- College of Veterinary Medicine, Northeast Agricultural University, Harbin, China
The number of co-infections with multiple porcine diarrhea viruses has increased in recent years. Inducing mucosal immunity through oral immunization is an effective approach for controlling these pathogens. To generate a multi-pathogen vaccine against viral co-infection, we employed the Lactobacillus vector platform, which was previously used to generate potent candidate vaccines against various diseases. Two strategies were used to test the protective efficiency of recombinant Lactobacillus against multiple diarrhea viruses. First, we used a mixture of recombinant Lactobacillus separately expressing antigens of transmissible gastroenteritis virus (TGEV), porcine epidemic diarrhea virus (PEDV), and porcine rotavirus (PoRV). Next, we used a recombinant Lactobacillus expressing an antigen fusion protein of the above viruses. Twenty-four newborn piglets were divided into three groups and orally immunized with a mixture of recombinant Lactobacillus, recombinant Lactobacillus expressing the antigen fusion protein, or sterile phosphate-buffered saline daily for seven consecutive days after birth. After immunization, the piglets were randomly selected from each group for oral administration of PEDV, and these piglets were then cohabited with piglets without PEDV infection for 7 days. The protective effect against PEDV was evaluated based on clinical symptoms, viral shedding, and intestinal pathological damage. Piglets immunized with recombinant Lactobacillus showed specific mucosal and humoral immune responses to the three viruses and were protected against severe diarrhea and intestinal pathology. Our results highlight the potential of an oral multi-pathogen vaccine based on Lactobacillus to prevent transmission and limit the severity of viral co-infection.
Introduction
Viral diarrhea, caused by enterovirus infections acquired via mucosal surfaces, is a problem in pig farms worldwide. Viral diarrhea causes high morbidity and mortality of neonatal piglets, resulting in large economic impacts (Sun et al., 2016). The main pathogens causing porcine viral diarrhea are porcine epidemic diarrhea virus (PEDV), porcine transmissible gastroenteritis virus (TGEV), and porcine rotavirus (PoRV), and, in recent years, the newly discovered porcine deltacoronavirus, porcine cristavirus, and swine acute diarrhea syndrome coronavirus (Song et al., 2015; Zhang et al., 2019; Wen et al., 2021). Co-infection with multiple viruses has been reported in clinical samples of porcine diarrhea in recent years (Liu et al., 2013; Wang et al., 2014; Chang et al., 2016). Therefore, the development of multi-vaccines is urgently required. However, few multi-vaccines are currently available on the market, and most are inactivated or attenuated vaccines administered by injection.
The fecal-oral route is thought to be the main mode of transmission of porcine diarrheal viruses, with the intestinal villus as the primary site of infection (Kim et al., 2011; Thomas et al., 2015; Jiang et al., 2016), where an effective mucosal immune response is the first line of defense for neutralizing pathogens (Mestecky, 1987; Mcghee et al., 1992; Nirmal et al., 2014). Secretory IgA (sIgA) plays a key role against pathogen invasion at mucosal sites by causing the agglutination and neutralization of bacteria, viruses, and toxins, as well as by stimulating T and B cells, enhancing mucosal immunity (Austin et al., 2003). Most sIgA is released into the gastrointestinal fluid, saliva, tears, urine, and other secretions and is then secreted to the mucosal surface together with secretions to exert immunity-related effects (Renegar et al., 2004). Mucosal vaccination not only induces a strong sIgA response to defend against viral infection at the mucosal surface but also produces systemic serum IgG to neutralize newly generated viruses (Thomas et al., 2015). A previous study on the effects of different administration routes (oral, intranasal, and intramuscular) on systemic and mucosal immune responses induced by PEDV infection suggested that oral inoculation generates more comprehensive immune responses compared to those by the other routes (Yza et al., 2019). This may be because mucosal vaccination can be achieved via oral vaccination with antigens that can stimulate immune routes similar to those of viral infection (Staats et al., 1994). In addition, mucosal vaccination is needle-free, comparatively convenient, has a lower risk of causing hypersensitivity reactions, and is cost-effective (Mantis et al., 2011). Therefore, oral mucosal vaccination is a promising method for preventing porcine viral diarrhea.
Nevertheless, the bottleneck in the development of oral vaccines is that denaturation of antigens in the stomach and intestinal tract prevents them from stimulating the intestinal mucosa (Renukuntla et al., 2013). To overcome this difficulty, bacterial delivery systems that can survive in adverse environments have been used to deliver antigens to the intestinal tract (Bhuyan et al., 2018). Safety is an important factor in qualified delivery systems, and food-grade lactic acid bacteria (LAB) are an excellent platform for fulfilling this requirement (Craig et al., 2019). Most LAB are “generally recognized as safe” according to the United States Food and Drug Administration and fulfill the criteria of the Qualified Presumption of Safety notion developed by the European Food Safety Authority (Daniel et al., 2011). In addition, LAB perform numerous beneficial functions, such as improving nutrient absorption, adhering to deleterious substances, increasing the immune response, and inhibiting viral replication (Martín et al., 2010). A previous study showed that LAB can survive in the presence of bile and low pH (Pfeiler et al., 2007; Wall et al., 2007) and thus can adapt to the conditions encountered during transit through the gastrointestinal tract. Therefore, many researchers have transformed LAB into delivery vectors for live vaccines to transport heterogeneous antigens (Rajoka et al., 2017; Chiabai et al., 2019; Liu et al., 2019; Natalia et al., 2019). In most studies, oral administration of the LAB vaccine elicited both antigen-specific systemic and sIgA immune responses that could eradicate the pathogen in post-immunization pathogen challenge models (Daniel et al., 2011). We previously demonstrated that recombinant Lactobacillus expressing PEDV or TGEV antigens induced specific mucosal and humoral immune responses in piglets (Liu et al., 2011; Hou et al., 2018). To prevent co-infection with porcine diarrhea virus, we developed a multi-vaccine based on Lactobacillus in this study.
The purpose of this study was to investigate the immunogenicity of Lactobacillus expressing multiple antigens simultaneously and to determine whether oral Lactobacillus multi-vaccines can prevent viral infection. Three types of porcine diarrhea viruses (TGEV, PEDV, and PoRV) were selected as research models. Two strategies (mixed group and fused group) were adopted to explore the feasibility and effectiveness of the Lactobacillus multi-vaccine. We observed humoral responses in the mucosa and systemically against all three viruses in newborn piglets. The piglets were protected against viral challenge and cohabitation infection following oral immunization with recombinant Lactobacillus casei. Notably, the effect of the mixed group was slightly better than that of the fused group. Our results suggest that using recombinant Lactobacillus is a promising vaccine strategy against coinfection with multiple viruses.
Materials and Methods
Strain, Plasmid, and Virus
Lactobacillus casei ATCC 393 was kindly provided by the Netherlands NIZO Institute and grown anaerobically in de Man, Rogosa, and Sharpe (MRS) broth at 37°C without shaking. The Lactobacillus constitutive expression plasmid pPG-T7g10-PPT (Song et al., 2014; encoded resistance to chloramphenicol and contained an HCE promoter, PgsA anchor, T7g10 enhancer, and rrnBT1T2 terminator), Escherichia coli pMD19-T-6Ds/TG1, pMD19-T-COE/TG1, and pMD19-T-VP4/TG1 were constructed and preserved in our laboratory; the PEDV GT/2017 strain, belonging to the G2b subtype, was isolated from clinical samples and identified in 2017 in our laboratory; the TGEV TH-98 and PoRV JL94 strains were isolated in our laboratory.
Animals
Twenty-four antibody-seronegative, healthy newborn piglets were purchased from the Acheng Experimental Practice Base of Northeast Agricultural University (Harbin, China). The piglets were breastfed until day 7 after birth and then fed with animal milk powder every 6 h. Animal studies were performed according to the regulations of the Animal Experiment Ethics Committee of the Northeast Agricultural University, China (review number: NEAUEC20210337).
Plasmids and Recombinant Strain Construction
A schematic diagram of the recombinant plasmid construction is shown in Figure 1. The details of primers used in this paper are shown in Table 1. Briefly, primers F1 and R1 were used to amplify the 6Ds gene using the plasmid pMD19-T-6Ds as a template. Primers F2 and R2 were used to amplify the VP4 gene using pMD19-T-VP4 as a template. Using plasmid pMD19-T-COE as a template, the primers F3/R3 and F4/R4 were used to amplify the COE gene, linker sequence, and SacI, KpnI, BamHI, SphI, and ApaI sites. After digesting the vector at restriction enzyme sites SacI and ApaI, the genes 6Ds, VP4, and COE were inserted into the expression plasmid pPG-T7g10-PPT, giving rise to recombinant plasmids pPG-T7g10-6Ds, pPG-T7g10-VP4, and pPG-T7g10-COE, respectively. The 6Ds fragment was inserted into the KpnI/BamHI sites of 19 T-simple-COE to generate the plasmid pMD19-T-6Ds-COE. The VP4 fragment was inserted into the SphI/ApaI sites of the recombinant plasmid pMD19-T-6Ds-COE to generate the plasmid pMD19-T-6Ds-COE-VP4. The 6Ds-COE-VP4 fragment was inserted into the SacI and ApaI sites of the expression plasmid pPG-T7g10-PPT to generate the plasmid pPG-T7g10-6Ds-COE-VP4. All four recombinant expression plasmids were verified using polymerase chain reaction (PCR) and sequencing. Finally, the recombinant plasmids were transformed into L. casei 393 via electroporation to generate the recombinant strains pPG-T7g10-6Ds/LC393, pPG-T7g10-COE/LC393, pPG-T7g10-VP4/LC393, and pPG-T7g10-6Ds-COE-VP4/LC393.
