- 1Department of Human Parasitology, School of Basic Medicine, Hubei University of Medicine, Shiyan, China
- 2Key Laboratory of Zoonosis Research, Ministry of Education, Institute of Zoonosis, College of Veterinary Medicine, Jilin University, Changchun, China
- 3Key Laboratory of Tropical Translational Medicine of Ministry of Education, Hainan Medical University, Haikou, China
- 4Hainan Medical University-The University of Hong Kong Joint Laboratory of Tropical Infectious Diseases, Hainan Medical University, Haikou, China
- 5Department of Pathogen Biology, Hainan Medical University, Haikou, China
Trichinellosis caused by Trichinella spiralis is a worldwide food-borne parasitic zoonosis. Several approaches have been performed to control T. spiralis infection, including veterinary vaccines, which contribute to improving animal health and increasing public health by preventing the transmission of trichinellosis from animals to humans. In the past several decades, many vaccine studies have been performed in effort to control T. spiralis infection by reducing the muscle larvae and adult worms burden. Various candidate antigens, selected from excretory-secretory (ES) products and different functional proteins involved in the process of establishing infection have been investigated in rodent or swine models to explore their protective effect against T. spiralis infection. Moreover, different types of vaccines have been developed to improve the protective effect against T. spiralis infection in rodent or swine models, such as live attenuated vaccines, natural antigen vaccines, recombinant protein vaccines, DNA vaccines, and synthesized epitope vaccines. However, few studies of T. spiralis vaccines have been performed in pigs, and future research should focus on exploring the protective effect of different types of vaccines in swine models. Here, we present an overview of the strategies for the development of effective T. spiralis vaccines and summarize the factors of influencing the effectiveness of vaccines. We also discuss several propositions in improving the effectiveness of vaccines and may provide a route map for future T. spiralis vaccines development.
Introduction
At present, the Trichinella genus comprises nine species and three genotypes that can be divided into encapsulated clade (Trichinella spiralis, T1; Trichinella nativa, T2; Trichinella britovi, T3; Trichinella murrelli, T5; Trichinella nelsoni, T7; Trichinella patagoniensis, T12; and Trichinella genotypes T6, T8, and T9) and non-encapsulated clade (Trichinella pseudospiralis, T4; Trichinella papuae, T10; and Trichinella zimbabwensis, T11) (Li et al., 2020; Feng et al., 2021). T. spiralis is an intracellular parasitic nematode that can infect a wide variety of mammals (Gottstein et al., 2009). Trichinellosis caused by T. spiralis is regarded as an emerging and re-emerging zoonotic parasitic disease in some parts of the world, such as in Argentina, Eastern Europe, and Asia (Cuperlovic et al., 2005; Devleesschauwer et al., 2017). Human can become infected with T. spiralis by ingestion of raw or poorly cooked meat containing the infective larvae of T. spiralis (Pozio, 2015). In humans, the clinical symptoms range from diarrhea and abdominal pain (intestinal phase) to fever, myalgia, myocarditis, and allergic reaction (muscular phase); in serious cases, facial edema and encephalitis may develop (Gottstein et al., 2009; Faber et al., 2015). Murrell and Pozio (2011) reported that 261 reports were selected from 494 reports for data extraction after applying strict criteria for relevance and reliability, and there are a total of 65,818 cases and 42 deaths were reported in 41 countries between 1986 and 2009. Although the husbandry condition of animals, meat inspection and public health and safety education have been enhanced to prevent trichinellosis, the International Commission on Trichinellosis (ICT) states that more than 11 million people are chronically infected with T. spiralis worldwide (Murrell and Pozio, 2011). China is one of the countries with the higher number of trichinellosis cases in the world. As the main source of human infection is domestic pigs and pork-related products, there is a need to develop an effective strategy to prevent T. spiralis transmission from pigs to humans (Cui et al., 2011; Cui et al., 2013a; Jiang et al., 2016). It is well-known that vaccines are among the most important ways to control diseases, and developing a vaccine against T. spiralis infection in pigs is a promising strategy to control T. spiralis infection.
To date, no effective vaccines are available to control T. spiralis infection, and therefore vaccine research should be intensified to prevent T. spiralis infection. The lack of progress in vaccine research against T. spiralis infection reflects both scientific obstacles, such as the complexity of life cycles and diversity of antigens, and policy deficiencies that have resulted in trichinellosis receiving little attention (Hewitson and Maizels, 2014). The developmental cycles of T. spiralis include three major stages: adult worms (AD), newborn larvae (NBL), and muscle larvae (ML) (Gottstein et al., 2009). Various antigenic components are expressed at each stage, and as such, a large number of antigens are available for vaccine research against T. spiralis infection. Furthermore, different life cycles of T. spiralis often occur in distinct tissues causing different immunological environments and pathological consequence. In general, vaccines should aim to block the development of infected larvae in the intestine, interrupt the growth of infected larvae to AD and expel AD from the intestine. Overall, the selection of adjuvants and the route of vaccination are associated with the effects of vaccines (Sander et al., 2020). Most studies of T. spiralis vaccines have been performed in murine models, whereas few studies have been conducted in swine models. Nevertheless, pigs are the natural host of T. spiralis, and studies have found that the immune responses induced by the same antigen are likely to differ between pigs and mice (Feng et al., 2013; Habiela et al., 2014; Xu et al., 2021). Feng et al. reported that mice vaccinated with a T. spiralis serine protease (rTs-Adsp) induced Th1-Th2 mixed immune response with Th2 predominant. However, Xu et al. reported that a Th1-Th2 mixed immune response with Th1 predominant was induced by rTs-Adsp after vaccination. Consequently, future studies of the development of T. spiralis vaccines should focus on pigs instead of mice.
Human trichinellosis is treated with anthelmintic drugs, such as mebendazole or albendazole. These drugs are considered relatively safe, except for pregnant women due to teratogenic effects (Shimoni and Froom, 2015). A vaccine for foodborne infection is the best method to control parasite infection, improve public health, and promote socioeconomic development (Hewitson and Maizels, 2014; McAllister, 2014). Compared with chemical treatment, vaccines have several advantages in the prevention of T. spiralis infection (Zhang N. et al., 2018). For example, vaccines provide long-term protection and eliminate the phenomenon of drug resistance of T. spiralis. Moreover, vaccines improve food safety by reducing drug residues in meat (Joachim, 2016; Sander et al., 2020). Studies have found that a window period between T. spiralis infection and anti-Trichinella IgG positivity (Gamble et al., 2004; Cui J. et al., 2015; Wang et al., 2017). There have been great efforts over the past few decades with respect to investigating the protective effect of T. spiralis vaccines through different strategies (Zhang N. et al., 2018). Vaccine development for T. spiralis infection is mainly divided into live attenuated vaccines, natural antigen vaccines, recombinant protein vaccines, DNA vaccines, and synthetic peptide vaccines. Although live attenuated vaccines elicit a strong immune response and protective immunity, their safety is doubtful. Inactivated vaccines are safe and have high protective effects, but antigen resources are limited due to the requirements of numerous animals. Currently, the development of T. spiralis vaccines focuses on recombinant vaccines and DNA vaccines. In this review, we will summarize the advances and future innovations in the development of T. spiralis vaccines as a strategy against trichinellosis.
Vaccines Against T. spiralis Infection
Traditional antigens of T. spiralis vaccines are derived from crude extracts of whole worms, excretory-secretory (ES) products. It is well-known that live attenuated vaccines and inactivated vaccines are first generation vaccines. With the development of genetic engineering methods, the strategy of genome, proteome, transcriptome, and immunoproteomics have been used to screen novel candidate antigens of T. spiralis vaccines (Figure 1). Based on these methods, second and third generation vaccines have been performed in rodent and swine models to evaluate their protective effect, such as recombinant protein vaccines, synthetic peptide vaccines and DNA vaccines. The strategies and protective effect of vaccines candidates against T. spiralis infection in different models are provided in Table 1.
