thioalkalivibrio species. Here, we show that N-containing glycine betaine is a main compatible solute in Thioalkalivibrio versutus. We also show that the glycine N-methyltransferase (TvGMT) and sarcosine dimethylglycine N-methyltransferase (TvSDMT) are responsible for the conversion of glycine to glycine betaine by adding three methyl groups to the amino group of glycine.
Materials and Methods
Bacterial Strains and Growth Conditions
Thioalkalivibrio versutus D301 was grown aerobically on a slightly modified TD medium supplemented with 10 g/L NaHCO3 (Low-salt medium, 0.4 M Na+) or 10 g/L NaHCO3 plus 152 g/L NaCl (High-salt medium, 3.0 M Na+) at 30°C and 200 rpm (Mu et al., 2016, 2017). The pH of the modified TD media was adjusted to 9.5 with 2 M hydrochloric acid. E. coli BL21(DE3) grown in Luria-Bertani (LB) medium was used to overexpress the target enzymes of interest. Cultures of E. coli growing at 37° and 200 rpm were switched to incubation at 30° and 160 rpm for overexpression of proteins. When appropriate, E. coli cultures were supplemented with kanamycin at 50 μg/mL.
Transcriptome Sequencing and Functional Enrichment Analysis of Differentially Expressed Genes
Thioalkalivibrio versutus cultures grown under low-salt and high-salt conditions were collected at the late exponential growth phase for transcriptome sequencing, which was carried out by Majorbio Bio-Pharm Technology Co., Ltd. (Shanghai, China). The transcriptome sequencing data were deposited in NCBI1 with BioProjects accession number PRJNA812740. After the quality control of raw reads was completed, clean reads were obtained and then mapped to the reference genome of T. versutus D301 (CP011367) using Bowtie 2 (version 2.3.5) (Mu et al., 2016). The fragments per kilobase of transcript per million mapped reads (FPKM) calculated by RSEM (version 1.3.1) was used to represent the expression level of genes under low-salt and high-salt conditions. Differentially expressed genes (DEGS) of T. versutus D301 were identified using DESeq2 (version 1.24.0) based on the value of | log2 fold change| > 2 and an adjusted P < 0.05. The Gene Ontology (GO) functional enrichment analysis of DEGS were performed by Goatools.
LC/MS Analysis of Intracellular Metabolites
For the LC/MS analysis of intracellular metabolites, 50 mL cultures of T. versutus D301 grown under low-salt and high-salt conditions, respectively, were harvested at the late exponential growth phase. Firstly, the cultures were centrifuged at 10,000 rpm for 20 min to collect the pellets, then the cell pellets were washed twice with 0.4 or 3 M NaCl solution, and finally 1 mL ultrapure water was used to resuspend the pellets. The cell lysates were prepared by treating the obtained cell suspensions with four freeze-thaw cycles: froze at −80°C for 15 min and thawed at 65°C for 2 min. Cell lysates were centrifuged at 12,000 rpm for 30 min to collect the supernatants, which were further mixed with acetonitrile in a ratio of 3:7 (v/v). After the mixtures were centrifuged at 12,000 rpm for 10 min, the supernatants filtered with a 0.22 μm nylon filter membrane were analyzed with LC/MS (ESI). Chromatography was performed with an Agilent 1260/6460 LC/Triple Quad MS system, using a TSKgel NH2-100 column (2.0 × 150 mm, 3 μm; TOSOH, Tokyo, Japan) with guard column (2.0 × 10 mm, 3 μm). Mobile phase A was 10 mM ammonium formate supplemented with 0.07% (v/v) formic acid, while mobile phase B was pure acetonitrile. The following method was used with a flow rate of 0.25 ml/min: 85% mobile phase B for 2 min; decrease of 1.5% mobile phase B/min to 55% mobile phase B; holding at 55% mobile phase B for 5 min; increase of 15% mobile phase B/min to 85% mobile phase B; holding at 85% mobile phase B for 15 min. Mass spectra were acquired in positive ionization mode, with a fragmentor of 80 V and a scan range of 70.0–1000.0 m/z. Data analysis was performed using Agilent MassHunter Qualitative Analysis B.04.00 Workstation Software.
Quantification of Glycine Betaine by HPLC
Samples used for quantification of intracellular glycine betaine were prepared using the same method as what mentioned in “LC/MS analysis of intracellular metabolites”. Agilent 1260 Infinity II system equipped with a Inertsil NH2 column (4.6 × 250 mm, 5 μm, GL Sciences, Tokyo, Japan) was used to measure the glycine betaine. Acetonitrile/ultrapure water (70:30, v/v) was used as the mobile phase at a flow rate of 1 mL/min. The detection wavelength of 196 nm was used to measure the glycine betaine. Total protein concentrations were determined using the Bradford method.
Protein Expression and Purification
The TVD_RS00875 and TVD_RS00880 genes, coding for the putative glycine methylation pathway, were inserted into BamHI-digested pET-28a(+), respectively, using the T5 exonuclease-dependent assembly system (Xia et al., 2019). The obtained E. coli strains grown with 50 μg/mL kanamycin were used to overexpress TVD_RS00875 (TvGMT) and TVD_RS00880 (TvSDMT) after addition of 0.05 mM IPTG. Cell extracts were prepared by high pressure homogenization in buffer A (20 mM Tris–HCl, 300 mM NaCl, 20 mM imidazole, 2 mM DTT, pH 7.5). His-tagged proteins were purified by an affinity column packed with Ni Sepharose (Cytiva, Uppsala, Sweden). After the pretreated sample loaded onto the affinity column was washed with 10 column volumes of buffer A and then 5 column volumes of 5% buffer B (20 mM Tris–HCl, 300 mM NaCl, 44 mM imidazole, 2 mM DTT, pH 7.5), TvGMT or TvSDMT was eluted using 30% buffer B (20 mM Tris–HCl, 300 mM NaCl, 164 mM imidazole, 2 mM DTT, pH 7.5). Protein concentrations were determined by Bradford assay using BSA as standard. The purities of TvGMT and TvSDMT were examined based on SDS-PAGE analysis with Coomassie staining.
