- 1Department of Surgical and Radiological Sciences, School of Veterinary Medicine, University of California, Davis, Davis, CA, United States
- 2William R. Pritchard Veterinary Medical Teaching Hospital, School of Veterinary Medicine, University of California, Davis, Davis, CA, United States
- 3Department of Ophthalmology & Vision Science, School of Medicine, University of California, Davis, Davis, CA, United States
Microbial keratitis is a common cause of ocular pain and visual impairment worldwide. The ocular surface has a relatively paucicellular microbial community, mostly found in the conjunctiva, while the cornea would be considered relatively sterile. However, in patients with microbial keratitis, the cornea can be infected with multiple pathogens including Staphylococcus aureus, Pseudomonas aeruginosa, and Fusarium sp. Treatment with topical antimicrobials serves as the standard of care for microbial keratitis, however, due to high rates of pathogen resistance to current antimicrobial medications, alternative therapeutic strategies must be developed. Multiple studies have characterized the expression and activity of antimicrobial peptides (AMPs), endogenous peptides with key antimicrobial and wound healing properties, on the ocular surface. Recent studies and clinical trials provide promise for the use of AMPs as therapeutic agents. This article reviews the repertoire of AMPs expressed at the ocular surface, how expression of these AMPs can be modulated, and the potential for harnessing the AMPs as potential therapeutics for patients with microbial keratitis.
Introduction
Microbial keratitis, or an infection of the cornea, can result in devastating visual impairment or even permanent vision loss if an effective, targeted therapy is not instituted in a timely manner. Damage to the corneal epithelium opens a portal for the entry of pathogenic microbes. This damage is often associated with contact lens use but can also be caused by a multitude of ocular surface traumas (Austin et al., 2017). With an incidence of one million visits to medical providers annually in the United States and an estimated $175 million in health expenditures, infectious keratitis places an enormous burden on patients and the healthcare system alike (Ung et al., 2019).
Antimicrobial peptides (AMPs) are key effector molecules with broad spectrum antimicrobial activity against bacteria, fungi, and viruses (Leonard et al., 2012; Boparai and Sharma, 2020). In addition to their direct antimicrobial activity, AMPs have been shown to modulate a wide array of critical cell behaviors including chemotaxis, cytokine production, epithelial cell proliferation, promotion of cell migration, angiogenesis, apoptosis, wound healing, and they even modulate coat color in dogs (Leonard et al., 2012). AMPs are small peptides, ranging anywhere from 12 to 50 amino acids in length, composed of cationic residues including arginine and lysine which are thought to exert the majority of their antimicrobial activity (McDermott, 2009). Additionally, these peptides contain hydrophilic and hydrophobic regions. The amphipathic nature of these peptides allows them to exert their antipathogenic action where they interact and directly incorporate into microbial cell walls and membranes (McDermott, 2009; Leonard et al., 2012).
AMPs are a component of the innate immune system expressed by epithelial cells (Fulton et al., 1997; Bals et al., 1998; Haynes et al., 1999; O’Neil et al., 1999) and leukocytes (Rivas-Santiago et al., 2008). Most epithelial cells that reside at the interface between pathogens and the host (i.e., mucosal surfaces such as the ocular surface, gastrointestinal tract, respiratory tract). These sites are where AMPs are constitutively expressed and increased expression can be induced by the presence of pathogenic or inflammatory stimuli (Haynes et al., 1999; McDermott et al., 2003; Rivas-Santiago et al., 2008). AMP expression can be upregulated to augment the antimicrobial activity at a body surface.
Given the rise in multidrug resistance, alternative and adjunctive treatment strategies for microbial keratitis are needed and the use of topically applied exogenous (natural and synthetic) AMPs and/or upregulation of endogenous AMPs may prove to be a critical therapeutic intervention. This review highlights the specific functions of AMPs expressed by the ocular surface and discusses the potential for exogenous treatment or modulation of host AMP expression as a therapeutic intervention for microbial keratitis.
Antimicrobial Peptides at the Ocular Surface
There are many AMPs expressed throughout the body, however cathelicidin, defensins, histatin, thymosin, psoriasin, and ribonuclease-7 are the main AMPs that play key roles in the innate immune system of the ocular surface (Figure 1).
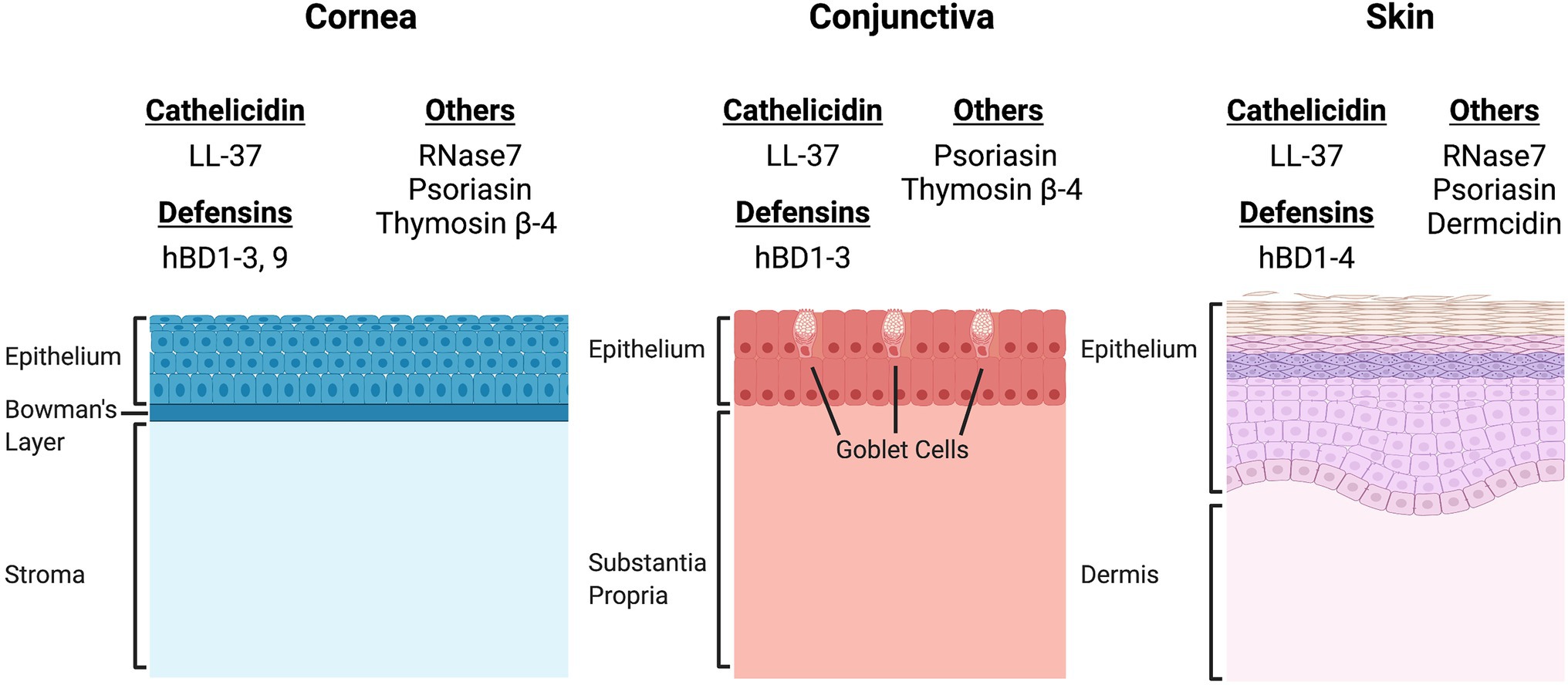
Figure 1. Comparison of the site-specific expression of antimicrobial peptides (AMPs) expressed by epithelial cells of the cornea, conjunctiva, and skin. There is significant overlap in the expression patterns of AMPs at both the ocular surface and the skin. Cathelicidin (LL-37), β-defensins 1, 2, and 3, and psoriasin are expressed by epithelial cells of the cornea, conjunctiva and skin (Herman and Herman, 2019). However, site specific expression has been documented for β-defensin 4 (hBD4, skin), hBD9 (cornea), RNase7 (cornea, skin), thymosin β-4 (cornea, conjunctiva) and dermcidin (skin). The differences in AMP expression patterns may directly influence the microbial niches of the cornea, conjunctiva, and skin.
Cathelicidins
Cathelicidins, named for their highly conserved cathelin domain at the N-terminus, were first discovered in bovine neutrophils and have since been documented in 133 other vertebrate species (Zanetti et al., 1990, 1995; Mookherjee et al., 2013). Many vertebrate species have multiple cathelicidins; but humans, mice, cats, and dogs are known to possess only one (Sang et al., 2007; Leonard et al., 2011; Mookherjee et al., 2013). The human cathelicidin antimicrobial peptide (hCAP18), encoded by the CAMP gene, is transcribed in a pro-form and is activated by specialized proteinases to become LL-37 (Sorensen et al., 2001; Bandurska et al., 2015). The LL-37 peptide is composed of 37 amino acids and is named for the two leucine residues found in the N-terminus of the mature peptide (Bandurska et al., 2015). Since its discovery in neutrophilic granules, LL-37 expression has been identified in various epithelial tissues including eye, skin, lung, mouth, tongue, esophagus, vagina, cervix, and sweat glands (Wang, 2014). At the ocular surface, LL-37 has both constitutive and inducible expression by conjunctival and corneal epithelial cells (McIntosh et al., 2005). The importance of their role in the innate immunity of the ocular surface was demonstrated through a study by Huang et al. (2007b), in which cathelicidin-deficient mice had increased susceptibility to corneal infection by Pseudomonas aeruginosa, delayed clearance of P. aeruginosa, and increased corneal infiltrating neutrophils when compared to wild type animals.
Defensins
The defensins are encoded by multiple genes mainly clustered on human chromosome 8 (Jia et al., 2001, p. 22–23). The primary structure of defensin peptides differs from cathelicidins in that they range from 29 to 45 amino acids and contain six cysteine residues, creating three disulfide bridges that form its secondary beta-pleated sheet structure (Selsted et al., 1985; Ganz, 2003; Lehrer et al., 2005). The three subgroups of defensins are α-, β-, and θ-defensins, categorized by their disulfide bridging array (Fruitwala et al., 2019). Four of the six α-defensins that have been identified are produced by neutrophils, thus they have been denoted human neutrophil peptides (HNP), while α-defensins 5 and 6 are predominantly found in Paneth cells of the small intestine in a subset of species (Jones and Bevins, 1992; Fruitwala et al., 2019). While there have been 34 human β-defensins (hBD) identified using gene analysis, only a small subset of the peptides has been well-characterized (Fruitwala et al., 2019). Human β-defensins 1–3 are secreted by epithelial cells and mononuclear cells while hBD4-6 are designated to specific tissues (Fruitwala et al., 2019). The β-defensins are considered the oldest evolutionary subcategory of the defensins as they can be found in a wide range of species from horseshoe crabs to mammals (Cheng et al., 2014). The α-defensins are thought to have evolved from the β-defensins and have been described in many mammals such as humans, primates, horses, rats, mice, rabbits, and guinea pigs (Wang, 2014). Lastly, the θ-defensins are a unique subcategory of defensins with respect to the synthesis of the peptide. The θ-defensins are translated as two separate peptides that fuse into a circular peptide and are only found in non-human primates (Tang et al., 1999; Cheng et al., 2014).
Of the defensins discovered, it is α-defensins and hBDs that have been shown to play key roles in ocular surface immunity. The α-defensins 1–4 are secreted into the tear film via passing and resident neutrophils along the ocular surface (Haynes et al., 1999). Alternatively, hBD 1–3 can be consistently detected in corneal and conjunctival epithelium, and hBD2 and 3 have been found in measurable amounts in human tears (Haynes et al., 1999; McIntosh et al., 2005; Garreis et al., 2010). Additionally, hBD1 can be found in the lacrimal gland and intraocular tissues (Haynes et al., 2000). Human β-defensin 1 is constitutively expressed while hBD2 and 3 are inducible by pathogenic and inflammatory stimuli (McDermott et al., 2003). To highlight their role in ocular surface immunity, an in vitro study on human corneal epithelial multilayers demonstrated reduced susceptibility to P. aeruginosa penetration following pre-exposure to bacterial antigens, known stimulants of AMPs (Augustin et al., 2011). The same study demonstrated a 3-fold increase in P. aeruginosa transversal across multilayered human corneal epithelia following hBD1-3 knockdown (Augustin et al., 2011). Similar in vivo effects were seen in corneal epithelia of murine BD3 deficient mice, the ortholog of hBD2, with increased susceptibility and adherence by P. aeruginosa when compared to wild-type mice (Augustin et al., 2011). Interestingly, not all hBDs expressed by the ocular surface appear to have a clear antimicrobial effect. Human β-defensin 9 is expressed by ocular surface epithelium, with particularly high concentration in conjunctival epithelium (Mohammed et al., 2010). However, hBD9 has been shown have a different expression pattern entirely and is often downregulated during infection (Otri et al., 2012; Dua et al., 2014).
Histatins
Histatins are cationic peptides that were first discovered in 1988 when Oppenheim et al. (1988) isolated histatins 1, 3, and 5 from human parotid salivary glands (Oppenheim et al., 1988; Khurshid et al., 2017). Histatin 1 and 3 are encoded on genes HTN1 and HTN3, while histatin 5 is a proteolytic product derived from histatin 3 (Oppenheim et al., 1988). Histatins, named for their histidine-rich composition, are well known for their wound healing effects in saliva, and have similar wound healing effects on the ocular surface, particularly histatin-1 and -5 (Oudhoff et al., 2008; Shah et al., 2017, 2020). Histatin-1 is expressed by both the main and accessory lacrimal glands, and can be detected in human tears, with lower levels of expression found in patients with aqueous deficient dry eye disease (Shah et al., 2016; Kalmodia et al., 2019). When applied to human corneal epithelial cells (HCEC) in vitro, histatin-1 showed increased cell surface area and faster wound closure, indicative of enhanced corneal epithelial spreading with minimal toxicity (Shah et al., 2017). Similar results of enhanced wound healing have been replicated in a rabbit model (Oydanich et al., 2018).
Thymosin
Thymosins contain three subgroups of families denoted as α, β, and γ polypeptides, that were first extracted from the thymus gland of the calf (Goldstein, 2007; Sosne et al., 2016). Thymosin β-4, -10, and -15 are found in humans, with thymosin β-4 being the most abundant and active polypeptide with expression in all tissues except red blood cells (Sosne et al., 2016). Thymosin β-4 was identified as a 43 amino acid G-actin-sequestering peptide with wound healing and anti-inflammatory properties (Goldstein and Badamchian, 2004; Sosne et al., 2016). After detection of thymosin β-4 in HCEC and conjunctival epithelial cells, it was hypothesized that thymosin β-4 could play a role in ocular surface health (Huang et al., 2007a). In an in vivo murine model, thymosin β-4 treated corneas had decreased expression of matrix metalloproteinase levels and leukocyte infiltration correlating to decreased inflammation and enhanced corneal wound repair following alkali injury (Sosne et al., 2005). Another study found that thymosin β-4 treated HCEC had increased ability to scavenge reactive oxygen species when compared to controls (Ho et al., 2008). To elucidate an activation pathway responsible for its anti-inflammatory properties, researchers compared thymosin β-4 treated to untreated HCEC in vitro following stimulation by TNF-α, a pro-inflammatory cytokine (Sosne et al., 2007). Results demonstrated that thymosin β-4 treated cells inhibited NF-κB phosphorylation and translocation, a commonly utilized pro-inflammatory cascade (Sosne et al., 2004). These anti-inflammatory properties of thymosin β-4 would be of particular benefit for the treatment of ocular surface disease. Treatment with thymosin β-4 significantly reduced signs of dryness in a murine adverse environmental model of dry eye, so appreciably that its effects are now being tested in phase 2 randomized trials (Sosne et al., 2015a,b). It also had significant healing effects and reduced ocular irritation for patients with refractory neurotrophic corneal ulcers (Dunn et al., 2010).
