- 1Univ. Bordeaux, CNRS, Bordeaux INP, ICMCB, UMR 5026, Pessac, France
- 2CNRS, Univ. Brest, Ifremer, IRP 1211 MicrobSea, Unité de Biologie et Ecologie des Ecosystèmes Marins Profonds BEEP, IUEM, Plouzané, France
Here, we present a novel methodology based on high-pressure microfluidics to rapidly perform temperature-based phenotyping of microbial strains from deep-sea environments. The main advantage concerns the multiple on-chip temperature conditions that can be achieved in a single experiment at pressures representative of the deep-sea, overcoming the conventional limitations of large-scale batch metal reactors to conduct fast screening investigations. We monitored the growth of the model strain Thermococcus barophilus over 40 temperature and pressure conditions, without any decompression, in only 1 week, whereas it takes weeks or months with conventional approaches. The results are later compared with data from the literature. An additional example is also shown for a hydrogenotrophic methanogen strain (Methanothermococcus thermolithotrophicus), demonstrating the robustness of the methodology. These microfluidic tools can be used in laboratories to accelerate characterizations of new isolated species, changing the widely accepted paradigm that high-pressure microbiology experiments are time-consuming.
Introduction
The study of the deep biosphere is particularly concerned with the discovery and investigation of the deep microbial life inhabiting this remote environment. The case of marine environment is particularly interesting since if we consider that the deep biosphere begins in the ocean at depths greater than 1,000 m (Jannasch and Taylor, 1984) the deep-sea represents 65% of the Earth’s surface and 95% of its habitable space. The deep biosphere is estimated to account for more than ~15% of the total biomass on Earth (Whitman et al., 1998; Bar-On et al., 2018), and is known to be a major contributor to biogeochemical cycles (D'Hondt et al., 2004; Kallmeyer et al., 2012). In recent years, the deep ocean has been the focus of much attention because it hosts various ecosystems, contains biological resources—especially microbial—and mineral resources, and plays key functions for our planet (e.g., carbon storage, climate regulation, microbial degradation, receptacle of pollutants, etc.). However, despite its importance, the deep-sea remains sparsely documented, as does the deep microbial biosphere (Ramirez-Llodra et al., 2010). Knowledge of the deep biosphere is notably hampered by the difficulties inherent to its access, the technical difficulties to sample it, and the investigations at laboratory scale under realistic pressure conditions (up to 100 MPa), which require adapted equipment (Cario et al., 2019; Garel et al., 2019). Therefore, phenotyping (i.e., determining the detectable physical and biochemical characteristics of an organism or microbial strain) of microbial strains from deep-sea environments is time-consuming, given the large number of long and distinct experiments that must be performed—and repeated—in order to characterize a new strain, a mutant or simply to perform basic growth studies.
Since microorganisms in the deep biosphere experience diverse and severe conditions (Jørgensen and Boetius, 2007; Orcutt et al., 2013), characterization of microbial species at the laboratory scale requires specific high-pressure equipment and robust methodologies. Various culture-based and culture-independent approaches have attempted to describe both the microbial diversity and the array of metabolic capabilities present in these singular ecosystems, but with more effort devoted to omics-based studies in recent years at the expense of time-consuming culture-based approaches (Connon and Giovannoni, 2002; DeSantis et al., 2007; Rinke et al., 2013; Chen et al., 2021). The cutting-edge approaches for isolation and cultivation of prokaryotes such as microencapsulation, single-cell and droplet-based cultivation, and cell sorting based cultivation (e.g., reverse genomics; Zengler et al., 2005; Nichols et al., 2010; Boitard et al., 2015; Jiang et al., 2016; Berdy et al., 2017; Terekhov et al., 2018; Hu et al., 2020; Lewis et al., 2021) are very promising but cannot be applied to all microbial taxa as they do not mimic all environmental conditions, and in particular high temperature and pressure. Despite these significant achievements, the vast majority of the genera and phyla of bacteria and archaea on Earth, including those from the deep biosphere, do not have cultured representatives (Whitman et al., 1998; Lloyd et al., 2018). The low cultivation scores can be explained in part by the fact that many cultures are grown at atmospheric pressure, whereas in their in situ habitat, microorganisms are subjected to high hydrostatic pressures. Together with temperature and chemistry, pressure is one of the parameters driving the distribution and the microbial activities in the biosphere (Fry et al., 2008; Reith, 2011; Picard and Daniel, 2013; Jebbar et al., 2015) and is of great importance to isolate new strains and to study their physiology. Having a larger number of deep biosphere isolates grown under different catabolic conditions and characterized in terms of temperature range and growth pressure would shed light on their putative function in their natural habitat and in the geochemical cycles of their ecosystem.
To reproduce the extreme conditions of pressure, temperature and geochemistry at the laboratory scale, the conventional equipment is large reactors (from a few tens of milliliters to a few liters) made of stainless steel or other metals (e.g., Inconel, Hastelloy, titanium alloys, etc.), which have demonstrated excellent thermo-mechanical properties along with acceptable chemical compatibility and bio compatible properties (Kato et al., 1996; Deusner et al., 2010; Czernichowski-Lauriol et al., 2017; Garel et al., 2019). Such reactors are used to achieve desired culture conditions representative of the deep biosphere environment to mimic the conditions encountered by deep environment microbes, while beneficiating from a laboratory-scale environment (Yayanos, 1986; Jannasch et al., 1996; Nauhaus et al., 2002; Kato, 2011). Although several new and/or updated sampling equipment and bioreactors have been developed allowing pressure and temperature conditions to be maintained (Garel et al., 2019; Peoples et al., 2019), there is still a lack of research equipment that allows for both (i) pressure-retention during analysis, (ii) ability to implement in situ characterization techniques—such as simple visualization, and (iii) fast screening capabilities. Several recent developments have overcome some of these limitations by allowing equi-pressure transfer of samples from reactor to reactor (Garel et al., 2019; Oliver et al., 2021) or by inserting sapphire windows within metal reactors, allowing performing microscopic or spectroscopy investigations (Bao et al., 2010; Maldonado et al., 2016). Such developments have somehow reduced the need for depressurization during analysis but remain scattered. Besides, some limitations remain: large-scale reactors can generate undesirable gradients—temperature, chemical composition—which could induce a bias in the analysis. In addition, these experimental set-ups do not allow for rapid screening experimentation, generally requiring weeks or even months to fully characterize a strain, which significantly slows the speed at which phenotyping can be performed. Therefore, the field of high-pressure microbiology needs to focus on new approaches and instruments to overcome the classical limitations of culturing and studying deep biosphere microorganisms, which are critical for advancing environmental microbiological research. An interesting option to address this gap involves the use of microfluidic reactors.
Over the past 20 years, microfluidics has greatly contributed to the progress of (micro)biology research, including the integration of analytical techniques (Demello, 2006) with cell handling (El-Ali et al., 2006), biochemical assays, which have been dedicated to various research areas such as medical investigations (Sackmann et al., 2014), environmental microbiology (Dusny and Schmid, 2015; Kaminski et al., 2016), etc. These tools offer several advantages over conventional large-scale devices. Indeed, microreactors offer a solution for temperature and feed flow control, reproducibility, in situ monitoring (Demello, 2006), rapid parameter screening (Jensen et al., 2000), fast mass and heat transfer (Gervais and Jensen, 2006), and low sample consumption. The combination of size reduction, single-phase and/or multi-phase in situ flows and improved reliability, can be recorded in a fast screening methodology development policy. Indeed, microfluidics generates a large amount of data and is already used as a high-throughput cultivation platform for conventional microbiology (i.e., non-extremophilic microorganisms) where hundreds to thousands of microbial colonies at a single-cell level can be studied by automated time-lapse microscopy, leading to a better understanding of microbial behavior and physiology (Moffitt et al., 2012; Grünberger et al., 2015). Conventional microfluidics uses PDMS (polydimethylsiloxane) materials, a polymer that is not compatible with extreme cultivation conditions (e.g., anoxic, high salinity, and high-pressure conditions). Nevertheless, microreactors have been already used to study environmental soil microbiology (Pucetaite et al., 2021) but also marine microbiology, under normal conditions (i.e., non-extremophilic) using droplet-based microfluidics (Girault et al., 2019). Meanwhile, technological developments have been made to use microfluidics for geochemical and microbiological investigations in deep-sea waters, considering microreactors as in situ (bio)chemical sensors/detectors (Wang et al., 2009; Beaton et al., 2022). In that case, the microreactor undergoes isostatic pressure both externally and internally, therefore not requiring mechanically resistant materials for its fabrication. Besides, microflows have also permitted to develop flow cytometry (Adan et al., 2017) at single cell level for the sorting and further identification of strains in complex environmental samples using either shape and/or fluorescence (fluorescent labeling molecules) detection, although no demonstration has been reported so far for high-pressure conditions. In order to extend the range of microfluidics to high-pressure microbiology investigations as well as deep environmental microbiology at laboratory scale, two strategies have been considered. First, researchers have used transparent capillary tubings (e.g., glass, silica, and sapphire), either with circular or square crossed section, for studying deep environment microorganisms using visible or fluorescence imaging (Beney et al., 1997; Raber et al., 2006; Bourges et al., 2020). Such capillaries offer affordable and easy strategies to access microbial characterization under high pressure, up to 100 MPa. Secondly, the use of diamond anvil cell or specific high-pressure cells, combined to spectroscopy techniques are also used for in situ monitoring and characterization studies under high-pressure conditions (Kato and Fujisawa, 1998; Molina-Gutierrez et al., 2002; Oger et al., 2006; Picard et al., 2007; Peters et al., 2014) but are not yet widespread because of specific and costly equipment.
Despite their ease of implementation, capillaries suffer from difficulties in accessing elaborate fluidic designs and controlling temperature. Therefore, it is difficult to envision their use for high throughput screening studies, in particular, for microbial phenotyping.
To address these limitations, on chip high-pressure microreactors have been recently developed (Marre et al., 2010) and utilized for instance for thermodynamics investigations (Xu et al., 2017; Gavoille et al., 2019) or deep underground fluids flow and geochemistry studies (Morais et al., 2016, 2020). High-pressure microreactors can be made out of silicon and Pyrex (semi-transparent), glass–glass (Murphy et al., 2007; Tiggelaar et al., 2007), or even sapphire (i.e., fully transparent; Marre et al., 2021). Such tools benefit from all the advantages of microfluidics, while being compatible with the representative pressure and temperature conditions inherent to deep environment. With the same ability than their room pressure counter-parts, high-pressure on-chip microreactors provide the ability to screen multiple conditions in a single experiment, paving the way for the study of the deep biosphere in the laboratory using new rapid screening methodologies.
In this article, we demonstrate for the first time the use of a novel transparent, high-pressure, biocompatible microfluidic platform for ultra-fast screening of temperature-dependent growth conditions of microorganisms from deep environments. The concept is demonstrated using two marine species with different metabolic strategies: Thermococcus barophilus MPT (Marteinsson et al., 1999), a model piezo-hyperthermophilic and heterotrophic strain isolated from a deep-sea hydrothermal vent and Methanothermococcus thermolithotrophicus, a thermophilic and a model hydrogenotrophic methanogen strain isolated from a seafloor geothermal spring (Huber et al., 1982). After introducing the overall methodology, design and fabrication of the microreactor, we present the investigation protocol and the obtained results, which are compared to the literature data.
Materials and Methods
Model Strains and Growth Media
We detailed hereafter the two considered strains along with the associated growth media, which were used in this study.