Methyltransferase Assay
Methyltransferase activity was measured using SAM as the methyl donor and glycine, sarcosine, or dimethylglycine as methyl receptor. The reaction mixture contained 100 mM Tris–HCl (pH 7.5), 12.5 μmol MgCl2, 0.5 mM DTT, 10 mM SAM, 250 mM glycine/30 mM sarcosine (TvGMT) or 60 mM sarcosine/50 mM dimethylglycine (TvSDMT) (Nyyssola et al., 2001). Reactions that initiated by the addition of TvGSMT or TvSDMT were conducted at 37°C for 30 min and then quenched by heating with boiling water for 10 min. The supernatants obtained by centrifugation were collected for HPLC analysis of products. The standard curves used for quantification of products were made with the commercially available sarcosine, dimethylglycine, and glycine betaine, respectively.
Results
Genes Involved in Nitrogen Metabolism Are Significantly Upregulated Under High-Salt Conditions
The comparative transcriptomic analysis was carried out to examine the response of T. versutus to high-salt stress. T. versutus cultures were firstly grown under low-salt and high-salt conditions, and cells were then harvested at the late exponential phase. After the cDNA libraries were constructed, they were sequenced using the Illumina HiSeq platforms. Around 4 Gb clean data for each sample was obtained after the quality control of raw data. The information of transcriptome sequencing data was shown in Table 1. Clean reads of each sample were mapped to the reference genome of T. versutus D301 (CP011367), with alignment rates ranging from 98.77 to 99.25%. The functional information of T. versutus D301 genome was obtained through the annotation of non-redundant protein (NR), Swiss-Prot, Pfam, COG, GO and KEGG Database (Figure 2). The FPKM was used to measure the expression levels of genes or transcripts. A total of 184 differentially expressed genes (DEGs) were obtained between T. versutus cultures grown under high-salt and low-salt conditions, with 90 genes significantly upregulated and 94 genes significantly downregulated (Figure 3A).
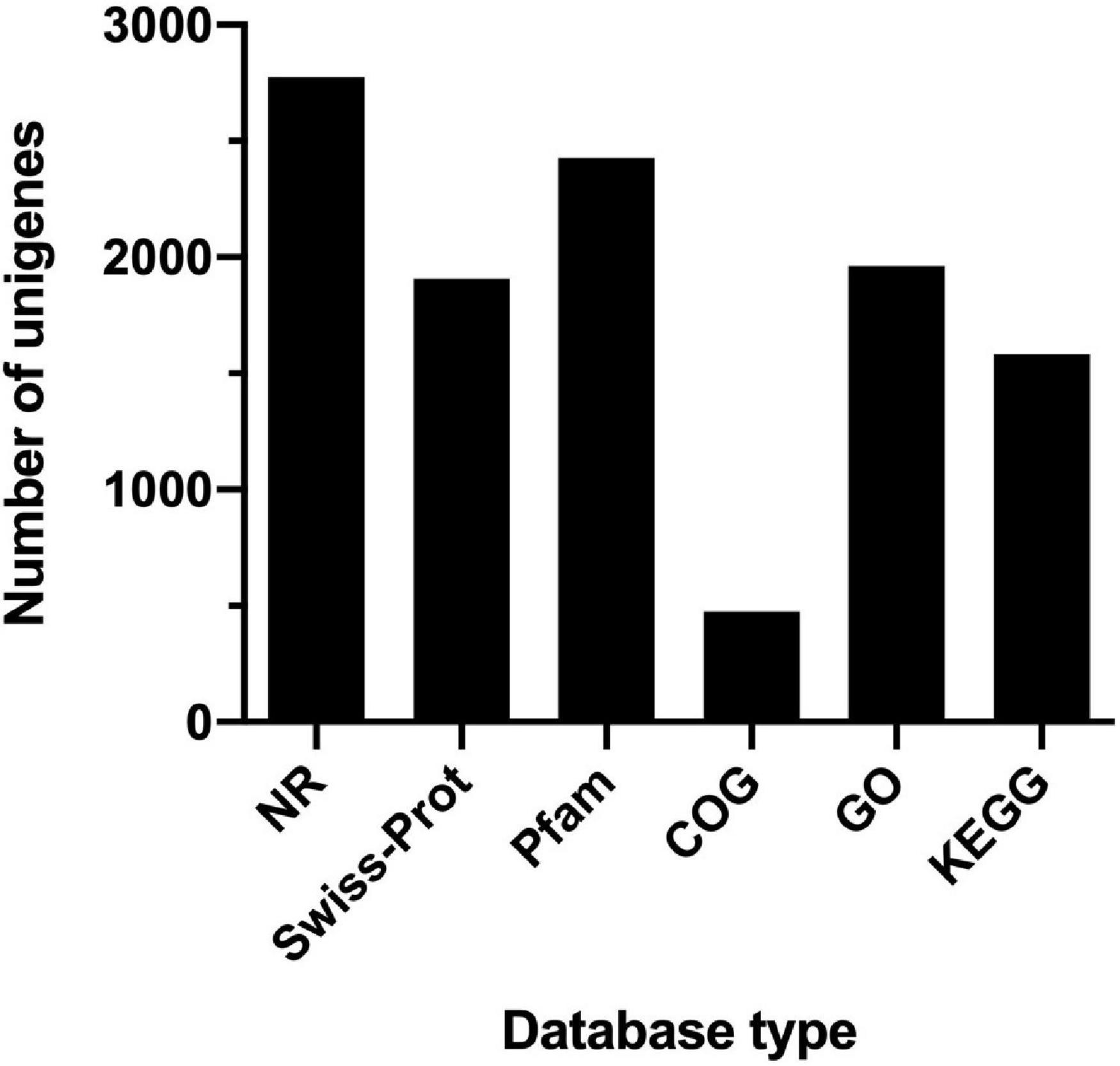
Figure 2. The statistic histogram of basic functional annotation of genes in T. versutus D301. The horizontal axis represents different database used for annotation. The vertical axis represents the number of genes annotated by different database. The total number of coding genes is 2,788 in T. versutus D301.
