- 1College of Biological and Pharmaceutical Engineering, West Anhui University, Lu’an, China
- 2School of Life Science, Jiaying University, Meizhou, China
Unhealthy diet, in particular high-fat diet (HFD) intake, can cause the development of several metabolic disorders, including obesity, hyperlipidemia, type 2 diabetes mellitus (T2DM), non-alcoholic fatty liver disease (NAFLD), and metabolic syndrome (MetS). These popular metabolic diseases reduce the quality of life, and induce premature death worldwide. Evidence is accumulating that the gut microbiota is inextricably associated with HFD-induced metabolic disorders, and dietary intervention of gut microbiota is an effective therapeutic strategy for these metabolic dysfunctions. Polysaccharides are polymeric carbohydrate macromolecules and sources of fermentable dietary fiber that exhibit biological activities in the prevention and treatment of HFD-induced metabolic diseases. Of note, natural polysaccharides are among the most potent modulators of the gut microbiota composition. However, the prebiotics-like effects of polysaccharides in treating HFD-induced metabolic diseases remain elusive. In this review, we introduce the critical role of gut microbiota human health and HFD-induced metabolic disorders. Importantly, we review current knowledge about the role of natural polysaccharides in improving HFD-induced metabolic diseases by regulating gut microbiota.
Introduction
Diet can affect multiple aspects of human health, and high-fat diet (HFD) contributes to the epidemics of obesity and obesity-associated chronic metabolic diseases, such as diabetes, hyperlipidemia, hypertension, non-alcoholic fatty liver disease (NAFLD), and metabolic syndrome (MetS; Li et al., 2016). These metabolic diseases remain a serious global burden that kill at least 2.5 million people annually (Li et al., 2016). Interestingly, metabolic diseases patients are highly associated with increased risk of developing the severe coronavirus disease 2019 (COVID-19) (Le Roux, 2021; Steenblock et al., 2021). In addition, over-consumption of high-fat directly promotes the tumorigenesis, such as prostate cancer, colorectal cancer (CRC), and hepatocellular carcinoma (Beyaz et al., 2016; Labbe et al., 2019; Yang et al., 2022).
Gut microbiota is considered as an invisible “forgotten organ” of human beings that is composed of trillions of microorganisms (Ursell et al., 2012). As a real organ, it is host-specific, and affects almost all aspects of host physiology, which can be effectively modified by diet, or surgery (Marchesi et al., 2016). Due to the development of next-generation sequencing technologies, the genomic blueprint of human gut microbiota is visualized, and our understanding the functions of intestinal microbiota in human health becomes more accurate (Almeida et al., 2019). The gut microbiota plays important role in nearly all aspects of human health and disease. For example, alterations of intestinal microbiota are highly correlated with levels of SARS-CoV-2, and severity of patients with COVID-19 (Zuo et al., 2020). In addition, maternal gut microbiota also influences the metabolic phenotype and social behavior of offspring (Buffington et al., 2016; Kimura et al., 2020; Laursen et al., 2021). Notably, in a phase 3, double-blind and randomized trial, oral administration of gut microbiome-derived drug (SER-109) reduces the risk of recurrent infection of Clostridioides difficile infection (Feuerstadt et al., 2022).
The intestinal microbiota composition is strongly associated with human diseases, especially chronic metabolic disorders, such as obesity and type 2 diabetes mellitus (T2DM; Gentile and Weir, 2018). Gut microbiota dysbiosis, including reduced diversity, stability, and increased adverse bacteria, is frequently observed in patients with metabolic diseases, and gut microbiota-targeting intervention is an effective treatment for these diseases (Tilg and Moschen, 2014; Liu et al., 2021b). Alterations of intestinal microbiota are highly associated with tumorigenesis, especially CRC, and the intestinal microbiota have the potential as CRC biomarkers (Wong and Yu, 2019). The phenotypes of intestinal microbiota are distinct stage-specific in CRC patients, and the metabolites of the entire gut microbiota substantially influence the progression of CRC (Yachida et al., 2019). Accordingly, administration of probiotic, Lactobacillus gallinarum that is depleted in the intestine of CRC patients, protects against CRC tumorigenesis through producing protective metabolites (Sugimura et al., 2021). In addition, the gut microbiota affects human immune system, and the link between gut microbiota and the immune system is based on peripheral white blood cell (WBC) dynamics (Schluter et al., 2020). In cancer immunotherapy, the intestinal microbiota influences the toxicity of combined immune checkpoint blockade treatment (Andrews et al., 2021). Accordingly, diet intervention can impact human immune status by modulating “diet-microbiota-immune” axis, and high fermented food diet, for example, increases gut microbiome diversity and reduces inflammation (Wastyk et al., 2021). Moreover, the gut microbiota substantially affects the nervous system disorders through the “gut-brain” axis (Aaldijk and Vermeiren, 2022). For example, germ-free mice exhibit autism spectrum disorder (ASD)-like behaviors when harbored with intestinal microbiota from ASD patients, and the abnormalities of social behavioral can be improved by the administration of specific metabolites (Sharon et al., 2019).
Natural polysaccharides, one of the most the abundant dietary components, are polymeric carbohydrate macromolecules and sources of fermentable dietary fiber that exhibit biological activities with low toxicity, such as, anti-oxidation, anti-inflammatory, anti-tumor, and antiviral (Chaisuwan et al., 2021; Li et al., 2021a; Mirzadeh et al., 2021). Of note, natural polysaccharides are among the most potent modulators of the gut microbiota composition and benefit for human health (Yin et al., 2020; Song et al., 2021). However, the prebiotics-like activities of polysaccharides in treating HFD-induced metabolic diseases remain elusive. In this review, we introduce the critical role of gut microbiota human health and HFD-induced metabolic disorders. Notably, we focus on the role of natural polysaccharides in treating HFD-induced metabolic diseases by the “polysaccharides-gut microbiota” manner.
High-Fat Diet Induces Gut Microbiota Dysbiosis
Increased HFD substantially alters intestinal microbiota composition and metabolites. Mice fed with HFD for short-term (2 days) exhibit altered gut microbiota composition at the phylum level, and longer-time HFD feeding (12 days) induces the gut microbiota dysbiosis (Ke et al., 2021). Regardless, after 2 or 12 days HFD feeding, the abundance of beneficial bacteria, Bacteroidetes is reduced, while the abundance of adverse bacteria, Firmicutes is substantially increased (Ke et al., 2021). The Firmicutes/Bacteroidetes ratio is increased in HFD-fed animals and humans and is proposed as a hallmark of obesity (Magne et al., 2020). In addition, the abundances of Firmicutes genus, including Blautia, Bilophila, Enterorhabdus, Erysipelatoclostridium, Lactobacillus, and Oscillibacter, is increased by HFD feeding, and these bacteria positively correlates with chronic metabolic diseases, such as obesity (Jo et al., 2021). Moreover, in zebrafish model, HFD feeding for 6 h increases intestinal microbiota abundance by 20 folds, and causes adverse effects in the bacterial communities (Ye et al., 2019). Importantly, germ-free mice prevent HFD-induced the insulin resistance, and increased cholesterol biosynthesis, suggesting that gut microbiota is involved in HFD-induced metabolic abnormalities (Rabot et al., 2010). In a randomized controlled trial, healthy young adults with high-fat consumption show adverse changes in gut microbiota, including increased Alistipes and Bacteroides, and decreased Faecalibacterium (Wan et al., 2019). In addition, HFD alters the functional integrity of the ileum, and one mechanism by which HFD induces gut microbes dysbiosis by modifying peroxisome proliferator-activated receptor-γ (PPAR-γ) pathway (Tomas et al., 2016). Of note, HFD-induced gut microbiome alterations are strongly associated with metabolic diseases (Kang et al., 2022). Currently, intervention of gut microbiota by prebiotics can prevent or ameliorate HFD-induced metabolic diseases (Kang et al., 2022). Of note, characterization of intestinal microbiota profiles prior to dietary intervention by prebiotics will increase the positive outcome in patients with metabolic disorders (Rodriguez et al., 2020). Thus, over-consumption of high-fat induces gut microbiota dysbiosis, and manipulation of gut microbiota can reverse HFD-induced metabolic diseases.
Supplementation of Natural Polysaccharides Ameliorate HFD-Induced Metabolic Disorders by Modulating Gut Microbiota
Carbohydrates and polysaccharides are important composition of our daily diet that are not directly digested by the gastrointestinal enzymes (Shang et al., 2018). Carbohydrate fermentation is a critical function of the human gut microbiota, and then the fermented carbohydrates produce important metabolites in gut, such as short-chain fatty acids (SCFAs), and succinate (Canfora et al., 2019). Mechanistically, the gut microbiome harbors distinct enzymatic systems, including a glycoside hydrolase family of β-galactosidases and a carbohydrate-binding module family, to degrade plant-derived polysaccharides (Cabral et al., 2022). These processes are essential to maintain gut microbiota that depends mostly on non-digestively polysaccharides and fibers as energy sources (Sonnenburg and Sonnenburg, 2014). In turn, notably, polysaccharides are primary modulators of the function and composition of gut microbiota (Sonnenburg and Backhed, 2016; Yang et al., 2021a). Importantly, accumulating evidence demonstrates that supplementation of natural polysaccharides is an effective gut-microbiota-targeted treatment for HFD-induced metabolic disorders (Wang et al., 2018a; Su et al., 2021).
HFD-Induced Obesity
The prevalence of obesity in children and adults, continues to increase and has tripled since 1975, and the rate of obese or overweight adults will account for 57.8% of global population by 2030 (Kelly et al., 2008; Loos and Yeo, 2021). Obesity is a serious public health concern that can induce premature death, and it is associated with increased risk of development of metabolic diseases, including diabetes, hypertension, hyperlipidemia, cardiovascular disease, and NAFLD. Obesity is a typical diet-related illness, and excess food uptake, especially HFD, is the most important master in the development of obesity (Kahn et al., 2006; Miyamoto et al., 2019). Increasing evidences demonstrate that HFD-induced obesity can cause adverse changes in intestinal microbial composition (Dalby et al., 2017; Miyamoto et al., 2019). Notably, obese individuals show increased the adverse bacteria, Firmicutes, Fusobacteria, Proteobacteria, and Lactobacillus, and decreased the beneficial bacteria, Bacteroidetes, Verrucomicrobia, Faecalibacterium, and Lactobacillus plantarum (Crovesy et al., 2020). One of the direct evidences for the role of gut microbiota in obesity is that, germ-free mice protects against obesity, despite the high-calories food intake (Bäckhed et al., 2004; Torres-Fuentes et al., 2017). In addition, germ-free mice colonized by intestinal microbiota from obese donors, display more increase of total body fat than lean donors (Turnbaugh et al., 2006). Mechanistically, gut bacteria and their metabolites regulate obesity through the “microbiota-gut-brain” axis (Torres-Fuentes et al., 2017; Cryan et al., 2019). Since intestinal microbiota dysbiosis can cause diet-related obesity, dietary intervention of gut microbiota is a therapeutic strategy of obesity (Zhang et al., 2015).
