- 1Infectious Diseases Department, National Institute of Health Dr. Ricardo Jorge (INSA), Lisbon, Portugal
- 2Faculty of Veterinary Medicine, Lusófona University, Lisbon, Portugal
- 3CBIOS – Lusófona University Research Centre for Biosciences & Health Technologies, Lisbon, Portugal
- 4National Zootechnical Station, National Institute for Agrarian and Veterinarian Research, Santarém, Portugal
The recent increase in community-acquired Clostridioides difficile infections discloses the shift in this bacterium epidemiology. This study aimed at establishing a transmission network involving One Health components, as well as assessing the zoonotic potential and genomic features of dominant clones. Samples were collected from different compartments of animal, human and environmental origin, from an animal production unit. C. difficile isolates were characterized for toxigenic profile by multiplex-PCR, while genetic diversity was evaluated by PCR-ribotyping and whole genome-based analysis. The overall C. difficile prevalence was 37.2% (70/188), and included samples from environmental (58.3%, 35/60) and animal (31.5%, 35/111) compartments; human samples (n = 17) taken from healthy workers were negative. A predominant clone from RT033 was found in almost 90% of the positive samples, including samples from all compartments connected to the pig production unit, with core-genome single nucleotide variant (SNV)-based Analysis supporting a clonal transmission between them (mean distance of 0.1 ± 0.1 core-SNVs). The isolates from this clone (herein designated PT RT033) were positive for all C. difficile toxin genes (tcdA, tcdB, cdtA/cdtB). The phyloGenetic positioning of this clone was clearly distinct from the classical RT033 cluster, suggesting a different evolutionary route. This new clone shares genomic features with several RTs from the clade 5 Sequence Type (ST) 11, including a complete pathogenicity locus (PaLoc) that is more similar to the one found in toxigenic strains and contrasting to the less virulent classical RT033 (tcdA-, tcdB-, cdtA + /cdtB +). The presence of a tcdA gene truncated into two ORFs, not previously described, requires further evaluation concerning toxin functionality. We hypothesize that the unique combination of genetic elements found in the PT RT033 clone may contribute to host tropism and environmental dissemination and maintenance. This study constitutes the first report of a toxigenic RT033 clone and adds to the overall knowledge on Clade 5 sequence type 11, considered the C. difficile evolutionary lineage with the highest zoonotic potential. The presence of this clone in all compartments associated with the pig production unit suggests a transmission chain involving these animals and contributes to unveil the role played by animal and environmental reservoirs in this pathogen epidemiology.
Introduction
Clostridioides difficile is a Gram-positive spore-forming anaerobic bacterium usually isolated from the feces of asymptomatic young animal and humans. The immature gut microbiota of these individuals allows the overgrowth of C. difficile over other enterobacterium. Overlooked as a major enteric pathogen for many years, a surge in diarrheic hospitalized patients was observed in recent years with the increase of antibiotic usage in healthcare facilities. C. difficile infection (CDI) severity can vary from mild diarrhea to life threatening pseudomembranous colitis, affecting mostly elderly hospitalized patients with a recent history of antimicrobial treatment. Nosocomial symptomatic infections were first associated with clindamycin consumption (Lance George et al., 1978). Other antibiotic groups that strongly associate with the development of symptomatic infection are second and third generation cephalosporins and fluoroquinolones (Brown et al., 2013; Slimings and Riley, 2014). The antibiotic-mediated alterations result in a gut microbiome derangement with a reduction of competitive flora that promote a shift in the gastrointestinal metabolic state to one that favors C. difficile germination, growth and toxin production (Giel et al., 2010; Buffie et al., 2012; Francis et al., 2013).
The C. difficile pathogenic potential is linked to the expression of two major cytotoxins encoded in the pathogenicity locus (PaLoc), the toxin A (TcdA) and the toxin B (TcdB; Voth and Ballard, 2005). Three supplementary regulatory genes are also present in the PaLoc: tcdE, tcdR, and tcdC (Chandrasekaran and Lacy, 2017). In addition, some strains of C. difficile produce a binary toxin (CDT) that contributes for the bacteria adhesion to the intestinal epithelial cells. Although its presence does not directly correlate to the severity of the clinical outcome (Berry et al., 2017), this toxin is present in the globally spread hypervirulent PCR ribotypes (RTs) 027 and 078.
The recent increase in community-acquired CDI affecting patients with no previous history of hospitalization discloses a shift in C. difficile epidemiology (Ofori et al., 2018) and unveils the urge to better understand the transmission dynamics taking place in the community, as well as identifying possible contamination sources and dissemination networks (De Roo and Regenbogen, 2020). Multiple studies have been published reporting the isolation of both toxigenic and non-toxigenic strains of C. difficile, similar to those found in humans. They include isolates from food-producing animal feces (Koene et al., 2012; Rodriguez et al., 2012, 2013; Schneeberg et al., 2013), from several foodstuff of animal origin, such as carcasses and abdominal viscera from abattoir samples (Rodriguez et al., 2013), retail meat products, seafood, fish and vegetable products (reviewed in Rodriguez-Palacios et al., 2020), and also from environmental samples (Janezic et al., 2016; Rodriguez et al., 2016). The extensive genetic overlap between strains was evidenced by the isolation of genetically related strains in animals and humans proving zoonotic transmission is possible (Knetsch et al., 2014, 2018; Werner et al., 2021). This is particularly notable in regard to the RT078, which is the most common RT isolated from pigs (Rodriguez et al., 2012; Stein et al., 2017), and also one of the most prevalent types found in hospitalized patients in Europe, with an increasing trend in prevalence (Bauer et al., 2011; Davies et al., 2016). In fact, whole-genome phylogenetic analysis reported by Knetsch et al. (2014) showing that pigs and farmers from the same farm were colonized with clonally related RT078 strains, support the possibility of interspecies transmission via fecal-oral route or a shared environmental source of infection. The RT078 belongs to clade 5, Multilocus Sequence Type (MLST) ST11, being highly divergent from other C. difficile clades, and comprising the RTs with the highest zoonotic potential which have recently emerged as common human pathogens (Knight et al., 2015). The close contact between humans and animals is enhanced in rural and farming environments, making it the ideal scenario to evaluate animal-human transmission.
Colonized animals and humans can shed oxygen resistant spores through the feces, allowing for an ubiquitarian presence of C. difficile in the environment. The ability of these spores to survive for a long period of time maintaining their infectious potential transforms contaminated soil and water into important reservoirs of public health concern (Kim et al., 1981; Al Saif and Brazier, 1996). The consumption of contaminated meat products, direct fecal-oral route (animal to person or person to person) and the ingestion of spores released into the environment are all possible transmission routes that need to be taken into account when assessing CDI risk factors (Janezic et al., 2016; Rodriguez Diaz et al., 2018). All these aspects make CDI a prime example of the interaction between animal, human and environmental health, disclosing the importance of the One Health approach when dealing with community circulating pathogens. Our research aimed at trying to establish a C. difficile transmission network involving all the One Health components using a pig farm as a proof-of-concept, as well as to assess the diversity of potential zoonotic types and to unveil genomic features of dominant clones.
Materials and Methods
Sampling Site Description and Sample Collection
To evaluate the transmission dynamics of C. difficile, samples from multiple environmental, human and animal compartments from a single farm were studied. The sampling site, a zootechnical station located in Santarém, Portugal, was selected based on the possibility of assessing the interaction between all One Health components (Figure 1). The animal research nature of the chosen zootechnical station allowed to obtain biological material from production animals in conditions mimicking intensive animal farming. Multiple units from the 250 hectares were covered in the sampling process. Each unit was distant from the others and the animals were separated by species with no possibility of interspecies interaction or fecal contamination. From the pig production unit, both fattening and reproduction sows were included, as well as the piglets from different maternities of the reproduction unit. All the waste products from the pig production unit converged to the same collection area from where the cesspool samples were taken. The resulting waste was then subjected to solid and liquid phase separation. The manure derived from the separation process was then used for the agricultural soil fertilization. The liquid part was diverted to a wastewater treatment plant from which samples were also collected. After the appropriate treatment, the water was discharged into the river. The other group of manure samples comprised manure samples from cattle and sheep that were separately collected.
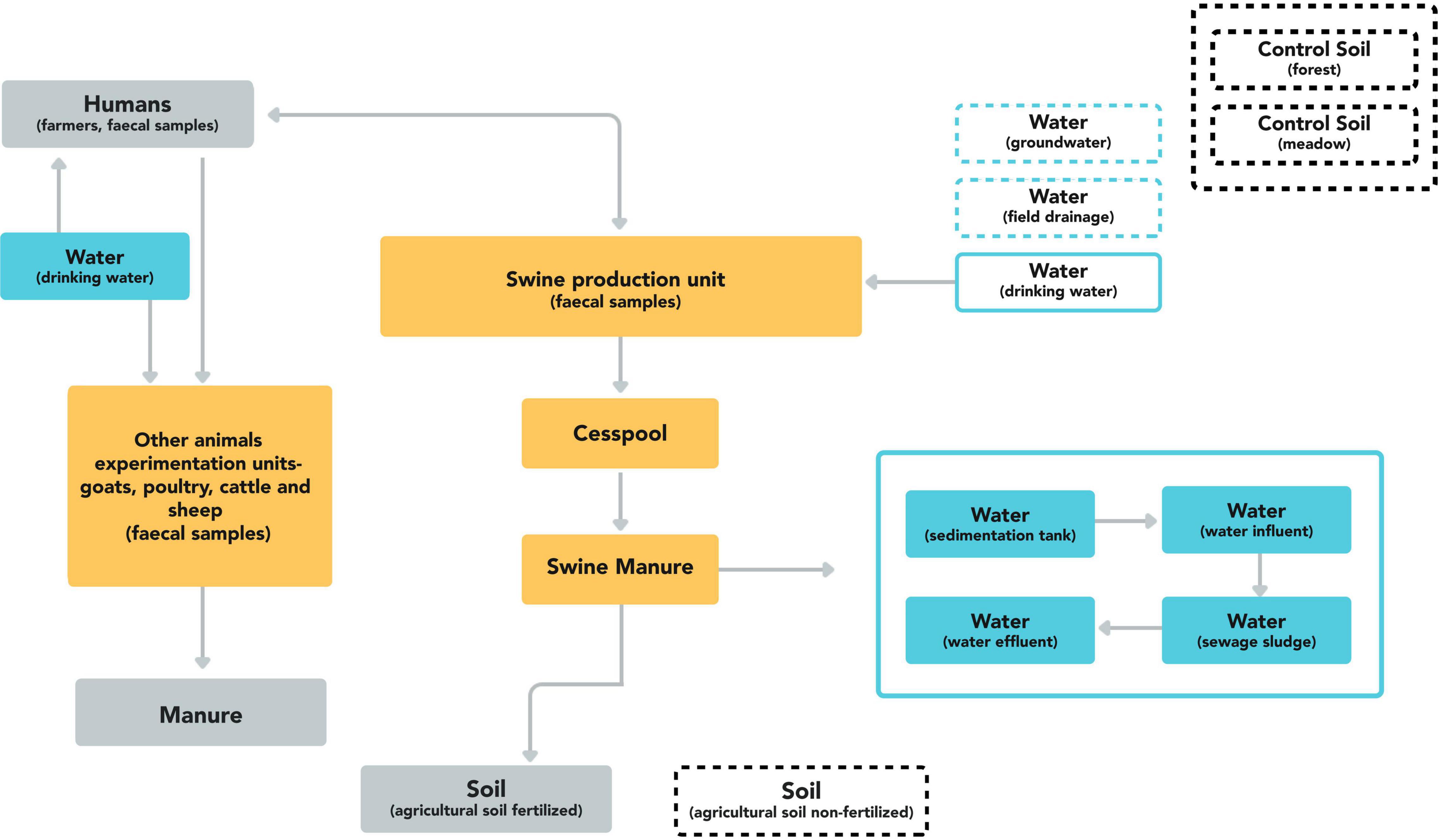
Figure 1. Schematic representation of the environmental, human, and animal compartments and the respective connection between them. The dashed line boxes indicate sampled compartments with no connection to the animal production units.