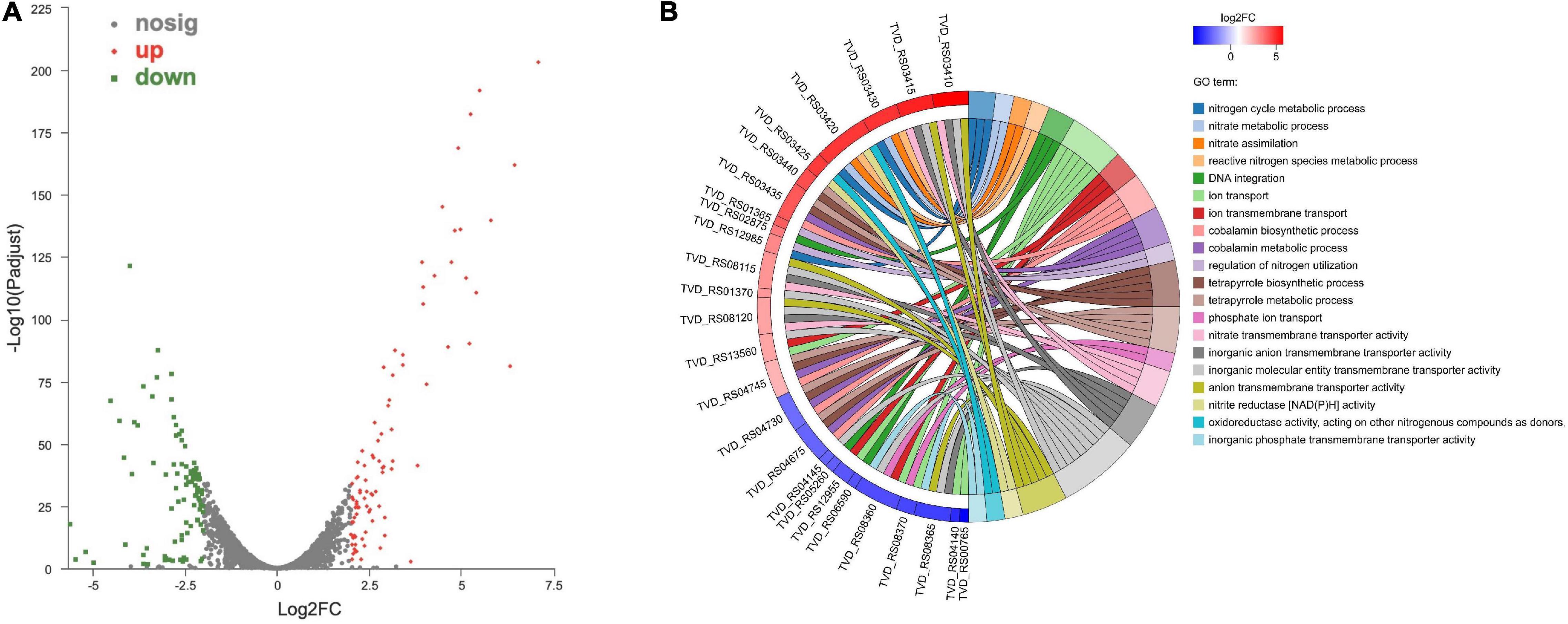
Figure 3. Transcriptomic analysis of differentially expressed genes (DEGs) between high-salt and low-salt conditions. (A) Volcano plot of the DEGs. Red dots indicate significantly upregulated genes, while green squares indicate significantly downregulated genes. The |log2FC| > 2 and an adjusted P < 0.05 was used as threshold values. (B) The top twenty GO enrichment terms from DEGs.
To further understand the physiological response of T. versutus to high-salt stress, we performed GO enrichment analysis of DEGs (Figure 3B). The nitrate transmembrane transporter activity, nitrogen cycle metabolic process, nitrate metabolic process, nitrate assimilation, reactive nitrogen species metabolic process and nitrite reductase [NAD(P)H] activity were among the top 20 GO terms. As shown in Table 2, as much as two-thirds of the top 20 significantly upregulated genes were involved in nitrogen metabolism or related regulation process.
Glycine betaine and ectoine/hydroxyectoine are N-containing compatible solutes commonly found in halophiles. Two candidate genes TVD_RS00875 and TVD_RS00880 probably encoding the enzymes for glycine methylation pathway were found in the genome of T. versutus, with the putative genes coding for choline oxidation pathway and ectoine/hydroxyectoine biosynthetic pathway absent. However, no significant difference was observed in transcriptional levels of TVD_RS00875 and TVD_RS00880 between high-salt and low-salt conditions. The transcriptional levels 6∼14 times higher than the rpoN gene encoding sigma 54.
N-Containing Glycine Betaine Is a Main Compatible Solute in Thioalkalivibrio versutus D301
To confirm that the glycine betaine was responsible for the high-salt tolerance in T. versutus D301, we measured the glycine betaine in T. versutus D301 grown in high-salt (3.0 M Na+) and low-salt (0.4 M Na+) media, respectively. Intracellular metabolites of T. versutus D301 were firstly analyzed qualitatively by LC/MS. T. versutus D301 grown under high-salt conditions produced a compound with an m/z ratio of 118 and a liquid chromatography retention time corresponding to glycine betaine (Figure 4A). The glycine betaine contents were then determined quantitatively by HPLC. T. versutus D301 produced much more glycine betaine under high-salt conditions (8.1 μmol/mg total protein) compared to the production under low-salt conditions (0.2 μmol/mg total protein), demonstrating that glycine betaine is indeed a compatible solute to resist the high-salt stress in T. versutus D301 (Figure 4B). However, in this range of salt concentrations, the contents of glycine betaine increases were not linearly with an increase in salt concentration.
