Corrigendum: Atypical carboxysome loci: JEEPs or junk?
- 1Integrative Biology Department, University of South Florida, Tampa, FL, United States
- 2MSU-DOE Plant Research Laboratory, Michigan State University, East Lansing, MI, United States
- 3Environmental Genomics and Systems Biology and Molecular Biophysics and Integrated Bioimaging Divisions, Lawrence Berkeley National Laboratory, Berkeley, CA, United States
Carboxysomes, responsible for a substantial fraction of CO2 fixation on Earth, are proteinaceous microcompartments found in many autotrophic members of domain Bacteria, primarily from the phyla Proteobacteria and Cyanobacteria. Carboxysomes facilitate CO2 fixation by the Calvin-Benson-Bassham (CBB) cycle, particularly under conditions where the CO2 concentration is variable or low, or O2 is abundant. These microcompartments are composed of an icosahedral shell containing the enzymes ribulose 1,5-carboxylase/oxygenase (RubisCO) and carbonic anhydrase. They function as part of a CO2 concentrating mechanism, in which cells accumulate HCO3− in the cytoplasm via active transport, HCO3− enters the carboxysomes through pores in the carboxysomal shell proteins, and carboxysomal carbonic anhydrase facilitates the conversion of HCO3− to CO2, which RubisCO fixes. Two forms of carboxysomes have been described: α-carboxysomes and β-carboxysomes, which arose independently from ancestral microcompartments. The α-carboxysomes present in Proteobacteria and some Cyanobacteria have shells comprised of four types of proteins [CsoS1 hexamers, CsoS4 pentamers, CsoS2 assembly proteins, and α-carboxysomal carbonic anhydrase (CsoSCA)], and contain form IA RubisCO (CbbL and CbbS). In the majority of cases, these components are encoded in the genome near each other in a gene locus, and transcribed together as an operon. Interestingly, genome sequencing has revealed some α-carboxysome loci that are missing genes encoding one or more of these components. Some loci lack the genes encoding RubisCO, others lack a gene encoding carbonic anhydrase, some loci are missing shell protein genes, and in some organisms, genes homologous to those encoding the carboxysome-associated carbonic anhydrase are the only carboxysome-related genes present in the genome. Given that RubisCO, assembly factors, carbonic anhydrase, and shell proteins are all essential for carboxysome function, these absences are quite intriguing. In this review, we provide an overview of the most recent studies of the structural components of carboxysomes, describe the genomic context and taxonomic distribution of atypical carboxysome loci, and propose functions for these variants. We suggest that these atypical loci are JEEPs, which have modified functions based on the presence of Just Enough Essential Parts.
Introduction
Autotrophic organisms that use the Calvin-Benson-Bassham cycle (CBB) for carbon dioxide fixation must grapple with the catalytic constraints of ribulose 1,5-bisphosphate carboxylase/oxygenase (RubisCO). This enzyme has poor substrate specificity; it catalyzes both the carboxylase reaction of the CBB, as well as a wasteful oxygenase reaction, which results in added energetic expense to regenerate the ribulose 1,5 bisphosphate (RuBP) necessary for the CBB (Tabita, 1999). In addition, RubisCO enzymes have relatively low affinities for CO2 (5–250 μM; Tabita, 1999). RubisCO affinities for CO2 are particularly low for autotrophic bacteria (25–250 μM; tabulated in Horken and Tabita, 1999). Furthermore, RubisCO is not able to use HCO3− (Cooper and Filmer, 1969), the predominant form in the equilibrium between CO2 and HCO3− at the circumneutral pH typical for cytoplasm.
In order to grow while using CO2 as a major carbon source, many autotrophic bacteria using the CBB cycle have CO2-concentrating mechanisms (CCMs). CCMs consist of two components: (1) membrane transporters for dissolved inorganic carbon (DIC; = CO2 + HCO3− + CO32−), which generate high concentrations of cytoplasmic HCO3−, and (2) carboxysomes, which are present in the cytoplasm and facilitate high rates of CO2 fixation by RubisCO (reviewed in Price et al., 2009; Long et al., 2016). Carboxysomes are a type of bacterial microcompartment, and consist of a protein shell filled with RubisCO and a trace of carbonic anhydrase activity (reviewed in Kerfeld et al., 2018). Cytoplasmic HCO3− enters carboxysomes, where carbonic anhydrase converts some of it to CO2, which is then fixed by RubisCO. CO2 is prevented from escaping from the carboxysome before fixation because the shell is impermeable to this gas (Dou et al., 2008; Cai et al., 2009). The components of CCMs, including carboxysomes, are often upregulated when autotrophic microorganisms are cultivated under low DIC conditions (Dobrinski et al., 2012; Esparza et al., 2019; Scott et al., 2019).
Two types of carboxysomes (α and β) are currently recognized (reviewed in Cannon et al., 2010; Kerfeld and Melnicki, 2016). Members of Proteobacteria and certain marine members of Cyanobacteria have α-carboxysomes, while the remaining members of Cyanobacteria have β-carboxysomes (Price et al., 2009; Scott et al., 2019). These types can be distinguished by the form of RubisCO they carry (α-carboxysomes carry form IA RubisCO; β-carboxysomes carry form IB RubisCO), as well as differences in carbonic anhydrases, scaffolding proteins, and carboxysome shell components (Kerfeld and Melnicki, 2016).
The composition of α-carboxysomes from members of phyla Proteobacteria and Cyanobacteria is mostly conserved (Kinney et al., 2011; Roberts et al., 2012; Sutter et al., 2021). The icosahedral shells of carboxysomes are comprised of (1) hexagonal units, consisting of hexamers of CsoS1 proteins that assemble into single-layers (Tsai et al., 2007), as well as trimers of CsoS1D proteins that assemble into single and double layers (Klein et al., 2009; Roberts et al., 2012), and (2) pentamers of CsoS4 proteins which assemble into pentagonal truncated pyramids and cap the vertices of the icosahedral shells (Tanaka et al., 2008; Cai et al., 2009; Zhao et al., 2019). Hexamers, trimers, and pentamers typically have central pores, which in some cases open and close. The size and charge of these pores are likely to dictate the selective permeability of carboxysome shells (Tsai et al., 2007; Kinney et al., 2011), which are impermeable to CO2 (Dou et al., 2008; Cai et al., 2009), and permeable to protons (Menon et al., 2010). α-carboxysomes contain RubisCO and carbonic anhydrase, as described above. Based on amino acid sequence, α-carboxysomal carbonic anhydrase (CsoSCA) was initially believed to be a new form of this enzyme, but its structure clarified that it is a deeply divergent β-carbonic anhydrase (So et al., 2004; Sawaya et al., 2006). α-carboxysomes also contain CsoS2, which facilitates the assembly of these microcompartments by binding to RubisCO and CsoS1 (Cai et al., 2015; Oltrogge et al., 2020). The conserved nature of α-carboxysome shell proteins and contents is reflected in gene synteny apparent in the loci encoding them; typical gene order in α-carboxysome loci is cbbL, cbbS, csoS2, csoSCA, csoS4AB, and csoS1ABC, with csoS1D genes, when present, often encoded a few genes downstream or elsewhere (Figure 1; Cannon et al., 2002; Cai et al., 2008; Roberts et al., 2012; Axen et al., 2014; Sutter et al., 2021).
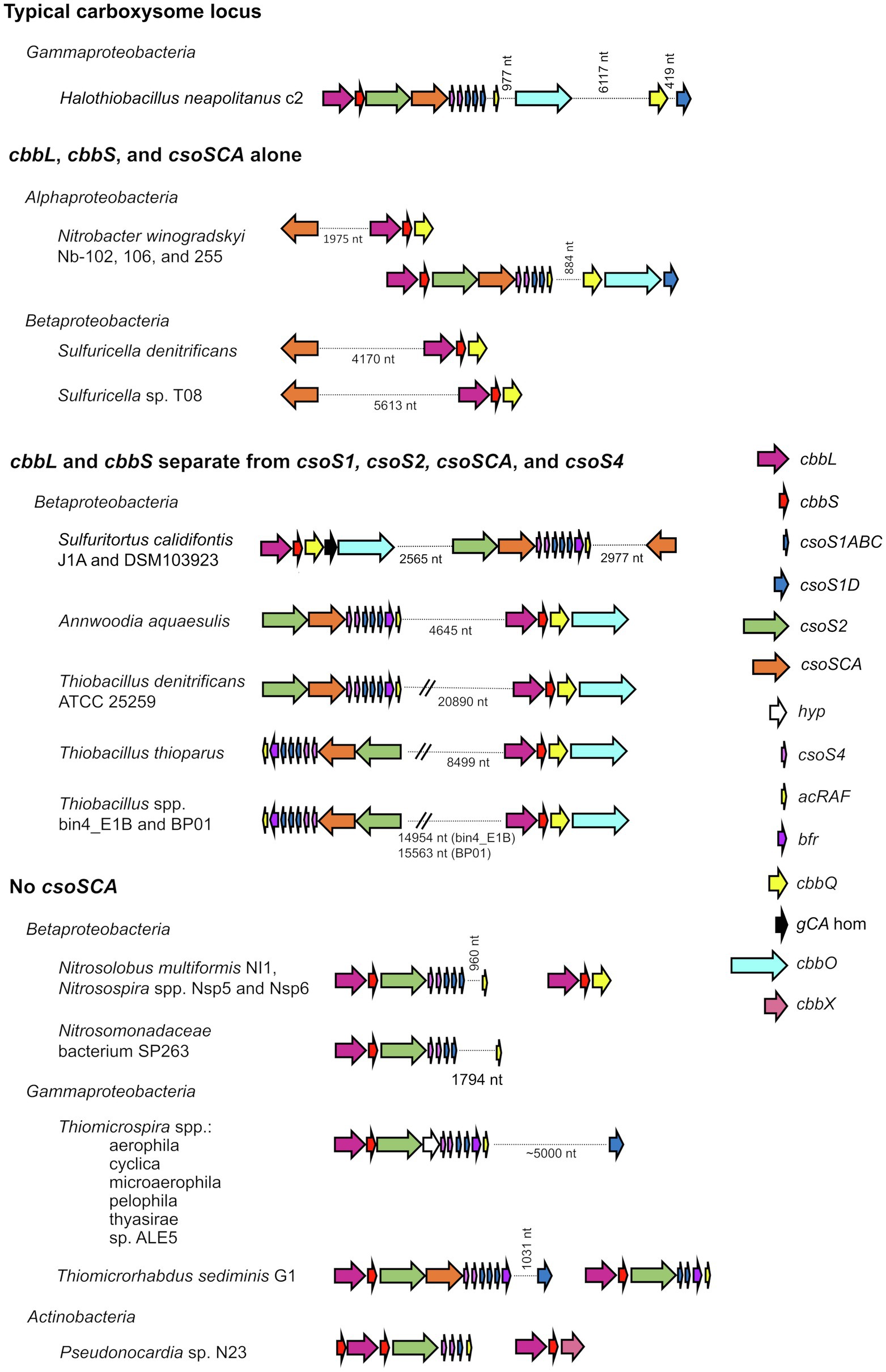
Figure 1. Atypical carboxysome loci. Arrows connected by dotted lines are collocated on the genome, and the distance between them is indicated in nucleotides (nt). cbbL, ribulose 1,5-carboxylase/oxygenase (RubisCO) large subunit; cbbS, RubisCO small subunit; csoS1ABC, hexamer shell proteins; csoS1D, pseudohexamer shell protein; csoS2, carboxysome assembly protein; csoSCA, carboxysomal carbonic anhydrase; hyp, hypothetical protein; csoS4, pentameric shell protein; acRAF, RubisCO assembly factor; bfr, bacterioferritin-like protein; cbbQ, RubisCO activase; gCA hom: gamma carbonic anhydrase homolog; cbbO: adaptor for CbbQ protein; and cbbX, RubisCO activase.