The sampling process took place between July 2020 and June 2021, at 2–3 time points. Environmental samples (n = 60) included agricultural soil samples, fertilized (n = 6) and non-fertilized (n = 9), non-agricultural soil, comprising the surrounding forest ground (n = 9) and the meadow (n = 9), swine manure (liquid and solid phases) (n = 6), pig production unit waste cesspools (n = 11) and manure from cattle (n = 2) and sheep (n = 1). The water samples were retrieved from the field drainage system, groundwater and drinking water, one of each (n = 3). Samples from the wastewater treatment plant (WTP) (n = 4) were also included, comprising one sample of each WTP influent, WTP effluent, WTP sedimentation tank and WTP sewage sludge. Animal fecal samples (n = 111) were taken from poultry (n = 9), sheep (n = 4), goats (n = 4), cattle (n = 8), and pigs, including fattening pigs (n = 18), reproduction sows (n = 25) and piglets (n = 43) from three different maternities of the reproduction unit. All animal stools were sampled from fresh droppings, except the piglets that were individually sampled by rectal swab. All samples were obtained with the farmers consent and collaboration following the animal wellbeing protocols in place in the animal production unit approved by the Portuguese Animal Welfare Authority (DGAV). Human fecal samples (n = 17) from healthy farmers and workers exposed to animal husbandry were also collected anonymously and voluntarily, the same individuals were sampled at two different times. None of the animals and humans included were showing gastrointestinal signs of disease at sampling time. Ethical approval for the study was obtained from the Health Ethics Commission from the National Institute of Health Dr Ricardo Jorge.
Isolation of Clostridioides difficile
Regarding the fecal and manure samples, 0.5 g of each were enriched in 5 ml of C. difficile enrichment broth (proteose peptone 40.0 g/L, disodium hydrogen phosphate 5.0 g/L, potassium dihydrogen phosphate 1.0 g/L, magnesium sulfate 0.1 g/L, sodium chloride 2.0 g/L, fructose 6.0 g/L, sodium taurocholate 1.0 g/L, D-cycloserine 0.25 g/L, Cefoxitin 0.008 g/L) for a week, under anaerobic conditions generated using the anaerobic cultivation system Anoxomat (Anoxomat, Mart, Netherlands), at 37°C, changing the atmosphere every 48 h. This step was followed by ethanol shock (2.5 ml of the enrichment mixture in 2.5 ml of 96–100% ethanol for 1 h at room temperature) and centrifugation (2300 RCF for 10 min) before inoculation of the pellet onto ChromID® C. difficile agar (bioMérieux, Marcy l’Etoile, France). The plates were incubated for 48 h under anaerobic conditions at 37°C. The rectal swabs were placed directly in 5 ml of C. difficile enrichment broth, and the following procedure was the same as described above.
The water samples were treated according to Janezic et al. (2016). Briefly, 50 ml of water was subjected to heat shock at 70°C for 20 min, followed by filtration through a 0.2 μm mixed cellulose ester membrane filter (Advantec®, California). Each filter was then enriched in 10 ml of C. difficile broth and incubated anaerobically at 37°C for 7 days, changing the atmosphere every 48 h. After enrichment, the filter was removed, and 5 ml of the mixture were centrifuged (2300 RCF for 10 min). The supernatant was removed and the pellet resuspended in 1 ml of 96–100% ethanol for 1 h before placing 2–3 drops on ChromID® C. difficile agar and incubated anaerobically for 48 h.
The soil samples were treated according to Janezic et al. (2016) with slight modifications. The samples were broth enriched (25 g soil in 90 ml broth) for a week under the previously described conditions, followed by centrifugation of 50 ml of the suspension. The resulting supernatant was then subjected to heat shock at 70°C for 20 min and the entire volume was then filtered through a 0.2 μm mixed cellulose ester membrane filter (Advantec®). The filters were directly placed on ChromID® C. difficile agar and incubated anaerobically at 37°C for 72 h. After incubation, between 2–5 presumptive colonies, were picked and cultured onto brucella blood agar with hemin and vitamin K1 (BBA) (BD BBL™, Heidelberg, Germany). The remaining colonies were swabbed from the filter, subjected to ethanol shock (1 ml 96100% ethanol for 1 h) and centrifuged (10500 RCF for 1 min). The pellet was then inoculated onto ChromID® C. difficile agar and incubated anaerobically for 48 h.
Toxins’ Profile, Ribotyping and Antimicrobial Susceptibility Testing
From the presumptively positive samples, based on colony morphologic characteristics, 3 to 5 colonies were picked, with exception of the piglets from which only 2 colonies were considered, and sub-cultured onto BBA under anaerobic conditions for 24 h at 37°C. Species confirmation was performed by MALDI-TOF (VITEK® MS, bioMérieux). For each confirmed C. difficile positive colony, genomic DNA was extracted using the Isolate II Genomic DNA kit (Bioline, London, United Kingdom), according to manufacturer’s instructions. C. difficile isolates were first characterized by multiplex PCR, targeting gluD and the tcdA, tcdB, cdtA and cdtB toxin genes, according to Persson et al. (2009). Then, isolates were subjected to PCR-ribotyping using Bidet primers followed by capillary gel-based electrophoresis, according to Fawley et al. (2015). Antimicrobial susceptibility to moxifloxacin (5 μg), vancomycin (5 μg), metronidazole (5 μg) and rifampicin (30 μg) was performed by disk diffusion (Oxoid™, Hampshire, United Kingdom), and by Etest® strips (bioMérieux) to clindamycin, using BBA and 24 h incubation under anaerobic conditions. C. difficile isolates were categorized as susceptible or resistant according to Erikstrup et al. (2012) for disk diffusion, and to the Clinical and Laboratory Standards Institute breakpoint for clindamycin (≥8 mg/L) [Clinical and Laboratory Standards Institute (CLSI), 2016].
Whole Genome Sequencing
A total of 26 C. difficile RT033 isolates (here designated as PT RT033) were selected (see results for details; Supplementary Table 1) for WGS. In short, after quantitation using Qubit Fluorometer (Thermo Fisher Scientific, Porto Salvo), high-quality DNA samples were subjected to dual-indexed Nextera XT Illumina library preparation, cluster generation and paired-end short-read high throughput sequencing (2 × 150 bp) on an Illumina NextSeq550 equipment (Ilumina, San Diego) available at the National Institute of Health (INSA), according to the manufacturer’s instructions.
Reads quality control, species confirmation and bacterial de novo assembly were performed using the INNUca v4.2.2 pipeline1 (Llarena et al., 2018). Briefly, after reads’ quality analysis (FastQC v0.11.52) and cleaning (Trimmomatic v0.38) (Bolger et al., 2014), genomes were assembled with SPAdes v3.14 (Bankevich et al., 2012) and subsequently improved using Pilon v1.23 (Walker et al., 2014). Species confirmation and contamination screening were assessed using Kraken v.2.0.7 (with 8GB database available at3) for both raw reads and final polished assemblies. MLST prediction was determined using mlst v2.18.1 software4. The 26 obtained draft genome sequences were annotated with RAST server v2.05 (Aziz et al., 2008).
In order to understand the phylogenetic positioning of PT RT033 isolates, other clade 5 ST11 isolates (n = 57) (Knight et al., 2019) belonging to multiple RTs from environmental, animal and human sources from different geographic regions (n = 57) were also selected (Supplementary Table 1), and their paired reads were downloaded from ENA and de novo assembled as described above. The genetic relatedness among isolates was evaluated by a reference-based mapping strategy using Snippy v.4.5.1 software6. Quality improved reads of all 83 isolates were individually mapped against the reference C. difficile RT033 DSM 101085 strain (GenBank accession number: CP021219.1). Single-nucleotide variant (SNV) calling was performed on variant sites that filled the following criteria: (i) minimum mapping quality and minimum base quality of 20; (ii) minimum number of reads covering the variant position ≥10; and (iii) minimum proportion of 90% of reads differing from the reference. Core-SNVs were extracted using Snippy’s core module (snippy-core), by masking repetitive regions, mobile genetic elements (MGE) [like transposons and prohages (Riedel et al., 2020)] and recombinant regions (like both the PaLoc region and the S-layer cassette) of C. difficile DSM 101085 [encompassing a total of ∼6.5% (∼260 kb) of the genome], as their inclusion would bias the phylogeny. All reported SNVs/indels were visually inspected and carefully confirmed using the Integrative Genomics Viewer v2.11.07 (Robinson et al., 2011). MEGA7 software8 (Kumar et al., 2016) was applied to calculate matrices of nucleotide distances and perform phylogenetic reconstructions over the obtained core-genome SNV alignment by using the Neighbor-Joining (NJ) method (Saitou and Nei, 1987) with the Maximum Composite Likelihood model to compute genetic distances (Tamura et al., 2004) and bootstrapping (1000 replicates) (Felsenstein, 1985).
Extended Genetic Diversity Analysis
In order to identify virulence and putative antimicrobial resistance (AMR) genetic determinants, PT genome assemblies were queried against the following publicly available databases, using ABRIcate v1.0.09 : CARD10, ResFinder11, ARG-ANNOT12 (Gupta et al., 2014), NCBI AMRFinderPlus13, MEGARES14, and VFDB15. The presence/absence and/or variability of each identified hit was confirmed by Snippy reference-based mapping, while BLASTp against the non-redundant (nr) protein sequences database was used to assess the potential protein function. The predicted AMR results were further compared with antimicrobial susceptibility testing results.