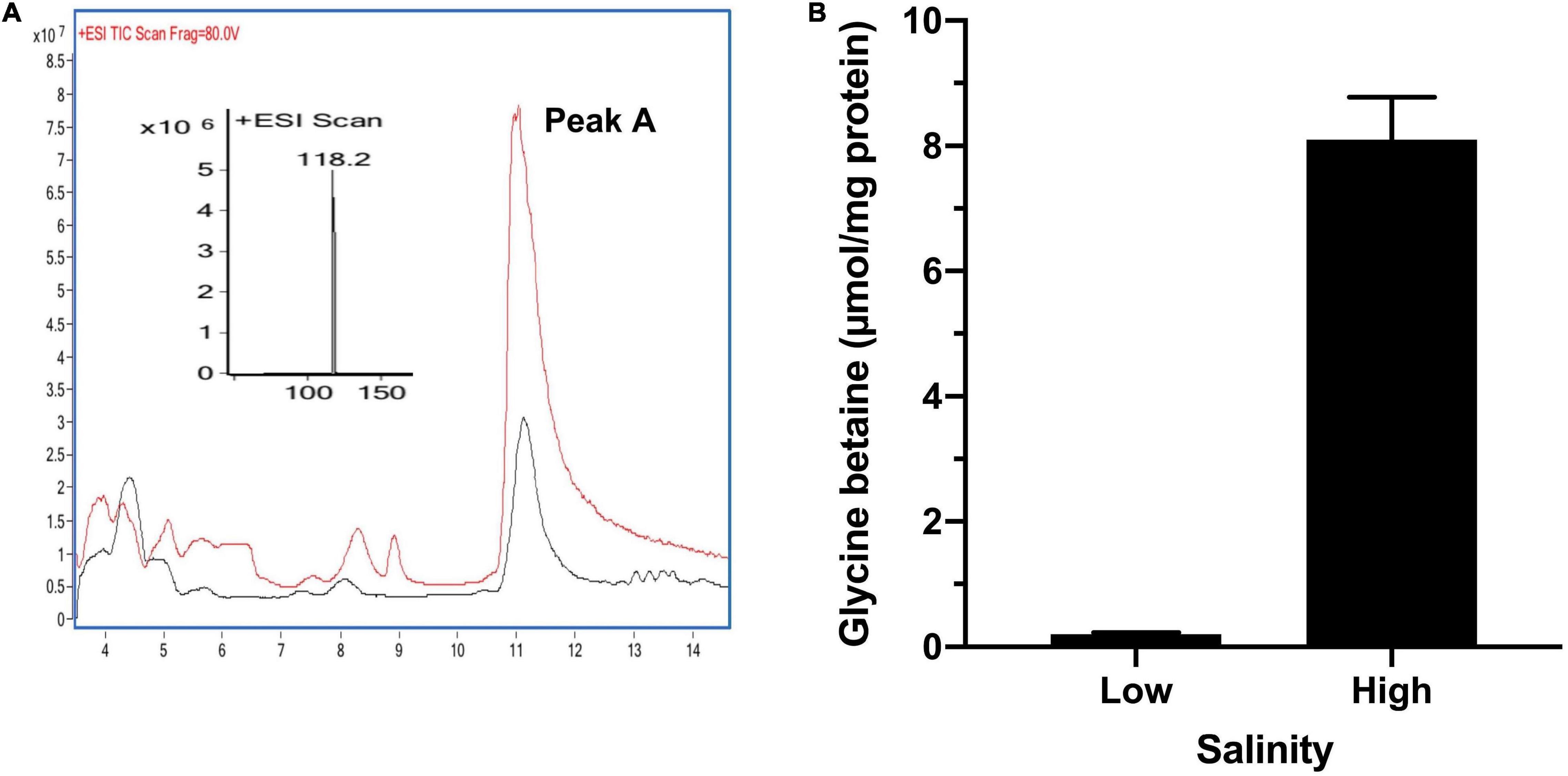
Figure 4. Qualitative and quantitative analysis of glycine betaine in T. versutus D301 grown under high-salt (3.0 M) and low-salt (0.4 M) conditions, respectively. (A) The red and black traces are the LC-MS data for monitoring protonated glycine betaine (m/z = 118) under high-salt and low-salt conditions, respectively. (B) Quantitative analysis of glycine betaine production by HPLC. Data are the average of three biological replicates and the error bars represent the s.d.
Glycine Betaine Is Synthesized via Glycine Methylation Pathway in Thioalkalivibrio versutus D301
To determine if glycine methylation pathway is used by T. versutus D301 for the biosynthesis of glycine betaine, His-tagged versions of the TVD_RS00875 and TVD_RS00880 were overexpressed and purified from E. coli BL21(DE3) (Figure 5A). The calculated molecular masses based on the amino acid sequences of the TVD_RS00875 and TVD_RS00880 are both 32 kDa. However, the molecular mass of TVD_RS00875 estimated from the SDS-PAGE gel was slightly higher than its calculated molecular mass. A similar phenomenon was also observed for HhGSMT from H. halochloris (Nyyssola et al., 2001). The molecular masses of HhGSMT estimated from the SDS-PAGE and analytical gel filtration were 42 kDa and 40 kDa, both of which were far higher than the calculated molecular mass of 31 kDa.
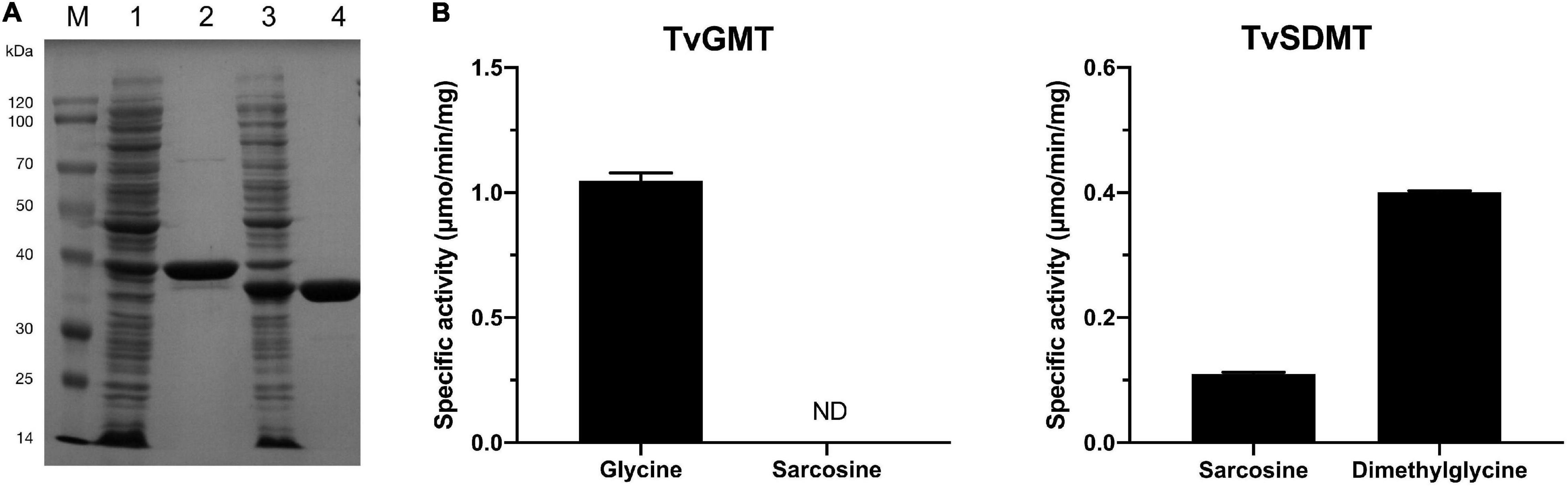
Figure 5. Glycine N-methyltransferase (TvGMT) and sarcosine dimethylglycine N-methyltransferase (TvSDMT) overexpressed and purified from E. coli convert glycine to glycine betaine by sequentially transferring three methyl groups to glycine. (A) SDS-PAGE analysis of TvGMT and TvSDMT. Lane M, protein marker; Lane 1, crude extract of TvGMT; Lane 2, purified TvGMT; Lane 3, crude extract of TvSDMT; Lane 4, purified TvSDMT. (B) Specific activities of TvGMT and TvSDMT using glycine, sarcosine, and dimethylglycine as substrates, respectively. Data are the average of three biological replicates and the error bars represent the s.d.