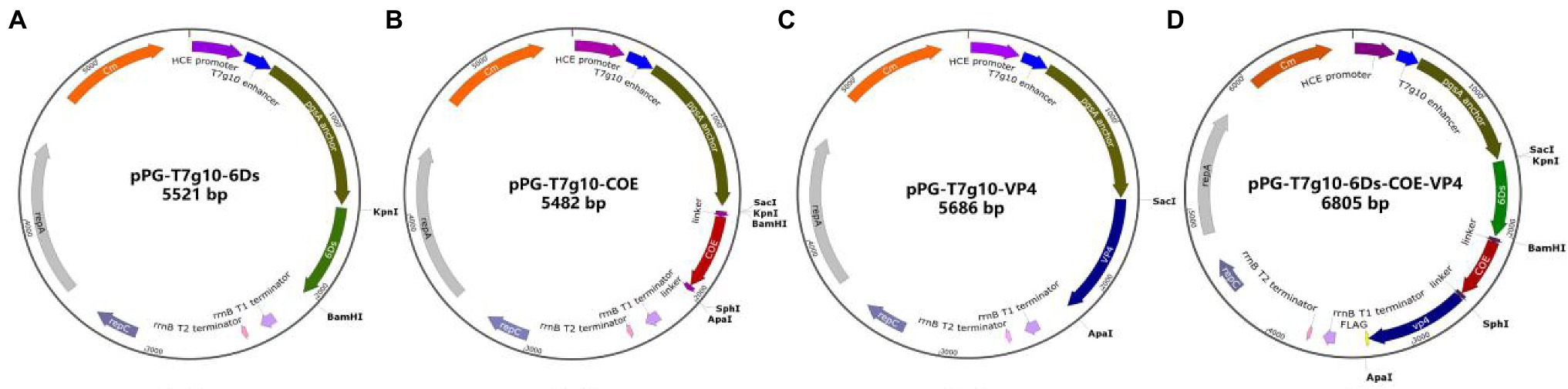
Figure 1. Schematic diagram showing the construction of recombinant plasmids. The fragments 6Ds, COE, VP4, and 6Ds-COE-VP4 were inserted into the vector pPG-T7g10-PPT digested by SacI and ApaI to generate the recombinant plasmids pPG-T7g10-6Ds (A), pPG-T7g10-COE (B), pPG-T7g10-VP4 (C), and pPG-T7g10-6Ds-COE-VP4 (D).
Identification of Antigen Expression in Recombinant Lactobacillus Using Western Blot
To analyze the expression of the proteins of interest in recombinant strains pPG-T7g10-6Ds/LC393, pPG-T7g10-COE/LC393, pPG-T7g10-VP4/LC393, and pPG-T7g10-6Ds-COE-VP4/LC393, the identified strains were cultured in MRS medium containing chloramphenicol (10 mg/ml) at 37°C for 16 h and then harvested by centrifugation (Heraeus Pico17 centrifuge, Thermo Fisher Scientific, Waltham, MA, United States) at 10000 × g for 2 min. After cell lysis with lysozyme (10 mg/ml) and washing with sterile deionized water, the bacterial precipitate was resuspended in phosphate-buffered saline (PBS) and mixed with 5× sodium dodecyl sulfate buffer to obtain the protein samples. These samples were subjected to western blotting using mouse anti-6Ds serum, rabbit anti-COE serum and rabbit anti-VP4 serum (1:200 dilution) prepared in our laboratory. Anti-FLAG tag mouse monoclonal antibody (1:1000 dilution) was used as the primary antibody, and horseradish peroxidase (HRP)-conjugated goat anti-mouse IgG and HRP-conjugated goat anti-rabbit IgG (1:5000 dilution) were used as secondary antibodies. Finally, the target protein was detected and visualized using western enhanced chemiluminescence substrate and visualized.
Identification of Antigen Expression in Recombinant Lactobacillus Using Immunofluorescence
To further evaluate the expression of the target protein, recombinant strains were cultured in MRS medium at 37°C for 16 h, and 500 μl of the culture was collected and centrifuged at 3500 × g for 5 min. The bacterial precipitate was washed three times with PBS, and the bacterial sediment was resuspended in mouse anti-6Ds serum, rabbit anti-COE serum, rabbit anti-VP4 serum, and anti-FLAG tag mouse monoclonal antibody (1:100 dilution) at 37°C for 1 h. Subsequently, the cells were incubated with fluorescein isothiocyanate-conjugated goat anti-mouse IgG and fluorescein isothiocyanate-conjugated goat anti-rabbit IgG (1:200 dilution) at 37°C for 45 min in the dark. The cells were visualized using fluorescence microscopy.
Animal Grouping and Immunizing Procedure
Recombinant Lactobacillus strains were cultured in MRS medium for 16 h without agitation, washed with sterile PBS, and resuspended in PBS to a final concentration of 1010 colony-forming units (CFU)/ml. Twenty-four newborn piglets were divided into three groups. Six piglets were orally immunized with a mixture of 2 × 1010 CFU/ml pPG-T7g10-6Ds/LC393, pPG-T7g10-COE/LC393, and pPG-T7g10-VP4/LC393 (mixed group); six piglets were orally immunized with 2 × 1010 CFU/ml pPG-T7g10-6Ds-COE-VP4/LC393 (fused group), and the remaining 12 piglets were orally administered 2 ml of sterile PBS (PBS group). The immunization program was conducted daily for seven consecutive days after birth.
Anti-TGEV/PEDV/PoRV Specific sIgA Levels of Immunized Piglets
After immunization, anal and nasal swabs of piglets were collected daily and soaked in 1 ml of cold sterile PBS at 4°C overnight. After centrifugation at 10000 × g for 10 min at 4°C, the supernatant was stored at −40°C. Specific sIgA levels in the samples were detected using enzyme-linked immunosorbent assay (ELISA) (Zhao et al., 2017). Briefly, polystyrene microtiter plates were coated with purified TGEV/PEDV/PoRV for 12 h at 4°C, washed three times with PBS containing 0.05% Tween-20 (PBST), blocked at 37°C for 2 h with 5% skim milk in PBST, washed with PBST, and incubated for 2 h at 37°C. After washing, commercial HRP-conjugated goat anti-porcine sIgA diluted to 1:5000 was added to the plate, incubated at 37°C for 1 h, and washed with PBST, followed by color development using 3,3′,5,5′ tetramethylbenzidine chromogenic solution at 37°C for 10 min. The reaction was stopped by adding 2 N H2SO4, and the optical density at 450 nm was determined using an ELISA reader (SpectraMax® ABS, Molecular Devices, Sunnyvale, CA, United States). Each sample was tested in triplicate.
Anti-TGEV/PEDV/PoRV Serum-Specific IgG Levels of Immunized Piglets
Blood flow from the anterior vena cava was collected from piglets in each group on days between 0 and 7 post-immunization. Serum was prepared, and serum-specific IgG responses were measured using ELISA. The method was the same as that for sIgA, but the secondary antibody was HRP-conjugated goat anti-porcine IgG (1:5000).
Grouping of Piglets
After 7 days of continuous immunization, piglets were randomly selected from the mixed, fused, and PBS groups (three piglets per group) for oral administration of 4 ml PEDV (107 of the median tissue culture infectious dose), and the piglets were grouped as shown in Figure 2.