Thermococcus barophilus
Thermococcus barophilus strain MPT (DSM 11836) was obtained from the DSMZ collection (Deutsche Sammlung von Mikroorganismen und Zellkulturen, Braunschweig, Germany). It is a piezo-hyperthermophilic strain, which was isolated from a deep-sea hydrothermal vent (Snake Pit) on the Mid-Atlantic Ridge (depth, 3,550 m; Marteinsson et al., 1999). It grows from ambient pressure to 80 MPa and exhibits an optimal temperature for growth of 85°C over a wide pressure range (0.3–40 MPa; Marteinsson et al., 1999; Zeng et al., 2009). In this study, the T. barophilus cultures were carried out in a Thermococcales Rich Medium (TRM, pH 6.8; Zeng et al., 2009), under anoxic conditions. After autoclaving at 121°C during 30 min, the medium was aliquoted into several sterile Hungate tubes (10 ml), sealed with butyl rubber septa, reduced by adding 0.5% reductant (v/v, polysulfides stock solution of 0.05 M; Ikeda et al., 1972), and placed under an inert atmosphere (Ar, 30 kPa). The reductant was also used as a soluble source of sulfur for T. barophilus cells.
Methanothermococcus thermolithotrophicus
Methanothermococcus thermolithotrophicus strain SN-1T (DSM 2095) was obtained from the DSMZ collection (Deutsche Sammlung von Mikroorganismen und Zellkulturen, Braunschweig, Germany). It is a thermophilic and hydrogenotrophic methanogen archaeon isolated from a geothermally heated seafloor in Italy (Huber et al., 1982). This strain is able to grow on a wide temperature range, from 30°C to 70°C (Huber et al., 1982) with an optimum temperature at 65°C, and displays a piezophilic behavior when growing under elevated pressure conditions (i.e., 50 MPa; Bernhardt et al., 1988). In this study, M. thermolithotrophicus was cultivated in the AGW (Artificial Ground Water) medium (Dupraz et al., 2013) supplemented with 120 mM HEPES. The anoxic cultures were performed optimally at 65°C in serum flasks and with a 0.2 MPa gas mixture (mol/mol) of 80% H2 and 20% CO2, with a final cell density in stationary phase reaching 2×108 cells.ml−1. When growing at elevated pressure conditions, the H2/CO2 partial pressure was established either at 2 or 3 MPa and supplemented with inert gas (Nitrogen) to reach the desired total pressure in the set up (i.e., 5 and 10 MPa, respectively). Only the initial (T0) and end-points (T = 18 h of growth) were considered to evaluate the growth of this strain into the high-pressure microfluidic temperature gradient setup.
In all cases, the pre-cultures in mid-exponential phase of growth were used to inoculate Hungate tube and then transferred to the high-pressure microfluidic reactors with an initial cell concentration of ~5.106 cells.ml−1. In the case of T. barophilus, for which a full phenotyping study was performed, cell concentrations in control culture tubes at 85°C (Hungate tube for ambient pressure condition and high-pressure vessels for high-pressure conditions) were assessed by direct cell counting onto a Thoma chamber (Preciss, France; surface area: 0.0025 mm2, depth: 0.1 mm) using a DM2000 LED phase contrast optical microscope (Leica Microsystems CMS GmbH, Germany).
Microreactor Design and Set-up
The overall strategy for performing multi-temperature culture of microorganisms under pressure on a chip is to take advantage of microfluidic pools, which will serve as micro-wells, each exposed to a particular temperature condition. Microbial growth can then be monitored directly in situ for each pool by microscopic counting. We detail hereafter the microreactor design and dimensioning strategy employed to achieve this goal.
The microreactors were fabricated using the well-known silicon/Pyrex technology (Marre et al., 2010). This microfabrication technology is chosen for: (i) the optical access through the Pyrex side, allowing in situ characterization by optical microscopy, (ii) the excellent thermomechanical properties of silicon and Pyrex, allowing to work in a wide range of pressure and temperature conditions, and (iii) the biochemical inertness of both materials ensuring a good biocompatibility. The microreactors were fabricated using standard photolithography, wet etching and anodic bonding process, as previously described in the literature (Marre et al., 2010). The microreactor (Figure 1A) consists of one inlet and one outlet, used to inject the inoculated growth medium. It displays a U-shaped central microchannel (width: w = 200 μm, depth: d = 30 μm and length: L = 95 mm), which is used to deliver the fluid to 10 pairs of regularly spaced quasi-circular culture micropools on each branch, in which the microorganisms grow (diameter: D = 300 μm, depth: d = 30 μm, V = 1.5 nl or 1.9 nl, depending on the experiments). Each pool are placed on either side of the main channel and connected by a restriction channel (50 μm wide).
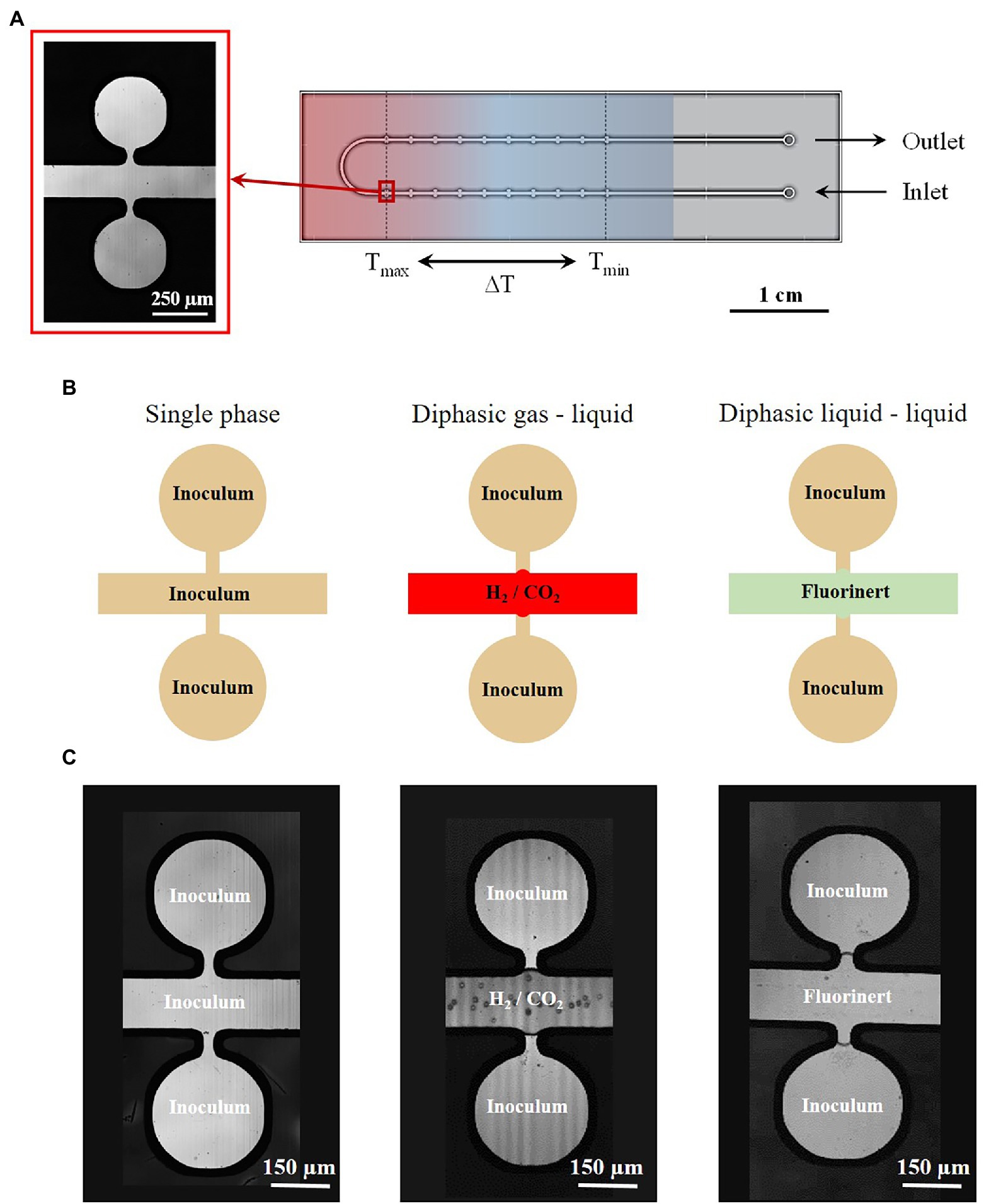
Figure 1. (A) Design of the temperature-gradient microreactor developed and used in this study with a microscope picture of the micropools used for both Thermococcus barophilus and Methanothermococcus thermolithotrophicus cultivation. (B) Different feeding strategies implemented into the microfluidic setup using growth medium phases and several interfaces. (C) Pictures of the concerning cultivation interfaces into the microreactor.
The main interest of this design stands in the possibility not only to work in a single phase mode were the inoculum occupied both the micro-chambers and the main channel but also to work in diphasic mode aiming at using a fluid non miscible with the inoculum for both “sealing” and feeding the micro-chambers with molecules through phase transfer (Figure 1B). In these cases, both liquids and gases may be considered. The microreactor surface being hydrophilic, the wetting phase is the inoculum. Hence, the wettability properties favor the inoculum to stay inside the micro-chamber (Figure 1B). However, the restriction channels are used to prevent any invasion of the micro-chambers with the second phase due to fluid flows during the microreactor filling procedure.
The microreactor is in contact with a temperature gradient generating device ensuring a linear temperature gradient between a Tmin (either 60.1°C or 72.5°C for M. thermolithotrophicus and T. barophilus, respectively) and a Tmax (either 70°C or 95°C for M. thermolithotrophicus and T. barophilus, respectively) along the microchip (Figure 2; Supplementary Information 1). Thus, 10 different temperatures could be examined simultaneously in a single experiment, while four different microchambers were subjected to the same temperature, providing a fourfold measurement to estimate the variability of results (Supplementary Figure S1). For T. barophilus, experiments were performed at three different pressures, 0.1, 5, and 10 MPa, in microreactors with 1.5 nl chambers. In parallel, experiments at 0.1 MPa (control) and 15 MPa were performed in microreactors with 1.9 nl chambers to demonstrate the robustness of the approach, independent of the microreactor itself. The microreactor with the 1.9 nl chambers was used for M. thermolithotrophicus experiments.
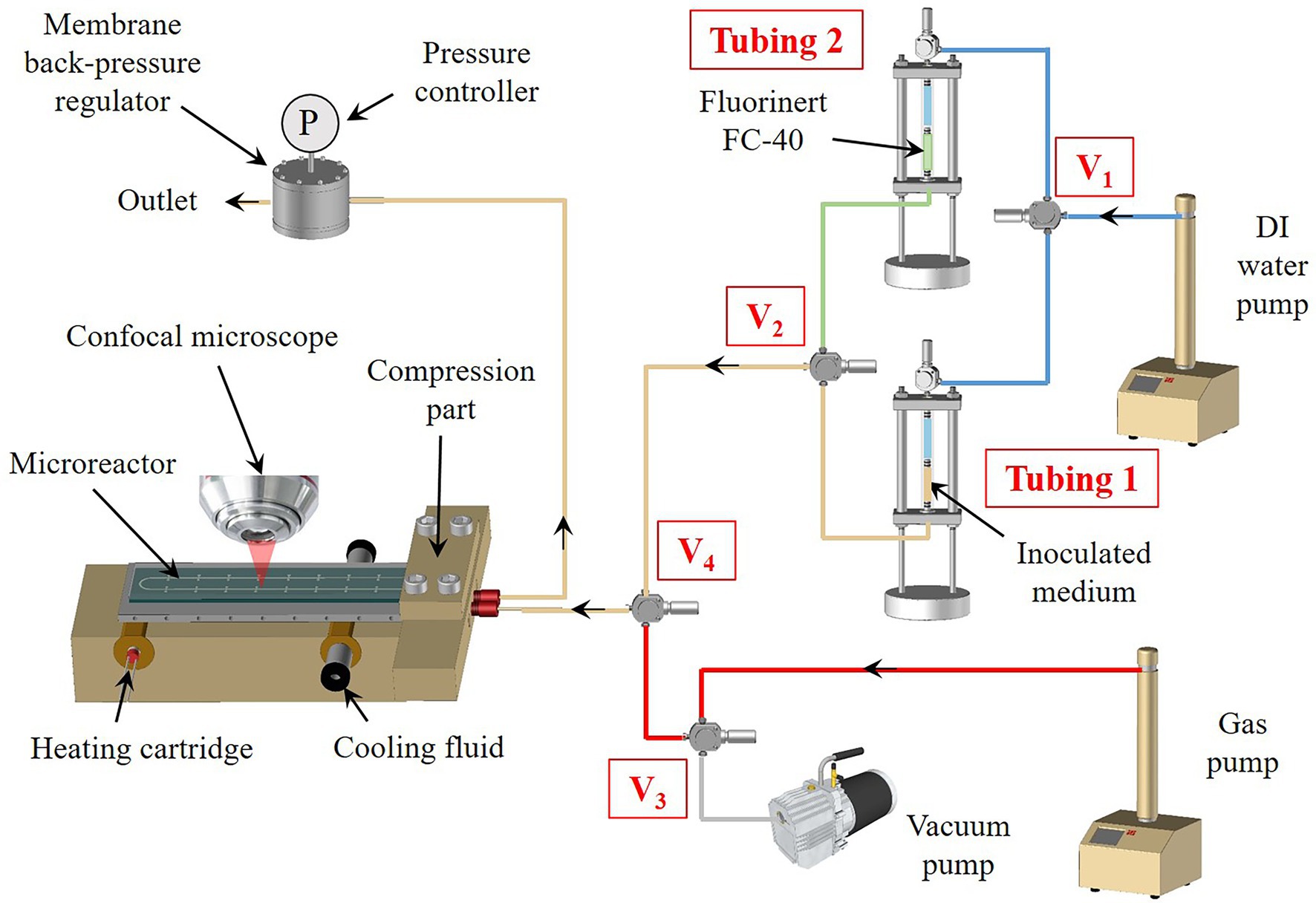
Figure 2. Set-up of the high-pressure microfluidics platform for phenotyping microorganisms at temperature (see the description in the text).