Psoriasin
Psoriasin (S100A7) is a subset of the S100 proteins family of Ca2+ binding proteins, encoded by genes of the epidermal differentiation complex (EDC) located on chromosome 1 (1q21; Mischke et al., 1996; Lesniak and Graczyk-Jarzynka, 2015). Its structure contains two EF-hands, or Ca2+ binding motifs, with a high affinity C-terminal containing a 12 amino acid canonical Ca2+ binding loop and a 14 amino acid low affinity N-terminal (Lesniak and Graczyk-Jarzynka, 2015). Given its name, psoriasin was first discovered in psoriatic keratinocytes and it is markedly overexpressed in psoriatic tissues compared to normal epidermis (Madsen et al., 1991; Broome et al., 2003; Martinsson et al., 2005). In normal epithelium, it is expressed by superficial, differentiated keratinocytes rather than the basal cells, suggesting its involvement in keratinocyte differentiation (Martinsson et al., 2005). Psoriasin has in vitro and in vivo Escherichia coli-killing properties in human keratinocytes that is mediated by Zn2+ sequestration (Glaser et al., 2005). The toll-like receptor (TLR)-5 ligand, flagellin, was identified as the essential bacterial component needed to induce psoriasin expression in epidermal keratinocytes (Abtin et al., 2008). Psoriasin expression was also induced during epidermal barrier disruption and by cytokines IL-17, IL-22, and TNF-α in in vitro and in vivo atopic dermatitis models (Glaser et al., 2009a). Besides its antimicrobial activity, psoriasin can influence innate immunity by regulating neutrophil function to produce cytokines and chemokines via phosphorylation of mitogen-activated protein kinase (MAPK) p38 and extracellular signal-regulated kinase (ERK; Zheng et al., 2008). In regard to the ocular surface, psoriasin was constitutively expressed in cornea, conjunctiva, nasolacrimal ducts, and lacrimal gland (Garreis et al., 2011). Psoriasin also demonstrated strong staining in meibomian glands on immunohistochemistry (Garreis et al., 2011). Interestingly, the same study detected induction of psoriasin in HCEC following stimulation with Staphylococcus aureus and Haemophilus influenzae supernatants, but not following stimulation by E. coli supernatants (Garreis et al., 2011). Both IL-1β and, to a lesser extent, TNF-α were able to upregulate psoriasin gene expression in HCEC (Garreis et al., 2011).
Ribonuclease-7
Ribonucealse-7 (RNase-7) is a part of the Ribonuclease A Superfamily, a group of peptides originally isolated from the bovine pancreas (Becknell and Spencer, 2016). RNase-7 is the most potent of the antimicrobial ribonucleases identified and, in addition to its ribonucleolytic activity, also has both angiogenic and immunomodulatory properties (Becknell and Spencer, 2016). RNase-7 has demonstrated antimicrobial activity against vancomycin-resistant Enteroccocus facium and can control cutaneous growth of P. aeruginosa in an in vitro skin keratinocyte model (Harder and Schroder, 2002; Rademacher et al., 2017). RNase-7 also has in vitro antibacterial activity against E. coli, P. aeruginosa, and S. aureus, and is upregulated with inflammation caused by UV-B radiation and in the context of atopic dermatitis (Zhang et al., 2003; Gambichler et al., 2008; Glaser et al., 2009b). RNase-7 expression has since been detected in human corneal epithelial cells and upregulation of RNase-7 appears to be mediated through the TAK-1/MAPK pathway following stimulation by the inflammatory cytokine IL-1β (Mohammed et al., 2011).
Functional Properties of Antimicrobial Peptides
There are myriad functions ascribed to AMPs. This review paper will focus on the functional properties of AMPs that are relevant to microbial keratitis. However, for the functional properties that are less relevant to ocular surface health, such as angiogenesis and apoptosis, please see the following reviews for their current state in the field (Chen et al., 2018; Jafari et al., 2022).
Antibacterial
There is a general consensus that AMPs exert their direct antimicrobial activity through disruption of the pathogen cellular membrane, resulting in cellular content leakage (Matsuzaki, 2019). However, there are multiple proposed theories to explain how this mechanism leads to cell death. These theories include the carpet model, the barrel stave model, and the more widely accepted toroidal pore model (Matsuzaki, 2019). The initial interaction of the AMPs is similar in all three proposed models, whereby AMPs are both electrostatically and hydrophobically attracted to bacterial cell membranes (Matsuzaki et al., 1995). After the initial attraction, the mechanistic theories for membrane disruption diverge. The carpet model describes AMPs as coating the outside surface of the cellular membrane, where they induce negative membrane curvature and charge neutralizing conditions increasing membrane strain, allowing additional AMPs to congregate, resulting in membrane collapse (Figure 2A; Silvestro et al., 1997; Matsuzaki, 2019). By contrast, both the barrel stave model and toroidal pore models focus on insertion of AMPs into the membrane to induce pore formation as the mechanism of action for membrane leakage. However, in the barrel stave model, the walls of the pore are made of purely AMPs, creating a channel for intracellular contents to leak out (Figure 2B; Baumann and Mueller, 1974). This is slightly different from the more widely accepted toroidal pore model, in which the walls of the pore are lined by both polar faces of AMPs and the lipid headgroups of the membrane (Figure 2C; Matsuzaki, 2019). This results in the transient existence of a torus-type pore, allowing an efflux of intracellular contents due to the positive pressure exerted on membrane curvature by the AMPs (Matsuzaki et al., 1998). In general, the cationic nature of the AMPs is beneficial to host immune defenses since it dictates their heavy attraction to the negatively charged bacterial cell membranes that contain acidic phospholipids and abundant lipopolysaccharide (LPS), compared to the zwitterionic phospholipids of host cell membranes (Matsuzaki et al., 1995). In addition, bacterial membranes lack sterols, making them much less resistant to the pore forming effects of AMPs when compared to the cholesterol-rich membrane of host cells (Matsuzaki et al., 1995).
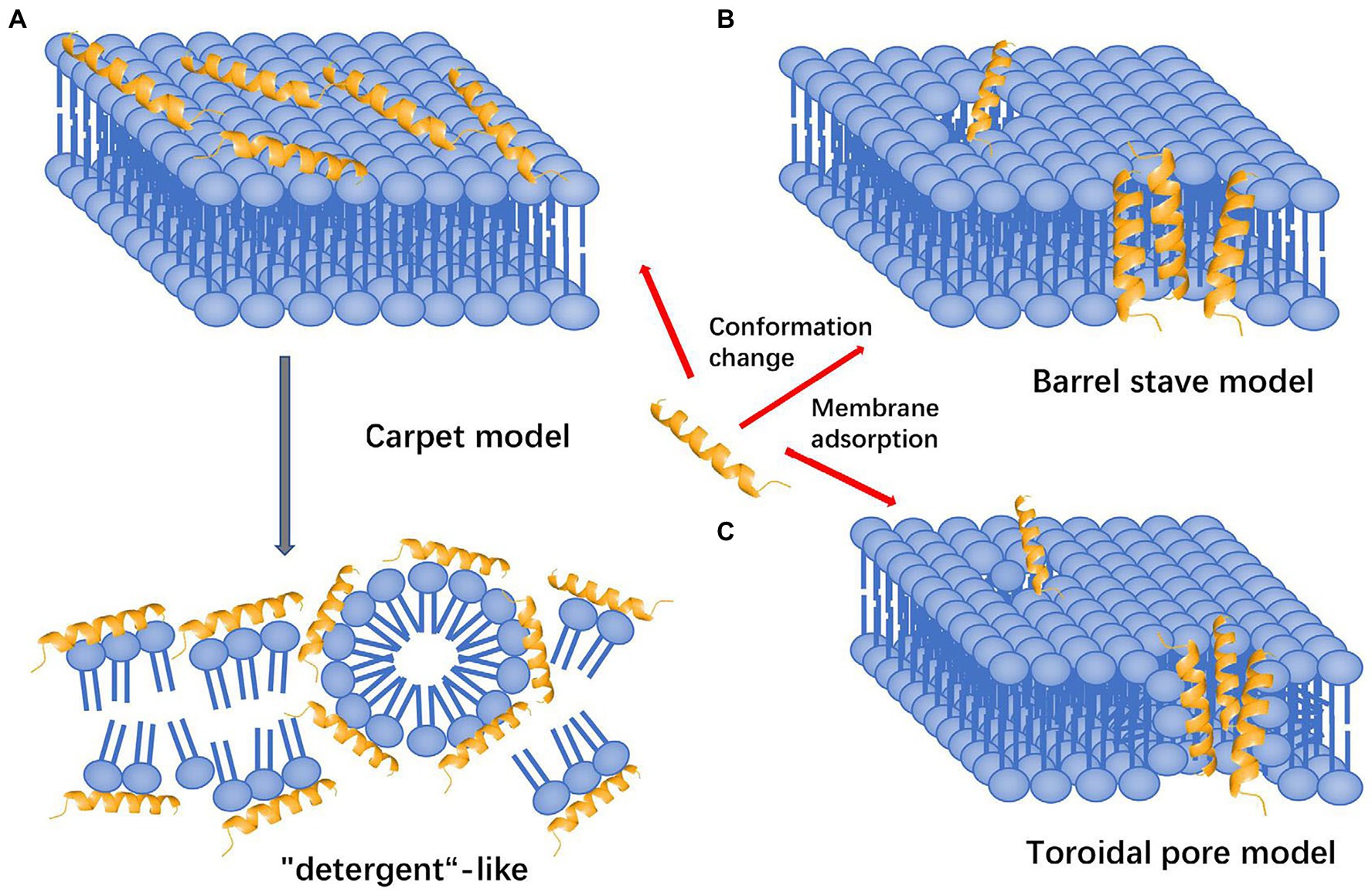
Figure 2. Mechanisms of action attributed to AMPs that lead to disruption of the cell membrane and subsequent death. (A) Carpet model: AMPs are attracted to the surface and disrupt the membrane in a detergent-like fashion, destabilizing the phospholipid packaging. (B) Barrel stave model: AMPs are attracted to the surface and inserted into the membrane, forming transmembrane helical channels resulting in killing via expulsion of water and intracellular contents. (C) Toroidal pore model: AMPs are attracted to the surface, embed into the cell membrane and bend to form a ring pore resulting in efflux of ions and small molecules. All models result in death of cell via different mechanisms (adapted from Huan et al., 2020).
Although the mechanism for membrane disruption primarily involves forced cell lysis and intracellular leakage, these models may not reflect the entirety of AMP mediated microbe killing. Recent studies suggest AMPs can also cause membrane permeabilization by direct inhibition of cell wall biosynthesis and activation of enzymes to lyse cell walls (Bierbaum and Sahl, 1987; Wiedemann et al., 2001; Sass et al., 2010). For example, hBD3 was shown to induce lysis similar to that of the antibiotic vancomycin through an experiment identifying AMP binding to the bacterial membrane precursor in Staphylococcus sp., lipid II (Sass et al., 2010). Human β-defensin 3 also induced morphological changes in S. aureus similar to treatment with penicillin, whose mechanism of action is known to inhibit cross-linking of peptidoglycans during cell wall synthesis (Harder et al., 2001). Additionally, through ionic interaction, the cationic AMPs can also cause membrane disruption by associating with inhibitors of autolytic enzymes, and therefore activate cell wall lysis (Bierbaum and Sahl, 1987). However, killing kinetics by AMPs seems to be largely dependent on individual microbial strains, peptide concentration and structure, and physiological conditions (Sahl et al., 2005).
Antiviral
Antimicrobial peptides have been shown to prevent and treat viral infections, however their antiviral activity is not as clearly defined as their bactericidal activity (Park et al., 2018). Rather than simply disrupting membrane integrity, AMPs prevent viral infection at multiple levels including: reduction of infectivity, direct viral inactivation, capsid proteins binding, and inhibition of viral attachment, entry, and replication (Park et al., 2018). This is not an exhaustive list as their antiviral activity appears to be dependent on virus type and physiological conditions (Park et al., 2018).
Human cathelicidin LL-37 has demonstrated protection against human immunodeficiency virus (HIV), influenza A virus (IAV), herpes simplex virus (HSV), rhinovirus (HRV), vaccinia virus (VACV), hepatitis C virus (HCV), respiratory syncytial virus (RSV), zika virus (ZIKV), dengue virus (DENV), and hepatitis C virus (HCV; Ahmed et al., 2019). For the majority of these viruses, LL-37 mediates its attack through the carpet model, in which the viral envelope is dissolved when threshold concentrations of LL-37 are reached (Dean et al., 2010). In a study focused on the antiviral activity of LL-37 against HSV, researchers saw that expression levels of TLR3 and LL-37 were higher in herpetic vesicles in the skin compared to normal controls (Takiguchi et al., 2014). With an understanding of direct viral killing by LL-37, the researchers treated HSV infected human keratinocytes with LL-37 and poly (I:C), a synthetic analog of viral double stranded RNA and a TLR3 ligand (Howell et al., 2004; Takiguchi et al., 2014). Results demonstrated that keratinocytes co-treated with LL-37 and poly (I:C) significantly reduced viral plaque assays via TLR3 signaling (Takiguchi et al., 2014).
Like cathelicidins, defensins have also been shown to inhibit HIV, IAV, RSV, and HSV. Additionally, defensins have been found to impede human adenovirus (HAdV), papilloma virus (HPV), and severe acute respiratory syndrome coronavirus (SARSC; Ahmed et al., 2019). Defensins have a wide repertoire of antiviral activity in which they directly or indirectly intervene in the viral life cycle. Some of the major studies of AMP antiviral activity of the ocular surface investigated the effect of defensins against HSV, a double-stranded DNA virus known to cause epithelial keratitis of the cornea (Koujah et al., 2019). Additionally, all human defensins (with the exception of hBD1 and 2) significantly inhibit HSV-2 infection in human cervical epithelial cells at 25 and 50 μg/ml (Hazrati et al., 2006). The methods of viral inhibition include prevention of viral binding, entry, and post-entry events (Hazrati et al., 2006). Specifically, by binding the cell surface receptors glycoprotein D or heparin sulfate, the α-defensins and hBD3 were able to inhibit infection by HSV (Hazrati et al., 2006). Especially intriguing were the post-entry effects seen as HSV infected cells expressed less viral fusion protein when treated with human alpha defensins [human neutrophilic peptide 1 (HNP-1) or human alpha defensin 5 (HD5)], compared to treatment with a defensin-free buffer (Hazrati et al., 2006). Studies such as these show the exciting potential for AMPs to be effective microbicides against pervasive viruses known to commonly infect the eye, including HSV.
