- 1College of Pharmacy, Sahmyook University, Seoul, South Korea
- 2College of Pharmacy, Chungbuk National University, Cheongju, South Korea
- 3College of Animal Biotechnology and Resource, Sahmyook University, Seoul, South Korea
Knowledge of the impact of the gut microbiota on human health has increased, and modulation of the bacterial community is now considered a therapeutic target for various diseases. Certain novel bacterial species have probiotic properties associated with improvement in obesity and related metabolic disorders. The relative abundance of Butyricimonas spp. is correlated with metabolic parameters; however, the physiological role of Butyricimonas in metabolic improvement is unclear. In this study, live and heat-killed Butyricimonas virosa were administered to mice with high-fat diet (HFD)-induced obesity. Both live and heat-killed B. virosa ameliorated HFD-impaired body weight, serum glucose level, insulin resistance, and liver steatosis. Moreover, activation of the glucagon-like peptide-1 receptor (GLP-1R) and peroxisome proliferator-activated receptor α (PPARα) was observed in the liver, and the expression levels of insulin receptor substrate (IRS)-1, IRS-2, Toll-like receptor 5 (TLR5), and zonula occludens-1 (ZO-1) were upregulated in the ileum. Finally, we demonstrated that the effect of B. virosa treatment on glucose regulation may be linked to the upregulation of GLP-1R in the liver and is not a result of colonization of the gut by B. virosa or B. virosa-produced butyrate. Our results provide a rationale for the development of Butyricimonas spp.-based therapeutics and prophylactics for hyperglycemia.
Introduction
The effect of the gut microbiota on human health has been investigated in the past decade. The intestinal microbial ecology is considered an important factor in energy metabolism and the immune responses to various diseases (Boulange et al., 2016). Importantly, novel microbes residing in the human gut were identified by next-generation sequencing. Certain of these microbes were named pharmabiotics because they exerted therapeutic effects beyond those of probiotics. For example, Akkermansia muciniphila and Faecalibacterium prausnitzii are considered therapeutic targets for multiple diseases (Derrien et al., 2017; Martin et al., 2017; Depommier et al., 2019). Moreover, a recent human clinical study demonstrated that A. muciniphila significantly improved metabolic parameters (including insulin sensitivity and total cholesterol level), such that it is considered a pharmabiotic (Depommier et al., 2019).
Microbes with therapeutic effects on metabolic disorders including obesity, hyperglycemia, and hypercholesterolemia have been reported (Okubo et al., 2018). Potential pharmabiotic candidates typically have common properties, such as the ability to produce short-chain fatty acids (SCFAs), enhance gut barrier function, improve gut microbial imbalance, and regulate inflammatory immune responses (Nguyen et al., 2017). These beneficial effects are linked to improvements in insulin resistance and lipid metabolism, as well as reduced inflammation. Pharmabiotics in clinical and animal studies demonstrated multiple beneficial effects, indicating that targeting the gut microbiota is an intriguing therapeutic target for metabolic disorders (Okubo et al., 2018).
Butyricimonas is a Gram-negative anaerobic bacterial genus of the family Odoribacteraceae. They are present in the intestinal tract of several mammals, including rat and human (Sakamoto et al., 2009, 2014), but few species have been isolated (B. faecihominis, B. synergistica, B. paravirosa, B. virosa, and B. phoceensis). Treatment of metabolic disorders with metformin and statins significantly increased the relative abundance of Butyricimonas spp. in the gut, which was significantly correlated with metabolic parameters (Lee et al., 2018; Kim et al., 2019). However, the direct effect of Butyricimonas on metabolic improvements, and the underlying physiological mechanisms, have not been investigated.
The characteristics of Butyricimonas and our previous results support the hypothesis that Butyricimonas species are involved in commensal homeostasis between the gut microbiota and host, and exert a beneficial effect on host energy metabolism. To test this hypothesis, we investigated the metabolic effects induced by oral administration of live and heat-killed Butyricimonas virosa to mice with high-fat diet (HFD)-induced obesity. Furthermore, we conducted comparative analysis of metabolism-related transcripts.
Materials and Methods
Butyricimonas
Butyricimonas virosa (KCTC 15148) was purchased from the Korean Collection for Type Cultures (KCTC) and cultured in Columbia broth supplemented with 5% horse serum under anaerobic conditions (i.e., in an anaerobic jar) at 37°C for 5 days. Cultured B. virosa (estimated 5 × 108 CFU/ml optical density) was washed in phosphate-buffered saline (PBS) and stored at −70°C until inoculation, and their viability was confirmed using the spread plate method. In addition, heat-killed B. virosa were prepared by autoclaving for 15 min at 121°C and 15 lb.
Animal Model
Male 4-week-old C57BL/6 N mice were purchased from Samtako Co., Ltd. (Osan, South Korea) and acclimated to laboratory conditions for 1 week, during which the animals were housed in a temperature and humidity-controlled animal facility under a 12 h light–dark cycle at 22 ± 2°C and 55 ± 5% humidity, with ad libitum access to water and food. Mice were fed a 60% kcal HFD (FeedLab, Inc., Guri, South Korea) to induce metabolic disorders—such as obesity, hyperglycemia, and hyperlipidemia—for 16 weeks. The mice were treated with B. virosa by oral gavage at 1 × 108 CFU/200 μl (HFD-Bu: live B. virosa during HFD, n = 6, or HFD-hk-Bu: heat-killed B. virosa during HFD, n = 6) daily for the final 6 weeks of HFD feeding. A regular diet (RD, 10% kcal; Purina Korea, Inc., Seoul, South Korea)-fed group (n = 6) and HFD-fed group (HFD without B. virosa, n = 5) were included as normal and disease controls, respectively.
Metabolic Analysis
Body weight, serum glucose level, and food intake were recorded weekly. The serum glucose level was determined using the Accu-Chek Performa system (Roche Diagnostics, Mannheim, Germany) following fasting for 12 h (dark cycle). Intraperitoneal glucose tolerance testing (IPGTT) was performed 6 weeks after treatment with B. virosa. The mice were intraperitoneally injected with glucose solution (2 g/kg in PBS) after fasting 12 h (dark cycle), and the glucose level was measured by tail vein sampling using blood lancet at 15, 30, 60, and 120 min after injection. The incremental area under the curve (iAUC) for glucose was calculated for comparison of IPGTT results among time points. The Homeostatic Model Assessment of Insulin Resistance (HOMA-IR) index was used to evaluate insulin resistance (Matthews et al., 1985). Blood samples were collected by cardiac puncture and centrifuged at 10,000 rpm for 5 min to isolate serum. The serum levels of apolipoprotein B (ApoB), low-density lipoprotein (LDL), high-density lipoprotein (HDL), aspartate aminotransferase (AST), and alanine aminotransferase (ALT) were determined using a biochemical analyzer (AU480; Beckman Coulter, Brea, CA).
Liver Histology
Hepatic tissue was fixed in 10% neutral formalin. Tissue samples were filtered and embedded in paraffin, cut into 4-μm sections, and stained with hematoxylin and eosin (H&E). Sections were observed using a microscope (Nikon, Tokyo, Japan), and micrographs were taken at ×100 magnification. The percentage of steatosis in images was assessed using ImageJ software (Bethesda, MD).