The genomic structure, organization and diversity of the PaLoc, binary toxin, skinCd element, S-layer cassette and accessory gene regulator (agr) regions on PT isolates was confirmed by Snippy reference-based mapping against C. difficile RT033 DSM 101085 or RT078 M120 (GenBank accession number: NC017174.1) strains and by RAST annotation. For comparative purposes, all the other clade 5 ST11 isolates (n = 57) were included in this analysis. Whenever it was possible, allele identification was conducted at the PubMLST platform (16 accessed in November 2021). Phylogeny of S-layer cassette was based on the concatenated sequences of splA, secA, cwp2, lmbE-like and cwp66 genes, with NJ tree being generated using MEGA7 as described above.
As a means to potentiate isolate discrimination, assemblies of PT RT033 isolates were analysed using PHASTER web server17 (Arndt et al., 2016) to determine the presence of prophages. Only hits with intact phages were considered for further analysis, given that the detection of phage fragments could result from the assembly process hampering the distinction between presence of intact phages and phage remnants that were excised during the evolutionary process (for which the biological importance is even more uncertain). All identified hits were manually curated and when possible closely defined by predicting their attachment sites. The presence of plasmids was assessed through PlasmidFinder 2.118, using default parameters with both trimmed reads and assemblies. All 83 ST-11 isolates were interrogated for the presence of C. difficile DSM 101085 plasmid (GenBank accession number: CP021320.1) by Snippy reference-based mapping.
The identification and structure of CRISPR-Cas systems (clustered regularly interspaced short palindromic repeats/CRISPR associated proteins) was predicted for all PT assembled genomes with CRISPRCasFinder19 web tool (accessed in September 2021). Only intact elements with the highest confidence score level were considered as legitimate hits. The occurrence and structure of each hit CRISPR-Cas system was confirmed by RAST annotation.
Data Availability
Raw sequencing reads of all 26 C. difficile PT RT033 isolates used in the present study were deposited in the European Nucleotide Archive (ENA) under the BioProject accession number PRJEB49792.
Results
Clostridioides difficile Distribution and Characteristics by Compartment
A total of 188 samples were included in this study (Table 1). The overall C. difficile positivity rate was 37.2% (70/188), and included samples from environmental (58.3%, 35/60) and animal (31.5%, 35/111) compartments. No C. difficile was found in any of the 17 human samples analysed, taken from the same farmers in two distinct time points.
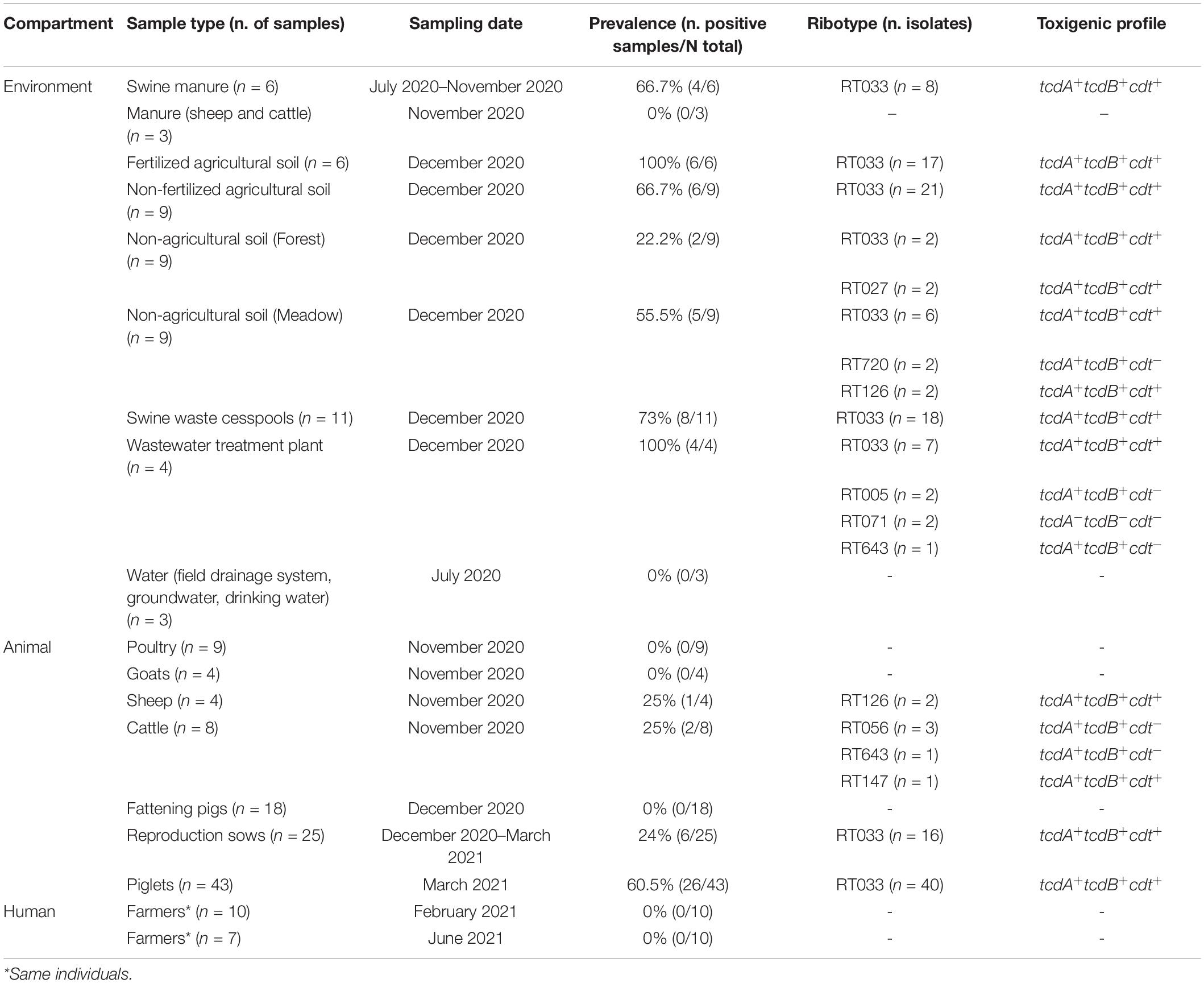
Table 1. Description of the samples analysed in the several compartments of the pig production unit and main findings.
Regarding environmental samples, four out of six (66.7%) swine manure samples were positive for C. difficile, while the three manure samples from cattle and sheep were negative. All the six fertilized agricultural soil samples were positive for C. difficile and the positivity rate for the non-fertilized agricultural soil was 66.7% (6/9). Concerning the non-agriculture soil samples, C. difficile was found in 22.2% (2/9) and 55.5% (5/9) of the forest and meadow samples, respectively. From the pig’s waste cesspools, eight of 11 (73%) samples were positive for C. difficile. The four water samples from the WTP were positive for C.diff, contrasting with the three water samples collected from other sources that were all negative (Table 1).
Among the 35 positive samples from the environment, a total of 90 C. difficile isolates belonging to different toxigenic RTs were obtained (Table 1): eight isolates from swine manure (all RT033, tcdA +, tcdB +, cdtA + /cdtB +); 17 from fertilized agricultural soil and 21 from non-fertilized agricultural soil (all RT033, tcdA +, tcdB +, cdtA + /cdtB +); four isolates from the forest ground (two RT027, tcdA +, tcdB +, cdtA + /cdtB +, two RT033, tcdA +, tcdB +, cdtA + /cdtB +); 10 from the meadow (six RT033, tcdA +, tcdB +, cdtA + /cdtB +, two RT720, tcdA +, tcdB +, cdtA-/cdtB-, and two RT126, tcdA +, tcdB +, cdtA + /cdtB +); 18 from the swine cesspool (all RT033, tcdA +, tcdB +, cdtA + /cdtB +); 12 isolates from the WTP (seven RT033, tcdA +, tcdB +, cdtA + /cdtB +, two RT005, tcdA +, tcdB +, cdtA-/cdtB-, two RT071, tcdA-, tcdB-, cdtA-/cdtB-, and one belonging to RT643, tcdA +, tcdB +, cdtA-/cdtB-) (Table 1). Regarding antimicrobial resistance, only the two RT027 isolates from forest were resistant to moxifloxacin.
While none of the samples from poultry (n = 9) or goats (n = 4) revealed the presence of C. difficile, this pathogen was found in sheep (1/4, 25%) and cattle (2/8, 25%) samples. The swine population included in this study represented the majority of the animal samples collected, totalizing 86 out of 111 samples. The positivity rate of C. difficile in pigs was assessed separately according to category and age group. None of the 18 samples from fattening pigs was positive for C. difficile, contrasting to a positivity rate of 24% (6/25) and 60.5% (26/43) detected in the reproduction sows and in the piglets’ group, respectively. Regarding the piglet population, three maternity units were evaluated, in which the piglets were separated by age groups. Two litters from each maternity unit were sampled. The presence of C. difficile was associated with the piglet’s age, being more frequent in the ones aged between 1–2 weeks (12/14, 85.7%), followed by the 3-weeks old (9/14, 64.3%) and less frequent in the older ones with 1-month of age (5/15, 33.3%).
Among the 35 positive animal samples, a total of 63 C. difficile isolates belonging to different toxigenic RTs were obtained (Table 1): two from sheep (all RT126, tcdA +, tcdB +, cdtA + /cdtB +), both presenting resistance to moxifloxacin; five from cattle (three RT056, tcdA +, tcdB +, cdtA-/cdtB-, one RT643, tcdA +, tcdB +, cdtA-/cdtB-, and one RT147, tcdA +, tcdB +, cdtA + /cdtB-); 16 from the reproduction sows (all RT033, tcdA +, tcdB +, cdtA + /cdtB +); 40 from piglets (all RT033, tcdA +, tcdB +, cdtA + /cdtB +).
Overall, all compartments connected to the swine production unit (pigs, swine cesspool/manure, agricultural soil and WTP) presented the highest C. difficile positivity rate, and RT033 was the predominant type found in those compartments. All the RT033 isolates were susceptible to the five antibiotics tested.
Genomic Characterization of the Predominant RT033
Considering the predominant distribution of RT033 (89.5%, 137/153) in all C. difficile positive compartments, a detailed genomic characterization of the isolates from this RT was undertaken. A group of 26 PT RT033 isolates representative of the different compartments (WTP, agricultural and control soils, manure, sows and piglets) was analysed (Supplementary Table 1).