Atypical carboxysome loci are scattered among several phyla of Bacteria (Table 1). Most are present in genomes from members of Proteobacteria, as expected, given the abundance of organisms from this phylum with typical α-carboxysome loci (Cannon et al., 2002; Axen et al., 2014; Sutter et al., 2021). The atypical loci described here fall into four categories: (1) csoSCA is present without any of the other carboxysome-associated genes; (2) cbbL and cbbS and csoSCA are present, without genes encoding shell proteins; (3) genes encoding RubisCO are missing from the locus, with cbbL and cbbS encoded in a location distinct from csoS1, csoS2, csoSCA, and csoS4; and (4) csoSCA is absent, though the other carboxysome-associated genes are present (Figure 1). It seems likely that these atypical loci originated from typical loci, and were selected for in some lineages. The objective of this review is to assess the likelihood that the genes of these atypical loci are functional, predict the function of the loci, and describe how they may have originated.
Do the Genes From Atypical Carboxysome Loci Encode Functional Proteins?
The majority of genes from atypical carboxysome loci appear to encode proteins that could function similarly to their homologs from typical carboxysome loci, based on the presence of conserved amino acids predicted from their sequences (Table 2). For CbbS sequences from members of genus Nitrobacter, conserved residue Y25 (tyrosine) is replaced with histidine; given that both are large polar amino acids, this substitution may not disrupt the function of CbbS in these organisms. Pseudonocardia sp. N23 has two cbbS genes, with one (IMG gene ID 2868417193) immediately upstream of cbbL, and the other (IMG gene ID 2868417191) immediately downstream (Figure 1). The protein encoded by the upstream cbbS is only 63 amino acids long, shorter than is typical for CbbS (~90 amino acids), and is missing several conserved amino acids (L53, P54, and F56) in the portion that is present. The protein encoded by the cbbS gene downstream cbbL, as annotated in IMG, has a truncated amino terminus, but selecting an alternative start codon results in a predicted amino acid sequence including S2, L11, and P12. Based on these observations, the cbbS gene downstream of cbbL in Pseudonocardia sp. N23 is likely to be functional, while the cbbS upstream is not.
Genes encoding CsoS1A-C from atypical carboxysome loci have some amino acid substitutions at conserved positions. In many cases the substitutions are biochemically similar; e.g., V36 (valine) is replaced with an isoleucine, R70 (arginine) is replaced with a lysine, I80 (isoleucine) is replaced with a valine, which suggests similar functionality. However, there are some instances, e.g., for Pseudonocardia sp. N23, where the amino acids are not biochemically similar [V36 (valine) is replaced with glutamate; G37 (glycine) is replaced with aspartate]; given that these are core residues of CsoS1 monomers, folding may be problematic, suggesting that these carboxysomes may not be able to assemble.
Genes encoding CsoS2 from atypical carboxysome loci have features that have been found to be conserved among sequences from typical carboxysome loci. All have at least one N-terminal [RK]XXXXX[HKR]R motif, which binds RubisCO (Cai et al., 2015; Oltrogge et al., 2020). Of the six repetitive motifs from the M (middle) region of CsoS2 (Cai et al., 2015), M1–M4 and M6 are present, while M5 is less conserved. All share a conserved carboxy terminus as described in (Cai et al., 2015).
α-Carboxysomal carbonic anhydrase encoded by atypical carboxysome loci, including those from loci consisting solely of csoSCA homologs, have all of the active site residues. In typical carboxysome loci, csoSCA follows csoS2. Members of genus Thiomicrospira have a gene following csoS2 which in some cases matches weakly with Pfam08936 (see section “No csoSCA” below), but lack all of the active site residues necessary for carbonic anhydrase activity.
Amino acid sequences predicted from genes encoding CsoS4A and B from all of the atypical carboxysome loci include all of the conserved residues, though in some cases S56 (serine) is replaced with threonine; given that a hydroxyl moiety is present in both of these amino acids, this substitution is less likely to be disruptive to the function of these proteins.
Taxonomic Distribution, Origin, and Potential Function of the Four Types Of Atypical Carboxysome Loci
As described above, based on predicted amino acid sequences, most of the individual genes of atypical carboxysome loci appear to encode proteins sufficiently conserved to be capable of the same function as their homologs from typical carboxysome loci. Below are detailed descriptions of the taxonomic distribution of atypical loci, possible mechanisms for their origins, and predictions of how the proteins encoded by atypical carboxysome loci could function together.
csoSCA Alone
Genes homologous to those encoding CsoSCA are quite widespread beyond carboxysome loci, and are present in genomes from autotrophic (e.g., Sulfuritortus caldifontis, Nitrospia marina) and heterotrophic (e.g., Cand. Accumulibacter phosphatis; Chrysiogenes arsenatis) bacteria. Given their widespread distribution, it is surprising that they have yet to be studied (Table 1; Figure 2; referred to as csoSCA2 to distinguish them from those present in carboxysome loci). The amino acid sequences predicted from csoSCA2 genes share many features with CsoSCA proteins; they include both an active and defunct domain (Sawaya et al., 2006), and the active domain includes all of the residues necessary for catalytic activity as carbonic anhydrase (Table 2; Figure 3).
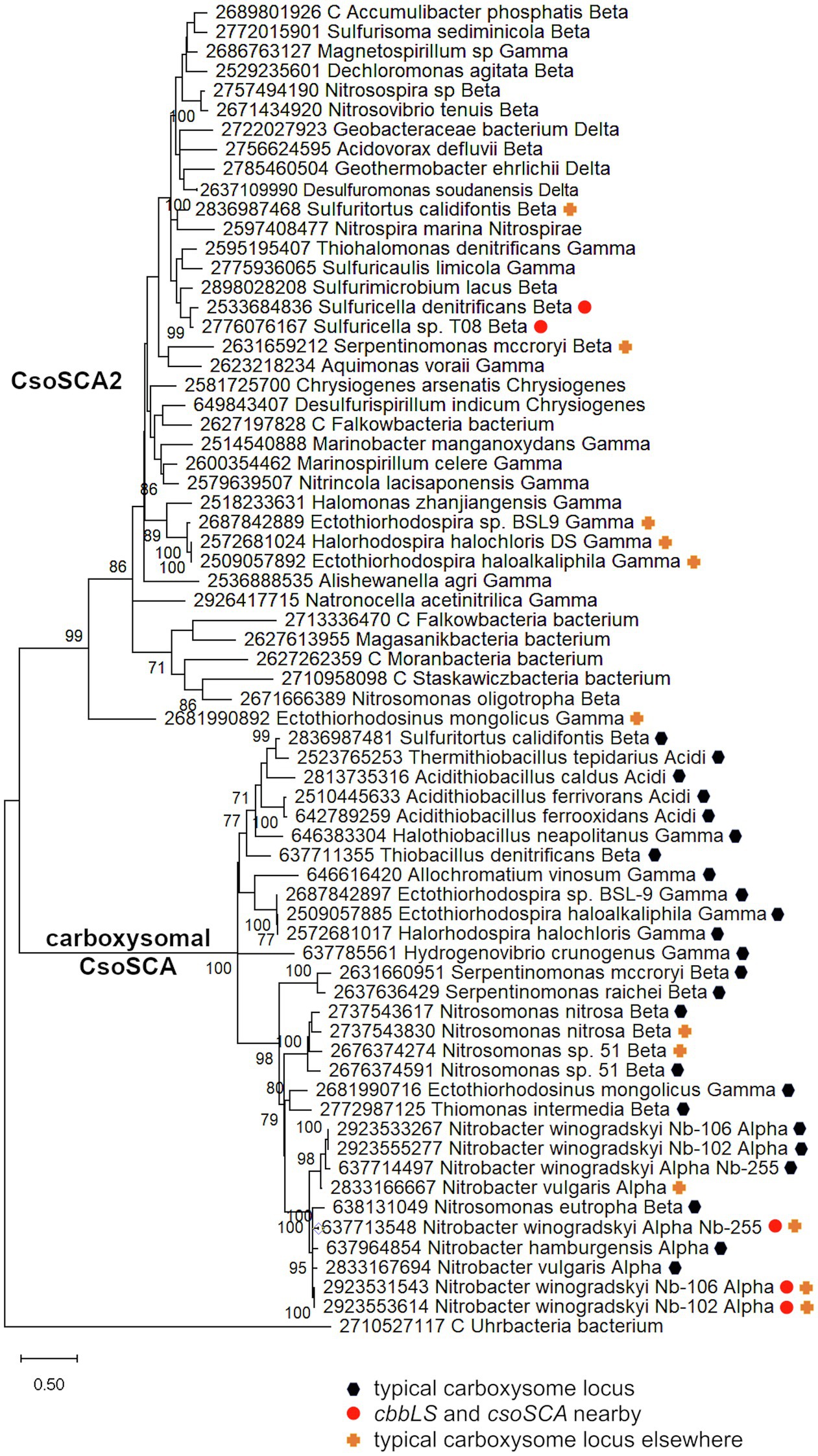
Figure 2. Maximum likelihood analysis of CsoSCA homologs from carboxysome loci and elsewhere (CsoSCA2). Amino acid sequences were gathered from the IMG database, aligned by MUSCLE in MEGA 11, and trimmed via GBLOCKS to a final length of 278 aa (Edgar, 2004; Talavera and Castresana, 2007; Tamura et al., 2021). The maximum likelihood tree was constructed with partial deletion of gaps (95% cut-off) and the JTT model (Jones et al., 1992; discrete Gamma distribution with five categories, gamma parameter = 1.9314, 3.55% of sites evolutionarily invariant; this model had the lowest AIC calculated via the Find Best DNA/Protein Models feature in MEGA 11; Hurvich and Tsai, 1989; Akaike, 1998). Branch lengths are proportional to the number of substitutions (scale bar = substitutions per site). Bootstrap values are based on 500 resamplings of the alignment, with values <70% omitted. Taxon labels include abbreviated names of classes of Proteobacteria (Alpha, Beta, Gamma, and Delta; Acidi = Acidithiobacillia), and full names of phyla beyond Proteobacteria. “C” indicates candidate status of species or phylum names. Taxon names also include symbols indicating the position of CsoSCA homologs relative to carboxysome-related genes, if present in the genomes. “Typical carboxysome locus” indicates that the CsoSCA homolog is part of a typical carboxysome locus, “cbbLS and csoSCA nearby” indicates that genes encoding RubisCO and a CsoSCA homolog are juxtaposed on the genome, and “typical carboxysome locus elsewhere” indicates that a typical carboxysome locus is present elsewhere on the genome.
