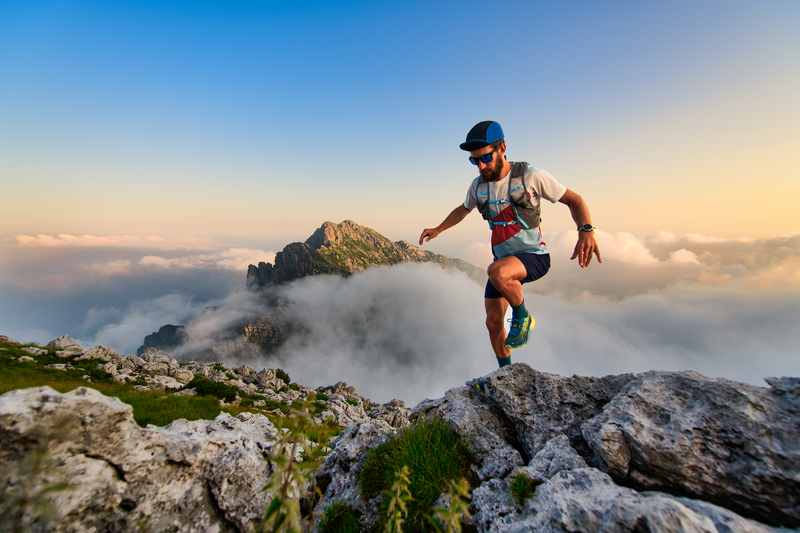
94% of researchers rate our articles as excellent or good
Learn more about the work of our research integrity team to safeguard the quality of each article we publish.
Find out more
ORIGINAL RESEARCH article
Front. Microbiol. , 17 May 2022
Sec. Extreme Microbiology
Volume 13 - 2022 | https://doi.org/10.3389/fmicb.2022.867340
This article is part of the Research Topic Insights in Extreme Microbiology: 2021 View all 16 articles
The extent to which the full diversity of the subsurface microbiome can be captured via cultivation is likely hindered by the inevitable loss of cellular viability from decompression during sampling, enrichment, and isolation. Furthermore, the pressure tolerance of previously isolated strains that span surface and subsurface ecosystems can shed light into microbial activity and pressure adaptation in these transition zones. However, assessments of the effects of elevated pressure on the physiology of piezotolerant and piezosensitive species may be biased by high-pressure enrichment techniques. Here, we compared two high-pressure cultivation techniques—one that requires decompression of the whole cultures during sampling and one that employs the previously described isobaric PUSH devices—to explore the effects of repeated decompression during incubations performed to characterize isolates from deep environments. Two model sulfate-reducing prokaryotes were used to test the effects of decompression/repressurization cycles on growth rates, cell yields, and pressure tolerance. The mesophilic bacterium Desulfovibrio salexigens was cultivated from 0.1 to 50 MPa, and the hyperthermophilic archaeon Archaeoglobus fulgidus was tested from 0.1 to 98 MPa. For both cultivation methods, D. salexigens showed exponential growth up to 20 MPa, but faster growth rates were observed for isobaric cultivation. Furthermore, at 30 MPa minor growth was observed in D. salexigens cultures only for isobaric conditions. Isobaric conditions also extended exponential growth of A. fulgidus to 60 MPa, compared to 50 MPa when cultures were decompressed during subsampling. For both strains, growth rates and cell yields decreased with increasing pressures, and the most pronounced effects of decompression were observed at the higher end of the pressure ranges. These results highlight that repeated decompression can have a significant negative impact on cell viability, suggesting that decompression tolerance may depend on habitat depth. Furthermore, sampling, enrichment, and cultivation in isobaric devices is critical not only to explore the portion of the deep biosphere that is sensitive to decompression, but also to better characterize the pressure limits and growth characteristics of piezotolerant and piezosensitive species that span surface and subsurface ecosystems.
Most of the bacterial and archaeal biomass on Earth is in deep-sea and subsurface environments at elevated pressures (Whitman et al., 1998; Kallmeyer et al., 2012; Parkes et al., 2014; Bar-On et al., 2018). These microorganisms have been shown to be well-adapted to the elevated pressures of their natural habitats (e.g., Somero, 1992; Allen and Bartlett, 2002; Simonato et al., 2006; Jebbar et al., 2015; Peoples and Bartlett 2017). Despite this, pressure seems to be one of the least explored parameters for microbial growth, as relatively few microorganisms from these deep environments have been isolated and/or grown under in situ pressures. To date, fewer than 100 species have been reported to be piezotolerant or piezophilic (Picard and Daniel, 2013; Jebbar et al., 2015; Cario et al., 2019; Oliver et al., 2020; Alain et al., 2021; Courtine et al., 2021; Li et al., 2021; Yu et al., 2021; Zhang et al., 2022), and few obligate piezophiles have been identified (Bartlett, 2002; Zeng et al., 2009). Therefore, our knowledge of active microbial species, their physiological and metabolic potential, and community diversity in these deep environments is limited.
Exploration of high-pressure life is limited by the difficulty in both sampling from high-pressure environments and replicating those pressure conditions in the laboratory during cultivation, isolation, and characterization. For obligate piezophiles, high-pressure sampling and cultivation is the only route to isolate novel species (Yayanos and Dietz, 1983; Jannasch and Wirsen, 1984). However, facultative piezophiles and piezotolerant microorganisms can often withstand lower sampling and transfer pressures (Jannasch and Wirsen, 1984). In these cases, the total change in pressure, duration of decompression, and number of subsequent decompression/repressurization cycles can impact the successful isolation of piezophiles (Yayanos, 2001; Park and Clark, 2002; Peoples and Bartlett, 2017). The pressure condition of cultivation experiments may also impose a selection bias on enrichment and isolation experiments that favor species more tolerant to decompression or lower growth pressures (Jannasch et al., 1992; Yayanos, 1995; Grossart and Gust, 2009). Furthermore, Park and Clark (2002) showed that rates of decompression could also impact microbial survival during decompression. For example, accelerated rates of decompression (26 MPa/s) caused the piezophile, Methanococcus jannaschii cells to rupture while slower rates of decompression (5.2 MPa/min) over the same pressure range increased viable cell yields (Park and Clark, 2002). Additionally, many piezophiles recovered from the intestinal systems of deep-sea macro fauna (Yayanos et al., 1979; Deming et al., 1981; Jannasch and Wirsen, 1984), show a greater tolerance to sample decompression, but the loss of species from decompression has yet to be quantified and any correlation with habitat depth has yet to be explored (Yayanos, 1978).
Deep biosphere species are often exposed to decompression during sample recovery, as well as during transfer, enrichment, and isolation. Therefore, significant effort has gone into developing pressure-retaining vessels to sample from the deep ocean habitats and carry out enrichment and isolation experiments without decompression (Tabor and Colwell, 1976; Jannasch and Wirsen, 1977; Yayanos, 1977; Cahet et al., 1990; Bianchi et al., 1999; Tamburini et al., 2003; Kato et al., 2008; McNichol et al., 2016; Cario et al., 2019). Such variable volume, floating piston devices can maintain elevated pressure during subsampling, inoculation, and/or transfer (Bianchi et al., 1999; Tamburini et al., 2003; Garel et al., 2019). Recent application of these new technologies has confirmed that higher rates of microbial activity and cell growth are achieved in incubation studies of bathypelagic samples maintained at in situ pressures without decompression, compared to parallel, decompressed, ambient pressure experiments (Tamburini, 2006; Tamburini et al., 2013; Garel et al., 2019, 2021). These high-pressure incubation experiments emphasize the need to study deep-sea microbes under in situ pressure conditions in order to accurately quantify deep-sea prokaryotic activity.
For enrichments and isolation, common methods involve growth in syringes, plastic bulbs, or heat-sealed plastic bags, in static pressure vessels (reviewed in Yayanos, 2001). However, subsampling to monitor cell growth over time requires decompression of the entire system followed by repressurization for continued cultivation. Inoculating such devices for high-pressure enrichment experiments also usually requires ambient pressure conditions. Other alternatives to study microorganisms under in situ pressure conditions include (i) the implementation of optic windows in the high-pressure vessels to monitor growth and motility (Maldonado et al., 2016; Garel et al., 2019); (ii) using high-pressure capillaries or other high-pressure cells coupled with microscopy to study molecular behaviors under extreme conditions with specific high-pressure cell using fluorescence microscopy (Raber et al., 2006; Usui et al., 2012; Patra et al., 2017; Bourges et al., 2020); and (iii) application of spectroscopy techniques to characterize microbial metabolism under high-pressure conditions (Kato and Fujisawa, 1998; Picard et al., 2007, 2015; Peters et al., 2014; Martinez et al., 2016; Osman et al., 2021). These alternatives are promising but require both specific and costly equipment and expertise to perform high-pressure in situ monitoring, and are not-yet widespread.