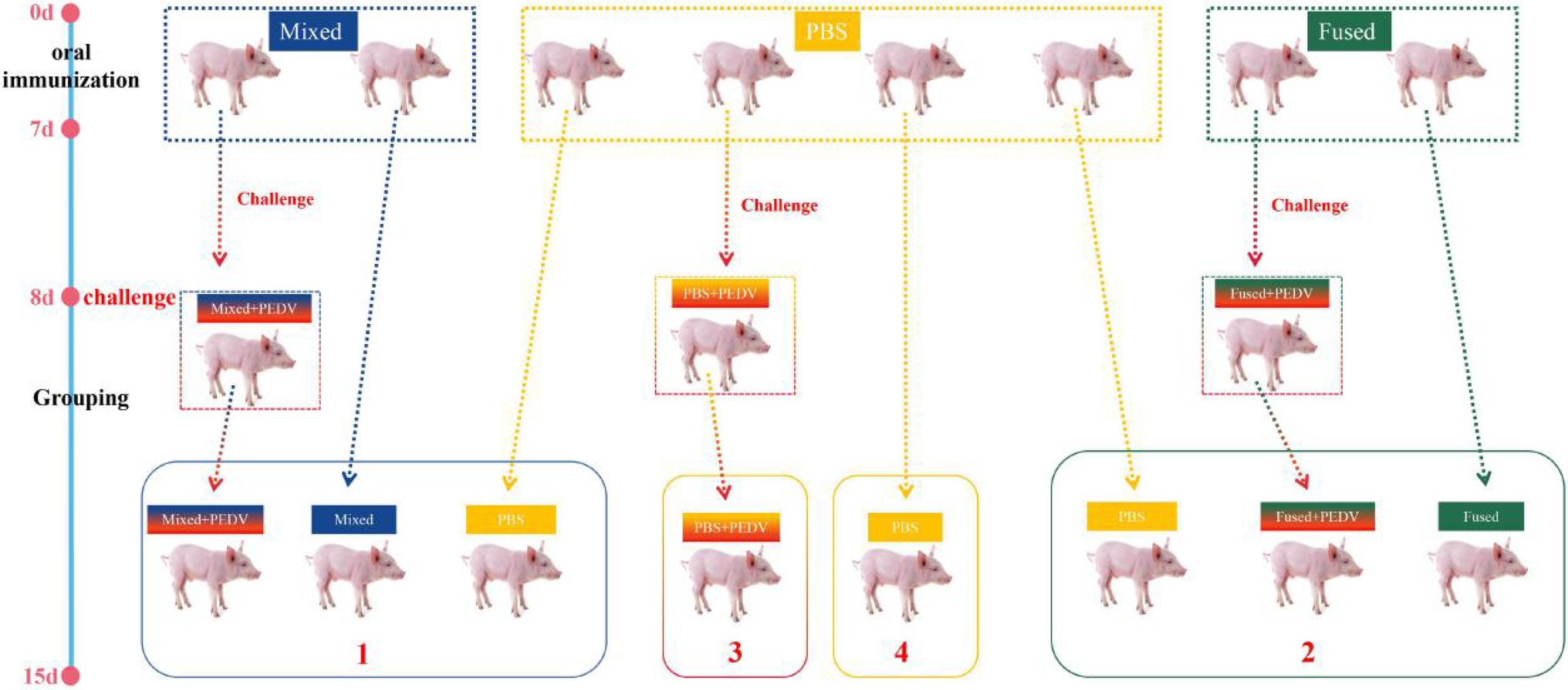
Figure 2. Schematic diagram of the immunization, challenge, and cohabitation of piglets. Twenty-four newborn piglets were divided into three groups for immunization. The immunization program was conducted daily for 7 consecutive days after birth. indicates three piglets. The blue dotted box indicates six piglets orally immunized with a mixture of 2 × 1010 CFU/ml pPG-T7g10-6Ds/LC393, pPG-T7g10-COE/LC393, and pPG-T7g10-VP4/LC393 (mixed group); the yellow dotted box indicates 12 piglets orally administered with PBS (PBS-group); and the green dotted box indicates 6 piglets orally immunized with 2 × 1010 CFU/ml pPG-T7g10-6Ds-COE-VP4/LC393 (fused-group).
indicates three piglets randomly selected from each group after immunization for PEDV challenge on day 8. The piglets were regrouped into four different isolators (numbers 1–4) for cohabiting until they were euthanized on day 15.
Clinical Symptoms and Weight Changes
The piglets were weighed prior to challenge and at 7 days post-challenge to calculate body weight gain, and the clinical symptoms of piglets were recorded daily and scored according to the guidelines in Table 2.
Virus Shedding Through Feces of Piglets
Anal swabs were collected from the piglets daily after the challenge, and virus in the anal swabs was detected using reverse transcription PCR (RT-PCR). Briefly, one anal swab from each pig was suspended in 1 ml PBS at 4°C overnight, and the supernatant was harvested by centrifugation at 5000 × g for 3 min. RNA was extracted and then reverse transcribed into cDNA, and PCR was performed using primers ORF3-F and ORF3-R.
Macroscopic Examination and Histopathology Assessment
All experimental piglets were euthanized on day 8 after challenge and dissected to observe lesions in the intestinal tract. In addition, jejunum samples were embedded in paraffin wax, and pathological sections were prepared and stained with hematoxylin and eosin.
Statistical Analysis
The data were analyzed using GraphPad Prism 6 software (GraphPad, Inc., La Jolla, CA, United States), and all data are expressed as the mean ± standard deviation. Data were analyzed using two-way analysis of variance, with at least three independent experiments. The results were considered as significant at p < 0.05 and highly significant at p < 0.01.
Results
Construction of Recombinant Expression Vectors of Lactobacillus
To improve the expression of antigens and ensure their localization on the surface of LAB, the previously constructed expression vector pPG-T7g10-PPT was used. This vector contains the enhancer T7g10 fragment, HCE constitutive promoter, and PgsA anchor sequence (Liu et al., 2011). The protective antigen (6Ds, COE, and VP4) genes of TGEV, PEDV, and PoRV were inserted into pPG-T7g10-PPT separately or combined into the same vector (Figure 1). Four types of recombinant lactobacilli expression vectors were identified using PCR and sequencing. The sequencing results showed that the above vectors were successfully constructed.
Expression of Viral Antigens in Recombinant Lactobacillus
Recombinant Lactobacillus was obtained by electroporation of the recombinant plasmid into Lactobacillus cells. Cell lysates of the recombinant strains were analyzed using western blotting after 16 h of culture. Correctly sized proteins were detected in all inserts of pPG-T7g10-6Ds/LC393, pPG-T7g10-COE/LC393, pPG-T7g10-VP4/LC393, and pPG-T7g10-6Ds-COE-VP4/LC393. The negative control, pPG-T7g10-PPT/LC393, did not display a corresponding immunoreactive band (Figure 3A). Immunofluorescence assays were performed to analyze the expression of the proteins of interest. As shown in Figure 3B, green fluorescence was observed for recombinant Lactobacillus but not for pPG-T7g10-PPT/LC393. These results indicate that the proteins of interest were successfully expressed in recombinant Lactobacillus.
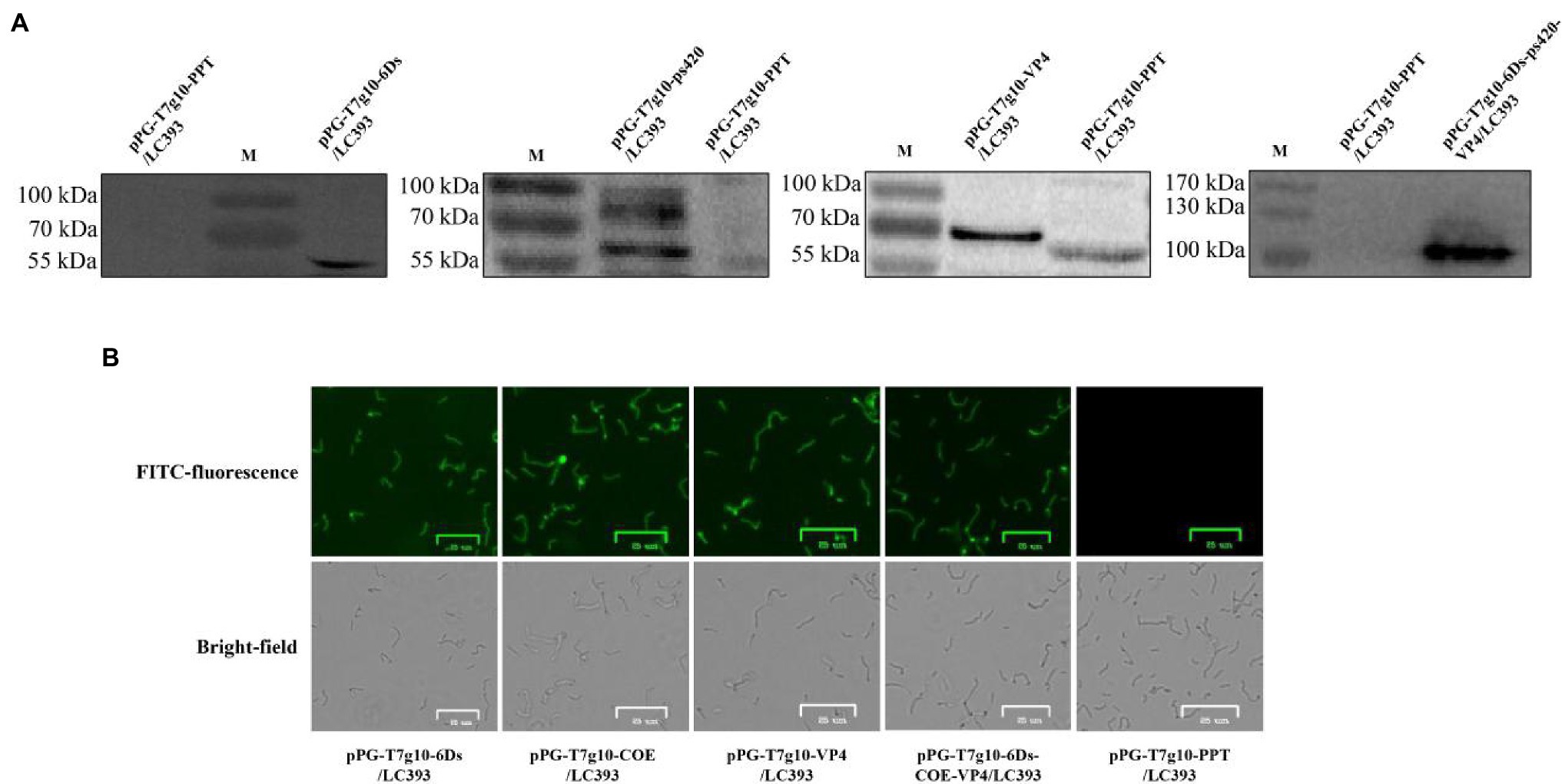
Figure 3. Identification of proteins of interest expressed in recombinant Lactobacillus. (A) Identification of the proteins of interest using western blotting. The relevant immunoreactive bands were detected in the cell pellets of strain pPG-T7g10-6Ds/LC393, pPG-T7g10-COE/LC393, pPG-T7g10-VP4/LC393, and pPG-T7g10-6Ds-COE-VP4/LC393 but not in those of strain pPG-T7g10-PPT/LC393. (B) Identification of proteins of interest using immunofluorescence assay. Green fluorescence was observed in strain pPG-T7g10-6Ds/LC393, pPG-T7g10-COE/LC393, pPG-T7g10-VP4/LC393, and pPG-T7g10-6Ds-COE-VP4/LC393 but not in strain pPG-T7g10-PPT/LC393.
