- 1Department of Clinical Sciences, Institute of Tropical Medicine, Antwerp, Belgium
- 2Laboratory of Medical Microbiology, Vaccine and Infectious Disease Institute, University of Antwerp, Antwerp, Belgium
- 3Department of Medicine, University of Cape Town, Cape Town, South Africa
Resistance acquisition via natural transformation is a common process in the Neisseria genus. Transformation has played an important role in the emergence of resistance to many antimicrobials in Neisseria gonorrhoeae and Neisseria meningitidis. In a previous study, we found that currently circulating isolates of Neisseria subflava had acquired an msr(D) gene that has been found to result in macrolide resistance in other bacteria but never found in Neisseria species before. To determine if this resistance mechanism is transferable among Neisseria species, we assessed if we could transform the msr(D) gene into other commensal and pathogenic Neisseria under low dose azithromycin pressure. Intraspecies recombination in commensal N. subflava was confirmed with PCR and resulted in high-level macrolide resistance. Whole-genome sequencing of these transformed strains identified the complete uptake of the msr(D) integration fragment. Sequence analysis showed that a large fragment of DNA (5 and 12 kb) was transferred through a single horizontal gene transfer event. Furthermore, uptake of the msr(D) gene had no apparent fitness cost. Interspecies transformation of msr(D) from N. subflava to N. gonorrhoeae was, however, not successful.
Introduction
Transformation is one of the genetic recombination methods Neisseria gonorrhoeae has used to acquire resistance to every class of antimicrobials used to treat it (Unemo and Shafer, 2014). Via this process, Neisseria species are able to take up environmental DNA and incorporate it into their chromosomes (Hamilton and Dillard, 2006; Rotman and Seifert, 2014). Neisseria species preferably take up DNA from closely related species, especially those that use the same DNA uptake sequence (DUS) for transformation (Duffin and Seifert, 2010). An important consequence of transformation is the transfer of resistance-associated DNA fragments from commensal Neisseria towards pathogenic Neisseria (Nakayama et al., 2016; Wadsworth et al., 2018). Commensal Neisseria are important members of a healthy oral microbiome and hence are present in all humans (Liu et al., 2015; Tedijanto et al., 2018). This high prevalence means they are more likely to be exposed to antimicrobials used for any indication (bystander selection; Kenyon et al., 2021). As a result, commensal Neisseria are particularly at risk for developing antimicrobial resistance (AMR) to commonly used antimicrobials. Along these lines, recent studies have found alarmingly high minimum inhibitory concentrations (MIC) values for fluoroquinolones, macrolides and β-lactams in commensal Neisseria (Dong et al., 2020; Laumen et al., 2021b). Studies have confirmed that transformation of DNA from commensal Neisseria has played an important role in the genesis of resistance to a number of classes of antimicrobials in pathogenic Neisseria: macrolides (mtrR, mtrCDE, rplD and rplY; Wadsworth et al., 2018; Manoharan-Basil et al., 2021), β-Lactams (penA; Bowler et al., 1994; Ito et al., 2005), sulphonamides (folP) and fluoroquinolones (gyrA; Unemo and Shafer, 2014; Chen et al., 2020).
An additional pathway used by the pathogenic Neisseria to acquire AMR has been the uptake of whole genes from other species. Examples of these are the acquisition of the tetM, ermB/C and blaTEM genes that confer resistance to tetracyclines, macrolides and β-Lactams, respectively (Roberts et al., 1999; Unemo and Shafer, 2014). In a previous study, we identified the recent acquisition of a new ribosomal protection protein (MsrD) in N. subflava as a novel resistance mechanism in Neisseria (de Block et al., 2021). The msr(D) gene is part of the antibiotic resistance ATP-binding cassette F (ABC-F) protein family. The four classes of Msr proteins (A, C, D and E) operate as ribosomal protection proteins by displacing macrolides and ketolides from the ribosome. Macrolide resistance conferring msr genes have been identified in various species of Streptococcus, Staphylococcus, Enterococcus, Pseudomonas and Corynebacterium (Sharkey et al., 2016; Dinos, 2017). Complementation studies in these species have clearly established that msr(D) has a powerful effect on macrolide MICs (Daly et al., 2004; Nunez-Samudio and Chesneau, 2013; Zhang et al., 2016; Iannelli et al., 2018; Fostier et al., 2020). In our previous study, we found that the msr(D) in N. subflava was likely derived from the macrolide efflux genetic assembly (MEGA) element in Streptococcus pneumoniae, with whom it shared 100% sequence homology (de Block et al., 2021). As already described in other species, we found that the presence of the msr(D) gene in N. subflava was associated with higher azithromycin MICs (Iannelli et al., 2018; Fox et al., 2021).