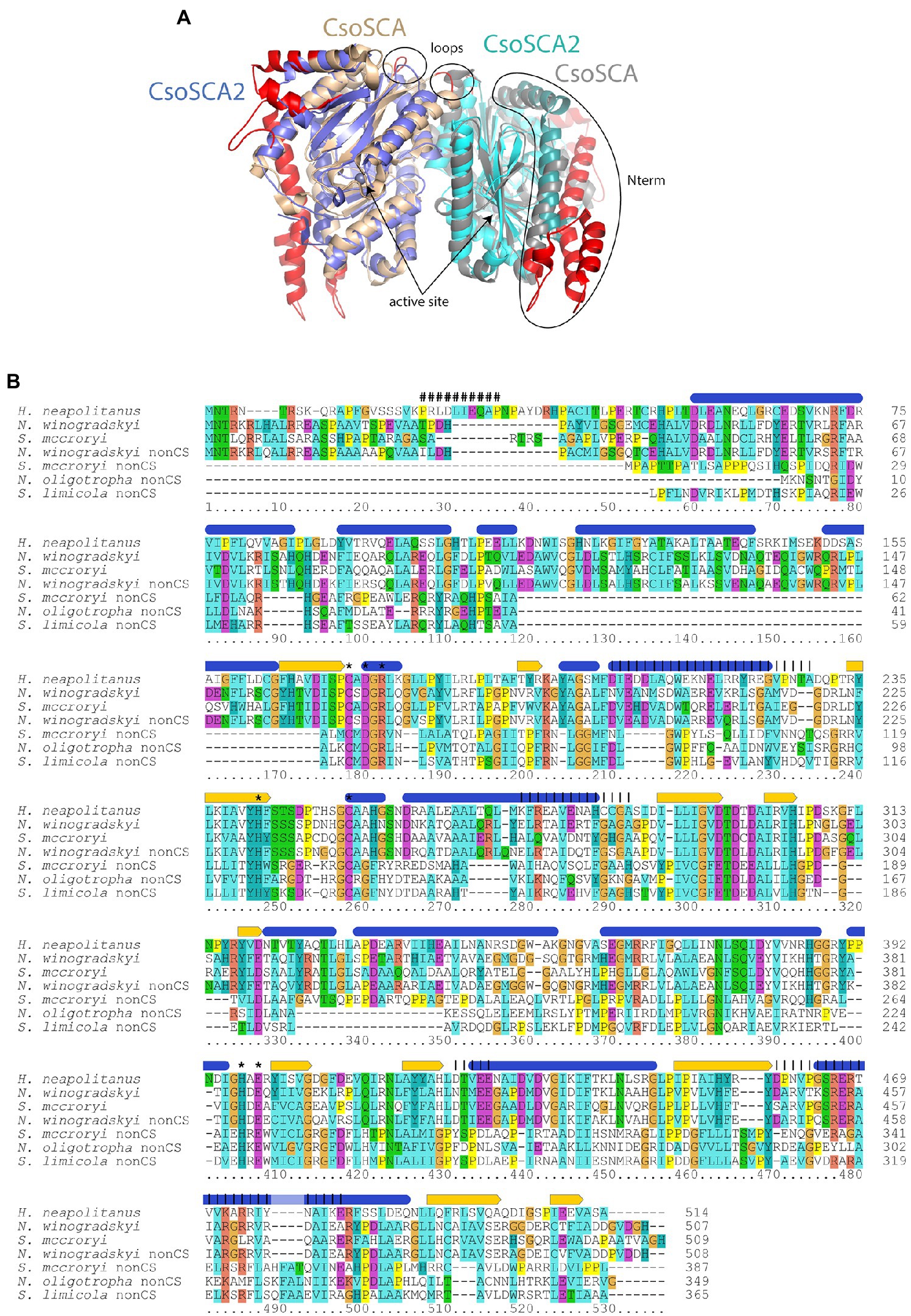
Figure 3. Comparison of carboxysomal CsoSCA and CsoSCA2. (A) Cartoon representation (www.pymol.org) of a dimer of CsoSCA subunits (pdb id 2FGY) in gray and wheat, and AlphaFold2 model of CsoSCA2 in blue and cyan. The active site zinc is labeled and shown as a sphere. Differences due to truncations are shown on the CsoSCA in red. The two N-terminal helices of CsoSCA2 are shown as slightly darker blue/cyan. Loop regions with truncations at the dimer interface are also labeled. (B) Alignment of carboxysomal CsoSCA and CsoSCA2 sequences. Structural and functional information from Halothiobacillus neapolitanus CsoSCA is indicated above the alignment: Blue ovals = alpha-helices, yellow arrows = beta strands, “#” = RubisCO binding site, “*” = active site residue, vertical lines = regions involved in dimerization. Coloring of conserved residues is according to chemical properties. Sequences from typical carboxysome loci included in the alignment are: Halothiobacillus neapolitanus, H. neapolitanus from Gammaproteobacteria, IMG gene object ID 646383304; Nitrobacter winogradskyi, N. winogradskyi from Alphaproteobacteria, IMG gene object ID 2923555277; Serpentimonas mccroryi, S. mccroryi from Betaproteobacteria, IMG gene object ID 2631660951. CsoSCA2 sequences included in the alignment are: N. winogradskyi nonCS, N. winogradskyi from Alphaproteobacteria, IMG gene object ID 2923553614; Serpentimonas mccroryi nonCS, S. mccroryi from Betaproteobacteria, IMG gene object ID 2631659212; Nitrosomonas oligotropha nonCS, N. oligotropha from Betaproteobacteria, IMG gene object ID 2671666389; Sulfuricaulis limicola nonCS, S. limicola from Gammaproteobacteria, IMG gene object ID 2775936065.
There are two variants of CsoSCA2. The first variant closely resembles carboxysomal CsoSCA (found in Nitrobacter vulgaris, Nitrobacter winogradskyi, Nitrosomonas nitrosa, and Nitrosomonas sp. 51), clustering with carboxysomal CsoSCA in phylogenetic analyses, but missing the N-terminal 40 residues (Figure 2; Figure 3). The second variant is further truncated at the N-terminus, is missing short stretches of sequence throughout, and does not cluster with carboxysomal CsoSCA sequences (Figure 2; Figure 3). The more substantially truncated version has an N-terminal domain of only 40 amino acids (instead of 144 for the CsoSCA from Halothiobacillus neapolitanus) that does not align with the CsoSCA equivalent on a sequence level but is also predicted to form two short alpha helices in an AlphaFold2 model (Jumper et al., 2021; Figure 3). Further truncations include shorter loops connecting secondary structure elements (Figure 3). Some of those extra elements are involved in dimer contacts (Sawaya et al., 2006), so it is possible that this homolog has lost the ability to form dimers, which would be unusual for a β-carbonic anhydrase (Cannon et al., 2010); however, this would need to be verified experimentally. The N-terminus of CsoSCA from Htb. neapolitanus facilitates interaction between CsoSCA and RubisCO (Blikstad et al., 2021). Presumably, since CsoSCA2 proteins do not interact with RubisCO, this N-terminal region is not necessary for CsoSCA2 to function outside of carboxysomes. Altogether, this form of CsoSCA2 seems to be a more compact version, possibly due to the fact that it is not necessary to encapsulate this protein in a carboxysome. This is particularly interesting for genomes that include both csoSCA and csoSCA2 genes (e.g., members of Nitrobacter, Nitrosomonas, Ectothiorhodospira, and Halorhodospira). Presumably, the CsoSCA2 proteins cannot assemble within the carboxysomes present in these organisms.
The presence of CsoSCA2 sequences in numerous phyla, and the more restricted distribution of CsoSCA, suggest that CsoSCA may have originated from CsoSCA2. However, in some cases, the reverse appears to be the case. Nitrobacter vulgaris, Nb. winogradskyi (Nb-102, 106, and 255), Ns. nitrosa, and Nitrosomonas sp. 51 have genes encoding both a CsoSCA (encoded in a typical carboyxsome locus), and a CsoSCA2 encoded elsewhere. The two copies cluster together within the larger clade of carboxysomal CsoSCA sequences, despite having the truncated N-termini seen in other CsoSCA2 sequences (Figure 2; Figure 3). Sequence similarities between CsoSCA and CsoSCA2 proteins in these organisms suggest that these CsoSCA2 sequences duplicated and diverged from CsoSCA.
One wonders why these deeply divergent β-carbonic anhydrases are so widespread, and what the features of these proteins might be that make them particularly useful to their host organisms. Though CsoSCA2 proteins lack the residues needed to associate with RubisCO, they may have residues that facilitate the formation of other types of enzyme complexes. Alternatively, CsoSCA2 proteins may not require aggregation with other proteins for activity. Indeed, even carboxysomal CsoSCA is active when expressed in the absence of other carboxysomal proteins (Heinhorst et al., 2006), which suggests that “free” CsoSCA2 could also be active in the cytoplasm of its host organisms.
cbbL, cbbS, and csoSCA Alone
In genomes from some members of Alpha- and Betaproteobacteria, csoSCA homologs are present near genes encoding RubisCO (Figure 1; Figure 4). In Alphaproteobacteria, three strains of Nb. winogradskyi share this arrangement of genes (though the average nucleotide identities of strains Nb-102 and Nb-106 versus Nb-255 are 94.6%, suggesting they may be a different species; Richter and Rosselló-Móra, 2009). Among Betaproteobacteria, two species of Sulfuricella have cbbL and cbbS genes near csoSCA homologs (Figure 4).
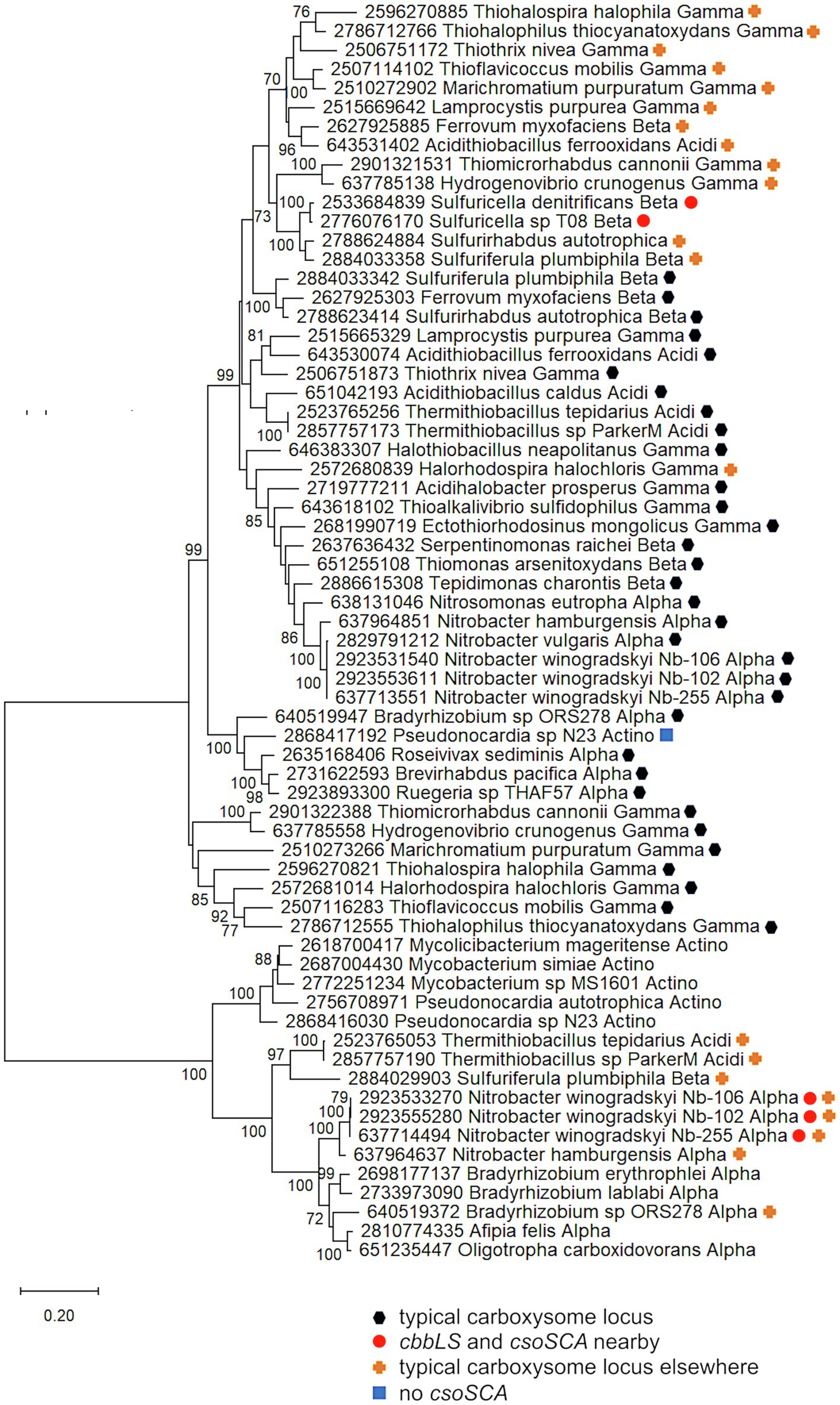
Figure 4. Ribulose 1,5-carboxylase/oxygenase subunits (CbbL and CbbS) encoded by genes collocated with csoSCA homologs. Maximum likelihood analysis of CbbL and CbbS sequences was undertaken on sequences gathered from the IMG database, aligned by MUSCLE in MEGA 11, and trimmed via GBLOCKS (Edgar, 2004; Talavera and Castresana, 2007; Tamura et al., 2021). CbbL and CbbS alignments were then concatenated using the FASTA alignment joiner feature at FABOX (https://birc.au.dk/~palle/php/fabox/index.php), resulting in an alignment of 527 residues. The tree was constructed with partial deletion of gaps (95% cut-off) and the Le_Gascuel model (Le and Gascuel, 2008; discrete Gamma distribution with five categories, gamma parameter = 0.5775, 17.46% of sites evolutionarily invariant; this model had the lowest AIC calculated via the Find Best DNA/Protein Models feature in MEGA 11; Hurvich and Tsai, 1989; Akaike, 1998). Branch lengths are proportional to the number of substitutions (scale bar = substitutions per site). Bootstrap values are based on 500 resamplings of the alignment, with values <70% omitted. Taxon labels include abbreviated names of classes of Proteobacteria (Alpha, Beta, Gamma, and Delta; Acidi = Acidithiobacillia), and members of Actinobacteria (Actino). Taxon names also include symbols indicating the position of cbbL and cbbS genes relative to carboxysome-related genes, if present in the genomes. “Typical carboxysome locus” indicates that the cbbL and cbbS genes are part of a typical carboxysome locus, “cbbLS and csoSCA nearby” indicates that genes encoding RubisCO and a CsoSCA homolog are juxtaposed on the genome, “typical carboxysome locus elsewhere” indicates that a typical carboxysome locus is present elsewhere on the genome, and “No CsoSCA” indicates that the carboxysome locus lacks csoSCA.