Methyltransferase assays were conducted according to a published method with minor modifications (Figure 5B; Nyyssola et al., 2001). Given that the reaction product S-adenosylhomocysteine (AdoHcy) is a strong competitive inhibitor of many methyltransferases (Heady and Kerr, 1973; Upmeier et al., 1988; Nyyssola et al., 2001), as high as 10 mM SAM was used as the methyl donor in the reaction system. The purified TVD_RS00875 converted glycine to sarcosine with a relatively high specific activity (1.0 U/mg protein). The substrate specificity of TVD_RS00875 is different from HhGSMT, which transfers methyl group to both glycine and sarcosine (Nyyssola et al., 2001). Therefore, the TVD_RS00875 was designated as TvGMT. The purified TVD_RS00880, designated as TvSDMT, exhibited activities on both sarcosine and dimethylglycine, showing a higher specific activity toward dimethylglycine. The sequential action of TvGMT and TvSDMT resulted in the biosynthesis of glycine betaine from glycine in a process of three-step methylation.
Discussion
The comparative transcriptomic analysis showed that genes involved in nitrogen metabolism were significantly upregulated under high-salt conditions. The upregulated genes for nitrate ABC transporters (NrtABC) (Frias et al., 1997), with log2FC ranging from 2.6 to 7.1, could result in the transport of more extracellular nitrate into the cytosol of T. versutus, where nitrate is sequentially reduced to ammonia by nitrate reductase (NasA, TVD_RS03430) and nitrite reductase (NirBD, TVD_RS03425 and TVD_RS03420), respectively, with nitrite as the intermediate (Harborne et al., 1992; Ogawa et al., 1995). The nasA and nirBD genes were all significantly upregulated (log2FC > 4) in response to high-salt stress. Besides the nitrate reduction, ammonia transport and assimilation genes such as amt (TVD_RS01370) and glnA (TVD_RS13790), whose gene products ammonia transporter (Amt) and glutamine synthetase (GlnA) are responsible for the transport of extracellular ammonia into cells and for the conversion of ammonia and glutamate to glutamine, respectively, were also significantly upregulated. Besides the genes directly involved in nitrogen metabolism, genes associated with their regulation were also upregulated, such as two-component system GlnLG (TVD_RS12990 and TVD_RS12985) that responds to the nitrogen limitation and then activate the expression of glnA (Reitzer, 2003). Therefore, pathways associated with nitrogen metabolism could play a key role in resisting the high-salt stress.
Sufficient supply of nitrogen is required to guarantee the biosynthesis of N-containing compatible solutes. Marine bacterium Dinoroseobacter shibae, which normally uses both N-containing glutamate and N-free α-glucosylglycerate/α-glucosylglycerol as compatible solutes, prefers to synthesize α-glucosylglycerate when nitrogen is limiting (Kleist et al., 2017). Besides D. shibae, halophilic bacterium H. halochloris produces more trehalose and less glycine betaine to maintain the intra- and extracellular osmotic balance under nitrogen-limited conditions (Galinski and Herzog, 1990). The obvious upregulation of genes associated with nitrogen metabolism suggests that a variety of nitrogen sources are mobilized for use to guarantee the biosynthesis of N-containing compatible solutes. Given that N-containing glycine betaine is a compatible solute commonly used in Thioalkalivibrio species (Banciu et al., 2004a,2005; Ahn et al., 2021), the rapid mobilization of biologically available nitrogen is probably used for the biosynthesis of glycine betaine.
Based on the genomic analysis of T. versutus D301, TVD_RS00875 and TVD_RS00880 gene products that homologous to the GSMT and SDMT from H. halochloris were considered to be responsible for the biosynthesis of glycine betaine by the three-step methylation of glycine. No genes involved in choline oxidation pathway were found in the genome of T. versutus D301. However, transcriptomic analysis showed that the expression levels of both TVD_RS00875 and TVD_RS00880 under high-salt conditions did not increase compared to that under low-salt conditions. It demonstrates the biosynthesis of glycine betaine are probably regulated by posttranslational modification, and nitrogen metabolism related genes are regulated at transcriptional level. In addition to de novo biosynthesis of glycine betaine, T. versutus is also able to transport glycine betaine across membranes by glycine betaine/proline ABC transporters (TVD_RS10550, TVD_RS10555, and TVD_RS10560) when glycine betaine is available in environment (Ko and Smith, 1999). When glycine betaine is unavailable in extreme environment, T. versutus will synthesize compatible solutes to overcome the challenge of environmental osmolarity.