In the current paper, we aimed to address four questions that emerged from the previous research: (1) Can the msr(D) gene be transformed into other strains of N. subflava? (2) If so, does this occur at the same insertion site? (3) Does uptake of msr(D) confer a fitness cost? (4) Can the msr(D) gene be transformed into N. gonorrhoeae?
Materials and methods
Intra- and Interspecies Transformation in Plates
The strains used in this experiment were all isolated from oropharyngeal swabs taken from men who have sex with men (MSM) attending our Sexually Transmitted Infections (STI) clinic in Antwerp, Belgium in 2019 (Laumen et al., 2021b). Nine N. subflava strains containing the msr(D) gene (azithromycin MIC ≥24 mg/L) were used as donor and two N. subflava and one N. gonorrhoeae strains without this gene were used as recipients (MIC <1 mg/L; Table 1). Genomic DNA was extracted using the EpiCentre® kit. The DNA concentration (ng/μl) was determined using the NanoDrop® ND-1000 spectrophotometer (Thermo Scientific). One hundred μicroliter of three different donor pools (P1–P3), each containing a mix of three donor DNA extracts of N. subflava (150 ng/μl), were separately mixed with 100 μl (4.0 McFarland) of the mid-log phase growth (6 h) of three recipient strains: (i) N. subflava (ITM_Ns_9/1: azithromycin MIC 3), (ii) N. subflava (ITM_Ns_45/1: azithromycin MIC 6 mg/L and (iii) N. gonorrhoeae (ITM_Ng_38/1: azithromycin MIC 0.19 mg/L; Table 2). Azithromycin concentration of 1.5× MIC was added as a stress factor. Control experiments did not contain azithromycin and/or DNA. The reaction mixtures were plated on blood agar and incubated for 48 h. One colony from each blood agar culture was selected for azithromycin MIC determination E-test gradient strips (bioMerieux, France). All the experiments were conducted at 36°C and 6% CO2.
Inter-species Transformation in Morbidostat
The transformation experiment was performed in a NGmorbidostat. The construction, optimalisation and use of the NGmorbidostat have been described in detail elsewhere (Verhoeven et al., 2019; Laumen et al., 2021a). In brief, the NGmorbidostat is a bioreactor that measures bacterial growth via optical density measurements and is used to assess the evolution of antimicrobial resistance (AMR) over time within a constant temperature (35°C–36°C) and CO2 range (5.5%–6%). In this experiment, we only used the incubator and turbidity measurement functions with the programme MATLAB, to record the growth rate of N. gonorrhoeae (The Math Works, Inc. MATLAB, version R2015b).
The experiment was conducted in four flasks with a total volume of 15 ml in each of gonococcal (GC) broth supplemented with (1%) IsoVitaleX, henceforth referred as GC medium. The conditions were as: (1) 1.5× MIC azithromycin + DNA from msr(D) containing N. subflava, (2) 1.5× MIC azithromycin, (3) DNA from msr(D) containing N. subflava and (4) GC medium (Supplementary Figure 1). To achieve this, firstly we added 200 μl of N. gonorrhoeae (ITM_Ng_21.021 with Azithromycin MIC 1 mg/L; Table 1) at 4.0–5.0 McFarland in all four flasks. After 6 h, the growth curve reached the mid-log phase and 100 μl of HLR-Azithromycin DNA from pool 1 of N. subflava (150 ng/μl) was added to flasks 1 and 3. At the same time point, azithromycin was added to a final concentration of 1.5 mg/L in flasks 1 and 2. After 24 h, 7.5 ml of the old medium was replaced by fresh medium and an additional 100 μl of HLR-Azithromycin DNA from N. subflava (150 ng/μl; flasks 1 and 3) and 1.5 mg/L of azithromycin of the ITM_Ng_21.021 was added (flasks 1 and 2; Table 1). This process was repeated daily for 7 days, after which the azithromycin concentration was increased to 3 mg/L for another 7 days.
Inter-species Transformation With msr(D)-DUS DNA Fragment
Msr(D) was PCR amplified from N. subflava isolate ITM_Ns_41/1 (Table 1) using primers containing a AT-DUS tag to facilitate inter-species transformation to N. gonorrhoeae, forward primer (5’-GAT GCC GTC TGA ACA AAT GAT AAC TGA GG-3’) and reverse primer (5’-GAA TCA ATA CTG ACC AGC GAC-3’). This amplification was carried out as a touchdown PCR: the initial denaturation consisted of 5 min at 95°C, followed by amplification for 10 cycles at 94°C for 30 s, 55°C for 30 s and 72°C for 3 min. The next stage consisted of 35 cycles, lasting 5 more seconds at each cycle, at 94°C for 30 s, 60°C for 30 s and 72°C for 3 min. A final extension step was carried at 72°C for 7 min. The PCR fragment size was analysed on an agarose gel. The concentration of the amplicon was determined using the NanoDrop® ND-1000 spectrophotometer (Thermo Scientific). The PCR product was used for transformation using the ‘Transformation in plates’ methodology as described above with 100 μl (150 ng/μl) as DNA donor.