Here, we compare microbial growth in traditional static pressure vessels with cultivation in variable volume, floating piston isobaric high-pressure vessels to explore how decompression affects microbial growth patterns and the range of growth pressures at HHP for two model subsurface sulfate-reducing prokaryotes. Building upon previous designs (Bianchi et al., 1999; Tamburini et al., 2003), we recently collaborated with TOP Industrie© (Vaux Le Peńil, France) to develop a high-pressure, high temperature (HT; 100 MPa, 121°C), floating piston device with a 50 ml PEEK-lined reservoir (Cario et al., 2019). These pressurized underwater sample handler (PUSH) vessels were designed to sample from deep-sea environments and enable subsequent high-pressure enrichment and isolation without decompression (Oliver et al., 2021). These PUSH vessels were used to grow two sulfate-reducing prokaryotes, in high hydrostatic pressure (HHP) batch cultivation experiments that compared growth rates between cultures that experienced several cycles of decompression/repressurization, and those that were maintained at constant pressures throughout the experiment. High-pressure growth of Desulfovibrio salexigens, a mesophilic bacterium previously reported to be piezosensitive (Bale et al., 1997) was tested up to 50 MPa at 30°C (optimum temperature at 0.1 MPa). We have previously reported piezotolerant growth of Archaeoglobus fulgidus (Oliver et al., 2020), a hyperthermophilic archaeon, under high-pressure conditions with cycles of decompression/repressurization, and here explore growth at constant incubation pressures from 0.1 to 98 MPa at 83°C (optimum temperature at 0.1 MPa).
The PUSH vessels, similar to those vessels described in Bianchi et al. (1999) and Garel et al. (2019), and purchased through TOP Industrie©, were used for HT and HHP microbial batch cultivation without whole sample decompression and repressurization cycles during subsampling periods (Oliver et al., 2020). The PUSH vessels have a maximum pressure and temperature range of 0.1–100 MPa and 25°C–160°C, respectively, (as detailed in Oliver et al., 2021). For HT conditions, the temperature was controlled by a heating jacket and thermocouple system constructed for each PUSH vessel. The heating jackets, thermocouples, and temperature controllers were purchased from OMEGA™. Each vessel had an insulation wrap over the heating jacket and thermocouple. This heating system can be moved into an anaerobic chamber and plugged into a DC to AC converter powered by a 12-volt battery to maintain HT conditions during anaerobic preparations so that stable pressures could be reached upon pressurization (Supplementary Figures S1B,C). A decompression line was constructed to mitigate rapid pressure changes during subsampling that might induce cell shearing or death (Park and Clark, 2002; Foustoukos and Pérez-Rodríguez, 2015). The line included a micrometering valve to slowly subsample while operating the HHP screw pump to maintain vessel pressures (Supplementary Figure S1E).
Similar to several previous high-pressure growth experiments (e.g., Marteinsson et al., 1997; Takai et al., 2008; Oliver et al., 2020) syringes were used as reaction vessels in heated static pressure vessels, which were decompressed and repressurized at each sampling point. Desulfovibrio salexigens was grown in plastic syringes, and A. fulgidus was grown in glass syringes (Hamilton© 10 ml, 1,000 series luer lock gastight glass syringes) to maintain anoxia in the A. fulgidus growth medium, as previously described (Oliver et al., 2020; glass syringes were required at the higher incubation temperature for this strain to limit oxygen diffusion into the anoxic growth medium during incubation). To prevent syringe leakage at HT-HHP, custom-made butyl rubber stoppers served as an extra seal between the growth medium and the syringe piston. For incubation in both plastic and glass syringes with Luer lock fittings, the needle hub was embedded in butyl rubber stoppers before being transferred into the pressure vessels. Four High Pressure Equipment Co.© (HiP©), OC-1 O-Ring static pressure vessels were equipped with individual pressure gauges, and heating systems were similar to those described above for the PUSH vessels. Each 125 ml vessel held one 10 ml glass syringe. The temperature range of this system is 25°C–121°C and accommodates pressures from 0.1 to 100 MPa (based on the maximum working pressure capacities of the HiP© vessels and the temperature range of the BUNA O-Rings).
The choice of D. salexigens and A. fulgidus allowed for a comparison between two strains with similar metabolic strategies (heterotrophic sulfate reduction) over a range of temperatures (D. salexigens is a mesophile, while A. fulgidus is a thermophile) for two genera that are ubiquitous in subsurface environments and also represent both prokaryote. Each of these strains have had previous indications of growth at elevated pressure, belong to genera with other piezotolerant/piezophilic species, and also have been identified in high-pressure ecosystems. For example, a previous study, based on sulfide production measurements, showed that D. salexigens was active up to 5 MPa (Bale et al., 1997), and three other Desulfovibrio species have been isolated from subsurface environments (D. profundus, D. hydrothermalis, and D. piezophiles; Bale et al., 1997; Alazard et al., 2003; Khelaifia et al., 2011). While A. fulgidus type strain VC-16 was isolated from a shallow marine vent (Stetter et al., 1987, 1988), this strain has been identified in a number of deep-sea and deep subsurface environments (1–4 km and ~10–40 MPa; Stetter et al., 1987; Beeder et al., 1994; L'Haridon et al., 1995; Nakagawa et al., 2005; Fardeau et al., 2009). Additional species within the genus Archaeoglobus have been isolated from deep-sea environments (e.g., Archaeoglobus veneficus was isolated from the Mid Atlantic Ridge at 3.5 km depth, Huber et al., 1997), and archaeal sequences belonging to Archaeoglobaceae have been identified at the Mid-Cayman Rise, the deepest known hydrothermal system that reaches 4.96 km depths (~50 MPa; Reveillaud et al., 2016). Further, we have previously reported high-pressure growth of A. fulgidus (Oliver et al., 2020), showing that this strain is tolerant up to ~30–40 MPa (with decompression), which is consistent with the depths and pressures of deep-sea and deep subsurface environments where it has been identified.
Desulfovibrio salexigens (DSM 2638), a marine sulfate-reducing bacterium (Postgate and Campbell, 1966) was obtained from the Deutsche Sammlung von Mikroorganismen und Zellkulturen (Germany) and grown anaerobically in DSMZ medium 163 (Solution A: NaCl 25 g L−1, K2HPO4 0.5 g L−1, NH4Cl 1.0 g L−1, Na2SO4 1.0 g L−1, CaCl2.2H2O 2.0 g L−1, MgSO4.7H2O 2.0 g L−1, Na-DL-lactate 2.0 g L−1, Yeast Extract 1.0 g L−1, resazurin solution (0.1% w/v) 0.5 ml L−1, distilled water 980 ml; Solution B: FeSO4.7H2O 0.5 g/10 ml and Solution C: Na-thioglycolate 0.1 g/10 ml, ascorbic acid 0.1 g/10 ml), similar to Postgate and Campbell (1966), with the exception that the media was enriched in calcium (1.5 times) and reduced in iron (1:100). Solutions B and C were added to solution A under N2 and the pH was adjusted to 7.8 with NaOH.
Archaeoglobus fulgidus strain VC-16 (DSM 4304) was obtained from the Deutsche Sammlung von Mikroorganismen und Zellkulturen GmbH (DSMZ, Braunschweig, Germany). Archaeoglobus fulgidus VC-16 is an anaerobic, hyperthermophilic sulfate-reducing archaeon. Here, A. fulgidus was grown chemolithoheterotrophically in a lactate–sulfate-rich medium. The composition of the culture medium followed Hartzell and Reed (2006) and sterile anaerobic conditions were maintained following Balch et al. (1979). The medium was reduced prior to inoculation by adding Na2S.9H2O to a final concentration of 0.1% prior to inoculation (Cario et al., 2016).
Desulfovibrio salexigens cultivations with or without decompression (static pressure vessels, and PUSH vessels, respectively) were tested at 0.1, 10, 20, 30, 40, and 50 MPa. Subsamples were taken at ~12 h intervals for 72 h to obtain a robust growth curve. For the higher pressures (30–50 MPa), a cell count after 150 h of growth was performed to enumerate cell density (death or survival) according to the pressure cultivation technique. Cell recovery experiments were performed for this strain in order to evaluate the impact of elevated pressure on cell growth recovery. The protocol and the obtained results are detailed in Table 1. Archaeoglobus fulgidus VC-16 was cultivated from 0.1 to 98 MPa in ~10 MPa increments at 83°C in PUSH vessels and in static pressure vessels. Six to eight subsamples were taken at regular time intervals for standard growth curves. Archaeoglobus fulgidus cells grown in the glass syringes were decompressed and repressurized a maximum of nine times throughout each batch culture experiment.
Table 1. The percentage of D. salexigens cell recovery after 36 h of growth at different elevated pressures, with or without decompression steps, and transfer at ambient pressure.
