- The Novo Nordisk Foundation Center for Biosustainability, Technical University of Denmark, Kgs. Lyngby, Denmark
Lack of active export system often limits the industrial bio-based production processes accumulating the intracellular product and hence complexing the purification steps. L-lysine, an essential amino acid, is produced biologically in quantities exceeding two million tons per year; yet, L-lysine production is challenged by efficient export system at high titers during fermentation. To address this issue, new exporter candidates for efficient efflux of L-lysine are needed. Using metagenomic functional selection, we identified 58 genes encoded on 28 unique metagenomic fragments from cow gut microbiome library that improved L-lysine tolerance. These genes include a novel L-lysine transporter, belonging to a previously uncharacterized EamA superfamily, which is further in vivo characterized as L-lysine exporter using Xenopus oocyte expression system as well as Escherichia coli host. This novel exporter improved L-lysine tolerance in E. coli by 40% and enhanced yield, titer, and the specific production of L-lysine in an industrial Corynebacterium glutamicum strain by 7.8%, 9.5%, and 12%, respectively. Our approach allows the sequence-independent discovery of novel exporters and can be deployed to increase titers and productivity of toxicity-limited bioprocesses.
Introduction
The global chemical industry is transitioning from reliance majorly on petrochemical processes to more sustainable bio-based production. This development holds promise to improve the sustainability of the chemical industry while also reducing the overall production costs of chemical products. In order to establish a cost-competitive bioprocess, titers of fermentations frequently exceed 100 gl−1, which leads to a significant stress on the host organism. Indeed, a majority of industrial bioprocesses are limited in production due to several stresses, for example, osmotic stress, leading to product toxicity.
One of the most significant bio-based chemical products is L-lysine. The global bio-based L-lysine production is estimated to reach 3.0 million tons in 2022 corresponding to 5.6 billion USD of market value according to the current L-lysine market report (Elder, 2018). Industrial L-lysine bioprocesses entirely rely on Corynebacterium glutamicum and Escherichia coli production strains that achieve titers over 1.2 M (Becker and Wittmann, 2012; Lee and Kim, 2015). It was observed that the L-lysine export rate is inhibited by 50% upon exceeding the extracellular concentration of 400 mM compared to that at 80 mM (Kelle et al., 1996), indicating the substantial inhibition of the L-lysine-specific export in industrial fermentation. Indeed, several studies have demonstrated the benefit of incorporating active efflux systems to address intracellular product accumulation in bio-based production (Malla et al., 2010; Hemberger et al., 2011; Borodina, 2019). Hence, L-lysine export system is an obvious target to maintain the producer organism at high lysine concentration as well as easing the downstream process. Despite its importance, there are only two identified lysine-specific exporters: (i) LysE as a member of the lysine efflux permease (LysE; 2.A.75) family (Vrljic et al., 1999; Bellmann et al., 2001) and (ii) lysine outward permease (LysO or YbjE in E. coli; Vrljic et al., 1996; Pathania and Sardesai, 2015) achieved five-fold higher lysine export rate upon overexpression of LysE in C. glutamicum (Vrljic et al., 1996). Similarly, Yasueda and coworker have deployed this strategy for 10-fold improvement in L-lysine production by expressing a spontaneously mutated LysE from C. glutamicum in Methylophilis methylotrophus (Gunji and Yasueda, 2006). In addition to the rational engineering of the existing exporters, there is an urgent demand for new genetic building blocks to further improve L-lysine tolerance and production.
Functional metagenomic selection is an effective approach to discover novel genes and enzymes due to its ability to access the wide range of genetic elements present in a particular environmental niche (Sommer et al., 2009, 2010; Munck et al., 2015; Forsberg et al., 2016). Hence, we set out to use functional metagenomic selection to identify novel L-lysine transporter candidates from a cow fecal library, with the goal of improving industrial L-lysine production (Figure 1A). Using C13-labeled L-lysine and mRNA expression of the screened transporter in Xenopus oocyte, the transporter candidate was confirmed as L-lysine exporter. Expression of the metagenomic derived L-lysine transporter improved titer and productivity in both Gram-positive and Gram-negative production strains.
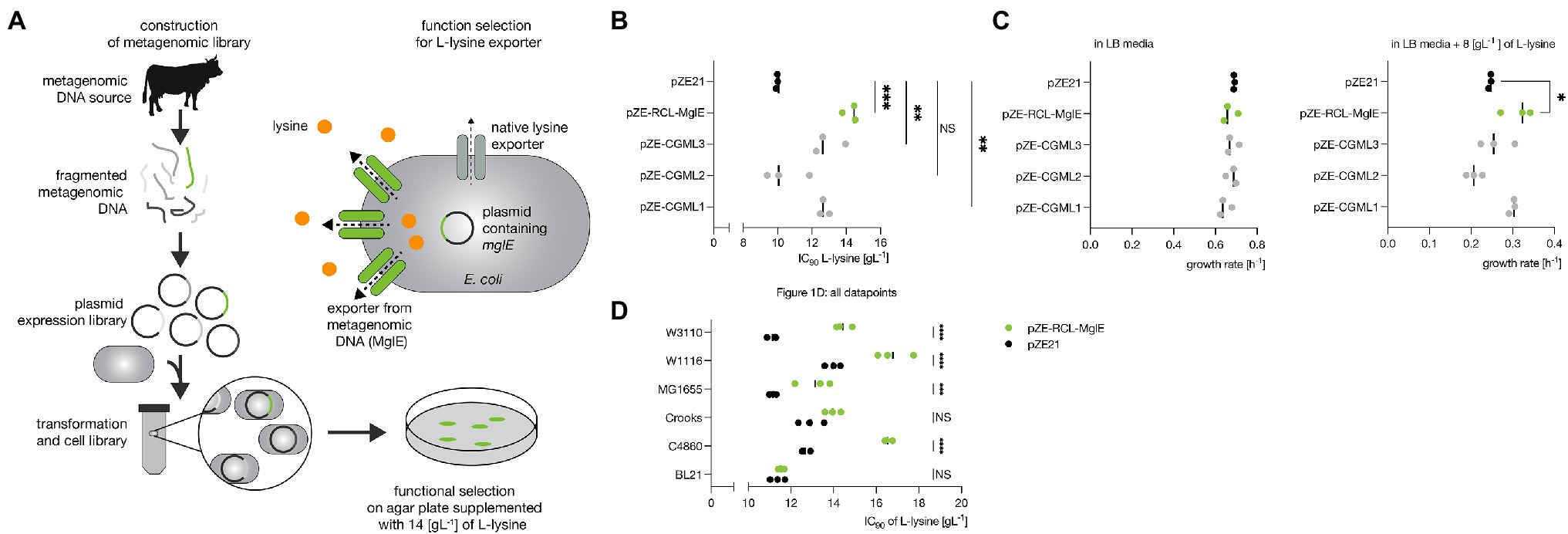
Figure 1. Functional metagenomic screening and Lysine tolerance of the screened metagenomics clones. (A) Functional metagenomic screening for lysine transporters. Cow fecal metagenomic DNA library construction and its functional selection for L-lysine exporters. (B) L-lysine IC90 values for the selected E. coli C4860 resistant clones harboring putative transporters. A one-way ANOVA with Dunnett’s multiple testing correction of the L-Lysine IC90 was applied to compare the metagenomic inserts vs. the empty vector (pZE21). (C) The growth rates of E. coli C4860 harboring pZE-RCL-MglE and pZE21 (control) in LB-Km and LB-Km supplemented with 8 gl−1 of L-lysine. A one-way ANOVA with Dunnett’s correction for multiple testing was used to compare the growth rate of the metagenomic gene inserts vs. the empty vector (pZE21). A significantly different of growth rate (p = 0.0375) was found between MglE and the empty vector control pZE21 at 8 gl−1 of L-lysine. (D) Improved L-lysine tolerance (IC90 values) by expression of metagenomics insert carrying MglE transporter in industrially relevant E. coli strains. A two way ANOVA with Bonferroni correction for multiple testing of the IC90 values was applied per background strain comparing the empty vector (pZE21) against MglE metagenomic insert (pZE-RCL-MglE). In C4860, MG1655, W1116 and W3110 there was a significant difference between the empty vector and MglE (p = <0.0001). For BL21 and Crooks, no significant difference was found (p > 0.05).