In a typical experimental set-up (Figure 2), the high-pressure temperature-gradient microreactor is connected to external fluid management equipment using a compression part, as described previously (Marre et al., 2010), made of polyetheretherketone (PEEK) for its biocompatibility characteristics and thermomechanical stability. The microreactor is placed on the temperature gradient system, which consists of an insulating block made of PEEK (for its thermal insulation properties) inside which two copper cylinders are inserted. A heating cartridge is inserted in the first cylinder, to generate a stable hot temperature zone using a Eurotherm temperature controller. The second cylinder is cooled to a targeted low temperature using a water flow provided by a circulating cryostat bath. To ensure a perfectly linear thermal gradient, an aluminum plate is placed in contact between the two copper cylinders, right below the microreactor (Figure 2; Supplementary Figure S1). A thermal paste is deposited between the aluminum and the microreactor to ensure excellent heat transfer. The whole assembly (i.e., microreactor + compression part + temperature gradient device) is placed under a confocal LASER scanning microscope. The compression part is connected to transparent tubes containing the fluids and equipped with movable pistons, in order to push the fluids into the microreactor.
Experimental Protocol
We have used two distinct protocols depending on both the considered strain and their growth strategy. Each protocol corresponds to a specific method depending on whether we considered a single fluid, a diphasic liquid–liquid or a gas–liquid approach. In a typical high-pressure microfluidic experiment, the inoculation was performed in sterile and anoxic ways with either T. barophilus or M. thermolithotrophicus.
First, a Hungate tube containing 10 ml of either TRM medium supplemented with polysulfides for T. barophilus or AGW medium for M. thermolithotrophicus (see the growth medium above) was inoculated from a mid-exponential phase pre-culture to reach a final cell concentration of 5 × 106 cells.ml−1. This cell concentration was chosen to ensure filling all the micropools in the microfluidic setup with 5–10 cells. Indeed, preliminary tests have shown that by considering a lower cell concentration, some micropools were empty. After inoculation, the Hungate tube was homogenized and 2.0 ml were transferred in a 5 ml high-pressure transparent tube equipped with a movable piston (Tubing 1, Figure 2), which had been first degassed (five cycles of N2−30 kPa/vacuum) to maintain anoxic conditions. This latter was then closed and connected via PEEK caps and connectors to a Teledyne ISCO high-pressure piston pump, previously filled with DI water as a pressurization fluid through a 3-way valve (V1, Figure 2). The pressure was applied by pushing with DI water on the mobile piston. The microreactor was first vacuumed to remove the air phase inside the device and later flushed with inert gas (N2) by opening valves V3 and V4 (Figure 2). This cycle was reproduced three times and the microreactor was finally left under vacuum to ensure getting rid of any gas bubbles in the micro-chambers during the filling procedure. Then, the microreactor was filled by delivering the inoculated growth medium at a flowrate of 50 μl.min−1 by opening V2 and V4 (Figure 2). The pressure inside the full set-up was maintained thanks to a membrane back pressure regulator (Equilibar) placed downstream the microreactor, enabling precise control of the pressure down to ultra-low flowrates (Figure 2).
When considering single-phase growth strategy (i.e., with T. barophilus), the pressure was slowly increased using the ISCO syringe pump (30 MPa.h−1) to the desired conditions. The pump was then set into a pressure constant mode at the working pressure. The remaining 8.0 ml of the inoculated medium was divided to serve as both a high-pressure positive control (4.0 ml corresponding to the high-pressure experimental pressure conditions) and a room pressure control (4.0 ml), at 85°C. In parallel, a 10-ml negative control (growth medium without inoculum) was performed to ensure there would be no contamination issues during the experiment.
When considering a diphasic gas–liquid growth strategy (i.e., with M. thermolithotrophicus), once the microreactor has been filled with the liquid inoculum, the pressure was set to the desired operating pressure and valve V2 was closed. Then, the gas phase (H2/CO2 mixture, 80/20 mol/mol, supplemented with N2 to reach the desired total pressure) was first pressurized inside the high-pressure syringe pump (Gas pump, Figure 2) up to the working pressure. Valve V3 was opened in order to inject the gas phase inside the microreactor at a slow flowrate (1 μl.min−1) to avoid any undesirable invasion of the microchambers by the gas phase, resulting in gas–liquid interfaces between the microchambers and the main microchannel (Figures 1B,C).
Potentially, in the case of a diphasic liquid/liquid growth strategy (not utilized in this study), the same procedure can be applied: first, a pressurization of the microreactor with the inoculum, followed by the injection of an immiscible liquid (e.g. Fluorinert FC40), through the switch of the three-way valves V1 and V2 to inject the Fluorinert from Tubing 2 (Figure 2) to the microreactor. Similarly, as for gases, a low flowrate is applied (1 μl.min−1) to avoid any invasion of the microchambers by the inert fluid (Figures 1B,C).
Image Analysis: Pool Area Measurement and Cell Counting
The initial cell concentration (about 4–5 × 106 cells.ml−1 depending on the micropool volume) was experimentally confirmed by counting the cells in each micropool. As a starting point, the number of cells per pool was typically 8 ± 3 cells. Then, the growth rate in each micropool was determined as follows (Figure 3A). First, the volume of each pool was accurately determined using 3D confocal imaging followed by image analysis (ImageJ©), as detailed in Supplementary Information 3. Cell counting overtime (Figure 3B) was then performed based on image captures made with a confocal microscope (Leica Microsystems, SP8), at 40× objective (in reflection mode) using the Leica LasX© software. OpenCFU software (Geissmann, 2013) was used for semi-automatic counting and for more accurate cell counting. Since both T. barophilus and M. thermolithotrophicus cells are tiny cocci (0.8–2.0 μm diameter; Huber et al., 1982; Marteinsson et al., 1999), the processing parameters of OpenCFU were adjusted to detect both species in the micro-chambers (see Supplementary Information 3 for details concerning the choosen OpenCFU parameters). Data were then combined to plot the growth curve for each micropool (Figure 3C), which all exhibit the classical shape of microbial growth curve (Supplementary Figure S2; i.e., lag phase, exponential phase and stationary phase—the death phase was not detected in here due to the “short” incubation time of 24 h max considered in our experiments). Finally, an extrapolation of the number of cells in the micropools was performed to obtain a normalized cell concentration (cells.ml−1) over time (Supplementary Figure S2). This extrapolation was done using the following formula:
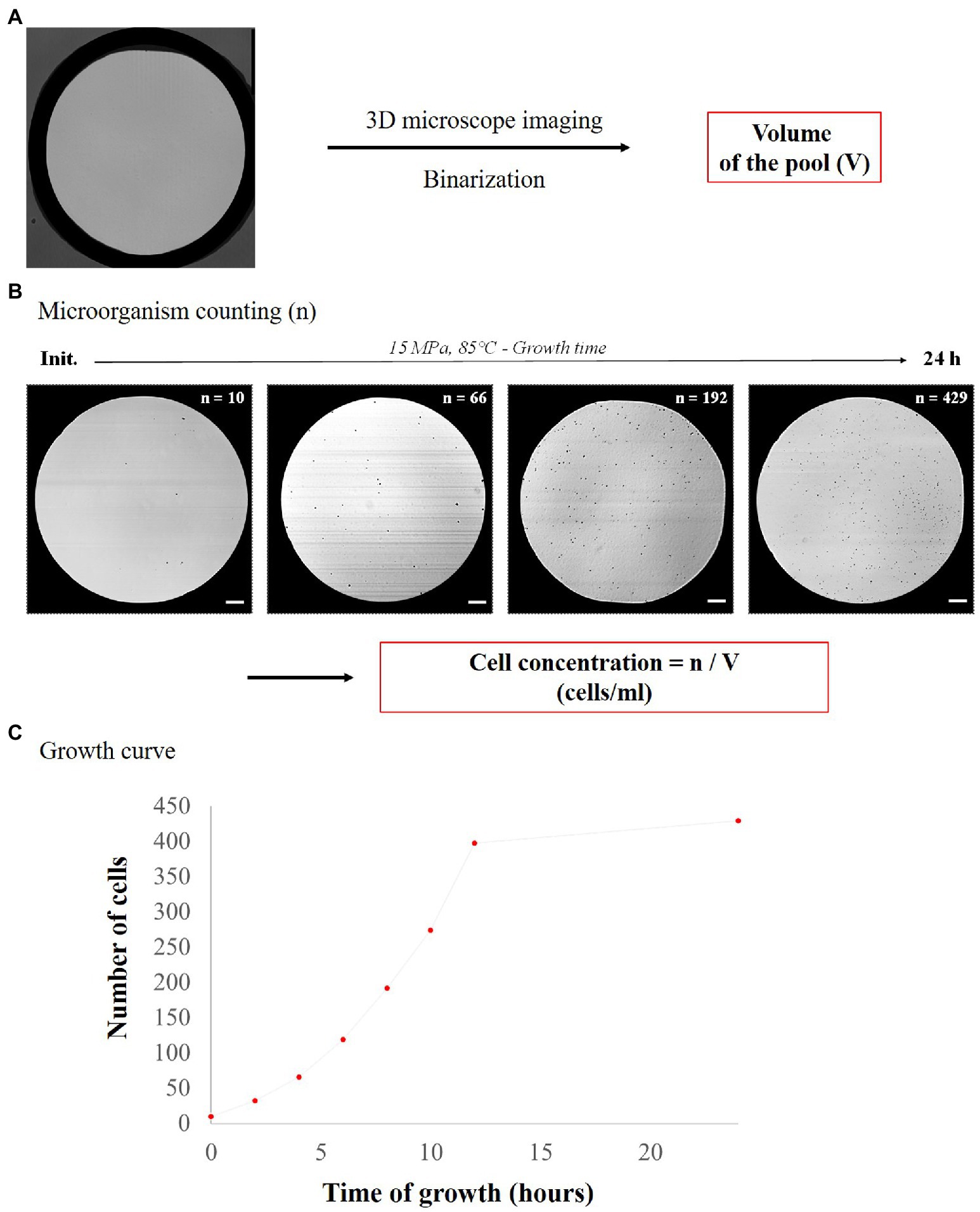
Figure 3. Schematic flowsheet for determination of cell concentration in each pool. (A) Determination of micropool volumes using 3D confocal microscopy and ImageJ analysis and (B) examples of pictures of Thermococcus barophilus growth over time with the corresponding cell number (n) at 15 MPa and 85°C (scale bar = 25 μm), allowing determination of cell concentration (cells.ml−1). (C) Growth curve with cell number versus time (15 MPa, 85°C).