For both the Alphaproteobacteria and Betaproteobacteria, if these genomically juxtaposed cbbL, cbbS, and csoSCA genes are the fragments of a single degraded carboxysome locus, one would anticipate that phylogenetic analysis would place them among genes encoding their carboxysomal cognates from taxonomically affiliated organisms. For the Nitrobacter spp. and Sulfuricella spp., the cbbLS genes cluster with noncarboxysomal RubisCO genes (Figure 4). The situation is more complicated for the csoSCA homologs (Figure 2). For the Nitrobacter spp., the csoSCA homologs appear to be recent duplicates of those present in the typical carboxysome loci in their genomes. For the Sulfuricella spp., the csoSCA homologs fall within the csoSCA2 clade and are unlikely to have arisen from carboxysome loci. Given the noncarboxysomal origin of the cbbLS genes in both classes, and csoSCA gene in the Sulfuricella spp., these are not fragments of a single degraded carboxysome locus.
Despite the likelihood that they do not share evolutionary history cohabitating carboxysomes, it is still possible that these two enzymes might function together in the cytoplasm to facilitate CO2 fixation in their host organisms, all of which are capable of autotrophic growth (Winogradsky, 1892; Kojima and Fukui, 2010). Coregulation is possible for both, but seems more likely for the members of Nitrobacter, since their genes are <2 kb apart (Figure 1). The juxtaposition of noncarboxysomal RubisCO genes to those encoding typical β-carbonic anhydrase has been noted for two members of Hydrogenovibrio (Yoshizawa et al., 2004; Scott et al., 2006), and is apparent in genome data from in many other members of Hydrogenovibrio and Thiomicrorhabdus,1 suggesting such juxtaposition may be selected for in some organisms. While the expression of cytoplasmic carbonic anhydrase results in a high CO2-requiring phenotype in organisms with CCMs (Price and Badger, 1989), there is evidence for carbonic anhydrase activity in the chloroplasts of certain algae and plants (reviewed in Moroney et al., 2001). If these enzymes do function together in Nitrobacter and Sulfuricella, perhaps the carbonic anhydrase facilitates RubisCO-mediated CO2 fixation by maintaining intracellular HCO3− and CO2 near chemical equilibrium, preventing RubisCO from diminishing the concentration of intracellular CO2 under conditions where CCMs are not induced (e.g., moderate environmental CO2 concentrations; Yoshizawa et al., 2004).
cbbL and cbbS Separate From csoS1, csoS2, csoSCA, and csoS4
Many members of family Thiobacillaceae (Boden et al., 2017; Boden, 2019) have csoS1–S4 genes in a separate genomic locus from cbbL and cbbS genes (Figure 1), as has previously been described for Thiobacillus denitrificans (Cannon et al., 2003; Beller et al., 2006a). Of the eight genome sequences from cultivated members of this family, all of which grow autotrophically (Boden et al., 2017; Boden, 2019), five include a homolog to csoS2 (Pfam012288; Annwoodia aquaesulis, Sulfuritortus calidifontis DSM103923 and J1A, Thiobacillus denitrificans ATCC25259, and Thiobacillus thioparus). In all cases, these csoS2 genes do not have cbbL and cbbS genes immediately upstream. Instead, cbbL and cbbS are located 2.6–21 kb away from csoS1-4 (Figure 1). The other three genome sequences lack csoS2 homologs; since these three sequences are incomplete (38–98 scaffolds), it is not possible to know whether csoS2 is truly absent from these organisms. Nine genomes (15–407 scaffolds) inferred to belong to members of Thiobacillaceae have been gathered from metagenomes, and five of these include csoS2 homologs. Three of these genes are present at the ends of scaffolds, making it impossible to determine whether cbbL and cbbS genes are nearby. For the two remaining (Thiobacillus spp. Bin4_E1B and BP01), cbbL and cbbS are encoded separately from csoS1–4. Based on these observations, it seems likely that having csoS1–4 genes apart from cbbL and cbbS genes may be a trait shared by all members of this family.
There are two mechanisms that could have resulted in the separate cbbLS and csoS1-4 loci found in members of Thiobacillaceae. In the first scenario, a typical ancestral carboxysome locus containing all of these genes was severed by genome re-arrangement. In the second scenario, cbbLS and csoS1-4 did not share an ancestral locus. Instead, carboxysomal cbbLS genes could have been lost from the genome entirely, and the cbbLS genes currently located 2–20 kb away are noncarboxysomal in origin. An additional possibility is that either cbbLS or csoS1-4 were acquired via horizontal gene transfer.
To provide evidence for different mechanisms for formation of cbbLS and csoS1-4 loci, phylogenetic analyses were conducted using concatenated alignments of cbbL and cbbS genes (cbbLS), and csoS2, S3, S4a, and S4b (csoS2-4). Genes encoding CsoS1A-C were omitted from these analyses, due to difficulties distinguishing the three types of csoS1A-C genes. The results of these analyses raise the possibility that cbbLS and csoS1-4 loci did not originate from a single ancestral typical carboxysome locus in these organisms (Figure 5). In Sf. calidifontis (here collapsed to strain J1A, since sequences for the two strains are identical), cbbLS genes fall in a small well-supported clade with Tb. denitrificans, and with other members of Thiobacillaceae in larger clades, though these larger clades are not as well-supported (Figure 5). The csoS2-4 genes from S. calidifontis fall among completely different taxa than its cbbLS genes, suggesting independent origins for its two loci. For all four isolates from Thiobacillaceae, cbbLS genes do not fall among those from typical carboxysome loci, though it should be noted that carboxysomal and non-carboxysomal cbbLS genes are not distinguished by two distinct, well-supported clades (Figure 5). Together, these observations suggest independent origins for cbbLS and csoS2-4 loci in Thiobacillaceae, but low bootstrap values for these phylogenetic analyses compromise the confidence of this assertion. If the cbbL and cbbS genes in members of Thiobacillaceae did not originate from a typical carboxysome locus, it would be very interesting to verify that they were capable of being packed into carboxysomes, as thus far it seems that noncarboxysomal RubisCO from other organisms cannot be packed into carboxysomes (Menon et al., 2008).
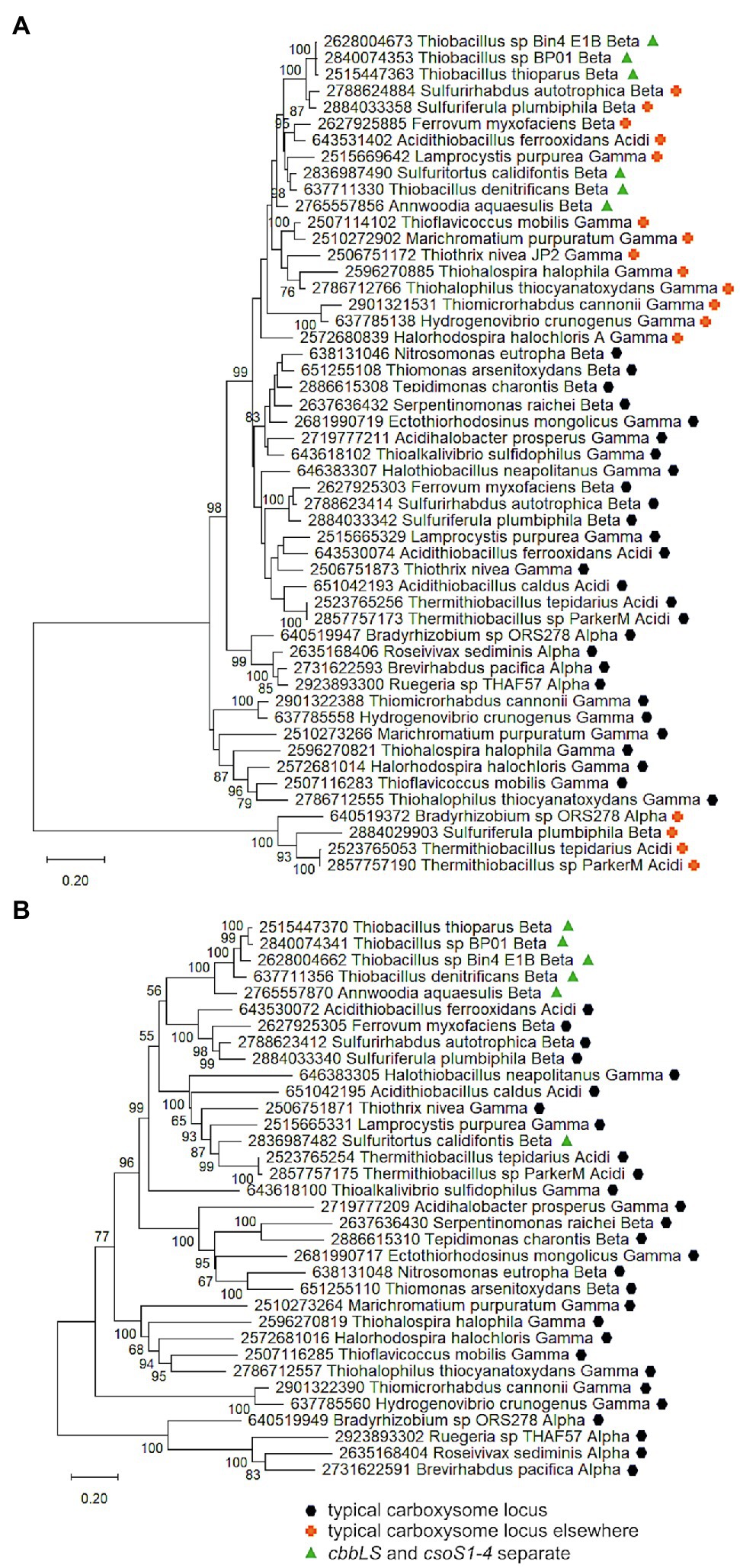
Figure 5. Analysis of RubisCO subunits (CbbL and CbbS) and carboxysome shell proteins (CsoS2, SCA, S4A, and S4B) that are encoded at two separate loci. Maximum likelihood analysis of amino acid sequences of (A). RubisCO subunits and (B). carboxysome shell proteins was undertaken on sequences that were gathered from the IMG database, aligned by MUSCLE in MEGA 11, and trimmed via GBLOCKS (Edgar, 2004; Talavera and Castresana, 2007; Tamura et al., 2021). CbbL and CbbS alignments were then concatenated using the FASTA alignment joiner feature at FABOX (https://birc.au.dk/~palle/php/fabox/index.php), as were CsoS2, SCA, S4A, and S4B, resulting in alignments of 550 (CbbLS) and 997 (CsoS2–4) residues. The trees were constructed with partial deletion of gaps (95% cut-off) and the Le_Gascuel model [Le and Gascuel, 2008; discrete Gamma distribution with five categories, gamma parameter = 0.5298, 17.64% of sites evolutionarily invariant (CbbLS); gamma parameter = 1.2921, 7.86% of sites evolutionarily invariant (CsoS2-4); this model had the lowest AIC calculated via the Find Best DNA/Protein Models feature in MEGA 11; Hurvich and Tsai, 1989; Akaike, 1998]. Branch lengths are proportional to the number of substitutions (scale bar = substitutions per site). Bootstrap values are based on 500 resamplings of the alignment, with values <70% omitted. Taxon labels include abbreviated names of classes of Proteobacteria (Alpha, Beta, Gamma, and Delta; Acidi = Acidithiobacillia). Taxon names also include symbols indicating the position of genes relative to carboxysome-related genes, if present in the genomes. “Typical carboxysome locus” indicates that the genes are part of a typical carboxysome locus, “cbbLS and csoS1-4 separate” indicates that genes encoding RubisCO and CsoS1–4 are encoded by separate loci, and “typical carboxysome locus elsewhere” indicates that a typical carboxysome locus is present elsewhere on the genome.