Different from T. versutus D301, which had an about 40-fold increase of glycine betaine content in response to high-salt stress as shown by quantification, T. versutus ALJ 15 grown in medium supplemented with a high concentration of sodium carbonate/sodium bicarbonate (4 M Na+, 0.1 M NaCl, and 3.9 M Na2CO3/NaHCO3) only produced six-fold more glycine betaine than in low-salt medium (0.6 M Na+, 0.1 M NaCl, and 0.5 M Na2CO3/NaHCO3) (Banciu et al., 2005). As measured by Banciu et al., the osmotic pressure of 4 M NaCl was almost two times higher than that of 4 M Na2CO3/NaHCO3 (Banciu et al., 2004a). Based on this measurement, the osmotic pressure of 2.6 M NaCl is slightly higher than that of 3.4 M Na2CO3/NaHCO3. Given that glycine betaine in T. halophilus grown with 4 M NaCl and 4 M soda medium accounted for 19.8% (w/w) and 12.4% (w/w) of biomass, respectively, such a small difference in osmolarity will not make such a large difference in the content of glycine betaine (Banciu et al., 2004a). It is worth noting that T. versutus ALJ 15 also produced a certain amount of sucrose under high-salt conditions (4 M Na+), accounting for 1.7% of the cell dry weight (Banciu et al., 2005). The N-free sucrose may partly contribute to the salinity tolerance of T. versutus ALJ 15. However, no differential expression of the putative sucrose-phosphate synthase gene (TVD_RS01115), which is responsible for the biosynthesis of sucrose in T. versutus D301, was observed between low-salt and high-salt conditions. Moreover, no sucrose could be detected in T. versutus D301 cells grown under low-salt and high-salt conditions, respectively. Therefore, different from the situation in T. versutus ALJ 15, glycine betaine probably plays a major role in resisting the high-salt stress in T. versutus D301 (Banciu et al., 2004a,2005).
This study shows that glycine methylation pathway is used by T. versutus D301 for the biosynthesis of glycine betaine, in which TvGMT and TvSDMT sequentially catalyze the three-step methylation of glycine. It is worth noting that the specific activity of TvGMT was over six-fold higher than that of HhGSMT (Nyyssola et al., 2001). Therefore, TvGMT can be used as a promising gene for the heterologous synthesis of glycine betaine in transgenic plants, which would be more tolerant to halo-alkaline environments (Chen and Murata, 2002). TvGMT and TvSDMT have about 77 and 59% sequence identities to HhGSMT and HhSDMT from H. halochloris, which were already known to participate in biosynthesis of glycine betaine via glycine methylation pathway (Nyyssola et al., 2001). In addition, the gene clusters encoding glycine methylation pathway were also present in the genomes of Thioalkalivibrio sp. and T. sulfidophilus (Muyzer et al., 2011a,b), suggesting that biosynthesis of glycine betaine via glycine methylation pathway is a general mechanism employed by Thioalkalivibrio species. However, the substrate specificity of TvGMT was quite different from HhGSMT. It suggests enzymes for glycine methylation pathway have evolved for different bacteria to adapt to changing environments. Characterization of these enzymes will contribute to a better understanding of the environmental adaptation mechanism of Thioalkalivibrio. The conversion of glycine to glycine betaine is energy intensive because of the requirement for SAM, which is involved in methyl group transfers as cosubstrate. As much as 12 ATP equivalents are required to regenerate an active SAM (Atkinson, 1977), so 36 ATP equivalents are needed to form one molecule of glycine betaine, which requires three active SAMs. T. versutus D301 grows slowly with low biomass, which limits its practical application in biodesulfurization industry (Sharshar et al., 2019). The decreased growth rate of T. versutus D301 under high-salt conditions could be attributed to the energy burden caused by the biosynthesis of glycine betaine.
Conclusion
Glycine betaine was found to be a main compatible solute in T. versutus D301, which is widely used as a biocatalyst for desulfurization. Genes associated with nitrogen metabolism of T. versutus D301 were significantly upregulated under high-salt conditions, causing the enhanced production of glycine betaine that functions as a main compatible solute to resist osmotic pressure and prevent osmotic lysis. Glycine betaine was synthesized from glycine by TvGMT and TvSDMT in a three-step process of methylation. This work has given us an improved understanding how Thioalkalivibrio adapts to extreme environments, which may further contribute to the engineering of T. versutus D301 to improve the process of biodesulfurization.
Data Availability Statement
The datasets presented in this study can be found in online repositories. The names of the repository/repositories and accession number(s) can be found below: National Center for Biotechnology Information (NCBI) BioProject database under accession number PRJNA812740.
Author Contributions
ML and YZ designed the research. ML, HL, FM, NY, DZ, and GA performed the research and analyzed the data. ML wrote the manuscript under the guidance of YZ. DZ, GA, and HX participated in discussion and revision. All authors have read and approved the final manuscript.
Funding
This study was funded by the National Key R&D Program of China (2020YFA0906800), the National Natural Science Foundation of China (91851102 and 32070034), the Senior User Project of RV KEXUE, Center for Ocean Mega-Science, Chinese Academy of Sciences (KEXUE2019GZ05), and the Strategic Priority Research Program of the Chinese Academy of Sciences (XDA28030201).
Conflict of Interest
The authors declare that the research was conducted in the absence of any commercial or financial relationships that could be construed as a potential conflict of interest.
Publisher’s Note
All claims expressed in this article are solely those of the authors and do not necessarily represent those of their affiliated organizations, or those of the publisher, the editors and the reviewers. Any product that may be evaluated in this article, or claim that may be made by its manufacturer, is not guaranteed or endorsed by the publisher.
Acknowledgments
We would like to thank all the members of Zheng Lab for their contributions on literature collection and critical reading of the manuscript.