Antifungal
AMPs have demonstrated antifungal activity as well with action against Fusarium, Aspergillus, Cryptococcus, and Candida, which are increasingly resistant fungal pathogens of the eye. In a murine model of Fusarium keratitis, researchers observed increased expression of murine β-defensins and cathelin-related antimicrobial peptide (CRAMP) at the onset of infection and faster recovery rates when compared to knockdown or knockout mice that experienced significantly augmented disease severity (Kolar et al., 2013). Another study concluded that defensins have also been shown to significantly damage Cryptococcus species. After treatment with various AMPs, the β-defensins proved to be among the most effective AMPs at slowing metabolic activity and decreasing fungal mass with planktonic cryptococci being more susceptible to damage than cryptococcal biofilms (Martinez and Casadevall, 2006). The mechanism for how AMPs interfere with fungal invasion has yet to be fully understood, but researchers have observed cell membrane disruption and interference with fungal adhesion (Tsai et al., 2011; Goncalves et al., 2017). For example, human cathelicidin (LL-37) has been shown to target C. albicans by binding mannan, the carbohydrate component of yeast cell walls, inhibiting fungal adhesion and aggregation in both in vitro and in vivo models (Tsai et al., 2011). Current treatment for fungal keratitis includes topical natamycin, amphotericin, or, for deep penetrating hyphae, voriconazole (Joseph and Sharma, 2016). However, with current increases in resistance to antifungal medications, researchers are pursuing the use of AMPs as an attractive alternative in patients undergoing corneal transplantation (Sara et al., 2016).
Chemotaxis
Chemoattraction is the movement of a cell in response to a chemical gradient in which the cell moves toward a high concentration of ligands. AMPs have demonstrated chemotactic properties for various cells of the innate and adaptive immune system. Based on the observation that interleukin-8 (IL-8) induced neutrophilic degranulation followed by T-cell infiltration, a 1996 study attempted to identify the neutrophil-derived chemoattractant factors, postulating that these were the cause for T-cell migration. The chemoattractant factors identified were hBD1-3, with the first two defensins having potent chemotactic capabilities for human T cells (Chertov et al., 1996). More specifically, mouse beta-defensin 14 (mBD14), the orthologue of hBD3, has been shown to be chemotactic for cells expressing mouse CC-chemokine receptor 6 (CCR6; Rohrl et al., 2008). The same chemoattractant effect was seen for hBD2, which utilized CCR6 to recruit human neutrophils that were pretreated with TNF-α (Niyonsaba et al., 2004).
After the discovery of defensin-mediated chemoattraction, researchers hypothesized that LL-37 would also have potent chemotactic activity. Indeed, LL-37 promoted the chemotaxis of various leukocytes, through Ca2+ mobilization in human monocytes, as well as the recruitment of neutrophils and T-cells via formyl peptide receptor-like 1 (FPRL1; Yang et al., 2000). Additionally, pulmonary researchers demonstrated that LL-37 attracts human peripheral blood eosinophils and neutrophils, cell types that characterize respiratory diseases such as asthma or chronic obstructive pulmonary disease, via FPRL1 (Tjabringa et al., 2006). This finding was confirmed when FPR antagonists inhibited LL-37-mediated chemotaxis (Tjabringa et al., 2006). In regard to the ocular surface, LL-37 has been shown to promote HCEC chemotaxis via the protein kinase pathways (PKC; Griffith et al., 2013). The ability of AMPs to chemoattract cells of the adaptive immune system creates a more streamlined inflammatory response between the innate and adaptive immunity, however, more studies are needed to further investigate the role of AMP-mediated chemotaxis on the protection of the ocular surface.
Wound Healing
Of the AMPs present on the ocular surface, thymosin β-4 and histatins have been promoted corneal wound healing. For re-epithelialization to occur, there must be epithelial cell migration and proliferation. In an in vitro study on human conjunctival epithelial cells, thymosin β-4 stimulated cell migration in a dose-dependent manner when compared to controls (Sosne et al., 2002). Thymosin β-4 treated cells also expressed increased laminin-5 deposition, an extracellular protein that initiates hemidesmosome formation and basement membrane attachment (Sosne et al., 2002). Similar benefits were demonstrated in the in vivo murine model following alkali burns. Thymosin β-4 treated mice had improved corneal clarity and decreased matrix metalloproteinases and infiltrating leukocytes, thereby promoting corneal wound repair following injury (Sosne et al., 2005). Researchers discovered that thymosin β-4 utilizes the ERK1/2 signaling pathway via a P2X7 receptor-mediated calcium influx to enhance HCEC proliferation and migration (Yang et al., 2020). Besides thymosin β-4, the histatin family also has potent wound healing capabilities. In vitro HCECs treated with histatin-1 demonstrated increased corneal epithelial spreading and pathfinding during re-epithelialization of a scratch wound (Shah et al., 2017). Histatin-5 had similar in vitro properties, demonstrating enhanced cellular migration of HCEC in a sprouting assay and faster rate of scratch closure in human corneal limbal epithelium (Shah et al., 2020). Histatin-5 also promotes cellular spreading, exhibited by an increase in surface area of treated HCECs (Shah et al., 2020). In the same in vitro study, histatin-5 was determined to utilize the phosphorylated ERK1/2 pathway (Shah et al., 2020). Histatins have similar efficacy in the in vivo rabbit corneal injury model. Following corneal injury with ethyl alcohol, rabbits treated with varying concentrations of histatin-1 demonstrated a faster recovery rate and a higher percent recovered area when compared with controls (Oydanich et al., 2018). Besides thymosin β-4 and histatins, LL-37 and hBD2 and 3 also play a role in wound healing. In vivo skin wounds demonstrated high levels of LL-37 in both inflammatory cells and migrating epithelium, and treatment with LL-37 induced cell proliferation and migration in airway epithelial cells (Heilborn et al., 2003b; Shaykhiev et al., 2005). In a study on corneal epithelialization in the context of diabetes, high-glucose attenuated wounds had enhanced LL-37 expression, which in response, prolonged the effects of epidermal growth factor receptor (EGFR) signaling and its ability to hasten scratch wound closure (Yin and Yu, 2010). Interestingly, low levels of LL-37 are present in chronic corneal ulcers, indicating that re-epithelialization may be partially dependent on LL-37 concentration (Heilborn et al., 2003a). Human β-defensin 2 has shown to augment wound healing in keratinocytes and hBD3 can stimulate intestinal epithelial migration in vitro (Otte et al., 2008; Hirsch et al., 2009). Additionally, hBD2 has shown to be upregulated in the re-epithelialized cornea, leaving researchers to hypothesize that it plays a key role in wound healing (McDermott et al., 2001). The ability of AMPs to function as immunomodulators and accelerate wound healing is fundamental to their role as modulators of the host immune system and can be leveraged in the future for therapeutic use.
Antibiofilm
Biofilms are aggregations of microbes that secrete extracellular polymeric substances (EPS) that allow for the adhesion of carbohydrates, proteins, lipids, and nucleic acids (Khatoon et al., 2018). This mixture of nutrients serves as a platform for bacteria to engage with, resulting in the upregulation of intracellular signaling and proliferation (Khatoon et al., 2018). Biofilms present a foreboding clinical problem with their growing antibiotic resistance and their tendency to accumulate on medical devices, such as contact lenses (Khatoon et al., 2018). Researchers have observed that biofilm formation of S. epidermidis was inhibited by human cathelicidin and that bacterial attachment to artificial surfaces was diminished at concentrations of LL-37 similar to human plasma levels (Hell et al., 2010). Using microarray technology, it was revealed that the mechanism by which LL-37 inhibits bacterial strains, like P. aeruginosa, involves the down-regulation of genes responsible for quorum sensing which are essential for biofilm formation (Overhage et al., 2008). The ability of AMPs to disrupt biofilm formation will become increasingly relevant as more therapeutic interventions for the ocular surface will rely on drug delivery devices on which bacteria could form biofilms.
Regulation of Antimicrobial Peptide Expression
AMPs are considered frontline defenses of the innate immune system, as they are constitutively expressed by epithelial cells of the ocular surface, intestine, skin, respiratory, and the reproductive systems to kill pathogens directly (Gallo and Hooper, 2012). However, many pathogenic stimuli, inflammatory mediators, and some small molecules can initiate a signaling cascade resulting in the upregulation of endogenous AMP expression. Given this knowledge, the ability to modulate AMP expression could serve as a therapeutic strategy to mitigate the pathologic consequences of infection. The following sections will include studies from multiple organ systems, including the ocular surface, to provide a more complete overview of how AMP gene expression is regulated and how modulation of their expression could be employed for therapeutic use.
Pathogenic Stimulation
AMP expression can be induced through the activation of pathogen recognition receptors (PRRs), such as TLRs, which recognize conserved structural pathogen motifs known as pathogen associated molecular patterns (PAMPs; Mogensen, 2009; Gallo and Hooper, 2012). Some TLRs reside at the cell surface (TLR1, -2, -4, -5, -6, -10) and recognize unique bacterial products, such a LPS or flagellin, while others reside in endosomes (TLR3, -7, -8, -9) and recognize exogenous nucleic acids (Mogensen, 2009). Once bound to its endogenous or exogenous ligand, TLRs will induce an intracellular signaling cascade that is classified as either myeloid differentiation primary response protein 88 (MyD88)-dependent or MyD88-independent. MyD88 is an adapter molecule used by TLRs as a co-stimulatory protein that helps shape the cellular response to PAMPs. Depending on the TLR activated, the subsequent signaling cascade involves multiple protein kinases, such as mitogen-activated protein kinase (MAPK). Ultimately, this cascade activates transcription factors, such as nuclear factor kB (NF-κB), activator protein-1 (AP-1), and interferon regulatory factors 3 and/or 7 (IRF3/7), resulting in the coordinated activation of both the host’s innate and adaptive immune response by producing proinflammatory cytokines, type 1 interferons, and AMPs (Figure 3A; Gomariz et al., 2010; Prasad et al., 2019).
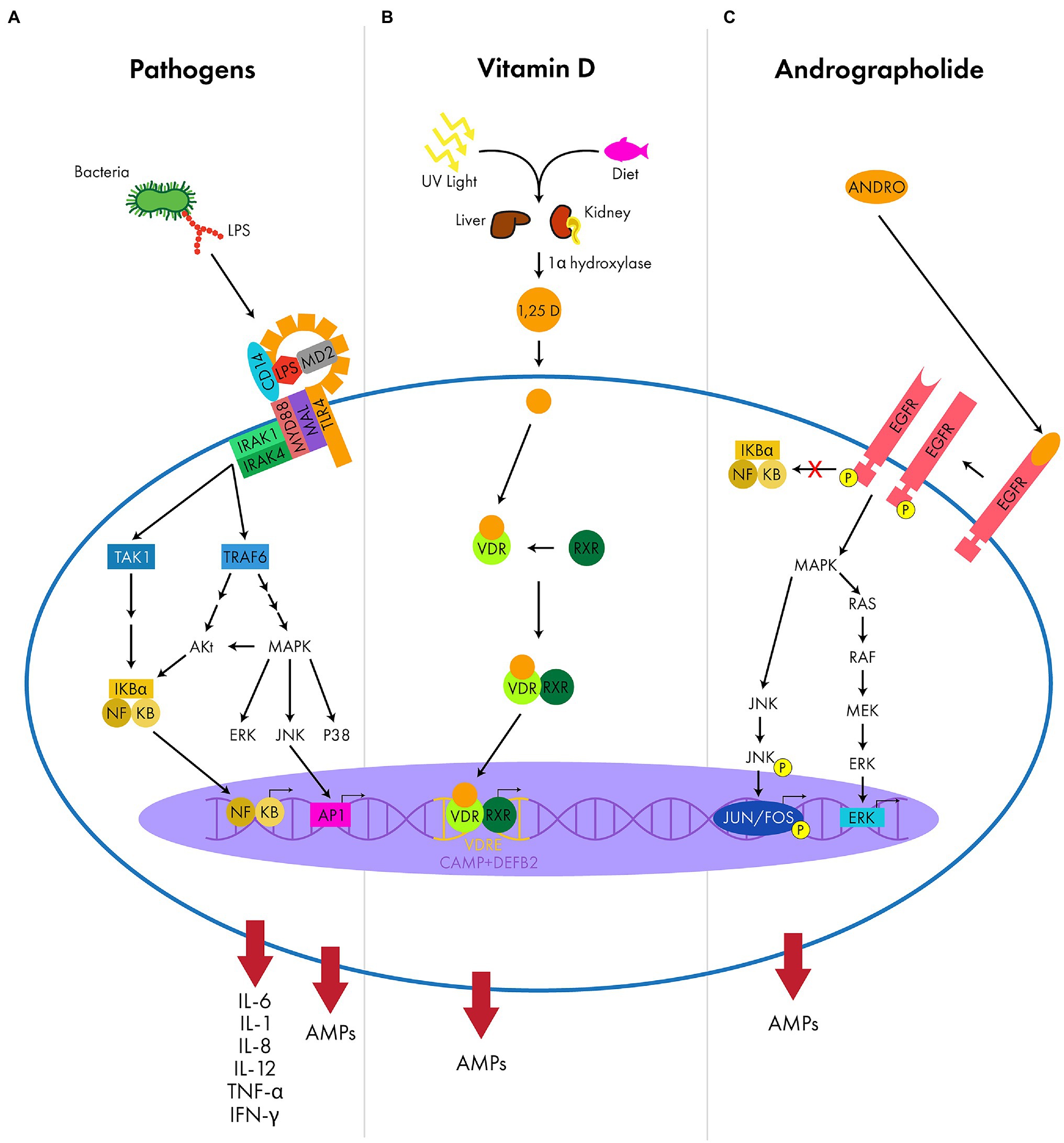
Figure 3. Examples of the signaling molecules and pathways that result in upregulation of AMP expression. (A) The LPS complex (TLR4, CD14, and MD2) recognizes PAMPS (LPS) and uses adapter proteins (MyD88 and MAL) to activate intracellular protein kinases (IRAK1 and IRAK4). These intracellular kinases trigger a signaling cascade (TAK1, TRAF6, Akt, MAPK and the subfamilies ERK/JNK/P38) initiating transcription factors (NF-κB and AP1) to upregulate AMPs and pro-inflammatory cytokines (IL-6, IL-1, IL-8, IL-12, TNF-α, and IFN-γ). (B) Vitamin D is absorbed from the conversion of UV light in the skin or from the diet and is metabolized by various enzymes (1α-hydroxylase) of the kidney and liver to produce the active metabolite, calcitriol (1,25 D). Calcitriol will bind intracellular receptors (VDR and RXR) that heterodimerize and target specific DNA sequences with VDREs, such as CAMP and DEFB2, to upregulate AMP expression. (C) Andrographolide (ANDRO) activates a ligand-induced dimerization of epidermal growth factor receptor (EGFR), allowing for tyrosine kinase activity and auto-phosphorylation. This triggers an intracellular signaling cascade (MAPK and subfamilies RAS/RAF/MEK/ERK and JNK), activating transcription factors (JUN/FOS and ERK) to upregulate AMP expression without the activation of the pro-inflammatory NF-κB pathway.
Given this activation pathway, there have been multiple studies demonstrating upregulation of AMP expression at the ocular surface and in other organ systems with pathogenic stimulation. Defensins and LL-37 become upregulated in various leukocyte and epithelial systems, such as the skin and intestine, following exposure to pathogens (O’Neil et al., 1999; Rivas-Santiago et al., 2008; Lai et al., 2010; Koon et al., 2011). Similar trends can be seen at the ocular surface. AMPs are consistently upregulated in HCEC in the presence of common bacterial keratitis pathogens such as Streptococcus pneumoniae, Corynebacterium pseudodiphtheriticum, P. aeruginosa (Augustin et al., 2011; Redfern et al., 2011; Evans and Fleiszig, 2013; Roy et al., 2015; Sharma et al., 2019). In HCEC treated with TLR agonists, there was an upregulation of hBD2 and LL-37 expression, with LL-37 having efficacy killing P. aeruginosa (Redfern et al., 2011). Similarly, in HCEC infected with S. pneumonia, LL-37 expression was induced via transcription factor STAT3 activation and had effective killing in vitro (Sharma et al., 2019). Induction of LL-37 and hBD1-3 was also seen in limbo-corneal fibroblasts post-infection with mycobacteria (Castañeda-Sánchez et al., 2013). PAMPs from fungal pathogens also induce AMP expression. Human corneal epithelial cells upregulated hBD2 and LL-37 following exposure to heat inactivated Fusarium solani (Kolar et al., 2017). This upregulation was determined to be dependent on TLR2 and dectin-1, a PRR, since knockdown of TLR2 and dectin-1 in HCEC abrogated this response. Similarly, heat-killed Candida albicans dose-dependently stimulated LL-37, hBD2, and hBD3 production in HCEC (Hua et al., 2014).