C = n/V.
with C corresponding to the cell concentration (cells.ml−1), V to the volume of the micropool and n to the number of cells per pool. After conversion of cell concentrations to logarithmic values, growth rates were calculated from the logarithmic growth phase slopes of quadruplicate micropool culture experiments using the LINEST function in Excel. Error bars indicate the standard error from linear regressions of quadruplicate experiments (four micropools for one temperature data point) using the microfluidic temperature gradient chip (Figure 4). Maximum cell densities were measured from the stationary phase for all pressure conditions and error bars indicate standard deviation of quadruplicate experiments (Figure 4 for T. barophilus and Figure 5 for M. thermolithotrophicus). For T. barophilus, an overnight positive control (batch culture in 10 ml flask for atmospheric pressure and 5 ml for high-pressure millifluidic vessel under high-pressure conditions) at 85°C with the same cell inoculum as in the microfluidic experiment was always performed to ensure cell viability. Since the conventional high-pressure experiment requires decompression, only an endpoint measurement (after 12 h of growth) was considered for cell counting and compared to microfluidic growth at the same time-period, to avoid any growth bias due to decompression.
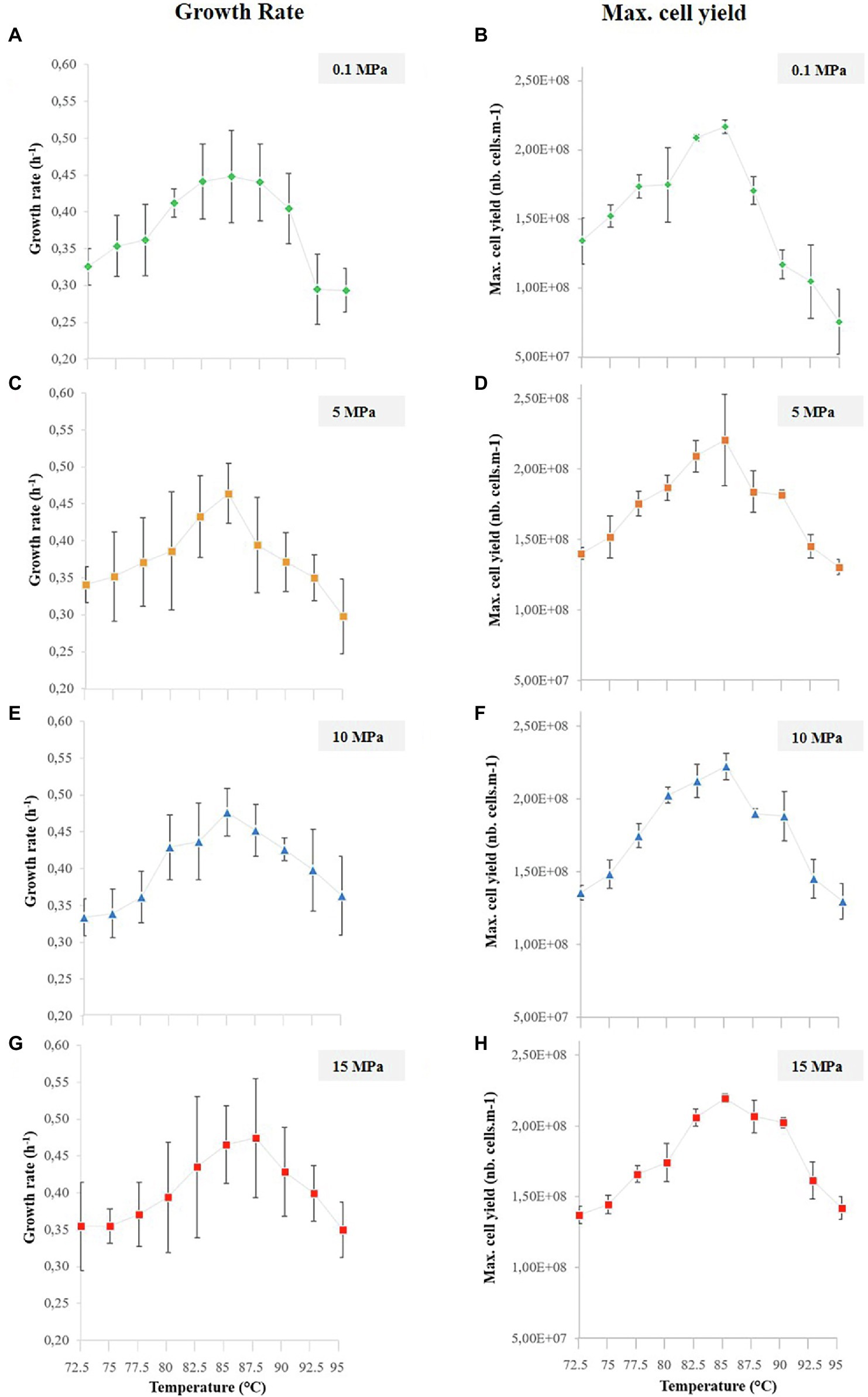
Figure 4. Thermococcus barophilus growth rates (left column) and maximal cell yields (right column) over 10 temperature conditions (i.e., 72.5°C–95°C) for several pressure conditions, while growing in the temperature gradient on-a-chip: (A) T. barophilus growth rates at 0.1 MPa. (B) T. barophilus maximum cell densities at 0.1 MPa. (C) T. barophilus growth rates at 5 MPa. (D) T. barophilus maximum cell densities at 5 MPa. (E) T. barophilus growth rates at 10 MPa. (F) T. barophilus maximum cell densities at 10 MPa. (G) T. barophilus growth rates at 15 MPa. (H) T. barophilus maximum cell densities at 15 MPa. Error bars represent the SD from the four replicates on a single experiment using the gradient on chip microfluidic setup.
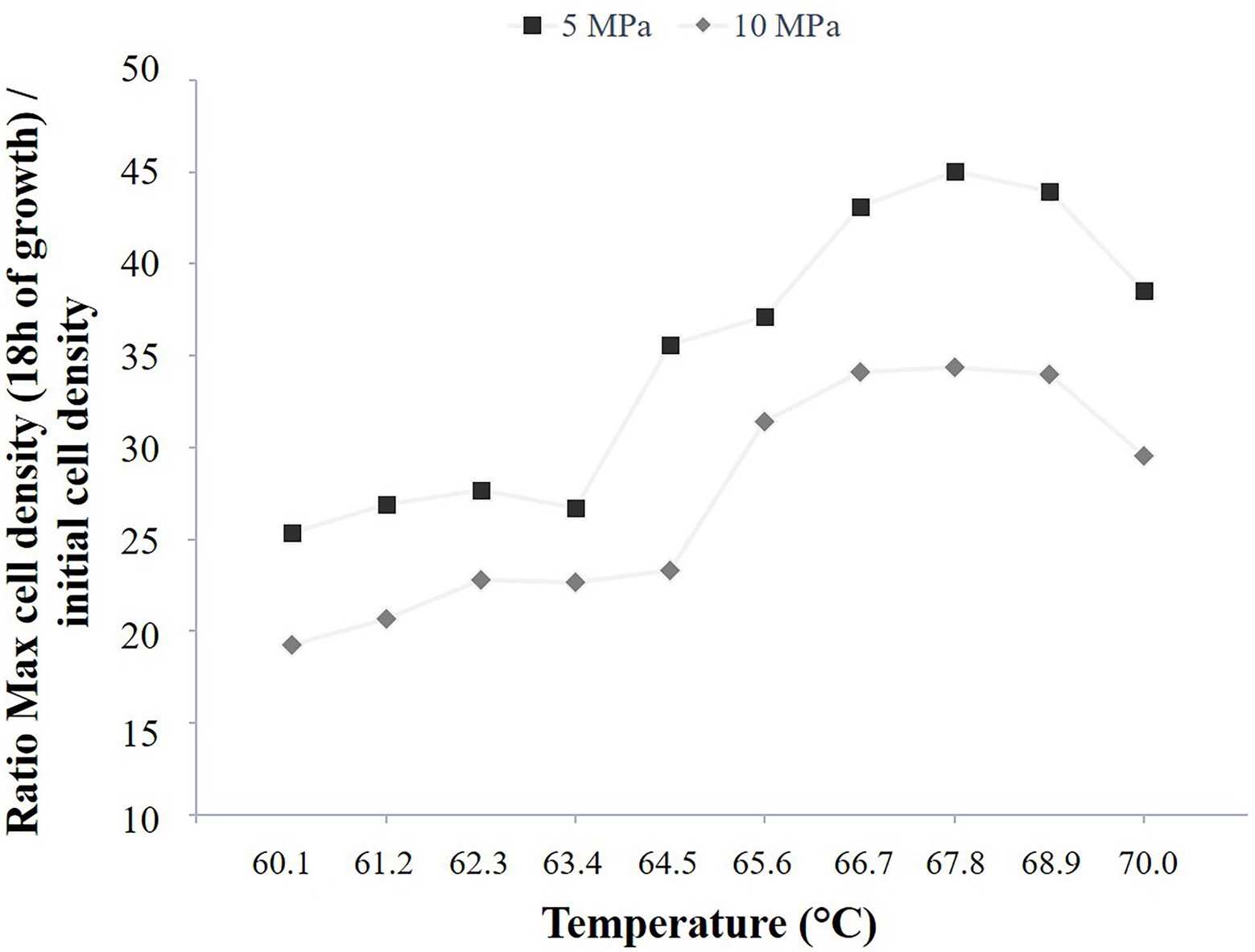
Figure 5. Methanothermococcus thermolithotrophicus growth comparison in a temperature gradient-on-a-chip between two-pressure conditions, 5 and 10 MPa total pressure (2 and 3 MPa of partial pressure of H2/CO2, respectively). Ratio of the mean cell density after 18 h of growth to the corresponding mean initial cell density, for two temperature gradient microfluidic experiments (temperature: 60.1°C–70°C).
Results
Thermococcus barophilus Phenotyping
Images taken overtime showed an increasing number of cells in the micropools (up to 24 h of growth), regardless of the pressure or temperature considered. This allowed us to estimate the evolutionary growth of T. barophilus (Figures 3B,C, 4) as a function of temperature for different pressures. Each experiment is performed at a single pressure (0.1, 5, 10, and 15 MPa, respectively), but gathers 10 different temperature conditions (72.5°C–95°C) in quadruplicate (i.e., 40 micro-batch experiments in a single run). Based on these images, cell numbers were correlated to the corresponding cell concentrations in cells.ml−1, allowing the phenotype of T. barophilus to be screened under 40 different P/T conditions and obtaining 40 growth curves in only 1 week of experiments (Supplementary Figure S2).
To compare the combined effects of high pressure (without decompression) and temperature on T. barophilus growth already reviewed in the literature, we plotted the growth rates and maximum cell yields (Figure 4) obtained in each pressure experiments on the temperature gradient on-a-chip. Average growth rates and maximal cell yields correspond to replicate of four microbial growths in a single experiment. The average growth rates ranged from 0.29 ± 0.03 to 0.45 ± 0.06, 0.30 ± 0.05 to 0.46 ± 0.04, 0.33 ± 0.02 to 0.48 ± 0.03 h, and 0.35 ± 0.04 to 0.47 ± 0.05 h−1 for 0.1, 5, 10, and 15 MPa pressure conditions, respectively (Figures 4A,C,E,G left column). The temperature-dependent pattern was similar for all four pressure conditions: growth rates were the highest for a temperature close to the known optimal temperature for T. barophilus (i.e., 85°C; Marteinsson et al., 1999), and decreased when moving away from the optimum, which is in accordance with previously published data. It is interesting to note that, due to the large amount of data available, the growth rate curves could be plotted with slightly better accuracy (2.5°C shift between each condition) than that obtained with the classical approach. It can also be observed that the temperature evolution of the growth rates shifted slightly to higher temperature values when growth was performed at higher pressure conditions (5, 10, and 15 MPa), however, the optimal growth temperature was not affected by the pressure variations in the explored range.