Currently, evidence for the presence of carboxysomes in members of Thiobacillaceae is limited to Tb. thioparus. Transmission electron micrographs have only been published for Tb. thioparus and Tb. denitrificans; polyhedral inclusions are apparent in Tb. thioparus cells, but not in Tb. denitrificans (Shively et al., 1970). Given the synteny of the carboxysome loci among Thiobacillus sp. Bin4 E1B, Thiobacillus sp. BP01, and Tb. thioparus, as well as the placement of their cbbLS and csoS2-4 genes together in clades (Figure 4), it seems likely that all three of these organisms are capable of synthesizing carboxysomes. For Tb. denitrificans, the absence of carboxysomes despite the presence of csoS1-4 is puzzling, and cannot be attributed to strain-level differences, since both ultrastructure and genome sequence were obtained from the same strain (ATCC25259). Perhaps their synthesis could be induced under growth conditions different from those used to cultivate the cells for ultrastructural study.
If carboxysomes are indeed synthesized by these organisms, one possible advantage of having separate loci would be independent regulation of cbbLS and csoS1-4 loci. Transcriptome analysis of Tb. denitrificans is consistent with this possibility. Based on hybridization with microarrays, transcripts of the Tb. denitrificans cbbL and cbbS genes are particularly abundant under aerobic conditions, but no such changes are apparent for csoS1-4 (Beller et al., 2006b). Other organisms have two sets of cbbLS, with one in a typical carboxysome locus and the other located elsewhere on the genome. These organisms synthesize noncarboxysomal RubisCO when CO2 concentrations are moderate, and selectively synthesize carboxysomal RubisCO and shell proteins when CO2 concentrations are very low (Yoshizawa et al., 2004). Perhaps some members of Thiobacillaceae upregulate the cbbLS locus when CO2 concentrations are low to moderate, and reserve upregulation of the csoS1-4 locus for low CO2 conditions, or other circumstances where carboxysomes facilitate growth.
No csoSCA
Carboxysome loci lacking csoSCA genes arose multiple times; they are present in some autotrophic organisms from Beta- and Gammaproteobacteria. A carboxysome locus lacking csoSCA is also present in Pseudonocardia sp. N23, a member Actinobacteria (Figure 1); though it has not been determined whether this organism could grow autotrophically, other members of its genus can (e.g., Pseudonocardia autotrophica; Takamiya and Tubaki, 1956). None of these organisms have csoSCA homologs elsewhere in their genomes (aside from Thiomicrorhabdus sediminis, which has a copy in its “typical” carboxysome operon). In Betaproteobacteria, they are present in Nitrosospira muliformis and also Nitrosospira spp. Nsp5 and Nsp6, which may be strains of Nsp. multiformis, based on average nucleotide identities >99% (Richter and Rosselló-Móra, 2009). Within Gammaproteobacteria, they appear to have arisen independently three times. All members of Thiomicrospira have carboxysome loci lacking csoSCA. Within Thiomicrorhabdus, such loci seem to have arisen twice. In Thiomicrorhabdus sediminis, two carboxysome loci are present; one is typical, while the second appears to be a recent duplicate of the typical locus. The amino acid sequences predicted from both cbbL and cbbS genes are 100% identical. Both copies of CsoS2 are 100% identical at amino termini; however, at residue 330, they diverge, and this continues to the carboxy termini. CsoS1 sequences also are identical at the amino termini and have small differences at their carboxy termini. The carboxysome locus from Thiomicrorhabdus aquaedulcis does not fall within a clade with those from other members of its genus (Figure 6), suggesting that it may have been acquired via horizontal gene transfer.
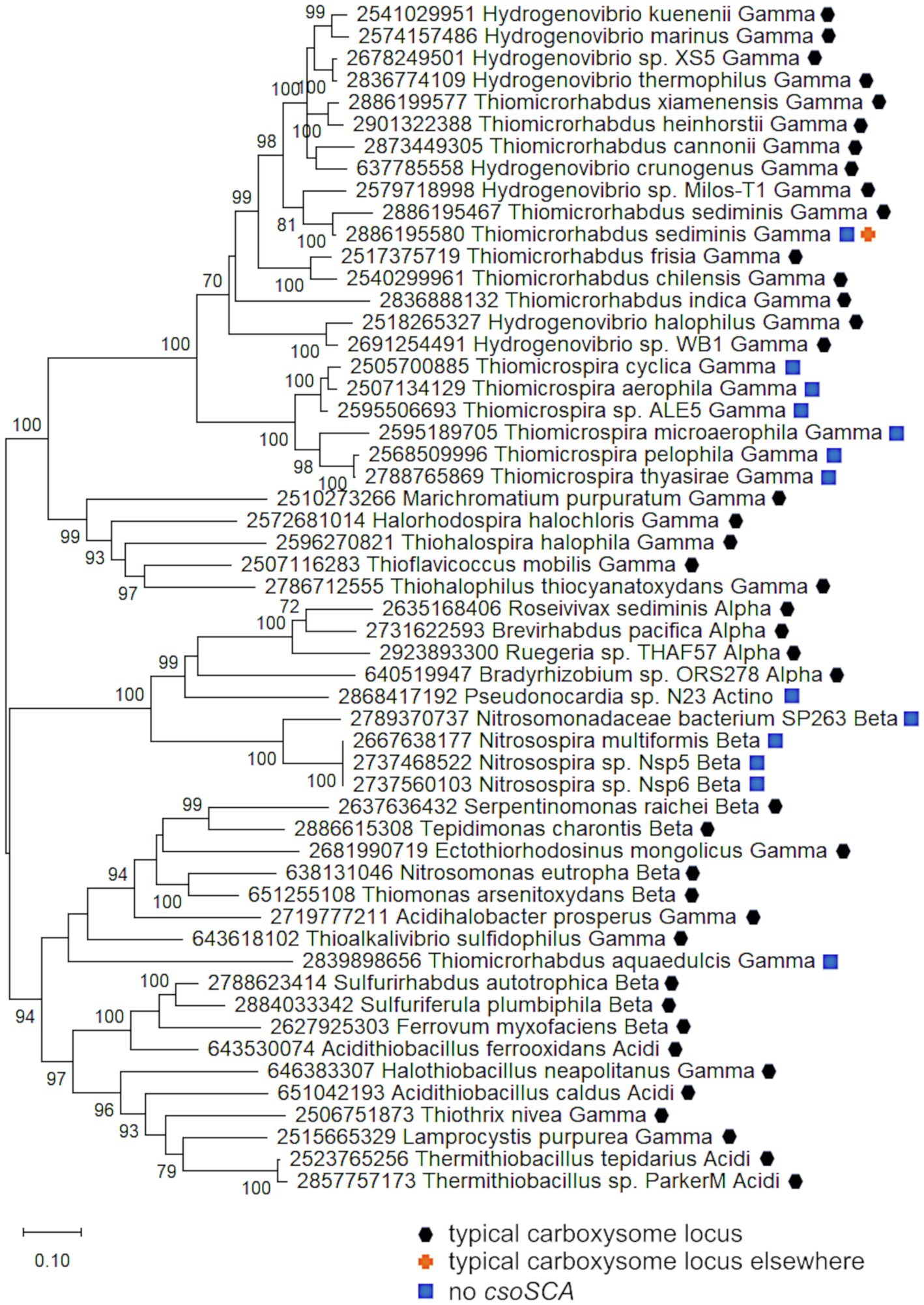
Figure 6. Carboxysome loci lacking csoSCA genes. Maximum likelihood analysis was undertaken on amino acid sequences of RubisCO subunits (CbbL and CbbS) and carboxysome shell proteins (CsoS2, S4A, and S4B) gathered from the IMG database, aligned by MUSCLE in MEGA 11, and trimmed via GBLOCKS (Edgar, 2004; Talavera and Castresana, 2007; Tamura et al., 2021). CbbL, CbbS, CsoS2, CsoS4A, and CsoS4B alignments were then concatenated using the FASTA alignment joiner feature at FABOX (https://birc.au.dk/~palle/php/fabox/index.php), resulting in an alignment of 940 residues. The trees were constructed with partial deletion of gaps (95% cut-off) and the Le_Gascuel model (Le and Gascuel, 2008; discrete Gamma distribution with five categories, gamma parameter = 0.7037, 16.40% of sites evolutionarily invariant; this model had the lowest AIC calculated via the Find Best DNA/Protein Models feature in MEGA 11; Hurvich and Tsai, 1989; Akaike, 1998). Branch lengths are proportional to the number of substitutions (scale bar = substitutions per site). Bootstrap values are based on 500 resamplings of the alignment, with values <70% omitted. Taxon labels include abbreviated names of classes of Proteobacteria (Alpha, Beta, Gamma, and Delta; Acidi = Acidithiobacillia) and members of Actinobacteria (Actino). Taxon names also include symbols indicating the position of genes relative to carboxysome-related genes, if present in the genomes. “Typical carboxysome locus” indicates that the genes are part of a typical carboxysome locus, “typical carboxysome locus elsewhere” indicates that a typical carboxysome locus is present elsewhere on the genome, and “No CsoSCA” indicates that the carboxysome locus lacks csoSCA.
The carboxysome locus from Pseudonocardia sp. N23 includes cbbL and cbbS genes distinct from those present in other members of phylum Actinobacteria (Figure 4). Other members of this phylum carry cbbL and cbbS, and Pseudonocardia sp. N23 does include a copy that falls within a clade of these sequences (Figure 4). However, the cbbL and cbbS genes present in the carboxysome locus, as well as csoS2, csoS4A, and csoS4B, fall among genes from carboxysome loci from members of Alpha- and Betaproteobacteria (Figures 4, 6), suggesting this locus was acquired via horizontal gene transfer.