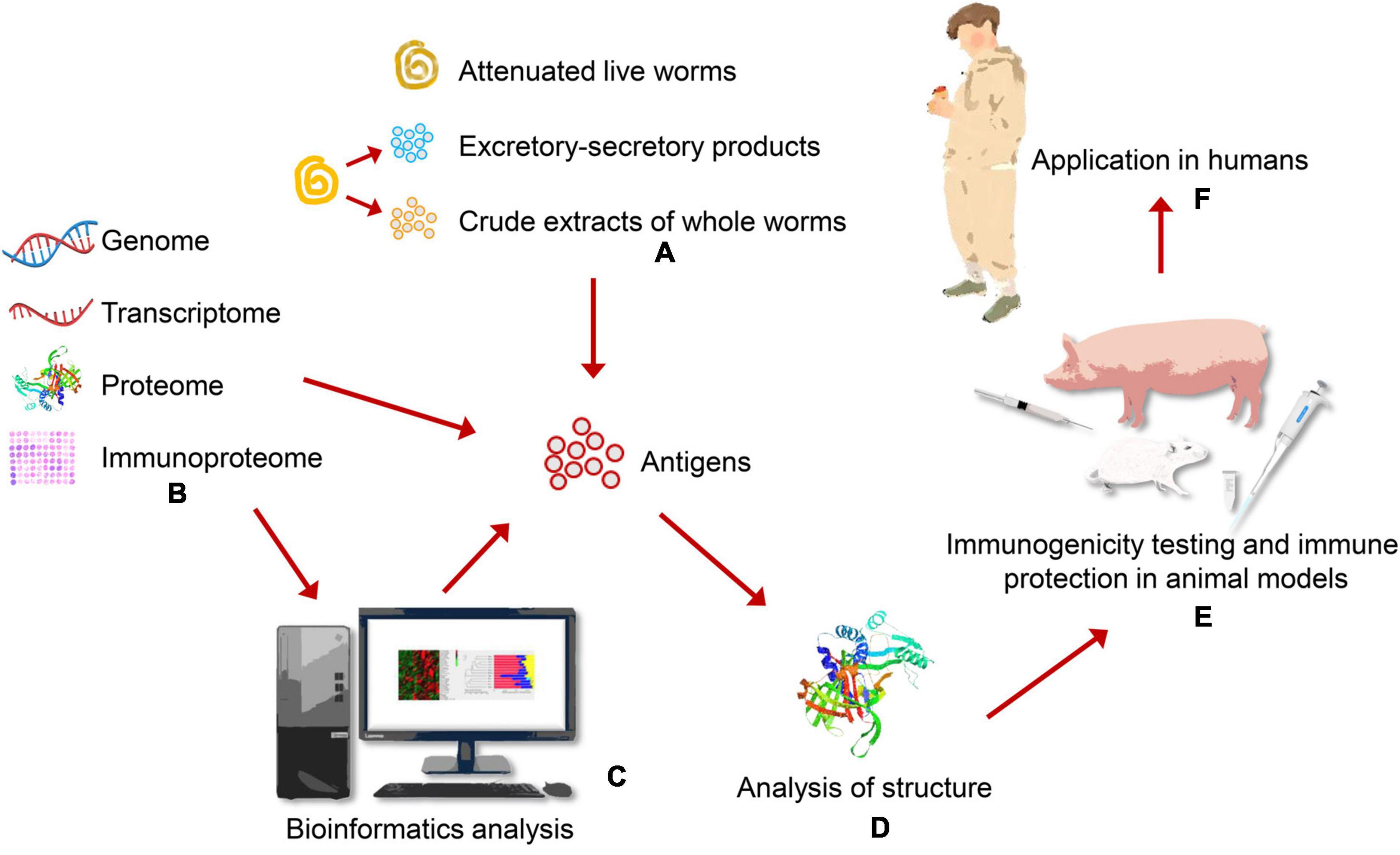
Figure 1. Discovery and identification vaccine candidates through different strategies in animal models. (A) Traditional Trichinella spiralis vaccines are derived from live attenuated vaccines, crude extracts of whole worms, excretory-secretory (ES) products. (B) Vaccine candidates can be screened through different strategies, including genome, transcriptome, proteome, immunoproteome. (C) Candidate antigens are selected for T. spiralis vaccines through bioinformatics analysis. (D) Analyzing structure of antigens contributes to designing effective vaccines in the future. (E) Immunogenicity and immune protection of vaccine candidates are identified through vitro and animal experiments. (F) High effective vaccines translate to meet people need and are applied in humans.
Live Attenuated Vaccines
Live attenuated vaccines were produced with radiation or drugs to reduce the pathogenicity of T. spiralis while maintaining immunogenicity. Experimental animals vaccinated with radiation-attenuated larvae exhibited an exciting reduction in muscle larvae burden (Cabrera and Gould, 1964; Agyei-Frempong and Catty, 1983; Hafez et al., 2020). For instance, Hafez et al. (2020) reported that mice vaccinated with radiation-attenuated larvae show a 72.5% reduction in muscle larvae burden, and Nakayama et al. (1998) found significant immunity in mice vaccinated with radiation-attenuated T. britovi larvae, with a strong worm reduction. Live attenuated vaccines perform high protective efficacy because they are similar to natural infection, mimicking an approximate microenvironment of T. spiralis infection. However, their safety is doubtful due to the potential of pathogenicity. The approach of live attenuated vaccines is gradually abandoned, even though it is associated with strong protective immunity.
Natural Antigens Vaccines
Crude Extracts of Whole Worms
Trichinella spiralis has three major antigenic stages in the same host, namely, AD, NBL, and ML. Mice inoculated with adult worms soluble antigens showed an 89% reduction in adult worms and a 96% reduction in muscle larvae (Gamble, 1985a). Marti et al. (1987) reported that crude T. spiralis newborn larvae antigens were treated under freeze-thawing condition resulted in 78% muscle larvae reduction in pigs vaccinated with the antigens. Immunofluorescence results have shown that antibodies from the serum of immunized pigs bind to the surface of newborn larvae. Conversely, it was reported that soluble components of newborn larvae treated by ultrasonication have not protective effect against T. spiralis infection (Marti et al., 1987). Eissa et al. (2003) reported that mice vaccinated with the autoclaved T. spiralis larvae exhibit a significant reduction in adult worms and muscle larvae. The results of histopathological examination showed degeneration and hyalinization in the T. spiralis cyst wall accompanied by pericystic fibrosis. Another study found that the combination of antigens from adult worms and muscle larvae elicited a 73% reduction in T. spiralis female fecundity, a 96% reduction in adult worms and an 86% reduction in muscle larvae (Darwish et al., 1996). Although worm antigens from different stages induce a surprising protective effect, antigen resources are limited due to the requirement of numerous animals. Moreover, worm antigens are mainly presented via MHC-II pathway and mostly induce humoral immune responses (Aida et al., 2021). High effective vaccines usually induce strong humoral and cell-mediated responses to expel parasites from the host’s intestine. With the development of technology, second and third generation vaccines spring up due to these disadvantages of inactivated vaccines.
Excretory-Secretory Products
The major origin of ES antigens are the stichosome and stichocytes (Gamble et al., 1983; Gamble et al., 1988). Here, we summarize the protective effect induced by crude ES products and components of ES products with a defined molecular size. After T. spiralis infection, ES antigens are directly exposed to the immune system of the host, and they are the target antigens that induce an immune response of host (Lightowlers and Rickard, 1988). Thus, ES antigens play a critical role in diagnosing and preventing trichinellosis (Todorova, 2000; Korinkova et al., 2008). According to Isao et al., the main components of ES products in muscle larvae consist of 43 kDa, 53 kDa, and 45 kDa glycoproteins, which are derived from stichosomes (Nagano et al., 2009). Studies on ES products of T. spiralis contribute to understanding the interaction between host and parasite, and in developing vaccines against T. spiralis infection.
Gamble et al. (1986) reported that pigs vaccinated with the ES antigen of muscle larvae had a significant reduction in female fecundity and a 57% reduction in muscle larvae. Moreover, Gamble (1985b) found significant levels of protection in mice immunized with an antigen isolated from ES products. Although ES antigens keep natural activity and have excellent immunogenicity, ES antigen resources require large numbers of animals. With the development of science and technology, some new strategies have been applied to screen antigens in ES products, such as proteomics and immunoblotting (Liu et al., 2016a,b), and in most studies, the antigen genes screened from ES products are cloned and expressed in a prokaryotic expression system. In the future, more potential immunogenic antigens should be selected from among ES products, and the protective effect of these antigens should be further explored.