Materials and Methods
Bacterial Strains, Growth Conditions, and Chemicals
All bacterial strains, vectors, and plasmids used in this study are listed in Table 1. All oligonucleotide primers (synthesized by Integrated DNA Technologies, Inc.) used are presented in Table 2. E. coli strains were routinely cultured at 37°C in Luria-Bertani (LB) broth or on agar supplemented with kanamycin (35 μg mL−1) when necessary (hereafter referred as LB-km). For the L-lysine production in E. coli LB as well as M9 minimal media supplemented with 2 gl−1 of yeast extract were used and the strains were cultivated in 96-deep well plate in 600 μl of media. Corynebacterium glutamicum strains were cultured in modified CGXII medium (Keilhauer et al., 1993) supplemented with 5% sucrose, 1% BHI, 0.5 mM IPTG, and kanamycin (when necessary), at 30°C and 250 RPM. Growth media were supplemented with 1.5% agar for plate assays.
DNA manipulations were carried out following standard protocols (Sambrook and Russell, 2001). All chemicals were purchased from Sigma-Aldrich (St. Louis, MO, United States). Restriction enzymes and T4 DNA ligase were purchased from Fermentas (Denmark) and New England Biolabs (Hertfordshire, United Kingdom). DNA sequencing was performed using an automated DNA sequence analyzer.
Metagenomic Library Construction and Functional Screening for L-Lysine
A metagenomic expression library of total DNA extracted from a cow fecal sample was constructed as described previously (Sommer et al., 2009). Briefly, library construction involves (i) the isolation of total DNA from 5 g of fecal material using the PowerMax Soil DNA Isolation Kit (MO BIO Laboratories Inc.), (ii) fragmentation of extracted DNA into pieces of an average size of 2 kb by sonication using a Covaris E210 (Massachusetts, United States) followed by end-repair using the End-It end-repair kit (Epicentre), and (iii) blunt-end ligation into the pZE21 MCS1 expression vector with constitutive promoter pLteo-1(Lutz and Bujard, 1997) at the HincII site using the Fast Link ligation kit (Epicentre), and (iv) transformation of the ligated sample into electrocompetent E. coli top10 cells by the standard method (One Shot TOP10 Electrocomp Cells, Invitrogen).
After electroporation, cells were recovered in 1 ml of SOC medium for 1 h at 37°C, and the library was titered by plating out 1, 0.1, and 0.01 μl of recovered cells onto LB-km plates. The insert size distribution was estimated by gel electrophoresis of the PCR products obtained by amplifying inserts using primers annealing to the vector backbone flanking the HincII site. The average insert size for the library was 1.7 kb. The total size of the metagenomics library was determined by multiplying the average insert size by the number of colony-forming units (CFU) per ml (5 × 108 bp). The remainder of the recovered cells were inoculated into 10 ml of LB-km liquid media and grown overnight; the library was frozen down in 15% glycerol and stored at −80°C.
The E. coli C4860 strain was used for the functional screening of L-lysine exporters. First, a plasmid prep of the metagenomics library was carried out from the E. coli top10 cells harboring the library. Then, 400 ng of metagenomics plasmid DNA was transformed into electrocompetent E. coli C4860 cells, and the library titer was determined as described above. On the basis of the determined library sizes and the titer of the library, 106 cells (i.e., 100 μl of the library cells) were plated on LB-km agar supplemented with L-lysine at the selective concentration (14 gl−1). Plates were incubated at 37°C, and the growth of colonies (likely lysine-tolerant clones) was assayed after 48–65 h of incubation.
The metagenomic inserts present in L-lysine-tolerant clones were Sanger sequenced using the pZE21_F and pZE21_R primer pair, which annealed to the vector backbone. The resulting raw sequencing chromatogram files were analyzed and functionally annotated using the deFUME web server (van der Helm et al., 2015): http://www.cbs.dtu.dk//services/deFUME/.
Minimum Inhibitory Concentration and IC90 Determination
For minimum inhibitory concentration (MIC) determination, the E. coli strains were cultured in LB liquid media at 37°C overnight, and then approximately 1 × 104 cells were inoculated from the overnight cultures into LB liquid media and grown at 30°C and 300 RPM in 96-well microtiter plates containing 150 μl of medium per well. MICs were determined using a logarithmic L-lysine (or chemical) concentration gradient with two-fold serial dilutions. Endpoint absorbance measurements (A600nm) were taken with a plate reader (Synergy H1, BioTek) after 24 or 48 h of incubation and were background-subtracted. Growth inhibition of the E. coli strains was plotted against L-lysine (chemical) concentration with a polynomial interpolation between neighboring data points using R software.1 The percentage of inhibition was calculated using the formula: 1 − [A600nm lysine (or chemical)/A600nm control]. The inhibitory concentration was defined as the lowest concentration of the chemical that inhibited 90% of the growth of the strain tested (IC90).
Growth Experiments
Single colonies of the tolerant clone(s) harboring transporter homologues were grown overnight at 37°C with shaking in liquid LB-km medium. The OD values at 600 nm [(OD)600nm] were determined by 10-fold dilution. Then, the cultures were diluted to adjust the (OD)600nm to 0.1, and 5 μl of each culture was inoculated in 150 μl fresh media containing L-lysine (0 and 8 gl−1) and kanamycin in a 96-well microtiter plate. The plate was incubated at 37°C for 24 h in an automated spectrophotometer (ELx808, BioTek) that recorded the (OD)630nm at an interval of 60 min. The data were subsequently retrieved and analyzed to determine growth rates. The growth rate data are the average of triplicate experiments, with error bars representing the standard error of the mean (SEM).
PCR and Escherichia coli Transformation
PCR was performed in a total volume of 50 μl under the following DNA amplification conditions: 95°C for 5 min, followed by 30 cycles of 95°C for 30 s, 50°C–65°C for 30 s, and 72°C for 1 min, and finally 72°C for 5 min. Electrocompetent or chemically competent E. coli cells were transformed with the ligation mixture using a standard protocol and plated onto LB-km agar plates for selection.
In silico Analysis
The amino acid sequence of MglE (Metagenomics gene for lysine Export) was analyzed using Interpro,2 its 2D membrane topology was predicted using Phobius (Käll et al., 2007) and visualized using Protter (Omasits et al., 2014), and the phylogenetic tree was plotted using iTOL. The UniProtKB database (UniProt Consortium, 2014) was accessed on June 1 2016 to query the closest homologs of MglE. Pfam version 29.0 (December 2015; Finn et al., 2014) was used to extract the LysE family (Pfam id: PF01810) members. The Maximum Likelihood Phylogenetic tree was constructed using CLC Main Workbench 7 with the neighbor-joining construction method.
Transport Assays in Xenopus Oocytes
The Xenopus laevis oocytes were obtained from EcoCyte Bioscience (Germany). Oocytes were kept in Kulori buffer (pH 7.4) and at 18°C. A linear cassette (including T7 promoter, mglE as the gene of interest, and 3’UTR) was amplified from the plasmid pUSER016-T7-MglE using Phusion Hot Start polymerase (Thermo Fisher Scientific) and the primers linear_cassette F and linear_cassette R. The linear cassette was used as template for in vitro transcription. Capped cRNAs was synthesized using the mMESSAGE mMACHINE T7 Transcription Kit (AM1344; Thermo Fisher). The quality and quantity of the RNA were determined by Agilent 2,100 Bioanalyzer. For microinjection of cRNA and 13C-labeled L-lysine, we used the RoboInject (Multi Channel Systems, Reutlingen, Germany) automatic injection system (Darbani et al., 2019). Injection needles with opening of 25 μm were used (Multi Channel Systems, MCS GmbH). For expression in oocytes, 25 ng of the in vitro transcribed cRNA for the MglE transporter was microinjected into the oocytes 3 days prior to transport assays. To perform the export assay, 50 nl of the 13C-labeled L-lysine stock solution was injected into the oocytes to obtain estimated internal concentrations of 6 mM, assuming an after-injection dilution factor of 20 (Darbani et al., 2016). Following four washing steps, each batch of 20 oocytes was incubated for 180 min in 90 μl Kulori buffer at pH 5 or pH 7.4. After incubation, 70 μl of the medium was collected from each batch with intact oocytes and added onto 70 μl of 60% MeOH before LC–MS analysis. The oocytes were washed four times with Kulori buffer pH 7.4 and intracellular metabolites were extracted in 30% MeOH to analyzed on LC–MS.