Natural polysaccharides from herbs and foods are important players in regulating gut microbiota to prevent or treat obesity (Lyu et al., 2017). Natural polysaccharides extracted from the Artemisia sphaerocephala Krasch seeds, alleviate HFD-induced obesity in mice by preventing HFD-induced gut microbiota dysbiosis, notably by reducing Proteobacteria, AF12, and Helicobacter (Li et al., 2020b). Dictyophora indusiata polysaccharides (DIP) shows anti-obesity effect in HFD-fed mice by reversing HFD-induced gut microbiota dysbiosis, notably by decreasing the ratio of bacteria, Firmicutes/Bacteroidetes (Kanwal et al., 2020). Microalgae polysaccharides isolated from Chlorella pyrenoidosa, Spirulina platensis, ameliorate obesity in HFD-fed mice by increasing the beneficial gut bacteria, including Clostridia, Bacterioidia, and Mollicutes, and reducing the unfavorable bacteria, Actinobacteria and Verrucomicrobia (Guo et al., 2021a). Modified apple polysaccharides (MAP) suppress HFD-induced obesity in mice by restoring HFD-induced gut microbiota disorder, notably by enriching the beneficial bacteria, Bacteroidetes, Bacteroides, and Lactobacillus, and inhibiting the adverse bacteria, Fusobacterium (Li et al., 2020d). Natural polysaccharides derived from Raphanus sativus display anti-obesity property in HDF-fed mice, and by reversing HFD-induced intestinal microbial dysbiosis via reducing the ratio of Firmicutes/Bacteroidetes, and increasing Verrucomicrobia (Do et al., 2021). Natural polysaccharides derived from WuGuChong ameliorate HDF-induced obesity in mice through decreasing the ratio of gut microbiota, Firmicutes/Bacteroidetes and the abundance of Proteobacteria (Wang et al., 2020). Polysaccharides derived from Hirsutella sinensis mycelium show anti-obesogenic effect in HFD-fed mice by modulating the gut microbiota composition, especially by enriching the gut bacteria, Parabacteroides goldsteinii. Importantly, oral administration of P. goldsteinii reduces obesity in HFD-fed mice (Wu et al., 2019b). Polysaccharides obtained from Momordica charantia, ameliorate HFD-induced obesity in mice by increasing the beneficial bacteria, such as Actinobacteria, Coprococcus, and Lactobacillus, and reducing the harmful bacteria, Proteobacteria and Helicobacter (Wen et al., 2021). Natural polysaccharides extracted from the food Laminaria japonica, alleviate HFD-induced obesity in mice by normalizing intestinal microbiota, notably by increasing the abundances of Bacteroidales and Rikenellaceae (Duan et al., 2019).
Intestinal microbial metabolites, particularly SCFAs, play crucial roles in the communication between gut microbes and the host (van de Wouw et al., 2018). Acetate, propionate, and butyrate are the most abundant SCFAs (>95%) in human body, and they are rapidly absorbed by the colonocytes (Dalile et al., 2019; He et al., 2020). The beneficial effects of SCFAs in obesity have been demonstrated, and SCFAs can protect against HFD-induced obesity (Den Besten et al., 2015). Notably, natural polysaccharides can promote intestinal SCFAs productions to ameliorate HFD-induced obesity. Alginate is the most abundant natural polysaccharides in brown seaweed that improves HFD-induced obesity in mice by increasing the SCFAs production, and gut bacteria, Bacteroidales, and reducing Clostridiales (Zheng et al., 2021). Mushroom polysaccharides isolated from Pleurotus eryngii display anti-obesity in HFD-fed mice through increasing the SCFAs-producing gut bacteria, Anaerostipes and Clostridium (Nakahara et al., 2020). Dietary Enteromorpha clathrata polysaccharides (ECP) attenuates obesity in HFD-fed mice by increasing the abundance of SCFAs, in particular, butyrate-producing gut bacterium, Eubacterium xylanophilum (Wei et al., 2021). Lycium barbarum polysaccharides (LBP) prevent obesity in HFD-fed mice, increase SCFAs, butyric acid and SCFAs-producing gut bacteria, Lacticigenium, Lachnospiraceae, and Butyricicoccus (Yang et al., 2021b). Polygonatum odoratum Polysaccharides attenuate HFD-induced obesity in rats by increasing the SCFAs, isobutyric acid, butyric acid, valeric acid, and reducing the adverse bacteria, Actinobacteria, Proteobacteria, and Sutterella abundance in intestine (Wang et al., 2018b). Ganoderma lucidum polysaccharides (GLP) inhibit HFD-fed obese mice by reversing HFD-induced gut microbiota disorder, and increasing SCFAs, acetate, and butyrate (Sang et al., 2021). Sulfated polysaccharides from Stichopus japonicus, prevents HFD-induced obesity in mice through enriching the probiotic Akkermansia and reducing the endotoxin-bearing Proteobacteria, and improving the SCFAs contents (Zhu et al., 2018). Mulberry leaf polysaccharides ameliorate HFD-induced obesity in mice by increasing the content of SCFAs, and reducing the Firmicutes/Bacteroidetes ratio (Li et al., 2021c). Taken together, natural polysaccharides exert prebiotics-like activities in preventing HFD-induced obesity by modulating the gut microbiota composition and metabolites.
HFD-Induced Hyperlipidemia
Hyperlipidemia is an obesity-related metabolic disease characterized by high levels of total cholesterol, or triglyceride, or low-density lipoprotein (LDL) cholesterol. Hyperlipidemia is strongly associated with diet, and HFD accelerates de novo lipogenesis and increases the biogenesis of LDL (Yuan et al., 2019). Hyperlipidemia is an important risk factor for atherosclerotic cardiovascular diseases, metabolic disorders, and stroke (Soh et al., 2013). Recent studies show that gut microbiota is highly associated with hyperlipidemia and its related diseases, and gut microbiota can regulate lipid-metabolism homeostasis to develop hyperlipidemia (Jia et al., 2021). As such, manipulation of gut microbiota is an effective therapeutic option for hyperlipidemia (Kim et al., 2019). Currently, statins are major hypolipidemic drug, but with increased glioma risk (Cote et al., 2019). Natural polysaccharides can regulate gut microbiota to prevent or ameliorate HFD-induced hyperlipidemia.
Natural polysaccharides isolated from Cordyceps militaris, alleviate hyperglycemia in high-fat/sucrose diet-fed mice by improving gut microbiota dysbiosis, and promoting the abundance of probiotics, Akkermansia muciniphila (Lee et al., 2021). Natural polysaccharides isolated from seafood Ostrea rivularis, attenuate hyperlipidemia in HFD-fed zebrafish by improving gut microbiota imbalance, notably by enriching the beneficial gut bacteria, Acidobacteria, Bacteroidetes, and Verrucomicrobia, and reducing the harmful bacteria, Proteobacteria and Cohaesibacter (Kong et al., 2021). Selenium-rich polysaccharides extracted from Cordyceps militaris prevent HFD-induced hyperlipidemia in mice by reducing obesity-correlated gut bacteria, including Dorea, Clostridium, Lactobacillus, and Ruminococcus, and increasing the beneficial gut bacteria, Akkermansia (Yu et al., 2021). Quinoa polysaccharides derived from Inca food Chenopodium quinoa, ameliorate HFD-induced hyperlipidemia in rats by reducing Firmicutes/Bacteroides ratio, and the abundance of Proteobacteria, Desulfovibrio, and Allobaculum (Cao et al., 2020). Natural polysaccharides extracted from mushroom Grifola frondose, alleviate HFD-induced hyperlipidemia in rats by reversing HFD-induced intestinal flora dysbiosis, notably by increasing the abundance of Helicobater, Barnesiella, Parasutterella, and Flavonifracter, and decreasing the harmful bacteria, Butyricicoccus and Turicibacter (Li et al., 2019a). Oral administration of natural polysaccharides obtained from Holothuria leucospilota ameliorate hyperlipidemia in HFD-fed rats by increasing the SCFAs-producing gut microbiota (Yuan et al., 2019). Overall, natural polysaccharides can display probiotics-like activity in improving HFD-induced hyperlipidemia.
HFD/Streptozotocin-Induced Type 2 Diabetes Mellitus
Diabetes mellitus is extremely common metabolic disease that affects the health of 460 million people in the world, and the number of diabetic patients will account for 9.9% of the global population by 2045 (Merino et al., 2019; Siehler et al., 2021). It seriously reduces quality of life, with many poor outcomes, including recurrent, longer hospital stays, and higher mortality rate (Nazzal et al., 2021). Approximately 90% of diabetic patients have T2DM, while T1DM represents 10% of all diabetes cases (Siehler et al., 2021). T2DM is an obesity-related disease, and increased dietary monounsaturated fat is linked with a higher risk of T2DM (Merino et al., 2019; Lingvay et al., 2021). Thus, combination of HFD and streptozotocin (STZ) is the most frequently used to establish alternative animal model of T2DM (Srinivasan et al., 2005). Currently, increasing evidence demonstrates that the gut microbiota can contribute to T2DM, and microbiota composition is altered in diabetic patients (Adeshirlarijaney and Gewirtz, 2020; Fan and Pedersen, 2021). In addition, the human microbiome contributes to the drug resistance of antidiabetic drug, acarbose (Balaich et al., 2021). Thus, targeting of gut microbiota is a defensive strategy against T2DM.
Natural polysaccharides can display prebiotics-like activities to improve T2DM by regulating the gut microbiota composition. Cyclocarya paliurus polysaccharides (CPP) alleviate T2DM symptoms in HFD/STZ-fed mice by increasing SCFAs contents, and promoting the SCFAs-producing gut species, such as Ruminococcus and Anaerotruncus (Yao et al., 2020). Natural polysaccharides derived from Nigella sativa seed, show antidiabetic effect in HFD/STZ-induced T2DM mice by increasing the abundance of intestinal microbiota Bacteroides and Muribaculaceae (Dong et al., 2020). Grifola frondosa polysaccharides (GFP) exhibit hypoglycemic and hypolipidemic effects in HFD/STZ-induced T2DM mouse model by reversing HFD/STZ-induced gut microbial dysbiosis, notably increasing Alistipes, and reducing Streptococcus, Staphylococcus, and Enterococcus (Guo et al., 2020). Tea polysaccharides extracted from Camellia sinensis L. possess hypolipidemic and hypoglycemic effect in HFD/STZ-induced T2DM rats by restoring the abundance of gut microbiota, such as Lachnospira, and Victivallis, and increasing the SCFAs contents (Li et al., 2020a). Polysaccharides isolated from Cyclocarya paliurus leaves attenuate diabetic symptoms in HFD/STZ-induced T2DM rats by increasing the SCFAs contents and the beneficial gut bacteria Ruminococcaceae (Li et al., 2021b). Natural polysaccharides from Momordica charantia ameliorate hyperglycemia, hyperlipidemia, and hyperinsulinemia in HFD/STZ-induced T2DM rats through increasing the SCFAs contents and the abundance of Prevotella loescheii and Lactococcus laudensis (Gao et al., 2018). Natural pumpkin polysaccharides extracted from popular vegetable Cucurbita moschata, show hypoglycemic effect in HFD/STZ-induced T2DM by increasing the biomarker, Akkermansia, and reducing Erysipelotrichaceae (Wu et al., 2021). In addition, pumpkin polysaccharides also increase the gut production of SCFAs in T2DM model (Liu et al., 2018). Ganoderma lucidum polysaccharides (GLP) display antidiabetic effects by restoring HFD/STZ-induced intestinal microbiota dysbiosis, notably by increasing Blautia, Bacteroides, Dehalobacterium, and Parabacteroides, and reducing the harmful gut bacteria, Aerococcus, Corynebactrium, Ruminococcus, and Proteus (Chen et al., 2020b). Natural polysaccharides from Coix seed, exhibit hypoglycemic activity in HFD/STZ-induced T2DM mouse model by reducing the Firmicutes/Bacteroidetes ratio, and increasing the contents of SCFAs (Xia et al., 2021). Glucomannans, as natural polysaccharides from Dendrobium officinale, Aloe vera, and konjac, ameliorates metabolic disorder of T2DM in HFD/STZ-fed rats through increasing the abundance of Firmicutes, and decreasing the abundance of Bacteroidetes, Proteobacteria (Chen et al., 2021a). Taken together, natural polysaccharides can prevent or treat T2DM by regulating gut microbiota composition and metabolites.