Surface Antigens
Trichinella spiralis surface proteins are directly exposed to the immune system of the host and may play an important role in the process of T. spiralis invasion (Cui et al., 2013b; Liu R. D. et al., 2014). Grencis et al. (1986) reported that several surface antigens are stripped from the cuticle of T. spiralis larvae and that the relative molecular masses of the antigens are 100 kDa, 90 kDa, 69 kDa, 55 kDa, 46 kDa, and 35 kDa. Moreover, mice immunized with surface antigens showed a reduction in adult worms, muscle larvae and female fecundity. In the study of Ortega-Pierres et al. (1989), several antigens extracted from T. spiralis larvae displayed the same structural features and surface antigens conferred partial protection against T. spiralis infection in mice. Aquaporins (AQPs), also known as water channel proteins, are components of many parasite membranes (Benga, 2009; Faghiri and Skelly, 2009). An aquaporin gene from T. spiralis (TsAQP) has been identified, and the TsAQP protein is predicted to contain six linear B-cell epitopes, suggesting it is a promising antigen for vaccines (Cui J. M. et al., 2015).
Surface antigens of T. spiralis are complex, because they may change during the process of worm molting and growth (Bolas-Fernandez and Corral Bezara, 2006). Surface antigens comprise a series of proteins of different biological processes, such as immune reactions, adhesion molecules, and enzyme (Cui et al., 2013b). These antigens are important modulators of the host immune system, and studies of intestinal immunity have revealed that these antigens play a critical role during the process of T. spiralis invasion and development. Surface antigens of T. spiralis have been identified and characterized by two-dimensional gel electrophoresis (2-DE), mass spectrometry and immunoproteomics (Cui et al., 2013b; Liu R. D. et al., 2014). Research on surface antigens contributes to understanding the host-parasite interaction and identifying target antigens for detection, diagnosis and vaccine development (Bolas-Fernandez and Corral Bezara, 2006; Cui et al., 2013b; Liu R. D. et al., 2014).
Recombinant Protein Vaccines
Certain progress in the development of recombinant protein vaccines against T. spiralis infection has been achieved with the rapid development of genetic engineering. Candidate antigens have mainly been screened from ES products, functional proteins and antigens involved in the processes of T. spiralis invasion. Protease and protease inhibitor are the most important components of ES products involved in T. spiralis infection. In recent years, a large amount of protein vaccine research has been performed on serine proteases, serine proteases inhibitors, cystatins and deoxyribonuclease to control T. spiralis infection.
Proteases
Serine proteases from ES products are thought to be key factors in T. spiralis invasion of host cells and in processes of immune evasion (Dzik, 2006). Sun et al. (2019) reported that mice vaccinated with recombinant T. spiralis serine protease (rTsSP) showed 71.10 and 62.10% reduced worm burdens of AD and ML, and Wang et al. (2013) reported that mice immunized with recombinant T. spiralis serine protease (rTspSP-1.2) displayed 34.92 and 52.24% reduced worm burdens of AD and ML. A previous study in our laboratory has found that mice vaccinated with recombinant T. spiralis serine protease (rTs-Adsp) exhibited a 46.5% reduction in muscle larvae (Feng et al., 2013). Further research showed a 50.9% reduced worm burden of ML in pigs immunized with rTs-Adsp (Xu et al., 2021). Furthermore, cysteine proteases of parasites are also a focus of attention for parasite vaccines. Cysteine proteases are essential hydrolases present in most organisms, such as viruses and parasites. Cysteine proteases of parasites play an important role in invasion of host tissue and maintenance of parasite survival in the host, rendering them a major target for the development of parasite vaccines (Grote et al., 2018).
Proteases Inhibitor
Serine proteases inhibitors (serpins) are a superfamily of proteins that suppress the activity of serine proteases and play an important role in blood coagulation, inflammation, and complement activation (Molehin et al., 2012). The serpins secreted by worms protect them from the serine proteolysis of the host, and thus, they help parasites overcome defensive barriers and avoid host immune attack (Dzik, 2006). Mice vaccinated with recombinant T. spiralis serine protease inhibitor (rTsSPI) exhibited 62.2 and 57.25% reduced worm burdens of AD and ML, in the study by Song et al. (2018), and a 59.95% reduction in adult worms at 10 days post-infection (dpi) and a 46.41% reduction in muscle larvae were detected in mice immunized with recombinant T. spiralis serpin (rTs-Serpin) according to Xu et al. (2017b). Cystatins are a superfamily of proteins that specifically inhibit cysteine protease activity (Brown and Dziegielewska, 1997). In nematodes, cystatins play a crucial role in immune evasion and regulate the host immune response during parasite infection (Vray et al., 2002; Hartmann and Lucius, 2003). Tang et al. (2015) reported that mice vaccinated with a cystatin-like protein (Ts-CLP) exhibited a 64.28% reduction in adult worms at 5 dpi and a 61.21% reduction in muscle larvae. Liu X. D. et al. (2014) found that oral administration of a cystatin-like protein to mice resulted in a 91% reduction in the parasite female fecundity.
Deoxyribonuclease II
DNase II mainly exists in lysosomes and nuclei, and plays an important role in pathogen invasion and evasion of the immune response of the host. Compared to DNase II in other species, the DNase II protein family of T. spiralis has significantly enlarged. Moreover, studies have found that DNase enzymes of T. spiralis may play a key role in parasite-host interactions during infection, suggesting that they can be used as candidate antigens to control and prevent trichinellosis (Liu R. D. et al., 2015; Liu et al., 2016b). Qi et al. (2018) reported that mice subcutaneously vaccinated with rTsDNase II-1 and rTs-DNase II-7 showed 40.36 and 34.86% reductions in adult worms at 5 dpi and 50.43 and 42.33% reductions in muscle larvae, respectively. A previous study by our laboratory found that pigs vaccinated with DNase II-7 recombinant protein showed a 45.7% reduction in muscle larvae (Xu et al., 2020b). Although recombinant protein vaccines have become increasingly popular, the level of immunoprotection is still associated with the antigens, adjuvants, and delivery routes.
DNA Vaccines
DNA vaccines have become more attractive due to their ability to induce a broad immune response and long-lasting immunity (Gu et al., 2014). Additionally, compared to conventional protein vaccines, DNA vaccines are much more stable, cost efficient, simple to produce, and safe for use (Prazeres and Monteiro, 2014; Kumar and Samant, 2016). Compared to protein vaccines, the main disadvantage of DNA vaccines is poor immunogenicity (Li et al., 2012). Furthermore, designing a vaccine suitable for humans is a great challenge for DNA vaccines against parasites. Many studies of DNA vaccines against parasites are focused on murine models, but the results cannot be applied in humans (Kumar and Samant, 2016).
Many DNA vaccines against T. spiralis infection have recently been developed in murine models (Gu et al., 2014; Xu et al., 2017a,2020a). In the study of Wang L. et al. (2016), mice vaccinated with a Salmonella-delivered TsPmy DNA vaccine showed 44.8 and 46.6% reduced worm burdens of AD and ML, respectively; Xu et al. (2017a) found a 77.93% reduced worm burden of ML in mice vaccinated with the pcDNA3.1(+)-Ts-NBLsp DNA vaccine. Overall, compared to protein vaccines, the immunogenicity of DNA vaccines is poor due to low levels of antigens expression. Studies have found that DNA plus protein vaccination is an ideal strategy for improving the immune response and protective effect. Gu et al. (2014) reported that mice immunized with Ts87 in a DNA-prime/protein-boost strategy experienced a 46.1% reduced worm burden of ML. In a previous study in our laboratory, mice immunized with pcDNA3.1(+)-Adsp/rTs-Adsp exhibited a 69.50% reduced worm burden of ML (Xu et al., 2020a). With the rapid development of technologies, more methods will be performed to enhance the efficacy of DNA vaccines. The ultimate goal is to develop a DNA vaccine that can be safely applied in humans.