For triplicate HT-HHP culture experiments with and without sample decompression, the sterile media and pre-cultures were prepared for four PUSH vessels and four syringes incubated in static pressure vessels, corresponding to three inoculated culture replicates and one uninoculated experiment to serve as a negative control. Each strain was first grown from a frozen stock (−80°C) and transferred into sterile anaerobic growth medium. Exponential growth of this culture was used as inoculum for three independent pre-cultures. After reaching logarithmic-phase growth, each of these pre-cultures was used to inoculate fresh sterile growth medium in three serum bottles. Immediately following inoculation, 8–10 ml from each inoculated serum bottle were transferred into sterile syringes and the remaining 45–47 ml were transferred into each PUSH vessel. The D. salexigens culture started at 1.106 cells/ml and incubations were carried out in static pressure vessels and PUSH vessels at 30°C. Inoculation and transfer of A. fulgidus cultures were carried out in the anaerobic chamber in pre-heated PUSH vessels. Starting cell concentrations for A. fulgidus experiments were ~6.7 × 106 cells/ml, and incubations were carried out at 83°C.
For each batch culture HT-HHP experiment, four PUSH vessels were sterilized, assembled, and pre-heated before inoculation and transfer (Supplementary Information 1, Supplementary Figure S1). After pre-assembly, each PUSH was individually wrapped in an insulated temperature-controlled system with thermocouple and heating jacket to 30°C and 83°C, for D. salexigens and A. fulgidus, respectively (Supplementary Information 1, Supplementary Figure S1B). Once pre-heated, all four vessels were transferred with their respective temperature-control systems into an anaerobic chamber (Bactron Shellab), and each temperature-control system was plugged into the DC to AC power converter allowing for continual vessel heating throughout the anaerobic inoculation process. In the anaerobic chamber, the PEEK reservoirs of each of the four PUSH vessels were filled with ~45–47 ml of pre-inoculated growth medium (triplicate) or sterile growth medium (Supplementary Information 1, Supplementary Figure S1C). The PEEK reservoir screw cap and lid was then closed completely and the valves were closed while in the anaerobic chamber. The vessels were then transported out of the anaerobic chamber for pressurization.
Each PUSH vessel was individually pressurized to the target pressure with a hydraulic screw pump with an in-line pressure gauge connected to the PEEK piston valve (Supplementary Information 1, Supplementary Figure S1D). Once pressurized, an initial 0.5 ml subsample was taken and fixed in 2.5% glutaraldehyde from each PUSH vessel using the decompression line (see below). Initial sampling and pressurization of the all of the PUSH vessels and syringes were done within 1 h of inoculation in the anaerobic chamber. To assure pressure stability, potential pressure loss from vessel leakage was closely monitored for the first 3 h after inoculation. Up to nine subsamples were obtained from each PUSH vessel for every HT-HHP batch culture growth experiment. For subsampling, the average decompression rate was of 15–25 MPa/min (Supplementary Information 1, Supplementary Figure S1E). The first 3 ml of medium sampled were discarded as waste from flushing the decompression line before taking a 0.5 ml aliquot sample for enumeration. A maximum of 10% pressure loss occurred during subsampling, but in all cases the pressure was rapidly re-established. The decompression line was cleaned with 70% ethanol and ultrapure water (18.2 MΩ) before and after subsampling each PUSH vessel.
For both strains, D. salexigens and A. fulgidus, four 10 ml syringes were used for HT-HHP batch cultivation with sample decompression. Ten milliliter sterile plastic syringes were used for D. salexigens and 10 ml glass syringes were used for A. fulgidus. Both plastic and glass syringes were flushed with N2 and the syringe needles were then embedded into butyl stoppers (Supplementary Figure S2B). The assembled syringes were then inoculated in the anaerobic chamber by transferring 8–10 ml of inoculated medium into each of the three 10 ml glass/or plastic syringes. For a negative control, 8–10 ml of sterile medium was transferred in the fourth 10 ml glass/or plastic syringe (Supplementary Information 2 and Supplementary Figure S2C). The syringes were removed from the anaerobic chamber and an initial 0.5 ml subsample was fixed in 2.5% glutaraldehyde for enumeration. Finally, each syringe was placed in one of the four pre-heated HiP© vessels, filled with water, and pressurized by connecting each vessel to a HHP screw pump to obtain the target growth pressure (Supplementary Information 2, Supplementary Figure S2D). For syringe subsampling, each vessel was decompressed at an average rate of 19 MPa/min and a 0.5 ml aliquot sample was taken and fixed in 2.5% glutaraldehyde from each syringe. After subsampling, the syringes were returned to the vessels and again pressurized to the target growth pressure.
Cell enumeration was estimated by direct counts from fixed cells [2.5% (v/v) glutaraldehyde] in a Thoma-chamber (depth: 0.02 mm; Brand, Wertheim, Germany) using a light microscope (model XM: Olympus) under 80x magnification (e.g., Huber et al., 1989; Hei and Clark, 1994; Blöchl et al., 1997; Cario et al., 2016). Specific growth rates (μ-hr) were calculated from linear regressions of the exponential portion of the growth curves from triplicate experiments using the LINEST function in Excel. Error bars indicate the standard error from linear regressions of triplicate experiments. Cell densities quantified at ~36 h for A. fulgidus and 48 h for D. salexigens were used to evaluate the effects of different cultivation conditions on overall cell yields. As some pressure conditions led to cell death, maximum cell densities could not be used for comparison. Error bars indicate SD from triplicate experiments. Significant differences were determined by Student’s t-test (value of p < 0.01).
Elevated pressure experiments were performed from ambient (0.1 MPa) up to 50 MPa for D. salexigens using both static pressure vessels (i.e., with cyclic decompression) and variable volume PUSH vessels (i.e., isobaric) for experiments that lasted 76–150 h. Growth curves, growth rates, and cell density data are summarized in Figure 1, Supplementary Table S1. At some elevated pressures, cell numbers decreased with time (e.g., cell death). To account for both cell growth and cell death at different conditions, cell densities are compared at 48 h over the entire pressure range of the experiments (Figure 1D).
Figure 1. (A) Desulfovibrio salexigens growth curves in plastic syringes with multiple sample decompression and (B) D. salexigens growth curves in the pressurized underwater sample handler (PUSH) vessels without multiple sample decompression from 0.1 to 50 MPa. (C) Desulfovibrio salexigens specific growth rates in the PUSH vessels (orange squares) and in syringes (blue circles). (D) Desulfovibrio salexigens maximum log cell densities in the PUSH vessels (orange squares) and in syringes (blue circles), open orange squares and blue circle indicate maximum cell densities in samples with no observed growth or observed cell densities lower than the average initial cell densities (dashed lines in D). Error bars are the SDs from the average of triplicate experiments. Significant differences were determined by Student’s t-test (value of p < 0.01).
Exponential growth of D. salexigens was observed up to 20 MPa both in the static and PUSH vessels (Figures 1A,B). The highest growth rates were observed at ambient pressure (0.1 MPa) and 30°C, and were nearly identical for cultivation in serum bottles and the PUSH vessels (0.19 ± 0.005 h−1 and 0.19 ± 0.004 h−1, respectively). The same growth rate was confirmed with D. salexigens incubation, performed in syringes, at ambient pressure and 30°C (data not shown). Overall growth rates decreased with pressure, but declining growth rates were less pronounced for isobaric enrichments (Figure 1C; Supplementary Table S1). For example, at 10 MPa the growth rate for isobaric cultivation was 0.17 ± 0.2 h−1, ~10.5% lower than optimal conditions, while cultivation with decompression had a ~36.8% drop in growth rate to 0.12 ± 0.1 h−1. Furthermore, the upper pressure limit for growth was higher in isobaric experiments, with slow growth observed at 30 MPa in PUSH vessels (0.02 h−1), compared to distinct cell death in decompressed experiments (Figures 1A–C).
While differences in growth rates were notable between the two cultivation techniques at moderate pressures (0.1–20 MPa), there were negligible effects on cell yields in this pressure range (Figure 1D; Supplementary Table S1). However, at supra-optimal pressures (≥30 MPa for D. salexigens), differences in cell densities (quantified at 48 h) were more significant. For cultivation experiments that included cyclic decompression, cell numbers decreased markedly with time at 30, 40, and 50 MPa, indicating cell death. Conversely, isobaric cultivation at these pressures led to only minor changes in cell density with time. For example, at 40 MPa, cell density decreased from an initial value of 106.30–105.78 after 48 h, while the isobaric culture had a cell density of 106.34 after 48 h and no decompression (Figure 1D). When considering changes in cell yields, decompression had a more significant impact at supra-optimal pressures, inducing cell death, as opposed to isobaric conditions, which only limited growth.