HFD-Induced Non-Alcoholic Fatty Liver Disease
Non-alcoholic fatty liver disease remains a highly prevalent and largely underappreciated chronic liver disease that strongly affects about one-quarter of the global adults, and NAFLD is one of the most important risk factors of hepatocellular carcinoma (Foerster et al., 2021; Lazarus et al., 2021). The majority of patients suffering from NAFLD are obese, and NAFLD is considered as a further manifestation of metabolic syndrome (Tarantino and Finelli, 2015). Although the contributors of NAFLD are extremely complicated, emerging data demonstrates that HFD is a primary driver in the development of NAFLD (Jensen et al., 2018; Gao et al., 2020). Importantly, HFD induces adverse changes of intestinal microbiota composition in healthy young adults (Wan et al., 2019), and gut microbiota dysbiosis is highly associated with NAFLD (Leung et al., 2016). In this regard, HFD-induced gut microbiota disorder promotes the development of NAFLD by mediating the “gut-liver” axis (Le Roy et al., 2013). The communication between the gut and liver is through the biliary tract, portal vein, and systemic circulation (Tripathi et al., 2018). Currently, there is no approved drugs for the treatment of NAFLD, and several anti-NAFLD drugs are being undergoing various phases of recent clinical trials (Negi et al., 2022). However, natural polysaccharides extracted from plants exhibit great potential in alleviating NAFLD through manipulation of gut microbiota.
Gut microbe-derived microbial metabolites play pivotal roles in the development and progression of NAFLD, and these microbial metabolites include SCFAs, bile acids (BAs), choline metabolite trimethylamine (TMA), lithocholic acid (LCA), and succinate (Chu et al., 2019). SCFAs are the most abundant microbial metabolites that are generated from microbe fermentation of indigestible carbohydrates in gut (Dai et al., 2020). SCFAs are not only providers of nutrients and energy for intestinal epithelium, but also serve as signaling molecules to regulate intestinal lipogenesis and gluconeogenesis (Koh et al., 2016; Dai et al., 2020). Importantly, numerous human studies suggest that SCAFs are reduced in patients with NAFLD (Wang et al., 2016), and SCAFs supplementation is a therapeutic target for the prevention and treatment of HFD-associated NAFLD (Li et al., 2020c; Zhang et al., 2021a). Notably, natural polysaccharides play a major role in regulating gut microbiota to produce SCAFs (Liu et al., 2021a).
Astragalus polysaccharides (APS) are extracted from Astragalus mongholicus that attenuates NAFLD in HFD-fed mice, and mechanistically, APS enriches Desulfovibrio genus, especially Desulfovibrio vulgaris that is a generator of, SCFAs, acetic acid, and attenuates hepatic steatosis (Hong et al., 2021). Noni fruit polysaccharides are derived from Morinda citrifolia L. that alleviates NAFLD in HFD-fed mice through promoting SCFAs production, and reversing HFD-induced intestinal dysbiosis by improving gut microbiota diversity and composition (Yang et al., 2020b). Walnut green husk polysaccharides prevent obesity and NAFLD in HFD-fed rats by enhancing the SCFAs content and abundance of intestinal microbiota, including Prevotellaceae, Allobaculum (Wang et al., 2021). Lycium barbarum polysaccharides (LBP) are extracted from traditional Chinese herd and functional food, Lycium barbarum that combines with aerobic exercise to ameliorate NAFLD in HFD-fed rats by augmenting SCFAs contents and gut microbiota, Bacteroidetes (Gao et al., 2021b). In a randomized controlled trial, the potential prebiotics-like effect of LBP supplementation in treating NAFLD is being evaluated (Gao et al., 2021a). Natural polysaccharides, MDG-1, extracted from the roots of Ophiopogon japonicus, inhibits the progression of NAFLD in HFD-fed mice through restoring the gut microbiota balance by improving the abundance of SCFAs-producing the beneficial bacteria, Butyricimonas and Roseburia (Wang et al., 2019). Mussel polysaccharides, α-D-glucan (MPA) extracted from Mytilus coruscus protects NAFLD in HFD-fed rats, and mechanistically, MPA supplementation reverses HFD-inhibited microbial dysbiosis and SCFAs (Wu et al., 2019a). Soluble polysaccharides from Laminaria japonica attenuates NAFLD in HFD-fed mice by reducing the ratio of Firmicutes/Bacteroidetes, and promoting Verrucomicrobia and propionate-producing bacteria Bacteroides and Akkermansia (Zhang et al., 2021b).
Interestingly, the natural polysaccharides are not always safe for the treatment of NAFLD. For example, exopolysaccharides (EPS) derived from bacteria, Lactobacillus rhamnosus GG (LGG EPS) and L. casei BL23 (BL23 EPS), both ameliorate NAFLD and increase the acetate and propionate (SCFAs) in HFD-fed zebrafish, but BL23 EPS, not LGG EPS, induces liver inflammation and injury by intestinal microbiota dysbiosis (Zhang et al., 2019). In addition, Cordyceps sinensis polysaccharides (CSPs) exhibit obvious liver toxicity via aggravating liver steatosis and fibrosis in HFD-fed mice, and mechanistically, CSPs are digested by Actinobacteria and then excess Actinobacteria induces intestinal flora disorder and contributes to steatohepatitis (Chen et al., 2020a). Thus, using natural polysaccharides to treat NAFLD has a potential liver injury risk, and it is critical to select safe polysaccharides for treating NAFLD. Taken together, HFD induces gut microbiota dysbiosis that contributes to NAFLD, and intervention with natural polysaccharides can target the gut-liver axis, especially SCFAs, to alleviate or treat NAFLD. However, these findings on the efficacy of natural polysaccharides are consequence of studies in animal models, which is impossible completely mirror the human NAFLD. Thus, more clinical trials should be performed to further evaluate the efficacy of natural polysaccharides in NAFLD.
Sirtuins (SIRTs, SIRT1-7), ubiquitous deacetylases, are crucial metabolic regulators, and importantly, SIRTs are also emerging as the essential cause of NAFLD (Nassir and Ibdah, 2016; Palomer et al., 2021). SIRTs play master roles in a range of physiological functions, including fatty acid oxidation, mitochondrial oxidative metabolism, hepatic fat metabolism, and insulin secretion in hepatocytes (Nasrin et al., 2010; Laurent et al., 2013; Gupta et al., 2022). In NAFLD patients, SIRT1, SIRT2, SIRT3, SIRT5, and SIRT6 are dramatically down-regulated in livers (Wu et al., 2014; Ren et al., 2021), and plasma levels of SIRTs is highly associated with NAFLD (Mariani et al., 2015). In addition, low circulating levels of SIRT4 in obese NAFLD patients reduce oxidative capacity by reducing the mitochondrial ROS production in the liver, and in muscle (Tarantino et al., 2014). Therefore, SIRTs are the potential molecular targets for the treatment of NAFLD, as well as other metabolic diseases (Li et al., 2017). Notably, SIRTs can interact with the gut microbiota, and are linked with altered gut microbiota in NAFLD. For example, SIRT3 deficiency promotes HFD-induced NAFLD by impairing intestinal permeability through induction of the gut microbial dysbiosis (Chen et al., 2019). Thus, loss of SIRT3 interacts with the gut microbiota in the NAFLD progression. More importantly, natural polysaccharides regulate the activities of SIRTs, such as SIRT1 and SIRT3, in human diseases (Dimitrova-Shumkovska et al., 2020; Zhao et al., 2021b). However, the direct evidence that natural polysaccharides protect HFD-induced NAFLD through regulation of SIRTs-mediated gut microbiota is lacking. Therefore, SIRTs are novel research topics by which polysaccharides improve HFD-induced NAFLD by regulating gut microbiota.
Metabolic Syndrome
Metabolic syndrome remains the most common non-communicable disease that is characterized by central obesity, insulin resistance, hyperlipidaemia, and hypertension (Bishehsari et al., 2020). MetS increases the risk of developing several chronic diseases, including T2DM, NAFLD, cardiovascular diseases, and cancer (Chen et al., 2021b; Kao and Huang, 2021). The contributors of MetS include genetic factors, and lifestyle, such as dietary over-consumption of HFD (Wutthi-In et al., 2020). Increasing studies show that the gut plays an important role in MetS, and the gut-centric theory in MetS emerged since 2007 (Fandriks, 2017; Dabke et al., 2019). Strong evidence for the important role of gut in MetS is the potent efficacy of weight-loss in gastrointestinal surgery (Hughes, 2014). Currently, intestinal microbiota is highly associated with MetS, and the gut microbiota dysbiosis results in obesity, and consequently MetS (Croci et al., 2021). Therefore, intervention of gut microbiota composition is an effective option for treating MetS patients (Wutthi-In et al., 2020; Guo et al., 2021b).
Natural polysaccharides have been demonstrated to alleviate or prevent MetS by regulating gut microbiota. Fucoidan, as sulfated polysaccharides from Pearsonothuria graeffei, alleviates MetS in HFD-fed mice by increasing abundances of Actinobacteria and Bacteroidetes, and decreasing the adverse bacteria, Firmicutes and Proteobacteria (Li et al., 2018). Flaxseed polysaccharides from Linum usitatissimum, prevent MetS in HFD-fed mice by increasing SCFAs contents and the beneficial bacteria, Akkermansia, Bifidobacterium, and decreasing the obesity-associated intestinal bacteria, Oscillospira and Odoribacteraceae (Yang et al., 2020a). Sulfated polysaccharides extracted from pacific abalone, improve MetS in HFD-fed mice by reversing HFD-reduced contents of SCFAs, and reducing ratio of Firmicutes/Bacteroidetes (Wu et al., 2020). Sulfated polysaccharides from Isostichopus Badionotus, prevent MetS in high fat and sucrose diet-fed mice by reducing the ratio of Firmicutes/Bacteroidetes, and the abundances of Allobaculum, Lachnospiraceae, and increasing abundances of Barnesiella, Bacteroides, and Porphyromonadaceae (Li et al., 2019b). Fuzhuan brick tea (FBT) polysaccharides extracted from Camellia sinensis, attenuate MetS in HFD-fed mice by restoring HFD-induced gut microbiota disorder, notably by reducing the harmful intestinal bacteria, Coriobacteriaceae, Erysipelotrichaceae, and Streptococcaceae (Chen et al., 2018). Natural polysaccharides extracted from Flammulina velutipes, attenuate MetS-related obesity, hyperlipidemia, and insulin resistance in HFD-fed mice by restoring HDF-induced gut microbiota dysbiosis and improving intestinal function (Zhao et al., 2021a). Sulfated polysaccharides from seafood Undaria pinnatifida, improve MetS in HFD-fed mice by reversing HFD-induced intestinal microbiota disorder, notably by increasing the beneficial bacteria, Bacteroidetes, and reducing the adverse bacteria, Firmicutes (Jiang et al., 2021). Therefore, supplementation of natural polysaccharides restores HFD-induced microbiota dysbiosis, and thereby improves MetS.