Knowing that PAMPs stimulate AMP expression, researchers have turned to methods of topical application of de-activated PAMPs that could pre-treat the cornea to resist infection by microbes. Following topical application of flagellin, the principal protein of bacterial flagella, mice corneas had improved protection from candida keratitis (Gao et al., 2011). Using TLR5, flagellin was able to induce CAMP upregulation, and once CAMP was totally ablated the protective effects against C. albicans were abolished (Gao et al., 2011). In parallel, corneal fibroblasts that were pre-treated with LPS expressed elevated levels of thymosin β-4 upon subsequent challenge with Aspergillus fumigatus (Xiaoyan et al., 2011). These studies are just a few examples demonstrating the close relationship between activation of TLRs by PAMPs, induction of AMP synthesis, and how their induction can be modulated to augment the innate immune response.
Inflammatory Stimulation
Similar to the mechanisms used to stimulate AMP secretion via TLR activation, inflammatory cytokines and mediators have also been shown to induce AMP expression to modulate host immune defense. When innate immune cells encounter pathogens, a signaling cascade induces secretion of inflammatory cytokines to signal cells of the adaptive immune system (Kolls et al., 2008). These cytokines work not only to modulate the host’s immune response, but also to upregulate AMP expression (Kolls et al., 2008). The interleukin-1 (IL-1) family of cytokines is one of the best-known inducers of AMPs. Evidence of the regulatory pathway responsible for cytokine upregulation of AMPs in multiple organ systems, including the ocular surface, is thought to involve MAPK and NF-κB transcription factors (Krisanaprakornkit et al., 2002; McDermott et al., 2003; Kao et al., 2004). Relevant to the ocular surface, McDermott et al. demonstrated upregulation of hBD2 gene expression in HCEC when treated with IL-1β and TNF-α (McDermott et al., 2003). The upregulation of hBD2 expression in HCEC was abolished in the presence of NF-κB inhibitors and partially blocked with both MAP kinase and JNK inhibitors independently (McDermott et al., 2003). Interestingly, hBD2 is found to have higher expression in inflamed conjunctiva of patients with pterygium, likely due to stimulation by cytokines (Karadag et al., 2017). The importance of IL-1 as an AMP stimulant was highlighted in the murine model of experimental dry eye, where IL-1 receptor deficient mice demonstrated non-existent to decreased levels of mBD2 in cornea and conjunctiva when compared to wild type mice (Narayanan et al., 2008) Interestingly, local application of a newly discovered subfamily of cytokines, IL-36γ, stimulated expression of mBD3 in murine corneas while simultaneously inhibiting IL-1β (Gao et al., 2018). These findings demonstrate the need to further investigate the exact mechanisms of AMP upregulation by cytokines to better define the fundamental role of AMPs in modulating host immune function to better inform therapeutic strategies (Moon et al., 2002; Albanesi et al., 2007).
Small Molecules
There are many small molecules known to upregulate AMP expression including: vitamin D, fatty acids (ex. butyrate; Raqib et al., 2006; Nakatsuji et al., 2010; Jiang et al., 2013; Sunkara et al., 2014; Xiong et al., 2016; Wang et al., 2018; Schulthess et al., 2019; Takakuwa et al., 2019; Zhang et al., 2020; Schaefer et al., 2022), amino acids, (Sherman et al., 2006; Ren et al., 2016; Zhang et al., 2017; Schaefer et al., 2022) trace nutrients (ex. zinc; Wang et al., 2006; Talukder et al., 2011; Liu et al., 2014), and other less common molecules, like curcumin (Guo et al., 2013; Liu et al., 2020), resveratrol (Ravagnan et al., 2013), isoflavones (Srisomboon et al., 2017), genistein (Park et al., 2014), and andrographolide (Sechet et al., 2018). This section highlights the mechanisms of (1) vitamin D-induced upregulation of cathelicidin and (2) andrographolide upregulation of hBD3. Importantly, upregulation of AMP expression with vitamin D and andrographolide has been shown to occur without the release of inflammatory mediators that would exacerbate ocular surface disease and potentially impair vision.
Vitamin D
The vitamin D receptor (VDR) pathway proteins are widely expressed among epithelial and immune cells and the VDR pathway plays a critical role in the induction of AMP expression. There is evidence that activation of the VDR pathway directly upregulates AMP expression, specifically, LL-37 and hBD2 (Wang et al., 2004; Gombart et al., 2005). Vitamin D3 can either be ingested from the diet or synthesized by the skin with UV light exposure and subsequent conversion to the active form, 1,25 dihydroxyvitamin D(1,25D; Prosser and Jones, 2004). Binding of 1,25D to the cytosolic VDR results in the heterodimerization of the VDR with the retinoid X receptor (White, 2010; Svensson et al., 2016). Then this complex binds to the vitamin D response element (VDRE) located in the regulatory regions of the CAMP and DEFB2 genes, stimulating transcription and upregulation of AMP expression (Figure 3B; Wang et al., 2004; Svensson et al., 2016). Utilizing this pathway, vitamin D is a potent inducer of LL-37 and could serve as a therapeutic target. In fact, LL-37 plasma concentrations directly reflect systemic vitamin D3 concentrations with higher values of vitamin D3 correlating with higher levels of LL-37 expression (Dixon et al., 2012). Upregulation of LL-37 expression with vitamin D supplementation has been shown to reduce the growth of mycobacteria in vitro and aid wound healing in human skin in vivo (Martineau et al., 2007; Heilborn et al., 2010). The role of vitamin D in regulating LL-37 expression has also been demonstrated in corneal epithelial cells. Previous studies have applied vitamin D metabolites to corneal epithelial cells in vitro and observed the production of the active metabolite, 1,25 vitamin D3, which enhanced LL-37 expression while attenuating pro-inflammatory cytokines and metalloproteinases (Reins et al., 2015). Furthermore, topical application of vitamin D in a mouse model of corneal wound healing resulted in delayed wound closure, increased infiltration of neutrophils, and increased expression of CRAMP (Reins et al., 2016). The increased neutrophil infiltration may have been responsible for the delayed re-epithelialization as vitamin D also upregulated the neutrophil chemoattractant CXCL (Reins et al., 2016). Despite the delay in wound healing from the neutrophilic infiltration, the increased expression of CRAMP did exert the beneficial effect of blocking pathogen entry in a non-sterile wound environment, providing protection to the exposed epithelium (Reins et al., 2016). When corneal epithelial cells were infected by Fusarium solani, researchers observed an upregulation of the VDR via the TLR2/1-VDR pathway, identifying the role of the VDR pathway as a potential target for upregulating CAMP to treat fungal keratitis (Cong et al., 2015). As an inducer of LL-37, vitamin D shows promising potential as a topical therapeutic. However, not all studies have reached similar conclusions about the positive therapeutic effect of vitamin D, thus additional studies into the role of vitamin D on the ocular surface innate immune system is warranted (Sel et al., 2016).
Andrographolide
Andrographolide is a small molecule extracted from the herb Andrographis paniculata that has been used for centuries across some Asian cultures as a remedy to treat respiratory and gastrointestinal bacterial infections (Tan et al., 2017). A recent study identified the inducible effects of andrographolide on hBD3 in colonic epithelial and organoid cell lines (Sechet et al., 2018). There was a dose-dependent increase in mRNA expression of hBD3 when cells were treated with andrographolide and a 100-fold increase in hBD3 peptide expression was identified at 75 and 100 μM of andrographolide (Sechet et al., 2018). Using EGFR inhibitors, they determined that the EGFR-ERK (extracellular-signal-regulated kinase)-JNK (c-Jun N-terminal kinase) pathway was responsible for the upregulation of hBD3 expression. After inducing dimerization of EGFR, andrographolide activated the MAPK intracellular signaling cascade, a pathway responsible for cell proliferation, stress signaling, and AMP expression (Figure 3C; Sechet et al., 2018). Interestingly, andrographolide induced hBD3 expression without activating the pro-inflammatory effects of the NF-κB pathway (Sechet et al., 2018). They also observed that the effects of andrographolide in boosting hBD3 expression could be further increased when synergized with another molecule from natural pharmacopeia, isoliquiritigenin (Sechet et al., 2018). Similarly, other research groups found that andrographolide could also induce hBD2 expression in intestinal and lung epithelial cells (Shao et al., 2012; Xiong et al., 2015). In addition, these groups observed a less pronounced upregulation of hBD2 expression when co-treated with inhibitors of the MAPK pathway, p38 (Shao et al., 2012; Xiong et al., 2015). Moving forward, studies that utilize andrographolide to induce hBDs in corneal epithelial cells would serve as a proof-of-concept for implementing new therapeutics to target resistant infectious keratitis while decreasing the pro-inflammatory mediators that compromise vision.
Therapeutic Use of Antimicrobial Peptides
Exogenous Use of Antimicrobial Peptides
Given their great efficacy against pathogens, some researchers have taken the approach of directly applying both natural and synthetic AMPs to wounds (Clemens et al., 2017). Several studies have shown that application of exogenous natural AMPs can have immunomodulatory and protective effects against infectious keratitis (Gao and Yu, 2015; Kolar et al., 2015; Shah et al., 2017). Furthermore, it has been observed that AMPs can synergize with each other and additional antibacterial enzymes (Nagaoka et al., 2000; Singh et al., 2000; Wu et al., 2009; Chen et al., 2019). From this, researchers hypothesized that AMPs could synergize with current antibiotic therapies to potentiate bactericidal effects (Li et al., 2014; Carion et al., 2020). These effects can be seen at the ocular surface when researchers applied thymosin β-4 and ciprofloxacin to HCEC in vitro (Carion et al., 2020). While thymosin β-4 did not have direct antibacterial effects, it synergized with ciprofloxacin to upregulate other AMPs and related molecules for improved disease response (Carion et al., 2020). However, utilizing exogenous AMPs can cause cytotoxic effects at high concentrations, which are often necessary to see beneficial effects (Huang et al., 2006). Given this fine balance, some scientists have attempted altering AMP structure or creating synthetic AMPs (Kowalski et al., 2016; Clemens et al., 2017). For example, Brilcidin (PMX30063), a defensin mimetic, demonstrated in vitro activity against gram-positive bacteria in ocular isolates collected from patients with keratitis and endophthalmitis (Kowalski et al., 2016). In vivo, Brilcidin 0.5% was equally efficacious to vancomycin in reducing methicillin-resistant S. aureus (MRSA) in rabbit eyes (Kowalski et al., 2016). Given the success in studies on the exogenous application of AMPs, researchers have experimented with delivering AMPs in different vehicles, including contact lenses, hydrogels, and liposomes (Kumar et al., 2011; Dutta et al., 2016; Gallagher et al., 2016; Kennedy et al., 2020; Kalaiselvan et al., 2021). In an in vivo rabbit model, melimine-coated contact lenses significantly reduced P. aeruginosa microbial keratitis, had lower ocular scores, and improved ocular signs compared to controls (Dutta et al., 2016). However, researchers observed a corneal staining response seen with melimine-coated contact lenses, and decided to create Mel4, a shorter version of the melimine peptide that has the hydrophobic amino acids removed (Dutta et al., 2018; Willcox et al., 2020). The success of Mel4 coated contact lenses in preclinical testing, led to phase I/II/III clinical trials where the Mel4 contact lenses showed a 50% reduction in corneal infiltrative events, defined as inflammation caused by microbial colonization of contact lenses (Willcox et al., 2020). Studies such as these are promising, however, this therapeutic potential can be highly variable. Additionally, their utilization is limited by the cost of production, bioavailability, cytotoxicity, and pharmacokinetic stability (Mannis, 2002; Huang et al., 2006; Brandt et al., 2007; Dutta and Das, 2016). Until circumventions are made, such as using D amino acids to improve pharmacokinetic stability or creating shorter peptides to improve production costs and decrease cytotoxicity, the use of synthetic peptides may not be the most efficient therapeutic approach (McPhee et al., 2005; Liu et al., 2008). An alternative approach focused on the modulation of endogenous AMP expression may serve as a viable treatment option for patients with infectious disease, particularly through the topical application of AMP induction molecules at the ocular surface to upregulate the innate immune system.
Modulation of Endogenous Antimicrobial Peptide Expression
Through our understanding of AMP regulation pathways, there is a potential to modulate endogenous AMP expression with small molecules as either a prophylactic measure to prevent infection or as a method to treat infection. Outside of the use of narrowband ultraviolet B treatment to increase systemic vitamin D levels (Vahavihu et al., 2010) or the direct supplementation of vitamin D, (Peric et al., 2009) there is a paucity of studies focused on modulating AMP expression in vivo. This represents an unexplored area for developing a novel therapeutic approach for the treatment of microbial keratitis. Additionally, the antimicrobial activity of AMPs have been shown to be potentiated when combined with traditional antibiotic therapy (Darveau et al., 1991). More specifically, co-treatment of hBD3 with either amoxicillin, chlorhexidine or metronidazole resulted in synergistic antimicrobial activity against Streptococcus mutans, Actinobacillus actinomycetemcomitans, and Porphyromonas gingivalis compared with single agent treatment alone (Maisetta et al., 2003).
Conclusion
Antimicrobial peptides are critical to maintaining the health of the ocular surface and prevent infection. This review highlights the key AMPs expressed by the corneal and conjunctival epithelium in health, their method of action and regulation of expression. Future studies are warranted to evaluate the therapeutic benefit of exogenous AMP delivery as well as the modulation of endogenous peptide expression. The eye represents an ideal organ system for these therapeutic approaches due to the direct delivery of compounds to the site of infection and the cell types required to halt progression. Researchers should continue to explore the intricacies of AMP signaling pathways and regulation in addition to providing vehicles for extended drug delivery such as nano-particle based vectors, liposomes, and drug-eluting contact lenses (Lakhani et al., 2018; Xu et al., 2018; Bhattacharjee et al., 2019; Martin-Serrano et al., 2019). In doing this, ocular pharmacology could shepherd in a new therapeutic approach for the treatment of microbial keratitis while simultaneously limiting the potential for antimicrobial resistance.
Author Contributions
AS and BL: conceptualization and writing (original draft preparation). All authors contributed to the article and approved the submitted version.
Funding
This work was supported by the Summer Training in Advanced Research from University of California, Davis, School of Veterinary Medicine (AS) and the National Institutes of Health K08EY028199 (BL) and R01EY019970 (CM and ST).
Conflict of Interest
The authors declare that the research was conducted in the absence of any commercial or financial relationships that could be construed as a potential conflict of interest.
Publisher’s Note
All claims expressed in this article are solely those of the authors and do not necessarily represent those of their affiliated organizations, or those of the publisher, the editors and the reviewers. Any product that may be evaluated in this article, or claim that may be made by its manufacturer, is not guaranteed or endorsed by the publisher.
Acknowledgments
Figure 1 was created with BioRender.com. The authors thank Chrisoula Agape-Toupadakis Skouritakis for the illustration utilized in Figure 3.