This shift was also observed with the maximum cell yields which ranged from 7.54 ± 2.33 × 107 to 2.17 ± 0.05 × 108, 1.30 ± 0.05 × 108 to 2.17 ± 0.32 × 108, 1.30 ± 0.12 × 108 to 2.22 ± 0.09 × 108, and 1.37 ± 0.06 × 108 to 2.19 ± 0.30 × 108 cells.ml−1 for 0.1, 5, 10, and 15 MPa pressure conditions, respectively (Figures 4B,D,F,H right column). For comparison, parallel experiments in millifluidic high-pressure vessels were performed for each pressure at 85°C, as positive controls. T. barophilus growth was checked after one night of growth and reached the plateau of 2.108 cells.ml−1 for each high-pressure experiment (data not shown), thus in good agreement with the results of the microfluidic approach.
Experiments With Methanothermococcus thermolithotrophicus
The high-pressure microfluidic temperature gradient setup was also implemented with the cultivation of a methanogen strain in order to show the cultivation versatility of this setup. As the model strain is a thermophilic and hydrogenotrophic methanogen, it requires the supply of a gas phase (H2/CO2) to grow. The temperature range was chosen as a function of the literature (i.e., 60°C–70°C, Bernhardt et al., 1988) for two pressure conditions, 5 and 10 MPa (i.e., total pressure) with a partial gas pressure (H2/CO2, 80/20% mol/mol) of 2 MPa and 3 MPa, respectively. As this strain was previously described to better grow under elevated pressure conditions (Bernhardt et al., 1988), these partial pressures were chosen in order to screen rapidly the effects of dissolved gases (H2 and CO2) at 10 different temperatures on M. thermolithotrophicus growth (i.e., cell yield in stationary phase). To do so, a quadruplicate counting was performed for these 10 temperature conditions, both at the initial time point and after 18 h of growth, for both pressure conditions considered in this study (5 and 10 MPa; Figure 5; Supplementary Figure S3A).
Interestingly, the cell yields at 5 MPa (Pi = 2 MPa) reach the optimal cell density observed at atmospheric pressure conditions (about 2 × 108 cells.ml−1) for both the optimum growth temperature and the above ones (i.e., 65°C–70°C; Supplementary Figure S3A). However, for growth temperatures below the optimum (i.e., 60.1°C–63.4°C), the cell yields reach a lower cell density, approximatively two-fold less (about 1.25×108 cells.ml−1) with an intermediate temperature at 64.5°C (about 1.5 × 108 cells.ml−1; Supplementary Figure S3A). When considering the initial cell density into the micropools, M. thermolithotrophicus cells seem to grow better at temperatures between 66.7°C and 68.9°C (Figure 5). The same growth temperature pattern is observed when M. thermolithotrophicus is cultivated at 10 MPa (Pi = 3 MPa). Indeed, the strain displays a higher cell yield when growing at temperatures between 65.6°C and 68.9°C. However, the highest cell density is 2-fold lower than the optimum cell density known for this strain (Figure 5; Supplementary Figure S3A).
Discussion
In this study, we provide proof-of-concept for the operation of a novel high-pressure microfluidic culture approach and demonstrate that it can rapidly screen the effects of temperature on the growth of the piezo-hyperthermophilic model of deep hydrothermal origin T. barophilus strain MPT (Marteinsson et al., 1999) along with a marine thermophile strain Methanothermoccus thermolithotrophicus. Thermococcus barophilus uses molecular and structural adaptation to thrive in its harsh environment, being able to cope with high pressure and fluctuating salinity and temperature conditions (Vannier et al., 2015; Cario et al., 2015a,b, 2016). This piezophilic model is easy to manipulate (Thiel et al., 2014) and is a versatile microorganism with unique metabolic attributes for adaptation to deep-sea vent conditions (Le Guellec et al., 2021). In addition, this archaeal species exhibits several other deep-sea vent clones with other metabolic properties (Kozhevnikova et al., 2016; Oger et al., 2016) expanding the catabolic capabilities of this species. Therefore, this strain was a prime model for this study. Meanwhile, M. thermolithotrophicus is a model methanogenic strain, already cultivated and studied under elevated pressure conditions (Bernhardt et al., 1988; Jaenicke et al., 1988; Miller et al., 1988).
Microorganisms in the deep biosphere flourish under extreme environmental conditions and only a small fraction of them have been isolated to date. Working on isolates remains a technical challenge but the best opportunity to understand the microbial mechanisms enabling life at depth and the limits of life on Earth. Studying growth behaviors under such extreme conditions provides insight into the molecular and cellular processes developed by piezophilic strains for microbial adaptation to high pressure. Recreating the in situ pressure conditions require specific culture approaches and is extremely time-consuming for strain phenotyping. Classical microbiology approaches have overcome some of these time issues by using spectrophotometry and other automated counting methods (e.g. cell sorting and FACS) to estimate microbial growth and phenotype (Zuleta et al., 2014; García-Timermans et al., 2020). However, characterizing cells within a culture without disturbing conditions (i.e., microorganisms live imaging; Bourges et al., 2020) while mimicking in situ pressures (from the deep biosphere) remains a challenge whenever high-pressure vessels are not equipped with a sapphire or optic window for cell characterization.
In this study, the high-pressure microfluidic screening approach yielded 40 growth curves at different pressure and temperature conditions in a record time.
For T. barophilus, the final growth rates are comparable with the literature at ambient pressure and temperatures between 75°C and 90°C (Marteinsson et al., 1999) and at 85°C and for pressure conditions below 20 MPa (i.e., 0.35–0.45 h−1; Vannier et al., 2015). Marteinsson and colleagues reported that T. barophilus was an obligate piezophile for temperature above 95°C. Our study confirmed that T. barophilus shifts its tolerance to higher temperatures when growing under higher-pressure conditions (Figures 4, 6), and showed that it is still able to grow at atmospheric pressure and 95°C (well-defined environment with a precise temperature measurement, Figure 1; Supplementary Information 1). At atmospheric pressure, growth slowed as did cell density, which was the lowest at this extreme temperature. It is well-known in the literature that elevated pressures significantly increase the metabolism of deep-sea strains while growing at elevated temperatures (Miller et al., 1988; Takai et al., 2008). In addition, the high-pressure shifts the upper temperature range of hyperthermophilic strains (Miller et al., 1988; Pledger et al., 1994; Canganella et al., 1997; Marteinsson et al., 1999), which was also observed in this study (Figure 6). The culture approach is important for studying the upper temperature limit of life in hyperthermophilic microorganisms, and even more so when they are piezophilic, as high-pressure is an essential metabolic driving force for these strains isolated from the deep-sea (Takai et al., 2008; Oliver et al., 2020). In this study, combining several conditions of high temperature and pressure (without decompression) in a single experiment provided a rapid mean for characterizing the temperature range of a model strain of deep hydrothermal origin. Furthermore, this work demonstrates that high-pressure microfluidics is a robust and reproducible technique to examine microbial growth at different pressure conditions. Indeed, T. barophilus displayed the same temperature profile when growing in different micropools volume (1.5 and 1.9 nl; Figure 6).
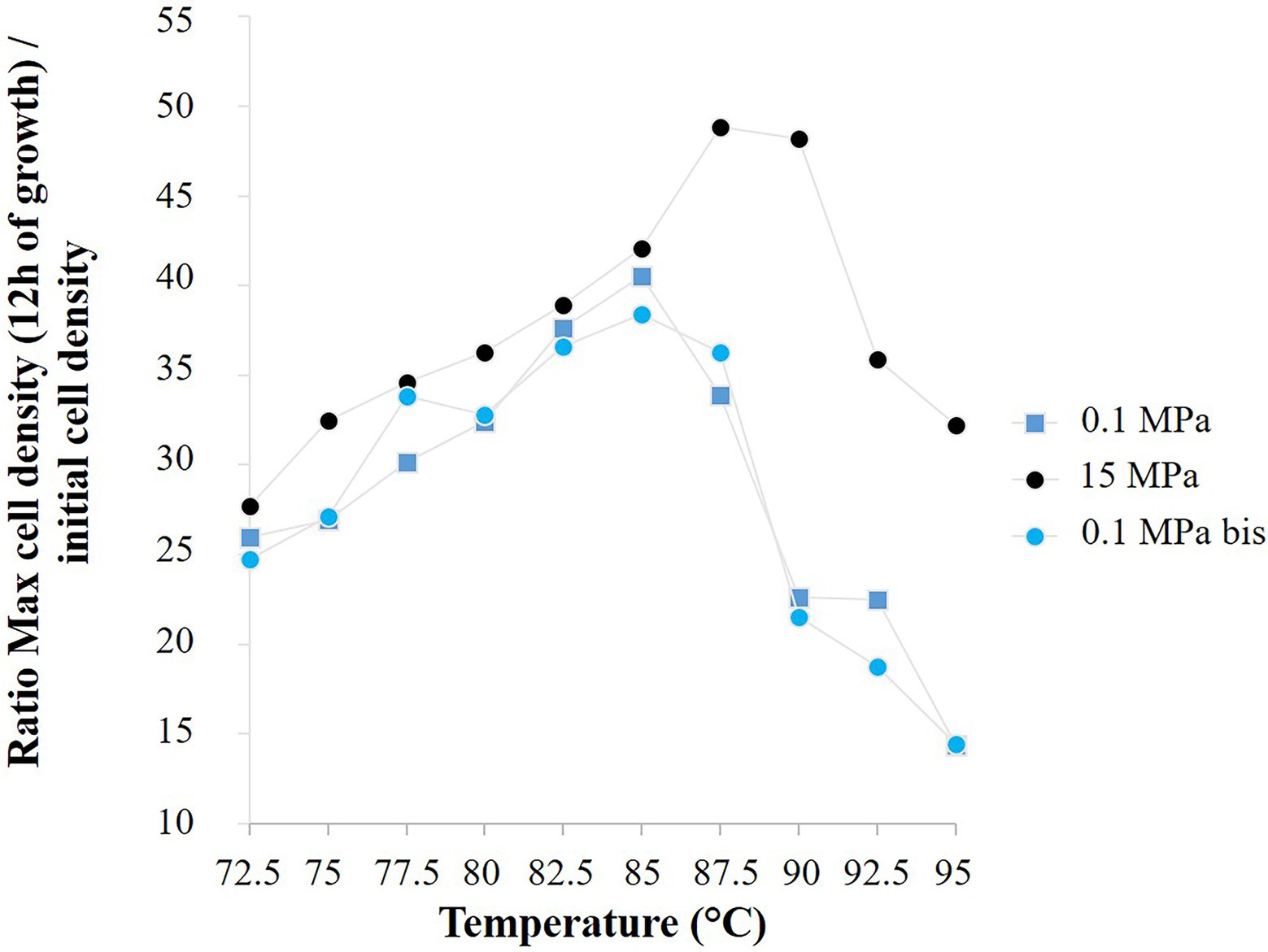
Figure 6. Ratio of the mean cell density of Thermococcus barophilus after 12 h of growth to the corresponding mean initial cell density, for three temperature gradient microfluidic experiments (72.5°C–95°C): atmospheric pressure (0.1 MPa) in a microchip of 1.5 nl pool volume, both 15 MPa and atmospheric pressure (0.1 MPa bis) in a microchip of 1.9 nl pool volume.