Conclusion and Perspectives
The composition of gut microbiota is highly linked with human health, and unhealthy lifestyles, in particular HFD, can induce chronic metabolic diseases by regulating gut microbiota. The plasticity of intestinal microbiota makes microbiome-targeted dietary intervention an important treatment for diseases. Natural polysaccharides are important components of human foods that are critical bidirectional regulators of gut microbiota. Gut microbiome ferments indigestible polysaccharides, and the fermented metabolites, in turn, affect intestinal microbiota composition and metabolites. Importantly, natural polysaccharides display probiotics-like activities in improving HFD-induced metabolic diseases (Figure 1; Table 1).
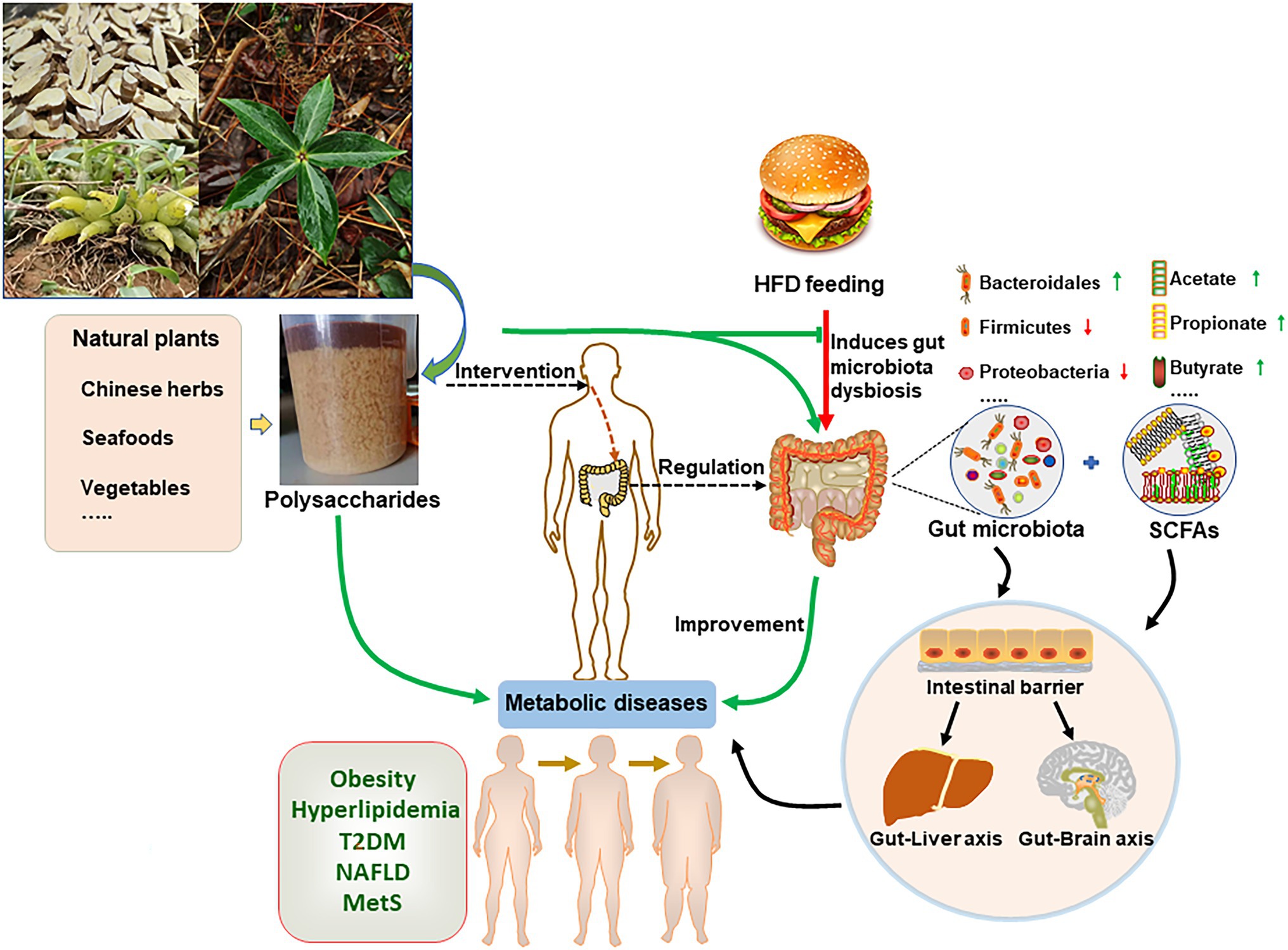
Figure 1. Natural polysaccharides improve high-fat diet (HFD)-induced metabolic diseases through regulating gut microbiota. Plants or animals-derived polysaccharides are fermented by gut microorganism, and produce metabolites, such as short-chain fatty acids (SCFAs). In turn, these metabolites regulate the intestinal microbiota composition. HFD induce gut microbiota dysbiosis that contributes to obesity-associated chronic metabolic diseases. In this regard, natural polysaccharides reverse HFD-induced metabolic diseases by regulating gut microbiota.
Nevertheless, a few limitations and challenges exist to use natural polysaccharides for the treatment of high-fat-associated metabolic diseases through gut microbiota. First, a comprehensive understanding of the interaction between gut microbiota and natural polysaccharides needs further studies. Second, because most of researches are in animal, it is critical to perform the clinical trials that examine the polysaccharides-microbiome-diseases interaction, and realization of clinical translation. Third, the adverse effects of polysaccharides, including hepatotoxicity, should be evaluated prior to clinical use.
Author Contributions
C-YS and Z-LZ drafted the manuscript. C-YS and B-WL revised the manuscript. C-WC and DL approved the final manuscript.
Funding
This study is supported by the grants from the Major Science and Technology Project of Anhui Province (Nos: 202003c08020004 and 202103b06020004).
Conflict of Interest
The authors declare that the research was conducted in the absence of any commercial or financial relationships that could be construed as a potential conflict of interest.
Publisher’s Note
All claims expressed in this article are solely those of the authors and do not necessarily represent those of their affiliated organizations, or those of the publisher, the editors and the reviewers. Any product that may be evaluated in this article, or claim that may be made by its manufacturer, is not guaranteed or endorsed by the publisher.
References
Aaldijk, E., and Vermeiren, Y. (2022). The role of serotonin within the microbiota-gut-brain axis in the development of Alzheimer’s disease: a narrative review. Ageing Res. Rev. 75:101556. doi: 10.1016/j.arr.2021.101556
Adeshirlarijaney, A., and Gewirtz, A. T. (2020). Considering gut microbiota in treatment of type 2 diabetes mellitus. Gut Microbes 11, 253–264. doi: 10.1080/19490976.2020.1717719
Almeida, A., Mitchell, A. L., Boland, M., Forster, S. C., Gloor, G. B., Tarkowska, A., et al. (2019). A new genomic blueprint of the human gut microbiota. Nature 568, 499–504. doi: 10.1038/s41586-019-0965-1
Andrews, M. C., Duong, C. P. M., Gopalakrishnan, V., Iebba, V., Chen, W. S., Derosa, L., et al. (2021). Gut microbiota signatures are associated with toxicity to combined CTLA-4 and PD-1 blockade. Nat. Med. 27, 1432–1441. doi: 10.1038/s41591-021-01406-6
Bäckhed, F., Ding, H., Wang, T., Hooper, L. V., Koh, G. Y., Nagy, A., et al. (2004). The gut microbiota as an environmental factor that regulates fat storage. Proc. Natl. Acad. Sci. U. S. A. 101, 15718–15723. doi: 10.1073/pnas.0407076101
Balaich, J., Estrella, M., Wu, G., Jeffrey, P. D., Biswas, A., Zhao, L., et al. (2021). The human microbiome encodes resistance to the antidiabetic drug acarbose. Nature 600, 110–115. doi: 10.1038/s41586-021-04091-0
Beyaz, S., Mana, M. D., Roper, J., Kedrin, D., Saadatpour, A., Hong, S. J., et al. (2016). High-fat diet enhances stemness and tumorigenicity of intestinal progenitors. Nature 531, 53–58. doi: 10.1038/nature17173
Bishehsari, F., Voigt, R. M., and Keshavarzian, A. (2020). Circadian rhythms and the gut microbiota: from the metabolic syndrome to cancer. Nat. Rev. Endocrinol. 16, 731–739. doi: 10.1038/s41574-020-00427-4
Buffington, S. A., Di Prisco, G. V., Auchtung, T. A., Ajami, N. J., Petrosino, J. F., and Costa-Mattioli, M. (2016). Microbial reconstitution reverses maternal diet-induced social and synaptic deficits in offspring. Cell 165, 1762–1775. doi: 10.1016/j.cell.2016.06.001
Cabral, L., Persinoti, G. F., Paixao, D. A. A., Martins, M. P., Morais, M. A. B., Chinaglia, M., et al. (2022). Gut microbiome of the largest living rodent harbors unprecedented enzymatic systems to degrade plant polysaccharides. Nat. Commun. 13:629. doi: 10.1038/s41467-022-28310-y
Canfora, E. E., Meex, R. C. R., Venema, K., and Blaak, E. E. (2019). Gut microbial metabolites in obesity, NAFLD and T2DM. Nat. Rev. Endocrinol. 15, 261–273. doi: 10.1038/s41574-019-0156-z
Cao, Y., Zou, L., Li, W., Song, Y., Zhao, G., and Hu, Y. (2020). Dietary quinoa (Chenopodium quinoa Willd.) polysaccharides ameliorate high-fat diet-induced hyperlipidemia and modulate gut microbiota. Int. J. Biol. Macromol. 163, 55–65. doi: 10.1016/j.ijbiomac.2020.06.241
Chaisuwan, W., Phimolsiripol, Y., Chaiyaso, T., Techapun, C., Leksawasdi, N., Jantanasakulwong, K., et al. (2021). The antiviral activity of bacterial, fungal, and algal polysaccharides as bioactive ingredients: potential uses for enhancing immune systems and preventing viruses. Front. Nutr. 8:772033. doi: 10.3389/fnut.2021.772033
Chen, M., Hui, S., Lang, H., Zhou, M., Zhang, Y., Kang, C., et al. (2019). SIRT3 deficiency promotes high-fat diet-induced nonalcoholic fatty liver disease in correlation with impaired intestinal permeability through gut microbial dysbiosis. Mol. Nutr. Food Res. 63:e1800612. doi: 10.1002/mnfr.201800612
Chen, H., Nie, Q., Hu, J., Huang, X., Yin, J., and Nie, S. (2021a). Multiomics approach to explore the amelioration mechanisms of glucomannans on the metabolic disorder of type 2 diabetic rats. J. Agric. Food Chem. 69, 2632–2645. doi: 10.1021/acs.jafc.0c07871
Chen, M., Xiao, D., Liu, W., Song, Y., Zou, B., Li, L., et al. (2020b). Intake of Ganoderma lucidum polysaccharides reverses the disturbed gut microbiota and metabolism in type 2 diabetic rats. Int. J. Biol. Macromol. 155, 890–902. doi: 10.1016/j.ijbiomac.2019.11.047
Chen, G., Xie, M., Wan, P., Chen, D., Dai, Z., Ye, H., et al. (2018). Fuzhuan brick tea polysaccharides attenuate metabolic syndrome in high-fat diet induced mice in association with modulation in the gut microbiota. J. Agric. Food Chem. 66, 2783–2795. doi: 10.1021/acs.jafc.8b00296
Chen, L., Zhang, L., Wang, W., Qiu, W., Liu, L., Ning, A., et al. (2020a). Polysaccharides isolated from Cordyceps sinensis contribute to the progression of NASH by modifying the gut microbiota in mice fed a high-fat diet. PLoS One 15:e0232972. doi: 10.1371/journal.pone.0232972
Chen, H., Zheng, X., Zong, X., Li, Z., Li, N., Hur, J., et al. (2021b). Metabolic syndrome, metabolic comorbid conditions and risk of early-onset colorectal cancer. Gut 70, 1147–1154. doi: 10.1136/gutjnl-2020-321661
Chu, H., Duan, Y., Yang, L., and Schnabl, B. (2019). Small metabolites, possible big changes: a microbiota-centered view of non-alcoholic fatty liver disease. Gut 68, 359–370. doi: 10.1136/gutjnl-2018-316307
Cote, D. J., Rosner, B. A., Smith-Warner, S. A., Egan, K. M., and Stampfer, M. J. (2019). Statin use, hyperlipidemia, and risk of glioma. Eur. J. Epidemiol. 34, 997–1011. doi: 10.1007/s10654-019-00565-8
Croci, S., D’apolito, L. I., Gasperi, V., Catani, M. V., and Savini, I. (2021). Dietary strategies for management of metabolic syndrome: role of gut microbiota metabolites. Nutrients 13:1389. doi: 10.3390/nu13051389
Crovesy, L., Masterson, D., and Rosado, E. L. (2020). Profile of the gut microbiota of adults with obesity: a systematic review. Eur. J. Clin. Nutr. 74, 1251–1262. doi: 10.1038/s41430-020-0607-6
Cryan, J. F., O’riordan, K. J., Cowan, C. S. M., Sandhu, K. V., Bastiaanssen, T. F. S., Boehme, M., et al. (2019). The microbiota-gut-brain axis. Physiol. Rev. 99, 1877–2013. doi: 10.1152/physrev.00018.2018
Dabke, K., Hendrick, G., and Devkota, S. (2019). The gut microbiome and metabolic syndrome. J. Clin. Invest. 129, 4050–4057. doi: 10.1172/JCI129194
Dai, X., Hou, H., Zhang, W., Liu, T., Li, Y., Wang, S., et al. (2020). Microbial metabolites: critical regulators in NAFLD. Front. Microbiol. 11:567654. doi: 10.3389/fmicb.2020.567654
Dalby, M. J., Ross, A. W., Walker, A. W., and Morgan, P. J. (2017). Dietary uncoupling of gut microbiota and energy harvesting from obesity and glucose tolerance in mice. Cell Rep. 21, 1521–1533. doi: 10.1016/j.celrep.2017.10.056
Dalile, B., Van Oudenhove, L., Vervliet, B., and Verbeke, K. (2019). The role of short-chain fatty acids in microbiota-gut-brain communication. Nat. Rev. Gastroenterol. Hepatol. 16, 461–478. doi: 10.1038/s41575-019-0157-3