Synthetic Peptide Vaccines
In the past several decades, much research has been carried out to develop vaccines against T. spiralis infection, such as crude antigens, recombinant proteins, and DNA vaccines. However, there are only a few studies on controlling T. spiralis infection utilizing peptide vaccines. Compared to recombinant protein vaccines, multiepitope peptides have advantages of being easy and fast to produce and may include of multiple protective epitopes (Gu et al., 2020). Robinson et al. (1995) screened a 40-mer synthetic peptide from the glycoprotein of T. spiralis, and mice vaccinated with the peptide vaccine exhibited a 64.3% reduction in adult worms, and McGuire et al. (2002) reported a 33.3% reduction in parasite female fecundity in mice immunized with a 30-mer peptide antigen.
In recent years, epitope vaccines against viral, bacterial or even parasitic infection have been rapidly developed. However, epitope vaccines also have some disadvantages: they have poor immunogenicity and need to be conjugated to a large carrier protein. A new strategy of multiple antigenic peptides was developed to improve the immunogenicity of epitope vaccines. Epitope-based vaccines can be constructed as chimeric vaccines by engineering multiple effective epitopes (Gu et al., 2013). Consequently, a chimeric vaccine may improve the level of epitope vaccine protection or prevent parasite immune evasion. Moreover, the life cycle of T. spiralis is complex leading to various antigens at different stages. A multiepitope vaccine is an effective way to control T. spiralis infection.
Factors of Vaccine Effectiveness
There are many factors that influence the effectiveness of vaccines, such as the composition of antigens, adjuvants, inoculation doses, delivery routes, infective doses, coinfection, animal species, and vaccination protocol (Figure 2). T. spiralis has a multistage life cycle, resulting in different antigens at different stages. The composition of antigens determines the effectiveness of vaccines thus identification of excellent antigens is crucial for developing T. spiralis vaccines. Different candidate antigens elicit distinct immune response and protective effects. During Trichinella infection, Th2-type cytokines are secreted by hosts to promote mast-cell activation and proliferation, which are important for expelling parasite from intestine (Zhang N. et al., 2018). Future studies of the development of T. spiralis vaccines should focus on the antigens that could elicit Th2-type immune response. Selecting a suitable adjuvant plays a very important role in vaccine development (Burakova et al., 2018). Adjuvants enhance immune responses induced by parasite antigens, and protect the antigens from being diluted, degraded and eliminated by the host (Stills, 2005). To date, the adjuvants used in experiments include Freund’s adjuvant, aluminum hydroxide and emulsions containing water in oil, oil in water, and multiphasic formulations. Freund’s adjuvant has been thought to be the gold standard adjuvant and has been used widely in many studies. Nevertheless, the application of Freund’s adjuvant is gradually limited due to its toxic effect and specific damage to experimental animals. Although few adjuvants surpass Freund’s adjuvant in inducing antibody production, many adjuvants can also induce high antibody responses with less inflammation and tissue destruction. In recent decades, alternative adjuvants have been evaluated against T. spiralis infection in murine models, including Montanide ISA series adjuvants and Montanide™ IMS series adjuvants. Montanide™ IMS series adjuvants containing water-dispersed liquid nanoparticles combined with an immunostimulating compound are comparatively non-toxic and have been employed in research on T. spiralis vaccines (Jang et al., 2011; Xu et al., 2017b). Yang et al. (2010) reported that recombinant Ts-Pmy formulated with Montanide ISA206 or ISA720 induced similar levels of immune responses and protection, compared to that of Freund’s adjuvant formulation group.
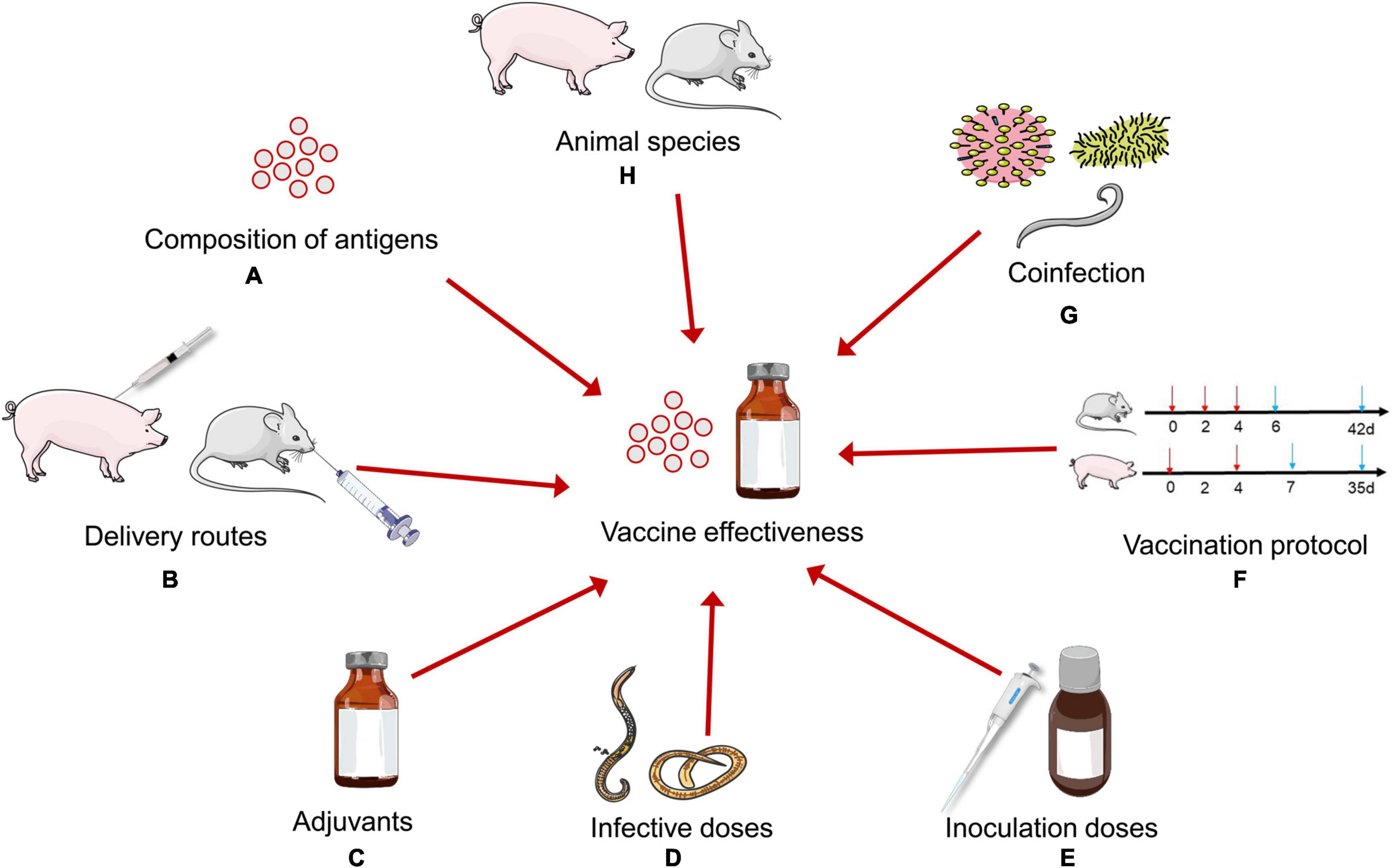
Figure 2. Factors influencing the effectiveness of Trichinella spiralis vaccines in animal models. (A) Various candidate antigens show different protective effects. (B) Several vaccine delivery routes have been used to improve the effectiveness of T. spiralis vaccines. (C) Suitable adjuvants play a very important role in vaccines effectiveness. (D) Infective doses of T. spiralis may determine the effectiveness of T. spiralis vaccines in animal models. (E) The efficiency of immunization also depends on inoculation doses of vaccines. (F) There is a lack of a standardized vaccination protocol in animal models. (G) Coinfection change the host’s immune response to affect the effectiveness of T. spiralis vaccines. (H) The same antigen may exhibit different protective effects in different animal models.