Determination of Anti-TGEV/PEDV/PoRV-Specific sIgA Level of Immunized Piglets
Anti-TGEV/PEDV/PoRV-specific sIgA was assessed to evaluate the ability of recombinant Lactobacillus strains to induce mucosal immune responses in piglets. Anal and nasal swabs were collected from the piglets at 0–7 days post-immunization, and specific sIgA levels in the samples were detected using ELISA. As shown in Figure 4, anti-TGEV/PEDV/PoRV-specific sIgA in the nasal swabs and anal swabs post-immunization was detected in both the mixed group and fused group and gradually increased over time. No significant differences were observed in the PBS group before and after immunization. Anti-TGEV/PEDV/PoRV-specific sIgA levels in the nasal swabs and anal swabs from piglets in the mixed and fused groups were significantly higher than those in the PBS group from days 3 to 7 post-immunization (p < 0.05). The levels of sIgA in the mixed and fused groups did not exhibit significant differences; however, sIgA was produced earlier in the mixed group than in the fused group.
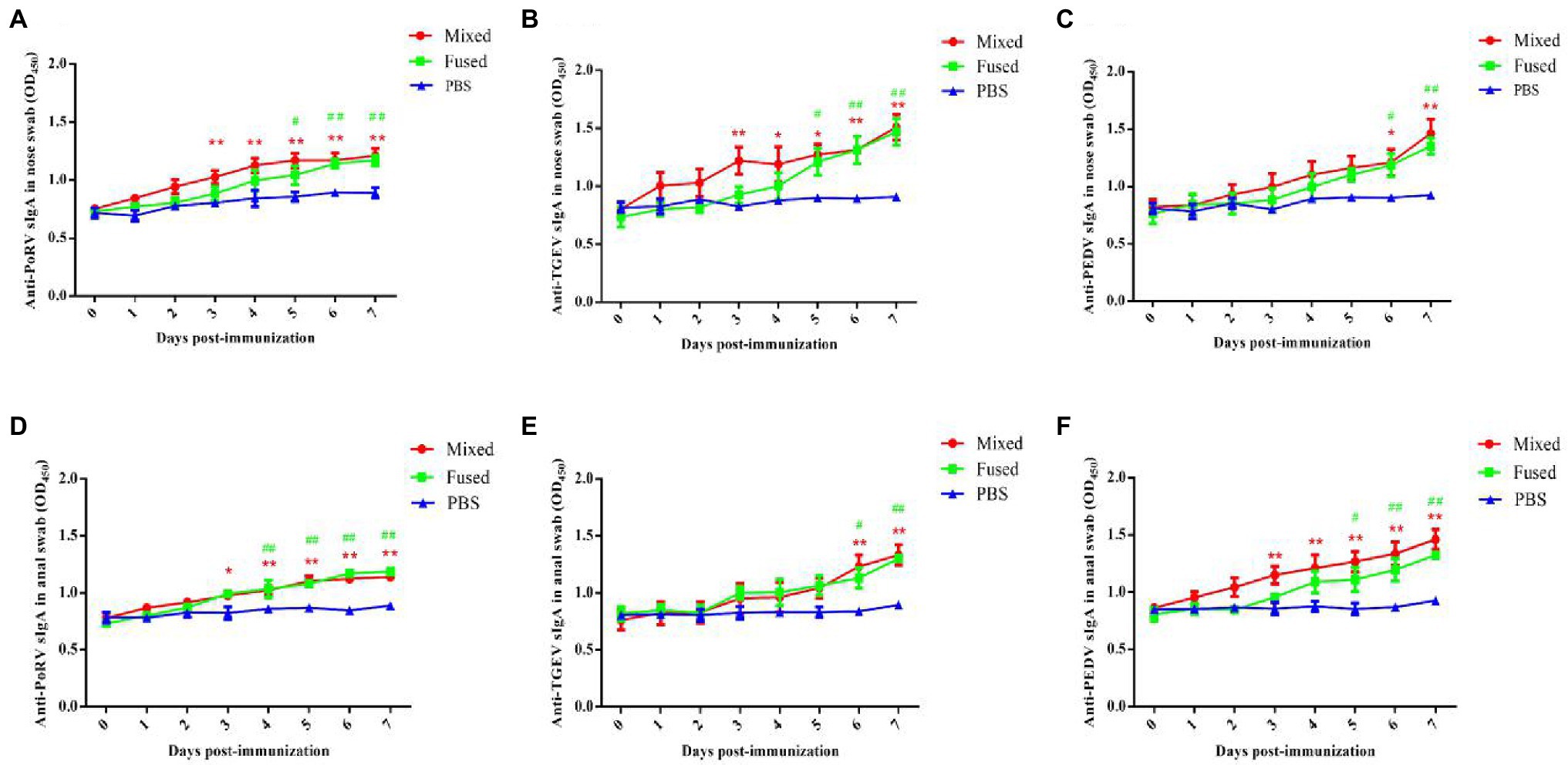
Figure 4. Levels of anti-TGEV/PEDV/PoRV specific sIgA in immunized piglets. The levels of anti-TGEV/PEDV/PoRV-specific sIgA was detected using ELISA. (A) Anti-PoRV specific sIgA in nose swab. (B) Anti-TGEV specific sIgA in nose swab. (C) Anti-PEDV specific sIgA in nose swab. (D) Anti-PoRV specific sIgA in anal swab. (E) Anti-TGEV specific sIgA in anal swab. (F) Anti-PEDV specific sIgA in anal swab. “*” Represents the comparison between mixed-group and PBS group, *p < 0.05, **p < 0.01. “#” represents the comparison between fused-group and PBS group, #p < 0.05, ##p < 0.01.
Determination of Anti-TGEV/PEDV/PoRV-Specific IgG Level in Serum of Immunized Piglets
Anti-TGEV/PEDV/PoRV-specific IgG was assessed to evaluate the ability of recombinant Lactobacillus to induce humoral immune responses in piglets. Twenty-four newborn piglets were divided into three groups for immunization (mixed, fused, and PBS groups). Serum collected from piglets between days 0 and 7 post-immunization was prepared, and serum-specific IgG responses were measured using ELISA. As shown in Figure 5, anti-TGEV/PEDV/PoRV-specific IgG was detected at day 7 post-immunization in both the mixed and fused groups. No significant differences were observed in the PBS group before and after immunization. The levels of serum anti-TGEV/PEDV/PoRV-specific IgG in the mixed and fused groups were significantly higher than those in the PBS group (p < 0.01). However, there was no significant difference between the mixed and fused groups.
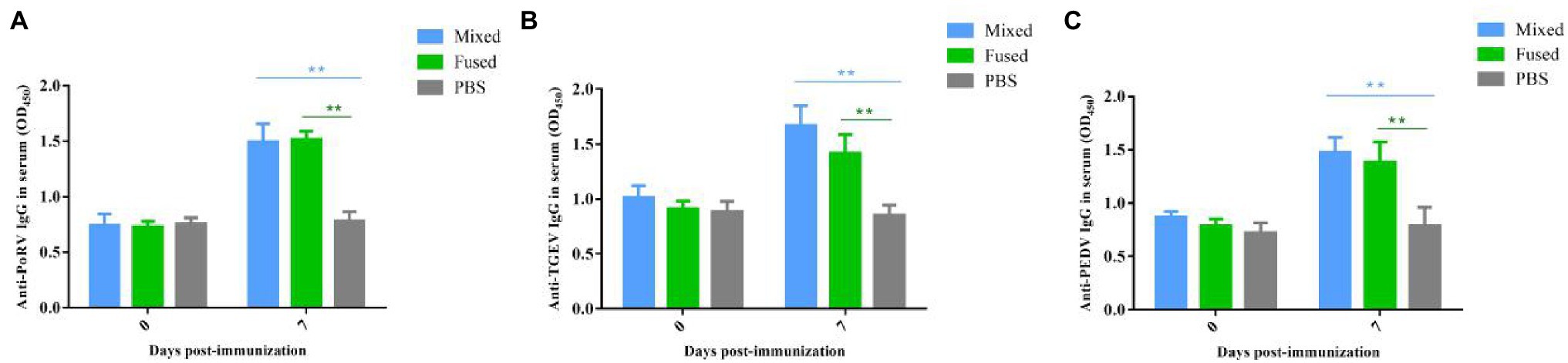
Figure 5. Levels anti-TGEV/PEDV/PoRV specific IgG in the serum of immunized piglets. Serum collected from piglets on days 0 and 7 post-immunization were prepared, and the serum specific IgG levels were measured using ELISA. (A) Anti-PoRV specific IgG in serum. (B) Anti-TGEV specific IgG in serum. (C) Anti-PEDV specific IgG in serum. **p < 0.01.
Clinical Symptoms and Weight Changes of Piglets After Challenge
The challenge test using PEDV as an example was carried out after seven consecutive days of immunization; the piglets were grouped as shown in Figure 2. The clinical symptoms of the piglets in each group were observed after challenge and scored as shown in Table 1. Briefly, the piglets that were orally administered PBS followed by PEDV challenge (PBS + PEDV3) showed typical PEDV infection symptoms, including acute watery diarrhea, depression, and drowsiness, with the most severe symptoms observed between days 4 and 5 after challenge (Figure 6A). The immunized piglets (mixed+PEDV1 and fused+PEDV2) had slight diarrhea and a normal appetite and showed a significant difference from the PBS + PEDV3 group after 2 days (Figure 6A). None of the cohabiting piglets (mixed1 and fused2) developed diarrhea during the experiment and showed significant differences from unimmunized piglets (PBS1 and PBS2). In addition, before and after challenge, the weight of immunized piglets was significantly higher than that of unimmunized piglets (Figure 6B).