Escherichia coli Double Deletion Mutant Construction
The chromosomal lysine decarboxylases ldcC and cadA in E. coli W3110 were successively knocked out by PCR targeting to create E.coli DMLC strain. The gene disruption process was carried out as described previously using an ampicillin-resistant pSIJ8 helper plasmid containing both the λ Red and FLP systems (Jensen et al., 2015). The primer pair IdcC_F2/IdcC_R (situated 64 bp away from the start/stop codon ldcC gene) was used to amplify the kanamycin cassette from the genomic DNA of the ldcC inframe knocked out Keio strain b0186 whereas the primer pair CadA_F2/CadA_R (situated 56 bp away from the start/stop codon cadA gene) was used to amplify the kanamycin cassette from the genomic DNA of the cadA inframe knock-out Keio strain b4131. These PCR products were used to delete ldcC and cadA in E. coli W3110 strain. The detailed process of construction of E. coli DMLC is given in Supplementary Material.
Plasmid Construction
Subcloning the Metagenomic Insert Implicated in Tolerance Phenotypes
The recombinant plasmids pZE-MglE and pZE0-P-MglE-T were constructed as described below. The construction of recombinant plasmids was verified by both restriction mapping and direct nucleotide sequencing of the respective genes in the recombinant plasmids.
Using the oligonucleotide pair MglE_F/MglE_R and the pZE-RCL-MglE plasmid as template DNA, the exact orf of the putative carboxylate/amino acid transporter homologue (referred to as MglE) was amplified. The amplicon was purified with a Qiagen PCR purification kit and digested with the restriction enzymes KpnI and HindIII (NEB, United Kingdom), followed by ligation using T4 DNA ligase (Fermentas, Denmark) into the corresponding restriction sites of the multiple cloning site (MCS) of the pZE21 vector to construct the recombinant plasmids pZE-MglE.
To construct the recombinant plasmid pZE0-P-MglE-T, USER cloning was applied. The pZE21-vector backbone without its promoter (pZE0) was amplified using the oligonucleotide pair pZE21_User_F/pZE21_User_R. The putative carboxylate/amino acid transporter homologue and the native promoter and terminator sequences (P-MglE-T fragment) were amplified from the pZE-RCL-MglE plasmid using the primer pair P-MglE-T_User_F/P-MglE-T_User_F. The amplified PCR products were ligated into the recombinant plasmid pZE0-P-MglE-T using the recommended standard USER cloning protocol (NEB, United Kingdom).
Codon-Optimized mglE Plasmids Construction for Oocytes Expression Study
For functional analysis of MglE in Xenopus oocytes, we constructed recombinant plasmid pUSER016-pT7-MglE by USER cloning. The pUSER016 vector backbone was digested with PacI for 18 h and the ends were further nicked with Nt.BbvCI for 2 h. The codon-optimized mglE (sequence provided in Supplementary Data) for Xenopus laevis was synthetized along with N-terminal linker sequence—GGCTTAAU and C-terminal linker sequences—ATTAAACC (in the complementary sequence, T is replaced by U for pairing with A) including uracil with compatible USER sites easing direct cloning into linearized and nicked pUSER016 vector by USER cloning (USER, NEB), resulting in plasmid pUSER016-pT7-MglE.
Recombinant Plasmids With Escherichia coli Lysine Exporter and Homologous Genes of mglE
The lysine exporter, ybjE (900 bp, GenBank accession no. CAQ31402), from E. coli BL21 (DE3) was amplified using oligonucleotides YbjE-F and YbjE-R. The PCR product of ybjE was cloned into pZE21 vector excised with KpnI and BamHI restriction enzymes to construct pZE-YbjE expression plasmid.
Similarly, four mglE homologous genes: (i) Gene1 (891 bp, 82% identity, GenBank accession no. CDC57518) from Bacteroides coprophilus CAG:333, (ii) Gene2 (891 bp, 78% identity, GenBank accession no. WP_018711839) from Bacteroides barnesiae, (iii) Gene3 (870 bp, 54% identity, GenBank accession no. CDC66277) from Bacteroides sp. CAG:770, and (iv) Gene4 (912 bp, 43% identity, GenBank accession no. CCZ76555) from Alistipes finegoldii CAG, were synthetized along with N-terminal linker sequence—AATTCATU AAAGAGGAGAAAGGTACC and C-terminal linker sequence—GTCGACGGTATCG ATAAGCTT (in the complementary sequence, T is replaced by U for pairing with A) including uracil easing direct user cloning. These four synthetized gene fragments were cloned into the linear PCR fragment of pZE21 vector amplified by using oligonucleotides pZE21_User-F4 and pZE21_User-R4 (Table 2) to construct pZE-Gene1, pZE-Gene2, pZE-Gene3 and pZE-Gene4 recombinant plasmids, respectively.
Construction of Recombinant Industrial Corynebacterium glutamicum Strains
The full metagenomics insert derived from pZE-RCL-MglE plasmid was amplified using the primer pair RCL-MglE-F/RCL-MglE-R. The resulting PCR product was cloned into pEKEx2 vector, using the KpnI and SacI restriction sites, yielding expression plasmid pEK-RCL-MglE. The newly constructed expression plasmid was introduced into the industrial L-lysine producing strain C. glutamicum VL5 by electroporation and concomitant selection on kanamycin containing 2xTY-Agar. Successful construction of the new strain, named C. glutamicum VL5/pEK-RCL-MglE, was verified by re-isolation of the plasmid, followed by control digest and PCR on the length of the insert. The same procedure was performed to introduce the empty pEKEx2 expression vector into C. glutamicum VL5, yielding control strain C. glutamicum VL5/pEKEx2.
Analysis of L-Lysine in Supernatant of Bacterial Culture
A calibration curve of an authentic standard was generated using concentrations ranging from 0.4 to 77 mg L−1. The accurate mass of L-lysine from 20-fold diluted samples (supernatants containing L-lysine for quantification) was analyzed using an LC–MS Fusion (Thermo Fisher Scientific, United States) with positive electrospray ionization (ESI+). The final concentration was adjusted for the dilution factor. Bracketing calibration was used for the quantification of the external concentration. For the quantification of L-lysine, an LC–MS/MS, EVOQ (Bruker, Fremont United States) was used with multiple reaction monitoring (MRM) transition in positive ionization mode (ESI+), with the quantified transition 147 → 84 (CE 10) and qualifier transition 147 → 130 (CE 7). The significance of the specific lysine production was calculated using a Student t-test.
Results and Discussion
Identification of Metagenomic L-Lysine Tolerance Genes
The gut microbiota from industrial livestock animals are conceivably enriched with the microbes capable to survive at higher L-lysine concentration which is supplied as food additives. A metagenomic library derived from cow feces was constructed and used to find candidate genes that could lead to a higher L-lysine tolerance in E. coli C4860 strain. The resulting library was screened for L-lysine tolerance by plating on LB agar plates supplemented with selective concentrations of L-lysine (14 gl−1; Figure 1A; section “Materials and Methods”). Colonies appeared only on the plates with the metagenomic library but not on the control plates with E. coli C4860 harboring empty vector. Eighty L-lysine-tolerant clones were selected for further analysis.
Metagenomic inserts present in those L-lysine-tolerant clones were PCR amplified, sequenced, and annotated using deFUME (van der Helm et al., 2015). In total, 28 unique clones containing 58 complete or partial open reading frames were identified in this analysis. The sequence analysis revealed that resistant clones contained orfs homologous to genes encoding L-lysine modification/degradation enzymes, membrane proteins, signaling proteins, and hypothetical proteins (Supplementary Table S1).