To further explore the impact of decompression on D. salexigens cell viability, cells were incubated at various elevated pressures (10–50 MPa) for 36 h, with or without decompression. They were then transferred to ambient pressure, incubated in fresh growth medium at several dilutions (1:1, 1:10, and 1:100, v/v), and cell recovery was estimated after 24 and 48 h of growth. Because the syringe cultivation technique requires decompression of the high-pressure vessels for subsampling, each vessel was subjected to six decompression/repressurization cycles with a minimum of 4 h between cycles. For both static pressure vessels and PUSH vessels, cells from the 10 and 20 MPa growth conditions were able to recover the optimal pressure cell density (see Table 1). However, when the cells were incubated at higher pressure (≥30 MPa) with cyclic decompression the cells were not able to recover and did not grow after 24 or 48 h of transfer at ambient pressure (nor after 1 week of incubation, data not shown). In comparison, cells exposed to 30–50 MPa in the PUSH without decompression remained viable, though were slower to recover as pressures increased. After transfer to and growth at ambient pressure (0.1 MPa) for 48 h, cell densities were only slightly lower (90% for 30 MPa and 70% for 50 MPa) than those observed for cells never exposed to elevated pressure (Table 1).
Archaeoglobus fulgidus VC-16 (type strain) was grown in batch cultivation experiments at 83°C over a range of pressures (0.1–98 MPa, at ~10 MPa increments) in PUSH vessels (isobaric) and in static pressure vessels (cyclic decompression) and cell densities were monitored up to 115 h. Growth curves, growth rates, and cell density data are summarized in Figure 2; Supplementary Table S1. To assess the impact of decompression on cell yields even in the cases of cell death, cell densities at 36 h are reported over the entire pressure range (Figure 2D). This time aligns with the generally faster growth of this strain, captures the exponential phase at lower pressures, and accurately represents cell death at elevated pressures.
Figure 2. (A) Archaeoglobus fulgidus growth curves in glass syringes with multiple sample decompression and (B) A. fulgidus growth curves in the PUSH vessels without multiple sample decompression from 0.1 to 98 MPa. (C) Archaeoglobus fulgidus specific growth rates in the PUSH vessels (orange squares) and in syringes (blue circles). (D) Archaeoglobus fulgidus maximum log cell densities in the PUSH vessels (orange squares) and in syringes (blue circles), open orange squares and blue circle indicate maximum cell densities in samples with no observed growth or observed cell densities lower than the average initial cell densities (dashed lines in D). Error bars are the SDs from the average of triplicate experiments. Significant differences were determined by Student’s t-test (value of p < 0.01).
Based on the growth curves shown in Figures 2A,B, A. fulgidus growth rates were calculated for pressures between 0.1 and 60 MPa (Figure 2C). Overall, A. fulgidus growth characteristics showed trends with pressure similar to those observed for D. salexigens. Exponential growth was observed in the PUSH vessels at 60 MPa until ~29 h, after which cell numbers decreased. In static pressure vessels at this pressure, only minor increases in cell density (1.34 ± 0.11 × 107 cells/ml) relative to initial values (6.03 × 106 ± 1.02 cells/ml) were observed, but were not consistent with a true exponential growth phase (Figure 2A). Growth was generally faster with higher maximum cell densities (with the exception at 10 MPa) in PUSH vessels compared to static pressure vessels, especially at the higher end of the pressure range (Figures 2C,D). The fastest growth rate in the PUSH vessels was measured at 0.1 MPa (0.326 ± 0.017 μ-hr), though growth rates were only slightly slower from 10 to 30 MPa (0.258 ± 0.026–0.302 ± 0.011 μ-hr). The largest difference in growth rates between the two cultivation methods was observed at 30 and 40 MPa (15.3% and 44%, respectively). Overall, growth rates indicate that A. fulgidus VC-16 is piezosensitive with a maximum exponential growth pressure of 60 MPa in isobaric conditions and 50 MPa for cultivation that included cyclic decompression.
Similar to the mesophilic strain, D. salexigens, the two cultivation techniques had a more significant impact on A. fulgidus cell density in the supra-optimal pressure range (≥50 MPa), while density was largely unaffected at the lower pressures. For example, from 0.1 to 40 MPa the largest difference in density at ~36 h was at 30 MPa (108.66, isobaric vs. 108.56, decompressed). Comparing isobaric growth to cultivation with cyclic decompression shows that the largest disparities in cell density (measured at ~36 h) were observed at 60 MPa when growth was observed and at 90 MPa when only cell death was recorded. Again, these data suggest that cycles of decompressions can accelerate loss of cell viability. Loss of cell viability was also tested via high-pressure incubation experiments in which A. fulgidus cells were incubated at 80 MPa for 115 h and subsequently transferred (10% v/v) to fresh growth medium and incubated at 0.1 MPa and 83°C. Ambient pressure cultures were monitored visually and no growth was observed after a week of incubation.
We also observed significant effects of elevated pressure on the mobility and the morphology of D. salexigens cells using a light microscope (Supplementary Figure S3). Under optimal pressure conditions (i.e., ambient pressure, 0.1 MPa), the cells were highly motile and formed 3–4 μm vibrios and up to 20 μm cell long when reaching the late exponential and stationary phases (Supplementary Figure S3). Under high-pressure cultivation conditions (10 and 20 MPa) and subsequent sampling, the cells were barely motile and formed short vibrios (2–3 μm). After longer incubation times they also formed filamentous cells (Supplementary Figure S1). At higher cultivation pressures without decompression steps (30–50 MPa) and subsequent sampling, the cells were also immotile. When they were transferred for growth at ambient pressure, the cell division appeared altered with formation of cells arranged in chains (Supplementary Figure S4). Irregular and elongated cell morphologies were previously observed in A. fulgidus at elevated pressures (Oliver et al., 2020). This morphological feature has been reported in several studies, where elevated pressure inhibited the cell division protein FtsZ (e.g., Ishii et al., 2004; Abe et al., 2013). Morphological changes are a common stress response (e.g., Zobell and Oppenheimer, 1950; Donaldson et al., 1989) and might be a global stress response to high-pressure (e.g., Bidle and Bartlett, 1999; Aertsen et al., 2004).
Additionally, for elevated pressure conditions (10–50 MPa) we noticed the presence of cyst-like cells (Supplementary Figure S3), a constitutive dormancy behavior characteristic of some non-spore forming bacteria (Suzina et al., 2004). As pressure increased from 10 to 50 MPa, we observed an increasing abundance (approximately 2-fold) of such pleiomorphic cells (Supplementary Figure S5). However, after transferring these cultures to ambient pressure, we observed the release of cells resembling the development of vegetative cells, suggesting that these structures could be cysts (Supplementary Figure S4). The formation of various types of structures, such as endospores, exospores, or cysts, is previously recognized survival strategy of many bacteria under unfavorable conditions induce (Sudo and Dworkin, 1973). The pressures at which we observed changes morphologies and structures are consistent with the growth data that indicated the onset global stress, as indicated by slower growth rates and decreasing cell numbers. Interestingly, among the dissimilatory sulfate-reducing bacteria, the genus Desulfovibrio was characterized as non-sporulating compared to the closely related genus Desulfotomaculum (Postgate and Campbell, 1966). Such behavior might help this species to cope with extreme growth conditions, such as elevated pressure conditions, and might be an important contribution for microbial dissemination in various environments. These specific structures deserve more investigations to determine the developmental steps and the ultrastructural properties of this species when growing under unfavorable growth conditions.
A majority of the Earth’s biosphere thrives in high-pressure, subsurface environments (Fang et al., 2010; Oger and Jebbar, 2010; Picard and Daniel, 2013), and like many natural ecosystems, studies of subsurface diversity reveal a vast number of uncultured species, often only identified through the presence of their genetic material. The difference between the diversity observed in molecular studies and the strains cultivated from natural systems is likely due to the loss of cell viability during sampling, or the enrichment and isolation protocols. The importance of using growth media compositions to target unique metabolic strategies and thus increase the diversity of cultured species has been discussed elsewhere (e.g., Alain and Querellou, 2009; Overmann et al., 2017). Furthermore, the idea of reducing sample exposure to conditions that cause cell death is regularly used, as is the case for obligate anaerobes, specifically methanogens. More recently, similar reasoning has been applied to the high-pressure biosphere; with novel technologies being deployed to capture and characterize microbes at elevated pressure (e.g., Garel et al., 2019; Peoples et al., 2019). Nonetheless, widespread application of high-pressure techniques throughout sampling, transfer, enrichment, and isolation is limited by challenges associated with cost, technology development, and technical expertise that are amplified when considering high-pressure sampling and cultivation (Cario et al., 2019). Furthermore, even when enrichments or isolates are cultivated at elevated pressures in laboratory settings, traditional methods that use static pressure vessels require decompression during subsampling (e.g., Takai et al., 2008), and the effects of decompression on experimental results for laboratory cultivation experiments has not been thoroughly or systematically investigated.