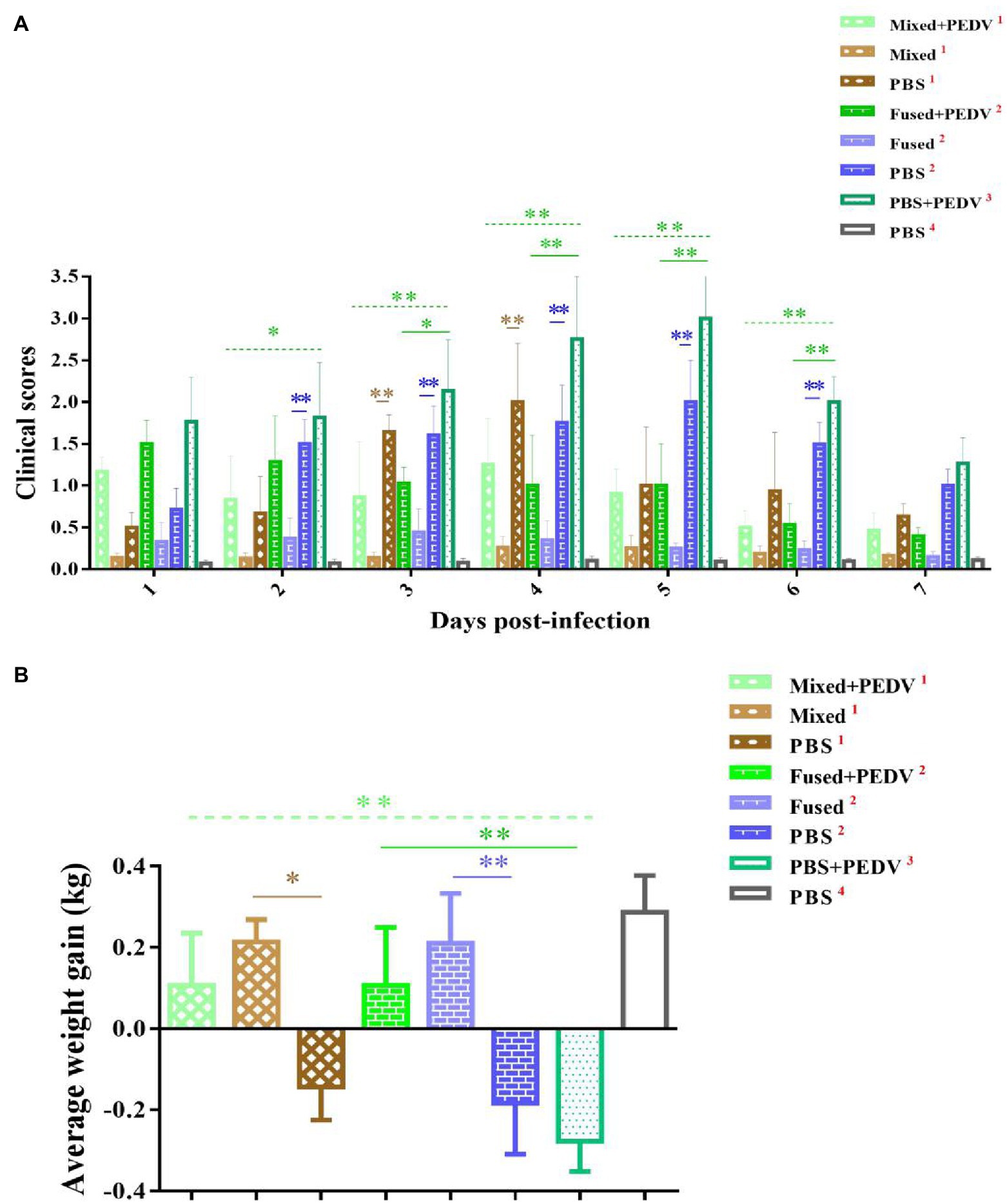
Figure 6. Clinical symptom scores and weight change of piglets. (A) Clinical symptom scores of piglets in different groups. (B) Average weight gain of piglets in different groups. *p < 0.05, **p < 0.01.
Analysis on the Regularity of Virus Shedding Through Feces of Piglets
Anal swabs were collected from the piglets daily after grouping, and PEDV RNA in the anal swabs was detected using RT-PCR. The PBS + PEDV3 group began shedding the virus on day 1, which continued for the next few days (Figure 7). However, PEDV RNA was detected at 1–4 days in immunized piglets (mixed+PEDV1 and fused+PEDV2) and then disappeared (Figure 7). Among the cohabiting piglets, PEDV RNA was detected in unimmunized piglets (PBS1 and PBS2) 2–4 days after cohabitation; almost no fecal virus shedding was observed in the mixed1 and fused2 groups (Figure 7). Oral immunization with recombinant Lactobacillus inhibited PEDV shedding through the feces of piglets and reduced the infection risk of the cohabiting piglets. The inhibitory effect on fecal PEDV shedding in the mixed group was slightly stronger than that in the fused group.
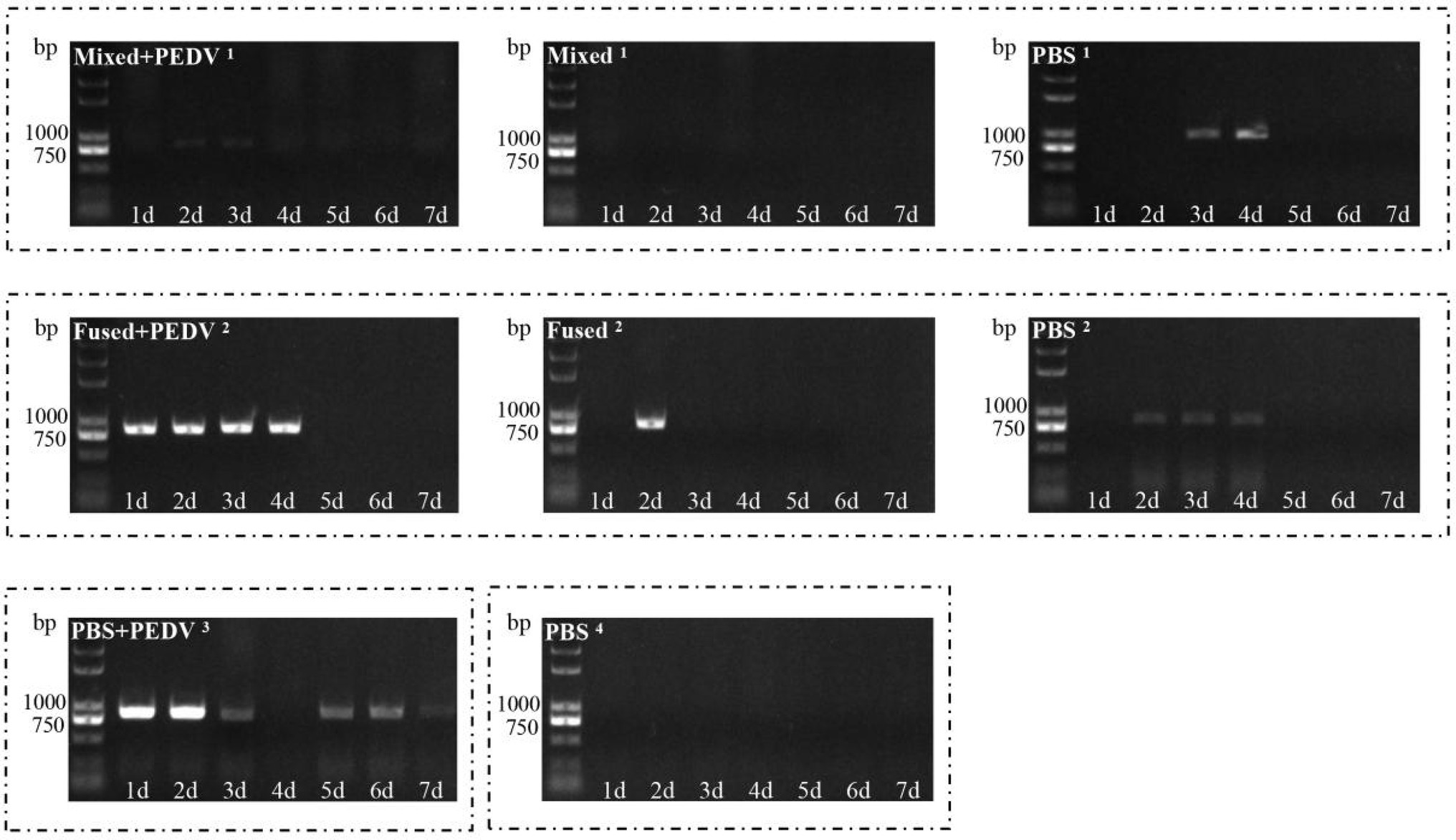
Figure 7. Detection of virus shedding through feces of piglets. PEDV RNA in anal swabs collected daily after grouping was detected using RT-PCR.