It is apparent that these carboxysome loci originated from typical carboxysome loci, given that they cluster with others that contain csoSCA genes (Figure 6). Indeed, in members of Thiomicrospira, a gene is present between csoS2 and csoS4A (Figure 1), which is likely to be a degraded form of csoSCA. In Thiomicrospiras pelophila, thyasirae, and microaerophila, these genes do match Pfam08936 (csoSCA), but e-values range from 0.006 to 4.5e−05, and none of the residues necessary for carbonic anhydrase activity are present. However, the amino termini of the proteins predicted from these genes align well with those from CsoSCA proteins. Given that the amino termini of CsoSCA proteins may facilitate interactions among carboxysome proteins (Blikstad et al., 2021), perhaps these degraded genes may still encode proteins that facilitate packing of RubisCO molecules into carboxysomes.
There is evidence that these carboxysome loci are transcribed and translated. Carboxysome locus genes are transcribed in Tms. pelophila (Scott et al., 2019), carboxysomes are visible in transmission electron micrographs of members of Thiomicrospira (Sorokin et al., 2001, 2002a,b; Scott et al., 2019), and have been purified from Tms. thyasirae (Lanaras et al., 1991). Electron dark inclusions are abundant in Nsp. muliformis, but staining patterns suggest these consist of glycogen (Watson et al., 1971). Ultrastructural studies of Pseudonocardia sp. N23, as well as Tmr. aquadulcis and sediminis, have not been published.
Given the presence of carboxysomes in at least some of these taxa, the conservation of residues necessary for the function of the CbbL, CbbS, CsoS2, and CsoS4A and B proteins, and the convergent evolution of this sort of carboxysome locus in multiple lineages of microorganisms, they are likely to be functional in their host organisms (however, see the comments on CsoS1 sequences from Pseudonocardia sp. N23 in Section “Do the Genes From Atypical Carboxysome Loci Encode Functional Proteins” above). The current understanding of carboxysome function requires the presence of carbonic anhydrase activity within these microcompartments in order for them to facilitate CO2 fixation by RubisCO (see above). One possibility is that these modified carboxysomes have shells that are permeable to CO2, allowing this gas to enter from the cytoplasm. CsoS4 proteins are necessary for carboxysome shell impermeability to CO2; the absence of CsoS4 to seal the vertices of their shells renders the microcompartments CO2-permeable (Cai et al., 2009). Their critical function perhaps accounts for their strong sequence conservation, hence redundancy, which is unusual for bacterial microcompartments that have multiple pentamer-forming paralogs (Melnicki et al., 2021). Interestingly, the carboxysome locus from Tmr. aquaedulcis lacks genes encoding CsoS4A, and the Tmr. sediminis locus lacking csoSCA lacks both csoS4A and csoS4B. Perhaps carboxysomes from these organisms operate without CsoS4 proteins, and are permeable to CO2. Given that carboxysome shells are assumed to require only 12 pentamers, and their pores are small (~4 Å in diameter), they are assumed to not play a significant role in metabolite conductance. However, a recent study of the protein stoichiometry of β-carboxysomes showed varying occupation of the vertices by the CcmL, the lone pentamer-forming gene product in beta carboxysome loci (Sun et al., 2019). The occupancy was correlated with environmental conditions, suggesting that pentamer association with shells is dynamic and perhaps serves as one way to alter permeability. Because Tmr. sediminis has two carboxysome loci (one typical, one lacking csoSCA, csoS4A, and csoS4B), determining the conditions under which it expresses typical, vs. atypical, carboxysomes could provide useful information about how its atypical carboxysomes might function, including whether pentamers and carbonic anhydrase are provided by the other locus. If these carboxysomes are permeable to CO2, cytoplasmic CO2 concentrations would need to be elevated in order to enhance RubisCO activity, running the risk of high rates of CO2 loss from the cells via diffusion, unless this loss is counterbalanced by living in a high CO2 habitat. These organisms have been cultivated in growth media supplemented with HCO3− (10–30 mM; Kojima and Fukui, 2019) or CO2 (20% headspace; Liu et al., 2021). For Tmr. sediminis, the lack of csoS4 genes suggests that this organism may not be capable of growth under low CO2 conditions, and it would be interesting to determine whether this is the case.
The other organisms lacking CsoSCA have loci including genes encoding CsoS1 and CsoS4; perhaps their shells are permeable to CO2 based on modifications to these two types of shell proteins. However, such differences are not detected when shell proteins from typical carboxysomes are compared to those from carboxysomes lacking carbonic anhydrase. For CsoS1ABC proteins, the sequence FVGGGY, corresponding to residues 40–45 from Htb. neapolitanus, comprises the narrowest part of the pore and the residues surrounding it (Tsai et al., 2007). In all of the CsoS1ABC sequences from atypical carboxysome loci lacking csoSCA, these residues are conserved, suggesting the pores have characteristics similar to those in typical carboxysomes. To determine whether there are other residues that vary systematically for these atypical carboxysome loci, and to detect changes in the sequence that are more likely due to the presence/absence of CsoSCA rather than evolutionary distance, CsoS1ABC sequences within Piscirickettsiaceae were compared, since genomes from this family include both typical (all 10 species of Hydrogenovibrio, 8/10 species from Thiomicrorhabdus) and atypical (2/10 species from Thiomicrorhabdus, all six members of Thiomicrospira) loci. Among all of these organisms, CsoS1ABC sequences are highly conserved throughout the sequences. Likewise, CsoS1D sequences from these organisms have small differences throughout, and mapping those differences on a homology model does not reveal significant patches of variability. CsoS4A and B sequences are also very similar across all three genera and there are no distinguishable differences between them. If these shell proteins actually are permeable to CO2, the mechanism mediating this change is not apparent from their sequences.
Another mechanism for preserving the activities of these carboxysomes would be their recruitment of a carbonic anhydrase encoded elsewhere on the genome, as may be the case for some β-carboxysomes from Cyanobacteria. β-carboxysomes carry homologs to γ-carbonic anhydrase (Dearaujo et al., 2014). In some cases, these homologs are enzymatically active as carbonic anhydrases, while in others, these homologs have apparently lost enzymatic activity (Cot et al., 2008), although the active site residues are intact. In these cases, the carboxysomes also carry a functional β-carbonic anhydrase (deeply divergent to CsoSCA; So et al., 2002; Cot et al., 2008; Rae et al., 2013), and the gene encoding this β-carbonic anhydrase is not present in or near the operon encoding the essential components of the carboxysome (Rae et al., 2013). Evaluation of these possibilities awaits purification of carboxysomes from organisms with carboxysome loci lacking csoSCA genes, to test the permeabilities of their shells and the potential presence of carbonic anhydrase activity within them.
Conclusion
The unusual carboxysome-related loci described here are common enough to suggest relevance. Genes encoding CsoSCA2 are extremely widespread. Colocalization of csoSCA homologs and cbbLS is present in genomes from two classes of Proteobacteria. “Split” carboxysome loci (cbbLS and csoS1-4) are likely present in all members of family Thiobacillaceae. Carboxysome loci lacking csoSCA homologs (or homologs unlikely to be active) are present in at least two classes of Proteobacteria and have been horizontally transferred to phylum Actinobacteria. Together, all of this indicates that modified carboxysome loci have been evolutionarily selected for in some lineages, and are not the tattered remnants of typical carboxysome loci, captured on their journey to degradation and loss. Understanding how the proteins encoded by these atypical carboxysome loci function could help us understand better how typical carboxysomes function (the exceptions that prove—or disprove—the rule), as well as the selective pressures driving their origins from the assembly of their components over time.
The nature of the selective advantage provided by these atypical loci is not apparent at this point. All of the organisms carrying these atypical carboxysome loci (except for csoSCA2) are chemolithoautotrophs, so these atypical loci are likely to play a role in CO2 fixation. The habitats from which they were isolated are very diverse, with CO2 concentrations ranging from extremely low (alkaline soda lakes; Sorokin et al., 2001, 2002a,b), to high (e.g., soils, marine sediments; Bock and Wagner, 2006; Kelly and Wood, 2006). Particularly for those organisms from low CO2 habitats, these atypical carboxysome loci are likely to play a role in CCMs. Consistent with this possibility, most of these organisms have genes for likely DIC transporters either associated with their atypical loci or elsewhere in their genomes (Scott et al., 2020). However, based on the current understanding of CCMs in bacteria, which requires that both RubisCO and carbonic anhydrase are present in carboxysomes, it is difficult to understand how organisms lacking carboxysomal RubisCO (as in section “cbbL and cbbS Separate From csoS1, csoS2, csoSCA, and csoS4” above) or carbonic anhydrase (as in section “No csoSCA” above) could have functioning CCMs. This conceptual gap may result from the relative paucity of studies on CCMs in organisms besides Cyanobacteria, in which CCMs have been well-studied (reviewed in Price et al., 2009). Though carboxysomes from chemolithoautotrophs have been well-studied (Kerfeld et al., 2010, 2018; Sutter et al., 2021), their integration with the other components of CCMs in these organisms (e.g., DIC transporters) has not. CCM function (carboxysome presence and elevated intracellular DIC concentration) has been demonstrated for only one bacterium beyond Cyanobacteria (Hydrogenovibrio crunogenus; Dobrinski et al., 2005). Upregulation of genes encoding both DIC transporters and carboxysomes under low DIC conditions has only been demonstrated for a handful of chemolithoautotrophic Gammaproteobacteria (Mangiapia et al., 2017; Desmarais et al., 2019; Scott et al., 2019). Despite this undersampling, it is already apparent that CCMs in Proteobacteria are more diverse than those from Cyanobacteria, in their reliance on a different arsenal of DIC transporters and multiple types of RubisCO (Dobrinski et al., 2012; Scott et al., 2019, 2020). Atypical carboxysomes could represent yet another layer of diversity in these CCMs; evaluating this possibility awaits further study of CCMs in these organisms as well as those in other members of Proteobacteria.
Author Contributions
KS contributed to conception and design of the manuscript. KS, USF GC 2020, USF GC 2021, MS, and CK ran the analyses. All authors contributed to manuscript writing and revision, and have read and approved the submitted version.
Funding
This work was supported by the National Science Foundation (NSF-MCB-1952676 to KS), and the Office of Science of the United States Department of Energy (DE-FG02-91ER20021 to MS and CK), and MSU AgBio Research (MS and CK).
Conflict of Interest
The authors declare that the research was conducted in the absence of any commercial or financial relationships that could be construed as a potential conflict of interest.
Publisher’s Note
All claims expressed in this article are solely those of the authors and do not necessarily represent those of their affiliated organizations, or those of the publisher, the editors and the reviewers. Any product that may be evaluated in this article, or claim that may be made by its manufacturer, is not guaranteed or endorsed by the publisher.
Acknowledgments
We are grateful to the University of South Florida for the resources to make it possible for the students in Genomics Class 2020 and 2021 to contribute to this manuscript. Genomics Class 2020 and 2021 are Salvador Ayala-Preciado, Alizain K. Bangash, Eleanor Brodrick, Deanna Byram, Madilyn Carney, Michael Colosi, Holly David, Gabriela Dolard, Anelys Figueroa, Christyn A. Geniesse, Lucy B. Gravitt, M. Andrew Glass, Rhiannon A. Hendrick, Arianna Henry, Thomas Henry, Alexis Hunt, Nicole Juern, Jason A. Kalathia, Taylor Kelsay, Jacob Kligman, Sydney T. Marshall, Ian M. Matosdeaza, Aaron S. Mohammed, Jeremias Munoz, Nicole Nauman, Dhanvi Patel, Alexandra Rodier, Beatriz Sanchez Uribazo, Logan Suits, Angel D. Valladares, Daniela Varon-Garcia, Alex Wagner, Lauren Wallace, and Jana Wieschollek.