References
Abtin, A., Eckhart, L., Mildner, M., Gruber, F., Schröder, J. M., and Tschachler, E. (2008). Flagellin is the principal inducer of the antimicrobial peptide S100A7c (psoriasin) in human epidermal keratinocytes exposed to Escherichia coli. FASEB J. 22, 2168–2176. doi: 10.1096/fj.07-104117
Ahmed, A., Siman-Tov, G., Hall, G., Bhalla, N., and Narayanan, A. (2019). Human antimicrobial peptides as therapeutics for viral infections. Viruses 11:704. doi: 10.3390/v11080704
Albanesi, C., Fairchild, H. R., Madonna, S., Scarponi, C., de Pità, O., Leung, D. Y. M., et al. (2007). IL-4 and IL-13 negatively regulate TNF-alpha- and IFN-gamma-induced beta-defensin expression through STAT-6, suppressor of cytokine signaling (SOCS)-1, and SOCS-3. J. Immunol. 179, 984–992. doi: 10.4049/jimmunol.179.2.984
Augustin, D. K., Heimer, S. R., Tam, C., Li, W. Y., le Due, J. M., Evans, D. J., et al. (2011). Role of defensins in corneal epithelial barrier function against Pseudomonas aeruginosa traversal. Infect. Immun. 79, 595–605. doi: 10.1128/IAI.00854-10
Austin, A., Lietman, T., and Rose-Nussbaumer, J. (2017). Update on the management of infectious keratitis. Ophthalmology 124, 1678–1689. doi: 10.1016/j.ophtha.2017.05.012
Bals, R., Wang, X., Zasloff, M., and Wilson, J. M. (1998). The peptide antibiotic LL-37/hCAP-18 is expressed in epithelia of the human lung where it has broad antimicrobial activity at the airway surface. Proc. Natl. Acad. Sci. U. S. A. 95, 9541–9546. doi: 10.1073/pnas.95.16.9541
Bandurska, K., Berdowska, A., Barczyńska-Felusiak, R., and Krupa, P. (2015). Unique features of human cathelicidin LL-37. Biofactors 41, 289–300. doi: 10.1002/biof.1225
Baumann, G., and Mueller, P. (1974). A molecular model of membrane excitability. J. Supramol. Struct. 2, 538–557. doi: 10.1002/jss.400020504
Becknell, B., and Spencer, J. D. (2016). A review of Ribonuclease 7’s structure, regulation, and contributions to host defense. Int. J. Mol. Sci. 17:423. doi: 10.3390/ijms17030423
Bhattacharjee, A., Das, P. J., Adhikari, P., Marbaniang, D., Pal, P., Ray, S., et al. (2019). Novel drug delivery systems for ocular therapy: with special reference to liposomal ocular delivery. Eur. J. Ophthalmol. 29, 113–126. doi: 10.1177/1120672118769776
Bierbaum, G., and Sahl, H. G. (1987). Autolytic system of Staphylococcus simulans 22: influence of cationic peptides on activity of N-acetylmuramoyl-L-alanine amidase. J. Bacteriol. 169, 5452–5458. doi: 10.1128/jb.169.12.5452-5458.1987
Boparai, J. K., and Sharma, P. K. (2020). Mini review on antimicrobial peptides, sources, mechanism and recent applications. Protein Pept. Lett. 27, 4–16. doi: 10.2174/0929866526666190822165812
Brandt, C. R., Akkarawongsa, R., Altmann, S., Jose, G., Kolb, A. W., Waring, A. J., et al. (2007). Evaluation of a theta-defensin in a murine model of herpes simplex virus type 1 keratitis. Invest. Ophthalmol. Vis. Sci. 48, 5118–5124. doi: 10.1167/iovs.07-0302
Broome, A. M., Ryan, D., and Eckert, R. L. (2003). S100 protein subcellular localization during epidermal differentiation and psoriasis. J. Histochem. Cytochem. 51, 675–685. doi: 10.1177/002215540305100513
Carion, T. W., Ebrahim, A. S., Alluri, S., Ebrahim, T., Parker, T., Burns, J., et al. (2020). Antimicrobial effects of thymosin Beta-4 and ciprofloxacin adjunctive therapy in Pseudomonas aeruginosa induced keratitis. Int. J. Mol. Sci. 21. doi: 10.3390/ijms21186840
Castañeda-Sánchez, J. I., García-Pérez, B. E., Muñoz-Duarte, A. R., Baltierra-Uribe, S. L., Mejia-López, H., López-López, C., et al. (2013). Defensin production by human limbo-corneal fibroblasts infected with mycobacteria. Pathogens 2, 13–32. doi: 10.3390/pathogens2010013
Chen, X., Chen, H., Zhang, H., Peng, Y., Deng, F., Gao, J., et al. (2019). Characterization of synergistic antibacterial effect of silver nanoparticles and ebselen. Artif. Cells Nanomed. Biotechnol. 47, 3338–3349. doi: 10.1080/21691401.2019.1648278
Chen, X., Zou, X., Qi, G., Tang, Y., Guo, Y., Si, J., et al. (2018). Roles and mechanisms of human cathelicidin LL-37 in cancer. Cell. Physiol. Biochem. 47, 1060–1073. doi: 10.1159/000490183
Cheng, D.-Q., Li, Y., and Huang, J.-F. (2014). Molecular evolution of the primate α-/θ-Defensin multigene family. PLoS One 9, 1–16. doi: 10.1371/journal.pone.0097425
Chertov, O., Michiel, D. F., Xu, L., Wang, J. M., Tani, K., Murphy, W. J., et al. (1996). Identification of defensin-1, defensin-2, and CAP37/azurocidin as T-cell chemoattractant proteins released from interleukin-8-stimulated neutrophils. J. Biol. Chem. 271, 2935–2940. doi: 10.1074/jbc.271.6.2935
Clemens, L. E., Jaynes, J., Lim, E., Kolar, S. S., Reins, R. Y., Baidouri, H., et al. (2017). Designed host defense peptides for the treatment of bacterial keratitis. Invest. Ophthalmol. Vis. Sci. 58, 6273–6281. doi: 10.1167/iovs.17-22243
Cong, L., Xia, Y. P., Zhao, G. Q., Lin, J., Xu, Q., Hu, L. T., et al. (2015). Expression of vitamin D receptor and cathelicidin in human corneal epithelium cells during Fusarium solani infection. Int. J. Ophthalmol. 8, 866–871. doi: 10.3980/j.issn.2222-3959.2015.05.03
Darveau, R. P., Cunningham, M. D., Seachord, C. L., Cassiano-Clough, L., Cosand, W. L., Blake, J., et al. (1991). Beta-lactam antibiotics potentiate magainin 2 antimicrobial activity in vitro and in vivo. Antimicrob. Agents Chemother. 35, 1153–1159. doi: 10.1128/AAC.35.6.1153
Dean, R. E., O’Brien, L. M., Thwaite, J. E., Fox, M. A., Atkins, H., and Ulaeto, D. O. (2010). A carpet-based mechanism for direct antimicrobial peptide activity against vaccinia virus membranes. Peptides 31, 1966–1972. doi: 10.1016/j.peptides.2010.07.028
Dixon, B. M., Barker, T., McKinnon, T., Cuomo, J., Frei, B., Borregaard, N., et al. (2012). Positive correlation between circulating cathelicidin antimicrobial peptide (hCAP18/LL-37) and 25-hydroxyvitamin D levels in healthy adults. BMC Dermatol. 5, 1–5. doi: 10.1186/1756-0500-5-575
Dua, H. S., Otri, A. M., Hopkinson, A., and Mohammed, I. (2014). In vitro studies on the antimicrobial peptide human beta-defensin 9 (HBD9): signalling pathways and pathogen-related response (an American ophthalmological society thesis). Trans. Am. Ophthalmol. Soc. 112, 50–73.
Dunn, S. P., Heidemann, D. G., Chow, C. Y., Crockford, D., Turjman, N., Angel, J., et al. (2010). Treatment of chronic nonhealing neurotrophic corneal epithelial defects with thymosin beta 4. Arch. Ophthalmol. 128, 636–638. doi: 10.1001/archophthalmol.2010.53
Dutta, P., and Das, S. (2016). Mammalian antimicrobial peptides: promising therapeutic targets against infection and chronic inflammation. Curr. Top. Med. Chem. 16, 99–129. doi: 10.2174/1568026615666150703121819
Dutta, D., Kamphuis, B., Ozcelik, B., Thissen, H., Pinarbasi, R., Kumar, N., et al. (2018). Development of silicone hydrogel antimicrobial contact lenses with Mel4 peptide coating. Optom. Vis. Sci. 95, 937–946. doi: 10.1097/OPX.0000000000001282
Dutta, D., Vijay, A. K., Kumar, N., and Willcox, M. D. P. (2016). Melimine-coated antimicrobial contact lenses reduce microbial keratitis in an animal model. Invest. Ophthalmol. Vis. Sci. 57, 5616–5624. doi: 10.1167/iovs.16-19882
Evans, D. J., and Fleiszig, S. M. (2013). Why does the healthy cornea resist Pseudomonas aeruginosa infection? Am J. Ophthalmol. 155, 961.e2–970.e2. doi: 10.1016/j.ajo.2013.03.001
Fruitwala, S., El-Naccache, D. W., and Chang, T. L. (2019). Multifaceted immune functions of human defensins and underlying mechanisms. Semin. Cell Dev. Biol. 88, 163–172. doi: 10.1016/j.semcdb.2018.02.023
Fulton, C., Anderson, G. M., Zasloff, M., Bull, R., and Quinn, A. G. (1997). Expression of natural peptide antibiotics in human skin. Lancet 350, 1750–1751. doi: 10.1016/S0140-6736(05)63574-X
Gallagher, A. G., Alorabi, J. A., Wellings, D. A., Lace, R., Horsburgh, M. J., and Williams, R. L. (2016). A novel peptide hydrogel for an antimicrobial bandage contact lens. Adv. Healthc. Mater. 5, 2013–2018. doi: 10.1002/adhm.201600258
Gallo, R. L., and Hooper, L. V. (2012). Epithelial antimicrobial defence of the skin and intestine. Nat. Rev. Immunol. 12, 503–516. doi: 10.1038/nri3228
Gambichler, T., Skrygan, M., Tomi, N. S., Othlinghaus, N., Brockmeyer, N. H., Altmeyer, P., et al. (2008). Differential mRNA expression of antimicrobial peptides and proteins in atopic dermatitis as compared to psoriasis vulgaris and healthy skin. Int. Arch. Allergy Immunol. 147, 17–24. doi: 10.1159/000128582
Ganz, T. (2003). Defensins: antimicrobial peptides of innate immunity. Nat. Rev. Immunol. 3, 710–720. doi: 10.1038/nri1180
Gao, N., Kumar, A., Guo, H., Wu, X., Wheater, M., and Yu, F. S. X. (2011). Topical flagellin-mediated innate defense against Candida albicans keratitis. Invest. Ophthalmol. Vis. Sci. 52, 3074–3082. doi: 10.1167/iovs.10-5928
Gao, N., Me, R., Dai, C., Seyoum, B., and Yu, F. S. X. (2018). Opposing effects of IL-1Ra and IL-36Ra on innate immune response to Pseudomonas aeruginosa infection in C57BL/6 mouse corneas. J. Immunol. 201, 688–699. doi: 10.4049/jimmunol.1800046
Gao, N., and Yu, F. S. (2015). Chitinase 3-Like 1 promotes Candida albicans killing and preserves corneal structure and function by controlling host antifungal responses. Infect. Immun. 83, 4154–4164. doi: 10.1128/IAI.00980-15
Garreis, F., Gottschalt, M., Schlorf, T., Gläser, R., Harder, J., Worlitzsch, D., et al. (2011). Expression and regulation of antimicrobial peptide psoriasin (S100A7) at the ocular surface and in the lacrimal apparatus. Invest. Ophthalmol. Vis. Sci. 52, 4914–4922. doi: 10.1167/iovs.10-6598
Garreis, F., Schlorf, T., Worlitzsch, D., Steven, P., Bräuer, L., Jäger, K., et al. (2010). Roles of human beta-defensins in innate immune defense at the ocular surface: arming and alarming corneal and conjunctival epithelial cells. Histochem. Cell Biol. 134, 59–73. doi: 10.1007/s00418-010-0713-y
Glaser, R., Harder, J., Lange, H., Bartels, J., Christophers, E., and Schröder, J.-M. (2005). Antimicrobial psoriasin (S100A7) protects human skin from Escherichia coli infection. Nat. Immunol. 6, 57–64. doi: 10.1038/ni1142
Glaser, R., Meyer-Hoffert, U., Harder, J., Cordes, J., Wittersheim, M., Kobliakova, J., et al. (2009a). The antimicrobial protein psoriasin (S100A7) is upregulated in atopic dermatitis and after experimental skin barrier disruption. J. Invest. Dermatol. 129, 641–649. doi: 10.1038/jid.2008.268
Glaser, R., Navid, F., Schuller, W., Jantschitsch, C., Harder, J., Schröder, J. M., et al. (2009b). UV-B radiation induces the expression of antimicrobial peptides in human keratinocytes in vitro and in vivo. J. Allergy Clin. Immunol. 123, 1117–1123. doi: 10.1016/j.jaci.2009.01.043
Goldstein, A. L. (2007). History of the discovery of the thymosins. Ann. N. Y. Acad. Sci. 1112, 1–13. doi: 10.1196/annals.1415.045
Goldstein, A. L., and Badamchian, M. (2004). Thymosins: chemistry and biological properties in health and disease. Expert. Opin. Biol. Ther. 4, 559–573. doi: 10.1517/14712598.4.4.559
Gomariz, R. P., Gutiérrez-Cañas, I., Arranz, A., Carrión, M., Juarranz, Y., and Leceta, J. (2010). Peptides targeting toll-like receptor signalling pathways for novel immune therapeutics. Curr. Pharm. Des. 16, 1063–1080. doi: 10.2174/138161210790963841
Gombart, A. F., Borregaard, N., and Koeffler, H. P. (2005). Human cathelicidin antimicrobial peptide (CAMP) gene is a direct target of the vitamin D receptor and is strongly up-regulated in myeloid cells by 1,25-dihydroxyvitamin D3. FASEB J. 19, 1067–1077. doi: 10.1096/fj.04-3284com
Goncalves, S., Silva, P. M., Felício, M. R., de Medeiros, L. N., Kurtenbach, E., and Santos, N. C. (2017). Psd1 effects on Candida albicans planktonic cells and biofilms. Front. Cell. Infect. Microbiol. 7:249. doi: 10.3389/fcimb.2017.00249
Griffith, G. L., Russell, R. A., Kasus-Jacobi, A., Thavathiru, E., Gonzalez, M. L., Logan, S., et al. (2013). CAP37 activation of PKC promotes human corneal epithelial cell chemotaxis. Invest. Ophthalmol. Vis. Sci. 54, 6712–6723. doi: 10.1167/iovs.13-12054
Guo, C., Rosoha, E., Lowry, M. B., Borregaard, N., and Gombart, A. F. (2013). Curcumin induces human cathelicidin antimicrobial peptide gene expression through a vitamin D receptor-independent pathway. J. Nutr. Biochem. 24, 754–759. doi: 10.1016/j.jnutbio.2012.04.002
Harder, J., Bartels, J., Christophers, E., and Schröder, J. M. (2001). Isolation and characterization of human beta -defensin-3, a novel human inducible peptide antibiotic. J. Biol. Chem. 276, 5707–5713. doi: 10.1074/jbc.M008557200
Harder, J., and Schroder, J. M. (2002). RNase 7, a novel innate immune defense antimicrobial protein of healthy human skin. J. Biol. Chem. 277, 46779–46784. doi: 10.1074/jbc.M207587200
Haynes, R. J., McElveen, J., Dua, H. S., Tighe, P. J., and Liversidge, J. (2000). Expression of human beta-defensins in intraocular tissues. Invest. Ophthalmol. Vis. Sci. 41, 3026–3031.