To explore the potential effects of decompression on microbial growth dynamics, we used two model sulfate reducers, D. salexigens and A. fulgidus to compare growth rates, cell yields, and maximum growth pressures between cultivation experiments that included several cycles of decompression and repressurization during subsampling, and those that maintained cultures at constant pressure throughout. Both strains showed significant exponential growth at elevated pressure for both types of cultivation conditions, although both had maximum growth rates at ambient pressure, classifying them as piezosensitive strains. We note that in previous studies we observed piezophilic behavior with maximum growth rates at 20 MPa (Oliver et al., 2020), and found that the growth rate measured at 0.1 MPa was particularly sensitive to the presence of a gas phase. Additionally, the maximum pressure for exponential growth for both strains was extended under isobaric conditions, confirming that cultivation techniques can impact experimentally determined growth characteristics of isolated strains.
In general, the impacts of the two cultivation techniques (isobaric vs. cyclic decompression) followed similar patterns for both D. salexigens and A. fulgidus, even though the specific pressure ranges, growth rates, and cell yields were strain-specific. Comparing cultivation techniques across both species, growth dynamics can be delineated into three different pressure regimes. The low-pressure regime (LP) has robust exponential growth with only marginal decreases in growth rates as pressures increase and little variation in maximum cell density. The high-pressure regime (HP) includes the range that exceeds the maximum pressure for exponential growth and is often characterized by cell death. The transitional pressure regime (TP) lies in between, is characterized by markedly lower growth rates and cell yields than those observed in the LP, and sometimes exponential growth is hard to discern. Within the TP the impacts of several cycles of decompression and repressurization are most noticeable for both growth rates and maximum cell yields.
The LP regime of D. salexigens is 0.1–20 and 0.1–40 MPa for A. fulgidus. For both strains, exponential growth rates decreased with increasing pressure, but maximum cell densities were similar at the end of the exponential growth phase. In this low-pressure regime, the effects of cyclic decompression are pronounced for growth rates, which are lower for cyclic decompression cultivation compares to isobaric experiments. However, little to no discernible impact was observed for cell densities. Growth rates and cell densities are more variable in the TP and HP regimes. For D. salexigens the transition pressure range is 30–40 MPa. While exponential growth is not discernible at 30 MPa for either condition, there are measurable increases in cell density late in the isobaric cultivation, while only cell death is observed with cyclic decompression cultivation (Figure 1A). It is also in the TP range that the largest difference in cell density is observed (Figure 1D). A similar pattern is observed for A. fulgidus for which the transition pressure range is 40–70 MPa. Exponential growth is observed in isobaric cultures up to 60 MPa, while the limit for cell division in cyclic decompression cultivation is 50 MPa. Overall, significantly slower growth rates (Figure 2C) were observed in A. fulgidus subjected to decompression from 40 to 60 MPa. Furthermore, the impact of decompression on cell density was also most apparent at 50 and 60 MPa (Figure 2D). Finally, while the HP regime is generally characterized by cell death (decreasing cell density with time) there are still noticeable differences between the two cultivation techniques. For D. salexigens no growth was obvious at 40 or 50 MPa, however cells subjected to cyclic decompression exhibited clearly higher rates of cell death (Figure 1A). Additionally for A. fulgidus, decreases in cell density with time were slower for isobaric cultivation.
The processes that might cause the largest differences in both growth rate and cell yield in the TP and HP are not immediately apparent. However, the robust exponential growth in the LP indicates that cells in this lower pressure range are not significantly impacted by these conditions. As both D. salexigens and A. fulgidus are piezosensitive strains that were isolated and optimized at ambient pressure conditions (0.1 MPa), it follows that decompression from moderate pressures to ambient pressure (their optimum pressure for growth) has little impact on growth rates and cell yields. However, for piezotolerant and piezophilic organisms, decompression would repeatedly expose cells to sub-optimal pressure conditions. In these cases, we hypothesize that cyclic decompression would have a more significant negative impact on growth. Additionally, if extended exposure to elevated pressure were inducing adaptation, even in a subpopulation of the culture, these cells would be selected against during decompression. Further characterization of these and other piezotolerant and piezophilic strains are critical next steps to better understand the effects of cyclic decompression on laboratory characterization of isolated species. Overall, we expect that the difference between isobaric cultivation and cultivation with cyclic decompression will be more pronounced in piezotolerant and piezophilic strains.
For two deep biosphere species investigated here, A. fulgidus and D. salexigens, growth was negatively impacted by sample decompression, and these detriments to growth were more significant at pressures further from optimum pressures. These results highlight the need to maintain constant pressure without decompression during cultivation of high-pressure strains. Such an approach is likely to increase the pressure ranges of piezotolerant species, potentially reclassify other strains as piezophilic, and ultimately expand our knowledge of the diversity of known piezophiles, putting into perspective the habitat constraints for many strains whose pressure ranges have been underestimated. Even the piezophilic and piezotolerant organisms that are in culture have likely been isolated after decompression, inevitably selecting against strains more sensitive to decompression. For high-pressure samples that are decompressed and subsequently enriched at elevated in situ pressures, those strains that are somewhat tolerant to decompression, like A. fulgidus, would outcompete more sensitive strains, and results of any subsequent analyses would not necessarily be representative of the active in situ microbial communities.
Furthermore, maintaining in situ pressures becomes even more critical when retrieving samples from deep ecosystems, for example, 5–6 km—habitats more likely to host obligate piezophiles—for which decompression would subject native communities to pressure changes of over 50 MPa. Thus far, most of the obligate piezophiles identified have been psychrophilic bacteria sampled from depths greater that 6 km (i.e., ~60 MPa pressures; for example, Yayanos et al., 1981; Deming et al., 1988; Kato et al., 1998; Nogi et al., 2004) and only one obligate piezophilic hyperthermophilic archaeon, Pyrococcus yayanosii, has been identified from a hydrothermal vent at 4.1 km depth (Birrien et al., 2011). Given the extent of the subsurface biosphere, it is unlikely that these few obligate piezophiles represent the full diversity of Earth’s largest microbiome. Variable volume, high-pressure devices like the PUSH and others (reviewed in Cario et al., 2019), can be used not only to retrieve samples from up to ~9–10 km water depth, but all can be connected in series (as shown here and in Garel et al., 2019), so that sample transfer and enrichment can also be carried out without decompression. Such an approach will be more selective for piezophiles and obligate piezophiles, and greatly expand our understanding of the activity and physiology of the deep biosphere microbiome.
The original contributions presented in the study are included in the article/Supplementary Material; further inquiries can be directed to the corresponding authors.
GO, AC, and KR designed this research project and analyzed data. GO and AC performed the experiments and collected data and wrote the original draft. KR edited and wrote sections of the manuscript. All authors contributed to revisions of the manuscript, tables, and figures and approved the submitted version.
Funding for this work was provided by the NASA Exobiology and PSTAR Programs (NNX13AP2G9 and 80NSSC17K0252 to KR), the Deep Carbon Observatory (Subawards: 10371-07, 10561-01, and 10311-11 to KR), an NSF Graduate Fellowship (FAIN 1247271 and 1744655 to GO), and a GSA Research grant to GO. Additional support was provided by startup funds from Rensselaer Polytechnic Institute to KR.
The authors declare that the research was conducted in the absence of any commercial or financial relationships that could be construed as a potential conflict of interest.
All claims expressed in this article are solely those of the authors and do not necessarily represent those of their affiliated organizations, or those of the publisher, the editors and the reviewers. Any product that may be evaluated in this article, or claim that may be made by its manufacturer, is not guaranteed or endorsed by the publisher.
We thank Bruce Watson for generously gifting us four pressure vessels that allowed us to perform these growth experiments.
The Supplementary Material for this article can be found online at: https://www.frontiersin.org/articles/10.3389/fmicb.2022.867340/full#supplementary-material
Abe, A., Furukawa, S., Migita, Y., Tanaka, M., Ogihara, H., and Morinaga, Y. (2013). Sublethal high hydrostatic pressure treatment reveals the importance of genes coding cytoskeletal protein in Escherichia coli morphogenesis. Curr. Microbiol. 67, 515–521. doi: 10.1007/s00284-013-0392-8
Aertsen, A., Van Houdt, R., Vanoirbeek, K., and Michiels, C. W. (2004). An SOS response induced by high pressure in Escherichia coli. J. Bacteriol. 186, 6133–6141. doi: 10.1128/JB.186.18.6133-6141.2004
Alain, K., and Querellou, J. (2009). Cultivating the uncultured: limits, advances and future challenges. Extremophiles 13, 583–594. doi: 10.1007/s00792-009-0261-3
Alain, K., Vince, E., Courtine, D., Maignien, L., Zeng, X., Shao, Z., et al. (2021). Thermococcus henrietii sp. nov., a novel extreme thermophilic and piezophilic sulfur-reducing archaeon isolated from a deep-sea hydrothermal chimney. Int. J. Syst. Evol. Microbiol. 71:004895. doi: 10.1099/ijsem.0.004895
Alazard, D., Dukan, S., Urios, A., Verhé, F., Bouabida, N., Morel, F., et al. (2003). Desulfovibrio hydrothermalis sp. nov., a novel sulfate-reducing bacterium isolated from hydrothermal vents. Int. J. Syst. Evol. Microbiol. 53, 173–178. doi: 10.1099/ijs.0.02323-0
Allen, E. E., and Bartlett, D. H. (2002). Piezophiles: microbial adaptation to the deep-sea environment. Extremophiles III, 231–255.