As all PT RT033 isolates were expectably found to belong to clade 5 ST11, their phylogenetic positioning within the major RT sublineages (RT078, RT126, RT127, RT033, and RT288) of clade 5 ST11 (Knight et al., 2019) was first investigated. The core-genome SNV-based phylogenetic analysis shows a clear segregation of strains into distinct clusters (I-VI), with all PT RT033 isolates grouping together in an independent tree branch (III) (Figure 2A). Indeed, despite they were isolated from distinct compartments, PT RT033 isolates were found to be highly genetically related among them, differing only by a mean of 0.1 ± 0.1 core-SNVs. On the other hand, they exhibited a mean distance that ranged from 235.0 ± 13.1 to 346.8 ± 14.4 core-SNVs to clusters II (RT127 cluster I) and V (RT078/RT126 cluster), respectively (Figure 2B). Surprisingly, when compared to the classical RT033 cluster (I), whose isolates displayed a mean of 8.0 ± 1.2 core-SNVs among them, the PT RT033 cluster distant a mean of 290.5 ± 14.1 core-SNVs. Overall, these results point for a clonal origin of PT RT033 isolates, clearly distinct from the remaining clade 5 ST11 sublineages, including the classical RT033 cluster.
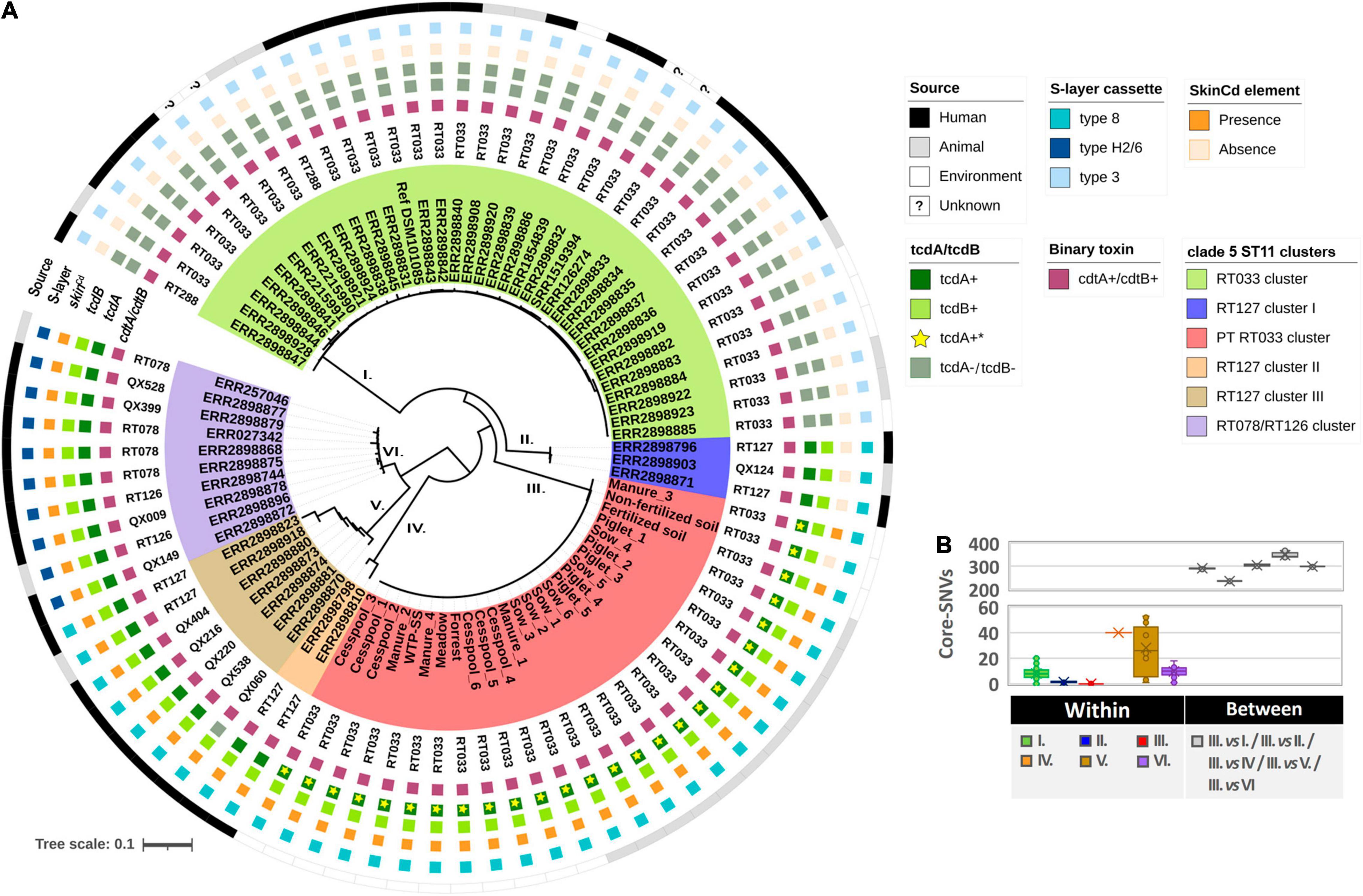
Figure 2. Phylogenetic positioning of PT RT033 clone within in the Clostridioides difficile clade 5 ST11 lineage. (A) The core-genome SNP-based phylogenetic tree was reconstructed using the Neighbor-Joining method (Saitou and Nei, 1987) with the Maximum Composite Likelihood model (Tamura et al., 2004). For all isolates (n = 84), the clade 5 ST11 sub-lineage (color-coded) is shown as well as the ribotype, the source, the S-layer cassette type, the skinCd element, the tcdA/tcdB and binary toxins. The tree was drawn using the iTOL website (https://itol.embl.de/) (Letunic and Bork, 2021). (B) Box Plots depicting the number of SNVs observed within and between all clade ST11 clusters. The horizontal line within each box marks the median, while the cross (“x”) represents the medium value.
A deeper genomic inspection of the PT RT033 cluster allowed to pinpoint several genomic features that reinforce the distance observed to the classical RT033 cluster. One of the major discrepancies was found in the PaLoc (Figure 3) since RT033 has been traditionally considered as non-toxigenic due to the insertion of a 10.4 kb Tn6218-like element that has resulted in a deletion of most genes involved in toxins’ production, regulation and secretion facilitation, leaving only a truncated tcdA pseudogene and a disrupted tcdC (Elliott et al., 2014). In contrast, the PT RT033 clone exhibit a large genomic region (∼58 kb) that include an 18.5 kb complete PaLoc as well as its immediately upstream region, with a genetic organization similar to the remaining clade 5 ST11 sublineages. Indeed, all tcdR, tcdB, tcdE, tcdL, and tcdA PaLoc genes, as well as the disrupted tcdC typically present in clade 5 sublineages, were found in PT RT033. However, some discrepancies were observed that make cluster’s PT RT033 PaLoc unique. Indeed, when compared with RT078 reference M120 strain (NC017174.1), both tcdR and tcdB displayed a missense substitution (533A > G| Tyr178Cys and 6658G > A| Asp22220Asn, respectively), while tcdA is truncated in two smaller ORFs of 2982 bp and 4938 bp due to a C→T alteration at position 2980, resulting in a prematurely gained stop codon (at 994aa). Moreover, three additional missense alterations (5333T > A| Met1778Lys; 5710A > G| Arg1901Gly and 5997T > C| Asp1999Asp) were found in the major tcdA ORF (Figure 3).
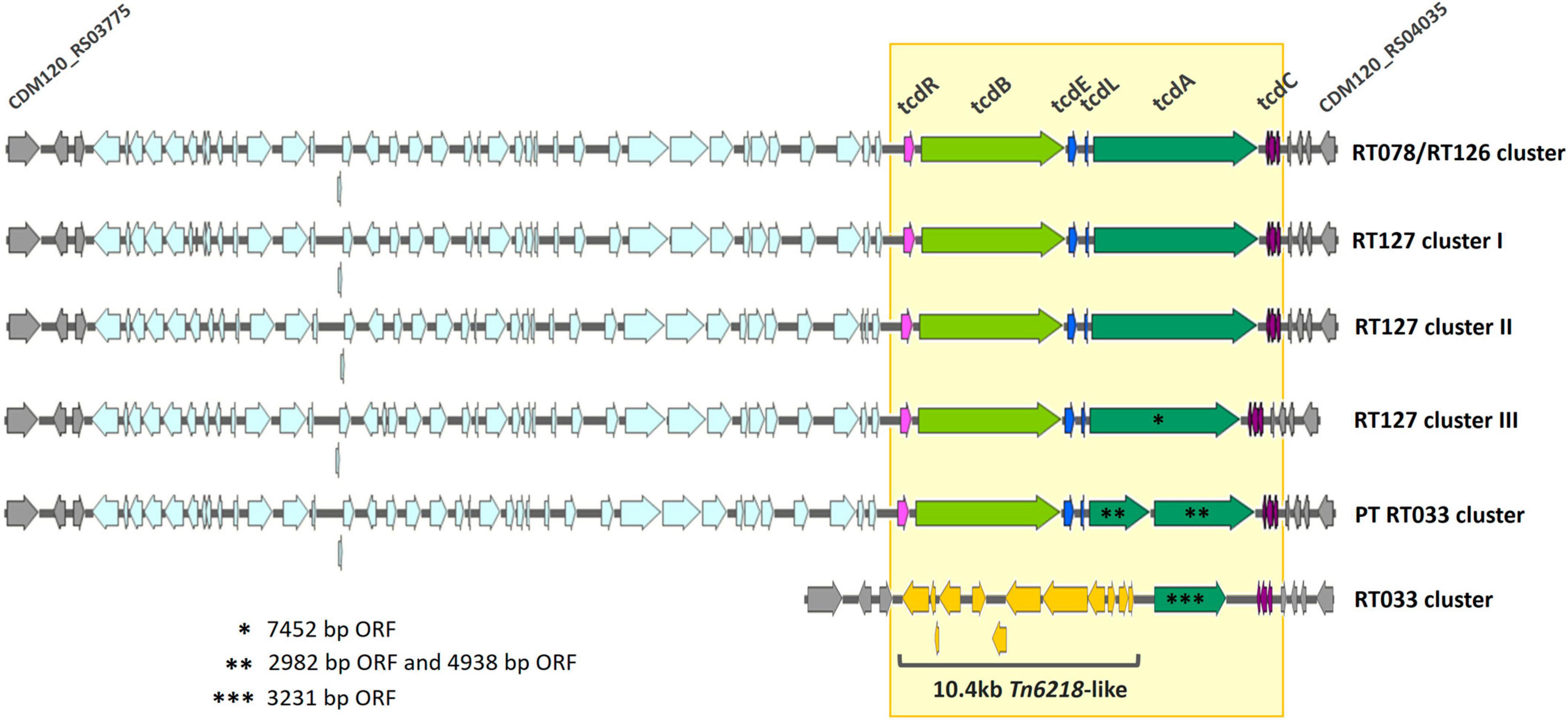
Figure 3. PaLoc genetic diversity within the Clostridioides difficile clade 5 ST11 lineage. For all clusters, ORFs orientation is depicted by arrows, while PaLoc region is highlighted by a yellow box with tcdR, tcdB, tcdE, tcdL and tcdA genes differently colored. The Tn6218-like element, exclusively found in the classic RT033 cluster, is shown in yellow arrows. Flanking genes (homologous to all clusters) of the PaLoc-containing region are represented by gray arrows, where extremes designated according to RT078 reference M120 genome (NC017174.1).