Footnotes
References
Akaike, H. (1998). “Information theory and an extension of the maximum likelihood principle,” in Selected Papers of Hirotugu Akaike. eds. E. Parzen, K. Tanabe, and G. Kitagawa (New York, NY: Springer New York), 199–213.
Axen, S. D., Erbilgin, O., and Kerfeld, C. A. (2014). A taxonomy of bacterial microcompartment loci constructed by a novel scoring method. PLoS Comput. Biol. 10:e1003898. doi: 10.1371/journal.pcbi.1003898
Beller, H. R., Chain, P. S., Letain, T. E., Chakicherla, A., Larimer, F. W., Richardson, P. M., et al. (2006a). The genome sequence of the obligately chemolithoautotrophic, facultatively anaerobic bacterium Thiobacillus denitrificans. J. Bacteriol. 188, 1473–1488. doi: 10.1128/JB.188.4.1473-1488.2006
Beller, H. R., Letain, T. E., Chakicherla, A., Kane, S. R., Legler, T. C., and Coleman, M. A. (2006b). Whole-genome transcriptional analysis of chemolithoautotrophic thiosulfate oxidation by Thiobacillus denitrificans under aerobic versus denitrifying conditions. J. Bacteriol. 188, 7005–7015. doi: 10.1128/JB.00568-06
Blikstad, C., Dugan, E. J., Laughlin, T. G., Liu, M. D., Shoemaker, S. R., Remis, J. P., et al. (2021). Discovery of a carbonic anhydrase-Rubisco supercomplex within the alpha-carboxysome. bioRxiv [Preprint]. doi: 10.1101/2021.11.05.467472
Bock, E., and Wagner, M. (2006). “Oxidation of inorganic nitrogen compounds as an energy source” in The Prokaryotes. ed. M. Dworkin 3rd ed (New York: Springer), 457–495.
Boden, R. (2019). “Sulfuritortus” in Bergey's Manual of Systematics of Archaea and Bacteria. ed. W. B. Whitman (John Wiley & Sons, Inc.), 1–3.
Boden, R., Hutt, L. P., and Rae, A. W. (2017). Reclassification of Thiobacillus aquaesulis (Wood & Kelly, 1995) as Annwoodia aquaesulis gen. nov., comb. nov., transfer of Thiobacillus (Beijerinck, 1904) from the Hydrogenophilales to the Nitrosomonadales, proposal of Hydrogenophilalia class. nov. within the 'Proteobacteria', and four new families within the orders Nitrosomonadales and Rhodocyclales. Int. J. Syst. Evol. Microbiol. 67, 1191–1205. doi: 10.1099/ijsem.0.001927
Cai, F., Dou, Z., Bernstein, S. L., Leverenz, R., Williams, E. B., Heinhorst, S., et al. (2015). Advances in understanding carboxysome assembly in Prochlorococcus and Synechococcus implicate CsoS2 as a critical component. Life 5, 1141–1171. doi: 10.3390/life5021141
Cai, F., Heinhorst, S., Shively, J. M., and Cannon, G. C. (2008). Transcript analysis of the Halothiobacillus neapolitanus cso operon. Arch. Microbiol. 189, 141–150. doi: 10.1007/s00203-007-0305-y
Cai, F., Menon, B. B., Cannon, G. C., Curry, K. J., Shively, J. M., and Heinhorst, S. (2009). The pentameric vertex proteins are necessary for the icosahedral carboxysome shell to function as a CO2 leakage barrier. PLoS One 4:e7521. doi: 10.1371/journal.pone.0007521
Cannon, G. C., Baker, S. H., Soyer, F., Johnson, D. R., Bradburne, C. E., Mehlman, J. L., et al. (2003). Organization of carboxysome genes in the thiobacilli. Curr. Microbiol. 46, 115–119. doi: 10.1007/s00284-002-3825-3
Cannon, G. C., Heinhorst, S., Bradburne, C. E., and Shively, J. M. (2002). Carboxysome genomics: a status report. Funct. Plant Biol. 29, 175–182. doi: 10.1071/PP01200
Cannon, G. C., Heinhorst, S., and Kerfeld, C. A. (2010). Carboxysomal carbonic anhydrases: structure and role in microbial CO2 fixation. Biochim. Biophys. Acta 1804, 382–392. doi: 10.1016/j.bbapap.2009.09.026
Chen, I. A., Chu, K., Palaniappan, K., Pillay, M., Ratner, A., Huang, J., et al. (2019). IMG/M v.5.0: an integrated data management and comparative analysis system for microbial genomes and microbiomes. Nucleic Acids Res. 47, D666–D677. doi: 10.1093/nar/gky901
Cooper, T. G., and Filmer, D. (1969). The active species of "CO2" utilized by ribulose diphosphate carboxylase. J. Biol. Chem. 244, 1081–1083. doi: 10.1016/S0021-9258(18)91899-5
Cot, S. S. W., So, A. K. C., and Espie, G. S. (2008). A multiprotein bicarbonate dehydration complex essential to carboxysome function in cyanobacteria. J. Bacteriol. 190, 936–945. doi: 10.1128/JB.01283-07
Dearaujo, C., Arefeen, D., Tadesse, Y., Long, B. M., Price, G. D., Rowlett, R. S., et al. (2014). Identification and characterization of a carboxysomal g-carbonic anhydrase from the cyanobacerium Nostoc sp. PCC 7120. Photosynth. Res. 121, 135–150. doi: 10.1007/s11120-014-0018-4
Desmarais, J. J., Flamholz, A. I., Blikstad, C., Dugan, E. J., Laughlin, T. G., Oltrogge, L. M., et al. (2019). DABs are inorganic carbon pumps found throughout prokaryotic phyla. Nat. Microbiol. 4, 2204–2215. doi: 10.1038/s41564-019-0520-8
Dobrinski, K. P., Enkemann, S. A., Yoder, S. J., Haller, E., and Scott, K. M. (2012). Transcription response of the sulfur chemolithoautotroph Thiomicrospira crunogena to dissolved inorganic carbon limitation. J. Bacteriol. 194, 2074–2081. doi: 10.1128/JB.06504-11
Dobrinski, K. P., Longo, D. L., and Scott, K. M. (2005). A hydrothermal vent chemolithoautotroph with a carbon concentrating mechanism. J. Bacteriol. 187, 5761–5766. doi: 10.1128/JB.187.16.5761-5766.2005
Dou, Z., Heinhorst, S., Williams, E., Murin, E., Shively, J., and Cannon, G. (2008). CO2 fixation kinetics of Halothiobacillus neapolitanus mutant carboxysomes lacking carbonic anhydrase suggest the shell acts as a diffusional barrier for CO2. J. Biol. Chem. 283, 10377–10384. doi: 10.1074/jbc.M709285200
Edgar, R. C. (2004). MUSCLE: multiple sequence alignment with high accuracy and high throughput. Nucleic Acids Res. 32, 1792–1797. doi: 10.1093/nar/gkh340
Esparza, M., Jedlicki, E., Gonzalez, C., Dopson, M., and Holmes, D. S. (2019). Effect of CO2 concentration on uptake and assimilation of inorganic carbon in the extreme acidophile Acidithiobacillus ferrooxidans. Front. Microbiol. 10:603. doi: 10.3389/fmicb.2019.00603
Heinhorst, S., Williams, E., Cai, F., Murin, D., Shively, J., and Cannon, G. (2006). Characterization of the carboxysomal carbonic anhydrase CsoCSA from Halothiobacillus neapolitanus. J. Bacteriol. 188, 8087–8094. doi: 10.1128/JB.00990-06
Horken, K., and Tabita, F. R. (1999). Closely related form I ribulose bisphosphate carboxylase/oxygenase molecules that possess different CO2/O2 substrate specificities. Arch. Biochem. Biophys. 361, 183–194. doi: 10.1006/abbi.1998.0979
Hurvich, C. M., and Tsai, C. L. (1989). Regression and time series model selection in small samples. Biometrika 76, 297–307. doi: 10.1093/biomet/76.2.297
Jones, D. T., Taylor, W. R., and Thornton, J. M. (1992). The rapid generation of mutation data matrices from protein sequences. Comput. Appl. Biosci. 8, 275–282. doi: 10.1093/bioinformatics/8.3.275
Jumper, J., Evans, R., Pritzel, A., Green, T., Figurnov, M., Ronneberger, O., et al. (2021). Highly accurate protein structure prediction with AlphaFold. Nature 596, 583–589. doi: 10.1038/s41586-021-03819-2
Kelly, D. P., and Wood, A. P. (2006). “The chemolithotrophic prokaryotes” in The Prokaryotes. ed. M. Dworkin 3rd ed. (New York: Springer), 441–456.