Selection of Transporter Candidates
For the purpose of improving industrial production strains, novel efflux systems constitute more relevant genetic building blocks than those involved in degradation and modification, which would counteract the objective of L-lysine production. Accordingly, we focused our subsequent analysis on orfs encoding potential efflux systems. We identified six unique metagenomic inserts encoding putative membrane proteins, some of which are annotated in GenBank as hypothetical proteins (Supplementary Table S1). Four inserts were selected for further testing based on the absence of putative degradation enzymes flanking the putative transporters on the metagenomic insert.
We determined the L-lysine IC90 values for E. coli transformants where each of the four selected metagenomic inserts have been introduced. The L-lysine IC90 values for these transformants ranged from 10.44 ± 1.277 to 14.25 ± 0.415 gl−1, corresponding to a more than 40% (value of p = 0.0001) increase in the L-lysine IC90 for the clone harboring pZE-RCL-MglE compared to the empty vector control strain (Figure 1B). All of these strains have similar growth profiles in LB medium, whereas the growth rates varied in the liquid LB supplemented with 8 gl−1 of L-lysine. At 8 gl−1 of L-lysine supplementation, the growth rate of the highest tolerant metagenomic clone harboring pZE-RCL-MglE was 30% higher (value of p = 0.0375) than that of the empty vector control (Figure 1C).
Lysine Tolerance by MglE in Escherichia coli Strains
To test whether the pZE-RCL-MglE plasmid carrying the mglE gene could confer L-lysine tolerance to industrially relevant E. coli strains, we introduced the plasmid into various E. coli strains; BL21 (DE3), MG1655, Crooks, W1116, and W3110 and IC90 values of L-lysine were determined for all of the constructed E. coli strains. Interestingly, the IC90 values of L-lysine for most of these E. coli strains (except Crooks and BL21) were increased (p = <0.0001) upon introduction of the pZE-RCL-MglE plasmid (Figure 1D). Of particular interest, the L-lysine IC90 was increased by 30% (p < 0.0001) in W3110, which is a widely used background strain for L-lysine production (Imaizumi et al., 2005). These data demonstrate that the mechanism of L-lysine tolerance mediated by pZE-RCL-MglE is effective across the industrially relevant E. coli strains and accordingly should be generally applicable to industrial E. coli-based L-lysine fermentations.
Protein Sequence Analysis of the Metagenomic Insert Carrying MglE
Sequence analysis of the 1.6 kb PCR amplicon from pZE-RCL-MglE metagenomics insert contained an open reading frame mglE flanked by native promoter and terminator sequences (Figure 2A). The MglE protein consists of 297 amino acids, and its closest homolog is a hypothetical protein from Bacteroides coprophilus (GenBank accession no. WP_008140691) with 82% identity at the amino acid level. The MglE protein is a member of RhaT/EamA-like transporter family of the drug/metabolite transporter (DMT; 2.A.7) superfamily. The MglE contains two copies of the EamA domain, which is found in transporters belonging to the EamA family, at 9–143 aa (pfam (Finn et al., 2014) e-value: 3e−11) and 152–292 aa (pfam e-value 9.6e−12). The members of the EamA family are diverse, and most of their functions are unknown (Franke et al., 2003). Nevertheless, a few proteins belonging to EamA family are well characterized as exporters, such as PecM exports a pigmented compound indigoidine in Erwinia chrysanthemi (Rouanet and Nasser, 2001) and YdeD export metabolites of the cysteine pathway in E. coli (Daßler et al., 2000). The predicted two-dimensional topology of the MglE transporter possesses six cytoplasmic regions, five periplasmic regions, and 10 transmembrane domains, with both the N- and C-terminals in the cytoplasmic region (Figure 2B).
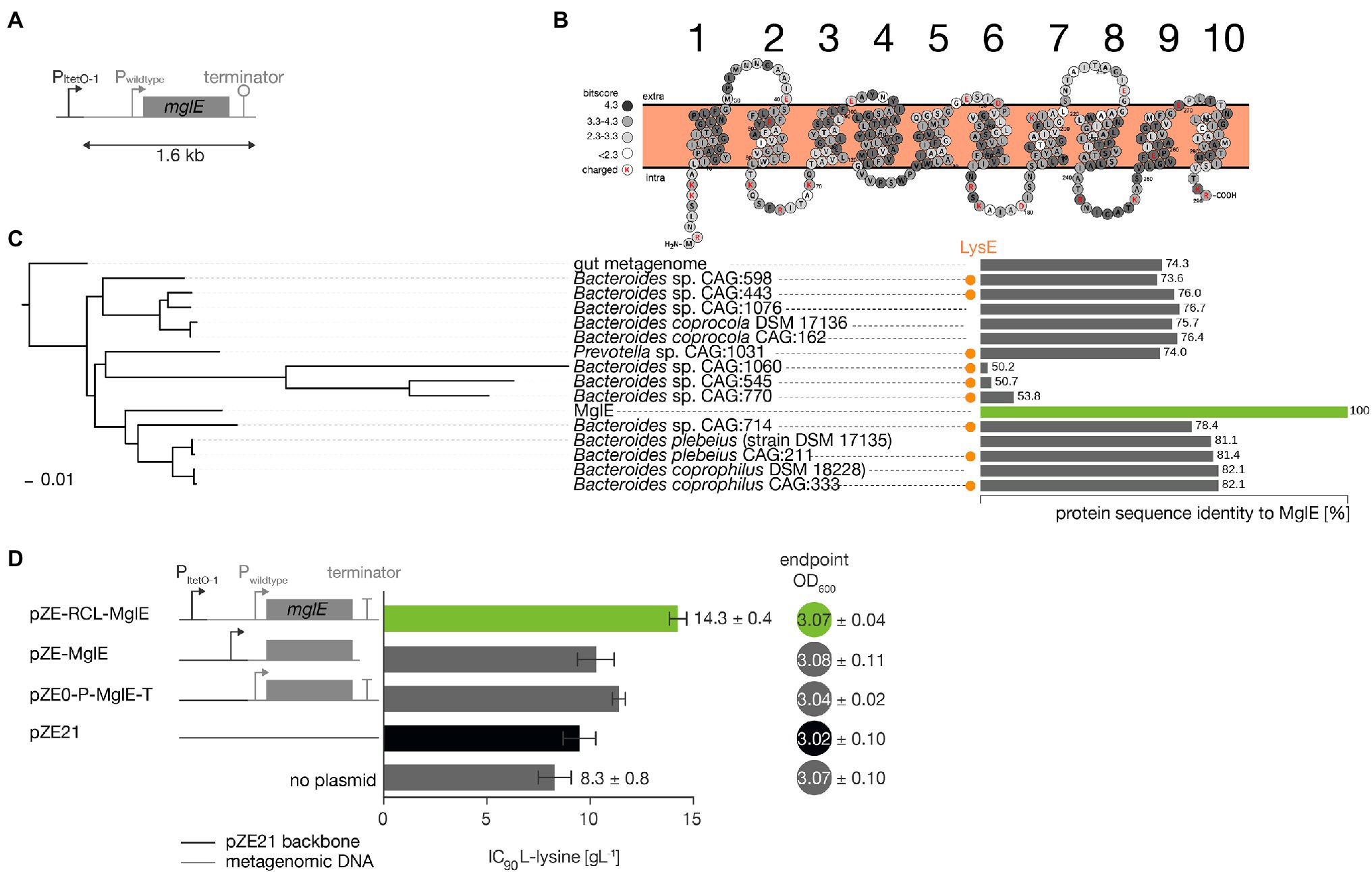
Figure 2. In silico analysis of the metagenomic insert contained in pZE-RCL-MglE plasmid and L-lysine tolerance of its subclones. (A) The metagenomic insert present in the pZE-RCL-MglE plasmid is shown. P: promoter region, MglE: membrane bound transporter protein, and T: terminator sequence. (B) The membrane topology of the MglE transporter protein showing 6 cytoplasmic regions, 5 periplasmic regions, and 10 transmembrane α-helices. The 15 BLASTp hits with a > 50% sequence identity were aligned to MglE and the conservation (bitscore) for each residues was calculated using the Biopython package (Cock et al., 2009). (C) A Maximum Likelihood phylogenetic protein tree containing all the significant (sequence identity >50%) BLASTp hits in the UniProtKB database against MglE. The percentage identity of the 15 hits is shown as horizontal bars. The presence of a LysE family member in the genomes is shown with an orange dot. The highest homology with MglE is found in Bacteroides coprophilus CAG:333, with 82% sequence identity. (D) The strategy for subcloning the MglE transporter with or without its native promoter and the resultant L-lysine tolerance phenotypes for the E. coli C4860 strain are shown. The biomass of the strains (at 24 h) cultured in LB media was also shown in terms of OD in closed circles.