Footnotes
References
Ahn, A. C., Jongepier, E., Schuurmans, J. M., Rijpstra, W. I. C., Damste, J. S. S., Galinski, E. A., et al. (2021). Molecular and physiological adaptations to low temperature in Thioalkalivibrio strains isolated from soda lakes with different temperature regimes. mSystems 6:e01202-20. doi: 10.1128/mSystems.01202-20
Banciu, H., Sorokin, D. Y., Galinski, E. A., Muyzer, G., Kleerebezem, R., and Kuenen, J. G. (2004a). Thialkalivibrio halophilus sp nov., a novel obligately chemolithoautotrophic, facultatively alkaliphilic, and extremely salt-tolerant, sulfur-oxidizing bacterium from a hypersaline alkaline lake. Extremophiles 8, 325–334. doi: 10.1007/s00792-004-0391-6
Banciu, H., Sorokin, D. Y., Kleerebezem, R., Muyzer, G., Galinski, E. A., and Kuenen, J. G. (2004b). Growth kinetics of haloalkaliphilic, sulfur-oxidizing bacterium Thioalkalivibrio versutus strain ALJ 15 in continuous culture. Extremophiles 8, 185–192. doi: 10.1007/s00792-004-0376-5
Banciu, H., Sorokin, D. Y., Rijpstra, W. I. C., Damste, J. S. S., Galinski, E. A., Takalchi, S., et al. (2005). Fatty acid, compatible solute and pigment composition of obligately chemolithoautotrophic alkaliphilic sulfur-oxidizing bacteria from soda lakes. FEMS Microbiol. Lett. 243, 181–187. doi: 10.1016/j.femsle.2004.12.004
Boyd, L. A., Adam, L., Pelcher, L. E., McHughen, A., Hirji, R., and Selvaraj, G. (1991). Characterization of an Escherichia coli gene encoding betaine aldehyde dehydrogenase (BADH): structural similarity to mammalian ALDHs and a plant BADH. Gene 103, 45–52. doi: 10.1016/0378-1119(91)90389-s
Brown, A. D. (1976). Microbial water stress. Bacteriol. Rev. 40, 803–846. doi: 10.1128/mmbr.40.4.803-846.1976
Chen, T. H. H., and Murata, N. (2002). Enhancement of tolerance of abiotic stress by metabolic engineering of betaines and other compatible solutes. Curr. Opin. Plant Biol. 5, 250–257. doi: 10.1016/s1369-5266(02)00255-8
Christian, J. H. B., and Waltho, J. A. (1962). Solute concentrations within cells of halophilic and non-halophilic bacteria. Biochim. Biophys. Acta 65, 506–508. doi: 10.1016/0006-3002(62)90453-5
Dennis, P. P., and Shimmin, L. C. (1997). Evolutionary divergence and salinity-mediated selection in halophilic archaea. Microbiol. Mol. Biol. Rev. 61, 90–104. doi: 10.1128/.61.1.90-104.1997
Eisenberg, H., and Wachtel, E. J. (1987). Structural studies of halophilic proteins, ribosomes, and organelles of bacteria adapted to extreme salt concentrations. Annu. Rev. Biophys. Biophys. Chem. 16, 69–92. doi: 10.1146/annurev.bb.16.060187.000441
Fan, F., Ghanem, M., and Gadda, G. (2004). Cloning, sequence analysis, and purification of choline oxidase from Arthrobacter globiformis: a bacterial enzyme involved in osmotic stress tolerance. Arch. Biochem. Biophys. 421, 149–158. doi: 10.1016/j.abb.2003.10.003
Frias, J. E., Flores, E., and Herrero, A. (1997). Nitrate assimilation gene cluster from the heterocyst-forming cyanobacterium Anabaena sp strain PCC 7120. J. Bacteriol. 179, 477–486. doi: 10.1128/jb.179.2.477-486.1997
Galinski, E. A., and Herzog, R. M. (1990). The role of trehalose as a substitute for nitrogen-containing compatible solutes (Ectothiorhodospira halochloris). Arch. Microbiol. 153, 607–613. doi: 10.1007/bf00245273
Galinski, E. A., and Truper, H. G. (1994). Microbial behaviour in salt-stressed ecosystems. FEMS Microbiol. Rev. 15, 95–108. doi: 10.1016/0168-6445(94)90106-6
Ginzburg, M., Sachs, L., and Ginzburg, B. Z. (1970). Ion metabolism in a Halobacterium. I. Influence of age of culture on intracellular concentrations. J. Gen. Physiol. 55, 187–207. doi: 10.1085/jgp.55.2.187
Gunde-Cimerman, N., Plemenitas, A., and Oren, A. (2018). Strategies of adaptation of microorganisms of the three domains of life to high salt concentrations. FEMS Microbiol. Rev. 42, 353–375. doi: 10.1093/femsre/fuy009
Harborne, N. R., Griffiths, L., Busby, S. J. W., and Cole, J. A. (1992). Transcriptional control, translation and function of the products of the five open reading frames of the Escherichia coli nir operon. Mol. Microbiol. 6, 2805–2813. doi: 10.1111/j.1365-2958.1992.tb01460.x
Heady, J. E., and Kerr, S. J. (1973). Purification and characterization of glycine N-methyltransferase. J. Biol. Chem. 248, 69–72.
Kageyama, H., Tanaka, Y., and Takabe, T. (2018). Biosynthetic pathways of glycinebetaine in Thalassiosira pseudonana; functional characterization of enzyme catalyzing three-step methylation of glycine. Plant Physiol. Biochem. 127, 248–255. doi: 10.1016/j.plaphy.2018.03.032
Kempf, B., and Bremer, E. (1998). Uptake and synthesis of compatible solutes as microbial stress responses to high-osmolality environments. Arch. Microbiol. 170, 319–330. doi: 10.1007/s002030050649
Kleist, S., Ulbrich, M., Bill, N., Schmidt-Hohagen, K., Geffers, R., and Schomburg, D. (2017). Dealing with salinity extremes and nitrogen limitation – an unexpected strategy of the marine bacterium Dinoroseobacter shibae. Environ. Microbiol. 19, 894–908. doi: 10.1111/1462-2920.13266
Ko, R., and Smith, L. T. (1999). Identification of an ATP-driven, osmoregulated glycine betaine transport system in Listeria monocytogenes. Appl. Environ. Microbiol. 65, 4040–4048. doi: 10.1128/AEM.65.9.4040-4048.1999
Lai, S.-J., and Lai, M.-C. (2011). Characterization and regulation of the osmolyte betaine synthesizing enzymes GSMT and SDMT from halophilic methanogen Methanohalophilus portucalensis. PLoS One 6:e25090. doi: 10.1371/journal.pone.0025090
Landfald, B., and Strom, A. R. (1986). Choline-glycine betaine pathway confers a high level of osmotic tolerance in Escherichia coli. J. Bacteriol. 165, 849–855. doi: 10.1128/jb.165.3.849-855.1986