Likewise, disparities were also found regarding the binary toxin, with the PT RT033 clone harboring a novel cdtA synonymous variant (936T > G) and a wild-type cdtB gene (allele 21) identical to all ST11 toxigenic clusters (except for RT127 cluster II, allele 48), differing from the classical RT033 cdtB by a synonymous substitution (allele 14). Regarding cdtR, PT RT033 isolates were found to harbor the wild-type 747 bp allele characteristically exhibited by non-RT078/RT126 strains.
The S-layer cassette diversity was also evaluated through phylogenetic characterization of concatenated slpA, secA, cwp2, lmbE-like and cwp66 gene sequences (totalizing ∼10 kb) (Supplementary Figure 1), as they were shown to be the most polymorphic within this region (Dingle et al., 2013). All PT RT033 isolates were clearly segregated within the RT127 cluster branch tree, for which S-layer cassette was classified as type 8 (Knight et al., 2019). Interestingly, they are distanced by 1807.8 ± 29.1 and 1342.6 ± 31.6 nucleotides from the classical RT033 cluster (S-layer type 3) and RT078/RT126 cluster (S-layer type H2/6), respectively.
Moreover, in PT RT033 isolates, the sporulation-specific gene sigK is interrupted by a 10 kb phage-like skinCd element with similar gene content and structure to that seen in RT078/RT126 cluster and RT127 clusters II and III (Figure 4). This element is composed of twelve ORFs, including its site-specific recombinase [required for excision in the mother cell during sporulation (Haraldsen and Sonenshein, 2003)] and of the vanZ1 gene [shown to confer low-level of resistance to the glycopeptide antibiotic teicoplanin (Woods et al., 2018)], as well as a CRISPR element without cas-associated genes. The only exception occurred for the PT RT033 non-fertilized soil isolate that possesses an intact sigk like both the classical RT033 cluster and the RT127 cluster I.
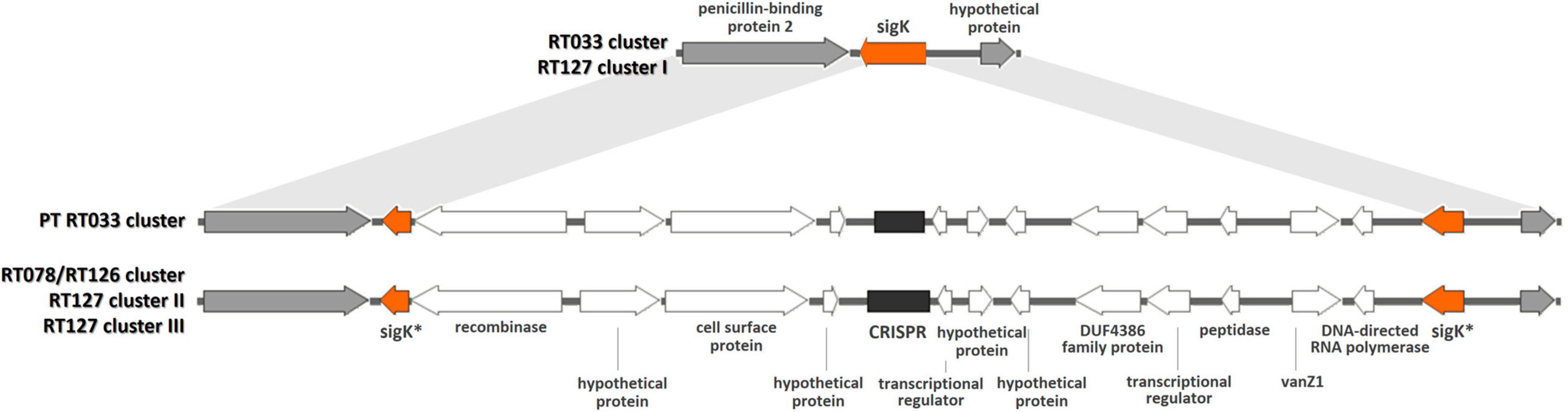
Figure 4. Genetic structure of the skinCd element of Clostridioides difficile PT RT033 clone. For all clade 5 ST11 clusters, ORFs orientation is depicted by arrows. Intact or interrupted (*) sigK genes are represented by orange arrows, while homologous flanking genes are shown in gray arrows. All 12 ORFs that compose skinCd element are represented by white arrows, and CRISPR element is depicted as a black box.
Regarding the presence of other MGEs, no plasmid was found in any PT RT033 draft genomes, including that present in the reference RT033 DSM101085 strain (CP021320.1). On the other hand, genome inspection of all 26 PT RT033 isolates revealed the presence of prophage regions with homology to four known Clostridium phages. Similarly to the reference RT033 DSM101085 strain (NZ_CP021329.1), all PT RT033 isolates were found to carry two distinct intact prophages, namely: (i) an ∼48–58 kb phiCDHM19-like prophage containing 76–83 ORFs and a predicted CRISPR array without cas genes, and (ii) an ∼46 kb phiMM03-like or phiMMP01-like prophage with 76 ORFs. The only exception was the Manure_3 isolate, for which a phiCP340-like prophage was additionally identified. However, no association was found between the presence or combination of phages and the different compartments from which samples were collected. There is, however, a difference concerning the insertion sites of the phiCDHM19-like prophage, which is inserted between ORFs CDIF101085_01985 (c-di-GMP-I riboswitch) and CDIF101085_02107 (hypothetical protein) for reference RT033, whereas it interrupts the homolog CDIF101085_00958 (∼1143 bp) that codes for an aldo/keto reductase in PT isolates, resulting in a smaller ORF (∼552 bp). On the other hand, PT RT033 isolates share the same insertion site as reference RT033 for phiMM03-like prophage, which disrupts an ABC transporter coding gene (CDIF101085_03033). The only exception occurred for Manure_2 isolate, in which a phiCDHM19-like prophage was found at this location instead of a phiMM03-like prophage (i.e., interrupting the CDIF101085_03033 homolog). Interestingly, both phiMM03- and phiMM01-like prophages were found to carry an additional agr1-like locus containing only agrB and agrD genes, which contrasts to the agr3 locus displayed by the classical RT033 cluster that also contains the agrC gene in phiMM03 phage (Riedel et al., 2020).
Finally, no AMR genetic determinants were found in silico for the 26 PT RT033 isolates, supporting and complementing the obtained phenotypic results.
Discussion
This study aimed firstly at evaluating the transmission dynamics of C. difficile focusing on the role played by environmental reservoirs, using a model centered on a pig farm. The overall positivity rate of 37.2% observed in this study is in agreement with other studies conducted in swine farms (Andrés-Lasheras et al., 2017; Krutova et al., 2018) and suggests a key role of animal production units in the CDI panorama, acting as possible reservoirs of toxigenic strains. The prevalence of C. difficile was found to be almost 2-fold higher in environmental samples (58.3%) than in animal samples (31.5%). The fact that most environmental samples included in this study were related to the pig production units may have accounted for the prevalence noted, since the isolation rate was higher in pigs, especially in piglets, than in other species, suggesting a transmission cycle involving these animals. The role of the environment in the transmission chain has been reported before (Al Saif and Brazier, 1996; Janezic et al., 2016), particularly the piggery enclosure with a study by O’Shaughnessy et al. (2019) also reporting a prevalence above 50%. Worth noting is the fact that all samples from the WTP and fertilized agricultural soil (a total of 10 samples), which were directly connected to the swine manure, were positive for C. difficile. This animal production by-product is integrated back into the environment, used for agricultural soil fertilization or released into water bodies, contributing to the environmental dissemination of toxigenic strains (Al Saif and Brazier, 1996; Le Maréchal et al., 2020; Lim et al., 2020).
Regarding animal samples, the positivity rate was around 25% in cattle, sheep and pigs, the exception being the piglet group from which 60.5% were positive. This higher prevalence might be attributed to microbiota immaturity which facilitates C. difficile overgrowth and isolation (Grześkowiak et al., 2019). When looking into the piglet population it is notable that the prevalence decreased with age, with a positivity rate of 85.7% in the 1–2 weeks old group, 64.3% at 3-weeks old and decreasing to 33.3% in 1 month old animals. Together with previous studies (Weese et al., 2010; Susick et al., 2012), our results corroborate the importance of intestinal flora maturity in CDI control. Considering the high prevalence of C. difficile found in the environment, it is likely that piglet colonization arises from suckling on contaminated teats or from direct fecal-oral route (Hopman et al., 2011).
Even though the humans were selected based on animal proximity, none of their fecal samples was positive for C. difficile. The fact that these were all healthy adults with no history of recent antimicrobial administration may have accounted for the negative results. It is also possible that the handling and hygiene practices adopted in the zootechnical station are effective in preventing workers from becoming colonized by the circulating pathogens. Nevertheless, the high prevalence of C. difficle isolated from the environment reveals the importance it may hold as a plausible reservoir of toxigenic strains that can potentially be the source of human community acquired CDI.
Despite the clear predominance of RT033, showing a prevalence of 89.5%, a high diversity of toxigenic RTs was found. The inclusion of different animal species as well as different environmental compartments justifies the diversity found and is supported by other farm related studies (Andrés-Lasheras et al., 2017; Krijger et al., 2019). Some of the remaining isolates observed in this study also belonged to RTs with zoonotic potential for which symptomatic infections are being increasingly reported in hospitalized patients, such as the case of RT126 [(Davies et al., 2016) and data from the laboratory-based epidemiological surveillance of CDI in Portugal, Portuguese National Institute of Health]. The resistance to moxifloxacin found in RT027 from the forest soil and RT126 from a sheep deserves special attention since fluoroquinolone resistance is mainly found in epidemic strains, particularly RT027 responsible for several outbreaks of nosocomial nature (Clements et al., 2010).
The PT RT033 isolates were found in all compartments connected to the pig production unit. The high genetic relatedness found among isolates (0.1 ± 0.1 core-SNVs) (Figure 2B) supports a clonal transmission between animal and environmental compartments, as a threshold of ≤2 SNV in the core genome is usually accepted to define clonally related strains (Bletz et al., 2018). Considering the almost ubiquitous presence of the isolated clone in the environmental compartments, especially in the ones linked to the swine production unit, the introduction of new animals that rapidly become colonized and continue to contribute to spore dissemination, allows for the maintenance of the farm transmission dynamic.