Phylogenetic analysis shows that the closest MglE homologs (>50% sequence identity) are present in the phyla bacteroidetes and are annotated as uncharacterized proteins (Figure 2C). Members of the LysE family (pfam id: PF01810) are mainly (>5 species per phyla) found in the phyla: Proteobacteria, Bacteroidetes, Firmicutes, Actinobacteria. The full list of phyla that contain species with a LysE domain are Proteobacteria: 102, Bacteroidetes: 73, Firmicutes: 45, Actinobacteria: 28, Chloroflexi: 1, Cyanobacteria: 3, Spirochaetes: 1, Verrucomicrobia: 1, and Gemmatimonadetes: 1. Interestingly, nine genomes containing the MglE homolog also contain additional LysE family (pfam id: PF01810, TC code: 2.A.75) genes (Figure 2C orange dot).
Effect of Regions in the Metagenomics Insert on L-Lysine Tolerance
To investigate the effects of promoter region of the metagenomic clone on lysine tolerance, the recombinant plasmids pZE-MglE (mglE cloned into pZE21), and pZE0-P-MglE-T (MglE along with its native promoter and terminator cloned into pZE21 without the pLteo-1 vector promoter) were subcloned from pZE-RCL-MglE plasmid. The constructed plasmids were transformed into E. coli C4860, and IC90 values of L-lysine were determined (Figure 2D). Although these recombinant C4860 strains grew in the same extent in LB media, they showed different L-lysine tolerance. We observed that the IC90 for the strain harboring the pZE0-P-MglE-T plasmid was higher than that of the strain harboring the pZE-MglE plasmid, indicating that the native promoter resulted in higher lysine tolerance than the vector promoter. However, the synergistic effect of these two promoters was better still (i.e., the IC90 values of the pZE-RCL-MglE plasmid were higher than those of the strains harboring pZE-MglE or the pZE0-P-MglE-T plasmids). Hence, the lysine tolerance of the E. coli C4860 strain was the highest upon harboring the full metagenomic insert (i.e., pZE-RCL-MglE).
MglE Exports L-Lysine When Expressed in Xenopus Oocytes
For functional analysis, MglE was expressed in Xenopus oocytes and the export of C13-labeled L-lysine was measured. The water-injected oocytes were used as negative control. L-lysine export assay was performed by injecting C13-labeled L-lysine to obtain a final cytosolic concentration of 6 mM in the oocytes. The oocytes were incubated in Kulori buffers with two different pH (pH 5 and 7.4) for 3 h, and L-lysine concentrations were measured in the buffer and within the oocytes. MglE-expressing oocytes resulted in 6.6-fold and 8.5-fold higher extracellular concentrations of C13-labeled L-lysine and natural C12 L-lysine, respectively, than control oocytes, when buffer with pH 5 was used (Figure 3Ai). The intracellular concentrations of C13-labeled and natural C12 L-lysine were correspondingly 2.1-fold and 1.4-fold lower than in control oocytes (Figure 3Ai). On the other hand, there was no significant change in L-lysine quantities when oocytes were incubated at pH 7.4 (Figure 3Aii). This indicates a proton-gradient dependent export mechanism for MglE transporter.
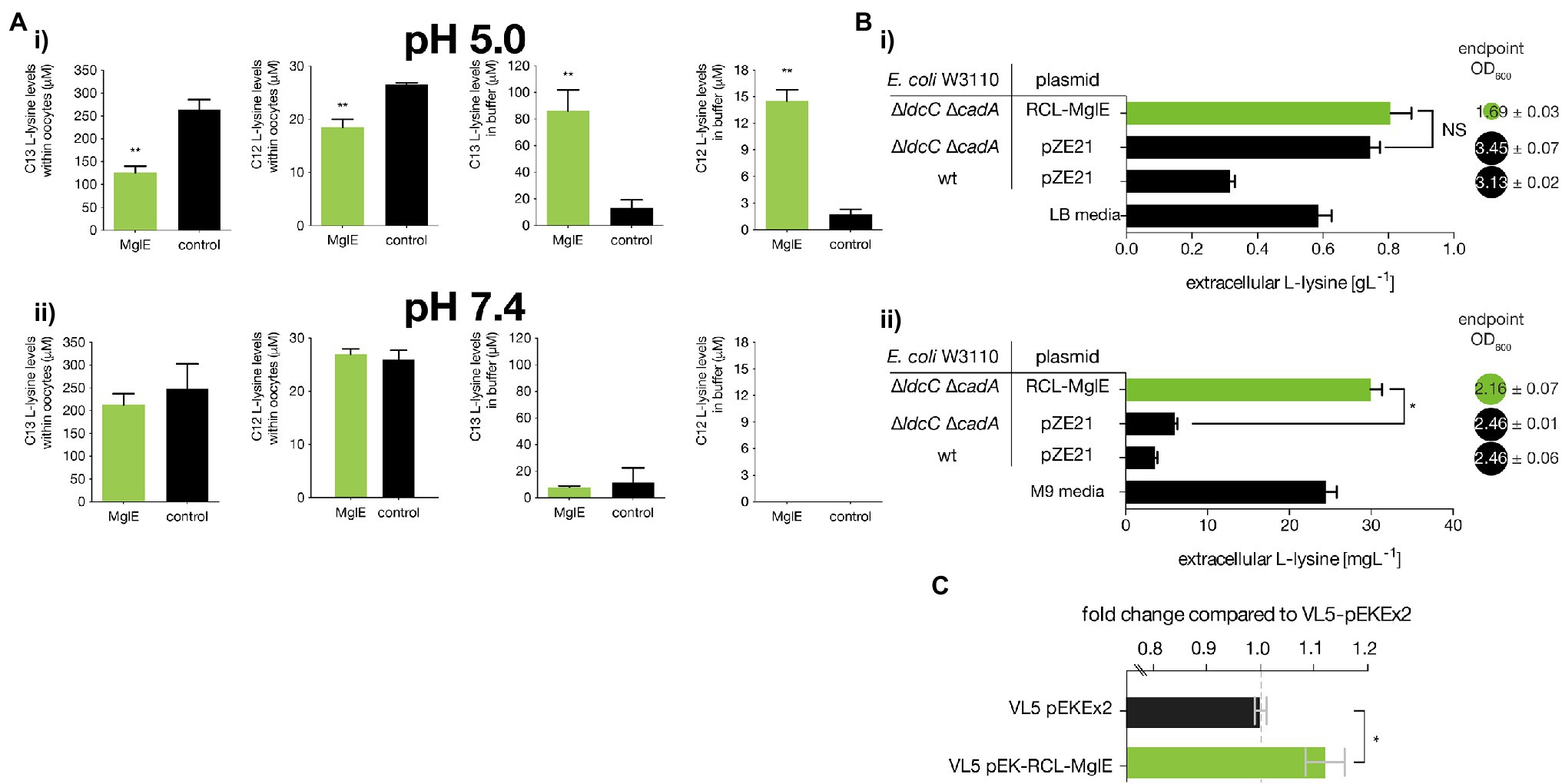
Figure 3. Functional characterization of MglE transporter activity in Xenopus oocytes, E.coli and Corynebacterium glutamicum. (A) L-lysine export assay of MglE transporter in Xenopus oocytes in Kulori buffer (i) at pH 5 and (ii) at pH 7.4. The bars represent lysine contents in the oocytes or the buffer (means ± SD, 3–4 biological replicates each involving 20 oocytes). Significant changes are in comparison with the controls marked by asterisks (** p < 0.01; Fishers one-way ANOVA). (B) The extracellular L-lysine concentration of reference E. coli W3110 and its isogenic mutant strain DMLC along with the cell OD600nm values confirming the exporter activity of the MglE protein in (i) LB media and (ii) in M9 minimal media supplemented with yeast extract (* p < 0.0001, t-test). (C) Fold enhancement of specific production of L-lysine by MglE protein in the industrial C. glutamicum VL5 strain compared to the empty vector control. Error bars are s.e.m., * denotes p = 0.023, n = 4.