Balch, W. E., Fox, G. E., Magrum, L. J., Woese, C. R., and Wolfe, R. S. (1979). Methanogens: reevaluation of a unique biological group. Microbiol. Rev. 43, 260–296. doi: 10.1016/j.watres.2010.10.010
Bale, S. J., Goodman, K., Rochelle, P. A., Marchesi, J. R., Fry, J. C., Weightman, A. J., et al. (1997). Desulfovibrio profundus sp. nov., a novel barophilic sulfate-reducing bacterium from deep sediment layers in the Japan Sea. Int. J. Syst. Evol. Microbiol. 47, 515–521.
Bar-On, Y. M., Phillips, R., and Milo, R. (2018). The biomass distribution on Earth. Proc. Natl. Acad. Sci. 115, 6506–6511. doi: 10.1073/pnas.1711842115
Bartlett, D. H. (2002). Pressure effects on in vivo microbial processes. Biochim. Biophys. Acta Protein Struct. Mol. Enzymol. 1595, 367–381. doi: 10.1016/S0167-4838(01)00357-0
Beeder, J., Nilsen, R. K., Rosnes, J. T., Torsvik, T., and Lien, T. (1994). Archaeoglobus fulgidus isolated from hot North Sea oil field waters. Appl. Environ. Microbiol. 60, 1227–1231. doi: 10.1128/aem.60.4.1227-1231.1994
Bianchi, A., Garcin, J., and Tholosan, O. (1999). A high-pressure serial sampler to measure microbial activity in the deep sea. Deep-Sea Res. I: Oceanogr. Res. Pap. 46, 2129–2142. doi: 10.1016/S0967-0637(99)00039-4
Bidle, K. A., and Bartlett, D. H. (1999). RecD function is required for high-pressure growth of a deep-sea bacterium. J. Bacteriol. 181, 2330–2337. doi: 10.1128/JB.181.8.2330-2337.1999
Birrien, J. L., Zeng, X., Jebbar, M., Cambon-Bonavita, M. A., Quérellou, J., Oger, P., et al. (2011). Pyrococcus yayanosii sp. nov., an obligate piezophilic hyperthermophilic archaeon isolated from a deep-sea hydrothermal vent. Int. J. Syst. Evol. Microbiol. 61, 2827–2881. doi: 10.1099/ijs.0.024653-0
Blöchl, E., Rachel, R., Burggraf, S., Hafenbradl, D., Jannasch, H. W., and Stetter, K. O. (1997). Pyrolobus fumarii, gen. and sp. nov., represents a novel group of archaea, extending the upper temperature limit for life to 113 degrees C. Extremophiles 1, 14–21. doi: 10.1007/s007920050010
Bourges, A. C., Lazarev, A., Declerck, N., Rogers, K. L., and Royer, C. A. (2020). Quantitative high-resolution imaging of live microbial cells at high hydrostatic pressure. Biophys. J. 118, 2670–2679. doi: 10.1016/j.bpj.2020.04.017
Cahet, G., Daumas, R., and Sibuet, M. (1990). In situ experimentation at the water/sediment interface in the deep sea: 2. Biotransformation of dissolved organic substrates by microbial communities at 2000m depth in the Bay of Biscay. Prog. Oceanogr. 24, 169–178. doi: 10.1016/0079-6611(90)90028-Z
Cario, A., Jebbar, M., Thiel, A., Kervarec, N., and Oger, P. M. (2016). Molecular chaperone accumulation as a function of stress evidences adaptation to high hydrostatic pressure in the piezophilic archaeon Thermococcus barophilus. Sci. Rep. 6:29483. doi: 10.1038/srep29483
Cario, A., Oliver, G. C., and Rogers, K. L. (2019). Exploring the deep marine biosphere: challenges, innovations, and opportunities. Front. Earth Sci. 7:225. doi: 10.3389/feart.2019.00225
Courtine, D., Vince, E., Maignien, L., Philippon, X., Gayet, N., Shao, Z., et al. (2021). Thermococcus camini sp. nov., a hyperthermophilic and piezophilic archaeon isolated from a deep-sea hydrothermal vent at the Mid-Atlantic Ridge. Int. J. Syst. Evol. Microbiol. 71:7. doi: 10.1099/ijsem.0.004853
Deming, J. W., Somers, L. K., Straube, W. L., Swartz, D. G., and Macdonell, M. T. (1988). Isolation of an obligately barophilic bacterium and description of a new genus, Colwellia gen. nov. Syst. Appl. Microbiol. 10, 152–160. doi: 10.1016/S0723-2020(88)80030-4
Deming, J. W., Tabor, P. S., and Colwell, R. R. (1981). Barophilic growth of bacteria from intestinal tracts of deep-sea invertebrates. Microb. Ecol. 7, 85–94. doi: 10.1007/BF02010480
Donaldson, E. C., Knapp, R. M., Yen, T. F., and Chilingarian, G. V. (1989). “The subsurface environment,” Developments in Petroleum Science Vol. 22. Elsevier. doi: 10.1016/S0376-7361(09)70090-1
Fang, J., Zhang, L., and Bazylinski, D. A. (2010). Deep-sea piezosphere and piezophiles: geomicrobiology and biogeochemistry. Trends Microbiol. 18, 413–422. doi: 10.1016/j.tim.2010.06.006
Fardeau, M.-L., Goulhen, F., Bruschi, M., Khelifi, N., Cayol, J.-L., Ignatiadis, I., et al. (2009). Thermophilic isolates from deep geothermal water of the Paris Basin. Geomicrobiol J. 26, 119–130. doi: 10.1080/01490450802674970
Foustoukos, D. I., and Pérez-Rodríguez, I. (2015). A continuous culture system for assessing microbial activities in the piezosphere. Appl. Environ. Microbiol. 81, 6850–6856. doi: 10.1128/AEM.01215-15
Garel, M., Bonin, P., Martini, S., Guasco, S., Roumagnac, M., Bhairy, N., et al. (2019). Pressure-retaining sampler and high-pressure systems to study deep-sea microbes under in situ conditions. Front. Microbiol. 10:453. doi: 10.3389/fmicb.2019.00453
Garel, M., Panagiotopoulos, C., Boutrif, M., Repeta, D., Sempere, R., Santinelli, C., et al. (2021). Contrasting degradation rates of natural dissolved organic carbon by deep-sea prokaryotes under stratified water masses and deep-water convection conditions in the NW Mediterranean Sea. Mar. Chem. 231:103932. doi: 10.1016/j.marchem.2021.103932
Grossart, H. P., and Gust, G. (2009). Hydrostatic pressure affects physiology and community structure of marine bacteria during settling to 4000 m: an experimental approach. Marine Ecology Progress Series 390, 97–104. doi: 10.3354/meps08201
Hartzell, P., and Reed, D. W. (2006). “The genus archaeoglobus,” in The Prokaryotes, (eds.) M. Dworkin, S. Falkow, E. Rosenberg, K.-H. Schleifer, and E. Stackebrandt (New York, NY: Springer) 82–100.
Hei, D. J., and Clark, D. S. (1994). Pressure stabilization of proteins from extreme thermophiles. Appl. Environ. Microbiol. 60, 932–939. doi: 10.1128/aem.60.3.932-939.1994
Huber, H., Jannasch, H., Rachel, R., Fuchs, T., and Stetter, K. O. (1997). Archaeoglobus veneficus sp. nov., a novel facultative chemolithoautotrophic hyperthermophilic sulfite reducer, isolated from abyssal black smokers.. Syst. Appl. Microbiol. 20, 374–380.