Most notably, the PT RT033 clone was clustered separately from the previously described RT033 highly suggesting a different evolutionary path (Figure 2A). One of the most significant disparities was found for the PaLoc with the PT RT033 clone displaying a complete locus similar to that harbored by other clade 5 ST11 toxigenic strains (Figure 3), where all the toxin and regulatory genes (tcdR, tcdB, tcdE, tcdL, and tcdA) are present, including an aberrant tcdC typical of some clade 5 sublineages. Nevertheless, some unique features like the truncation of the tcdA gene into two smaller ORFs and a few missense nucleotide substitutions, make this a distinctive PaLoc genotype not previously described. To our knowledge this is the first report of a toxigenic RT033 strain, contributing to the overall PaLoc diversity. The PaLoc loss in the classical RT033 is considered a fairly recent evolutionary event resulting from the acquisition of the Tn6218 mobile element by a previously toxigenic ancestral (Elliott et al., 2014). Altogether, considering that PT RT033 harbors a complete PaLoc and it belongs to a distinct genetic cluster, these findings suggest that it might be a more ancestral RT033 clone that underwent a separate evolution route which did not include the acquisition of the mobile element responsible for the typical deletion in the PaLoc.
Further phenotypical characterization is needed to evaluate Toxin A expression and cytotoxic effect. The role played by the binary toxin in CDI pathogenesis is still under debate but it is generally assumed as a virulence increasing factor that works synergistically with toxins A and B contributing to poorer prognosis (Gerding et al., 2013). Nonetheless, strains that exclusively produce the binary toxin are still considered as disease-causing agents (Riedel et al., 2020). Therefore, it is highly expected that the PT RT033 clone holds an increased pathogenic potential as compared the classic RT033 strains.
Regarding the S-layer cassette composition, all PT RT033 isolates, except one, were classified as having a type 8 S-layer similar to the one found in the RT127 cluster. These genetic elements behave independently from the genome and suffer a much higher recombination rate via homologous recombination. This phenomenon of S-layer cassette switching, occurring independently from the strains genotype, contrasts with the low mutation rate found within each cassette (Supplementary Figure 1), with very little diversity being reported between isolates with the same variant (Dingle et al., 2013). It is possible that PT RT033 clone acquired its S-layer cassette from other RT before establishing its dominance in the farm, decreasing the chances of further recombination events that would result in a higher cassette diversity. Considering the cell surface is closely related to the bacterial antigenic properties it is likely that the PT RT033 triggers a different antigenic response than the classic RT033 (Dingle et al., 2013). Given the role of the outer membrane in the host-pathogen interaction (Steinberg and Snitkin, 2020) it can also be speculated that the PT RT033 clone, having a S-layer cassette closer to the ones found in RTs more commonly found in pigs (e.g., RT078/RT126), may hold a similar host tropism.
Clostridioides difficile spore formation is a complex process that depends on many regulatory genes, one of which is the sigK gene. This sigma factor plays an essential role in the late stage of the spore formation chain of events, being responsible for endospore coat and exospore synthesis as well as being indispensable to the release of spores into the environment (Saujet et al., 2014). Unlike the classic RT033 strains, the presently described PT RT033 clone harbors a phage-like skinCd element that interrupts the sigK gene (Figure 4). The skinCd excision is needed for sigK and late sporulation related genes to be expressed. Previous studies suggest that skinCd– and skinCd + C. difficile strains display different sporulation properties, the latter presenting an increased sporulation efficiency (Haraldsen and Sonenshein, 2003). The presence of this phage-like element in the PT RT033 isolates may translate into an increased sporulation fitness when compared to the typical RT033, which can be partially responsible for the maintenance of the transmission network observed in the farm.
Regarding other MGE, the presence of phiMM01 phage in PT RT033 is noteworthy since it has not been described to date in this RT, with previous studies reporting its presence in other ST11 RTs (e.g., RT126 and RT127) (Knight et al., 2019). C. difficile prophages have been proven to influence gene expression, including virulence factors and antimicrobial resistance genes transduction (Brouwer et al., 2013; Wasels et al., 2014), and a more in-depth analysis of the genetic influence this phage may have in the newly described clone is worth exploring. In addition, PT RT033 shares the phiCDHM19 and phiMM03 prophages with the classical RT033, although the insertion site for the phiCDHM19 prophage is different, interrupting an aldo/keto reductase gene. This protein belongs to a superfamily of enzymes which functions are related to the reduction of aldehydes and ketones. Microorganisms capable of producing these enzymes hold a selective advantage, as these are mutagenic compounds that can be found in the environment and can represent a danger to microbial cells. Although it is important to explore if the insertion of a prophage in this ORF has any influence in AKR gene expression, it is plausible that, even if this ORF becomes dysfunctional by such event, other enzymes partially support the reaction resulting in no significant disadvantage for the cell (Ellis, 2002).
Regarding agr loci, the classical RT033 isolates harbor three agr loci, the typical agr1, one agr1-like, and an agr3, the last one located in phiMMP03 prophage (Riedel et al., 2020). However, the PT RT033 isolates, besides the two agr1 loci, harbors a third agr1-like locus located in either phiMM03 or phiMM01 prophages. To our knowledge, this is the first report of an agr1-like locus in an MGE. Considering the role agr genes pose in contributing to the virulence and colonization factors by quorum sensing regulation (Darkoh et al., 2017), the presence of such element in a prophage that can be subjected to horizontal gene transfer, may influence the behavior of the bacterial community considered in this study (Hargreaves et al., 2014; Okada et al., 2020). Further studies are still needed to uncover the role of this third agr1-like locus in the PT RT033 clone, but it is possible that it may influence biofilm production through quorum sensing regulation contributing to bacterial persistence in the environment, thus allowing for the maintenance of the transmission cycle.
Finally, no AMR determinants were found in any of the PT RT033 isolates, which may be due to the experimental nature of the zootechnical station, where animal exposure to antibiotics is much lower than in intensive animal farming. The low antimicrobial pressure may have allowed the clone to establish well in the ecosystem, not being overthrown by more resistance strains that would be expected to thrive in a more antibiotic saturated environment.
Conclusion
The present study comes as a valuable contribution to the overall knowledge on the diversity of the clade 5 ST11, shedding light on the evolutionary path, not only of this clade but also of the C. difficile as a species. The PT RT033 shows a unique combination of genetic features found in other clade 5 RTs but never before reported in RT033. Some of the newly described characteristics are closely related with sporulation properties, biofilm production and outer membrane properties, and could possibly be accountable for the generalized spread and environmental maintenance of this clone in the studied animal production unit. Considering this is the first report of a toxigenic RT033 strain, its pathogenic potential cannot be ignored. Still, further studies are still needed in order to understand the functionality of the PT RT033 clone PaLoc and its zoonotic potential. Given the close animal-human interaction taking place in animal production units, an interspecies leap can occur and human infections with circulating clones may arise as a consequence of environmental or fecal-oral contamination.
Data Availability Statement
The datasets presented in this study can be found in online repositories. The names of the repository/repositories and accession number(s) can be found in the article/Supplementary Material.
Ethics Statement
The studies involving human participants were reviewed and approved by Comissão de Ética do Instituto Nacional de Saúde Doutor Ricardo Jorge, I. P. The patients/participants provided their written informed consent to participate in this study.
Author Contributions
FA, RC, and MO conceptualized the study. FA, AN, RC, AS, OM, RM, JR, LS, JG, and MO contributed to the methodology. FA, AN, and MO worked on the original draft of the manuscript. FA, AN, LS, JG, and MO reviewed and edited the manuscript. All authors have read and agreed to the published version of the manuscript.
Funding
This work was supported by funding from the European Union’s Horizon 2020 Research and Innovation programme under grant agreement No. 773830: One Health European Joint Programme. FA and RC were recipients of fellowships from the same programme.
Conflict of Interest
The authors declare that the research was conducted in the absence of any commercial or financial relationships that could be construed as a potential conflict of interest.
Publisher’s Note
All claims expressed in this article are solely those of the authors and do not necessarily represent those of their affiliated organizations, or those of the publisher, the editors and the reviewers. Any product that may be evaluated in this article, or claim that may be made by its manufacturer, is not guaranteed or endorsed by the publisher.
Acknowledgments
WGS was performed at Unit of Innovation and Technology, Human genetic Department, National Institute of Health Dr. Ricardo Jorge, Lisbon, Portugal.