MglE Assists L-Lysine Export in Escherichia coli
To analyze the function of MglE for L-lysine efflux, E. coli DMLC strain was constructed by knocking out two lysine decarboxylase genes, constitutive (ldcC) and acid-inducible (cadA). These deletions should prevent L-lysine degradation, thereby leading to increased L-lysine production (Kikuchi et al., 1997). Then, the pZE-RCL-MglE plasmid and pZE21 empty vector were transformed into E. coli DMLC, yielding E. coli DMLC/pZE-RCL-MglE and E. coli DMLC/pZE21, respectively. Subsequently, these strains along with E. coli W3110/pZE21, were cultured in LB media as well as M9 minimal media supplemented with yeast extract in 96 deep well plates with culture volume of 600 μl.
The bacterial growth and extracellular L-lysine concentrations were measured after 24 h.
In both media, upon knocking out ldcC and cadA, there were higher L-lysine concentration in supernatant as compared to the wild-type strain and further the extracellular L-lysine concentration was increased upon expression of MglE operon in the deletion mutant. However, the difference of extracellular L-lysine concentration between E. coli DMLC and E. coli DMLC/pZE-RCL-MglE is not significant in LB media as compared to M9 minimal media because of the presence of higher amount of L-lysine in LB media.
We found that in LB media the absolute extracellular L-lysine titer was increased from 744 to 806 mgl−1 in the presence of the metagenomic insert consisting MglE operon that is, 8.3% higher L-lysine production in the E. coli DMLC/pZE-RCL-MglE strain as compared to E. coli DMLC/pZE21 in LB. However, the biomass of the strain expressing MglE was decreased by about 50% as compared to the control strain (Figure 3Bi). Furthermore, the L-lysine production was analyzed in minimal media supplementing yeast extract. In minimal media, the extracellular L-lysine accumulation was increased from 6 to 30 mgl−1 (p < 0.0001, t-test) whereas there was 12% reduced in biomass upon expressing the MglE metagenomic fragment (Figure 3Bii). The difference in extracellular L-lysine concentration among the strain expressing MglE and the control strain is prominent in minimal media (*p < 0.0001, t-test) than in LB media (p = 0.1997, t-test). Hence, the above results demonstrate that MglE assists exporting L-lysine from E. coli.
The reduced biomass might be due to the combined effect of expression of membrane protein in high copy number and active export of L-lysine by MglE which could have resulted in less available energy for the cell to build biomass. It is worth noting that a big proportion of total cellular energy-demand is for the transport machinery which has accordingly been under evolutionary selection toward a higher energetic efficiency (Darbani et al., 2018).
Improvement of L-Lysine Productivity in Industrial Corynebacterium glutamicum Strain
Expression of a functionally active gene(s) from one strain to another often requires special optimizations. For the practical application in industrial L-lysine bioprocesses, retaining the activity of the discovered exporter into the production host, C. glutamicum, is very essential. To test whether the MglE can boost the industrial L-lysine bioprocesses, we cloned and expressed it in C. glutamicum production strain.
The full metagenomic insert carrying the MglE along with its native promoter and terminator was amplified from the pZE-RCL-MglE plasmid and cloned into pEKEx2 expression vector to construct pEK-RCL-MglE expression plasmid. The constructed plasmid was introduced into C. glutamicum VL5 strain (Materials and methods), a producer strain currently used for industrial L-lysine production. Using sucrose as a carbon source, L-lysine production from the constructed C. glutamicum recombinant strain was analyzed at 30 h. The C. glutamicum VL5 parent strain and VL5 transformed with empty plasmid (i.e., VL5/pEKEx2) produced 26.59 and 27.13 gl−1 of L-lysine respectively, whereas 29.72 gl−1 of L-lysine titer was produced by the strain expressing MglE operon (i.e., VL5/pEX-RCL-MglE). Furthermore, L-lysine yield with respect to amount of sucrose consumed were calculated for these three strains which resulted 0.76, 0.77, and 0.83 gg−1 for the strains VL5, VL5/pEKEx2, and VL5/pEK-RCL-MglE, respectively. Hence, expression of MglE operon into C. glutamicum VL5 improved the L-lysine yield and titer by 7.8% and 9.5%, respectively in comparison to the empty vector control. Unlike in E. coli strains, expression of the full metagenomics insert harboring MglE did not have any growth inhibition effect as the vector control and the recombinant strains both have nearly equal biomass. Notably, upon incorporation of the MglE operon into C. glutamicum, the specific L-lysine production was improved by 12% ± 0.07% relative to the empty vector control (Figure 3C). By expressing the operon in this highly efficient producer strain, we showed not only the functionality of the MglE in a Gram-positive species but also the benefit of the efflux system on an already highly optimized producer strain.
Comparison of L-Lysine Tolerance Conferred by MglE and Its Homologous Genes
The YbjE transporter from E. coli is one of the functionally characterized lysine-specific exporters. Based upon the homology search, four genes in the NCBI database having different homologies (ranging from 43% to 82% in amino acid level) with MglE were also selected and gene synthetized. To find out the lysine tolerance of MglE as compared to that of YbjE and the four selected MglE homologous proteins (with 82%, 78%, 54%, and 43% amino acid sequence identity), recombinant expression plasmids pZE-YbjE, pZE-Gene1, pZE-Gene2, pZE-Gene3, and pZE-Gene4 were constructed. The nucleotide sequences of these genes are provided in the Supplementary Information. The constructed plasmids, pZE-MglE and pZE21 vector control were introduced into E. coli C4860 and E. coli DMLC strains and determined the IC90 of L-lysine for the constructed recombinant strains as described in early experiments.
Interestingly, we found that the expression of MglE provided similar L-lysine tolerance in E. coli C4860 strain as that of YbjE whereas in E. coli DMLC strain, MglE displayed even higher tolerance than YbjE. On the other hand, all of the four MglE homologs showed lower lysine tolerance than MglE in both strains (Figure 4).
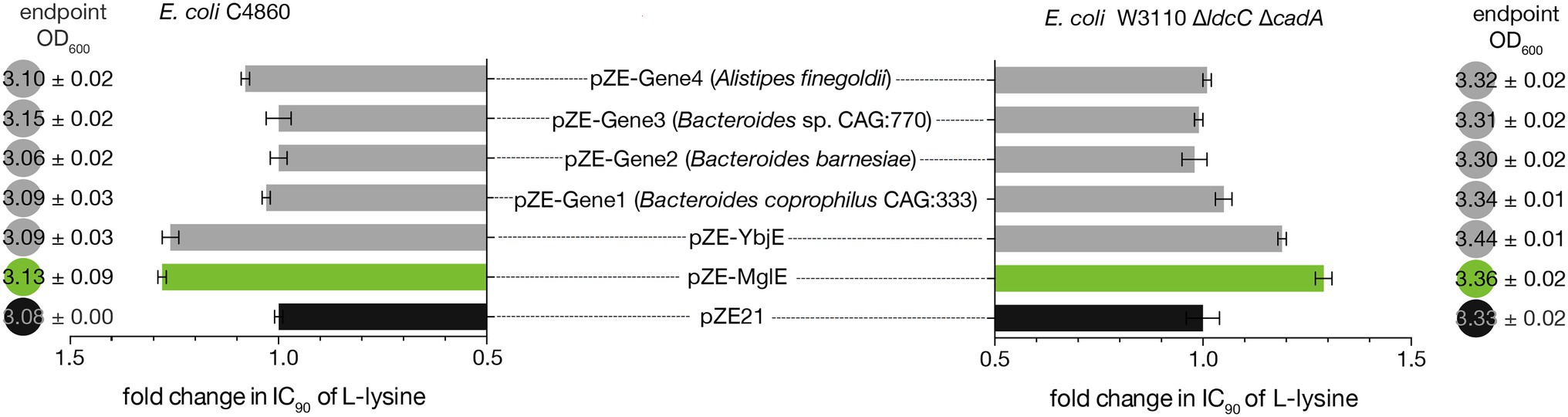
Figure 4. Comparison of lysine tolerance (presented in relative IC90 values) displayed by MglE, YbjE (Lysine-specific exporter from E. coli), and four synthetic MglE homologs. The biomass of the strains (at 24 h) cultured in LB media was also shown in closed circles.