Transcriptome Analysis
Ileum and liver tissues were immediately frozen in liquid nitrogen and stored at −70°C for later analysis of transcript levels. Total RNA was extracted using the RiboEx™ Kit (GeneAll, Seoul, South Korea). cDNA was synthesized using HyperScript™ RT Premix (GeneAll) according to the manufacturer’s instructions. SYBR Green PCR Master Mix and the StepOnePlus™ Real-Time PCR System (Applied Biosystems, Waltham, MA) were used to quantify mRNA levels. The following primer sets were used as: insulin receptor substrate (IRS)-1 (forward: 5′-TCCTATCCCGAAGAGGGTCT-3′; reverse: 5′-TGGGCATATAGCCATCATCA-3′), IRS-2 (forward: 5′-TCCAGAACGGCCTCAACTAT-3′; reverse: 5′-AGTGATGGGACAGGAAGTCG-3′), glucagon-like peptide-1 receptor (GLP-1R; forward: 5′-TCAGAGACGGTGCAGAAATG-3′; reverse: 5′-CAGCTGACATTCACGAAGGA-3′), Dipeptidyl peptidase-4 (DPP4; forward: 5′-TTGTGGATAGCAAGCGAGTTG-3′; reverse: 5′-CACAGCTATTCCGCACTTGAA-3′), zonula occludens-1 (ZO-1; forward: 5′-GCTCATAGTTCAACACAGCCTCCAG-3′; reverse: 5′-TTCTTCCACAGCTGAAGGACTCACAG-3′), peroxisome proliferator-activated receptor α (PPARα, forward: 5′-TCGGCGAACTATTCGGCTG-3′; reverse: 5′-GCACTTGTGAAAACGGCAGT-3′), PPAR𝛾 (forward: 5′-TGTGGGGATAAAGCATCAGGC-3′; reverse: 5′-CCGGCAGTTAAGATCACACCTAT-3′), Toll-like receptor 5 (TLR5; forward: 5′-AAGTTCCGGGGAATCTGTTT-3′; reverse: 5′-GCATAGCCTGAGCCTGTTTC-3′), and glyceraldehyde 3-phosphate dehydrogenase (GAPDH; forward: 5′-AACTTTGGCATTGTGGAAGG-3′; reverse: 5′-ACACATTGGGGGTAGGAACA-3′).
Relative Abundance of Butyricimonas
The relative abundance of Butyricimonas in fecal samples was evaluated using SYBR® Green PCR Master Mix and the StepOnePlus™ Real-Time PCR System (Applied Biosystems). Fecal samples were collected 6 weeks after treatment with B. virosa. Total DNA was extracted using the PowerSoil DNA Isolation Kit (MO BIO Laboratories Inc., Carlsbad, CA) according to the manufacturer’s instructions. Primer sets for total bacteria (515f, forward: 5′-GTGCCAGCMGCCGCGGTAA-3′; 806r, reverse: 5′-GGACTACHVHHHTWTCTAAT-3′) and Butyricimonas (Buty1f, forward: 5′-GGTGAGTAACACGTGTGCAAC-3′; Buty1r, reverse: 5′-TACCCCGCCAACTACCTAATG-3′) were used for amplification.
Butyrate Analysis
Butyrate in feces was quantified using a modified version of a method published elsewhere (Cuervo et al., 2013; Jang et al., 2020). Homogenized mouse feces (10 mg/50 μl of PBS) was dried in a dry oven at 60°C to remove water. The dried sample was extracted with 500 μl of MeOH for 10 min in a shaking incubator. An internal standard, 4-methylvaleric acid (Sigma-Aldrich, St. Louis, MO), was added to the supernatant. The SCFA concentration was calculated using standard reagents (acetate, propionate, and butyrate; Sigma-Aldrich) with Autochro-3,000 software and the YL6100 GC system, which was equipped with a flame ionization detector (FID) and capillary column (DB-Wax column, 30 m × 0.25 mm × 0.25 μm; Agilent Technology, Santa Clara, CA). The inlet and detector temperatures were set to 220°C. Samples (2 μl) were introduced by splitless injection. The oven temperature was initially maintained at 50°C for 1 min and then increased to 220°C at a rate of 10°C/min.
Western Blotting
Total liver protein was extracted using RIPA lysis buffer (GenDEPOT, Katy, TX) supplemented with a protease inhibitor cocktail solution (GenDEPOT). The homogenate was incubated at 4°C for 2 h. The protein concentration was measured by the Bradford method. Protein samples (20 μg) were subjected to 10% SDS-polyacrylamide gel electrophoresis and transferred to nitrocellulose membranes. The membranes were blocked with 5% bovine serum albumin (BSA) in Tris-buffered saline containing 0.1% Tween-20 (TBST) for 2 h. They were then incubated at 4°C overnight with the primary anti-GAPDH antibody and anti-GLP-1R polyclonal antibody (1:1,000; Abcam, Cambridge, UK). After incubation with an anti-rabbit IgG horse radish peroxidase (HRP)-conjugated antibody (1:5,000; GenDEPOT) for 1 h, bands were detected using a chemiluminescent peroxidase substrate (ECL Plus; GenDEPOT) and imaged using the ChemiDoc XRS System (Bio-Rad, Hercules, CA).
Statistical Analysis
Data are presented as means ± standard error of mean (SEM). To quantify the in vivo mRNA levels relative to the internal control (GAPDH), the 2–ΔΔCt relative quantification method (ΔΔCt = (Ct.Target − Ct.GAPDH)Group1 − (Ct.Target − Ct.GAPDH)Group2) was used. Statistical significance was assessed by Kruskal-Wallis H test, followed by Duncan’s post-hoc test. Statistical analysis was performed using RStudio (R Development Core Team, Vienna, Austria). A p-value of <0.05 was considered indicative of statistical significance.
Results
Effect of B. virosa on Metabolic Disorders
Feeding the mice an HFD for 16 weeks induced weight gain; the average body weight of mice in the HFD group (46.5 ± 0.5 g) was significantly increased compared to that of mice in the RD group (32.0 ± 3.6 g). After treatment with B. virosa for 6 weeks, the body weight of mice in the HFD-Bu (44.7 ± 3.6 g) and HFD-hk-Bu (43.7 ± 5.7 g) groups was decreased compared to mice in the HFD group (48.2 ± 3.6 g; Figure 1A). The mice in the HFD group gained 2.2 ± 1.2 g after the 6-week HFD, whereas those in the HFD-Bu and HFD-hk-Bu groups lost 2.2 ± 1.7 and 2.0 ± 0.8 g, respectively (Figure 1B). Daily food intake was not significantly different among the groups.