Supplementary Material
The Supplementary Material for this article can be found online at: https://www.frontiersin.org/articles/10.3389/fmicb.2022.858310/full#supplementary-material
Footnotes
- ^ https://github.com/B-UMMI/INNUca
- ^ http://www.bioinformatics.babraham.ac.uk/projects/fastqc/
- ^ https://ccb.jhu.edu/software/kraken/
- ^ https://github.com/tseemann/mlst
- ^ http://rast.nmpdr.org/
- ^ https://github.com/tseemann/snippy
- ^ http://software.broadinstitute.org/software/igv/
- ^ http://www.megasoftware.net
- ^ https://github.com/tseemann/abricate
- ^ https://card.mcmaster.ca/
- ^ https://cge.cbs.dtu.dk/services/ResFinder/
- ^ https://www.mediterranee-infection.com/acces-ressources/base-de-donnees/arg-annot-2/
- ^ http://www.ncbi.nlm.nih.gov/pathogens/antimicrobial-resistance/AMRFinder/
- ^ https://megares.meglab.org/
- ^ http://www.mgc.ac.cn/VFs/
- ^ http://pubmlst.org/
- ^ https://phaster.ca/
- ^ http://cge.cbs.dtu.dk/services/PlasmidFinder/
- ^ http://crisprcas.i2bc.paris-saclay.fr/
References
Al Saif, N., and Brazier, J. S. (1996). The distribution of Clostridium difficile in the environment of South Wales. J. Med. Microbiol. 45, 133–137. doi: 10.1099/00222615-45-2-133
Andrés-Lasheras, S., Bolea, R., Mainar-Jaime, R. C., Kuijper, E., Sevilla, E., Martín-Burriel, I., et al. (2017). Presence of Clostridium difficile in pig faecal samples and wild animal species associated with pig farms. J. Appl. Microbiol. 122, 462–472. doi: 10.1111/jam.13343
Arndt, D., Grant, J. R., Marcu, A., Sajed, T., Pon, A., Liang, Y., et al. (2016). PHASTER: a better, faster version of the PHAST phage search tool. Nucleic Acids Res. 44, W16–W21. doi: 10.1093/nar/gkw387
Aziz, R. K., Bartels, D., Best, A., DeJongh, M., Disz, T., Edwards, R. A., et al. (2008). The RAST Server: rapid annotations using subsystems technology. BMC Genomics 9:75. doi: 10.1186/1471-2164-9-75
Bankevich, A., Nurk, S., Antipov, D., Gurevich, A. A., Dvorkin, M., Kulikov, A. S., et al. (2012). SPAdes: a new genome assembly algorithm and its applications to single-cell sequencing. J. Comput. Biol. 19, 455–477. doi: 10.1089/cmb.2012.0021
Bauer, M. P., Notermans, D. W., Van Benthem, B. H. B., Brazier, J. S., Wilcox, M. H., Rupnik, M., et al. (2011). Clostridium diffi cile infection in Europe : a hospital-based survey. Lancet 377, 63–73. doi: 10.1016/S0140-6736(10)61266-4
Berry, C. E., Davies, K. A., Owens, D. W., and Wilcox, M. H. (2017). Is there a relationship between the presence of the binary toxin genes in Clostridium difficile strains and the severity of C. difficile infection (CDI)? Eur. J. Clin. Microbiol. Infect. Dis. 36, 2405–2415. doi: 10.1007/s10096-017-3075-8
Bletz, S., Janezic, S., Harmsen, D., Rupnik, M., and Mellmann, A. (2018). Defining and evaluating a core genome multilocus sequence typing scheme for genome-Wide typing of clostridium difficile. J. Clin. Microbiol. 56:e01987-17. doi: 10.1128/JCM.01987-17
Bolger, A. M., Lohse, M., and Usadel, B. (2014). Trimmomatic: a flexible trimmer for Illumina sequence data. Bioinformatics 30, 2114–2120. doi: 10.1093/bioinformatics/btu170
Brouwer, M. S. M., Roberts, A. P., Hussain, H., Williams, R. J., Allan, E., and Mullany, P. (2013). Horizontal gene transfer converts non-toxigenic Clostridium difficile strains into toxin producers. Nat. Commun. 4:2601. doi: 10.1038/ncomms3601
Brown, K. A., Khanafer, N., Daneman, N., and Fisman, D. N. (2013). Meta-analysis of antibiotics and the risk of community-associated Clostridium difficile infection. Antimicrob. Agents Chemother. 57, 2326–2332. doi: 10.1128/AAC.02176-12
Buffie, C. G., Jarchum, I., Equinda, M., Lipuma, L., Gobourne, A., Viale, A., et al. (2012). Profound alterations of intestinal microbiota following a single dose of clindamycin results in sustained susceptibility to Clostridium difficile-induced colitis. Infect. Immun. 80, 62–73. doi: 10.1128/IAI.05496-11
Chandrasekaran, R., and Lacy, D. B. (2017). The role of toxins in clostridium difficile infection. FEMS Microbiol. Rev. 41, 723–750. doi: 10.1093/femsre/fux048
Clements, A. C., Magalhães, R. J. S., Tatem, A. J., Paterson, D. L., and Riley, T. V. (2010). Clostridium difficile PCR ribotype 027: assessing the risks of further worldwide spread. Lancet Infect. Dis. 10, 395–404. doi: 10.1016/S1473-3099(10)70080-3
Clinical and Laboratory Standards Institute (CLSI) (2016). Subcommittee on Antimicrobial Susceptibility Testing. Philadelphia: CLSI. Available online at: https://clsi.org/media/1700/clsi-newswinter-2016.pdf
Darkoh, C., Odo, C., and DuPont, H. L. (2017). Erratum for Darkoh, et al., “Accessory Gene Regulator-1 Locus Is Essential for Virulence and Pathogenesis of Clostridium difficile.”. MBio 8:e01643-17. doi: 10.1128/mBio.01643-17
Davies, K. A., Ashwin, H., Longshaw, C. M., Burns, D. A., Davis, G. L., and Wilcox, M. H. (2016). Diversity of clostridium difficile PCR ribotypes in europe: results from the European, multicentre, prospective, biannual, point-prevalence study of clostridium difficile infection in hospitalised patients with diarrhoea (EUCLID), 2012 and 2013. Eurosurveillance 21:30294. doi: 10.2807/1560-7917.ES.2016.21.29.30294
Dingle, K. E., Didelot, X., Ansari, M. A., Eyre, D. W., Vaughan, A., Griffiths, D., et al. (2013). Recombinational switching of the Clostridium difficile S-layer and a novel glycosylation gene cluster revealed by large-scale whole-genome sequencing. J. Infect. Dis. 207, 675–686. doi: 10.1093/infdis/jis734
Elliott, B., Dingle, K. E., Didelot, X., Crook, D. W., and Riley, T. V. (2014). The complexity and diversity of the pathogenicity locus in Clostridium difficile clade 5. Genome Biol. Evol. 6, 3159–3170. doi: 10.1093/gbe/evu248
Ellis, E. M. (2002). Microbial aldo-keto reductases. FEMS Microbiol. Lett. 216, 123–131. doi: 10.1016/S0378-1097(02)01014-5
Erikstrup, L. T., Danielsen, T. K. L., Hall, V., Olsen, K. E. P., Kristensen, B., Kahlmeter, G., et al. (2012). Antimicrobial susceptibility testing of Clostridium difficile using EUCAST epidemiological cut-off values and disk diffusion correlates. Clin. Microbiol. Infect. 18, E266–E272. doi: 10.1111/j.1469-0691.2012.03907.x
Fawley, W. N., Knetsch, C. W., MacCannell, D. R., Harmanus, C., Du, T., Mulvey, M. R., et al. (2015). Development and validation of an internationally-standardized, high-resolution capillary gel-based electrophoresis PCR-ribotyping protocol for Clostridium difficile. PLoS One 10:e0118150. doi: 10.1371/journal.pone.0118150
Felsenstein, J. (1985). Confidence Limits on Phylogenies: an Approach Using the Bootstrap. Evolution 39, 783–791. doi: 10.1111/j.1558-5646.1985.tb00420.x
Francis, M. B., Allen, C. A., Shrestha, R., and Sorg, J. A. (2013). Bile Acid Recognition by the Clostridium difficile Germinant Receptor, CspC, Is Important for Establishing Infection. PLoS Pathog. 9:e1003356. doi: 10.1371/journal.ppat.1003356
Gerding, D. N., Johnson, S., Rupnik, M., and Aktories, K. (2013). Clostridium difficile binary toxin CDT: mechanism, epidemiology, and potential clinical importance. Gut Microbes 5, 15–27. doi: 10.4161/gmic.26854
Giel, J. L., Sorg, J. A., Sonenshein, A. L., and Zhu, J. (2010). Metabolism of bile salts in mice influences spore germination in clostridium difficile. PLoS One 5:e8740. doi: 10.1371/journal.pone.0008740
Grześkowiak, Ł, Dadi, T. H., Zentek, J., and Vahjen, W. (2019). Developing gut microbiota exerts colonisation resistance to clostridium (Syn. clostridioides) difficile in piglets. Microorganisms 7:218. doi: 10.3390/microorganisms7080218
Gupta, S. K., Padmanabhan, B. R., Diene, S. M., Lopez-Rojas, R., Kempf, M., Landraud, L., et al. (2014). ARG-annot, a new bioinformatic tool to discover antibiotic resistance genes in bacterial genomes. Antimicrob. Agents Chemother. 58, 212–220. doi: 10.1128/AAC.01310-13
Haraldsen, J. D., and Sonenshein, A. L. (2003). Efficient sporulation in Clostridium difficile requires disruption of the σK gene. Mol. Microbiol. 48, 811–821. doi: 10.1046/j.1365-2958.2003.03471.x
Hargreaves, K. R., Kropinski, A. M., and Clokie, M. R. J. (2014). What does the talking? PLoS One 9:e85131. doi: 10.1371/journal.pone.0085131
Hopman, N. E. M., Keessen, E. C., Harmanus, C., Sanders, I. M. J. G., van Leengoed, L. A. M. G., Kuijper, E. J., et al. (2011). Acquisition of Clostridium difficile by piglets. Vet. Microbiol. 149, 186–192. doi: 10.1016/j.vetmic.2010.10.013
Janezic, S., Potocnik, M., Zidaric, V., and Rupnik, M. (2016). Highly divergent Clostridium difficile strains isolated from the environment. PLoS One 11:e0167101. doi: 10.1371/journal.pone.0167101
Kim, K. H., Fekety, R., Batts, D. H., Brown, D., Cudmore, M., Silva, J., et al. (1981). Isolation of Clostridium difficile from the Environment and Contacts of Patients with Antibiotic-Associated Colitis. J. Infect. Dis. 143, 42–50. doi: 10.1093/infdis/143.1.42
Knetsch, C. W., Connor, T. R., Mutreja, A., van Dorp, S. M., Sanders, I. M., Browne, H. P., et al. (2014). Whole genome sequencing reveals potential spread of Clostridium difficile between humans and farm animals in the Netherlands, 2002 to 2011. Eurosurveillance 19:20954. doi: 10.2807/1560-7917.es2014.19.45.20954
Knetsch, C. W., Kumar, N., Forster, S. C., Connor, T. R., Browne, H. P., Harmanus, C., et al. (2018). Zoonotic transfer of Clostridium difficile harboring antimicrobial resistance between farm animals and humans. J. Clin. Microbiol. 56, e1384–e1317. doi: 10.1128/JCM.01384-17
Knight, D. R., Elliott, B., Chang, B. J., Perkins, T. T., and Riley, V. (2015). Diversity and Evolution in the Genome of Clostridium difficile. Clin. Microbiol. Rev. 28, 721–741. doi: 10.1128/CMR.00127-14
Knight, D. R., Kullin, B., Androga, G. O., Barbut, F., Eckert, C., Johnson, S., et al. (2019). Evolutionary and genomic insights into clostridioides difficile sequence type 11: a diverse zoonotic and antimicrobial-resistant lineage of global one health importance. MBio 10, 1–17. doi: 10.1128/MBIO.00446-19