Concerning M. thermolithotrophicus, this microfluidic setup allowed us to cultivate the methanogen strain under elevated pressure conditions (i.e., 5 and 10 MPa) with two different partial pressure conditions of H2/CO2 (i.e., 2 and 3 MPa, respectively). M. thermolithotrophicus cells were easily detected in the micropools using reflective mode (Visible), and can also be monitored thanks to their putative autofluorescence (Supplementary Figure S3). The growth of this strain is known to be enhanced under elevated pressure conditions (i.e., 50 MPa, see Bernhardt et al., 1988). Thus, we screened the effects of partial gas pressure (H2/CO2) on the cell yields, at two pressure conditions and over 10 temperature conditions. Interestingly, this strain seems to have a better growth under lower partial pressure of H2/CO2 over the range of temperature conditions tested (60°C–70°C). This study needs further investigations (e.g., gas solubility calculations according to the thermodynamic parameters) as well as to be confirmed with growth rate experiments in order to evaluate the toxicity of H2 and/or CO2 at higher partial pressures for this strain (Dupraz et al., 2013).
Beyond this first demonstration, this setup is adaptable and evolutive and will allow in the short term to cover a wider range of temperature and pressure conditions (0°C–150°C at up to 70 MPa with the new sapphire reactor technology; Marre et al., 2021) to better appreciate the growth (limits and tolerance) of a strain under extreme culture conditions. An interesting outcome is the implementation of various cultivation strategies in order to capture a wide variety of microorganisms with different metabolic requirements: (i) anaerobes and heterotrophs using a single-phase medium; (ii) autotrophs using a diphasic gas–liquid interface; and (iii) aerobes using a diphasic liquid–liquid interface where the immiscible oil serves as an oxygen supplier (Tanet et al., 2019; Figure 1). This microfluidic setup could provide new opportunities to screen known piezophiles and see how high pressure without decompression could extend the upper temperature range, e.g., for T. barophilus and other strict piezophiles such as Pyrococcus yayanosii CH1 (Zeng et al., 2009). In addition, high-pressure microfluidics could also be used as a fast-screening tool to characterize new isolates from the deep biosphere and to study the effect of pressure on growth in the vast majority of previously isolated strains in which this parameter has never been studied. Indeed, as an example, of the 129 bacterial species and 55 archaeal species that have been isolated from deep-sea hydrothermal vents to date and are recognized by the International Committee on Systematics of Prokaryotes (ICSP), only 23 have been examined for the effect of hydrostatic pressure on growth (Jebbar et al., 2015; Zeng et al., 2021).
Compared to conventionally studied batch microbial growth (i.e., large volume cultures at the ml or liter scale), microfluidics handles small volumes for batch culture (i.e., pl to μl) and such a volume variation could lead to a substantial change in microbial behavior when considering different situations such as surface area to volume ratio and nutritional aspect. The low cultivability scores in the laboratory of strains from natural environments have multiple explanations largely reviewed in the literature (e.g., Alain and Querellou, 2009; Lewis et al., 2021). Growth defects may be due to intrinsic environmental differences related, among others, to both accessible nutrients and the confined space available for growth. This variation in volume, known as the “bottle effect” is mainly reported in the literature for natural communities in aquatic environments (Hammes et al., 2010). Depending on the strain and its site of isolation, the effect of containment may be species-dependent and the size of the compartmentalization may trigger or constrain a microbial strain-specific phenotype. The model strain studied here, i.e. T. barophilus, comes from a deep hydrothermal vent, which is an environment with significant local chemical and physical gradients at the millimeter to the centimeter scale, changing in time and space, and containing a multitude of microniches for the growth of microorganisms (Dick, 2019). Microfluidics enables the handling of local gradients on a very small scale. It therefore appears to be a relevant cultivation approach to understand cell behavior (i.e., the microbial response to the local environment) or even cell interaction on a small scale. Such a confined environment for microbial cultivation does not account for larger scale processes (e.g., microbial dispersion and colonization) and depending on the type of study, multi-scale controls are needed to avoid biases induced by confined cultures. Here, T. barophilus and M. thermolithotrophicus cells were able to grow in a confined environment (mono- or diphasic) over several cell generations (about 2 log) in a similar fashion to dynamic growth in a “large” batch liquid culture (i.e., ml cultures in a closed vial). Thus, high-pressure microfluidics is a complementary approach to study microbial behavior under in situ pressure conditions.
Despite the reproducibility of this cultivation approach, such a confined environment presents a local nutrient gradient and motile microorganisms could use of their swimming abilities to seek out favorable available resources and/or conditions (Stocker and Seymour, 2012). Thermococcus barophilus is a motile microorganism but low-pressure conditions abolish its swimming capabilities (Vannier et al., 2015). Therefore, this approach on-a-chip is suitable at pressure conditions below 20 MPa, as T. barophilus cells are non-motile at these low pressure conditions and will not migrate from one pool to another. Concerning the application of this methodology to motile microorganisms, future developments will consider the use of non-miscible inert fluid (such as Fluorinert©) injected in the main microchannel to “seal” the micropools during the experiment, therefore preventing cell migration (see Figure 1 and the concerning protocol in the method section). Nevertheless, one can also think of taking advantage of connections between pore-like structures (e.g., porous media) on-a-chip with temperature and/or chemical gradients to get insights into cell motility towards better living conditions, which can be investigating in real time and under pressure with such approach. In summary, microspatial structures matter for phenotyping studies, thus it is important to design the appropriate microreactor in order to take into account variable parameters (e.g., cell migration, nutrient availability, diffusion coefficient) to ensure both the reproducibility and the closest representativeness of microbial habitats.
Conclusion and Perspectives
This study highlighted the potentialities of high-pressure microfluidics for microbial strain phenotyping and validated high-pressure microfluidics as a valuable complementary tool for temperature range screening of extremophilic microorganisms, under pressure. It demonstrated that microfluidics can be used in order to accelerate microbial phenotyping of microorganisms (here piezo-hyper/thermophilic models) and provided a proof-of-concept for the operation, efficiency and reliability of microfluidics to screen several parameters at a time (e.g., temperature, pressure, and also nutrients gradient) and get the overall and combined phenotype of a single strain in a record time. This set up also demonstrated its versatility with the ability to target various metabolic requirements, reflecting the wide variety of the microbial metabolisms in the deep biosphere.
In this study, one pressure condition experiment (in quadruplicate) took only a few days (pre-culture plus cultivation test) to perform 10 temperature conditions whereas the conventional high-pressure microbiology approach would have taken weeks or months to get these data. High-pressure microfluidic technology can acquire large amounts of data in a short time, resulting in a huge amount of data to process (i.e., image capture, image analysis, and data processing). Our future goal is to implement automation of cell image capture and cell counting using artificial intelligence (machine learning in particular). Indeed, machine learning could help speed up counting but also increase accuracy and reduce errors (Qu et al., 2019). Such a methodology coupled to high-pressure microfluidics, would promote in-depth investigations (e.g., morphologies, catabolisms, and effects of chemicals) of various microorganisms from the deep-biosphere and greatly increase our knowledge of this remote biosphere.
Microfluidics provides an excellent opportunity to better document microbial phenotypes in a short time. This technology is widely applicable to other extremophiles (e.g., halophiles, psychrophiles) and could be adapted to diverse and more challenging culture conditions in order to explore the vast extremophilic microbial realm on Earth. The development of resilient microfabrication materials for extreme microfluidics (e.g., sapphire microreactors – (Marre et al., 2021)) is worth considering and could afford unique features to capture the large as-yet uncultured microbial fraction.
Data Availability Statement
The original contributions presented in the study are included in the article/Supplementary Material, and further inquiries can be directed to the corresponding authors.
Author Contributions
AC and SM designed this research project, made the microreactor design, analyzed data, and wrote the original draft. AC, SM, and ON developed the set-up. ON developed the temperature gradient device. AC and ML performed the experiments and collected data. AC, ON, and KA edited and wrote sections of the manuscript. All authors contributed to revisions of the manuscript, tables, and figures and approved the submitted version.
Funding
Funding for this work was provided by the European Research Council (ERC) under the European Union’s Horizon 2020 research and innovation program (grant agreement no. 725100, project Big Mac).
Conflict of Interest
The authors declare that the research was conducted in the absence of any commercial or financial relationships that could be construed as a potential conflict of interest.
Publisher’s Note
All claims expressed in this article are solely those of the authors and do not necessarily represent those of their affiliated organizations, or those of the publisher, the editors and the reviewers. Any product that may be evaluated in this article, or claim that may be made by its manufacturer, is not guaranteed or endorsed by the publisher.
Acknowledgments
The authors are grateful to Fabien Palencia for the 3D printing of a specific microscopic frame enabling to fit the temperature gradient setup under the microscope and take pictures in the micropools over time.
Supplementary Material
The Supplementary Material for this article can be found online at: https://www.frontiersin.org/articles/10.3389/fmicb.2022.866681/full#supplementary-material
References
Adan, A., Alizada, G., Kiraz, Y., Baran, Y., and Nalbant, A. (2017). Flow cytometry: basic principles and applications. Crit. Rev. Biotechnol. 37, 163–176. doi: 10.3109/07388551.2015.1128876
Alain, K., and Querellou, J. (2009). Cultivating the uncultured: limits, advances and future challenges. Extremophiles 13, 583–594. doi: 10.1007/s00792-009-0261-3
Bao, C., Gai, Y., Lou, K., Jiang, C., and Ye, S. (2010). High-hydrostatic-pressure optical chamber system for cultivation and microscopic observation of deep-sea organisms. Aquat. Biol. 11, 157–162. doi: 10.3354/ab00303
Bar-On, Y. M., Phillips, R., and Milo, R. (2018). The biomass distribution on earth. Proc. Natl. Acad. Sci. U. S. A. 115, 6506–6511. doi: 10.1073/pnas.1711842115
Beaton, A. D., Schaap, A. M., Pascal, R., Hanz, R., Martincic, U., Cardwell, C. L., et al. (2022). Lab-on-chip for in situ analysis of nutrients in the deep sea. ACS sensors 7, 89–98. doi: 10.1021/acssensors.1c01685
Beney, L., Perrier-Cornet, J. M., Hayert, M., and Gervais, P. (1997). Shape modification of phospholipid vesicles induced by high pressure: influence of bilayer compressibility. Biophys. J. 72, 1258–1263. doi: 10.1016/S0006-3495(97)78772-1
Berdy, B., Spoering, A. L., Ling, L. L., and Epstein, S. S. (2017). In situ cultivation of previously uncultivable microorganisms using the ichip. Nat. Protoc. 12, 2232–2242. doi: 10.1038/nprot.2017.074
Bernhardt, G., Distèche, A., Jaenicke, R., Koch, B., Lüdemann, H. D., and Stetter, K. O. (1988). Effect of carbon dioxide and hydrostatic pressure on the pH of culture media and the growth of methanogens at elevated temperature. App. Microbiol. Biotechnol. 28, 176–181.
Boitard, L., Cottinet, D., Bremond, N., Baudry, J., and Bibette, J. (2015). Growing microbes in millifluidic droplets. Eng. Life Sci. 15, 318–326. doi: 10.1002/elsc.201400089
Bourges, A. C., Lazarev, A., Declerck, N., Rogers, K. L., and Royer, C. A. (2020). Quantitative high-resolution imaging of live microbial cells at high hydrostatic pressure. Biophys. J. 118, 2670–2679. doi: 10.1016/j.bpj.2020.04.017
Canganella, F., Gonzalez, J. M., Yanagibayashi, M., Kato, C., and Horikoshi, K. (1997). Pressure and temperature effects on growth and viability of the hyperthermophilic archaeon Thermococcus peptonophilus. Arch. Microbiol. 168, 1–7. doi: 10.1007/s002030050462
Cario, A., Grossi, V., Schaeffer, P., and Oger, P. M. (2015a). Membrane homeoviscous adaptation in the piezo-hyperthermophilic archaeon Thermococcus barophilus. Front. Microbiol. 6:1152. doi: 10.3389/fmicb.2015.01152
Cario, A., Jebbar, M., Thiel, A., Kervarec, N., and Oger, P. M. (2016). Molecular chaperone accumulation as a function of stress evidences adaptation to high hydrostatic pressure in the piezophilic archaeon Thermococcus barophilus. Sci. Rep. 6, 1–8. doi: 10.1038/srep29483
Cario, A., Lormières, F., Xiang, X., and Oger, P. (2015b). High hydrostatic pressure increases amino acid requirements in the piezo-hyperthermophilic archaeon Thermococcus barophilus. Res. Microbiol. 166, 710–716. doi: 10.1016/j.resmic.2015.07.004
Cario, A., Oliver, G. C., and Rogers, K. L. (2019). Exploring the deep marine biosphere: challenges, innovations, and opportunities. Front. Earth Sci. 7:225. doi: 10.3389/feart.2019.00225
Chen, P, Zhou, H, Huang, Y, Xie, Z, Zhang, M, Wei, Y, et al. (2021). Revealing the full biosphere structure and versatile metabolic functions in the deepest ocean sediment of the challenger deep. bioRxiv [Preprint].