Macroscopic Examination and Histopathological Detection of Small Intestine in Piglets
All piglets were euthanized for macroscopic examination at 7 days after the challenge. Piglets in the PBS + PEDV3 group exhibited moderately thin and transparent intestinal walls in the small intestine, and large amounts of fluid accumulated in the intestinal lumen (Figure 8A). Cohabiting piglets (PBS1 and PBS2) exhibited similar or even more serious symptoms. In contrast, there were no observable changes in the small intestines of immunized piglets (mixed+PEDV1, fused+PEDV2, mixed1, and fused2) compared with those of the piglets in the PBS4 group (Figure 8A). Histopathological observations indicated that infection by PEDV caused extensive damage to the jejunum of piglets in the PBS + PEDV3 group, characterized by large amounts of fragmentation and shedding of intestinal villi (Figure 8B). Interestingly, the PBS1 and PBS2 groups exhibited more severe pathological damage to the jejunum. In contrast, damage to the jejunum of immunized piglets (mixed+PEDV1 and fused+PEDV2) was minor compared to that in the PBS + PEDV3 group. No pathological damage was observed in the piglets of the mixed1 and fused2 groups (Figure 8B).
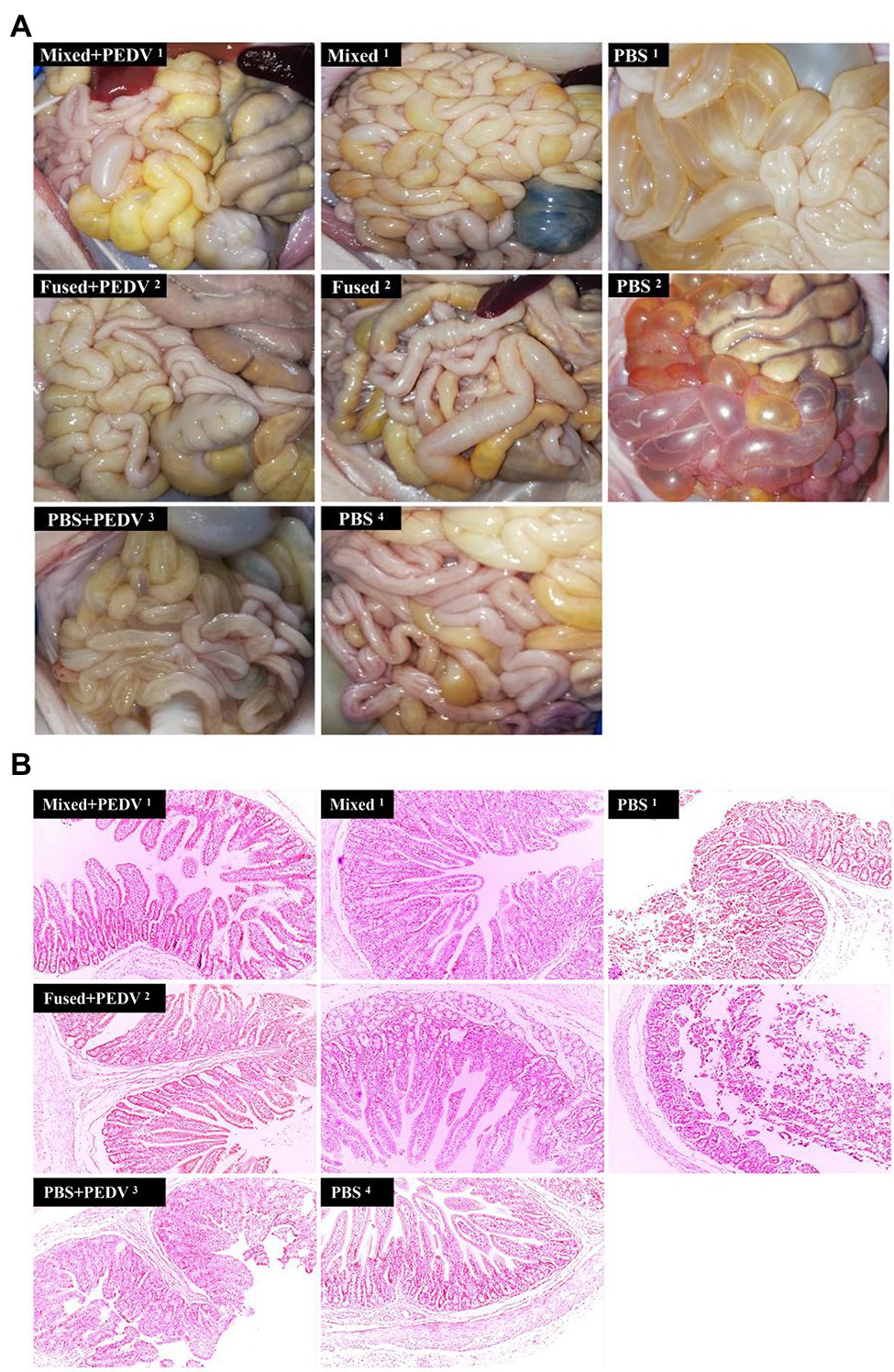
Figure 8. Intestinal macroscopic examination and histopathological observation of piglets. (A) Macroscopic examination of piglets in different groups. (B) Histopathological observation of jejunum of piglets in different groups. Original magnification: × 100.
Discussion
By harnessing the mucosal immune system for vaccine development, sIgA and serum IgG can be induced to provide two layers of defense against mucosal pathogens (Thomas et al., 2015). Mucosal immunization can be achieved through oral administration, which is comparatively convenient to administer and is a far more effective route for directly stimulating intestinal sIgA responses (Neutra and Kozlowski, 2006; Sung et al., 2018). Lactobacillus is an ideal bacterial delivery system for oral vaccines (Rajoka et al., 2017; Chiabai et al., 2019; Liu et al., 2019; Natalia et al., 2019). Thus, we investigated the feasibility of using a multi-pathogen vaccine based on Lactobacillus spp. against porcine viral diarrhea.
We chose TGEV, PEDV, and PoRV, which are common pathogens of porcine viral diarrhea and often occur as mixed infections, to explore the feasibility of multi-pathogen vaccines. Antigens are key factors for the development of bacterial vector vaccines. The D site (378–395 amino acids) of the TGEV S protein has been identified as an important immune target for the host to neutralize the virus (Posthumus et al., 1990; Uer et al., 1991). To enhance immunogenicity, 6D fragments of the TGEV S protein were linked to each other to form a tandem gene, which was named as 6Ds. We previously showed that recombinant Lactobacillus expressing 6Ds induced mucosal and systemic immune responses in mice (Liu et al., 2011). Similarly, the COE and VP4 genes have been identified as the antigenic neutralizing epitopes of PEDV and PoRV (Duarte and Laude, 1994; Gilbert and Greenberg, 1998), respectively, and their effectiveness has been confirmed in the Lactobacillus expression system (Li et al., 2010; Hou et al., 2018). Therefore, we used the proteins 6Ds, COE, and VP4 as antigens.
To improve the expression of antigens and ensure their localization on the surface of Lactobacillus, the previously constructed expression vector pPG-T7g10-PPT (Song et al., 2014; containing the enhancer T7g10 fragment, HCE constitutive promoter, and PgsA anchor sequence), which expresses foreign antigens on the surface (Xu and Li, 2007; Liu et al., 2011; Yu et al., 2017; Zhao et al., 2017; Hou et al., 2018), was used in this study. We previously confirmed that recombinant Lactobacillus expressing a single antigen had a strong immune effect (Li et al., 2010; Liu et al., 2011; Hou et al., 2018). Research has suggested that combining multiple vaccines can have numerous positive effects against mixed multi-virus infections (Sánchez-Matamoros et al., 2019). Data on the immunity and safety afforded by such combined administration have been published for other vaccines in piglets (Jeong et al., 2016; Garcia-Morante et al., 2019; Sánchez-Matamoros et al., 2019). In this context, we constructed a recombinant Lactobacillus expressing antigen 6Ds, COE, and VP4, and immunity was evaluated following combined administration. To simplify the vaccine production schedules and reduce the cost of bacterial culture, another strategy involves the construction of a recombinant Lactobacillus expressing these three antigens in a fused manner. A previous study confirmed that direct fusion of antigens may lead to many undesirable outcomes, including misfolding of the fusion proteins, low yield in protein production, or impaired bioactivity (Amet et al., 2009). Therefore, the selection or rational design of linkers to join fusion proteins is important in recombinant fusion protein technology (Chen et al., 2012). Here, we chose a rigid linker to preserve the stability and bioactivity of the fusion proteins, and recombinant Lactobacillus pPG-T7g10-6Ds-COE-VP4/LC393 fused expressing the three antigens was constructed. Our results confirmed that the antigens 6Ds, COE, and VP4 were successfully expressed in recombinant Lactobacillus using pPG-T7g10-PPT as a vector. These antigens were expressed on the surface of the recombinant Lactobacillus.
Immune responses of newborn piglets induced by recombinant Lactobacillus were evaluated via oral immunization. Specific sIgA and IgG levels symbolize mucosal and humoral immune responses (Holmgren and Czerkinsky, 2005; Suzuki et al., 2017). In this study, anti-TGEV/PEDV/PoRV-specific sIgA and IgG were significantly increased in both the mixed and fused groups after immunization compared to those in the PBS group. The results showed that recombinant L. casei induced not only a specific mucosal immune response but also a specific humoral immune response against all three viruses in piglets, which is consistent with our previous results (Li et al., 2010; Liu et al., 2011; Hou et al., 2018). In addition, the anti-TGEV/PEDV/PoRV-specific sIgA and serum IgG in partial samples of the mixed group were slightly higher than those of the fused group, but the difference was not significant.