Koene, M. G. J., Mevius, D., Wagenaar, J. A., Harmanus, C., Hensgens, M. P. M., Meetsma, A. M., et al. (2012). Clostridium difficile in Dutch animals: their presence, characteristics and similarities with human isolates. Clin. Microbiol. Infect. 18, 778–784. doi: 10.1111/j.1469-0691.2011.03651.x
Krijger, I. M., Meerburg, B. G., Harmanus, C., and Burt, S. A. (2019). Clostridium difficile in wild rodents and insectivores in the Netherlands. Lett. Appl. Microbiol. 69, 35–40. doi: 10.1111/lam.13159
Krutova, M., Zouharova, M., Matejkova, J., Tkadlec, J., Krejèí, J., Faldyna, M., et al. (2018). The emergence of Clostridium difficile PCR ribotype 078 in piglets in the Czech Republic clusters with Clostridium difficile PCR ribotype 078 isolates from Germany. Japan and Taiwan. Int. J. Med. Microbiol. 308, 770–775. doi: 10.1016/j.ijmm.2018.05.006
Kumar, S., Stecher, G., and Tamura, K. (2016). MEGA7 : molecular Evolutionary Genetics Analysis Version 7.0 for Bigger Datasets Brief communication. Mol. Biol. Evol. 33, 1870–1874. doi: 10.1093/molbev/msw054
Lance George, W., Goldstein, E. J. C., Sutter, V. L., Ludwig, S. L., and Finegold, S. M. (1978). Ætiology of Antimicrobial-Agent-Associated Colitis. Lancet 311, 802–803. doi: 10.1016/S0140-6736(78)93001-5
Le Maréchal, C., Gateau, C., Poezevara, T., Couturier, J., Rouxel, S., Syed Zaidi, R., et al. (2020). Characterization of Clostridioides difficile strains isolated from manure and digestate in five agricultural biogas plants. Anaerobe 62:102180. doi: 10.1016/j.anaerobe.2020.102180
Letunic, I., and Bork, P. (2021). Interactive tree of life (iTOL) v5: an online tool for phylogenetic tree display and annotation. Nucleic Acids Res. 49, W293–W296. doi: 10.1093/nar/gkab301
Lim, S. C., Knight, D. R., Moono, P., Foster, N. F., and Riley, T. V. (2020). Clostridium difficile in soil conditioners, mulches and garden mixes with evidence of a clonal relationship with historical food and clinical isolates. Environ. Microbiol. Rep. 12, 672–680. doi: 10.1111/1758-2229.12889
Llarena, A.-K., Ribeiro-Gonçalves, B. F. Nuno Silva, D., Halkilahti, J., Machado, M. P. Da Silva, M. S., et al. (2018). INNUENDO: a cross-sectoral platform for the integration of genomics in the surveillance of food-borne pathogens. EFSA Support Publ. 15:142. doi: 10.2903/sp.efsa.2018.en-1498
Ofori, E., Ramai, D., Dhawan, M., Mustafa, F., Gasperino, J., and Reddy, M. (2018). Community-acquired Clostridium difficile : epidemiology, ribotype, risk factors, hospital and intensive care unit outcomes, and current and emerging therapies. J. Hosp. Infect. 99, 436–442. doi: 10.1016/j.jhin.2018.01.015
Okada, Y., Okugawa, S., Ikeda, M., Kobayashi, T., Saito, R., Higurashi, Y., et al. (2020). Genetic diversity and epidemiology of accessory gene regulator loci in Clostridioides difficile. Access Microbiol. 2:acmi000134. doi: 10.1099/acmi.0.000134
O’Shaughnessy, R. A., Habing, G. G., Gebreyes, W. A., Bowman, A. S., Weese, J. S., Rousseau, J., et al. (2019). Clostridioides difficile on Ohio swine farms (2015): a comparison of swine and human environments and assessment of on-farm risk factors. Zoonoses Public Health 66, 861–870. doi: 10.1111/zph.12637
Persson, S., Torpdahl, M., and Olsen, K. E. P. (2009). Erratum: new multiplex PCR method for the detection of the Clostridium difficile toxin A (tcdA) and toxin B (tcdB) and the binary toxin (cdtA/cdtB) genes applied to a Danish strain collection (Clinical Microbiology and Infection (2008) vol. 14 (1057-1064). Clin. Microbiol. Infect. 15:296. doi: 10.1111/j.1469-0691.2009.02777_1.x
Riedel, T., Neumann-Schaal, M., Wittmann, J., Schober, I., Hofmann, J. D., Lu, C. W., et al. (2020). Characterization of Clostridioides difficile DSM 101085 with A-B-CDT+Phenotype from a Late Recurrent Colonization. Genome Biol. Evol. 12, 566–577. doi: 10.1093/gbe/evaa072
Robinson, J. T., Thorvaldsdóttir, H., Winckler, W., Guttman, M., Lander, E. S., Getz, G., et al. (2011). Integrative Genome Viewer. Nat. Biotechnol. 29, 24–26. doi: 10.1038/nbt.1754.Integrative
Rodriguez, C., Avesani, V., Van Broeck, J., Taminiau, B., Delmée, M., and Daube, G. (2013). Presence of Clostridium difficile in pigs and cattle intestinal contents and carcass contamination at the slaughterhouse in Belgium. Int. J. Food Microbiol. 166, 256–262. doi: 10.1016/j.ijfoodmicro.2013.07.017
Rodriguez, C., Taminiau, B., Van Broeck, J., Avesani, V., Delmée, M., and Daube, G. (2012). Clostridium difficile in young farm animals and slaughter animals in Belgium. Anaerobe 18, 621–625. doi: 10.1016/j.anaerobe.2012.09.008
Rodriguez, C., Taminiau, B., Van Broeck, J., Delmée, M., and Daube, G. (2016). Clostridium difficile in food and animals: a comprehensive review. Adv. Exp. Med. Biol. 932, 65–92. doi: 10.1007/5584_2016_27
Rodriguez Diaz, C., Seyboldt, C., and Rupnik, M. (2018). Non-human C. difficile reservoirs and sources: animals, food, environment. Adv. Exp. Med. Biol. 1050, 227–243. doi: 10.1007/978-3-319-72799-8_13
Rodriguez-Palacios, A., Mo, K. Q., Shah, B. U., Msuya, J., Bijedic, N., Deshpande, A., et al. (2020). Global and Historical Distribution of Clostridioides difficile in the Human Diet (1981–2019): systematic Review and Meta-Analysis of 21886 Samples Reveal Sources of Heterogeneity, High-Risk Foods, and Unexpected Higher Prevalence Toward the Tropic. Front. Med. 7:9. doi: 10.3389/fmed.2020.00009
De Roo, A. C., and Regenbogen, S. E. (2020). Clostridium dif fi cile Infection : an Epidemiology Update. Clin. Colon Rectal. Surg. 33, 49–57.
Saitou, N., and Nei, M. (1987). The Neighbor-joining Method : a New Method for Reconstructing Phylogenetic Trees’. Mol. Biol. Evol. 4, 406–425.
Saujet, L., Pereira, F. C., Henriques, A. O., and Martin-Verstraete, I. (2014). The regulatory network controlling spore formation in Clostridium difficile. FEMS Microbiol. Lett. 358, 1–10. doi: 10.1111/1574-6968.12540
Schneeberg, A., Neubauer, H., Schmoock, G., Baier, S., Harlizius, J., Nienhoff, H., et al. (2013). Clostridium difficile genotypes in piglet populations in germany. J. Clin. Microbiol. 51, 3796–3803. doi: 10.1128/JCM.01440-13
Slimings, C., and Riley, T. V. (2014). Antibiotics and hospital-acquired Clostridium difficile infection: update of systematic review and meta-analysis. J. Antimicrob. Chemother. 69, 881–891. doi: 10.1093/jac/dkt477
Stein, K., Egan, S., Lynch, H., Harmanus, C., Kyne, L., Herra, C., et al. (2017). PCR-ribotype distribution of Clostridium difficile in Irish pigs. Anaerobe 48, 237–241. doi: 10.1016/j.anaerobe.2017.10.004
Steinberg, H. D., and Snitkin, E. S. (2020). Homologous Recombination in Clostridioides difficile Mediates Diversification of Cell Surface Features and Transport Systems. mSphere 5, e799–e720. doi: 10.1128/msphere.00799-20
Susick, E. K., Putnam, M., Bermudez, D. M., and Thakur, S. (2012). Longitudinal study comparing the dynamics of Clostridium difficile in conventional and antimicrobial free pigs at farm and slaughter. Vet. Microbiol. 157, 172–178. doi: 10.1016/j.vetmic.2011.12.017
Tamura, K., Nei, M., and Kumar, S. (2004). Prospects for inferring very large phylogenies by using the neighbor-joining method. Proc. Natl. Acad. Sci. U.S.A. 101, 11030–11035. doi: 10.1073/pnas.0404206101
Voth, D. E., and Ballard, J. D. (2005). Clostridium difficile toxins: mechanism of action and role in disease. Clin. Microbiol. Rev. 18, 247–263. doi: 10.1128/CMR.18.2.247-263.2005
Walker, B. J., Abeel, T., Shea, T., Priest, M., Abouelliel, A., Sakthikumar, S., et al. (2014). Pilon: an integrated tool for comprehensive microbial variant detection and genome assembly improvement. PLoS One 9:e112963. doi: 10.1371/journal.pone.0112963
Wasels, F., Monot, M., Spigaglia, P., Barbanti, F., Ma, L., Bouchier, C., et al. (2014). Inter-and intraspecies transfer of a clostridium difficile conjugative transposon conferring resistance to MLSB. Microb. Drug Resist. 20, 555–560. doi: 10.1089/mdr.2014.0015
Weese, J. S., Wakeford, T., Reid-Smith, R., Rousseau, J., and Friendship, R. (2010). Longitudinal investigation of Clostridium difficile shedding in piglets. Anaerobe 16, 501–504. doi: 10.1016/j.anaerobe.2010.08.001
Werner, A., Mölling, P., Fagerström, A., Dyrkell, F., Arnellos, D., Johansson, K., et al. (2021). Whole genome sequencing of Clostridioides difficile PCR ribotype 046 suggests transmission between pigs and humans. PLoS One 15:e0244227. doi: 10.1371/journal.pone.0244227
Keywords: Clostridioides difficile, RT033, PaLoc, Pig, environment, One Health, transmission dynamics
Citation: Alves F, Nunes A, Castro R, Sequeira A, Moreira O, Matias R, Rodrigues JC, Silveira L, Gomes JP and Oleastro M (2022) Assessment of the Transmission Dynamics of Clostridioides difficile in a Farm Environment Reveals the Presence of a New Toxigenic Strain Connected to Swine Production. Front. Microbiol. 13:858310. doi: 10.3389/fmicb.2022.858310
Received: 19 January 2022; Accepted: 21 March 2022;
Published: 14 April 2022.
Edited by:
Ruiting Lan, University of New South Wales, AustraliaReviewed by:
Zuowei Wu, Iowa State University, United StatesEliane De Oliveira Ferreira, Federal University of Rio de Janeiro, Brazil
Copyright © 2022 Alves, Nunes, Castro, Sequeira, Moreira, Matias, Rodrigues, Silveira, Gomes and Oleastro. This is an open-access article distributed under the terms of the Creative Commons Attribution License (CC BY). The use, distribution or reproduction in other forums is permitted, provided the original author(s) and the copyright owner(s) are credited and that the original publication in this journal is cited, in accordance with accepted academic practice. No use, distribution or reproduction is permitted which does not comply with these terms.
*Correspondence: Mónica Oleastro, bW9uaWNhLm9sZWFzdHJvQGluc2EubWluLXNhdWRlLnB0
†These authors have contributed equally to this work