Connon, S. A., and Giovannoni, S. J. (2002). High-throughput methods for culturing microorganisms in very-low-nutrient media yield diverse new marine isolates. Appl. Environ. Microbiol. 68, 3878–3885. doi: 10.1128/AEM.68.8.3878-3885.2002
Czernichowski-Lauriol, I., Czop, V., Dupraz, S., Farret, R., Gombert, P., Husson, G., et al. (2017). “CO2 capture, transport and storage research facilities from the French node of ECCSEL available for access by the European scientific community.” in 9th “Trondheim Conference on CO2 Capture, Transport and Storage” (TCCS-9). June 2017 (Trondheim, Norway).
Demello, A. J. (2006). Control and detection of chemical reactions in microfluidic systems. Nature 442, 394–402. doi: 10.1038/nature05062
DeSantis, T. Z., Brodie, E. L., Moberg, J. P., Zubieta, I. X., Piceno, Y. M., and Andersen, G. L. (2007). High-density universal 16S rRNA microarray analysis reveals broader diversity than typical clone library when sampling the environment. Microb. Ecol. 53, 371–383. doi: 10.1007/s00248-006-9134-9
Deusner, C., Meyer, V., and Ferdelman, T. G. (2010). High-pressure systems for gas-phase free continuous incubation of enriched marine microbial communities performing anaerobic oxidation of methane. Biotechnol. Bioeng. 105, 524–533. doi: 10.1002/bit.22553
D'Hondt, S., Jørgensen, B. B., Miller, D. J., Batzke, A., Blake, R., Cragg, B. A., et al. (2004). Distributions of microbial activities in deep subseafloor sediments. Science 306, 2216–2221. doi: 10.1126/science.1101155
Dick, G. J. (2019). The microbiomes of deep-sea hydrothermal vents: distributed globally, shaped locally. Nat. Rev. Microbiol. 17, 271–283. doi: 10.1038/s41579-019-0160-2
Dupraz, S., Fabbri, A., Joulian, C., Dictor, M. C., Battaglia-Brunet, F., Menez, B., et al. (2013). Impact of CO2 concentration on autotrophic metabolisms and carbon fate in saline aquifers–A case study. Geochim. Cosmochim. Acta 119, 61–76. doi: 10.1016/j.gca.2013.05.027
Dusny, C., and Schmid, A. (2015). Microfluidic single-cell analysis links boundary environments and individual microbial phenotypes. Environ. Microbiol. 17, 1839–1856. doi: 10.1111/1462-2920.12667
El-Ali, J., Sorger, P. K., and Jensen, K. F. (2006). Cells on chips. Nature 442, 403–411. doi: 10.1038/nature05063
Fry, J. C., Parkes, R. J., Cragg, B. A., Weightman, A. J., and Webster, G. (2008). Prokaryotic biodiversity and activity in the deep subseafloor biosphere. FEMS Microbiol. Ecol. 66, 181–196. doi: 10.1111/j.1574-6941.2008.00566.x
García-Timermans, C., Rubbens, P., Heyse, J., Kerckhof, F. M., Props, R., Skirtach, A. G., et al. (2020). Discriminating bacterial phenotypes at the population and single-cell level: a comparison of flow cytometry and Raman spectroscopy fingerprinting. Cytometry A 97, 713–726. doi: 10.1002/cyto.a.23952
Garel, M., Bonin, P., Martini, S., Guasco, S., Roumagnac, M., Bhairy, N., et al. (2019). Pressure-retaining sampler and high-pressure systems to study deep-sea microbes under in situ conditions. Front. Microbiol. 10:453. doi: 10.3389/fmicb.2019.00453
Gavoille, T., Pannacci, N., Bergeot, G., Marliere, C., and Marre, S. (2019). Microfluidic approaches for accessing thermophysical properties of fluid systems. React. Chem. Eng. 4, 1721–1739. doi: 10.1039/C9RE00130A
Geissmann, Q. (2013). OpenCFU, a new free and open-source software to count cell colonies and other circular objects. PLoS One 8:e54072. doi: 10.1371/journal.pone.0054072
Gervais, T., and Jensen, K. F. (2006). Mass transport and surface reactions in microfluidic systems. Chem. Eng. Sci. 61, 1102–1121. doi: 10.1016/j.ces.2005.06.024
Girault, M., Beneyton, T., Del Amo, Y., and Baret, J.-C. (2019). Microfluidic technology for plankton research. Curr. Opin. Biotechnol. 55, 134–150. doi: 10.1016/j.copbio.2018.09.010
Grünberger, A., Probst, C., Helfrich, S., Nanda, A., Stute, B., Wiechert, W., et al. (2015). Spatiotemporal microbial single-cell analysis using a high-throughput microfluidics cultivation platform. Cytometry A 87, 1101–1115. doi: 10.1002/cyto.a.22779
Hammes, F., Vital, M., and Egli, T. (2010). Critical evaluation of the volumetric “bottle effect” on microbial batch growth. Appl. Environ. Microbiol. 76, 1278–1281. doi: 10.1128/AEM.01914-09
Hu, B., Xu, B., Yun, J., Wang, J., Xie, B., Li, C., et al. (2020). High-throughput single-cell cultivation reveals the underexplored rare biosphere in deep-sea sediments along the Southwest Indian Ridge. Lab Chip 20, 363–372. doi: 10.1039/C9LC00761J
Huber, H., Thomm, M., König, H., Thies, G., and Stetter, K. O. (1982). Methanococcus thermolithotrophicus, a novel thermophilic lithotrophic methanogen. Arch. Microbiol. 132, 47–50. doi: 10.1007/BF00690816
Ikeda, S., Satake, H., Hisano, T., and Terazawa, T. (1972). Potentiometric argentimetric method for the successive titration of sulphide and dissolved sulphur in polysulphide solutions. Talanta 19, 1650–1654. doi: 10.1016/0039-9140(72)80240-6
Jaenicke, R., Bernhardt, G., Lüdemann, H. D., and Stetter, K. O. (1988). Pressure-induced alterations in the protein pattern of the thermophilic archaebacterium Methanococcus thermolithotrophicus. Appl. Environ. Microbiol. 54, 2375–2380. doi: 10.1128/aem.54.10.2375-2380.1988
Jannasch, H. W., and Taylor, C. D. (1984). Deep-sea microbiology. Annu. Rev. Microbiol. 38:487. doi: 10.1146/annurev.mi.38.100184.002415
Jannasch, H. W., Wirsen, C. O., and Doherty, K. W. (1996). A pressurized chemostat for the study of marine barophilic and oligotrophic bacteria. Appl. Environ. Microbiol. 62, 1593–1596. doi: 10.1128/aem.62.5.1593-1596.1996
Jebbar, M., Franzetti, B., Girard, E., and Oger, P. (2015). Microbial diversity and adaptation to high hydrostatic pressure in deep-sea hydrothermal vents prokaryotes. Extremophiles 19, 721–740. doi: 10.1007/s00792-015-0760-3
Jensen, K. F., Ajmera, S. K., Firebaugh, S. L., Floyd, T. M., Franz, A. J., Losey, M. W., et al. (2000). Microfabricated Chemical Systems for Product Screening and Synthesis. Automated Synthetic Methods for Speciality Chemicals. ed. W. Hoyle (London: Royal Society of Chemistry), 14–24.
Jiang, C.-Y., Dong, L., Zhao, J.-K., Hu, X., Shen, C., Qiao, Y., et al. (2016). High-throughput single-cell cultivation on microfluidic streak plates. Appl. Environ. Microbiol. 82, 2210–2218. doi: 10.1128/AEM.03588-15
Jørgensen, B. B., and Boetius, A. (2007). Feast and famine—microbial life in the deep-sea bed. Nat. Rev. Microbiol. 5, 770–781. doi: 10.1038/nrmicro1745
Kallmeyer, J., Pockalny, R., Adhikari, R. R., Smith, D. C., and D’Hondt, S. (2012). Global distribution of microbial abundance and biomass in subseafloor sediment. Proc. Natl. Acad. Sci. 109, 16213–16216. doi: 10.1073/pnas.1203849109
Kaminski, T. S., Scheler, O., and Garstecki, P. (2016). Droplet microfluidics for microbiology: techniques, applications and challenges. Lab Chip 16, 2168–2187. doi: 10.1039/C6LC00367B
Kato, C. (2011). Cultivation Methods for Piezophiles. Extremophiles Handbook Tokyo: Springer, 719–726.
Kato, M., and Fujisawa, T. (1998). High-pressure solution X-ray scattering of protein using a hydrostatic cell with diamond windows. J. Synchrotron Radiat. 5, 1282–1286. doi: 10.1107/S0909049598000788
Kato, C., Inoue, A., and Horikoshi, K. (1996). Isolating and characterizing deep-sea marine microorganisms. Trends Biotechnol. 14, 6–12. doi: 10.1016/0167-7799(96)80907-3
Kozhevnikova, D., Taranov, E., Lebedinsky, A., Bonch-Osmolovskaya, E., and Sokolova, T. (2016). Hydrogenogenic and sulfidogenic growth of Thermococcus archaea on carbon monoxide and formate. Microbiology 85, 400–410. doi: 10.1134/S0026261716040135
Le Guellec, S., Leroy, E., Courtine, D., Godfroy, A., and Roussel, E. G. (2021). H2-dependent formate production by hyperthermophilic Thermococcales: an alternative to sulfur reduction for reducing-equivalents disposal. ISME J. 15, 3423–3436. doi: 10.1038/s41396-021-01020-x
Lewis, W. H., Tahon, G., Geesink, P., Sousa, D. Z., and Ettema, T. J. (2021). Innovations to culturing the uncultured microbial majority. Nat. Rev. Microbiol. 19, 225–240. doi: 10.1038/s41579-020-00458-8
Lloyd, K. G., Steen, A. D., Ladau, J., Yin, J., and Crosby, L. (2018). Phylogenetically novel uncultured microbial cells dominate earth microbiomes. mSystems 3, e00055–e00018. doi: 10.1128/mSystems.00055-18
Maldonado, J. A., Schaffner, D. W., Cuitiño, A. M., and Karwe, M. V. (2016). In situ studies of microbial inactivation during high pressure processing. High Pressure Res. 36, 79–89. doi: 10.1080/08957959.2015.1111887
Marre, S., Adamo, A., Basak, S., Aymonier, C., and Jensen, K. F. (2010). Design and packaging of microreactors for high pressure and high temperature applications. Ind. Eng. Chem. Res. 49, 11310–11320. doi: 10.1021/ie101346u
Marre, S., Lecoutre, C., Garrabos, Y., Fauveau, C., Cario, A., and Nguyen, O. (2021). Sapphire microreactors. International Patent No WO 2021/2051 15 A1. WIPO, PCT
Marteinsson, V. T., Birrien, J. L., Reysenbach, A. L., Vernet, M., Marie, D., Gambacorta, A., et al. (1999). Thermococcus barophilus sp. nov., a new barophilic and hyperthermophilic archaeon isolated under high hydrostatic pressure from a deep-sea hydrothermal vent. Int. J. Syst. Bacteriol. 49, 351–359.