Den Besten, G., Bleeker, A., Gerding, A., Van Eunen, K., Havinga, R., Van Dijk, T. H., et al. (2015). Short-chain fatty acids protect against high-fat diet-induced obesity via a PPARγ-dependent switch from lipogenesis to fat oxidation. Diabetes 64, 2398–2408. doi: 10.2337/db14-1213
Dimitrova-Shumkovska, J., Krstanoski, L., and Veenman, L. (2020). Potential beneficial actions of fucoidan in brain and liver injury, disease, and intoxication-potential implication of sirtuins. Mar. Drugs 18:242. doi: 10.3390/md18050242
Do, M. H., Lee, H. B., Oh, M. J., Jhun, H., Choi, S. Y., and Park, H. Y. (2021). Polysaccharide fraction from greens of Raphanus sativus alleviates high fat diet-induced obesity. Food Chem. 343:128395. doi: 10.1016/j.foodchem.2020.128395
Dong, J., Liang, Q., Niu, Y., Jiang, S., Zhou, L., Wang, J., et al. (2020). Effects of Nigella sativa seed polysaccharides on type 2 diabetic mice and gut microbiota. Int. J. Biol. Macromol. 159, 725–738. doi: 10.1016/j.ijbiomac.2020.05.042
Duan, M., Sun, X., Ma, N., Liu, Y., Luo, T., Song, S., et al. (2019). Polysaccharides from Laminaria japonica alleviated metabolic syndrome in BALB/c mice by normalizing the gut microbiota. Int. J. Biol. Macromol. 121, 996–1004. doi: 10.1016/j.ijbiomac.2018.10.087
Fan, Y., and Pedersen, O. (2021). Gut microbiota in human metabolic health and disease. Nat. Rev. Microbiol. 19, 55–71. doi: 10.1038/s41579-020-0433-9
Fandriks, L. (2017). Roles of the gut in the metabolic syndrome: an overview. J. Intern. Med. 281, 319–336. doi: 10.1111/joim.12584
Feuerstadt, P., Louie, T. J., Lashner, B., Wang, E. E. L., Diao, L., Bryant, J. A., et al. (2022). SER-109, an oral microbiome therapy for recurrent clostridioides difficile infection. N. Engl. J. Med. 386, 220–229. doi: 10.1056/NEJMoa2106516
Foerster, F., Gairing, S. J., Muller, L., and Galle, P. R. (2021). NAFLD-driven HCC: safety and efficacy of current and emerging treatment options. J. Hepatol. 76, 446–457. doi: 10.1016/j.jhep.2021.09.007
Gao, L. L., Li, Y. X., Ma, J. M., Guo, Y. Q., Li, L., Gao, Q. H., et al. (2021a). Effect of Lycium barbarum polysaccharide supplementation in non-alcoholic fatty liver disease patients: study protocol for a randomized controlled trial. Trials 22:566. doi: 10.1186/s13063-021-05529-6
Gao, L. L., Ma, J. M., Fan, Y. N., Zhang, Y. N., Ge, R., Tao, X. J., et al. (2021b). Lycium barbarum polysaccharide combined with aerobic exercise ameliorated nonalcoholic fatty liver disease through restoring gut microbiota, intestinal barrier and inhibiting hepatic inflammation. Int. J. Biol. Macromol. 183, 1379–1392. doi: 10.1016/j.ijbiomac.2021.05.066
Gao, H., Wen, J. J., Hu, J. L., Nie, Q. X., Chen, H. H., Xiong, T., et al. (2018). Polysaccharide from fermented Momordica charantia L. with Lactobacillus plantarum NCU116 ameliorates type 2 diabetes in rats. Carbohydr. Polym. 201, 624–633. doi: 10.1016/j.carbpol.2018.08.075
Gao, Y., Zhang, W., Zeng, L. Q., Bai, H., Li, J., Zhou, J., et al. (2020). Exercise and dietary intervention ameliorate high-fat diet-induced NAFLD and liver aging by inducing lipophagy. Redox Biol. 36:101635. doi: 10.1016/j.redox.2020.101635
Gentile, C. L., and Weir, T. L. (2018). The gut microbiota at the intersection of diet and human health. Science 362, 776–780. doi: 10.1126/science.aau5812
Guo, W. L., Deng, J. C., Pan, Y. Y., Xu, J. X., Hong, J. L., Shi, F. F., et al. (2020). Hypoglycemic and hypolipidemic activities of Grifola frondosa polysaccharides and their relationships with the modulation of intestinal microflora in diabetic mice induced by high-fat diet and streptozotocin. Int. J. Biol. Macromol. 153, 1231–1240. doi: 10.1016/j.ijbiomac.2019.10.253
Guo, Y., Luo, S., Ye, Y., Yin, S., Fan, J., and Xia, M. (2021b). Intermittent fasting improves cardiometabolic risk factors and alters gut microbiota in metabolic syndrome patients. J. Clin. Endocrinol. Metab. 106, 64–79. doi: 10.1210/clinem/dgaa644
Guo, W., Zhu, S., Li, S., Feng, Y., Wu, H., and Zeng, M. (2021a). Microalgae polysaccharides ameliorates obesity in association with modulation of lipid metabolism and gut microbiota in high-fat-diet fed C57BL/6 mice. Int. J. Biol. Macromol. 182, 1371–1383. doi: 10.1016/j.ijbiomac.2021.05.067
Gupta, R., Ambasta, R. K., and Kumar, P. (2022). Multifaced role of protein deacetylase sirtuins in neurodegenerative disease. Neurosci. Biobehav. Rev. 132, 976–997. doi: 10.1016/j.neubiorev.2021.10.047
He, J., Zhang, P., Shen, L., Niu, L., Tan, Y., Chen, L., et al. (2020). Short-chain fatty acids and their association with signalling pathways in inflammation, glucose and lipid metabolism. Int. J. Mol. Sci. 21:6356. doi: 10.3390/ijms21176356
Hong, Y., Sheng, L., Zhong, J., Tao, X., Zhu, W., Ma, J., et al. (2021). Desulfovibrio vulgaris, a potent acetic acid-producing bacterium, attenuates nonalcoholic fatty liver disease in mice. Gut Microbes 13, 1–20. doi: 10.1080/19490976.2021.1930874
Hughes, V. (2014). Weight-loss surgery: a gut-wrenching question. Nature 511, 282–284. doi: 10.1038/511282a
Jensen, V. S., Hvid, H., Damgaard, J., Nygaard, H., Ingvorsen, C., Wulff, E. M., et al. (2018). Dietary fat stimulates development of NAFLD more potently than dietary fructose in Sprague-Dawley rats. Diabetol. Metab. Syndr. 10:4. doi: 10.1186/s13098-018-0307-8
Jia, X., Xu, W., Zhang, L., Li, X., Wang, R., and Wu, S. (2021). Impact of gut microbiota and microbiota-related metabolites on hyperlipidemia. Front. Cell. Infect. Microbiol. 11:634780. doi: 10.3389/fcimb.2021.634780
Jiang, P., Zheng, W., Sun, X., Jiang, G., Wu, S., Xu, Y., et al. (2021). Sulfated polysaccharides from Undaria pinnatifida improved high fat diet-induced metabolic syndrome, gut microbiota dysbiosis and inflammation in BALB/c mice. Int. J. Biol. Macromol. 167, 1587–1597. doi: 10.1016/j.ijbiomac.2020.11.116
Jo, J. K., Seo, S. H., Park, S. E., Kim, H. W., Kim, E. J., Kim, J. S., et al. (2021). Gut microbiome and metabolome profiles associated with high-fat diet in mice. Metabolites 11:482. doi: 10.3390/metabo11080482
Kahn, S. E., Hull, R. L., and Utzschneider, K. M. (2006). Mechanisms linking obesity to insulin resistance and type 2 diabetes. Nature 444, 840–846. doi: 10.1038/nature05482
Kang, Y., Kang, X., Yang, H., Liu, H., Yang, X., Liu, Q., et al. (2022). Lactobacillus acidophilus ameliorates obesity in mice through modulation of gut microbiota dysbiosis and intestinal permeability. Pharmacol. Res. 175:106020. doi: 10.1016/j.phrs.2021.106020
Kanwal, S., Aliya, S., and Xin, Y. (2020). Anti-obesity effect of Dictyophora indusiata mushroom polysaccharide (DIP) in high fat diet-induced obesity via regulating inflammatory cascades and intestinal microbiome. Front. Endocrinol. 11:558874. doi: 10.3389/fendo.2020.558874
Kao, T. W., and Huang, C. C. (2021). Recent progress in metabolic syndrome research and therapeutics. Int. J. Mol. Sci. 22:6862. doi: 10.3390/ijms22136862
Ke, S., Yu, Y., Xu, Q., Zhang, B., Wang, S., Jin, W., et al. (2021). Composition-activity relationships of polysaccharides from Saccharina japonica in regulating gut microbiota in short-term high-fat diet-fed mice. J. Agric. Food Chem. 69, 11121–11130. doi: 10.1021/acs.jafc.1c04490
Kelly, T., Yang, W., Chen, C. S., Reynolds, K., and He, J. (2008). Global burden of obesity in 2005 and projections to 2030. Int. J. Obes. 32, 1431–1437. doi: 10.1038/ijo.2008.102
Kim, J., Lee, H., An, J., Song, Y., Lee, C. K., Kim, K., et al. (2019). Alterations in gut microbiota by statin therapy and possible intermediate effects on hyperglycemia and hyperlipidemia. Front. Microbiol. 10:1947. doi: 10.3389/fmicb.2019.01947
Kimura, I., Miyamoto, J., Ohue-Kitano, R., Watanabe, K., Yamada, T., Onuki, M., et al. (2020). Maternal gut microbiota in pregnancy influences offspring metabolic phenotype in mice. Science 367:eaaw8429. doi: 10.1126/science.aaw8429
Koh, A., De Vadder, F., Kovatcheva-Datchary, P., and Backhed, F. (2016). From dietary fiber to host physiology: short-chain fatty acids as key bacterial metabolites. Cell 165, 1332–1345. doi: 10.1016/j.cell.2016.05.041
Kong, Y., Li, Y., Dai, Z. R., Qin, M., Fan, H. L., Hao, J. G., et al. (2021). Glycosaminoglycan from Ostrea rivularis attenuates hyperlipidemia and regulates gut microbiota in high-cholesterol diet-fed zebrafish. Food Sci. Nutr. 9, 5198–5210. doi: 10.1002/fsn3.2492
Labbe, D. P., Zadra, G., Yang, M., Reyes, J. M., Lin, C. Y., Cacciatore, S., et al. (2019). High-fat diet fuels prostate cancer progression by rewiring the metabolome and amplifying the MYC program. Nat. Commun. 10:4358. doi: 10.1038/s41467-019-12298-z
Laurent, G., De Boer, V. C., Finley, L. W., Sweeney, M., Lu, H., Schug, T. T., et al. (2013). SIRT4 represses peroxisome proliferator-activated receptor alpha activity to suppress hepatic fat oxidation. Mol. Cell. Biol. 33, 4552–4561. doi: 10.1128/MCB.00087-13
Laursen, M. F., Sakanaka, M., Von Burg, N., Morbe, U., Andersen, D., Moll, J. M., et al. (2021). Bifidobacterium species associated with breastfeeding produce aromatic lactic acids in the infant gut. Nat. Microbiol. 6, 1367–1382. doi: 10.1038/s41564-021-00970-4
Lazarus, J. V., Mark, H. E., Villota-Rivas, M., Palayew, A., Carrieri, P., Colombo, M., et al. (2021). The global NAFLD policy review and preparedness index: are countries ready to address this silent public health challenge? J. Hepatol. doi: 10.1016/j.jhep.2021.10.025 [Epub ahead of print].