Haynes, R. J., Tighe, P. J., and Dua, H. S. (1999). Antimicrobial defensin peptides of the human ocular surface. Br. J. Ophthalmol. 83, 737–741. doi: 10.1136/bjo.83.6.737
Hazrati, E., Galen, B., Lu, W., Wang, W., Ouyang, Y., Keller, M. J., et al. (2006). Human alpha- and beta-defensins block multiple steps in herpes simplex virus infection. J. Immunol. 177, 8658–8666. doi: 10.4049/jimmunol.177.12.8658
Heilborn, J. D., Nilsson, M. F., Kratz, G., Weber, G., Sorensen, O., Borregaard, N., et al. (2003a). The cathelicidin anti-microbial peptide LL-37 is involved in re-epithelialization of human skin wounds and is lacking in chronic ulcer epithelium. J. Investig. Dermatol. 130, 379–389.
Heilborn, J. D., Nilsson, M. F., Sørensen, O., Ståhle-Bäckdahl, M., Kratz, G., Weber, G., et al. (2003b). The cathelicidin anti-microbial peptide LL-37 is involved in re-epithelialization of human skin wounds and is lacking in chronic ulcer epithelium. J. Invest. Dermatol. 120, 379–389. doi: 10.1046/j.1523-1747.2003.12069.x
Heilborn, J. D., Weber, G., Grönberg, A., Dieterich, C., and Ståhle, M. (2010). Topical treatment with the vitamin D analogue calcipotriol enhances the upregulation of the antimicrobial protein hCAP18/LL-37 during wounding in human skin in vivo. Exp. Dermatol. 19, 332–338. doi: 10.1111/j.1600-0625.2009.00997.x
Hell, E., Giske, C. G., Nelson, A., Romling, U., and Marchini, G. (2010). Human cathelicidin peptide LL37 inhibits both attachment capability and biofilm formation of Staphylococcus epidermidis. Appl. Microbiol. 50, 211–215. doi: 10.1111/j.1472-765X.2009.02778.x
Herman, A., and Herman, A. P. (2019). Antimicrobial peptides activity in the skin. Skin Res. Technol. 25, 111–117. doi: 10.1111/srt.12626
Hirsch, T., Spielmann, M., Zuhaili, B., Fossum, M., Metzig, M., Koehler, T., et al. (2009). Human beta-defensin-3 promotes wound healing in infected diabetic wounds. J. Gene Med. 11, 220–228. doi: 10.1002/jgm.1287
Ho, J. H., Tseng, K. C., Ma, W. H., Chen, K. H., Lee, O. K., and Su, Y. (2008). Thymosin beta-4 upregulates anti-oxidative enzymes and protects human cornea epithelial cells against oxidative damage. Br. J. Ophthalmol. 92, 992–997. doi: 10.1136/bjo.2007.136747
Howell, M. D., Jones, J. F., Kisich, K. O., Streib, J. E., Gallo, R. L., and Leung, D. Y. M. (2004). Selective killing of vaccinia virus by LL-37: implications for eczema vaccinatum. J. Immunol. 172, 1763–1767. doi: 10.4049/jimmunol.172.3.1763
Hua, X., Yuan, X., Tang, X., Li, Z., Pflugfelder, S. C., and Li, D. Q. (2014). Human corneal epithelial cells produce antimicrobial peptides LL-37 and β-defensins in response to heat-killed Candida albicans. Ophthalmic Res. 51, 179–186. doi: 10.1159/000357977
Huan, Y., Kong, Q., Mou, H., and Yi, H. (2020). Antimicrobial peptides: classification, design, application and research progress in multiple fields. Front. Microbiol. 11:582779. doi: 10.3389/fmicb.2020.582779
Huang, L. C., Jean, D., Proske, R. J., Reins, R. Y., and McDermott, A. M. (2007a). Ocular surface expression and in vitro activity of antimicrobial peptides. Curr. Eye Res. 32, 595–609. doi: 10.1080/02713680701446653
Huang, L. C., Petkova, T. D., Reins, R. Y., Proske, R. J., and McDermott, A. M. (2006). Multifunctional roles of human cathelicidin (LL-37) at the ocular surface. Invest. Ophthalmol. Vis. Sci. 47, 2369–2380. doi: 10.1167/iovs.05-1649
Huang, L. C., Reins, R. Y., Gallo, R. L., and McDermott, A. M. (2007b). Cathelicidin-deficient (Cnlp−/−) mice show increased susceptibility to Pseudomonas aeruginosa keratitis. Invest. Ophthalmol. Vis. Sci. 48, 4498–4508. doi: 10.1167/iovs.07-0274
Jafari, A., Babajani, A., Sarrami Forooshani, R., Yazdani, M., and Rezaei-Tavirani, M. (2022). Clinical applications and anticancer effects of antimicrobial peptides: from bench to bedside. Front. Oncol. 12:819563. doi: 10.3389/fonc.2022.819563
Jia, H. P., Schutte, B. C., Schudy, A., Linzmeier, R., Guthmiller, J. M., Johnson, G. K., et al. (2001). Discovery of new human b-defensins using a genomics-based approach. Gene 263, 211–218. doi: 10.1016/S0378-1119(00)00569-2
Jiang, W., Sunkara, L. T., Zeng, X., Deng, Z., Myers, S. M., and Zhang, G. (2013). Differential regulation of human cathelicidin LL-37 by free fatty acids and their analogs. Peptides 50, 129–138. doi: 10.1016/j.peptides.2013.10.008
Jones, D. E., and Bevins, C. L. (1992). Paneth cells of the human small intestine express an antimicrobial peptide gene. J. Biol. Chem. 267, 23216–23225. doi: 10.1016/S0021-9258(18)50079-X
Joseph, J., and Sharma, S. (2016). “Antifungal therapy in eye infections: new drugs, new trends,” in Recent Trends in Antifungal Agents and Antifungal Therapy. eds. A. Basak, R. Chakraborty, and S. M. Mandal (India, New Delhi: Springer), 217–236.
Kalaiselvan, P., Konda, N., Pampi, N., Vaddavalli, P. K., Sharma, S., Stapleton, F., et al. (2021). Effect of antimicrobial contact lenses on corneal infiltrative events: a randomized clinical trial. Transl. Vis. Sci. Technol. 10:32. doi: 10.1167/tvst.10.7.32
Kalmodia, S., Son, K. N., Cao, D., Lee, B. S., Surenkhuu, B., Shah, D., et al. (2019). Presence of histatin-1 in human tears and association with aqueous deficient dry eye diagnosis: a preliminary study. Sci. Rep. 9:10304. doi: 10.1038/s41598-019-46623-9
Kao, C. Y., Chen, Y., Thai, P., Wachi, S., Huang, F., Kim, C., et al. (2004). IL-17 markedly up-regulates beta-defensin-2 expression in human airway epithelium via JAK and NF-kappaB signaling pathways. J. Immunol. 173, 3482–3491. doi: 10.4049/jimmunol.173.5.3482
Karadag, R., Bayram, N., Oguztuzun, S., Bayramlar, H., Bozer, B., Simsek, G., et al. (2017). An investigation of human beta-defensins and cathelicidin expression in patients with pterygium. Arq. Bras. Oftalmol. 80, 277–280. doi: 10.5935/0004-2749.20170068
Kennedy, S. M., Deshpande, P., Gallagher, A. G., Horsburgh, M. J., Allison, H. E., Kaye, S. B., et al. (2020). Antimicrobial activity of poly-epsilon-lysine peptide hydrogels against Pseudomonas aeruginosa. Invest. Ophthalmol. Vis. Sci. 61:18. doi: 10.1167/iovs.61.10.18
Khatoon, Z., McTiernan, C. D., Suuronen, E. J., Mah, T. F., and Alarcon, E. I. (2018). Bacterial biofilm formation on implantable devices and approaches to its treatment and prevention. Heliyon 4:e01067.
Khurshid, Z., Najeeb, S., Mali, M., Moin, S. F., Raza, S. Q., Zohaib, S., et al. (2017). Histatin peptides: pharmacological functions and their applications in dentistry. Saudi Pharm. J. 25, 25–31. doi: 10.1016/j.jsps.2016.04.027
Kolar, S. S., Baidouri, H., and McDermott, A. M. (2017). Role of pattern recognition receptors in the modulation of antimicrobial peptide expression in the corneal epithelial innate response to F. solani. Invest. Ophthalmol. Vis. Sci. 58, 2463–2472. doi: 10.1167/iovs.16-20658
Kolar, S. S. N., Luca, V., Baidouri, H., Mannino, G., McDermott, A. M., and Mangoni, M. L. (2015). Esculentin-1a(1-21)NH2: a frog skin-derived peptide for microbial keratitis. Cell. Mol. Life Sci. 72, 617–627. doi: 10.1007/s00018-014-1694-0
Kolar, S. S., Baidouri, H., Hanlon, S., McDermott, A. M., and Deepe, G. S. (2013). Protective role of murine beta-defensins 3 and 4 and cathelin-related antimicrobial peptide in Fusarium solani keratitis. Infect. Immun. 81, 2669–2677. doi: 10.1128/IAI.00179-13
Kolls, J. K., McCray, P. B. Jr., and Chan, Y. R. (2008). Cytokine-mediated regulation of antimicrobial proteins. Nat. Rev. Immunol. 8, 829–835. doi: 10.1038/nri2433
Koon, H. W., Shih, D. Q., Chen, J., Bakirtzi, K., Hing, T. C., Law, I., et al. (2011). Cathelicidin signaling via the toll-like receptor protects against colitis in mice. Gastroenterology 141, 1852.e3–1863.e3. doi: 10.1053/j.gastro.2011.06.079
Koujah, L., Suryawanshi, R. K., and Shukla, D. (2019). Pathological processes activated by herpes simplex virus-1 (HSV-1) infection in the cornea. Cell. Mol. Life Sci. 76, 405–419. doi: 10.1007/s00018-018-2938-1
Kowalski, R. P., Romanowski, E. G., Yates, K. A., and Mah, F. S. (2016). An independent evaluation of a novel peptide mimetic, brilacidin (PMX30063), for ocular anti-infective. J. Ocul. Pharmacol. Ther. 32, 23–27. doi: 10.1089/jop.2015.0098
Krisanaprakornkit, S., Kimball, J. R., and Dale, B. A. (2002). Regulation of human beta-defensin-2 in gingival epithelial cells: the involvement of mitogen-activated protein kinase pathways, but not the NF-kappaB transcription factor family. J. Immunol. 168, 316–324. doi: 10.4049/jimmunol.168.1.316
Kumar, A., Kolar, S. S., Zao, M., McDermott, A. M., and Cai, C. (2011). Localization of antimicrobial peptides on polymerized liposomes leading to their enhanced efficacy against Pseudomonas aeruginosa. Mol. BioSyst. 7, 711–713. doi: 10.1039/c0mb00207k
Lai, Y., Cogen, A. L., Radek, K. A., Park, H. J., MacLeod, D. T., Leichtle, A., et al. (2010). Activation of TLR2 by a small molecule produced by Staphylococcus epidermidis increases antimicrobial defense against bacterial skin infections. J. Invest. Dermatol. 130, 2211–2221. doi: 10.1038/jid.2010.123
Lakhani, P., Patil, A., and Majumdar, S. (2018). Recent advances in topical nano drug-delivery systems for the anterior ocular segment. Ther. Deliv. 9, 137–153. doi: 10.4155/tde-2017-0088
Lehrer, R. I., Bevins, C. L., and Ganz, T. (2005). “Defensins and other antimicrobial peptides and proteins,” Mucosal Immunology. 3rd Edn. eds. J. Mestecky, M. E. Lamm, J. R. McGhee, J. Bienenstock, L. Mayer, and W. Strober (Academic Press), 95–110.
Leonard, B. C., Affolter, V. K., and Bevins, C. L. (2012). Antimicrobial peptides: agents of border protection for companion animals. Vet. Dermatol. 23:177.e36. doi: 10.1111/j.1365-3164.2012.01037.x
Leonard, B. C., Chu, H., Johns, J. L., Gallo, R. L., Moore, P. F., Marks, S. L., et al. (2011). Expression and activity of a novel cathelicidin from domestic cats. PLoS One 6:e18756. doi: 10.1371/journal.pone.0018756
Lesniak, W., and Graczyk-Jarzynka, A. (2015). The S100 proteins in epidermis: topology and function. Biochim. Biophys. Acta 1850, 2563–2572. doi: 10.1016/j.bbagen.2015.09.015
Li, S. A., Liu, J., Xiang, Y., Wang, Y. J., Lee, W. H., and Zhang, Y. (2014). Therapeutic potential of the antimicrobial peptide OH-CATH30 for antibiotic-resistant Pseudomonas aeruginosa keratitis. Antimicrob. Agents Chemother. 58, 3144–3150. doi: 10.1128/AAC.00095-14
Liu, C. H., Lee, G. W., Wu, W. C., and Wang, C. C. (2020). Encapsulating curcumin in ethylene diamine-beta-cyclodextrin nanoparticle improves topical cornea delivery. Colloids Surf. B: Biointerfaces 186:110726. doi: 10.1016/j.colsurfb.2019.110726
Liu, P., Pieper, R., Tedin, L., Martin, L., Meyer, W., Rieger, J., et al. (2014). Effect of dietary zinc oxide on jejunal morphological and immunological characteristics in weaned piglets. J. Anim. Sci. 92, 5009–5018. doi: 10.2527/jas.2013-6690
Liu, S., Zhou, L., Li, J., Suresh, A., Verma, C., Foo, Y. H., et al. (2008). Linear analogues of human beta-defensin 3: concepts for design of antimicrobial peptides with reduced cytotoxicity to mammalian cells. Chembiochem 9, 964–973. doi: 10.1002/cbic.200700560
Madsen, P., Rasmussen, H. H., Leffers, H., Honoré, B., Dejgaard, K., Olsen, E., et al. (1991). Molecular cloning, occurrence, and expression of a novel partially secreted protein "psoriasin" that is highly up-regulated in psoriatic skin. J. Invest. Dermatol. 97, 701–712. doi: 10.1111/1523-1747.ep12484041
Maisetta, G., Batoni, G., Esin, S., Luperini, F., Pardini, M., Bottai, D., et al. (2003). Activity of human beta-defensin 3 alone or combined with other antimicrobial agents against oral bacteria. Antimicrob. Agents Chemother. 47, 3349–3351. doi: 10.1128/AAC.47.10.3349-3351.2003
Mannis, M. J. (2002). The use of antimicrobial peptides in ophthalmology: an experimental study in corneal preservation and the management of bacterial keratitis. Trans. Am. Ophthalmol. Soc. 100, 243–271.