Miller, J. F., Shah, N. N., Nelson, C. M., Ludlow, J. M., and Clark, D. S. (1988). Pressure and temperature effects on growth and methane production of the extreme thermophile Methanococcus jannaschii. Appl. Environ. Microbiol. 54, 3039–3042. doi: 10.1128/aem.54.12.3039-3042.1988
Moffitt, J. R., Lee, J. B., and Cluzel, P. (2012). The single-cell chemostat: an agarose-based, microfluidic device for high-throughput, single-cell studies of bacteria and bacterial communities. Lab Chip 12, 1487–1494. doi: 10.1039/c2lc00009a
Molina-Gutierrez, A., Stippl, V., Delgado, A., Gänzle, M. G., and Vogel, R. F. (2002). In situ determination of the intracellular pH of Lactococcus lactis and Lactobacillus plantarum during pressure treatment. Appl. Environ. Microbiol. 68, 4399–4406. doi: 10.1128/AEM.68.9.4399-4406.2002
Morais, S., Cario, A., Liu, N., Bernard, D., Lecoutre, C., Garrabos, Y., et al. (2020). Studying key processes related to CO 2 underground storage at the pore scale using high pressure micromodels. React. Chem. Eng. 5, 1156–1185. doi: 10.1039/D0RE00023J
Morais, S., Liu, N., Diouf, A., Bernard, D., Lecoutre, C., Garrabos, Y., et al. (2016). Monitoring CO 2 invasion processes at the pore scale using geological labs on chip. Lab Chip 16, 3493–3502. doi: 10.1039/C6LC00830E
Murphy, E. R., Inoue, T., Sahoo, H. R., Zaborenko, N., and Jensen, K. F. (2007). Solder-based chip-to-tube and chip-to-chip packaging for microfluidic devices. Lab Chip 7, 1309–1314. doi: 10.1039/b704804a
Nauhaus, K., Boetius, A., Krüger, M., and Widdel, F. (2002). In vitro demonstration of anaerobic oxidation of methane coupled to sulphate reduction in sediment from a marine gas hydrate area. Environ. Microbiol. 4, 296–305. doi: 10.1046/j.1462-2920.2002.00299.x
Nichols, D., Cahoon, N., Trakhtenberg, E., Pham, L., Mehta, A., Belanger, A., et al. (2010). Use of ichip for high-throughput in situ cultivation of “uncultivable” microbial species. Appl. Environ. Microbiol. 76, 2445–2450. doi: 10.1128/AEM.01754-09
Oger, P. M., Daniel, I., and Picard, A. (2006). Development of a low-pressure diamond anvil cell and analytical tools to monitor microbial activities in situ under controlled P and T. Biochim Biophys Acta 1764, 434–442. doi: 10.1016/j.bbapap.2005.11.009
Oger, P., Sokolova, T. G., Kozhevnikova, D. A., Taranov, E. A., Vannier, P., Lee, H. S., et al. (2016). Complete genome sequence of the hyperthermophilic and piezophilic archaeon Thermococcus barophilus Ch5, capable of growth at the expense of hydrogenogenesis from carbon monoxide and formate. Genome Announc. 4, e01534–e01515. doi: 10.1128/genomeA.01534-15
Oliver, G. C., Cario, A., and Rogers, K. L. (2020). Rate and extent of growth of a model extremophile, Archaeoglobus fulgidus, under high hydrostatic pressures. Front. Microbiol. 11:1023. doi: 10.3389/fmicb.2020.01023
Oliver, G. C., Cario, A., and Rogers, K. L. (2021). High temperature and high hydrostatic pressure cultivation, transfer, and filtration Systems for Investigating Deep Marine Microorganisms. Preprints 2021, 2021040453. doi: 10.20944/preprints202104.0453.v1
Orcutt, B. N., LaRowe, D. E., Biddle, J. F., Colwell, F. S., Glazer, B. T., Reese, B. K., et al. (2013). Microbial activity in the marine deep biosphere: progress and prospects. Front. Microbiol. 4:189. doi: 10.3389/fmicb.2013.00189
Peoples, L. M., Norenberg, M., Price, D., McGoldrick, M., Novotny, M., Bochdansky, A., et al. (2019). A full-ocean-depth rated modular lander and pressure-retaining sampler capable of collecting hadal-endemic microbes under in situ conditions. Deep-Sea Res. I Oceanogr. Res. Pap. 143, 50–57. doi: 10.1016/j.dsr.2018.11.010
Peters, J., Martinez, N., Michoud, G., Cario, A., Franzetti, B., Oger, P., et al. (2014). Deep sea microbes probed by incoherent neutron scattering under high hydrostatic pressure. Z. Phys. Chem. 228, 1121–1133. doi: 10.1515/zpch-2014-0547
Picard, A., and Daniel, I. (2013). Pressure as an environmental parameter for microbial life—a review. Biophys. Chem. 183, 30–41. doi: 10.1016/j.bpc.2013.06.019
Picard, A., Daniel, I., Montagnac, G., and Oger, P. (2007). In situ monitoring by quantitative Raman spectroscopy of alcoholic fermentation by Saccharomyces cerevisiae under high pressure. Extremophiles 11, 445–452. doi: 10.1007/s00792-006-0054-x
Pledger, R. J., Crump, B. C., and Baross, J. A. (1994). A barophilic response by two hyperthermophilic, hydrothermal vent Archaea: an upward shift in the optimal temperature and acceleration of growth rate at supra-optimal temperatures by elevated pressure. FEMS Microbiol. Ecol. 14, 233–241. doi: 10.1111/j.1574-6941.1994.tb00109.x
Pucetaite, M., Ohlsson, P., Persson, P., and Hammer, E. (2021). Shining new light into soil systems: spectroscopy in microfluidic soil chips reveals microbial biogeochemistry. Soil Biol. Biochem. 153:108078. doi: 10.1016/j.soilbio.2020.108078
Qu, K., Guo, F., Liu, X., Lin, Y., and Zou, Q. (2019). Application of machine learning in microbiology. Front. Microbiol. 10:827. doi: 10.3389/fmicb.2019.00827
Raber, E. C., Dudley, J. A., Salerno, M., and Urayama, P. (2006). Capillary-based, high-pressure chamber for fluorescence microscopy imaging. Rev. Sci. Instrum. 77:096106. doi: 10.1063/1.2349303
Ramirez-Llodra, E, Brandt, A, Danovaro, R, De Mol, B, Escobar, E, German, CR, et al. (2010). Deep, diverse and definitely different: unique attributes of the world’s largest ecosystem. Biogeosciences 7, 2851–2899. doi: 10.5194/bg-7-2851-2010
Rinke, C., Schwientek, P., Sczyrba, A., Ivanova, N. N., Anderson, I. J., Cheng, J.-F., et al. (2013). Insights into the phylogeny and coding potential of microbial dark matter. Nature 499, 431–437. doi: 10.1038/nature12352
Sackmann, E. K., Fulton, A. L., and Beebe, D. J. (2014). The present and future role of microfluidics in biomedical research. Nature 507, 181–189. doi: 10.1038/nature13118
Stocker, R., and Seymour, J. R. (2012). Ecology and physics of bacterial chemotaxis in the ocean. Microbiol. Mol. Biol. Rev. 76, 792–812. doi: 10.1128/MMBR.00029-12
Takai, K., Nakamura, K., Toki, T., Tsunogai, U., Miyazaki, M., Miyazaki, J., et al. (2008). Cell proliferation at 122°C and isotopically heavy CH4 production by a hyperthermophilic methanogen under high-pressure cultivation. Proc. Natl. Acad. Sci. 105, 10949–10954. doi: 10.1073/pnas.0712334105
Tanet, L., Tamburini, C., Baumas, C., Garel, M., Simon, G., and Casalot, L. (2019). Bacterial bioluminescence: light emission in Photobacterium phosphoreum is not under quorum-sensing control. Front. Microbiol. 10:365. doi: 10.3389/fmicb.2019.00365
Terekhov, S. S., Smirnov, I. V., Malakhova, M. V., Samoilov, A. E., Manolov, A. I., Nazarov, A. S., et al. (2018). Ultrahigh-throughput functional profiling of microbiota communities. Proc. Natl. Acad. Sci. 115, 9551–9556. doi: 10.1073/pnas.1811250115
Thiel, A., Michoud, G., Moalic, Y., Flament, D., and Jebbar, M. (2014). Genetic manipulations of the hyperthermophilic piezophilic archaeon Thermococcus barophilus. Appl. Environ. Microbiol. 80, 2299–2306. doi: 10.1128/AEM.00084-14
Tiggelaar, R. M., Benito-López, F., Hermes, D. C., Rathgen, H., Egberink, R. J., Mugele, F. G., et al. (2007). Fabrication, mechanical testing and application of high-pressure glass microreactor chips. Chem. Eng. J. 131, 163–170. doi: 10.1016/j.cej.2006.12.036
Vannier, P., Michoud, G., Oger, P., þór Marteinsson, V., and Jebbar, M. (2015). Genome expression of Thermococcus barophilus and Thermococcus kodakarensis in response to different hydrostatic pressure conditions. Res. Microbiol. 166, 717–725. doi: 10.1016/j.resmic.2015.07.006
Wang, F., Zhou, H., Meng, J., Peng, X., Jiang, L., Sun, P., et al. (2009). GeoChip-based analysis of metabolic diversity of microbial communities at the Juan de Fuca Ridge hydrothermal vent. Proc. Natl. Acad. Sci. 106, 4840–4845. doi: 10.1073/pnas.0810418106
Whitman, W. B., Coleman, D. C., and Wiebe, W. J. (1998). Prokaryotes: The unseen majority. Proc. Natl. Acad. Sci. 95, 6578–6583. doi: 10.1073/pnas.95.12.6578
Xu, Y., Riordon, J., Cheng, X., Bao, B., and Sinton, D. (2017). The full pressure–temperature phase envelope of a mixture in 1000 microfluidic chambers. Angew. Chem. 129, 14150–14155. doi: 10.1002/ange.201708238
Yayanos, A. A. (1986). Evolutional and ecological implications of the properties of deep-sea barophilic bacteria. Proc. Natl. Acad. Sci. 83, 9542–9546. doi: 10.1073/pnas.83.24.9542
Zeng, X., Alain, K., and Shao, Z. (2021). Microorganisms from deep-sea hydrothermal vents. Marine Life Sci. Technol. 3, 204–230.
Zeng, S., Birrien, J., Fouquet, Y., Cherkashov, G., Jebbar, M., Querellou, J., et al. (2009). Pyrococcus CH1, an obligate piezophilic hyperthermophile: extending the upper pressure-temperature limits for life. ISME J. 3, 873–876. doi: 10.1038/ismej.2009.21
Zengler, K., Walcher, M., Clark, G., Haller, I., Toledo, G., Holland, T., et al. (2005). High-throughput cultivation of microorganisms using microcapsules. Methods Enzymol. 397, 124–130. doi: 10.1016/S0076-6879(05)97007-9
Keywords: high-pressure microfluidics, deep-sea microorganisms, real time investigations, phenotyping, fast screening
Citation: Cario A, Larzillière M, Nguyen O, Alain K and Marre S (2022) High-Pressure Microfluidics for Ultra-Fast Microbial Phenotyping. Front. Microbiol. 13:866681. doi: 10.3389/fmicb.2022.866681
Edited by:
Andreas Teske, University of North Carolina at Chapel Hill, United StatesReviewed by:
Philippe M. Oger, UMR5240 Microbiologie, Adaptation et Pathogenie (MAP), FranceKrishnendu Chakrabarty, Government College of Engineering and Ceramic Technology, India
Copyright © 2022 Cario, Larzillière, Nguyen, Alain and Marre. This is an open-access article distributed under the terms of the Creative Commons Attribution License (CC BY). The use, distribution or reproduction in other forums is permitted, provided the original author(s) and the copyright owner(s) are credited and that the original publication in this journal is cited, in accordance with accepted academic practice. No use, distribution or reproduction is permitted which does not comply with these terms.
*Correspondence: Anaïs Cario, YW5haXMuY2FyaW9AY25ycy5mcg==; Samuel Marre, c2FtdWVsLm1hcnJlQGNucnMuZnI=