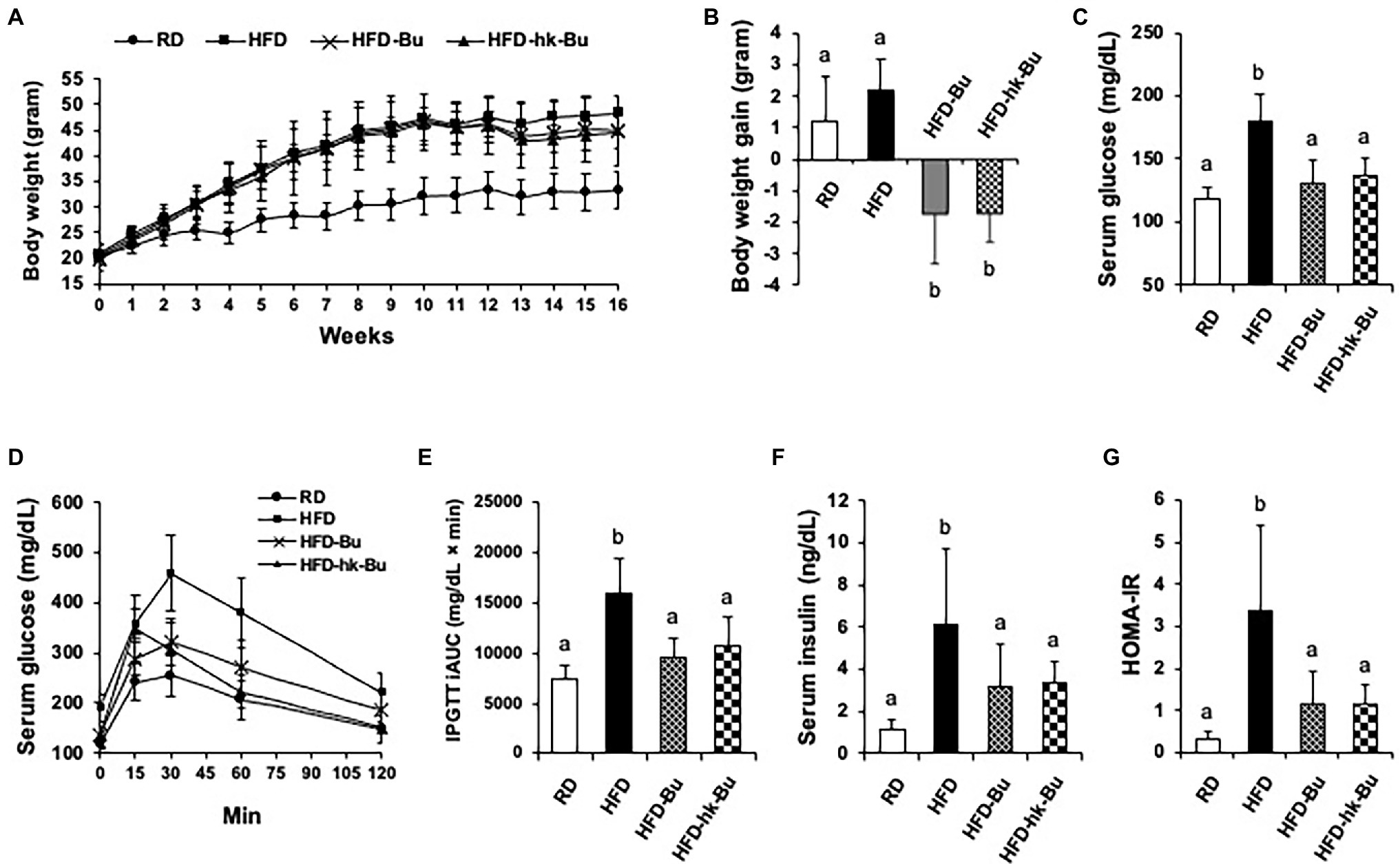
Figure 1. Effect of Butyricimonas virosa treatment on body weight (A, B), fasting serum glucose level (C-E), and insulin resistance (F, G). Five-week-old C57BL/6 N mice were fed a HFD (60% lipid) for 16 weeks to induce metabolic disorders. Then, live and heat-killed B. virosa were orally administered daily for the final 6 weeks of HFD feeding. Body weight change was measured at 6 weeks after treatment with B. virosa. Treatment with B. virosa significantly improved metabolic profiles. RD: Regular diet (N = 6); HFD: High-fat diet (N = 5); HFD-Bu: Live B. virosa administration during HFD feeding (N = 6); and HFD-hk-Bu: Heat-killed B. virosa administration during HFD feeding (N = 6). Different superscript letters indicate significant differences (p < 0.05) according to Duncan’s post-hoc test.
Treatment with B. virosa significantly improved serum glucose regulation. B. virosa significantly reduced the HFD-induced increase in fasting serum glucose levels in mice in the HFD-Bu (131.2 ± 17.6 mg/dl) and HFD-hk-Bu (137.1 ± 13.5 mg/dl) groups compared to the HFD group (180.2 ± 21.3 mg/dl; Figure 1C) after 6 weeks of B. virosa treatment and significantly ameliorated glucose tolerance (IPGTT; Figures 1D,E). Moreover, B. virosa reduced the serum insulin level in the HFD-Bu (3.2 ± 2.0 ng/dl) and HFD-hk-Bu (3.4 ± 1.0 ng/dl) groups compared to the HFD group (6.1 ± 3.6 ng/dl) and significantly improved the HOMA-IR scores (HFD: 3.4 ± 2.0, HFD-Bu: 1.1 ± 0.8 and HFD-hk-Bu: 1.2 ± 0.4; Figures 1F,G).
A significant decrease in the HFD-induced increased ALT and ApoB levels was observed in the HFD-Bu and HFD-hk-Bu groups compared to the HFD group, but the AST/ALT ratio was significantly improved only in the HFD-Bu group (Figures 2A–C). In addition, the LDL/HDL ratio was significantly decreased only in the HFD-Bu group (Figure 2D). Daily food intake was not significantly different among the groups.
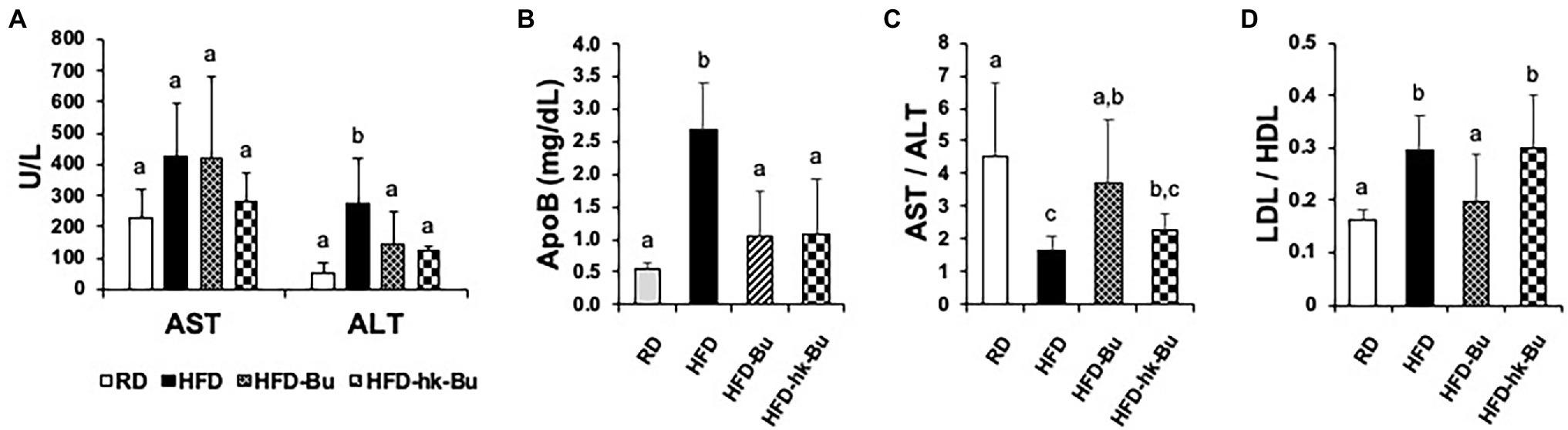
Figure 2. Biochemical analysis of serum. Significant recovery of the serum ALT (A) and ApoB (B) levels was observed in both the HFD-Bu and HFD-hk-Bu groups. The AST/AST (C) and LDL/HDL (D) ratios were significantly recovered only in the HFD-Bu group. Blood samples were collected via cardiac puncture, biochemical analysis was performed using a biochemical analyzer. Different superscript letters indicate significant differences (p < 0.05) according to Duncan’s post-hoc test. ALT, Alanine aminotransferase; ApoB, Apolipoprotein B, LDL, HFD-Bu, Live B. virosa administration during HFD feeding; HFD-hk-Bu, Heat-killed B. virosa administration during HFD feeding; Low-density lipoprotein HDL, High-density lipoprotein; and AST, Aspartate aminotransferase.
Effect of B. virosa on Liver Histology
The HFD-induced increased liver weight was significantly decreased by B. virosa treatment compared to the HFD group (Figure 3A). The severe liver steatosis in the HFD group was recovered by both live and heat-killed B. virosa (Figures 3B,C).