Conclusion
We deployed functional metagenomic selections to identify novel genetic building blocks encoding product efflux systems. Through applying this approach to the problem of L-lysine toxicity at high concentration, we discovered MglE, which is confirmed as a novel type of L-lysine exporter that belongs to the EamA superfamily and this gene could not have been identified by only sequence analysis or homology to known L-lysine transporters. We demonstrated the benefits of MglE in both Gram-negative (E. coli) and Gram-positive (C. glutamicum) strains and showed that MglE expression improves the productivity of an industrial L-lysine production strain. If incorporated into industrial-scale bioprocesses, this discovery has the potential to enhance L-lysine production by about 10%–12%, representing an increased profit on the order of 200–500 million USD per year. Our approach of using functional metagenomics to identify novel transporters is independent of prior knowledge of transporter gene sequences and can be generally applied to most of the toxic compounds/chemicals production by fermentation.
Protein Accession Numbers
The sequence information of full metagenomics insert harboring mglE is deposited in the NCBI GenBank database with the accession number KU708839.
Data Availability Statement
The datasets presented in this study can be found in online repositories. The names of the repository/repositories and accession number(s) can be found in the article/Supplementary Material.
Author Contributions
MS and SM conceived the study and drafted the manuscript. SM designed and performed the E. coli experiments. BD and IB conceived the experiments on Xenopus oocytes. BD performed the experiments. SW performed C. glutamicum experiments. EH performed in silico analysis. MS, IB, and JF led the research teams. EH, BD, SW, JF, and IB contributed to the writing. All authors contributed to the article and approved the submitted version.
Funding
The research leading to these results was funded by the Novo Nordisk Foundation (grant agreement no. NNF10CC1016517) and the European Union Seventh Framework Programme (FP7-KBBE-2013-7-single-stage) under grant agreement no. 613745, Promys. This work was further supported by the European Union Seventh Framework Programme-ITN (FP7/2012/317058 to EH). IB acknowledges the financial support of the European Research Council under the European Union’s Horizon 2020 research and innovation programme (YEAST-TRANS project, grant agreement no. 757384).
Conflict of Interest
The authors declare that the research was conducted in the absence of any commercial or financial relationships that could be construed as a potential conflict of interest.
Publisher’s Note
All claims expressed in this article are solely those of the authors and do not necessarily represent those of their affiliated organizations, or those of the publisher, the editors and the reviewers. Any product that may be evaluated in this article, or claim that may be made by its manufacturer, is not guaranteed or endorsed by the publisher.
Acknowledgments
SW acknowledges funding from Højteknologifonden. The authors would like to thank Vitalys I/S for providing the VL5 strain for lab-scale testing and Christian Munk for helping with R-script to calculate IC90 values.
Supplementary Material
The Supplementary Material for this article can be found online at: https://www.frontiersin.org/articles/10.3389/fmicb.2022.855736/full#supplementary-material
Footnotes
References
Archer, C. T., Kim, J. F., Jeong, H., Park, J., Vickers, C. E., Lee, S., et al. (2011). The genome sequence of E. coli W (ATCC 9637): comparative genome analysis and an improved genome-scale reconstruction of E. coli. BMC Genomics 12:9. doi: 10.1186/1471-2164-12-9
Baba, T., Ara, T., Hasegawa, M., Takai, Y., Okumura, Y., Baba, M., et al. (2006). Construction of Escherichia coli K-12 in-frame, single-gene knockout mutants: the Keio collection. Mol. Syst. Biol. 2:2006.0008. doi: 10.1038/msb4100050
Becker, J., and Wittmann, C. (2012). Systems and synthetic metabolic engineering for amino acid production—the heartbeat of industrial strain development. Curr. Opin. Biotechnol. 23, 718–726. doi: 10.1016/j.copbio.2011.12.025
Bellmann, A., Vrljić, M., Patek, M., Sahm, H., Kramer, R., and Eggeling, L. (2001). Expression control and specificity of the basic amino acid exporter LysE of Corynebacterium glutamicum. Microbiology 147, 1765–1774. doi: 10.1099/00221287-147-7-1765
Blattner, F. R., Plunkett, G. I., Bloch, A. C., Perna, T. N., Burland, V., Riley, M., et al. (1997). The complete genome sequence of Escherichia coli K-12. Science 277, 1453–1462. doi: 10.1126/science.277.5331.1453
Borodina, I. (2019). Understanding metabolite transport gives an upper hand in strain development. Microb. Biotechnol. 12, 69–70. doi: 10.1111/1751-7915.13347
Cock, P. J. A., Antao, T., Chang, J. T., Chapman, B. A., Cox, C. J., Dalke, A., et al. (2009). Biopython: freely available python tools for computational molecular biology and bioinformatics. Bioinformatics 25, 1422–1423. doi: 10.1093/bioinformatics/btp163
Darbani, B., Kell, D. B., and Borodina, I. (2018). Energetic evolution of cellular Transportomes. BMC Genomics 19:418. doi: 10.1186/s12864-018-4816-5
Darbani, B., Motawia, M. S., Olsen, C. E., Nour-Eldin, H. H., Møller, B. L., and Rook, F. (2016). The biosynthetic gene cluster for the cyanogenic glucoside dhurrin in Sorghum bicolor contains its co-expressed vacuolar MATE transporter. Sci. Rep. 6, 1–8. doi: 10.1038/srep37079
Darbani, B., Stovicek, V., van der Hoek, S. A., and Borodina, I. (2019). Engineering energetically efficient transport of dicarboxylic acids in yeast Saccharomyces cerevisiae. Proc. Natl. Acad. Sci. U. S. A. 116, 19415–19420. doi: 10.1073/pnas.1900287116
Daßler, T., Maier, T., Winterhalter, C., and Böck, A. (2000). Identification of a major facilitator protein from Escherichia coli involved in efflux of metabolites of the cysteine pathway. Mol. Microbiol. 36, 1101–1112. doi: 10.1046/j.1365-2958.2000.01924.x
Eikmanns, B. J., Kleinertz, E., Liebl, W., and Sahm, H. (1991). A family of Corynebacterium glutamicum/Escherichia coli shuttle vectors for cloning, controlled gene expression, and promoter probing. Gene 102, 93–98. doi: 10.1016/0378-1119(91)90545-M
Elder, M. (2018). World markets for fermentation ingredients, BCC Research: Market Research Reports. FOD020E. Available at: https://www.bccresearch.com/market-research/food-and-beverage/world-markets-for-fermentation-ingredients-fod020e.html (Accessed January 08, 2022).