Martineau, A. R., Wilkinson, K. A., Newton, S. M., Floto, R. A., Norman, A. W., Skolimowska, K., et al. (2007). IFN-gamma- and TNF-independent vitamin D-inducible human suppression of mycobacteria: the role of cathelicidin LL-37. J. Immunol. 178, 7190–7198. doi: 10.4049/jimmunol.178.11.7190
Martinez, L. R., and Casadevall, A. (2006). Cryptococcus neoformans cells in biofilms are less susceptible than planktonic cells to antimicrobial molecules produced by the innate immune system. Infect. Immun. 74, 6118–6123. doi: 10.1128/IAI.00995-06
Martin-Serrano, A., Gómez, R., Ortega, P., and de la Mata, F. J. (2019). Nanosystems as vehicles for the delivery of antimicrobial peptides (AMPs). Pharmaceutics 11. doi: 10.3390/pharmaceutics11090448
Martinsson, H., Yhr, M., and Enerback, C. (2005). Expression patterns of S100A7 (psoriasin) and S100A9 (calgranulin-B) in keratinocyte differentiation. Exp. Dermatol. 14, 161–168. doi: 10.1111/j.0906-6705.2005.00239.x
Matsuzaki, K. (2019). Membrane permeabilization mechanisms. Adv. Exp. Med. Biol. 1117, 9–16. doi: 10.1007/978-981-13-3588-4_2
Matsuzaki, K., Sugishita, K., Fujii, N., and Miyajima, K. (1995). Molecular basis for membrane selectivity of an antimicrobial peptide, magainin 2. Biochemist 34, 3423–3429. doi: 10.1021/bi00010a034
Matsuzaki, K., Sugishita, K.-I., Ishibe, N., Ueha, M., Nakata, S., Miyajima, K., et al. (1998). Relationship of membrane curvature to the formation of pores by Magainin 2. Biochemist 37, 11856–11863. doi: 10.1021/bi980539y
McDermott, A. M. (2009). The role of antimicrobial peptides at the ocular surface. Ophthalmic Res. 41, 60–75. doi: 10.1159/000187622
McDermott, A. M., Redfern, R. L., and Zhang, B. (2001). Human beta-defensin 2 is up-regulated during re-epithelialization of the cornea. Curr. Eye Res. 22, 64–67. doi: 10.1076/ceyr.22.1.64.6978
McDermott, A. M., Redfern, R. L., Zhang, B., Pei, Y., Huang, L., and Proske, R. J. (2003). Defensin expression by the cornea: multiple signalling pathways mediate IL-1beta stimulation of hBD-2 expression by human corneal epithelial cells. Invest. Ophthalmol. Vis. Sci. 44, 1859–1865. doi: 10.1167/iovs.02-0787
McIntosh, R. S., Cade, J. E., al-Abed, M., Shanmuganathan, V., Gupta, R., Bhan, A., et al. (2005). The spectrum of antimicrobial peptide expression at the ocular surface. Invest. Ophthalmol. Vis. Sci. 46, 1379–1385. doi: 10.1167/iovs.04-0607
McPhee, J. B., Scott, M. G., and Hancock, R. E. (2005). Design of host defence peptides for antimicrobial and immunity enhancing activities. Comb. Chem. High Throughput Screen. 8, 257–272. doi: 10.2174/1386207053764558
Mischke, D., Korge, B. P., Marenholz, I., Volz, A., and Ziegler, A. (1996). Genes encoding structural proteins of epidermal cornification and S100 calcium-binding proteins form a gene complex ("epidermal differentiation complex") on human chromosome 1q21. J. Invest. Dermatol. 106, 989–992. doi: 10.1111/1523-1747.ep12338501
Mogensen, T. H. (2009). Pathogen recognition and inflammatory signaling in innate immune defenses. Clin. Microbiol. Rev. 22, 240–273. doi: 10.1128/CMR.00046-08
Mohammed, I., Suleman, H., Otri, A. M., Kulkarni, B. B., Chen, P., Hopkinson, A., et al. (2010). Localization and gene expression of human beta-defensin 9 at the human ocular surface epithelium. Invest. Ophthalmol. Vis. Sci. 51, 4677–4682. doi: 10.1167/iovs.10-5334
Mohammed, I., Yeung, A., Abedin, A., Hopkinson, A., and Dua, H. S. (2011). Signalling pathways involved in ribonuclease-7 expression. Cell. Mol. Life Sci. 68, 1941–1952. doi: 10.1007/s00018-010-0540-2
Mookherjee, N., Brown, K. L., and Hancock, R. E. W. (2013). “Cathelicidins,” in Handbook of Biologically Active Peptides. 2nd Edn. ed. J. Kastin (Academic Press), 77–84.
Moon, S. K., Lee, H. Y., Li, J. D., Nagura, M., Kang, S. H., Chun, Y. M., et al. (2002). Activation of a Src-dependent Raf–MEK1:2–ERK signaling pathway is required for IL-1a-induced upregulation of h-defensin 2 in human middle ear epithelial cells. Biochem. Biophys. Acta 1590, 41–51. doi: 10.1016/S0167-4889(02)00196-9
Nagaoka, I., Hirota, S., Yomogida, S., Ohwada, A., and Hirata, M. (2000). Synergistic actions of antibacterial neutrophil defensins and cathelicidins. Inflamm. Res. 49, 73–79. doi: 10.1007/s000110050561
Nakatsuji, T., Kao, M. C., Zhang, L., Zouboulis, C. C., Gallo, R. L., and Huang, C. M. (2010). Sebum free fatty acids enhance the innate immune defense of human sebocytes by upregulating beta-defensin-2 expression. J. Invest. Dermatol. 130, 985–994. doi: 10.1038/jid.2009.384
Narayanan, S., Corrales, R. M., Farley, W., McDermott, A. M., and Pflugfelder, S. C. (2008). Interleukin-1 receptor-1-deficient mice show attenuated production of ocular surface inflammatory cytokines in experimental dry eye. Cornea 27, 811–817. doi: 10.1097/ICO.0b013e31816bf46c
Niyonsaba, F., Ogawa, H., and Nagaoka, I. (2004). Human beta-defensin-2 functions as a chemotactic agent for tumour necrosis factor-alpha-treated human neutrophils. Immunology 111, 273–281. doi: 10.1111/j.0019-2805.2004.01816.x
O’Neil, T. A., Porter, E. M., Elewaut, D., Anderson, G. M., Eckmann, L., Ganz, T., et al. (1999). Expression and regulation of the human beta-defensins hBD-1 and hBD-2 in intestinal epithelium. J. Immunol. 163, 6718–6724.
Oppenheim, F. G., Xu, T., McMillian, F. M., Levitz, S. M., Diamond, R. D., Offner, G. D., et al. (1988). Histatins, a novel family of histidine-rich proteins in human parotid secretion. Isolation, characterization, primary structure, and fungistatic effects on Candida albicans. J. Biol. Chem. 263, 7472–7477. doi: 10.1016/S0021-9258(18)68522-9
Otri, A. M., Mohammed, I., al-Aqaba, M. A., Fares, U., Peng, C., Hopkinson, A., et al. (2012). Variable expression of human beta defensins 3 and 9 at the human ocular surface in infectious keratitis. Invest. Ophthalmol. Vis. Sci. 53, 757–761. doi: 10.1167/iovs.11-8467
Otte, J. M., Werner, I., Brand, S., Chromik, A. M., Schmitz, F., Kleine, M., et al. (2008). Human beta defensin 2 promotes intestinal wound healing in vitro. J. Cell. Biochem. 104, 2286–2297. doi: 10.1002/jcb.21787
Oudhoff, M. J., Bolscher, J. G. M., Nazmi, K., Kalay, H., Hof, W., Amerongen, A. V. N., et al. (2008). Histatins are the major wound-closure stimulating factors in human saliva as identified in a cell culture assay. FASEB J. 22, 3805–3812. doi: 10.1096/fj.08-112003
Overhage, J., Campisano, A., Bains, M., Torfs, E. C. W., Rehm, B. H. A., and Hancock, R. E. W. (2008). Human host defense peptide LL-37 prevents bacterial biofilm formation. Infect. Immun. 76, 4176–4182. doi: 10.1128/IAI.00318-08
Oydanich, M., Epstein, S. P., Gadaria-Rathod, N., Guers, J. J., Fernandez, K. B., and Asbell, P. A. (2018). In vivo efficacy of histatin-1 in a rabbit animal model. Curr. Eye Res. 43, 1215–1220. doi: 10.1080/02713683.2018.1490772
Park, M. S., Kim, J. I., Lee, I., Park, S., Bae, J. Y., and Park, M. S. (2018). Towards the application of human defensins as antivirals. Biomol. Ther. 26, 242–254. doi: 10.4062/biomolther.2017.172
Park, K., Kim, Y. I., Shin, K. O., Seo, H. S., Kim, J. Y., Mann, T., et al. (2014). The dietary ingredient, genistein, stimulates cathelicidin antimicrobial peptide expression through a novel S1P-dependent mechanism. J. Nutr. Biochem. 25, 734–740. doi: 10.1016/j.jnutbio.2014.03.005
Peric, M., Koglin, S., Dombrowski, Y., Groß, K., Bradac, E., Büchau, A., et al. (2009). Vitamin D analogs differentially control antimicrobial peptide/"alarmin" expression in psoriasis. PLoS One 4:e6340. doi: 10.1371/journal.pone.0006340
Prasad, S. V., Fiedoruk, K., Daniluk, T., Piktel, E., and Bucki, R. (2019). Expression and function of host defense peptides at inflammation sites. Int. J. Mol. Sci. 21. doi: 10.3390/ijms21010104
Prosser, D. E., and Jones, G. (2004). Enzymes involved in the activation and inactivation of vitamin D. Trends Biochem. Sci. 29, 664–673. doi: 10.1016/j.tibs.2004.10.005
Rademacher, F., Simanski, M., Schröder, L., Mildner, M., and Harder, J. (2017). The role of RNase 7 in innate cutaneous defense against Pseudomonas aeruginosa. Exp. Dermatol. 26, 227–233. doi: 10.1111/exd.13166
Raqib, R., Sarker, P., Bergman, P., Ara, G., Lindh, M., Sack, D. A., et al. (2006). Improved outcome in shigellosis associated with butyrate induction of an endogenous peptide antibiotic. Proc. Natl. Acad. Sci. U. S. A. 103, 9178–9183. doi: 10.1073/pnas.0602888103
Ravagnan, G., de Filippis, A., Cartenì, M., de Maria, S., Cozza, V., Petrazzuolo, M., et al. (2013). Polydatin, a natural precursor of resveratrol, induces beta-defensin production and reduces inflammatory response. Inflammation 36, 26–34. doi: 10.1007/s10753-012-9516-8
Redfern, R. L., Reins, R. Y., and McDermott, A. M. (2011). Toll-like receptor activation modulates antimicrobial peptide expression by ocular surface cells. Exp. Eye Res. 92, 209–220. doi: 10.1016/j.exer.2010.12.005
Reins, R. Y., Baidouri, H., and McDermott, A. M. (2015). Vitamin D activation and function in human corneal epithelial cells during TLR-induced inflammation. Invest. Ophthalmol. Vis. Sci. 56, 7715–7727. doi: 10.1167/iovs.15-17768
Reins, R. Y., Hanlon, S. D., Magadi, S., and McDermott, A. M. (2016). Effects of topically applied vitamin D during corneal wound healing. PLoS One 11:e0152889. doi: 10.1371/journal.pone.0152889
Ren, M., Zhang, S., Liu, X., Li, S., Mao, X., Zeng, X., et al. (2016). Different lipopolysaccharide branched-chain amino acids modulate porcine intestinal endogenous beta-defensin expression through the Sirt1/ERK/90RSK pathway. J. Agric. Food Chem. 64, 3371–3379. doi: 10.1021/acs.jafc.6b00968
Rivas-Santiago, B., Hernandez-Pando, R., Carranza, C., Juarez, E., Contreras, J. L., Aguilar-Leon, D., et al. (2008). Expression of cathelicidin LL-37 during Mycobacterium tuberculosis infection in human alveolar macrophages, monocytes, neutrophils, and epithelial cells. Infect. Immun. 76, 935–941. doi: 10.1128/IAI.01218-07
Rohrl, J., Yang, D., Oppenheim, J. J., and Hehlgans, T. (2008). Identification and biological characterization of mouse beta-defensin 14, the orthologue of human beta-defensin 3. J. Biol. Chem. 283, 5414–5419. doi: 10.1074/jbc.M709103200
Roy, S., Marla, S., and Praneetha, D. C. (2015). Recognition of Corynebacterium pseudodiphtheriticum by toll-like receptors and up-regulation of antimicrobial peptides in human corneal epithelial cells. Virulence 6, 716–721. doi: 10.1080/21505594.2015.1066063
Sahl, H. G., Pag, U., Bonness, S., Wagner, S., Antcheva, N., and Tossi, A. (2005). Mammalian defensins: structures and mechanism of antibiotic activity. J. Leukoc. Biol. 77, 466–475. doi: 10.1189/jlb.0804452
Sang, Y., Teresa Ortega, M., Rune, K., Xiau, W., Zhang, G., Soulages, J. L., et al. (2007). Canine cathelicidin (K9CATH): gene cloning, expression, and biochemical activity of a novel pro-myeloid antimicrobial peptide. Dev. Comp. Immunol. 31, 1278–1296. doi: 10.1016/j.dci.2007.03.007
Sara, S., Sharpe, K., and Morris, S. (2016). Multidrug-resistant Fusarium keratitis: diagnosis and treatment considerations. BMJ Case Rep. 2016:bcr2016215401. doi: 10.1136/bcr-2016-215401
Sass, V., Schneider, T., Wilmes, M., Körner, C., Tossi, A., Novikova, N., et al. (2010). Human beta-defensin 3 inhibits cell wall biosynthesis in staphylococci. Infect. Immun. 78, 2793–2800. doi: 10.1128/IAI.00688-09
Schaefer, L., Hernandez, H., Coats, R. A., Yu, Z., Pflugfelder, S. C., Britton, R. A., et al. (2022). Gut-derived butyrate suppresses ocular surface inflammation. Sci. Rep. 12:4512. doi: 10.1038/s41598-022-08442-3
Schulthess, J., Pandey, S., Capitani, M., Rue-Albrecht, K. C., Arnold, I., Franchini, F., et al. (2019). The short chain fatty acid butyrate imprints an antimicrobial program in macrophages. Immunity 50, 432.e7–445.e7. doi: 10.1016/j.immuni.2018.12.018
Sechet, E., Telford, E., Bonamy, C., Sansonetti, P. J., and Sperandio, B. (2018). Natural molecules induce and synergize to boost expression of the human antimicrobial peptide beta-defensin-3. Proc. Natl. Acad. Sci. U. S. A. 115, E9869–E9878. doi: 10.1073/pnas.1805298115
Sel, S., Trau, S., Paulsen, F., Kalinski, T., Stangl, G. I., and Nass, N. (2016). 1,25-dihydroxyvitamin D3 inhibits corneal wound healing in an ex-vivo mouse model. Graefes Arch. Clin. Exp. Ophthalmol. 254, 717–724. doi: 10.1007/s00417-016-3267-4
Selsted, M. E., Harwig, S. S., Ganz, T., Schilling, J. W., and Lehrer, R. I. (1985). Primary structures of three human neutrophil defensins. J. Clin. Invest. 76, 1436–1439. doi: 10.1172/JCI112121
Shah, D., Ali, M., Pasha, Z., Jaboori, A. J., Jassim, S. H., Jain, S., et al. (2016). Histatin-1 expression in human lacrimal epithelium. PLoS One 11:e0148018. doi: 10.1371/journal.pone.0148018
Shah, D., Son, K. N., Kalmodia, S., Lee, B. S., Ali, M., Balasubramaniam, A., et al. (2020). Wound healing properties of Histatin-5 and identification of a functional domain required for histatin-5-induced cell migration. Mol. Ther. - Methods Clin. Dev. 17, 709–716. doi: 10.1016/j.omtm.2020.03.027