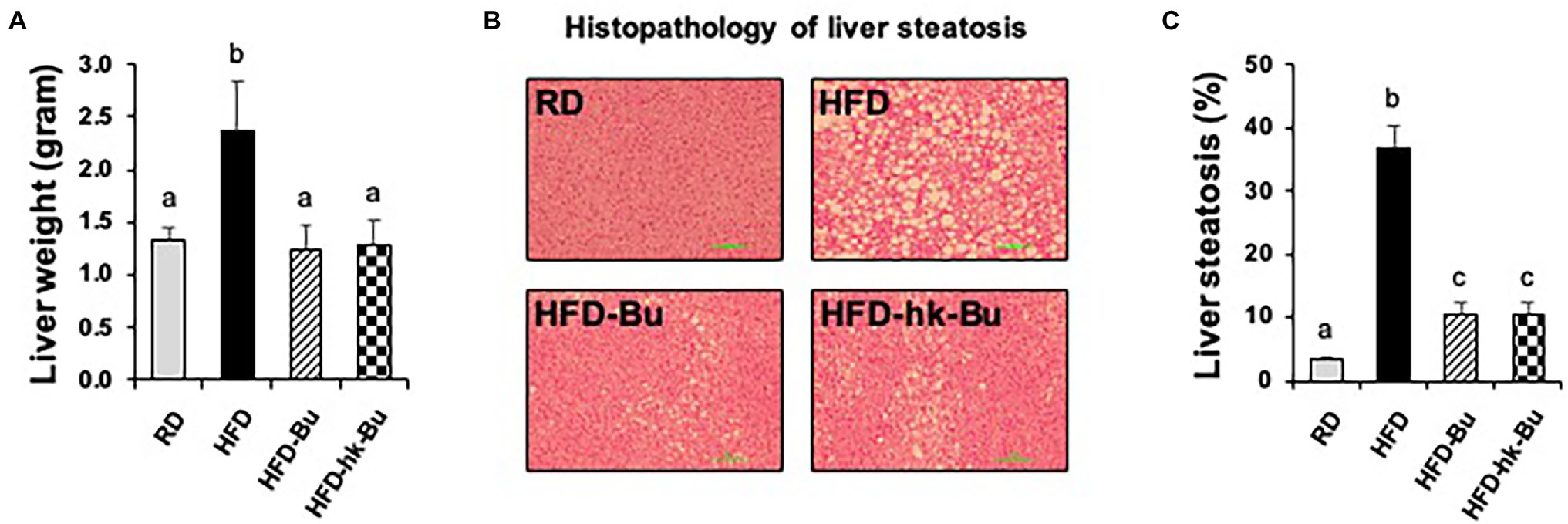
Figure 3. Liver histology. C57BL/6 N mice fed a HFD (60% lipid) for 16 weeks showed an increase in the total liver weight (A) and lipid deposition in the liver (B). Both treatment with live and heat-killed Butyricimonas virosa for 6 weeks significantly reduced the liver weight and lipid deposition. (C) Percentage of steatosis in liver histology images was analyzed with ImageJ software. Histological analysis of liver steatosis was performed using H&E staining. Different superscript letters indicate significant differences (p < 0.05) according to Duncan’s post-hoc test. HFD, High-fat diet; H&E, Hematoxylin and eosin.
Quantification of B. virosa and Butyrate in Feces
The relative abundance of B. virosa was 6.3 ± 5.7% and 6.2 ± 1.3% in fecal samples from mice in the HFD-Bu and HFD-hk-Bu groups, respectively, which were significantly higher than in the HFD group (2.8 ± 1.2%; Figure 4A). Furthermore, the concentration of butyrate in fecal samples was significantly decreased in the HFD group (0.21 ± 0.08 mM) compared to the RD group (0.78 ± 0.35 mM; Figure 4B). The difference in concentration of butyrate between HFD and HFD-Bu groups was not statistically significant (Figure 4B). The difference in the levels of acetate and propionate between groups were also not significant (data not shown).
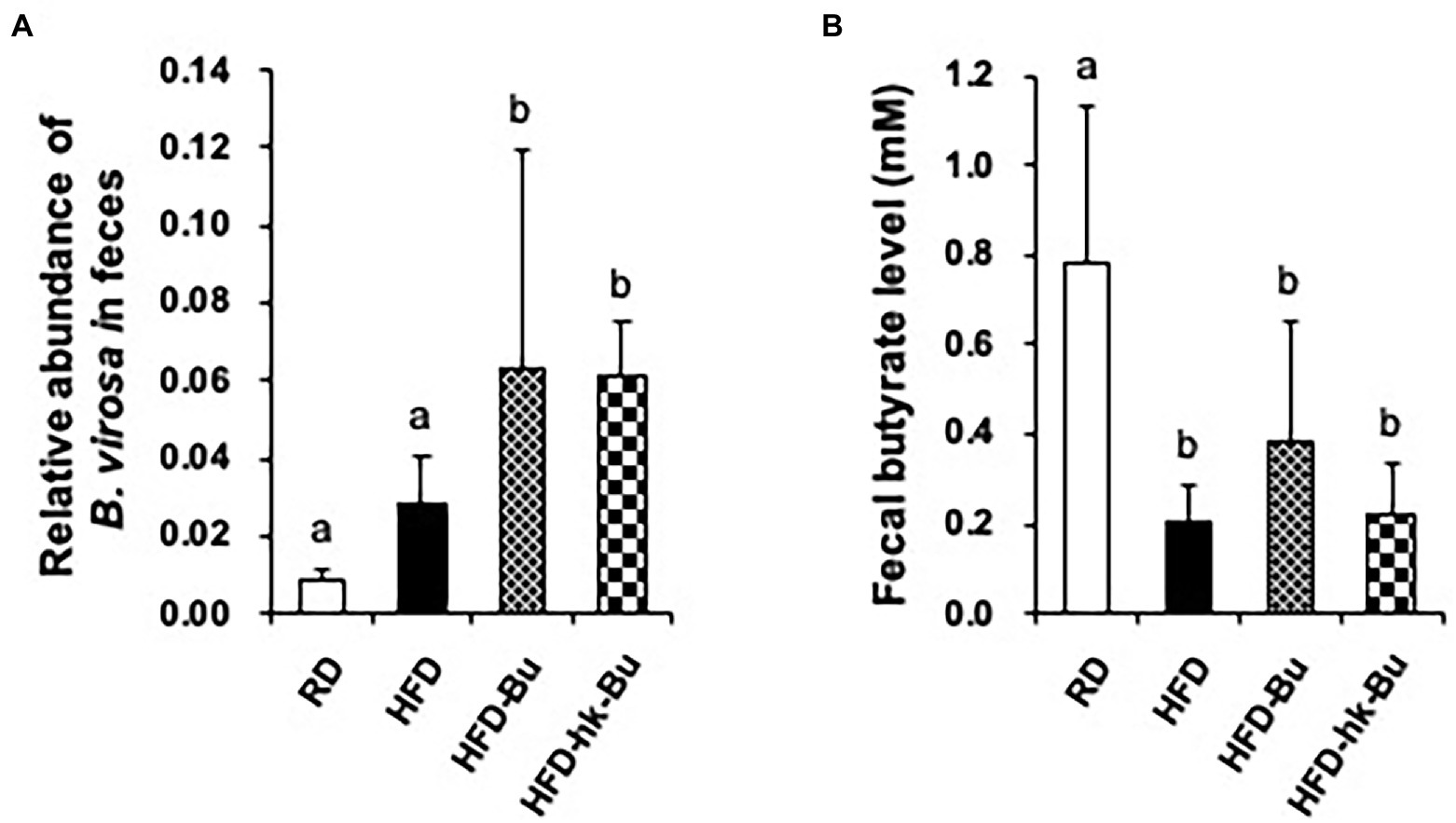
Figure 4. Quantification of Butyricimonas virosa and butyrate levels in feces. Fecal samples were collected at 6 weeks after treatment with B. virosa. Relative abundance of B. virosa analyzed using quantitative PCR (A), the concentration of butyrate was measured using gas chromatography (B). The relative abundance of B. virosa and the concentration of butyrate in fecal samples were significantly increased after treatment of B. virosa compared to HFD group.
Transcriptome Analysis in the Liver and Ileum
HFD significantly downregulated the levels of GLP-1R (0.50 ± 0.23) and PPARα (0.62 ± 0.24) in the liver compared with RD (GLP-1R: 1.08 ± 0.38 and PPARα: 1.02 ± 0.24; Figure 5A). Treatment with B. virosa significantly upregulated the liver levels of GLP-1R in the HFD-Bu (0.67 ± 0.17) and HFD-hk-Bu (0.72 ± 0.22) groups, and of PPARα in the HFD-Bu (0.96 ± 0.14) and HFD-hk-Bu (1.15 ± 0.32) groups, compared to the HFD group (Figure 5A). The levels of DPP4 and PPAR𝛾 were not significantly different between the B. virosa-treated and HFD groups.