Le Roux, C. W. (2021). COVID-19 alters thinking and management in metabolic diseases. Nat. Rev. Endocrinol. 17, 71–72. doi: 10.1038/s41574-020-00449-y
Le Roy, T., Llopis, M., Lepage, P., Bruneau, A., Rabot, S., Bevilacqua, C., et al. (2013). Intestinal microbiota determines development of non-alcoholic fatty liver disease in mice. Gut 62, 1787–1794. doi: 10.1136/gutjnl-2012-303816
Lee, B. H., Chen, C. H., Hsu, Y. Y., Chuang, P. T., Shih, M. K., and Hsu, W. H. (2021). Polysaccharides obtained from Cordyceps militaris alleviate hyperglycemia by regulating gut microbiota in mice fed a high-fat/sucrose diet. Foods 10:1870. doi: 10.3390/foods10081870
Leung, C., Rivera, L., Furness, J. B., and Angus, P. W. (2016). The role of the gut microbiota in NAFLD. Nat. Rev. Gastroenterol. Hepatol. 13, 412–425. doi: 10.1038/nrgastro.2016.85
Li, S., Dou, X., Ning, H., Song, Q., Wei, W., Zhang, X., et al. (2017). Sirtuin 3 acts as a negative regulator of autophagy dictating hepatocyte susceptibility to lipotoxicity. Hepatology 66, 936–952. doi: 10.1002/hep.29229
Li, H., Fang, Q., Nie, Q., Hu, J., Yang, C., Huang, T., et al. (2020a). Hypoglycemic and hypolipidemic mechanism of tea polysaccharides on type 2 diabetic rats via gut microbiota and metabolism alteration. J. Agric. Food Chem. 68, 10015–10028. doi: 10.1021/acs.jafc.0c01968
Li, L., Guo, W. L., Zhang, W., Xu, J. X., Qian, M., Bai, W. D., et al. (2019a). Grifola frondosa polysaccharides ameliorate lipid metabolic disorders and gut microbiota dysbiosis in high-fat diet fed rats. Food Funct. 10, 2560–2572. doi: 10.1039/c9fo00075e
Li, Q., Hu, J., Nie, Q., Chang, X., Fang, Q., Xie, J., et al. (2021b). Hypoglycemic mechanism of polysaccharide from Cyclocarya paliurus leaves in type 2 diabetic rats by gut microbiota and host metabolism alteration. Sci. China Life Sci. 64, 117–132. doi: 10.1007/s11427-019-1647-6
Li, S., Li, J., Mao, G., Wu, T., Hu, Y., Ye, X., et al. (2018). A fucoidan from sea cucumber Pearsonothuria graeffei with well-repeated structure alleviates gut microbiota dysbiosis and metabolic syndromes in HFD-fed mice. Food Funct. 9, 5371–5380. doi: 10.1039/C8FO01174E
Li, S., Li, J., Mao, G., Wu, T., Lin, D., Hu, Y., et al. (2019b). Fucosylated chondroitin sulfate from Isostichopus badionotus alleviates metabolic syndromes and gut microbiota dysbiosis induced by high-fat and high-fructose diet. Int. J. Biol. Macromol. 124, 377–388. doi: 10.1016/j.ijbiomac.2018.11.167
Li, J., Pang, B., Shao, D., Jiang, C., Hu, X., and Shi, J. (2020b). Artemisia sphaerocephala Krasch polysaccharide mediates lipid metabolism and metabolic endotoxaemia in associated with the modulation of gut microbiota in diet-induced obese mice. Int. J. Biol. Macromol. 147, 1008–1017. doi: 10.1016/j.ijbiomac.2019.10.069
Li, J., Song, J., Zaytseva, Y. Y., Liu, Y., Rychahou, P., Jiang, K., et al. (2016). An obligatory role for neurotensin in high-fat-diet-induced obesity. Nature 533, 411–415. doi: 10.1038/nature17662
Li, X., Wang, Y., Xing, Y., Xing, R., Liu, Y., and Xu, Y. (2020c). Changes of gut microbiota during silybin-mediated treatment of high-fat diet-induced non-alcoholic fatty liver disease in mice. Hepatol. Res. 50, 5–14. doi: 10.1111/hepr.13444
Li, C., Wu, G., Zhao, H., Dong, N., Wu, B., Chen, Y., et al. (2021a). Natural-derived polysaccharides from plants, mushrooms, and seaweeds for the treatment of inflammatory bowel disease. Front. Pharmacol. 12:651813. doi: 10.3389/fphar.2021.651813
Li, Y., Xu, W., Sun, Y., Wang, Y., Tang, Y., Li, Y., et al. (2020d). Modified apple polysaccharide regulates microbial dysbiosis to suppress high-fat diet-induced obesity in C57BL/6J mice. Eur. J. Nutr. 59, 2025–2037. doi: 10.1007/s00394-019-02051-z
Li, R., Xue, Z., Li, S., Zhou, J., Liu, J., Zhang, M., et al. (2021c). Mulberry leaf polysaccharides ameliorate obesity through activation of brown adipose tissue and modulation of the gut microbiota in high-fat diet fed mice. Food Funct. 13, 561–573. doi: 10.1039/d1fo02324a
Lingvay, I., Sumithran, P., Cohen, R. V., and Le Roux, C. W. (2021). Obesity management as a primary treatment goal for type 2 diabetes: time to reframe the conversation. Lancet 399, 394–405. doi: 10.1016/S0140-6736(21)01919-X
Liu, C., Du, P., Cheng, Y., Guo, Y., Hu, B., Yao, W., et al. (2021a). Study on fecal fermentation characteristics of aloe polysaccharides in vitro and their predictive modeling. Carbohydr. Polym. 256:117571. doi: 10.1016/j.carbpol.2020.117571
Liu, X., Li, X., Xia, B., Jin, X., Zou, Q., Zeng, Z., et al. (2021b). High-fiber diet mitigates maternal obesity-induced cognitive and social dysfunction in the offspring via gut-brain axis. Cell Metab. 33, 923.e6–938.e6. doi: 10.1016/j.cmet.2021.02.002
Liu, G., Liang, L., Yu, G., and Li, Q. (2018). Pumpkin polysaccharide modifies the gut microbiota during alleviation of type 2 diabetes in rats. Int. J. Biol. Macromol. 115, 711–717. doi: 10.1016/j.ijbiomac.2018.04.127
Loos, R. J. F., and Yeo, G. S. H. (2021). The genetics of obesity: from discovery to biology. Nat. Rev. Genet. 23, 120–133. doi: 10.1038/s41576-021-00414-z
Lyu, M., Wang, Y. F., Fan, G. W., Wang, X. Y., Xu, S. Y., and Zhu, Y. (2017). Balancing herbal medicine and functional food for prevention and treatment of cardiometabolic diseases through modulating gut microbiota. Front. Microbiol. 8:2146. doi: 10.3389/fmicb.2017.02146
Magne, F., Gotteland, M., Gauthier, L., Zazueta, A., Pesoa, S., Navarrete, P., et al. (2020). The firmicutes/bacteroidetes ratio: a relevant marker of gut dysbiosis in obese patients? Nutrients 12:1474. doi: 10.3390/nu12051474
Marchesi, J. R., Adams, D. H., Fava, F., Hermes, G. D., Hirschfield, G. M., Hold, G., et al. (2016). The gut microbiota and host health: a new clinical frontier. Gut 65, 330–339. doi: 10.1136/gutjnl-2015-309990
Mariani, S., Fiore, D., Basciani, S., Persichetti, A., Contini, S., Lubrano, C., et al. (2015). Plasma levels of SIRT1 associate with non-alcoholic fatty liver disease in obese patients. Endocrine 49, 711–716. doi: 10.1007/s12020-014-0465-x
Merino, J., Guasch-Ferre, M., Ellervik, C., Dashti, H. S., Sharp, S. J., Wu, P., et al. (2019). Quality of dietary fat and genetic risk of type 2 diabetes: individual participant data meta-analysis. BMJ 366:l4292. doi: 10.1136/bmj.l4292
Mirzadeh, M., Lelekami, A. K., and Khedmat, L. (2021). Plant/algal polysaccharides extracted by microwave: a review on hypoglycemic, hypolipidemic, prebiotic, and immune-stimulatory effect. Carbohydr. Polym. 266:118134. doi: 10.1016/j.carbpol.2021.118134
Miyamoto, J., Igarashi, M., Watanabe, K., Karaki, S. I., Mukouyama, H., Kishino, S., et al. (2019). Gut microbiota confers host resistance to obesity by metabolizing dietary polyunsaturated fatty acids. Nat. Commun. 10:4007. doi: 10.1038/s41467-019-11978-0
Nakahara, D., Nan, C., Mori, K., Hanayama, M., Kikuchi, H., Hirai, S., et al. (2020). Effect of mushroom polysaccharides from Pleurotus eryngii on obesity and gut microbiota in mice fed a high-fat diet. Eur. J. Nutr. 59, 3231–3244. doi: 10.1007/s00394-019-02162-7
Nasrin, N., Wu, X., Fortier, E., Feng, Y., Bare, O. C., Chen, S., et al. (2010). SIRT4 regulates fatty acid oxidation and mitochondrial gene expression in liver and muscle cells. J. Biol. Chem. 285, 31995–32002. doi: 10.1074/jbc.M110.124164
Nassir, F., and Ibdah, J. A. (2016). Sirtuins and nonalcoholic fatty liver disease. World J. Gastroenterol. 22, 10084–10092. doi: 10.3748/wjg.v22.i46.10084
Nazzal, Z., Khatib, B., Al-Quqa, B., Abu-Taha, L., and Jaradat, A. (2021). The prevalence and risk factors of urinary incontinence among women with type 2 diabetes in the north west bank: a cross-sectional study. Lancet 398:S42. doi: 10.1016/S0140-6736(21)01528-2
Negi, C. K., Babica, P., Bajard, L., Bienertova-Vasku, J., and Tarantino, G. (2022). Insights into the molecular targets and emerging pharmacotherapeutic interventions for nonalcoholic fatty liver disease. Metabolism 126:154925. doi: 10.1016/j.metabol.2021.154925
Palomer, X., Aguilar-Recarte, D., Garcia, R., Nistal, J. F., and Vazquez-Carrera, M. (2021). Sirtuins: to be or not to be in diabetic cardiomyopathy. Trends Mol. Med. 27, 554–571. doi: 10.1016/j.molmed.2021.03.004
Rabot, S., Membrez, M., Bruneau, A., Gerard, P., Harach, T., Moser, M., et al. (2010). Germ-free C57BL/6J mice are resistant to high-fat-diet-induced insulin resistance and have altered cholesterol metabolism. FASEB J. 24, 4948–4959. doi: 10.1096/fj.10-164921
Ren, H., Hu, F., Wang, D., Kang, X., Feng, X., Zhang, L., et al. (2021). Sirtuin 2 prevents liver steatosis and metabolic disorders by deacetylation of hepatocyte nuclear factor 4α. Hepatology 74, 723–740. doi: 10.1002/hep.31773