Although the adjuvant is very important, the efficiency of immunization also depends on inoculation doses. Regarding dose, Gamble (1985a) reported that mice immunized with 10 μg antigen exhibited a 77% reduction in muscle larvae but that mice immunized with 100 μg antigen displayed a 98% reduction in muscle larvae. In most research, the dose of vaccination in a murine model has varied from 20 to 100 μg. Moreover, few studies have been performed in pig to explore the dose of vaccination and hence there are no standard inoculation doses in pig models. Although mice were immunized with the same antigen formulated with different adjuvants and delivery routes, the immune protection induced differed. At present, vaccine delivery routes include subcutaneous, intradermal, intraperitoneal, intramuscular, intranasal, and oral inoculation. The strategy of encoding T. spiralis antigens by the attenuated Salmonella and recombinant Lactobacillus plantarum has been widely used in the development of T. spiralis vaccines, which have been proven to induce mucosal immunity (Liu X. D. et al., 2014; Liu P. et al., 2015; Wang L. et al., 2016; Hu et al., 2021). The model of T. spiralis infection involves the intestinal mucosa, and its target is to induce a local immune response. Studies have found that oral or intranasal vaccination can activate systemic and mucosal immune responses. Therefore, vaccination through oral or intranasal routes can be effective, inducing a broad mucosal immune response (Ortega-Pierres et al., 2015).
In most research, the dose of infection in a murine model has varied from 200 to 400 T. spiralis ML. In a pig model, Marti et al. (1987) reported that pigs were challenged 2000 T. spiralis ML to evaluate the protective effect of antigen. However, Xu et al. (2020b,2021) reported that pigs were inoculated with 5000 T. spiralis ML by oral administration to evaluate the effectiveness of vaccines. The effectiveness of the same candidate antigen may be influenced by infective dose in animal models. Moreover, whether or not the dose of infection is similar to natural infection remains to be explored. In the natural setting, bacteria, viruses and parasites may exist in one host (Wang et al., 2019). Coinfection may change the host’s immune response to affect the effectiveness of T. spiralis vaccines. Whether or not immune response induced by T. spiralis vaccines can be suppressed or neutralized by other pathogens infection remains to be determined. Understanding the immune response induced by multiple pathogens should be a part of T. spiralis vaccines development in future. It is well known that animal models play crucial roles in developing vaccines. Most studies on protective effect of vaccines have focused on murine models rather than on pig models. However, the effectiveness of vaccines may vary depending on animal species. Previous studies of our laboratory have found that immune response induced by the same antigen is different between pigs and mice (Feng et al., 2013; Xu et al., 2021). Therefore, high levels of protection induced by candidate antigens in murine models should be further verified in swine models. Considerable efforts have been made to design and verify various vaccination strategies. Although there have been mature vaccination regimens in murine models, they may not be available for pigs or humans. So far, there has been a lack of a standardized protocol in swine models. Consequently, the factors influenced the effectiveness of vaccines should be strongly considered for T. spiralis vaccines development in future.
Future Perspectives and Challenges
Although substantial efforts and progresses have been made to search for candidate antigens and develop T. spiralis vaccines, there is still no effective vaccines to prevent T. spiralis infection. With the development of genomics, proteomics, transcriptomics, more immunogenic antigens have been isolated and identified to develop effective Trichinella vaccines. More and more strategies have been applied to increase the effectiveness of vaccines. Currently, DNA vaccines are becoming more appealing due to their several advantages, such as cost-effectiveness, stable, long-lasting immunity. Regarding toxoplasmosis vaccines, a DNA multicomponent vaccine showed an 80.22% reduction in the parasite cyst burden (Zhang N. G.et al., 2018). The cocktail DNA vaccine may be a promising strategy to improve the effectiveness of T. spiralis vaccines. Moreover, combination vaccination has been used as a promising approach to improve the effectiveness of T. spiralis vaccines in murine models. The strategy of DNA plus protein vaccination exerts the advantages of DNA and protein vaccines to induce high levels of immune response and immune protection. Virus-like particle (VLP) vaccines are one of most popular strategy due to their ability to induce strong immune responses (Pitoiset et al., 2015). The approach has been applied in the field of toxoplasmosis vaccines, and provides a novel idea for the development of T. spiralis vaccines. With the development of genetic engineering approaches, the strategy of gene editing has been used to develop live-attenuated toxoplasmosis vaccines (Wang J. L.et al., 2016). Although physiological characters are different between T. spiralis and Toxoplasma gondii, the strategy can be used as a reference for developing an attenuated Trichinella vaccine.
The life cycle of T. spiralis in the host is complex, including a diversity of antigens, immune evasion, and regulation of host response. These characteristics make it difficult to achieve the ideal protection with a single T. spiralis antigen. The ideal vaccine should promote the process of expelling AD and inhibit the production of NBL and the formation of ML. Moreover, the immune response induced by vaccines should have the ability to disable, degrade, and dislodge the parasites (Maizels et al., 2012). With the development of genetics, new research ideas are being proposed to improve the protection rate of vaccines against T. spiralis infection. In-depth research on immunosuppression and immune evasion caused by T. spiralis infection will contribute to the design of more effective vaccines against T. spiralis infection.
The main source of human T. spiralis infection is pork and pork-related products. To date, most research on T. spiralis vaccines has been performed in murine models, and more research in pigs should be conducted. The financial and technical issues associated with T. spiralis vaccines using swine models make research difficult, but this is a key factor in many vaccinology studies. As the risk of livestock infection with T. spiralis is negligible under reasonable management condition, the development of vaccines against T. spiralis infection has received little attention. Regardless, vaccines against T. spiralis infection are a safe tool that could avoid drug resistance. Therefore, it is crucial to educate the public on the importance of vaccination and its benefit.
Conclusion
A successful vaccine for trichinellosis depends on a thorough consideration of immune response caused by T. spiralis infection and the factors that influence the effectiveness of vaccines. Finally, research on the protective effect of T. spiralis vaccines should focus on pig infection models. To conclude, vaccines are a promising strategy to control trichinellosis.
Author Contributions
DX, BT, and TL provided the ideas and wrote the draft manuscript. JL, YX, WG, YZ, and SY contributed to the revising of the manuscript. ML and DX approved the version to be published. All authors read and approved the final manuscript.
Funding
This study was supported by Cultivating Project for Young Scholar at Hubei University of Medicine (2021QDJZR012) and the Principle Investigator Program of Hubei University of Medicine (HBMUPI202101).
Conflict of Interest
The authors declare that the research was conducted in the absence of any commercial or financial relationships that could be construed as a potential conflict of interest.
Publisher’s Note
All claims expressed in this article are solely those of the authors and do not necessarily represent those of their affiliated organizations, or those of the publisher, the editors and the reviewers. Any product that may be evaluated in this article, or claim that may be made by its manufacturer, is not guaranteed or endorsed by the publisher.
Acknowledgments
We thank the staff of the Pathogen biology laboratory. Our thanks are also extended to express our gratitude to all the people who made this work.
References
Agyei-Frempong, M., and Catty, D. (1983). The measurement of antigens released by radiation-attenuated Trichinella spiralis larvae. Parasite Immunol. 5, 289–303. doi: 10.1111/j.1365-3024.1983.tb00745.x
Aida, V., Pliasas, V. C., Neasham, P. J., North, J. F., McWhorter, K. L., Glover, S. R., et al. (2021). Novel vaccine technologies in veterinary medicine: a herald to human medicine vaccines. Front. Vet. Sci. 8:654289. doi: 10.3389/fvets.2021.654289
Benga, G. (2009). Water channel proteins (Later Called Aquaporins) and relatives: past, present, and future. IUBMB Life 61, 112–133. doi: 10.1002/iub.156
Bolas-Fernandez, F., and Corral Bezara, L. D. (2006). TSL-1 antigens of Trichinella: an overview of their potential role in parasite invasion, survival and serodiagnosis of trichinellosis. Res. Vet. Sci. 81, 297–303. doi: 10.1016/j.rvsc.2006.01.002
Brown, W. M., and Dziegielewska, K. M. (1997). Friends and relations of the cystatin superfamily–new members and their evolution. Protein Sci. 6, 5–12. doi: 10.1002/pro.5560060102
Burakova, Y., Madera, R., McVey, S., Schlup, J. R., and Shi, J. (2018). Adjuvants for Animal Vaccines. Viral Immunol. 31, 11–22.