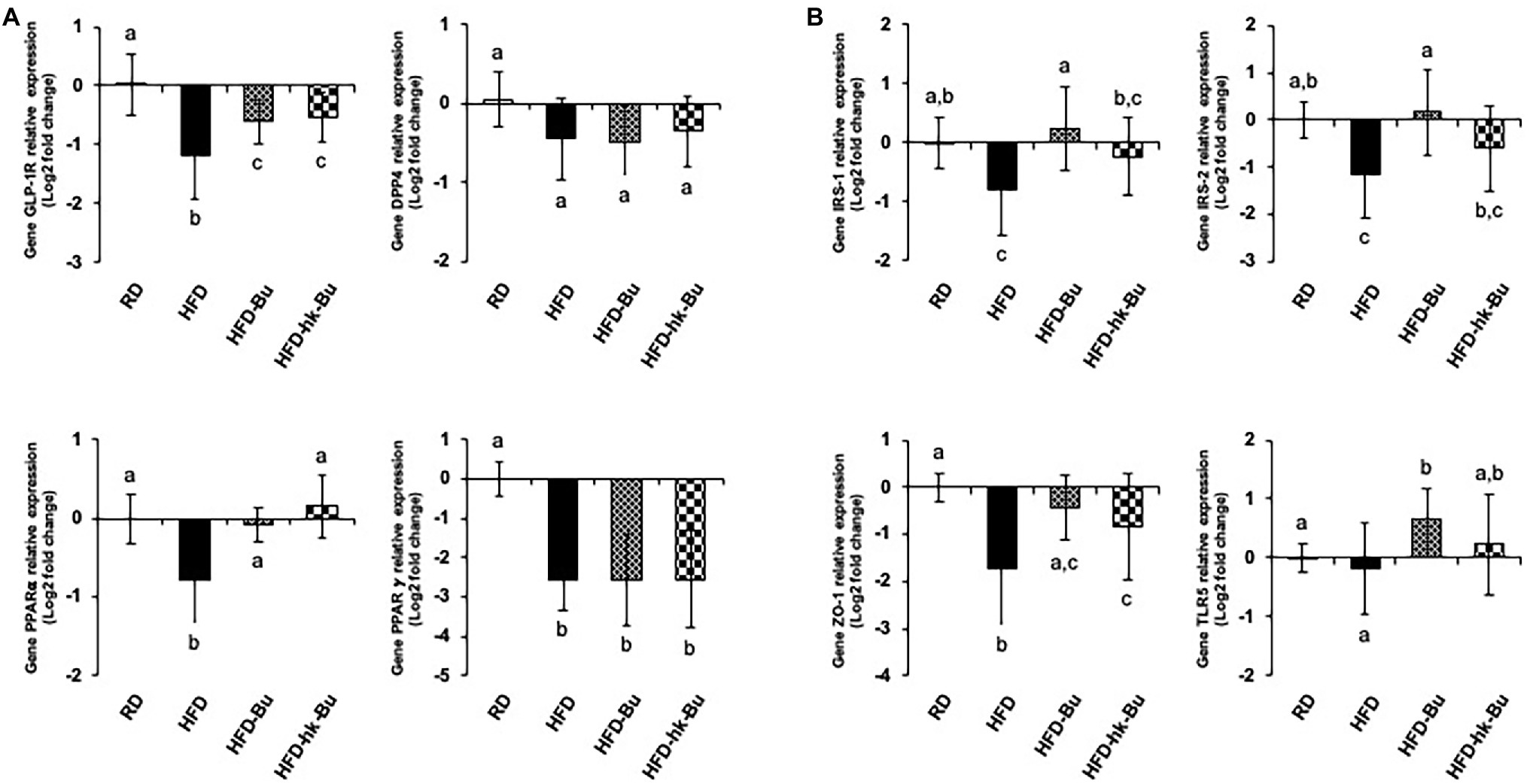
Figure 5. Transcriptome analysis in the liver (A) and ileum (B). Relative levels of mRNA associated with glucose regulation and lipid metabolism measured using quantitative PCR. The 2−ΔΔCT relative quantification method was used for analysis of the level of relative mRNA expression (log2 fold change) compared to that of GAPDH expression as an internal control. Different superscript letters indicate significant differences (p < 0.05) according to Duncan’s post-hoc test. GAPDH, Glyceraldehyde 3-phosphate dehydrogenase.
In the ileum, HFD significantly downregulated the levels of IRS-1 (0.64 ± 0.27), IRS-2 (0.54 ± 0.33), and ZO-1 (0.41 ± 0.31) compared with RD (IRS-1: 1.04 ± 0.30, IRS-2: 1.03 ± 0.29, and ZO-1: 1.02 ± 0.21), whereas the level of TLR5 was not significantly different between the RD (1.01 ± 0.17) and HFD (1.03 ± 0.64) groups (Figure 5B). Furthermore, B. virosa significantly upregulated the levels of IRS-1 in the HFD-Bu (1.35 ± 0.89) and HFD-hk-Bu (0.94 ± 0.47) groups, and those of IRS-2 in the HFD-Bu (1.32 ± 0.74) and HFD-hk-Bu (0.78 ± 0.42) groups, compared to the HFD group (Figure 5B). Moreover, B. virosa significantly upregulated the level of ZO-1 in the HFD-Bu (0.81 ± 0.34) and HFD-hk-Bu (0.71 ± 0.46) groups, and of TLR5 in the HFD-Bu (1.67 ± 0.68) and HFD-hk-Bu (1.37 ± 0.88) groups, compared to the HFD group (Figure 5B).
Regulation of GLP-1R in the Liver by B. virosa
The HFD-induced downregulation of the GLP-1R mRNA level in the liver was increased by heat-killed B. virosa (Figure 5A). In addition, the GLP-1R protein level in the liver, which was significantly decreased by the HFD, was upregulated by B. virosa compared to the HFD group (Figures 6A,B).
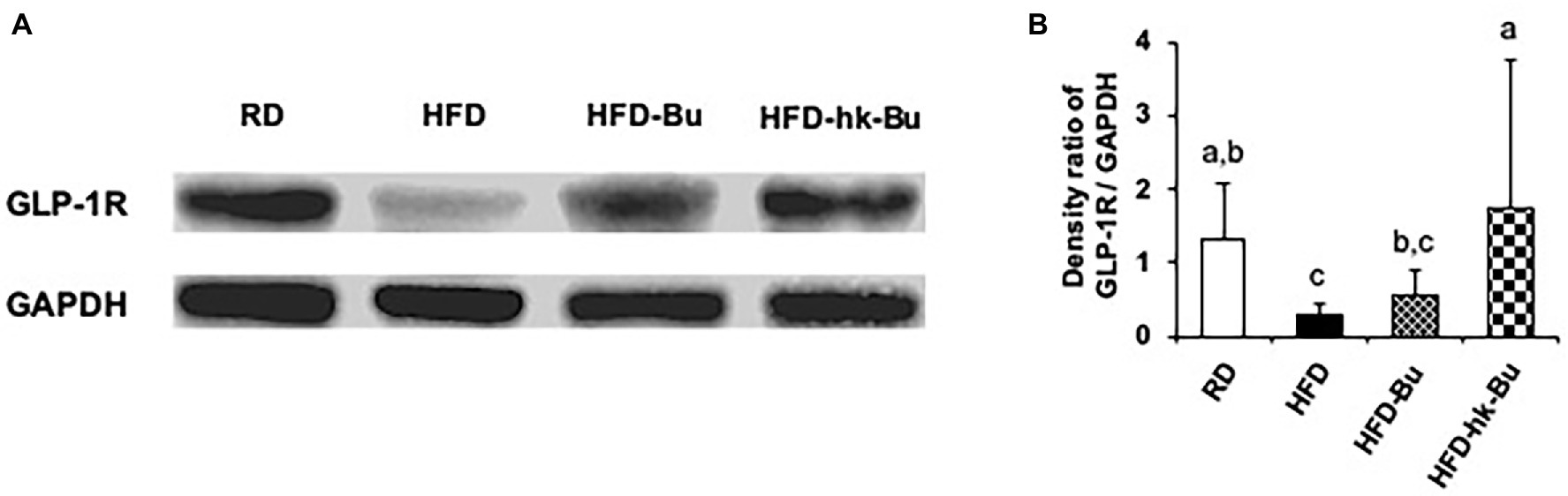
Figure 6. GLP-1R protein expression in the liver. Live and heat-killed Butyricimonas virosa were administered daily for the final 6 weeks of HFD feeding. Total protein extracted from liver lysates was used in Western blot GLP-1R analysis (A). GLP-1R protein was detected using anti-GLP-1R polyclonal antibodies; the expression ratio was quantified compared to the expression of GAPDH detected using anti-GAPDH polyclonal antibodies (B). Different superscript letters indicate significant differences (p < 0.05) according to Duncan’s post-hoc test. GLP-1R, Glucagon-like peptide-1 receptor; HFD, High-fat diet; GAPDH, Glyceraldehyde 3-phosphate dehydrogenase.