Huber, R., Woese, C. R., Langworthy, T. A., Fricke, H., and Stetter, K. O. (1989). Thermosipho africanus gen. Nov., represents a new genus of thermophilic eubacteria within the “Thermotogales”. Syst. Appl. Microbiol. 12, 32–37. doi: 10.1016/S0723-2020(89)80037-2
Ishii, A., Sato, T., Wachi, M., Nagai, K., and Kato, C. (2004). Effects of high hydrostatic pressure on bacterial cytoskeleton FtsZ polymers in vivo and in vitro. Microbiology 150, 1965–1972. doi: 10.1099/mic.0.26962-0
Jannasch, H. W., and Wirsen, C. O. (1977). Retrieval of concentrated and undecompressed microbial populations from the deep sea. Appl. Environ. Microbiol. 33, 642–646. doi: 10.1128/aem.33.3.642-646.1977
Jannasch, H. W., and Wirsen, C. O. (1984). Variability of pressure adaptation in deep-sea bacteria. Arch. Microbiol. 139, 281–288. doi: 10.1007/BF00408367
Jannasch, H. W., Wirsen, C. O., Molyneaux, S. J., and Langworthy, T. A. (1992). Comparative physiological studies on hyperthermophilic archaea isolated from deep-sea hot vents with emphasis on Pyrococcus strain GB-D. Appl. Environ. Microbiol. 58, 3472–3481. doi: 10.1128/aem.58.11.3472-3481.1992
Jebbar, M., Franzetti, B., Girard, E., and Oger, P. (2015). Microbial diversity and adaptation to high hydrostatic pressure in deep-sea hydrothermal vents prokaryotes. Extremophiles 19, 721–740. doi: 10.1007/s00792-015-0760-3
Kallmeyer, J., Pockalny, R., Adhikari, R. R., Smith, D. C., and D’Hondt, S. (2012). Global distribution of microbial abundance and biomass in subseafloor sediment. Proc. Natl. Acad. Sci. 109, 16213–16216. doi: 10.1073/pnas.1203849109
Kato, M., and Fujisawa, T. (1998). High-pressure solution X-ray scattering of protein using a hydrostatic cell with diamond windows. J. Synchrotron Radiat. 5, 1282–1286. doi: 10.1107/S0909049598000788
Kato, C., Li, L., Nakamura, Y., Nogi, Y., Tamaoka, J., and Horikoshi, K. (1998). Properties of hyper-barophilic bacteria isolated from the Mariana trench at a depth of 11,000m. Rev. High Press. Sci. Technol. (eds.) C. Michiels, D. H. Bartlett, and A. Aertsen (Washington, DC: © 2008 ASM Press). 7, 1274–1276. doi: 10.4131/jshpreview.7.1274
Kato, C., Nogi, Y., and Arakawa, S. (2008). “Isolation, cultivation, and diversity of deep-sea piezophiles” in High-Pressure Microbiology, 203–217.
Khelaifia, S., Fardeau, M. L., Pradel, N., Aussignargues, C., Garel, M., Tamburini, C., et al. (2011). Desulfovibrio piezophilus sp. nov., a piezophilic, sulfate-reducing bacterium isolated from wood falls in the Mediterranean Sea. Int. J. Syst. Evol. Microbiol. 61, 2706–2711. doi: 10.1099/ijs.0.028670-0
L'Haridon, S., Reysenbacht, A. L., Glenat, P., Prieur, D., and Jeanthon, C. (1995). Hot subterranean biosphere in a continental oil reservoir. Nature 377, 223–224. doi: 10.1038/377223a0
Li, X. G., Tang, H. Z., Zhang, W. J., Qi, X. Q., Qu, Z. G., Xu, J., et al. (2021). Thermococcus aciditolerans sp. nov., a piezotolerant, hyperthermophilic archaeon isolated from a deep-sea hydrothermal vent chimney in the southwest Indian ridge. Int. J. Syst. Evol. Microbiol. 71:004934. doi: 10.1099/ijsem.0.004934
Maldonado, J. A., Schaffner, D. W., Cuitiño, A. M., and Karwe, M. V. (2016). In situ studies of microbial inactivation during high pressure processing. High Pressure Res. 36, 79–89. doi: 10.1080/08957959.2015.1111887
Marteinsson, V. T., Moulin, P., Birrien, J., Gambacorta, A., Vernet, M., and Prieur, D. (1997). Physiological responses to stress conditions and barophilic behavior of the hyperthermophilic vent archaeon Pyrococcus abyssi. Appl. Environ. Microbiol. 63, 1230–1236. doi: 10.1128/aem.63.4.1230-1236.1997
Martinez, N., Michoud, G., Cario, A., Ollivier, J., Franzetti, B., Jebbar, M., et al. (2016). High protein flexibility and reduced hydration water dynamics are key pressure adaptive strategies in prokaryotes. Sci. Rep. 6, 1–11. doi: 10.1038/srep32816
McNichol, J., Sylva, S. P., Thomas, F., Taylor, C. D., Sievert, S. M., and Seewald, J. S. (2016). Assessing microbial processes in deep-sea hydrothermal systems by incubation at in situ temperature and pressure. Deep Sea Res. Part I Oceanogr. Res. Pap. 115, 221–232. doi: 10.1016/j.dsr.2016.06.011
Nakagawa, S., Takai, K., Inagaki, F., Chiba, H., Ishibashi, J. I., Kataoka, S., et al. (2005). Variability in microbial community and venting chemistry in a sediment-hosted backarc hydrothermal system: impacts of subseafloor phase-separation. FEMS Microbiol. Ecol. 54, 141–155. doi: 10.1016/j.femsec.2005.03.007
Nogi, Y., Hosoya, S., Kato, C., and Horikoshi, K.. (2004). Colwellia piezophila sp. nov., a novel piezophilic species from deep-sea sediments of the Japan Trench. Int. J. Syst. Evol. Microbiol. 54, 1627–1631. doi: 10.1099/ijs.0.03049-0
Oger, P. M., and Jebbar, M. (2010). The many ways of coping with pressure. Res. Microbiol. 161, 799–809. doi: 10.1016/j.resmic.2010.09.017
Oliver, G. C., Cario, A., and Rogers, K. L. (2020). Rate and extent of growth of a model extremophile, Archaeoglobus fulgidus, under high hydrostatic pressures. Front. Microbiol. 11:1023. doi: 10.3389/fmicb.2020.01023
Oliver, G. C., Cario, A., and Rogers, K. L. (2021). High temperature and high hydrostatic pressure cultivation, transfer, and filtration systems for investigating deep marine microorganisms. [Preprints]. doi: 10.20944/preprints202104.0453.v1.
Osman, J. R., Cardon, H., Montagnac, G., Picard, A., and Daniel, I. (2021). Pressure effects on sulfur-oxidizing activity of Thiobacillus thioparus. Environ. Microbiol. Rep. 13, 169–175. doi: 10.1111/1758-2229.12922
Overmann, J., Abt, B., and Sikorski, J. (2017). Present and future of culturing bacteria. Annu. Rev. Microbiol. 71, 711–730. doi: 10.1146/annurev-micro-090816-093449
Park, C. B., and Clark, D. S. (2002). Rupture of the cell envelope by decompression of the deep-sea methanogen Methanococcus jannaschii. Appl. Environ. Microbiol. 68, 1458–1463. doi: 10.1128/AEM.68.3.1458-1463.2002
Parkes, R. J., Cragg, B., Roussel, E., Webster, G., Weightman, A., and Sass, H. (2014). A review of prokaryotic populations and processes in sub-seafloor sediments, including biosphere: geosphere interactions. Mar. Geol. 352, 409–425. doi: 10.1016/j.margeo.2014.02.009
Patra, S., Anders, C., Erwin, N., and Winter, R. (2017). Osmolyte effects on the conformational dynamics of a DNA hairpin at ambient and extreme environmental conditions. Angew. Chem. 129, 5127–5131. doi: 10.1002/ange.201701420
Peoples, L. M., and Bartlett, Douglas H. (2017). “Ecogenomics of Deep-Ocean Microbial Bathytypes,” in Microbial Ecology of Extreme Environments. 7–50. doi: 10.1007/978-3-319-51686-8
Peoples, L. M., Norenberg, M., Price, D., McGoldrick, M., Novotny, M., Bochdansky, A., et al. (2019). A full-ocean-depth rated modular lander and pressure-retaining sampler capable of collecting hadal-endemic microbes under in situ conditions. Deep-Sea Res. I Oceanogr. Res. Pap. 143, 50–57. doi: 10.1016/j.dsr.2018.11.010
Peters, J., Martinez, N., Michoud, G., Cario, A., Franzetti, B., Oger, P., et al. (2014). Deep sea microbes probed by incoherent neutron scattering under high hydrostatic pressure. Z. Phys. Chem. 228, 1121–1133. doi: 10.1515/zpch-2014-0547
Picard, A., and Daniel, I. (2013). Pressure as an environmental parameter for microbial life: a review. Biophys. Chem. 183, 30–41. doi: 10.1016/j.bpc.2013.06.019
Picard, A., Daniel, I., Montagnac, G., and Oger, P. (2007). In situ monitoring by quantitative Raman spectroscopy of alcoholic fermentation by Saccharomyces cerevisiae under high pressure. Extremophiles 11, 445–452. doi: 10.1007/s00792-006-0054-x
Picard, A., Testemale, D., Wagenknecht, L., Hazael, R., and Daniel, I. (2015). Iron reduction by the deep-sea bacterium Shewanella profunda LT13a under subsurface pressure and temperature conditions. Front. Microbiol. 5:796. doi: 10.3389/fmicb.2014.00796
Postgate, J. R., and Campbell, L. L. (1966). Classification of Desulfovibrio species, the nonsporulating sulfate-reducing bacteria. Bacteriol. Rev. 30, 732–738. doi: 10.1128/br.30.4.732-738.1966
Raber, E. C., Dudley, J. A., Salerno, M., and Urayama, P. (2006). Capillary-based, high-pressure chamber for fluorescence microscopy imaging. Rev. Sci. Instrum. 77:096106. doi: 10.1063/1.2349303
Reveillaud, J., Reddington, E., McDermott, J., Algar, C., Meyer, J. L., Sylva, S., et al. (2016). Subseafloor microbial communities in hydrogen-rich vent fluids from hydrothermal systems along the mid-Cayman rise. Environ. Microbiol. 18, 1970–1987. doi: 10.1111/1462-2920.13173
Simonato, F., Campanaro, S., Lauro, F. M., Vezzi, A., D’Angelo, M., Vitulo, N., et al. (2006). Piezophilic adaptation: a genomic point of view. J. Biotechnol. 126, 11–25. doi: 10.1016/j.jbiotec.2006.03.038
Somero, G. N. (1992). Adaptations to high hydrostatic pressure. Annu. Rev. Physiol. 54, 557–577. doi: 10.1146/annurev.ph.54.030192.003013
Stetter, K. O. (1988). Archaeoglobus fulgidus gen. nov., sp. nov.: a new taxon of extremely thermophilic archaebacteria. Syst. Appl. Microbiol. 10, 172–173. doi: 10.1016/S0723-2020(88)80032-8
Stetter, K. O., Lauerer, G., Thomm, M., and Neuner, A. (1987). Isolation of extremely thermophilic sulfate reducers: evidence for a novel branch of archaebacteria. Science 236, 822–824. doi: 10.1126/science.236.4803.822
Sudo, S. Z., and Dworkin, M. (1973). Comparative biology of prokaryotic resting cells. Adv. Microb. Physiol. 9, 153–224. doi: 10.1016/S0065-2911(08)60378-1
Suzina, N. E., Mulyukin, A. L., Kozlova, A. N., Shorokhova, A. P., Dmitriev, V. V., Barinova, E. S., et al. (2004). Ultrastructure of resting cells of some non-spore-forming bacteria. Microbiology 73, 435–447. doi: 10.1023/B:MICI.0000036990.94039.af
Tabor, P., and Colwell, R. (1976). Initial investigations with a deep ocean in situ sampler. in IEEE OCEANS'76 September 1976, 324–327.