Rodriguez, J., Hiel, S., Neyrinck, A. M., Le Roy, T., Potgens, S. A., Leyrolle, Q., et al. (2020). Discovery of the gut microbial signature driving the efficacy of prebiotic intervention in obese patients. Gut 69, 1975–1987. doi: 10.1136/gutjnl-2019-319726
Sang, T., Guo, C., Guo, D., Wu, J., Wang, Y., Wang, Y., et al. (2021). Suppression of obesity and inflammation by polysaccharide from sporoderm-broken spore of Ganoderma lucidum via gut microbiota regulation. Carbohydr. Polym. 256:117594. doi: 10.1016/j.carbpol.2020.117594
Schluter, J., Peled, J. U., Taylor, B. P., Markey, K. A., Smith, M., Taur, Y., et al. (2020). The gut microbiota is associated with immune cell dynamics in humans. Nature 588, 303–307. doi: 10.1038/s41586-020-2971-8
Shang, Q., Jiang, H., Cai, C., Hao, J., Li, G., and Yu, G. (2018). Gut microbiota fermentation of marine polysaccharides and its effects on intestinal ecology: an overview. Carbohydr. Polym. 179, 173–185. doi: 10.1016/j.carbpol.2017.09.059
Sharon, G., Cruz, N. J., Kang, D. W., Gandal, M. J., Wang, B., Kim, Y. M., et al. (2019). Human gut microbiota from autism spectrum disorder promote behavioral symptoms in mice. Cell 177, 1600.e17–1618.e17. doi: 10.1016/j.cell.2019.05.004
Siehler, J., Blochinger, A. K., Meier, M., and Lickert, H. (2021). Engineering islets from stem cells for advanced therapies of diabetes. Nat. Rev. Drug Discov. 20, 920–940. doi: 10.1038/s41573-021-00262-w
Soh, J., Iqbal, J., Queiroz, J., Fernandez-Hernando, C., and Hussain, M. M. (2013). MicroRNA-30c reduces hyperlipidemia and atherosclerosis in mice by decreasing lipid synthesis and lipoprotein secretion. Nat. Med. 19, 892–900. doi: 10.1038/nm.3200
Song, Q., Wang, Y., Huang, L., Shen, M., Yu, Y., Yu, Q., et al. (2021). Review of the relationships among polysaccharides, gut microbiota, and human health. Food Res. Int. 140:109858. doi: 10.1016/j.foodres.2020.109858
Sonnenburg, J. L., and Backhed, F. (2016). Diet-microbiota interactions as moderators of human metabolism. Nature 535, 56–64. doi: 10.1038/nature18846
Sonnenburg, E. D., and Sonnenburg, J. L. (2014). Starving our microbial self: the deleterious consequences of a diet deficient in microbiota-accessible carbohydrates. Cell Metab. 20, 779–786. doi: 10.1016/j.cmet.2014.07.003
Srinivasan, K., Viswanad, B., Asrat, L., Kaul, C. L., and Ramarao, P. (2005). Combination of high-fat diet-fed and low-dose streptozotocin-treated rat: a model for type 2 diabetes and pharmacological screening. Pharmacol. Res. 52, 313–320. doi: 10.1016/j.phrs.2005.05.004
Steenblock, C., Schwarz, P. E. H., Ludwig, B., Linkermann, A., Zimmet, P., Kulebyakin, K., et al. (2021). COVID-19 and metabolic disease: mechanisms and clinical management. Lancet Diabetes Endocrinol. 9, 786–798. doi: 10.1016/S2213-8587(21)00244-8
Su, Y., Li, J., Wu, L., and Kuang, H. (2021). Polysaccharides from TCM herbs exhibit potent anti-obesity effect by mediating the community structure of gut microbiota. Pharmazie 76, 473–479. doi: 10.1691/ph.2021.1463
Sugimura, N., Li, Q., Chu, E. S. H., Lau, H. C. H., Fong, W., Liu, W., et al. (2021). Lactobacillus gallinarum modulates the gut microbiota and produces anti-cancer metabolites to protect against colorectal tumourigenesis. Gut doi: 10.1136/gutjnl-2020-323951 [Epub ahead of print].
Tarantino, G., and Finelli, C. (2015). Systematic review on intervention with prebiotics/probiotics in patients with obesity-related nonalcoholic fatty liver disease. Future Microbiol. 10, 889–902. doi: 10.2217/fmb.15.13
Tarantino, G., Finelli, C., Scopacasa, F., Pasanisi, F., Contaldo, F., Capone, D., et al. (2014). Circulating levels of sirtuin 4, a potential marker of oxidative metabolism, related to coronary artery disease in obese patients suffering from NAFLD, with normal or slightly increased liver enzymes. Oxidative Med. Cell. Longev. 2014:920676. doi: 10.1155/2014/920676
Tilg, H., and Moschen, A. R. (2014). Microbiota and diabetes: an evolving relationship. Gut 63, 1513–1521. doi: 10.1136/gutjnl-2014-306928
Tomas, J., Mulet, C., Saffarian, A., Cavin, J. B., Ducroc, R., Regnault, B., et al. (2016). High-fat diet modifies the PPAR-gamma pathway leading to disruption of microbial and physiological ecosystem in murine small intestine. Proc. Natl. Acad. Sci. U. S. A. 113, E5934–E5943. doi: 10.1073/pnas.1612559113
Torres-Fuentes, C., Schellekens, H., Dinan, T. G., and Cryan, J. F. (2017). The microbiota–gut–brain axis in obesity. Lancet Gastroenterol. Hepatol. 2, 747–756. doi: 10.1016/S2468-1253(17)30147-4
Tripathi, A., Debelius, J., Brenner, D. A., Karin, M., Loomba, R., Schnabl, B., et al. (2018). The gut-liver axis and the intersection with the microbiome. Nat. Rev. Gastroenterol. Hepatol. 15, 397–411. doi: 10.1038/s41575-018-0011-z
Turnbaugh, P. J., Ley, R. E., Mahowald, M. A., Magrini, V., Mardis, E. R., and Gordon, J. I. (2006). An obesity-associated gut microbiome with increased capacity for energy harvest. Nature 444, 1027–1031. doi: 10.1038/nature05414
Ursell, L. K., Clemente, J. C., Rideout, J. R., Gevers, D., Caporaso, J. G., and Knight, R. (2012). The interpersonal and intrapersonal diversity of human-associated microbiota in key body sites. J. Allergy Clin. Immunol. 129, 1204–1208. doi: 10.1016/j.jaci.2012.03.010
Van De Wouw, M., Boehme, M., Lyte, J. M., Wiley, N., Strain, C., O’sullivan, O., et al. (2018). Short-chain fatty acids: microbial metabolites that alleviate stress-induced brain-gut axis alterations. J. Physiol. 596, 4923–4944. doi: 10.1113/JP276431
Wan, Y., Wang, F., Yuan, J., Li, J., Jiang, D., Zhang, J., et al. (2019). Effects of dietary fat on gut microbiota and faecal metabolites, and their relationship with cardiometabolic risk factors: a 6-month randomised controlled-feeding trial. Gut 68, 1417–1429. doi: 10.1136/gutjnl-2018-317609
Wang, Y., Fei, Y., Liu, L., Xiao, Y., Pang, Y., Kang, J., et al. (2018b). Polygonatum odoratum polysaccharides modulate gut microbiota and mitigate experimentally induced obesity in rats. Int. J. Mol. Sci. 19:3587. doi: 10.3390/ijms19113587
Wang, B., Jiang, X., Cao, M., Ge, J., Bao, Q., Tang, L., et al. (2016). Altered fecal microbiota correlates with liver biochemistry in nonobese patients with non-alcoholic fatty liver disease. Sci. Rep. 6:32002. doi: 10.1038/srep32002
Wang, X., Shi, L., Wang, X., Feng, Y., and Wang, Y. (2019). MDG-1, an Ophiopogon polysaccharide, restrains process of non-alcoholic fatty liver disease via modulating the gut-liver axis. Int. J. Biol. Macromol. 141, 1013–1021. doi: 10.1016/j.ijbiomac.2019.09.007
Wang, X., Wang, X., Jiang, H., Cai, C., Li, G., Hao, J., et al. (2018a). Marine polysaccharides attenuate metabolic syndrome by fermentation products and altering gut microbiota: an overview. Carbohydr. Polym. 195, 601–612. doi: 10.1016/j.carbpol.2018.05.003
Wang, G., Yang, X., Wang, J., Zhong, D., Zhang, R., Zhang, Y., et al. (2021). Walnut green husk polysaccharides prevent obesity, chronic inflammatory responses, nonalcoholic fatty liver disease and colonic tissue damage in high-fat diet fed rats. Int. J. Biol. Macromol. 182, 879–898. doi: 10.1016/j.ijbiomac.2021.04.047
Wang, W., Zhong, M., Yu, T., Chen, L., Shi, L., Zong, J., et al. (2020). Polysaccharide extracted from WuGuChong reduces high-fat diet-induced obesity in mice by regulating the composition of intestinal microbiota. Nutr. Metab. 17:27. doi: 10.1186/s12986-020-00442-2
Wastyk, H. C., Fragiadakis, G. K., Perelman, D., Dahan, D., Merrill, B. D., Yu, F. B., et al. (2021). Gut-microbiota-targeted diets modulate human immune status. Cell 184, 4137.e14–4153.e14. doi: 10.1016/j.cell.2021.06.019
Wei, J., Zhao, Y., Zhou, C., Zhao, Q., Zhong, H., Zhu, X., et al. (2021). Dietary polysaccharide from Enteromorpha clathrata attenuates obesity and increases the intestinal abundance of butyrate-producing bacterium, Eubacterium xylanophilum, in mice fed a high-fat diet. Polymers 13:3286. doi: 10.3390/polym13193286
Wen, J. J., Li, M. Z., Gao, H., Hu, J. L., Nie, Q. X., Chen, H. H., et al. (2021). Polysaccharides from fermented Momordica charantia L. with Lactobacillus plantarum NCU116 ameliorate metabolic disorders and gut microbiota change in obese rats. Food Funct. 12, 2617–2630. doi: 10.1039/D0FO02600J
Wong, S. H., and Yu, J. (2019). Gut microbiota in colorectal cancer: mechanisms of action and clinical applications. Nat. Rev. Gastroenterol. Hepatol. 16, 690–704. doi: 10.1038/s41575-019-0209-8
Wu, T. R., Lin, C. S., Chang, C. J., Lin, T. L., Martel, J., Ko, Y. F., et al. (2019b). Gut commensal Parabacteroides goldsteinii plays a predominant role in the anti-obesity effects of polysaccharides isolated from Hirsutella sinensis. Gut 68, 248–262. doi: 10.1136/gutjnl-2017-315458
Wu, T., Liu, Y. H., Fu, Y. C., Liu, X. M., and Zhou, X. H. (2014). Direct evidence of sirtuin downregulation in the liver of non-alcoholic fatty liver disease patients. Ann. Clin. Lab. Sci. 44, 410–418.