Discussion
Butyricimonas virosa had a beneficial effect on metabolic disorders, including obesity and hyperglycemia, via GLP-1R in the liver in a mouse model of obesity. This finding is in agreement with a recent report that the abundance of Butyricimonas spp. is related to metabolic improvements (Lee et al., 2018; Kim et al., 2019). In addition, enrichment of B. virosa was observed in mice colonized with the microbiota of lean twins discordant for obesity (Ridaura et al., 2013). In a human study, a high level of the genus Butyricimonas was associated with significantly decreased triglyceride levels and body mass index (BMI; Fu et al., 2015). Interestingly, heat-killed B. virosa significantly ameliorated body weight gain, hyperglycemia, insulin resistance, and liver steatosis similarly to live B. virosa. Therefore, we considered that the metabolic improvements were not mediated by B. virosa-produced butyrate.
In this study, an increase in the relative abundance of B. virosa was identified in the fecal samples of mice in the HFD-Bu and HFD-hk-Bu groups but was significant only in the former group. We predicted that metabolic improvements would not be observed in the HFD-hk-Bu group. Butyricimonas is a butyrate-producing bacterial genus and SCFAs, such as acetate, propionate, and butyrate, regulate the epithelial expression of genes involved in energy metabolism (Den Besten et al., 2013). Moreover, the administration of butyrate improved metabolic disorders, including hyperglycemia and hepatic lipogenesis, in a manner mediated by AMPK, GLUT4, and GLP-1 (Gao et al., 2019; Zhang et al., 2019).
Previous studies on A. muciniphila support the effect of heat-killed B. virosa on metabolic improvements. Live and pasteurized A. muciniphila at 70°C improved the metabolism of rodents and humans with obesity and diabetes (Depommier et al., 2019, 2020). Although it is unclear why heat-killed B. virosa induced metabolic improvements in this study, there are several plausible mechanisms. First, the effect of B. virosa may be mediated by a protein stable to autoclaving. Certain active bacterial components that mitigate insulin resistance and glucose tolerance are unaffected by heat treatment. Moreover, heat-killed B. virosa may modulate the gut microbiota and metabolic improvements. Although the effect of B. virosa on the gut microbiota was not investigated in this study, bacterial components and metabolites can act as prebiotics, modulating the gut microbiota (Salminen et al., 2021). Live and heat-killed B. virosa may have different modes of action, on which further studies are needed.
GLP-1R was implicated in glucose homeostasis following B. virosa treatment. Activation of GLP-1R is involved in regulation of hyperglycemia, and GLP-1R agonists including exenatide, liraglutide, and lixisenatide are important therapeutics for type II diabetes (Burcelin et al., 2014) Especially, GLP-1 secreted from intestinal cells activates glucose-dependent insulin secretion and inhibits glucagon release (MacDonald et al., 2002). SCFAs increase the secretion of GLP-1 and GLP-1R (Tolhurst et al., 2012; Zhang et al., 2019). Therefore, we hypothesized that the metabolic improvements induced by Butyricimonas were caused by an increase in its SCFA production. However, the expression of GLP-1R in the intestine was not significantly increased by Butyricimonas (data not shown). Interestingly, GLP-1R was upregulated in the liver, in which its activation is implicated in glucose regulation by Butyricimonas. Indeed, GLP-1 is closely related to hepatocyte fatty acid β-oxidation and insulin sensitivity (Svegliati-Baroni et al., 2011), and GLP-1R in hepatocytes modulated insulin signaling, thereby ameliorating hepatic steatosis (Gupta et al., 2010). In this study, the hepatic steatosis induced by the HFD was improved by both live and heat-killed B. virosa; thus, Butyricimonas may modulate hepatic lipogenesis via GLP-1R activation.
In addition, DPP4, which inactivates GLP-1, is an important therapeutic target for hyperglycemia. DPP4 regulation was not observed in this study, so may not be involved in GLP-1R-mediated glucose regulation by Butyricimonas. Hepatic DPP4 is important in insulin resistance and the anti-diabetic effect of DPP4 inhibitors is mediated by upregulation of intact GLP-1 (Kim et al., 2016). However, an anti-glycemic effect of DPP4 inhibitors is apparent in mice lacking GLP-1R (Hansotia et al., 2004), and GLP-1R antagonism does not eliminate the anti-glycemic effect of DPP4 inhibitors in patients with T2D (Nauck et al., 2016). Moreover, GLP-1R agonists protect endogenous GLP-1 from degradation by DDP4 and are used in the treatment of T2D (Drucker et al., 2010).
PPARs are essential regulators of energy metabolism. Among them, PPARγ is a major therapeutic target for glucose regulation. Interestingly, the expression level of PPARα was significantly increased, instead of that of PPARγ. The role of PPARα in modulating glucose homeostasis is unclear. PPARα-null mice were protected against HFD-induced insulin resistance (Guerre-Millo et al., 2001), and PPARα deficiency reduced insulin resistance in apoE-null mice (Tordjman et al., 2001). In contrast, the absence of PPARα increased hepatic glucose production from lactate and glycerol irrespective of the insulin level (Xu et al., 2002). Moreover, fibrates, which are selective PPARα activators, improved HFD-impaired insulin sensitivity, thereby suppressing the expression of carcinoembryonic antigen-related cell adhesion molecule 1 (CEACAM1) and promoting insulin clearance (Guerre-Millo et al., 2000; Ramakrishnan et al., 2016). PPARα regulates hepatic lipogenesis by modulating fatty acid uptake and oxidation (Pawlak et al., 2015). Insulin resistance is associated with hepatic steatosis and is a hallmark of non-alcoholic fatty liver (Chao et al., 2019). In this study, the HFD-induced increased liver weight and steatosis were significantly ameliorated by B. virosa treatment compared to the HFD group. Therefore, hepatic glucose regulation may be affected by fatty acid homeostasis via PPARα in the liver.
HFD-induced dysbiosis of the gut microbiota affects the expression of various genes related to energy metabolism, inflammation, and lipid profiles (Boulange et al., 2016; Teixeira et al., 2021). In this study, live B. virosa ameliorated the HFD-induced downregulation of mRNA levels of IRS-1, IRS-2, ZO-1, and TLR5 in the ileum, likely related to the activation of GLP-1R for glucose regulation in the liver. A lack of insulin receptors in the intestine contributes to the pathophysiological changes seen in patients with type II diabetes (Ussar et al., 2017). The intestinal epithelial barrier, which is related to dysbiosis of the gut microbiota, affects liver function and insulin resistance (Damms-Machado et al., 2017). ZO-1 is an important intracellular tight junction protein, whose disruption is linked to hyperglycemia (Thaiss et al., 2018). TLR5-deficient mice showed features of metabolic syndrome, including hyperglycemia and insulin resistance, which were significantly correlated with the altered gut microbiota (Vijay-Kumar et al., 2010).
Our findings suggest that B. virosa is implicated in glucose regulation in obese mice, but the absence of a mechanistic analysis was a limitation. We evaluated crosstalk between the gut microbiota and GLP-1R in the liver. Although B. virosa upregulated several mRNA transcripts in the intestine, we did not analyze downstream effects of GLP-1R activation in the liver. In addition, to generalize the anti-hyperglycemic effect of Butyricimonas, intervention studies using other animal models, including a non-obese diabetic mouse, are needed. Furthermore, investigation of Butyricimonas-induced changes in the gut microbiota under a variety of conditions would provide insight into the effect of Butyricimonas on glucose regulation.