To determine whether the immune response of piglets induced by recombinant Lactobacillus could protect against viral infection, a challenge test was performed using PEDV as an example. Fecal-oral transmission is thought to be the main mode of PEDV transmission, and airborne transmission is considered as a potential route for dissemination (Alonso et al., 2014; Lin et al., 2016). The objectives of this study were to determine the efficacy of protecting piglets orally challenged with PEDV and to inhibit the horizontal spread of PEDV among piglets. For cohabiting piglets, the route of exposure to the virus is similar to that of natural infection rather than that from a high-dose oral challenge. Therefore, both oral challenge and cohabitation groups were examined in this study. Our results showed that recombinant Lactobacillus alleviated clinical symptoms, inhibited virus shedding, and reduced intestinal pathological damage in challenged piglets but could not prevent fecal virus shedding. The cohabiting piglets had no obvious symptoms, almost no fecal virus shedding, and no intestinal pathological damage, showing that recombinant Lactobacillus effectively protected cohabiting piglets. Thus, application of oral multi-pathogen recombinant Lactobacillus under field conditions may inhibit the horizontal transmission of PEDV and protect piglets from infection. Notably, protection of the mixed group was better than that of the fused group in terms of clinical symptoms and fecal virus shedding. The combination of multiple vaccines may have allowed the three recombinant Lactobacillus strains to play independent roles. In addition, because the specific sIgA and IgG levels induced by recombinant Lactobacillus against the three viruses were similar, the protective effect of these recombinant Lactobacillus against TGEV and PoRV may be similar to that of PEDV. To confirm this prediction, TGEV and PoRV challenge will be evaluated in further studies.
Overall, our results demonstrate that an oral multi-vaccine based on Lactobacillus can induce specific mucosal and humoral immune responses against multiple piglet diarrhea viruses and protect piglets from viral infection. Lactobacillus-based oral multi-vaccines show excellent potential as vaccines and should be further evaluated, which will have important implications for porcine management in highly convenient vaccination programs.
Data Availability Statement
The original contributions presented in the study are included in the article/supplementary material, and further inquiries can be directed to the corresponding authors.
Ethics Statement
The animal study was reviewed and approved by the Animal Experiment Ethics Committee of Northeast Agricultural University, China.
Author Contributions
YL and LW conceived and designed the study. TG, CG, JH, XL, KX, XW, JL, HZ, and WC performed the experiments. ZS, YJ, XQ, and LT interpreted and analyzed the data. TG wrote the manuscript. All authors contributed to the article and approved the submitted version.
Funding
This work was supported by the National Natural Science Foundation of China (31772779 and 31972718).
Conflict of Interest
The authors declare that the research was conducted in the absence of any commercial or financial relationships that could be construed as a potential conflict of interest.
Publisher’s Note
All claims expressed in this article are solely those of the authors and do not necessarily represent those of their affiliated organizations, or those of the publisher, the editors and the reviewers. Any product that may be evaluated in this article, or claim that may be made by its manufacturer, is not guaranteed or endorsed by the publisher.
References
Alonso, C., Goede, D. P., Morrison, R. B., and Davies, P. R. (2014). Evidence of infectivity of airborne porcine epidemic diarrhea virus and detection of airborne viral rna at long distances from infected herds. Vet. Res. 45:73. doi: 10.1186/s13567-014-0073-z
Amet, N., Lee, H. F., and Shen, W. C. (2009). Insertion of the designed helical linker led to increased expression of tf-based fusion proteins. Pharm. Res. 26, 523–528. doi: 10.1007/s11095-008-9767-0
Austin, A. S., Haas, K. M., Naugler, S. M., Bajer, A. A., Garcia-Tapia, D., and Estes, D. M. (2003). Identification and characterization of a novel regulatory factor: iga-inducing protein. J. Immunol. 171, 1336–1342. doi: 10.4049/jimmunol.171.3.1336
Bhuyan, A. A., Memon, A. M., Bhuiyan, A. A., Zhonghua, L., Zhang, B., Ye, S., et al. (2018). The construction of recombinant lactobacillus casei expressing bvdv e2 protein and its immune response in mice. J. Biotechnol. 270, 51–60. doi: 10.1016/j.jbiotec.2018.01.016
Chang, T. C., Chen, J. F., Feng, L., and Hong-Bo, N. I. (2016). Epidemiological survey of porcine epidemic diarrhea virus in 2014. Chin. J. Prev. Vet. Med. 38, 335–338.
Chen, X., Zaro, J. L., and Shen, W. C. (2012). Fusion protein linkers: property, design and functionality. Adv. Drug Deliv. Rev. 65, 1357–1369. doi: 10.1016/j.addr.2012.09.039
Chiabai, M. J., Almeida, J. F., Azevedo, M., Fernandes, S. S., and Brigido, M. M. (2019). Mucosal delivery of lactococcus lactis carrying an anti-tnf scfv expression vector ameliorates experimental colitis in mice. BMC Biotechnol. 19:38. doi: 10.1186/s12896-019-0518-6
Craig, K., Dai, X., Li, A., Lu, M., Xue, M., Rosas, L., et al. (2019). A lactic acid bacteria (lab)-based vaccine candidate for human norovirus. Viruses 11:213. doi: 10.3390/v11030213
Daniel, C., Roussel, Y., Kleerebezem, M., and Pot, B. (2011). Recombinant lactic acid bacteria as mucosal biotherapeutic agents. Trends Biotechnol. 29, 499–508. doi: 10.1016/j.tibtech.2011.05.002
Duarte, M., and Laude, H. (1994). Sequence of the spike protein of the porcine epidemic diarrhoea virus. J. Gen. Virol. 75, 1195–1200. doi: 10.1099/0022-1317-75-5-1195
Garcia-Morante, B., Noguera, M., Kraft, C., and Bridger, P. (2019). Field evaluation of the safety and compatibility of a combined vaccine against porcine parvovirus 1 and porcine reproductive and respiratory syndrome virus in breeding animals. Porcine Health Manag. 5:28. doi: 10.1186/s40813-019-0138-5
Gilbert, J. M., and Greenberg, H. B. (1998). Cleavage of rhesus rotavirus vp4 after arginine 247 is essential for rotavirus-like particle-induced fusion from without. J. Virol. 72, 5323–5327. doi: 10.1128/JVI.72.6.5323-5327.1998
Holmgren, J., and Czerkinsky, C. (2005). Mucosal immunity and vaccines. Nat. Med. 11, S45–S53. doi: 10.1038/nm1213
Hou, X., Jiang, X., Jiang, Y., Tang, L., Xu, Y., Qiao, X., et al. (2018). Oral immunization against pedv with recombinant lactobacillus casei expressing dendritic cell-targeting peptide fusing coe protein of pedv in piglets. Viruses 10:106. doi: 10.3390/v10030106
Jeong, J., Park, C., Choi, K., and Chae, C. (2016). A new single-dose bivalent vaccine of porcine circovirus type 2 and mycoplasma hyopneumoniae elicits protective immunity and improves growth performance under field conditions. Vet. Microbiol. 182, 178–186. doi: 10.1016/j.vetmic.2015.11.023
Jiang, X., Hou, X., Tang, L., Jiang, Y., Ma, G., and Li, Y. (2016). A phase trial of the oral lactobacillus casei vaccine polarizes th2 cell immunity against transmissible gastroenteritis coronavirus infection. Appl. Microbiol. Biotechnol. 100, 7457–7469. doi: 10.1007/s00253-016-7424-9
Kim, H. J., Park, J. G., Matthijnssens, J., Lee, J. H., Bae, Y. C., Alfajaro, M. M., et al. (2011). Intestinal and extra-intestinal pathogenicity of a bovine reassortant rotavirus in calves and piglets. Vet. Microbiol. 152, 291–303. doi: 10.1016/j.vetmic.2011.05.017
Li, Y. J., Ma, G. P., Li, G. W., Qiao, X. Y., Ge, J. W., Tang, L. J., et al. (2010). Oral vaccination with the porcine rotavirus vp4 outer capsid protein expressed by lactococcus lactis induces specific antibody production. J. Biomed. Biotechnol. 2010:708460. doi: 10.1155/2010/708460
Lin, C. M., Saif, L. J., Marthaler, D., and Wang, Q. (2016). Evolution, antigenicity and pathogenicity of global porcine epidemic diarrhea virus strains. Virus Res. 226, 20–39. doi: 10.1016/j.virusres.2016.05.023
Liu, D., Qiao, X., Ge, J., Tang, L., Jiang, Y., and Li, Y. (2011). Construction and characterization of lactobacillus pentosus expressing the d antigenic site of the spike protein of transmissible gastroenteritis virus. Can. J. Microbiol. 5, 392–397. doi: 10.1139/w11-027
Liu, M. L., Zhang, X., Hao, Y., Ding, J., Shen, J., Xue, Z., et al. (2019). Protective effects of a novel probiotic strain, lactococcus lactis ml2018, in colitis: in vivo and in vitro evidence. Food Funct. 10, 1132–1145. doi: 10.1039/C8FO02301H
Liu, Y. B., Zhao, H. C., Wang, Z. C., Gang, W. U., and Qi-Gai, H. E. (2013). Molecular epidemiological investigation of swine viral diarrhea. China Animal Husbandry and Veterinary Medicine 40, 204–207.