Wu, S., Liu, Y., Jiang, P., Xu, Y., Zheng, W., Song, S., et al. (2020). Effect of sulfate group on sulfated polysaccharides-induced improvement of metabolic syndrome and gut microbiota dysbiosis in high fat diet-fed mice. Int. J. Biol. Macromol. 164, 2062–2072. doi: 10.1016/j.ijbiomac.2020.08.010
Wu, H. Q., Ma, Z. L., Zhang, D. X., Wu, P., Guo, Y. H., Yang, F., et al. (2021). Sequential extraction, characterization, and analysis of pumpkin polysaccharides for their hypoglycemic activities and effects on gut microbiota in mice. Front. Nutr. 8:769181. doi: 10.3389/fnut.2021.769181
Wu, J., Shao, H., Zhang, J., Ying, Y., Cheng, Y., Zhao, D., et al. (2019a). Mussel polysaccharide alpha-D-glucan (MP-A) protects against non-alcoholic fatty liver disease via maintaining the homeostasis of gut microbiota and regulating related gut-liver axis signaling pathways. Int. J. Biol. Macromol. 130, 68–78. doi: 10.1016/j.ijbiomac.2019.02.097
Wutthi-In, M., Cheevadhanarak, S., Yasom, S., Kerdphoo, S., Thiennimitr, P., Phrommintikul, A., et al. (2020). Gut microbiota profiles of treated metabolic syndrome patients and their relationship with metabolic health. Sci. Rep. 10:10085. doi: 10.1038/s41598-020-67078-3
Xia, T., Liu, C. S., Hu, Y. N., Luo, Z. Y., Chen, F. L., Yuan, L. X., et al. (2021). Coix seed polysaccharides alleviate type 2 diabetes mellitus via gut microbiota-derived short-chain fatty acids activation of IGF1/PI3K/AKT signaling. Food Res. Int. 150:110717. doi: 10.1016/j.foodres.2021.110717
Yachida, S., Mizutani, S., Shiroma, H., Shiba, S., Nakajima, T., Sakamoto, T., et al. (2019). Metagenomic and metabolomic analyses reveal distinct stage-specific phenotypes of the gut microbiota in colorectal cancer. Nat. Med. 25, 968–976. doi: 10.1038/s41591-019-0458-7
Yang, C., Du, Y., Ren, D., Yang, X., and Zhao, Y. (2021a). Gut microbiota-dependent catabolites of tryptophan play a predominant role in the protective effects of turmeric polysaccharides against DSS-induced ulcerative colitis. Food Funct. 12, 9793–9807. doi: 10.1039/d1fo01468d
Yang, X., Mo, W., Zheng, C., Li, W., Tang, J., and Wu, X. (2020b). Alleviating effects of noni fruit polysaccharide on hepatic oxidative stress and inflammation in rats under a high-fat diet and its possible mechanisms. Food Funct. 11, 2953–2968. doi: 10.1039/d0fo00178c
Yang, J., Wei, H., Zhou, Y., Szeto, C. H., Li, C., Lin, Y., et al. (2022). High-fat diet promotes colorectal tumorigenesis through modulating gut microbiota and metabolites 162, 135.e2–149.e2. doi: 10.1053/j.gastro.2021.08.041
Yang, C., Xu, Z., Deng, Q., Huang, Q., Wang, X., and Huang, F. (2020a). Beneficial effects of flaxseed polysaccharides on metabolic syndrome via gut microbiota in high-fat diet fed mice. Food Res. Int. 131:108994. doi: 10.1016/j.foodres.2020.108994
Yang, M., Yin, Y., Wang, F., Zhang, H., Ma, X., Yin, Y., et al. (2021b). Supplementation with Lycium barbarum polysaccharides reduce obesity in high-fat diet-fed mice by modulation of gut microbiota. Front. Microbiol. 12:719967. doi: 10.3389/fmicb.2021.719967
Yao, Y., Yan, L., Chen, H., Wu, N., Wang, W., and Wang, D. (2020). Cyclocarya paliurus polysaccharides alleviate type 2 diabetic symptoms by modulating gut microbiota and short-chain fatty acids. Phytomedicine 77:153268. doi: 10.1016/j.phymed.2020.153268
Ye, L., Mueller, O., Bagwell, J., Bagnat, M., Liddle, R. A., and Rawls, J. F. (2019). High fat diet induces microbiota-dependent silencing of enteroendocrine cells. eLife 8:e48479. doi: 10.7554/eLife.48479
Yin, C., Noratto, G. D., Fan, X., Chen, Z., Yao, F., Shi, D., et al. (2020). The impact of mushroom polysaccharides on gut microbiota and its beneficial effects to host: a review. Carbohydr. Polym. 250:116942. doi: 10.1016/j.carbpol.2020.116942
Yu, M., Yue, J., Hui, N., Zhi, Y., Hayat, K., Yang, X., et al. (2021). Anti-hyperlipidemia and gut microbiota community regulation effects of selenium-rich Cordyceps militaris polysaccharides on the high-fat diet-fed mice model. Foods 10:2252. doi: 10.3390/foods10102252
Yuan, Y., Liu, Q., Zhao, F., Cao, J., Shen, X., and Li, C. (2019). Holothuria leucospilota polysaccharides ameliorate hyperlipidemia in high-fat diet-induced rats via short-chain fatty acids production and lipid metabolism regulation. Int. J. Mol. Sci. 20:4738. doi: 10.3390/ijms20194738
Zhang, Z., Ran, C., Ding, Q. W., Liu, H. L., Xie, M. X., Yang, Y. L., et al. (2019). Ability of prebiotic polysaccharides to activate a HIF1alpha-antimicrobial peptide axis determines liver injury risk in zebrafish. Commun. Biol. 2:274. doi: 10.1038/s42003-019-0526-z
Zhang, Y., Yang, L., Zhao, N., Hong, Z., Cai, B., Le, Q., et al. (2021b). Soluble polysaccharide derived from Laminaria japonica attenuates obesity-related nonalcoholic fatty liver disease associated with gut microbiota regulation. Mar. Drugs 19:699. doi: 10.3390/md19120699
Zhang, C., Yin, A., Li, H., Wang, R., Wu, G., Shen, J., et al. (2015). Dietary modulation of gut microbiota contributes to alleviation of both genetic and simple obesity in children. EBioMedicine 2, 968–984. doi: 10.1016/j.ebiom.2015.07.007
Zhang, S., Zhao, J., Xie, F., He, H., Johnston, L. J., Dai, X., et al. (2021a). Dietary fiber-derived short-chain fatty acids: a potential therapeutic target to alleviate obesity-related nonalcoholic fatty liver disease. Obes. Rev. 22:e13316. doi: 10.1111/obr.13316
Zhao, R., Ji, Y., Chen, X., Hu, Q., and Zhao, L. (2021a). Polysaccharide from Flammulina velutipes attenuates markers of metabolic syndrome by modulating the gut microbiota and lipid metabolism in high fat diet-fed mice. Food Funct. 12, 6964–6980. doi: 10.1039/d1fo00534k
Zhao, Y., Liu, X., Zheng, Y., Liu, W., and Ding, C. (2021b). Aronia melanocarpa polysaccharide ameliorates inflammation and aging in mice by modulating the AMPK/SIRT1/NF-kappaB signaling pathway and gut microbiota. Sci. Rep. 11:20558. doi: 10.1038/s41598-021-00071-6
Zheng, W., Duan, M., Jia, J., Song, S., and Ai, C. (2021). Low-molecular alginate improved diet-induced obesity and metabolic syndrome through modulating the gut microbiota in BALB/c mice. Int. J. Biol. Macromol. 187, 811–820. doi: 10.1016/j.ijbiomac.2021.08.003
Zhu, Z., Zhu, B., Sun, Y., Ai, C., Wang, L., Wen, C., et al. (2018). Sulfated polysaccharide from sea cucumber and its depolymerized derivative prevent obesity in association with modification of gut microbiota in high-fat diet-fed mice. Mol. Nutr. Food Res. 62:e1800446. doi: 10.1002/mnfr.201800446
Keywords: high-fat diet, metabolic diseases, gut microbiota, polysaccharides, obesity
Citation: Sun C-Y, Zheng Z-L, Chen C-W, Lu B-W and Liu D (2022) Targeting Gut Microbiota With Natural Polysaccharides: Effective Interventions Against High-Fat Diet-Induced Metabolic Diseases. Front. Microbiol. 13:859206. doi: 10.3389/fmicb.2022.859206
Edited by:
Marciane Magnani, Federal University of Paraíba, BrazilReviewed by:
Giovanni Tarantino, University of Naples Federico II, ItalyCarmine Finelli, Ospedale Cav. R. Apicella – ASL Napoli 3 Sud, Italy
Copyright © 2022 Sun, Zheng, Chen, Lu and Liu. This is an open-access article distributed under the terms of the Creative Commons Attribution License (CC BY). The use, distribution or reproduction in other forums is permitted, provided the original author(s) and the copyright owner(s) are credited and that the original publication in this journal is cited, in accordance with accepted academic practice. No use, distribution or reproduction is permitted which does not comply with these terms.
*Correspondence: Dong Liu, bGl1ZG9uZ0AxMjYuY29t