Cabrera, P. B., and Gould, S. E. (1964). Resistance to Trichinosis in Swine Induced by Administration of Irradiated Larvae. J. Parasitol. 50, 681–684.
Cui, J. M., Zhang, N. Z., Li, W. H., Yan, H. B., and Fu, B. Q. (2015). Cloning, identification, and bioinformatics analysis of a putative aquaporin TsAQP from Trichinella spiralis. Genet. Mol. Res. 14, 12699–12709. doi: 10.4238/2015.October.19.14
Cui, J., Jiang, P., Liu, L. N., and Wang, Z. Q. (2013a). Survey of Trichinella infections in domestic pigs from northern and eastern Henan, China. Vet. Parasitol. 194, 133–135. doi: 10.1016/j.vetpar.2013.01.038
Cui, J., Liu, R. D., Wang, L., Zhang, X., Jiang, P., Liu, M. Y., et al. (2013b). Proteomic analysis of surface proteins of Trichinella spiralis muscle larvae by two-dimensional gel electrophoresis and mass spectrometry. Parasites Vectors 6:355. doi: 10.1186/1756-3305-6-355
Cui, J., Wang, L., Sun, G. G., Liu, L. N., Zhang, S. B., Liu, R. D., et al. (2015). Characterization of a Trichinella spiralis 31 kDa protein and its potential application for the serodiagnosis of trichinellosis. Acta Trop. 142, 57–63. doi: 10.1016/j.actatropica.2014.10.017
Cui, J., Wang, Z. Q., and Xu, B. L. (2011). The epidemiology of human trichinellosis in China during 2004-2009. Acta Trop. 118, 1–5. doi: 10.1016/j.actatropica.2011.02.005
Cuperlovic, K., Djordjevic, M., and Pavlovic, S. (2005). Re-emergence of trichinellosis in southeastern Europe due to political and economic changes. Vet. Parasitol. 132, 159–166. doi: 10.1016/j.vetpar.2005.05.047
Darwish, R. A., Sanad, M. M., and Youssef, S. M. (1996). Immunization against Trichinella spiralis using antigens from different life-cycle stages experimental study in mice. J. Egypt. Soc. Parasitol. 26, 19–26.
Devleesschauwer, B., Bouwknegt, M., Dorny, P., Gabriel, S., Havelaar, A. H., Quoilin, S., et al. (2017). Risk ranking of foodborne parasites: state of the art. Food Waterborne Parasitol. 8–9, 1–13. doi: 10.1016/j.fawpar.2017.11.001
Dzik, J. M. (2006). Molecules released by helminth parasites involved in host colonization. Acta Biochim. Pol. 53, 33–64. doi: 10.18388/abp.2006_3361
Eissa, M. M., el-Azzouni, M. Z., Baddour, N. M., and Boulos, L. M. (2003). Vaccination trial against experimental trichrinellosis using autoclaved Trichinella spiralis larvae vaccine (ATSLV). J. Egypt. Soc. Parasitol. 33, 219–228.
Faber, M., Schink, S., Mayer-Scholl, A., Ziesch, C., Schonfelder, R., Wichmann-Schauer, H., et al. (2015). Outbreak of Trichinellosis due to wild boar meat and evaluation of the effectiveness of post exposure prophylaxis, Germany, 2013. Clin. Infect. Dis. 60, E98–E104. doi: 10.1093/cid/civ199
Faghiri, Z., and Skelly, P. J. (2009). The role of tegumental aquaporin from the human parasitic worm, Schistosoma mansoni, in osmoregulation and drug uptake. FASEB J. 23, 2780–2789. doi: 10.1096/fj.09-130757
Feng, S., Wu, X., Wang, X., Bai, X., Shi, H., Tang, B., et al. (2013). Vaccination of mice with an antigenic serine protease-like protein elicits a protective immune response against Trichinella spiralis infection. J. Parasitol. 99, 426–432. doi: 10.1645/12-46.1
Feng, Y. Y., Liu, X. L., Liu, Y. Q., Tang, B., Bai, X., Li, C., et al. (2021). Comparative epigenomics reveals host diversity of the Trichinella epigenomes and their effects on differential parasitism. Front. Cell Dev. Biol. 9:681839. doi: 10.3389/fcell.2021.681839
Gamble, H. R. (1985a). Comparison of immune effects in mice immunized with Trichinella spiralis adult and larval antigens. J. Parasitol. 71, 680–682. doi: 10.2307/3281444
Gamble, H. R. (1985b). Trichinella spiralis: immunization of mice using monoclonal antibody affinity-isolated antigens. Exp. Parasitol. 59, 398–404. doi: 10.1016/0014-4894(85)90095-5
Gamble, H. R., Murrell, K. D., and Marti, H. P. (1986). Inoculation of pigs against Trichinella spiralis, using larval excretory-secretory antigens. Am. J. Vet. Res. 47, 2396–2399.
Gamble, H. R., Pozio, E., Bruschi, F., Nockler, K., Kapel, C. M. O., and Gajadhar, A. A. (2004). International commission on trichinellosis: recommendations on the use of serological tests for the detection of Trichinella infection in animals and man. Parasite 11, 3–13. doi: 10.1051/parasite/20041113
Gamble, H., Anderson, W., Graham, C., and Murrell, K. D. (1983). Diagnosis of swine trichinosis by enzyme-linked immunosorbent assay (ELISA) using an excretory–secretory antigen. Vet. Parasitol. 13, 349–361. doi: 10.1016/0304-4017(83)90051-1
Gamble, H., Rapić, D., Marinculić, A., and Murrell, K. D. (1988). Evaluation of excretory-secretory antigens for the serodiagnosis of swine trichinellosis. Vet. Parasitol. 30, 131–137. doi: 10.1016/0304-4017(88)90160-4
Gottstein, B., Pozio, E., and Nockler, K. (2009). Epidemiology, Diagnosis, Treatment, and Control of Trichinellosis. Clin. Microbiol. Rev. 22, 127–145. doi: 10.1128/cmr.00026-08
Grencis, R. K., Crawford, C., Pritchard, D. I., Behnke, J. M., and Wakelin, D. (1986). Immunization of mice with surface antigens from the muscle larvae of Trichinella spiralis. Parasite Immunol. 8, 587–596. doi: 10.1111/j.1365-3024.1986.tb00872.x
Grote, A., Caffrey, C. R., Rebello, K. M., Smith, D., Dalton, J. P., and Lustigman, S. (2018). Cysteine proteases during larval migration and development of helminths in their final host. PLoS Negl. Trop. Dis. 12:e0005919. doi: 10.1371/journal.pntd.0005919
Gu, Y., Sun, X., Huang, J., Zhan, B., and Zhu, X. (2020). A multiple antigen peptide vaccine containing CD4(+) T cell epitopes enhances humoral immunity against Trichinella spiralis infection in mice. J. Immunol. Res. 2020:2074803. doi: 10.1155/2020/2074803
Gu, Y., Wei, J. F., Yang, J., Huang, J. J., Yang, X. D., and Zhu, X. P. (2013). Protective Immunity against Trichinella spiralis Infection Induced by a Multi-Epitope Vaccine in a Murine Model. PLoS One 8:e77238. doi: 10.1371/journal.pone.0077238
Gu, Y., Zhan, B., Yang, Y., Yang, X., Zhao, X., Wang, L., et al. (2014). Protective effect of a prime-boost strategy with the Ts87 Vaccine against Trichinella spiralis infection in mice. Biomed. Res. Int. 2014:326860. doi: 10.1155/2014/326860
Habiela, M., Seaga, J., Perez-Martin, E., Waters, R., Windsor, M., Salguero, F. J., et al. (2014). Laboratory animal models to study foot-and-mouth disease: a review with emphasis on natural and vaccine-induced immunity. J. Gen. Virol. 95, 2329–2345. doi: 10.1099/vir.0.068270-0
Hafez, E. N., El Kholy, W. A. E. S., and Amin, M. M. (2020). The potential protective role of gamma-irradiated vaccine versus Punica granatum treatment against murine trichinellosis. J. Radiat. Res. Appl. Sci. 13, 560–567.