Mantis, N. J., Rol, N., and Corthésy, B. (2011). Secretory iga's complex roles in immunity and mucosal homeostasis in the gut. Mucosal Immunol. 4, 603–611. doi: 10.1038/mi.2011.41
Martín, V., Maldonado, A., Fernández, L., Rodríguez, J. M., and Connor, R. I. (2010). Inhibition of human immunodeficiency virus type 1 by lactic acid bacteria from human breastmilk. Breastfeed. Med. 5, 153–158. doi: 10.1089/bfm.2010.0001
Mcghee, J. R., Mestecky, J., Dertzbaugh, M. T., Eldridge, J. H., and Kiyono, H. (1992). The mucosal immune system: from fundamental concepts to vaccine development. Vaccine 10, 75–88. doi: 10.1016/0264-410X(92)90021-B
Mestecky, J. (1987). The common mucosal immune system and current strategies for induction of immune responses in external secretions. J. Clin. Immunol. 7, 265–276. doi: 10.1007/BF00915547
Natalia, M. B., Priscilla, B., Gabriel, F., and Rodrigo, D. (2019). Oral delivery of pancreatitis-associated protein by lactococcus lactis displays protective effects in dinitro-benzenesulfonic-acid-induced colitis model and is able to modulate the composition of the microbiota. Environ. Microbiol. 21, 4020–4031. doi: 10.1111/1462-2920.14748
Neutra, M. R., and Kozlowski, P. A. (2006). Mucosal vaccines: the promise and the challenge. Nat. Rev. Immunol. 6, 148–158. doi: 10.1038/nri1777
Nirmal, M., Mariusz, S., and Istvan, T. (2014). Oral delivery of nanoparticle-based vaccines. Expert Rev. Vaccines 13, 1361–1376. doi: 10.1586/14760584.2014.936852
Pfeiler, E. A., Azcarate-Peril, M. A., and Klaenhammer, T. R. (2007). Characterization of a novel bile-inducible operon encoding a two-component regulatory system in lactobacillus acidophilus. J. Bacteriol. 189, 4624–4634. doi: 10.1128/JB.00337-07
Posthumus, W., Lenstra, J. A., Schaaper, W., Nieuwstadt, A., and Meloen, R. H. (1990). Analysis and simulation of a neutralizing epitope of transmissible gastroenteritis virus. J. Virol. 64, 3304–3309. doi: 10.1128/jvi.64.7.3304-3309.1990
Rajoka, M. R., Shi, J., Zhu, J., Shao, D., Huang, Q., Yang, H., et al. (2017). Capacity of lactic acid bacteria in immunity enhancement and cancer prevention. Appl. Microbiol. Biotechnol. 101, 35–45. doi: 10.1007/s00253-016-8005-7
Renegar, K. B., Small, P. A., Boykins, L. G., and Wright, P. F. (2004). Role of iga versus igg in the control of influenza viral infection in the murine respiratory tract. J. Immunol. 173, 1978–1986. doi: 10.4049/jimmunol.173.3.1978
Renukuntla, J., Vadlapudi, A. D., Patel, A., Boddu, S., and Mitra, A. K. (2013). Approaches for enhancing oral bioavailability of peptides and proteins. Int. J. Pharm. 447, 75–93. doi: 10.1016/j.ijpharm.2013.02.030
Sánchez-Matamoros, A., Camprodon, A., Maldonado, J., Pedrazuela, R., and Miranda, J. (2019). Safety and long-lasting immunity of the combined administration of a modified-live virus vaccine against porcine reproductive and respiratory syndrome virus 1 and an inactivated vaccine against porcine parvovirus and Erysipelothrix rhusiopathiae in breeding pigs. Porc. Heal. Manag. 2019, 1–10. doi: 10.1186/s40813-019-0118-9
Song, B. F., Ju, L. Z., Li, Y. J., and Tang, L. J. (2014). Chromosomal insertions in the lactobacillus casei upp gene that are useful for vaccine expression. Appl. Environ. Microbiol. 80, 3321–3326. doi: 10.1128/AEM.00175-14
Song, D., Zhou, X., Peng, Q., Chen, Y., Zhang, F., Huang, T., et al. (2015). Newly emerged porcine deltacoronavirus associated with diarrhoea in swine in China: identification, prevalence and full-length genome sequence analysis. Transboundary and Emerging Diseases 62, 575–580. doi: 10.1111/tbed.12399
Staats, H. F., Jackson, R. J., Marinaro, M., Takahashi, I., Kiyono, H., and Mcghee, J. R. (1994). Mucosal immunity to infection with implications for vaccine development. Curr. Opin. Immunol. 6, 572–583. doi: 10.1016/0952-7915(94)90144-9
Sun, D., Wang, X., Wei, S., Chen, J., and Feng, L. (2016). Epidemiology and vaccine of porcine epidemic diarrhea virus in China: a mini-review. J. Vet. Med. Sci. 78, 355–363. doi: 10.1292/jvms.15-0446
Sung, K., Seok, H., Yong-Kyu, L., and Sungpil, C. (2018). Oral vaccine delivery for intestinal immunity—biological basis, barriers, delivery system, and m cell targeting. Polymers 10:948. doi: 10.3390/polym10090948
Suzuki, T., Ainai, A., and Hasegawa, H. (2017). Functional and structural characteristics of secretory Iga antibodies elicited by mucosal vaccines against influenza virus. Vaccine 35, 5297–5302. doi: 10.1016/j.vaccine.2017.07.093
Thomas, J. T., Chen, Q., Gauger, P. C., Giménez-Lirola, L. G., Sinha, A., Harmon, K. M., et al. (2015). Effect of porcine epidemic diarrhea virus infectious doses on infection outcomes in nave conventional neonatal and weaned pigs. PLoS One 10: e0139266. doi: 10.1371/journal.pone.0139266
Uer, G. B., Posthumus, F., Correa, W., Sué, I. C., and Enjuanes, L. (1991). Residues involved in the antigenic sites of transmissible gastroenteritis coronavirus s glycoprotein. Virology 183, 225–238. doi: 10.1016/0042-6822(91)90135-X
Wall, T., Båth, K., Britton, R. A., Jonsson, H., Versalovic, J., and Roos, S. (2007). The early response to acid shock in lactobacillus reuteri involves the clpl chaperone and a putative cell wall-altering esterase. Appl. Environ.Microbiol. 73, 3924–3935. doi: 10.1128/AEM.01502-06
Wang, L., Beverly, B., and Yan, Z. (2014). New variant of porcine epidemic diarrhea virus, United States, 2014. Emerg. Infect. Dis. 20, 917–919. doi: 10.3201/eid2005.140195
Wen, F., Yang, J., Li, A., Gong, Z., and Huang, S. (2021). Genetic characterization and phylogenetic analysis of porcine epidemic diarrhea virus in Guangdong, China, between 2018 and 2019. PLoS One 16:e0253622. doi: 10.1371/journal.pone.0253622
Xu, Y., and Li, Y. (2007). Induction of immune responses in mice after intragastric administration of lactobacillus casei producing porcine parvovirus vp2 protein. Appl. Environ. Microbiol. 73, 7041–7047. doi: 10.1128/AEM.00436-07
Yu, M., Wang, L., Ma, S., Wang, X., Wang, Y., Xiao, Y., et al. (2017). Immunogenicity of egfp-marked recombinant lactobacillus casei against transmissible gastroenteritis virus and porcine epidemic diarrhea virus. Viruses 9:274. doi: 10.3390/v9100274
Yza, B., Cong, C. A., Yc, A., Zl, A., Jz, A., Tw, A., et al. (2019). Effect of route of inoculation on innate and adaptive immune responses to porcine epidemic diarrhea virus infection in suckling pigs. Vet. Microbiol. 228, 83–92. doi: 10.1016/j.vetmic.2018.11.019
Zhang, F., Luo, S., Gu, J., Li, Z., and Tang, Y. (2019). Prevalence and Phylogenetic Analysis of Porcine Diarrhea Associated Viruses in Southern China from 2012 to 2018. BMC Vet. Res. 15: 470. doi: 10.1186/s12917-019-2212-2
Keywords: porcine viral diarrhea, mucosal immunity, Lactobacillus casei, oral vaccine, multi-vaccine
Citation: Guo T, Gao C, Hao J, Lu X, Xie K, Wang X, Li J, Zhou H, Cui W, Shan Z, Jiang Y, Qiao X, Tang L, Wang L and Li Y (2022) Strategy of Developing Oral Vaccine Candidates Against Co-infection of Porcine Diarrhea Viruses Based on a Lactobacillus Delivery System. Front. Microbiol. 13:872550. doi: 10.3389/fmicb.2022.872550
Edited by:
Maryam Dadar, Razi Vaccine and Serum Research Institute, IranReviewed by:
Fanfan Zhang, Jiangxi Academy of Agricultural Sciences (CAAS), ChinaGui-Lian Yang, Jilin Agricultural University, China
Copyright © 2022 Guo, Gao, Hao, Lu, Xie, Wang, Li, Zhou, Cui, Shan, Jiang, Qiao, Tang, Wang and Li. This is an open-access article distributed under the terms of the Creative Commons Attribution License (CC BY). The use, distribution or reproduction in other forums is permitted, provided the original author(s) and the copyright owner(s) are credited and that the original publication in this journal is cited, in accordance with accepted academic practice. No use, distribution or reproduction is permitted which does not comply with these terms.
*Correspondence: Li Wang, d2FuZ2xpY2F1QDE2My5jb20=; Yijing Li, eWlqaW5nbGlAMTYzLmNvbQ==