Lanyi, J. K. (1974). Salt-dependent properties of proteins from extremely halophilic bacteria. Bacteriol. Rev. 38, 272–290. doi: 10.1128/mmbr.38.3.272-290.1974
Lu, W.-D., Chi, Z.-M., and Su, C.-D. (2006). Identification of glycine betaine as compatible solute in Synechococcus sp WH8102 and characterization of its N-methyltransferase genes involved in betaine synthesis. Arch. Microbiol. 186, 495–506. doi: 10.1007/s00203-006-0167-8
Mu, T., Yang, M., and Xing, J. (2021). Performance and characteristic of a haloalkaliphilic bio-desulfurizing system using Thioalkalivibrio verustus D301 for efficient removal of H2S. Biochem. Eng. J. 165:107812. doi: 10.1016/j.bej.2020.107812
Mu, T., Yang, M., Zhao, J., Sharshar, M. M., Tian, J., and Xing, J. (2017). Improvement of desulfurizing activity of haloalkaliphilic Thialkalivibrio versutus SOB306 with the expression of Vitreoscilla hemoglobin gene. Biotechnol. Lett. 39, 447–452. doi: 10.1007/s10529-016-2266-1
Mu, T., Zhou, J., Yang, M., and Xing, J. (2016). Complete genome sequence of Thialkalivibrio versutus D301 isolated from soda lake in northern China, a typical strain with great ability to oxidize sulfide. J. Biotechnol. 227, 21–22. doi: 10.1016/j.jbiotec.2016.04.019
Muyzer, G., Sorokin, D. Y., Mavromatis, K., Lapidus, A., Clum, A., Ivanova, N., et al. (2011a). Complete genome sequence of “Thioalkalivibrio sulfidophilus” HL-EbGr7. Stand. Genomic Sci. 4, 23–35. doi: 10.4056/sigs.1483693
Muyzer, G., Sorokin, D. Y., Mavromatis, K., Lapidus, A., Foster, B., Sun, H., et al. (2011b). Complete genome sequence of Thioalkalivibrio sp. K90mix. Stand. Genomic Sci. 5, 341–355. doi: 10.4056/sigs.2315092
Nyyssola, A., Kerovuo, J., Kaukinen, P., von Weymarn, N., and Reinikainen, T. (2000). Extreme halophiles synthesize betaine from glycine by methylation. J. Biol. Chem. 275, 22196–22201. doi: 10.1074/jbc.M910111199
Nyyssola, A., Reinikainen, T., and Leisola, M. (2001). Characterization of glycine sarcosine N-methyltransferase and sarcosine dimethylglycine N-methyltransferase. Appl. Environ. Microbiol. 67, 2044–2050. doi: 10.1128/aem.67.5.2044-2050.2001
Ogawa, K. I., Akagawa, E., Yamane, K., Sun, Z. W., Lacelle, M., Zuber, P., et al. (1995). The nasB operon and nasA gene are required for nitrate/nitrite assimilation in Bacillus subtilis. J. Bacteriol. 177, 1409–1413. doi: 10.1128/jb.177.5.1409-1413.1995
Reistad, R. (1970). On the composition and nature of the bulk protein of extremely halophilic bacteria. Arch. Mikrobiol. 71, 353–360. doi: 10.1007/bf00417131
Reitzer, L. (2003). Nitrogen assimilation and global regulation in Escherichia coli. Annu. Rev. Microbiol. 57, 155–176. doi: 10.1146/annurev.micro.57.030502.090820
Roberts, M. F. (2005). Organic compatible solutes of halotolerant and halophilic microorganisms. Saline Syst. 1:5. doi: 10.1186/1746-1448-1-5
Sharshar, M. M., Samak, N. A., Hao, X., Mu, T., Zhong, W., Yang, M., et al. (2019). Enhanced growth-driven stepwise inducible expression system development in haloalkaliphilic desulfurizing Thioalkalivibrio versutus. Bioresour. Technol. 288:121486. doi: 10.1016/j.biortech.2019.121486
Sorokin, D. Y., Lysenko, A. M., Mityushina, L. L., Tourova, T. P., Jones, B. E., Rainey, F. A., et al. (2001). Thioalkalimicrobium aerophilum gen. nov., sp nov and Thioalkalimicrobium sibericum sp nov., and Thioalkalivibrio versutus gen. nov., sp nov., Thioalkalivibrio nitratis sp nov and Thioalkalivibrio denitrificans sp nov., novel obligately alkaliphilic and obligately chemolithoautotrophic sulfur-oxidizing bacteria from soda lakes. Int. J. Syst. Evol. Microbiol. 51, 565–580. doi: 10.1099/00207713-51-2-565
Upmeier, B., Gross, W., Koster, S., and Barz, W. (1988). Purification and properties of S-adenosyl-L-methionine:nicotinic acid-N-methyltransferase from cell suspension cultures of Glycine-max L. Arch. Biochem. Biophys. 262, 445–454. doi: 10.1016/0003-9861(88)90396-7
Waditee, R., Tanaka, Y., Aoki, K., Hibino, T., Jikuya, H., Takano, J., et al. (2003). Isolation and functional characterization of N-methyltransferases that catalyze betaine synthesis from glycine in a halotolerant photosynthetic organism Aphanothece halophytica. J. Biol. Chem. 278, 4932–4942. doi: 10.1074/jbc.M210970200
Keywords: glycine betaine, biosynthetic pathway, Thioalkalivibrio versutus, glycine N-methyltransferase, sarcosine dimethylglycine N-methyltransferase
Citation: Liu M, Liu H, Mei F, Yang N, Zhao D, Ai G, Xiang H and Zheng Y (2022) Identification of the Biosynthetic Pathway of Glycine Betaine That Is Responsible for Salinity Tolerance in Halophilic Thioalkalivibrio versutus D301. Front. Microbiol. 13:875843. doi: 10.3389/fmicb.2022.875843
Received: 14 February 2022; Accepted: 25 March 2022;
Published: 18 April 2022.
Edited by:
Wen-Jun Li, Sun Yat-sen University, ChinaReviewed by:
Jianmin Xing, Institute of Process Engineering (CAS), ChinaShi-Hong Zhang, Jilin University, China
Rosa María Martínez-Espinosa, University of Alicante, Spain
Copyright © 2022 Liu, Liu, Mei, Yang, Zhao, Ai, Xiang and Zheng. This is an open-access article distributed under the terms of the Creative Commons Attribution License (CC BY). The use, distribution or reproduction in other forums is permitted, provided the original author(s) and the copyright owner(s) are credited and that the original publication in this journal is cited, in accordance with accepted academic practice. No use, distribution or reproduction is permitted which does not comply with these terms.
*Correspondence: Yanning Zheng, emhlbmd5bkBpbS5hYy5jbg==