Conclusion
Treatment with live and heat-killed B. virosa ameliorated changes in body weight, serum glucose level, insulin resistance, and liver steatosis in a mouse model of HFD-induced obesity. Moreover, GLP-1R in the liver was activated, which may be linked to in the metabolic improvements induced by Butyricimonas. Our results provide a rationale for the development of pharmabiotics based on Butyricimonas spp. for the prevention and treatment of type II diabetes.
Data Availability Statement
The original contributions presented in the study are included in the article/supplementary material, further inquiries can be directed to the corresponding author.
Ethics Statement
The animal study was reviewed and approved by Institutional Animal Care and Use Committee (IACUC) of Sahmyook University (SYUIACUC 2019-004).
Author Contributions
HL and KK: concept and design. JA, JK, DC, and HK: mouse model. HL, JA, JK, and DC: analysis or interpretation of data. HL: drafting of the manuscript. YS, CKL, HK, SBK, and KK: critical revision of the manuscript. All authors contributed to the article and approved the submitted version.
Conflict of Interest
The authors declare that the research was conducted in the absence of any commercial or financial relationships that could be construed as a potential conflict of interest.
Publisher’s Note
All claims expressed in this article are solely those of the authors and do not necessarily represent those of their affiliated organizations, or those of the publisher, the editors and the reviewers. Any product that may be evaluated in this article, or claim that may be made by its manufacturer, is not guaranteed or endorsed by the publisher.
Acknowledgments
This manuscript has been edited by a native English speaker.
References
Boulange, C. L., Neves, A. L., Chilloux, J., Nicholson, J. K., and Dumas, M. E. (2016). Impact of the gut microbiota on inflammation, obesity, and metabolic disease. Genome Med. 8:42. doi: 10.1186/s13073-016-0303-2
Burcelin, R., Gourdy, P., and Dalle, S. (2014). GLP-1-based strategies: a physiological analysis of differential mode of action. Physiology 29, 108–121. doi: 10.1152/physiol.00009.2013
Chao, H. W., Chao, S. W., Lin, H., Ku, H. C., and Cheng, C. F. (2019). Homeostasis of glucose and lipid in non-alcoholic fatty liver disease. Int. J. Mol. Sci. 20:298. doi: 10.3390/ijms20020298
Cuervo, A., Salazar, N., Ruas-Madiedo, P., Gueimonde, M., and Gonzalez, S. (2013). Fiber from a regular diet is directly associated with fecal short-chain fatty acid concentrations in the elderly. Nutr. Res. 33, 811–816. doi: 10.1016/j.nutres.2013.05.016
Damms-Machado, A., Louis, S., Schnitzer, A., Volynets, V., Rings, A., Basrai, M., et al. (2017). Gut permeability is related to body weight, fatty liver disease, and insulin resistance in obese individuals undergoing weight reduction. Am. J. Clin. Nutr. 105, 127–135. doi: 10.3945/ajcn.116.131110
Den Besten, G., Van Eunen, K., Groen, A. K., Venema, K., Reijngoud, D. J., and Bakker, B. M. (2013). The role of short-chain fatty acids in the interplay between diet, gut microbiota, and host energy metabolism. J. Lipid Res. 54, 2325–2340. doi: 10.1194/jlr.R036012
Depommier, C., Everard, A., Druart, C., Plovier, H., Van Hul, M., Vieira-Silva, S., et al. (2019). Supplementation with Akkermansia muciniphila in overweight and obese human volunteers: a proof-of-concept exploratory study. Nat. Med. 25, 1096–1103. doi: 10.1038/s41591-019-0495-2
Depommier, C., Van Hul, M., Everard, A., Delzenne, N. M., De Vos, W. M., and Cani, P. D. (2020). Pasteurized Akkermansia muciniphila increases whole-body energy expenditure and fecal energy excretion in diet-induced obese mice. Gut Microbes 11, 1231–1245. doi: 10.1080/19490976.2020.1737307
Derrien, M., Belzer, C., and De Vos, W. M. (2017). Akkermansia muciniphila and its role in regulating host functions. Microb. Pathog. 106, 171–181. doi: 10.1016/j.micpath.2016.02.005
Drucker, D. J., Sherman, S. I., Gorelick, F. S., Bergenstal, R. M., Sherwin, R. S., and Buse, J. B. (2010). Incretin-based therapies for the treatment of type 2 diabetes: evaluation of the risks and benefits. Diabetes Care 33, 428–433. doi: 10.2337/dc09-1499
Fu, J., Bonder, M. J., Cenit, M. C., Tigchelaar, E. F., Maatman, A., Dekens, J. A., et al. (2015). The gut microbiome contributes to a substantial proportion of the variation in blood lipids. Circ. Res. 117, 817–824. doi: 10.1161/CIRCRESAHA.115.306807
Gao, F., Lv, Y. W., Long, J., Chen, J. M., He, J. M., Ruan, X. Z., et al. (2019). Butyrate improves the metabolic disorder and gut microbiome Dysbiosis in mice induced by a high-fat diet. Front. Pharmacol. 10:1040. doi: 10.3389/fphar.2019.01040
Guerre-Millo, M., Gervois, P., Raspe, E., Madsen, L., Poulain, P., Derudas, B., et al. (2000). Peroxisome proliferator-activated receptor alpha activators improve insulin sensitivity and reduce adiposity. J. Biol. Chem. 275, 16638–16642. doi: 10.1074/jbc.275.22.16638
Guerre-Millo, M., Rouault, C., Poulain, P., Andre, J., Poitout, V., Peters, J. M., et al. (2001). PPAR-alpha-null mice are protected from high-fat diet-induced insulin resistance. Diabetes 50, 2809–2814. doi: 10.2337/diabetes.50.12.2809
Gupta, N. A., Mells, J., Dunham, R. M., Grakoui, A., Handy, J., Saxena, N. K., et al. (2010). Glucagon-like peptide-1 receptor is present on human hepatocytes and has a direct role in decreasing hepatic steatosis in vitro by modulating elements of the insulin signaling pathway. Hepatology 51, 1584–1592. doi: 10.1002/hep.23569
Hansotia, T., Baggio, L. L., Delmeire, D., Hinke, S. A., Yamada, Y., Tsukiyama, K., et al. (2004). Double incretin receptor knockout (DIRKO) mice reveal an essential role for the enteroinsular axis in transducing the glucoregulatory actions of DPP-IV inhibitors. Diabetes 53, 1326–1335. doi: 10.2337/diabetes.53.5.1326
Jang, E. Y., Ahn, Y., Suh, H. J., Hong, K. B., and Jo, K. (2020). Amylase-producing Maltooligosaccharide provides potential relief in rats with Loperamide-induced constipation. Evid. Based Complement. Alternat. Med. 2020, 1–9. doi: 10.1155/2020/5470268
Kim, T. H., Kim, M. K., Cheong, Y. H., Chae, Y. N., Lee, Y., Ka, S. O., et al. (2016). Hepatic role in an early glucose-lowering effect by a novel dipeptidyl peptidase 4 inhibitor, evogliptin, in a rodent model of type 2 diabetes. Eur. J. Pharmacol. 771, 65–76. doi: 10.1016/j.ejphar.2015.11.029
Kim, J., Lee, H., An, J., Song, Y., Lee, C. K., Kim, K., et al. (2019). Alterations in gut microbiota by statin therapy and possible intermediate effects on hyperglycemia and hyperlipidemia. Front. Microbiol. 10:1947. doi: 10.3389/fmicb.2019.01947
Lee, H., Lee, Y., Kim, J., An, J., Lee, S., Kong, H., et al. (2018). Modulation of the gut microbiota by metformin improves metabolic profiles in aged obese mice. Gut Microbes 9, 155–165. doi: 10.1080/19490976.2017.1405209