Hartmann, S., and Lucius, R. (2003). Modulation of host immune responses by nematode cystatins. Int. J. Parasitol. 33, 1291–1302. doi: 10.1016/s0020-7519(03)00163-2
Hewitson, J. P., and Maizels, R. M. (2014). Vaccination against helminth parasite infections. Expert. Rev. Vaccines 13, 473–487. doi: 10.1586/14760584.2014.893195
Hu, C. X., Xu, Y. X. Y., Hao, H. N., Liu, R. D., Jiang, P., Long, S. R., et al. (2021). Oral vaccination with recombinant Lactobacillus plantarum encoding Trichinella spiralis inorganic pyrophosphatase elicited a protective immunity in BALB/c mice. PLoS Negl. Trop. Dis. 15:e0009865. doi: 10.1371/journal.pntd.0009865
Jang, S. I., Lillehoj, H. S., Lee, S. H., Lee, K. W., Lillehoj, E. P., Bertrand, F., et al. (2011). Montanide IMS 1313 N VG PR nanoparticle adjuvant enhances antigen-specific immune responses to profilin following mucosal vaccination against Eimeria acervulina. Vet. Parasitol. 182, 163–170. doi: 10.1016/j.vetpar.2011.05.019
Jiang, P., Zhang, X., Wang, L. A., Han, L. H., Yang, M., Duan, J. Y., et al. (2016). Survey of Trichinella infection from domestic pigs in the historical endemic areas of Henan province, central China. Parasitol. Res. 115, 4707–4709. doi: 10.1007/s00436-016-5240-x
Joachim, A. (2016). Vaccination against parasites - status quo and the way forward. Porcine Health Manag. 2:30. doi: 10.1186/s40813-016-0047-9
Korinkova, K., Kovarcik, K., Pavlickova, Z., Svoboda, M., and Koudela, B. (2008). Serological detection of Trichinella spiralis in swine by ELISA (enzyme-linked immunosorbent assay) using an excretory-secretory (E/S) antigen. Parasitol. Res. 102, 1317–1320. doi: 10.1007/s00436-008-0911-x
Kumar, A., and Samant, M. (2016). DNA vaccine against visceral leishmaniasis: a promising approach for prevention and control. Parasite Immunol. 38, 273–281. doi: 10.1111/pim.12315
Li, L., Saade, F., and Petrovsky, N. (2012). The future of human DNA vaccines. J. Biotechnol. 162, 171–182. doi: 10.1016/j.jbiotec.2012.08.012
Li, T. T., Tang, B., Bai, X., Wang, X. L., Luo, X. N., Yan, H. B., et al. (2020). Development of genome-wide polymorphic microsatellite markers for Trichinella spiralis. Parasites Vectors 13:58. doi: 10.1186/s13071-020-3929-2
Lightowlers, M. W., and Rickard, M. D. (1988). Excretory-secretory products of helminth parasites: effects on host immune responses. Parasitology 96, S123–S166. doi: 10.1017/s0031182000086017
Liu, P., Wang, Z. Q., Liu, R. D., Jiang, P., Long, S. R., Liu, L. N., et al. (2015). Oral vaccination of mice with Trichinella spiralis nudix hydrolase DNA vaccine delivered by attenuated Salmonella elicited protective immunity. Exp. Parasitol. 153, 29–38. doi: 10.1016/j.exppara.2015.02.008
Liu, R. D., Cui, J., Liu, X. L., Jiang, P., Sun, G. G., Zhang, X., et al. (2015). Comparative proteomic analysis of surface proteins of Trichinella spiralis muscle larvae and intestinal infective larvae. Acta Trop. 150, 79–86. doi: 10.1016/j.actatropica.2015.07.002
Liu, R. D., Cui, J., Wang, L., Long, S. R., Zhang, X., Liu, M. Y., et al. (2014). Identification of surface proteins of Trichinella spiralis muscle larvae using immunoproteomics. Trop. Biomed. 31, 579–591.
Liu, R. D., Jiang, P., Wen, H., Duan, J. Y., Wang, L. A., Li, J. F., et al. (2016a). Screening and characterization of early diagnostic antigens in excretory-secretory proteins from Trichinella spiralis intestinal infective larvae by immunoproteomics. Parasitol. Res. 115, 615–622. doi: 10.1007/s00436-015-4779-2
Liu, R. D., Qi, X., Sun, G. G., Jiang, P., Zhang, X., Wang, L. A., et al. (2016b). Proteomic analysis of Trichinella spiralis adult worm excretory-secretory proteins recognized by early infection sera. Vet. Parasitol. 231, 43–46. doi: 10.1016/j.vetpar.2016.10.008
Liu, X. D., Wang, X. L., Bai, X., Liu, X. L., Wu, X. P., Zhao, Y., et al. (2014). Oral administration with attenuated Salmonella encoding a Trichinella cystatin-like protein elicited host immunity. Exp. Parasitol. 141, 1–11. doi: 10.1016/j.exppara.2014.03.015
Maizels, R. M., Hewitson, J. P., and Smith, K. A. (2012). Susceptibility and immunity to helminth parasites. Curr. Opin. Immunol. 24, 459–466. doi: 10.1016/j.coi.2012.06.003
Marti, H. P., Murrell, K. D., and Gamble, H. R. (1987). Trichinella spiralis: immunization of pigs with newborn larval antigens. Exp. Parasitol. 63, 68–73. doi: 10.1016/0014-4894(87)90079-8
McAllister, M. M. (2014). Successful vaccines for naturally occurring protozoal diseases of animals should guide human vaccine research. A review of protozoal vaccines and their designs. Parasitology 141, 624–640. doi: 10.1017/S0031182013002060
McGuire, C., Chan, W. C., and Wakelin, D. (2002). Nasal immunization with homogenate and peptide antigens induces protective immunity against Trichinella spiralis. Infect. Immun. 70, 7149–7152. doi: 10.1128/IAI.70.12.7149-7152.2002
Molehin, A. J., Gobert, G. N., and McManus, D. P. (2012). Serine protease inhibitors of parasitic helminths. Parasitology 139, 681–695. doi: 10.1017/S0031182011002435
Murrell, K. D., and Pozio, E. (2011). Worldwide Occurrence and Impact of Human Trichinellosis 1986-2009. Emerg. Infect. Dis. 17, 2194–2202. doi: 10.3201/eid1712.110896
Nagano, I., Wu, Z., and Takahashi, Y. (2009). Functional genes and proteins of Trichinella spp. Parasitol. Res. 104, 197–207. doi: 10.1007/s00436-008-1248-1
Nakayama, H., Inaba, T., Nargis, M., Chisty, M., Ito, M., and Kamiya, H. (1998). Immunization of laboratory animals with ultraviolet-attenuated larvae against homologous challenge infection with Trichinella britovi. Southeast Asian J. Trop. Med. Public Health 29, 563–566.
Ortega-Pierres, G., Muniz, E., Coral-Vazquez, R., and Parkhouse, R. M. (1989). Protection against Trichinella spiralis induced by purified stage-specific surface antigens of infective larvae. Parasitol. Res. 75, 563–567. doi: 10.1007/BF00931167
Ortega-Pierres, G., Vaquero-Vera, A., Fonseca-Linan, R., Bermudez-Cruz, R. M., and Argueello-Garcia, R. (2015). Induction of protection in murine experimental models against Trichinella spiralis: an up-to-date review. J. Helminthol. 89, 526–539. doi: 10.1017/S0022149X15000140
Pitoiset, F., Vazquez, T., and Bellier, B. (2015). Enveloped virus-like particle platforms: vaccines of the future? Expert. Rev. Vaccines 14, 913–915. doi: 10.1586/14760584.2015.1046440
Pozio, E. (2015). Trichinella spp. imported with live animals and meat. Vet. Parasitol. 213, 46–55. doi: 10.1016/j.vetpar.2015.02.017
Prazeres, D. M. F., and Monteiro, G. A. (2014). Plasmid Biopharmaceuticals. Microbiol. Spectrum 2:1128. doi: 10.1128/microbiolspec.PLAS-0022-2014
Qi, X., Yue, X., Han, Y., Jiang, P., Yang, F., Lei, J. J., et al. (2018). Characterization of two Trichinella spiralis Adult-Specific DNase II and their capacity to induce protective immunity. Front. Microbiol. 9:2504. doi: 10.3389/fmicb.2018.02504
Robinson, K., Bellaby, T., Chan, W. C., and Wakelin, D. (1995). High levels of protection induced by a 40-mer synthetic peptide vaccine against the intestinal nematode parasite Trichinella spiralis. Immunology 86, 495–498.