Shah, D., Ali, M., Shukla, D., Jain, S., and Aakalu, V. K. (2017). Effects of histatin-1 peptide on human corneal epithelial cells. PLoS One 12:e0178030
Shao, Z. J., Zheng, X. W., Feng, T., Huang, J., Chen, J., Wu, Y. Y., et al. (2012). Andrographolide exerted its antimicrobial effects by upregulation of human beta-defensin-2 induced through p38 MAPK and NF-kappaB pathway in human lung epithelial cells. Can. J. Physiol. Pharmacol. 90, 647–653. doi: 10.1139/y2012-050
Sharma, P., Sharma, N., Mishra, P., Joseph, J., Mishra, D. K., Garg, P., et al. (2019). Differential expression of antimicrobial peptides in Streptococcus pneumoniae keratitis and STAT3-dependent expression of LL-37 by Streptococcus pneumoniae in human corneal epithelial cells. Pathogens 8. doi: 10.3390/pathogens8010031
Shaykhiev, R., Beißwenger, C., Kändler, K., Senske, J., Püchner, A., Damm, T., et al. (2005). Human endogenous antibiotic LL-37 stimulates airway epithelial cell proliferation and wound closure. Am. J. Phys. Lung Cell. Mol. Phys. 289, L842–L848. doi: 10.1152/ajplung.00286.2004
Sherman, H., Chapnik, N., and Froy, O. (2006). Albumin and amino acids upregulate the expression of human beta-defensin 1. Mol. Immunol. 43, 1617–1623. doi: 10.1016/j.molimm.2005.09.013
Silvestro, L., Gupta, K., Weiser, J. N., and Axelsen, P. H. (1997). The concentration-dependent membrane activity of Cecropin A. Biochemist 36, 11452–11460. doi: 10.1021/bi9630826
Singh, P. K., Tack, B. F., McCray, P. B. Jr., and Welsh, M. J. (2000). Synergistic and additive killing by antimicrobial factors found in human airway surface liquid. Am. J. Phys. Lung Cell. Mol. Phys. 279, L799–L805. doi: 10.1152/ajplung.2000.279.5.L799
Sorensen, O. E., Follin, P., Johnsen, A. H., Calafat, J., Tjabringa, G. S., Hiemstra, P. S., et al. (2001). Human cathelicidin, hCAP-18, is processed to the antimicrobial peptide LL-37 by extracellular cleavage with proteinase 3. Blood 97, 3951–3959. doi: 10.1182/blood.V97.12.3951
Sosne, G., Christopherson, P. L., Barrett, R. P., and Fridman, R. (2005). Thymosin-beta4 modulates corneal matrix metalloproteinase levels and polymorphonuclear cell infiltration after alkali injury. Invest. Ophthalmol. Vis. Sci. 46, 2388–2395. doi: 10.1167/iovs.04-1368
Sosne, G., Dunn, S. P., and Kim, C. (2015a). Thymosin beta4 significantly improves signs and symptoms of severe dry eye in a phase 2 randomized trial. Cornea 34, 491–496. doi: 10.1097/ICO.0000000000000379
Sosne, G., Hafeez, S., Greenberry, A. L., and Kurpakus-Wheater, M. (2002). Thymosin beta4 promotes human conjunctival epithelial cell migration. Curr. Eye Res. 24, 268–273. doi: 10.1076/ceyr.24.4.268.8414
Sosne, G., Kim, C., and Kleinman, H. K. (2015b). Thymosin beta4 significantly reduces the signs of dryness in a murine controlled adverse environment model of experimental dry eye. Expert. Opin. Biol. Ther. 15(Suppl. 1), S155–S161. doi: 10.1517/14712598.2015.1019858
Sosne, G., Qiu, P., Christopherson, P. L., and Wheater, M. K. (2007). Thymosin beta 4 suppression of corneal NFkappaB: a potential anti-inflammatory pathway. Exp. Eye Res. 84, 663–669. doi: 10.1016/j.exer.2006.12.004
Sosne, G., Rimmer, D., Kleinman, H. K., and Ousler, G. (2016). Thymosin beta 4: a potential novel therapy for neurotrophic keratopathy, dry eye, and ocular surface diseases. Vitam. Horm. 102, 277–306. doi: 10.1016/bs.vh.2016.04.012
Sosne, G., Xu, L., Prach, L., Mrock, L. K., Kleinman, H. K., Letterio, J. J., et al. (2004). Thymosin beta 4 stimulates laminin-5 production independent of TGF-beta. Exp. Cell Res. 293, 175–183. doi: 10.1016/j.yexcr.2003.09.022
Srisomboon, Y., Poonyachoti, S., and Deachapunya, C. (2017). Soy isoflavones enhance beta-defensin synthesis and secretion in endometrial epithelial cells with exposure to TLR3 agonist polyinosinic-polycytidylic acid. Am. J. Reprod. Immunol. 78. doi: 10.1111/aji.12694
Sunkara, L. T., Zeng, X., Curtis, A. R., and Zhang, G. (2014). Cyclic AMP synergizes with butyrate in promoting beta-defensin 9 expression in chickens. Mol. Immunol. 57, 171–180. doi: 10.1016/j.molimm.2013.09.003
Svensson, D., Nebel, D., and Nilsson, B. O. (2016). Vitamin D3 modulates the innate immune response through regulation of the hCAP-18/LL-37 gene expression and cytokine production. Inflamm. Res. 65, 25–32. doi: 10.1007/s00011-015-0884-z
Takakuwa, A., Nakamura, K., Kikuchi, M., Sugimoto, R., Ohira, S., Yokoi, Y., et al. (2019). Butyric acid and leucine induce alpha-defensin secretion from small intestinal paneth cells. Nutrients 11. doi: 10.3390/nu11112817
Takiguchi, T., Morizane, S., Yamamoto, T., Kajita, A., Ikeda, K., and Iwatsuki, K. (2014). Cathelicidin antimicrobial peptide LL-37 augments interferon-beta expression and antiviral activity induced by double-stranded RNA in keratinocytes. Br. J. Dermatol. 171, 492–498. doi: 10.1111/bjd.12942
Talukder, P., Satho, T., Irie, K., Sharmin, T., Hamady, D., Nakashima, Y., et al. (2011). Trace metal zinc stimulates secretion of antimicrobial peptide LL-37 from Caco-2 cells through ERK and p38 MAP kinase. Int. Immunopharmacol. 11, 141–144. doi: 10.1016/j.intimp.2010.10.010
Tan, W. S. D., Liao, W., Zhou, S., and Wong, W. S. F. (2017). Is there a future for andrographolide to be an anti-inflammatory drug? Deciphering its major mechanisms of action. Biochem. Pharmacol. 139, 71–81. doi: 10.1016/j.bcp.2017.03.024
Tang, Y.-Q., Yuan, J., Osapay, G., Osapay, K., Tran, D., Miller, C. J., et al. (1999). A cyclic antimicrobial peptide produced in primate leukocytes by the ligation of two truncated α-defensins. Science 286, 498–502. doi: 10.1126/science.286.5439.498
Tjabringa, G. S., Ninaber, D. K., Drijfhout, J. W., Rabe, K. F., and Hiemstra, P. S. (2006). Human cathelicidin LL-37 is a chemoattractant for eosinophils and neutrophils that acts via formyl-peptide receptors. Int. Arch. Allergy Immunol. 140, 103–112. doi: 10.1159/000092305
Tsai, P. W., Yang, C. Y., Chang, H. T., and Lan, C. Y. (2011). Human antimicrobial peptide LL-37 inhibits adhesion of Candida albicans by interacting with yeast cell-wall carbohydrates. PLoS One 6:e17755. doi: 10.1371/journal.pone.0017755
Ung, L., Bispo, P. J. M., Shanbhag, S. S., Gilmore, M. S., and Chodosh, J. (2019). The persistent dilemma of microbial keratitis: global burden, diagnosis, and antimicrobial resistance. Surv. Ophthalmol. 64, 255–271. doi: 10.1016/j.survophthal.2018.12.003
Vahavihu, K., Ala-Houhala, M., Peric, M., Karisola, P., Kautiainen, H., Hasan, T., et al. (2010). Narrowband ultraviolet B treatment improves vitamin D balance and alters antimicrobial peptide expression in skin lesions of psoriasis and atopic dermatitis. Br. J. Dermatol. 163, 321–328. doi: 10.1111/j.1365-2133.2010.09767.x
Wang, G. (2014). Human antimicrobial peptides and proteins. Pharmaceuticals 7, 545–594. doi: 10.3390/ph7050545
Wang, J., Huang, N., Xiong, J., Wei, H., Jiang, S., and Peng, J. (2018). Caprylic acid and nonanoic acid upregulate endogenous host defense peptides to enhance intestinal epithelial immunological barrier function via histone deacetylase inhibition. Int. Immunopharmacol. 65, 303–311. doi: 10.1016/j.intimp.2018.10.022
Wang, T. T., Nestel, F. P., Bourdeau, V., Nagai, Y., Wang, Q., Liao, J., et al. (2004). Cutting edge: 1,25-dihydroxyvitamin D3 is a direct inducer of antimicrobial peptide gene expression. J. Immunol. 173, 2909–2912. doi: 10.4049/jimmunol.173.5.2909
Wang, Y., Shan, T., Xu, Z., Liu, J., and Feng, J. (2006). Effect of lactoferrin on the growth performance, intestinal morphology, and expression of PR-39 and protegrin-1 genes in weaned piglets. J. Anim. Sci. 84, 2636–2641. doi: 10.2527/jas.2005-544
White, J. H. (2010). Vitamin D as an inducer of cathelicidin antimicrobial peptide expression: past, present and future. J. Steroid Biochem. Mol. Biol. 121, 234–238. doi: 10.1016/j.jsbmb.2010.03.034
Wiedemann, I., Breukink, E., van Kraaij, C., Kuipers, O. P., Bierbaum, G., de Kruijff, B., et al. (2001). Specific binding of nisin to the peptidoglycan precursor lipid II combines pore formation and inhibition of cell wall biosynthesis for potent antibiotic activity. J. Biol. Chem. 276, 1772–1779. doi: 10.1074/jbc.M006770200
Willcox, M. D. P., Chen, R., Kalaiselvan, P., Yasir, M., Rasul, R., Kumar, N., et al. (2020). The development of an antimicrobial contact lens: from the laboratory to the clinic. Curr. Protein Pept. Sci. 21, 357–368. doi: 10.2174/1389203720666190820152508
Wu, M., McClellan, S. A., Barrett, R. P., and Hazlett, L. D. (2009). Beta-defensin-2 promotes resistance against infection with P. aeruginosa. J. Immunol. 182, 1609–1616. doi: 10.4049/jimmunol.182.3.1609
Xiaoyan, Z., Xinyi, W., and Li, G. (2011). Pretreatment with lipopolysaccharide modulates innate immunity in corneal fibroblasts challenged with Aspergillus fumigatus. Innate Immun. 17, 237–244. doi: 10.1177/1753425910365364
Xiong, H., Guo, B., Gan, Z., Song, D., Lu, Z., Yi, H., et al. (2016). Butyrate upregulates endogenous host defense peptides to enhance disease resistance in piglets via histone deacetylase inhibition. Sci. Rep. 6:27070. doi: 10.1038/srep27070
Xiong, W. B., Shao, Z. J., Xiong, Y., Chen, J., Sun, Y., Zhu, L., et al. (2015). Dehydroandrographolide enhances innate immunity of intestinal tract through up-regulation the expression of hBD-2. Daru 23:37. doi: 10.1186/s40199-015-0119-4
Xu, J., Xue, Y., Hu, G., Lin, T., Gou, J., Yin, T., et al. (2018). A comprehensive review on contact lens for ophthalmic drug delivery. J. Control. Release 281, 97–118. doi: 10.1016/j.jconrel.2018.05.020
Yang, D., Chen, Q., Schmidt, A. P., Anderson, G. M., Wang, J. M., Wooters, J., et al. (2000). LL-37, the neutrophil granule– and epithelial cell–derived cathelicidin, utilizes Formyl peptide receptor–like 1 (FPRL1) as a receptor to chemoattract human peripheral blood neutrophils, monocytes, and T cells. J. Exp. Med. 192, 1069–1074. doi: 10.1084/jem.192.7.1069
Yang, H. M., Kang, S. W., Sung, J., Kim, K., and Kleinman, H. (2020). Purinergic signaling involvement in thymosin beta4-mediated corneal epithelial cell migration. Curr. Eye Res. 45, 1352–1358. doi: 10.1080/02713683.2020.1748891
Yin, J., and Yu, F. S. (2010). LL-37 via EGFR transactivation to promote high glucose-attenuated epithelial wound healing in organ-cultured corneas. Invest. Ophthalmol. Vis. Sci. 51, 1891–1897. doi: 10.1167/iovs.09-3904
Zanetti, M., Gennaro, R., and Romeo, D. (1995). Cathelicidins: a novel protein family with a common proregion and a variable C-terminal antimicrobial domain. FEBS Lett. 374, 1–5. doi: 10.1016/0014-5793(95)01050-O
Zanetti, M., Litteri, L., Gennaro, R., Hostmann, H., and Romeo, D. (1990). Bactenecins, defense polypeptides of bovine neutrophils, are generated from precursor molecules sotred in the large granules. J. Cell. Biochem. 111, 1363–1371. doi: 10.1083/jcb.111.4.1363
Zhang, J., Dyer, K. D., and Rosenberg, H. F. (2003). Human RNase 7: a new cationic ribonuclease of the RNase A superfamily. Nucleic Acids Res. 31, 602–607. doi: 10.1093/nar/gkg157
Zhang, K., Hussain, T., Wang, J., Li, M., Wang, W., Ma, X., et al. (2020). Sodium butyrate abrogates the growth and pathogenesis of Mycobacterium bovis via regulation of cathelicidin (LL37) expression and NF-kappaB signaling. Front. Microbiol. 11:433. doi: 10.3389/fmicb.2020.00433
Zhang, W., Li, H., Ogando, D. G., Li, S., Feng, M., Price, F. W. Jr., et al. (2017). Glutaminolysis is essential for energy production and ion transport in human corneal endothelium. EBioMedicine 16, 292–301. doi: 10.1016/j.ebiom.2017.01.004
Keywords: antimicrobial peptides, cathelicidin (LL37), defensin, infectious keratitis, microbial keratitis
Citation: Shannon AH, Adelman SA, Hisey EA, Potnis SS, Rozo V, Yung MW, Li JY, Murphy CJ, Thomasy SM and Leonard BC (2022) Antimicrobial Peptide Expression at the Ocular Surface and Their Therapeutic Use in the Treatment of Microbial Keratitis. Front. Microbiol. 13:857735. doi: 10.3389/fmicb.2022.857735
Edited by:
Octavio Luiz Franco, Catholic University of Brasilia (UCB), BrazilReviewed by:
Savitri Sharma, LV Prasad Eye Institute, IndiaMichael Zegans, Dartmouth–Hitchcock Medical Center, United States
Sanhita Roy, LV Prasad Eye Institute, India
Copyright © 2022 Shannon, Adelman, Hisey, Potnis, Rozo, Yung, Li, Murphy, Thomasy and Leonard. This is an open-access article distributed under the terms of the Creative Commons Attribution License (CC BY). The use, distribution or reproduction in other forums is permitted, provided the original author(s) and the copyright owner(s) are credited and that the original publication in this journal is cited, in accordance with accepted academic practice. No use, distribution or reproduction is permitted which does not comply with these terms.
*Correspondence: Brian C. Leonard, YmNsZW9uYXJkQHVjZGF2aXMuZWR1