Takai, K., Nakamura, K., Toki, T., Tsunogai, U., Miyazaki, M., Miyazaki, J., et al. (2008). Cell proliferation at 122 C and isotopically heavy CH4 production by a hyperthermophilic methanogen under high-pressure cultivation. Proc. Natl. Acad. Sci. 105, 10949–10954. doi: 10.1073/pnas.0712334105
Tamburini, C. (2006). “Life under pressure. Deep-sea microbial ecology” in Life As We Know It. Series: Cellular Origin and Life in Extreme Habitats and Astrobiology ed. J. Seckbach (Berlin: Springer), 1–17.
Tamburini, C., Boutrif, M., Garel, M., Colwell, R. R., and Deming, J. W. (2013). Prokaryotic responses to hydrostatic pressure in the ocean–a review. Environ. Microbiol. 15, 1262–1274. doi: 10.1111/1462-2920.12084
Tamburini, C., Garcin, J., and Bianchi, A. (2003). Role of deep-sea bacteria in organic matter mineralization and adaptation to hydrostatic pressure conditions in the NW Mediterranean Sea. Aquat. Microb. Ecol. 32, 209–218. doi: 10.3354/ame032209
Usui, K., Hiraki, T., Kawamoto, J., Kurihara, T., Nogi, Y., Kato, C., et al. (2012). Eicosapentaenoic acid plays a role in stabilizing dynamic membrane structure in the deep-sea piezophile Shewanella violacea: a study employing high-pressure time-resolved fluorescence anisotropy measurement. Biochim. Biophys. Acta Biomembr. 1818, 574–583. doi: 10.1016/j.bbamem.2011.10.010
Whitman, W. B., Coleman, D. C., and Wiebe, W. J. (1998). Prokaryotes: the unseen majority. Proc. Natl. Acad. Sci. 95, 6578–6583. doi: 10.1073/pnas.95.12.6578
Yayanos, A. A. (1977). Simply actuated closure for a pressure vessel: design for use to trap deep-sea animals. Rev. Sci. Instrum. 48, 786–789. doi: 10.1063/1.1135150
Yayanos, A. A. (1978). Recovery and maintenance of live amphipods at a pressure of 580 bars from an ocean depth of 5700 meters. Science 200, 1056–1059. doi: 10.1126/science.200.4345.1056
Yayanos, A. A. (1995). “Microbiology to 10,500 Meters in the Deep Sea,” Annual Review of Microbiology 49, 777–805. doi: 10.1146/annurev.micro.49.1.777
Yayanos, A. A. (2001). 30 deep-sea piezophilic bacteria. Methods Microbiol. 30, 615–637. doi: 10.1016/S0580-9517(01)30065-X
Yayanos, A. A., and Dietz, A. S. (1983). Death of a Hadal deep-sea bacterium after decompression. Science 220, 497–498. doi: 10.1126/science.220.4596.497
Yayanos, A. A., Dietz, A. S., and Van Boxtel, R. (1979). Isolation of a deep-sea barophilic bacterium and some of its growth characteristics. Science 205, 808–810. doi: 10.1126/science.205.4408.808
Yayanos, A. A., Dietz, A. S., and Van Boxtel, R. (1981). Obligately barophilic bacterium from the Mariana trench. Proc. Natl. Acad. Sci. 78, 5212–5215. doi: 10.1073/pnas.78.8.5212
Yu, L., Jian, H., Gai, Y., Yi, Z., Feng, Y., Qiu, X., et al. (2021). Characterization of two novel psychrophilic and piezotolerant strains, Shewanella psychropiezotolerans sp. nov. and Shewanella eurypsychrophilus sp. nov, adapted to an extreme deep-sea environment. Syst. Appl. Microbiol. 44:126266. doi: 10.1016/j.syapm.2021.126266
Zeng, X., Birrien, J.-L., Fouquet, Y., Cherkashov, G., Jebbar, M., Querellou, J., et al. (2009). Pyrococcus CH1, an obligate piezophilic hyperthermophile: extending the upper pressure-temperature limits for life. ISME J. 3, 873–876. doi: 10.1038/ismej.2009.21
Zhang, H., Fang, J., Zhang, H., and Cao, J. (2022). Complete genome sequence of a psychrotolerant and piezotolerant bacterium Parasedimentitalea marina W43T, isolated from deep-sea water of the New Britain trench. Mar. Genomics 61:100915. doi: 10.1016/j.margen.2021.100915
Keywords: Desulfovibrio salexigens, Archaeoglobus fulgidus, high-pressure microbiology, microbial physiology, deep marine biosphere
Citation: Cario A, Oliver GC and Rogers KL (2022) Characterizing the Piezosphere: The Effects of Decompression on Microbial Growth Dynamics. Front. Microbiol. 13:867340. doi: 10.3389/fmicb.2022.867340
Received: 01 February 2022; Accepted: 20 April 2022;
Published: 17 May 2022.
Edited by:
Andreas Teske, University of North Carolina at Chapel Hill, United StatesReviewed by:
Philippe M. Oger, UMR5240 Microbiologie, Adaptation et Pathogenie (MAP), FranceCopyright © 2022 Cario, Oliver and Rogers. This is an open-access article distributed under the terms of the Creative Commons Attribution License (CC BY). The use, distribution or reproduction in other forums is permitted, provided the original author(s) and the copyright owner(s) are credited and that the original publication in this journal is cited, in accordance with accepted academic practice. No use, distribution or reproduction is permitted which does not comply with these terms.
*Correspondence: Anaïs Cario, YW5haXMuY2FyaW9AY25ycy5mcg==; Karyn L. Rogers, cm9nZXJrNUBycGkuZWR1
†Present addresses: Anaïs Cario, CNRS, Univ. Bordeaux, Bordeaux INP, ICMCB, Pessac, France
Gina C. Oliver, Department of Earth and Spatial Sciences San Bernardino Valley College, San Bernardino, CA, United States
‡These authors have contributed equally to this work
Disclaimer: All claims expressed in this article are solely those of the authors and do not necessarily represent those of their affiliated organizations, or those of the publisher, the editors and the reviewers. Any product that may be evaluated in this article or claim that may be made by its manufacturer is not guaranteed or endorsed by the publisher.
Research integrity at Frontiers
Learn more about the work of our research integrity team to safeguard the quality of each article we publish.