Sander, V. A., Sanchez Lopez, E. F., Mendoza Morales, L., Ramos Duarte, V. A., Corigliano, M. G., and Clemente, M. (2020). Use of veterinary vaccines for livestock as a strategy to control foodborne parasitic diseases. Front. Cell. Infect. Microbiol. 10:288. doi: 10.3389/fcimb.2020.00288
Shimoni, Z., and Froom, P. (2015). Uncertainties in diagnosis, treatment and prevention of trichinellosis. Expert. Rev. Anti Infect. Ther. 13, 1279–1288. doi: 10.1586/14787210.2015.1075394
Song, Y. Y., Zhang, Y., Yang, D. Q., Ren, H. N., Sun, G. G., Jiang, P., et al. (2018). The Immune Protection Induced by a Serine Protease Inhibitor From the Foodborne Parasite Trichinella spiralis. Front. Microbiol. 9:1544. doi: 10.3389/fmicb.2018.01544
Stills, H. F. (2005). Adjuvants and antibody production: dispelling the myths associated with Freund’s complete and other adjuvants. ILAR J. 46, 280–293. doi: 10.1093/ilar.46.3.280
Sun, G. G., Lei, J. J., Ren, H. N., Zhang, Y., Guo, K. X., Long, S. R., et al. (2019). Intranasal immunization with recombinant Trichinella spiralis serine protease elicits protective immunity in BALB/c mice. Exp. Parasitol. 201, 1–10. doi: 10.1016/j.exppara.2019.04.006
Tang, B., Liu, M., Wang, L., Yu, S., Shi, H., Boireau, P., et al. (2015). Characterisation of a high-frequency gene encoding a strongly antigenic cystatin-like protein from Trichinella spiralis at its early invasion stage. Parasites Vectors 8:78. doi: 10.1186/s13071-015-0689-5
Todorova, V. K. (2000). Proteolytic enzymes secreted by larval stage of the parasitic nematode Trichinella spiralis. Folia Parasitol. 47, 141–145. doi: 10.14411/fp.2000.027
Vray, B., Hartmann, S., and Hoebeke, J. (2002). Immunomodulatory properties of cystatins. Cell. Mol. Life Sci. 59, 1503–1512. doi: 10.1007/s00018-002-8525-4
Wang, B., Wang, Z. Q., Jin, J., Ren, H. J., Liu, L. N., and Cui, J. (2013). Cloning, expression and characterization of a Trichinella spiralis serine protease gene encoding a 35.5 kDa protein. Exp. Parasitol. 134, 148–154. doi: 10.1016/j.exppara.2013.03.004
Wang, J. L., Huang, S. Y., Behnke, M. S., Chen, K., Shen, B., and Zhu, X. Q. (2016). The past, present, and future of genetic manipulation in Toxoplasma gondii. Trends Parasitol. 32, 542–553. doi: 10.1016/j.pt.2016.04.013
Wang, J. L., Zhang, N. Z., Li, T. T., He, J. J., Elsheikha, H. M., and Zhu, X. Q. (2019). Advances in the development of Anti-Toxoplasma gondii vaccines: challenges, opportunities, and perspectives. Trends Parasitol. 35, 239–253. doi: 10.1016/j.pt.2019.01.005
Wang, L., Wang, X. H., Bi, K., Sun, X. M., Yang, J., Gu, Y., et al. (2016). Oral Vaccination with Attenuated Salmonella typhimurium-Delivered TsPmy DNA Vaccine Elicits Protective Immunity against Trichinella spiralis in BALB/c Mice. PLoS Negl. Trop. Dis. 10:e0004952. doi: 10.1371/journal.pntd.0004952
Wang, Z. Q., Shi, Y. L., Liu, R. D., Jiang, P., Guan, Y. Y., Chen, Y. D., et al. (2017). New insights on serodiagnosis of trichinellosis during window period: early diagnostic antigens from Trichinella spiralis intestinal worms. Infect. Dis. Poverty 6:41. doi: 10.1186/s40249-017-0252-z
Xu, D., Bai, X., Xu, J., Wang, X., Dong, Z., Shi, W., et al. (2021). The immune protection induced by a serine protease from the Trichinella spiralis adult against Trichinella spiralis infection in pigs. PLoS Negl. Trop. Dis. 15:e0009408. doi: 10.1371/journal.pntd.0009408
Xu, D., Tang, B., Yang, Y., Cai, X., Jia, W., Luo, X., et al. (2020b). Vaccination with a DNase II recombinant protein against Trichinella spiralis infection in pigs. Vet. Parasitol. 297:109069. doi: 10.1016/j.vetpar.2020.109069
Xu, D., Tang, B., Wang, Y., Zhang, L., Qu, Z., Shi, W., et al. (2020a). The immune protection induced by a serine protease from the Trichinella spiralis adult administered as DNA and protein vaccine. Acta Tropica 211, 105622–105622. doi: 10.1016/j.actatropica.2020.105622
Xu, J., Bai, X., Wang, L. B., Shi, H. N., Van Der Giessen, J. W. B., Boireau, P., et al. (2017a). Immune responses in mice vaccinated with a DNA vaccine expressing serine protease-like protein from the new-born larval stage of Trichinella spiralis. Parasitology 144, 712–719. doi: 10.1017/S0031182016002493
Xu, J., Bai, X., Wang, L. B., Shi, H. N., van der Giessen, J. W. B., Boireau, P., et al. (2017b). Influence of adjuvant formulation on inducing immune response in mice immunized with a recombinant serpin from Trichinella spiralis. Parasite Immunol. 39:e12437. doi: 10.1111/pim.12437
Yang, J., Gu, Y., Yang, Y., Wei, J., Wang, S., Cui, S., et al. (2010). Trichinella spiralis: immune response and protective immunity elicited by recombinant paramyosin formulated with different adjuvants. Exp. Parasitol. 124, 403–408. doi: 10.1016/j.exppara.2009.12.010
Zhang, N. G., Gao, Q., Wang, M., Elsheikha, H. M., Wang, B., Wang, J. L., et al. (2018). Immunization with a DNA vaccine cocktail encoding TgPF, TgROP16, TgROP18, TgMIC6, and TgCDPK3 genes protects mice against chronic toxoplasmosis. Front. Immunol. 9:1505. doi: 10.3389/fimmu.2018.01505
Keywords: Trichinella spiralis, trichinellosis, antigens, vaccines, effectiveness
Citation: Tang B, Li J, Li T, Xie Y, Guan W, Zhao Y, Yang S, Liu M and Xu D (2022) Vaccines as a Strategy to Control Trichinellosis. Front. Microbiol. 13:857786. doi: 10.3389/fmicb.2022.857786
Received: 19 January 2022; Accepted: 16 February 2022;
Published: 23 March 2022.
Edited by:
Lihua Xiao, South China Agricultural University, ChinaReviewed by:
M. Mabel Ribicich, University of Buenos Aires, ArgentinaRuo Dan Liu, Zhengzhou University, China
Copyright © 2022 Tang, Li, Li, Xie, Guan, Zhao, Yang, Liu and Xu. This is an open-access article distributed under the terms of the Creative Commons Attribution License (CC BY). The use, distribution or reproduction in other forums is permitted, provided the original author(s) and the copyright owner(s) are credited and that the original publication in this journal is cited, in accordance with accepted academic practice. No use, distribution or reproduction is permitted which does not comply with these terms.
*Correspondence: Mingyuan Liu, bGl1bXlAamx1LmVkdS5jbg==; Daoxiu Xu, eGRhb3hpdUAxNjMuY29t
†These authors have contributed equally to this work