Finn, R. D., Bateman, A., Clements, J., Coggill, P., Eberhardt, R. Y., Eddy, S. R., et al. (2014). Pfam: the protein families database. Nucleic Acids Res. 42, D222–D230. doi: 10.1093/nar/gkt1223
Forsberg, K. J., Patel, S., Witt, E., Wang, B., Ellison, T. D., and Dantas, G. (2016). Identification of genes conferring tolerance to lignocellulose-derived inhibitors by functional selections in soil metagenomes. Appl. Environ. Microbiol. 82, 528–537. doi: 10.1128/AEM.02838-15
Franke, I., Resch, A., Daßler, T., Maier, T., and Bock, A. (2003). YfiK from Escherichia coli promotes export of O-acetylserine and cysteine. J. Bacteriol. 185, 1161–1166. doi: 10.1128/JB.185.4.1161
Gunji, Y., and Yasueda, H. (2006). Enhancement of l-lysine production in methylotroph Methylophilus methylotrophus by introducing a mutant LysE exporter. J. Biotechnol. 127, 1–13. doi: 10.1016/j.jbiotec.2006.06.003
Hayashi, K., Morooka, N., Yamamoto, Y., Fujita, K., Isono, K., Choi, S., et al. (2006). Highly accurate genome sequences of Escherichia coli K-12 strains MG1655 and W3110. Mol. Syst. Biol. 2, 1–5. doi: 10.1038/msb4100049
Hemberger, S., Pedrolli, D. B., Stolz, J., Vogl, C., Lehmann, M., and Mack, M. (2011). RibM from Streptomyces davawensis is a riboflavin/roseoflavin transporter and may be useful for the optimization of riboflavin production strains. BMC Biotechnol. 11:119. doi: 10.1186/1472-6750-11-119
Imaizumi, A., Takikawa, R., Koseki, C., Usuda, Y., Yasueda, H., Kojima, H., et al. (2005). Improved production of L-lysine by disruption of stationary phase-specific rmf gene in Escherichia coli. J. Biotechnol. 117, 111–118. doi: 10.1016/j.jbiotec.2004.12.014
Jensen, S. I., Lennen, R. M., Herrgård, M. J., and Nielsen, A. T. (2015). Seven gene deletions in seven days: fast generation of Escherichia coli strains tolerant to acetate and osmotic stress. Sci. Rep. 5:17874. doi: 10.1038/srep17874
Käll, L., Krogh, A., and Sonnhammer, E. L. L. (2007). Advantages of combined transmembrane topology and signal peptide prediction-the Phobius web server. Nucleic Acids Res. 35, W429–W432. doi: 10.1093/nar/gkm256
Keilhauer, C., Eggeling, L., and Sahm, H. (1993). Isoleucine synthesis in Corynebacterium glutamicum: molecular analysis of the ilvB-ilvN-ilvC operon. J. Bacteriol. 175, 5595–5603. doi: 10.1128/jb.175.17.5595-5603.1993
Kelle, R., Laufer, B., Brunzema, C., Weuster-Botz, D., Krämer, R., and Wandrey, C. (1996). Reaction engineering analysis of L-lysine transport by Corynebacterium glutamicum. Biotechnol. Bioeng. 51, 40–50. doi: 10.1002/(SICI)1097-0290(19960705)51:1<40::AID-BIT5>3.0.CO;2-0
Kikuchi, Y., Kojima, H., Tanaka, T., Takatsuka, Y., and Kamio, Y. (1997). Characterization of a second lysine decarboxylase isolated from Escherichia coli. J. Bacteriol. 179, 4486–4492. doi: 10.1128/jb.179.14.4486-4492.1997
Lee, S. Y., and Kim, H. U. (2015). Systems strategies for developing industrial microbial strains. Nat. Biotechnol. 33, 1061–1072. doi: 10.1038/nbt.3365
Lutz, R., and Bujard, H. (1997). Independent and tight regulation of transcriptional units in Escherichia coli via the LacR/O, the TetR/O and AraC/I1-I2 regulatory elements. Nucleic Acids Res. 25, 1203–1210. doi: 10.1093/nar/25.6.1203
Malla, S., Niraula, N. P., Liou, K., and Sohng, J. K. (2010). Self-resistance mechanism in Streptomyces peucetius: overexpression of drrA, drrB and drrC for doxorubicin enhancement. Microbiol. Res. 165, 259–267. doi: 10.1016/j.micres.2009.04.002
Munck, C., Albertsen, M., Telke, A., Ellabaan, M., Nielsen, P. H., and Sommer, M. O. A. (2015). Limited dissemination of the wastewater treatment plant core resistome. Nat. Commun. 6:8452. doi: 10.1038/ncomms9452
Omasits, U., Ahrens, C. H., Müller, S., and Wollscheid, B. (2014). Protter: interactive protein feature visualization and integration with experimental proteomic data. Bioinformatics 30, 884–886. doi: 10.1093/bioinformatics/btt607
Pathania, A., and Sardesai, A. A. (2015). Distinct paths for basic amino acid export in Escherichia coli: YbjE (LysO) mediates export of L-lysine. J. Bacteriol. 197, 2036–2047. doi: 10.1128/JB.02505-14
Rouanet, C., and Nasser, W. (2001). The PecM protein of the phytopathogenic bacterium Erwinia chrysanthemi, membrane topology and possible involvement in the efflux of the blue pigment. J. Mol. Microbiol. Biotechnol. 3, 309–318.
Sambrook, J., and Russell, D. W. (2001). Molecular Cloning: A Laboratory Manual. 3rd Edn. Cold Spring Harbor, NY: Cold Spring Harbor Laboratory Press.
Sommer, M. O. A., Church, G. M., and Dantas, G. (2010). A functional metagenomic approach for expanding the synthetic biology toolbox for biomass conversion. Mol. Syst. Biol. 6:360. doi: 10.1038/msb.2010.16
Sommer, M. O. A., Dantas, G., and Church, G. M. (2009). Functional characterization of the antibiotic resistance reservoir in the human microflora. Science 325, 1128–1131. doi: 10.1126/science.1176950
UniProt Consortium (2014). UniProt: a hub for protein information. Nucleic Acids Res. 43, D204–D212. doi: 10.1093/nar/gku989
van der Helm, E., Geertz-Hansen, H. M., Genee, H. J., Malla, S., and Sommer, M. O. A. (2015). deFUME: dynamic exploration of functional metagenomic sequencing data. BMC. Res. Notes 8, 328–330. doi: 10.1186/s13104-015-1281-y
Vrljic, M., Garg, J., Bellmann, A., Wachi, S., Freudi, R., Malecki, M., et al. (1999). The LysE superfamily: topology of the lysine exporter LysE of Corynebacterium glutamicum, a paradyme for a novel superfamily of transmembrane solute translocators. J. Mol. Microbiol. Biotechnol. 1, 327–336.
Keywords: amino acid, transporter, E. coli, C. glutamicum, Xenopus oocytes
Citation: Malla S, van der Helm E, Darbani B, Wieschalka S, Förster J, Borodina I and Sommer MOA (2022) A Novel Efficient L-Lysine Exporter Identified by Functional Metagenomics. Front. Microbiol. 13:855736. doi: 10.3389/fmicb.2022.855736
Edited by:
Yu Wang, Tianjin Institute of Industrial Biotechnology (CAS), ChinaReviewed by:
Lothar Eggeling, Helmholtz Association of German Research Centres (HZ), GermanyHisashi Kawasaki, The University of Tokyo, Japan
Copyright © 2022 Malla, van der Helm, Darbani, Wieschalka, Förster, Borodina and Sommer. This is an open-access article distributed under the terms of the Creative Commons Attribution License (CC BY). The use, distribution or reproduction in other forums is permitted, provided the original author(s) and the copyright owner(s) are credited and that the original publication in this journal is cited, in accordance with accepted academic practice. No use, distribution or reproduction is permitted which does not comply with these terms.
*Correspondence: Morten Otto Alexander Sommer, bXNvbUBiaW8uZHR1LmRr; Sailesh Malla, ZGtzYWltQGNoci1oYW5zZW4uY29t
†Present addresses: Sailesh Malla, Strain Development Department, Discovery, R&D, Chr. Hansen (A/S), Hørshlom, Denmark
Eric van der Helm, SNIPR BIOME & ODity.bio, Copenhagen, Denmark
Behrooz Darbani, Department of Agroecology, Faculty of Technical Sciences, Aarhus University, Slagelse, Denmark
Jochen Förster, Biosyntia ApS Fruebjergvej, Copenhagen, Denmark