MacDonald, P. E., El-Kholy, W., Riedel, M. J., Salapatek, A. M., Light, P. E., and Wheeler, M. B. (2002). The multiple actions of GLP-1 on the process of glucose-stimulated insulin secretion. Diabetes 51:S434. doi: 10.2337/diabetes.51.2007.S434
Martin, R., Miquel, S., Benevides, L., Bridonneau, C., Robert, V., Hudault, S., et al. (2017). Functional characterization of novel Faecalibacterium prausnitzii strains isolated from healthy volunteers: A step forward in the use of F. prausnitzii as a next-generation probiotic. Front. Microbiol. 8:1226. doi: 10.3389/fmicb.2017.01226
Matthews, D. R., Hosker, J. P., Rudenski, A. S., Naylor, B. A., Treacher, D. F., and Turner, R. C. (1985). Homeostasis model assessment: insulin resistance and beta-cell function from fasting plasma glucose and insulin concentrations in man. Diabetologia 28, 412–419. doi: 10.1007/BF00280883
Nauck, M. A., Kind, J., Kothe, L. D., Holst, J. J., Deacon, C. F., Broschag, M., et al. (2016). Quantification of the contribution of GLP-1 to mediating insulinotropic effects of DPP-4 inhibition With Vildagliptin in healthy subjects and patients With type 2 diabetes using Exendin [9–39] as a GLP-1 receptor antagonist. Diabetes 65, 2440–2447. doi: 10.2337/db16-0107
Nguyen, T. T. B., Jin, Y. Y., Chung, H. J., and Hong, S. T. (2017). Pharmabiotics as an emerging medication for metabolic syndrome and its related diseases. Molecules 22:795. doi: 10.3390/molecules22101795
Okubo, H., Nakatsu, Y., Kushiyama, A., Yamamotoya, T., Matsunaga, Y., Inoue, M. K., et al. (2018). Gut microbiota as a therapeutic target for metabolic disorders. Curr. Med. Chem. 25, 984–1001. doi: 10.2174/0929867324666171009121702
Pawlak, M., Lefebvre, P., and Staels, B. (2015). Molecular mechanism of PPARalpha action and its impact on lipid metabolism, inflammation and fibrosis in non-alcoholic fatty liver disease. J. Hepatol. 62, 720–733. doi: 10.1016/j.jhep.2014.10.039
Ramakrishnan, S. K., Russo, L., Ghanem, S. S., Patel, P. R., Oyarce, A. M., Heinrich, G., et al. (2016). Fenofibrate decreases insulin clearance and insulin secretion to maintain insulin sensitivity. J. Biol. Chem. 291, 23915–23924. doi: 10.1074/jbc.M116.745778
Ridaura, V. K., Faith, J. J., Rey, F. E., Cheng, J., Duncan, A. E., Kau, A. L., et al. (2013). Gut microbiota from twins discordant for obesity modulate metabolism in mice. Science 341:1241214. doi: 10.1126/science.1241214
Sakamoto, M., Takagaki, A., Matsumoto, K., Kato, Y., Goto, K., and Benno, Y. (2009). Butyricimonas synergistica gen. Nov., sp. nov. and Butyricimonas virosa sp. nov., butyric acid-producing bacteria in the family 'Porphyromonadaceae' isolated from rat faeces. Int. J. Syst. Evol. Microbiol. 59, 1748–1753. doi: 10.1099/ijs.0.007674-0
Sakamoto, M., Tanaka, Y., Benno, Y., and Ohkuma, M. (2014). Butyricimonas faecihominis sp. nov. and Butyricimonas paravirosa sp. nov., isolated from human faeces, and emended description of the genus Butyricimonas. Int. J. Syst. Evol. Microbiol. 64, 2992–2997. doi: 10.1099/ijs.0.065318-0
Salminen, S., Collado, M. C., Endo, A., Hill, C., Lebeer, S., Quigley, E. M. M., et al. (2021). The international scientific Association of Probiotics and Prebiotics (ISAPP) consensus statement on the definition and scope of postbiotics. Nat. Rev. Gastroenterol. Hepatol. 18, 649–667. doi: 10.1038/s41575-021-00440-6
Svegliati-Baroni, G., Saccomanno, S., Rychlicki, C., Agostinelli, L., De Minicis, S., Candelaresi, C., et al. (2011). Glucagon-like peptide-1 receptor activation stimulates hepatic lipid oxidation and restores hepatic signalling alteration induced by a high-fat diet in nonalcoholic steatohepatitis. Liver Int. 31, 1285–1297. doi: 10.1111/j.1478-3231.2011.02462.x
Teixeira, L. D., Torrez Lamberti, M. F., Debose-Scarlett, E., Bahadiroglu, E., Garrett, T. J., Gardner, C. L., et al. (2021). Lactobacillus johnsonii N6.2 and blueberry Phytophenols affect Lipidome and gut microbiota composition of rats Under high-fat diet. Front. Nutr. 8:757256. doi: 10.3389/fnut.2021.757256
Thaiss, C. A., Levy, M., Grosheva, I., Zheng, D., Soffer, E., Blacher, E., et al. (2018). Hyperglycemia drives intestinal barrier dysfunction and risk for enteric infection. Science 359, 1376–1383. doi: 10.1126/science.aar3318
Tolhurst, G., Heffron, H., Lam, Y. S., Parker, H. E., Habib, A. M., Diakogiannaki, E., et al. (2012). Short-chain fatty acids stimulate glucagon-like peptide-1 secretion via the G-protein-coupled receptor FFAR2. Diabetes 61, 364–371. doi: 10.2337/db11-1019
Tordjman, K., Bernal-Mizrachi, C., Zemany, L., Weng, S., Feng, C., Zhang, F., et al. (2001). PPARalpha deficiency reduces insulin resistance and atherosclerosis in apoE-null mice. J. Clin. Invest. 107, 1025–1034. doi: 10.1172/JCI11497
Ussar, S., Haering, M. F., Fujisaka, S., Lutter, D., Lee, K. Y., Li, N., et al. (2017). Regulation of glucose uptake and Enteroendocrine function by the intestinal epithelial insulin receptor. Diabetes 66, 886–896. doi: 10.2337/db15-1349
Vijay-Kumar, M., Aitken, J. D., Carvalho, F. A., Cullender, T. C., Mwangi, S., Srinivasan, S., et al. (2010). Metabolic syndrome and altered gut microbiota in mice lacking toll-like receptor 5. Science 328, 228–231. doi: 10.1126/science.1179721
Xu, J., Xiao, G., Trujillo, C., Chang, V., Blanco, L., Joseph, S. B., et al. (2002). Peroxisome proliferator-activated receptor alpha (PPARalpha) influences substrate utilization for hepatic glucose production. J. Biol. Chem. 277, 50237–50244. doi: 10.1074/jbc.M201208200
Keywords: Butyricimonas virosa, gut microbiota, GLP-1 receptor, hyperglycemia, PPARα
Citation: Lee H, An J, Kim J, Choi D, Song Y, Lee C-K, Kong H, Kim SB and Kim K (2022) A Novel Bacterium, Butyricimonas virosa, Preventing HFD-Induced Diabetes and Metabolic Disorders in Mice via GLP-1 Receptor. Front. Microbiol. 13:858192. doi: 10.3389/fmicb.2022.858192
Edited by:
Franck Carbonero, Washington State University Health Sciences Spokane, United StatesReviewed by:
Tiphaine Le Roy, INSERM UMRS1269 Nutrition et Obésités (Nutriomique), FranceBret Rust, Washington State University Spokane, United States
Copyright © 2022 Lee, An, Kim, Choi, Song, Lee, Kong, Kim and Kim. This is an open-access article distributed under the terms of the Creative Commons Attribution License (CC BY). The use, distribution or reproduction in other forums is permitted, provided the original author(s) and the copyright owner(s) are credited and that the original publication in this journal is cited, in accordance with accepted academic practice. No use, distribution or reproduction is permitted which does not comply with these terms.
*Correspondence: Kyungjae Kim, a2lta2pAc3l1LmFjLmty