Kerfeld, C. A., Aussignargues, C., Zarzycki, J., Cai, F., and Sutter, M. (2018). Bacterial microcompartments. Nat. Rev. Microbiol. 16:277. doi: 10.1038/nrmicro.2018.10
Kerfeld, C. A., Heinhorst, S., and Cannon, G. C. (2010). Bacterial microcompartments. Annu. Rev. Microbiol. 64, 391–408. doi: 10.1146/annurev.micro.112408.134211
Kerfeld, C. A., and Melnicki, M. R. (2016). Assembly, function and evolution of cyanobacterial carboxysomes. Curr. Opin. Plant Biol. 31, 66–75. doi: 10.1016/j.pbi.2016.03.009
Kinney, J. N., Axen, S. D., and Kerfeld, C. A. (2011). Comparative analysis of carboxysome shell proteins. Photosynth. Res. 109, 21–32. doi: 10.1007/s11120-011-9624-6
Klein, M. G., Zwart, P., Bagby, S. C., Cai, F., Chisholm, S. W., Heinhorst, S., et al. (2009). Identification and structural analysis of a novel carboxysome shell protein with implications for metabolite transport. J. Mol. Biol. 392, 319–333. doi: 10.1016/j.jmb.2009.03.056
Kojima, H., and Fukui, M. (2010). Sulfuricella denitrificans gen. nov., sp. nov., a sulfur-oxidizing autotroph isolated from a freshwater lake. Int. J. Syst. Evol. Microbiol. 60, 2862–2866. doi: 10.1099/ijs.0.016980-0
Kojima, H., and Fukui, M. (2019). Thiomicrorhabdus aquaedulcis sp. nov., a sulfur-oxidizing bacterium isolated from lake water. Int. J. Syst. Evol. Microbiol. 69, 2849–2853. doi: 10.1099/ijsem.0.003567
Lanaras, T., Cook, C. M., Wood, A. P., Kelly, D. P., and Codd, G. A. (1991). Purification of ribulose 1,5-bisphosphate carboxylase/oxygenase from Thiobacillus thyasiris the putative symbiont of Thyasira flexuosa (Montagu). Arch. Microbiol. 156, 338–343. doi: 10.1007/BF00248707
Le, S. Q., and Gascuel, O. (2008). An improved general amino acid replacement matrix. Mol. Biol. Evol. 25, 1307–1320. doi: 10.1093/molbev/msn067
Liu, X., Chen, B., Lai, Q., Shao, Z., and Jiang, L. (2021). Thiomicrorhabdus sediminis sp. nov. and Thiomicrorhabdus xiamenensis sp. nov., novel sulfur-oxidizing bacteria isolated from coastal sediments and an emended description of the genus Thiomicrorhabdus. Int. J. Syst. Evol. Microbiol. 71:4660. doi: 10.1099/ijsem.0.004660
Long, B. M., Rae, B. D., Rolland, V., Förster, B., and Price, G. D. (2016). Cyanobacterial CO2-concentrating mechanism components: function and prospects for plant metabolic engineering. Curr. Opin. Plant Biol. 31, 1–8. doi: 10.1016/j.pbi.2016.03.002
Mangiapia, M., Microbialphysiology, U., Brown, T.-R. W., Chaput, D., Haller, E., Harmer, T. L., et al. (2017). Proteomic and mutant analysis of the CO2 concentrating mechanism of hydrothermal vent chemolithoautotroph Thiomicrospira crunogena. J. Bacteriol. 199, e00871–e00916. doi: 10.1128/JB.00871-16
Melnicki, M. R., Sutter, M., and Kerfeld, C. A. (2021). Evolutionary relationships among shell proteins of carboxysomes and metabolosomes. Curr. Opin. Microbiol. 63, 1–9. doi: 10.1016/j.mib.2021.05.011
Menon, B. B., Dou, Z., Heinhorst, S., Shively, J. M., and Cannon, G. C. (2008). Halothiobacillus neapolitanus carboxysomes sequester heterologous and chimeric RubisCO species. PLoS One 3:e3570. doi: 10.1371/journal.pone.0003570
Menon, B. B., Heinhorst, S., Shively, J. M., and Cannon, G. C. (2010). The carboxysome shell is permeable to protons. J. Bacteriol. 192, 5881–5886. doi: 10.1128/JB.00903-10
Moroney, J., Bartlett, S., and Samuelsson, G. (2001). Carbonic anhydrases in plants and algae. Plant Cell Environ. 24, 141–153. doi: 10.1111/j.1365-3040.2001.00669.x
Oltrogge, L., Chaijarasphong, T., Chen, A., Bolin, E., Marqusee, S., and Savage, D. (2020). Multivalent interactions between CsoS2 and Rubisco mediate α-carboxysome formation. Nat. Struct. Mol. Biol. 27, 281–287. doi: 10.1038/s41594-020-0387-7
Price, G. D., and Badger, M. R. (1989). Expression of human carbonic anhydrase in the cyanobacterium Synechococcus PCC7942 creates a high CO2-requiring phenotype. Plant Physiol. 91, 505–513. doi: 10.1104/pp.91.2.505
Price, G. D., Badger, M. R., Woodger, F. J., and Long, B. M. (2009). Advances in understanding the cyanobacterial CO2-concentrating-mechanism (CCM): functional components, Ci transporters, diversity, genetic regulation and prospects for engineering into plants. J. Exp. Bot. 59, 1441–1461. doi: 10.1093/jxb/erm112
Rae, B. D., Long, B. M., Badger, M. R., and Price, G. D. (2013). Functions, compositions, and evolution of the two types of carboxysomes: polyhedral microcompartments that facilitate CO2 fixation in cyanobacteria and some proteobacteria. Microbiol. Mol. Biol. Rev. 77, 357–379. doi: 10.1128/MMBR.00061-12
Richter, M., and Rosselló-Móra, R. (2009). Shifting the genomic gold standard for the prokaryotic species definition. Proc. Natl. Acad. Sci. U. S. A. 106, 19126–19131. doi: 10.1073/pnas.0906412106
Roberts, E. W., Cai, F., Kerfeld, C. A., Cannon, G. C., and Heinhorst, S. (2012). Isolation and characterization of the Prochlorococcus carboxysome reveal the presence of the novel shell protein CsoS1D. J. Bacteriol. 194, 787–795. doi: 10.1128/JB.06444-11
Sawaya, M. R., Cannon, G. C., Heinhorst, S., Tanaka, S., Williams, E. B., Yeates, T. O., et al. (2006). The structure of beta-carbonic anhydrase from the carboxysomal shell reveals a distinct subclass with one active site for the price of two. J. Biol. Chem. 281, 7546–7555. doi: 10.1074/jbc.M510464200
Scott, K. M., Harmer, T. L., Gemmell, B. J., Kramer, A. M., Sutter, M., Kerfeld, C. A., et al. (2020). Ubiquity and functional uniformity in CO2 concentrating mechanisms in multiple phyla of bacteria is suggested by a diversity and prevalence of genes encoding candidate dissolved inorganic carbon transporters. FEMS Microbiol. Lett. 367:fnaa106. doi: 10.1093/femsle/fnaa106
Scott, K. M., Leonard, J., Boden, R., Chaput, D., Dennison, C., Haller, E., et al. (2019). Diversity in CO2 concentrating mechanisms among chemolithoautotrophs from the genera Hydrogenovibrio, Thiomicrorhabdus, and Thiomicrospira, ubiquitous in sulfidic habitats worldwide. Appl. Environ. Microbiol. 85, e02096–e03018. doi: 10.1128/AEM.02096-18
Scott, K. M., Sievert, S. M., Abril, F. N., Ball, L. A., Barrett, C. J., Blake, R. A., et al. (2006). The genome of deep-sea vent chemolithoautotroph Thiomicrospira crunogena. PLoS Biol. 4:e383. doi: 10.1371/journal.pbio.0040383
Shively, J. M., Decker, G. L., and Greenawalt, J. W. (1970). Comparative ultrastructure of the thiobacilli. J. Bacteriol. 101, 618–627. doi: 10.1128/jb.101.2.618-627.1970
So, A. K., Espie, G. S., Williams, E. B., Shively, J. M., Heinhorst, S., and Cannon, G. C. (2004). A novel evolutionary lineage of carbonic anhydrase (epsilon class) is a component of the carboxysome shell. J. Bacteriol. 186, 623–630. doi: 10.1128/JB.186.3.623-630.2004
So, A. K., Johnmckay, M., and Espie, G. S. (2002). Characterization of a mutant lackin carboxysomal carbonic anhydrase from the cyanobacterium Synechocystis PCC6803. Planta 213, 456–467. doi: 10.1007/s004250100638
Sorokin, D. Y., Gorlenko, V. M., Tourova, T. P., Tsapin, A. I., Nealson, K. H., and Kuenen, G. J. (2002a). Thioalkalimicrobium cyclicum sp. nov. and Thioalkalivibrio jannaschii sp. nov., novel species of haloalkaliphilic, obligately chemolithoautotrophic sulfur-oxidizing bacteria from hypersaline alkaline mono Lake (California). Int. J. Syst. Evol. Microbiol. 52, 913–920. doi: 10.1099/00207713-52-3-913
Sorokin, D. Y., Lysenko, A. M., Mityushina, L. L., Tourova, T. P., Jones, B. E., Rainey, F. A., et al. (2001). Thioalkalimicrobium aerophilum gen. nov., sp. nov. and Thioalkalimicrobium sibericum sp. nov., and Thioalkalivibrio versutus gen. nov., sp. nov., Thioalkalivibrio nitratis sp. nov., novel and Thioalkalivibrio denitrificans sp. nov., novel obligately alkaliphilic and obligately chemolithoautotrophic sulfur-oxidizing bacteria from soda lakes. Int. J. Syst. Evol. Microbiol. 51, 565–580. doi: 10.1099/00207713-51-2-565
Sorokin, D. Y., Tourova, T. P., Kolganova, T. V., Sjollema, K. A., and Kuenen, J. G. (2002b). Thioalkalispira microaerophila gen. nov., sp nov., a novel lithoautotrophic, sulfur-oxidizing bacterium from a soda lake. Int. J. Syst. Evol. Microbiol. 52, 2175–2182. doi: 10.1099/00207713-52-6-2175
Spreitzer, R. J. (2003). Role of the small subunit in ribulose-1,5-bisphosphate carboxylase/oxygenase. Arch. Biochem. Biophys. 414, 141–149. doi: 10.1016/S0003-9861(03)00171-1
Sun, Y., Wollman, A. J. M., Huang, F., Leake, M. C., and Liu, L. N. (2019). Single-organelle quantification reveals stoichiometric and structural variability of carboxysomes dependent on the environment. Plant Cell 31, 1648–1664. doi: 10.1105/tpc.18.00787
Sutter, M., Melnicki, M. R., Schulz, F., Woyke, T., and Kerfeld, C. A. (2021). A catalog of the diversity and ubiquity of bacterial microcompartments. Nat. Commun. 12:3809. doi: 10.1038/s41467-021-24126-4
Tabita, F. R. (1999). Microbial ribulose 1,5-bisphosphate carboxylase/oxygenase: a different perspective. Photosynth. Res. 60, 1–28. doi: 10.1023/A:1006211417981
Tabita, F. R., Hanson, T. E., Satagopan, S., Witte, B. H., and Kreel, N. E. (2008). Phylogenetic and evolutionary relationships of RubisCO and the RubisCO-like proteins and the functional lessons provided by diverse molecular forms. Philos. Trans. R. Soc. Lond. Ser. B Biol. Sci. 363, 2629–2640. doi: 10.1098/rstb.2008.0023
Takamiya, A., and Tubaki, K. (1956). A new form of Streptomyces capable of growing autotrophically. Arch. Mikrobiol. 25, 58–64. doi: 10.1007/BF00424890
Talavera, G., and Castresana, J. (2007). Improvement of phylogenies after removing divergent and ambiguously aligned blocks from protein sequence alignments. Syst. Biol. 56, 564–577. doi: 10.1080/10635150701472164
Tamura, K., Stecher, G., and Kumar, S. (2021). MEGA11: molecular evolutionary genetics analysis version 11. Mol. Biol. Evol. 38, 3022–3027. doi: 10.1093/molbev/msab120
Tanaka, S., Kerfeld, C. A., Sawaya, M. R., Cai, F., Heinhorst, S., Cannon, G. C., et al. (2008). Atomic-level models of the bacterial carboxysome shell. Science 319, 1083–1086. doi: 10.1126/science.1151458
Tsai, Y., Sawaya, M. R., Cannon, G. C., Cai, F., Williams, E. B., Heinhorst, S., et al. (2007). Structural analysis of CsoS1A and the protein shell of the Halothiobacillus neapolitanus carboxysome. PLoS Biol. 5:e144. doi: 10.1371/journal.pbio.0050144
Watson, S. W., Graham, L. B., Remsen, C. C., and Valois, F. W. (1971). Lobular, ammonia-oxidizing bacterium, Nitrosolobus multiformis nov. gen. nov. sp. Arch. Mikrobiol. 76, 183–203. doi: 10.1007/BF00409115
Watson, G. M. F., Yu, J.-P., and Tabita, F. R. (1999). Unusual ribulose 1,5-bisphosphate carboxylase/oxygenase of anoxic Archaea. J. Bacteriol. 181, 1569–1575. doi: 10.1128/JB.181.5.1569-1575.1999
Winogradsky, S. (1892). Contributions à la morphologie des organismes de la nitrification. St. Petersbourg: L'Institut Imperial de Medecine Experimentale.
Yoshizawa, Y., Toyoda, K., Arai, H., Ishii, M., and Igarashi, Y. (2004). CO2-responsive expression and gene organization of three ribulose-1,5-bisphosphate carboxylase/oxygenase enzymes and carboxysomes in Hydrogenovibrio marinus strain MH-110. J. Bacteriol. 186, 5685–5691. doi: 10.1128/JB.186.17.5685-5691.2004
Keywords: carboxysome, microcompartment, carbonic anhydrase, carbon dioxide fixation, autotroph
Citation: USF Genomics Class 2020, USF Genomics Class 2021, Sutter M, Kerfeld CA and Scott KM (2022) Atypical Carboxysome Loci: JEEPs or Junk? Front. Microbiol. 13:872708. doi: 10.3389/fmicb.2022.872708
Edited by:
Biswarup Mukhopadhyay, Virginia Tech, United StatesReviewed by:
James Ferry, The Pennsylvania State University (PSU), United StatesIris Maldener, University of Tübingen, Germany
John Albert Raven, University of Dundee, United Kingdom
Copyright © 2022 Sutter, Kerfeld and Scott. This is an open-access article distributed under the terms of the Creative Commons Attribution License (CC BY). The use, distribution or reproduction in other forums is permitted, provided the original author(s) and the copyright owner(s) are credited and that the original publication in this journal is cited, in accordance with accepted academic practice. No use, distribution or reproduction is permitted which does not comply with these terms.
*Correspondence: Kathleen M. Scott, a21zY290dEB1c2YuZWR1