Confirmation of msr(D) Transformation With qPCR
Presence or absence of the msr(D) gene in transformant strains were confirmed using quantitative PCR (qPCR). The DNA of the recipient strains was extracted using the EpiCentre® kit. The primers used to amplify the internal region of the msr(D) (637–934) were as: Forward (5’-GCG GAG GAA AAG CGA AAA C-3’) and Reverse (5’-ACA GAG CCT TAT CCC CAA ATAC-3’). The master mix was composed by 10× EHF PCR buffer (5 μl), 2 Mm dNTPs (7 μl), 5 μM Primer Forward (3 μl), 5 μM Primer Reverse (3 μl), 3.5 U/μl EHF Taq Polymerase (0.5 μl), Rnase free water (21.5 μl) and DNA (10 μl).The qPCR protocol consisted of an initial denaturation stage at 95°C during 5 min followed by amplification for 45 cycles of 94°C for 30 s, 55°C for 30 s and 72°C for 3 min. This step was followed by the final stage consisting of a single cycle of 72°C for 7 min. The specificity of the amplicon was confirmed by conducting melting point analyses.
Assessment of Fitness Cost Based on MIC Stability
To test the stability of the transformed N. subflava, a single colony of HLR-azithromycin N. subflava strain (ITM_Ns_27/1; azithromycin MIC of 24 μg/ml) and a single colony of one transformant strain of N. subflava (ITM_Ns_9/1; azithromycin MIC of 256 μg/ml) were subcultured every 24 h in blood agar plates without additional azithromycin for 7 days, similar to the one described in O’Regan et al. (2010). The azithromycin MICs were tested daily on a single colony from each plate with E-tests (Table 3).

Table 3. Observation of the azithromycin MIC evolution in ITM_Ns_27/1 donor and ITM_Ns_9/1_P1 transformant strain (both Neisseria subflava) after serial subculturing in plates with absence of azithromycin as stress factor.
Evaluation of Fitness Cost in Transformants by Growth Curves Rate Variance
The NGmorbidostat was used to compare the growth curves of N. subflava recipient and transformant strain. In a total volume of 15 ml of GC broth supplemented with 1% IsoVitalex (BD BBL™) for each experiment, 100 μl of a 4.0 McFarland suspension in PBS of N. subflava recipient (ITM_Ns_9/1) or N. subflava transformant (ITM_Ns_9/1transformant) strain was added in triplicate. The growth curves were assessed for 18 h, via measurement of optical density every 20 min. Difference in growth curves was assessed via analysis with R (R Core Team, 2019) package ‘growthcurver’ (Sprouffske and Wagner, 2016) with the data obtained from the NGmorbidostat. R was also used to perform the t-test on the samples to confirm or deny or null hypothesis and to obtain the value of p (Supplementary Figure 2).
Whole-Genome Sequencing
For whole-genome sequencing (WGS) analysis, the following samples were chosen: (i) DNA recipients after transformation (ITM_Ns_9/1, ITM_Ns_45/1) and (ii) Transformation in morbidostat ITM_Ng_21.021 (Time points 1, 7 and 14 for AZM + DNA and day 14 for the controls). Genomic DNA was extracted using the MasterPure Complete DNA and RNA Purification Kit (Epicentre, Madison, Wisconsin, United States) and suspended in nuclease-free water. Indexed paired-end libraries were prepared using the Nextera XT DNA Library Prep Kit (Illumina, San Diego, CA, United States) and sequenced on an Illumina MiSeq instrument (Illumina, San Diego, CA, United States). Data are available in GenBank: https://www.ncbi.nlm.nih.gov/sra/PRJNA794044. Processed Illumina reads were de novo assembled with Shovill (v1.0.4; https://github.com/tseemann/shovill) which uses SPAdes (v3.14.0) using the following parameters: --trim --depth 150 --opts --isolate (Prjibelski et al., 2020). The quality of the contigs was verified with Quast (v5.0.2; Gurevich et al., 2013) followed by annotation using Prokka (v1.14.6; Seemann, 2014). WGS assemblies of the donor (ITM_Ns_3/2, ITM_Ns_27/1 and ITM_Ns_36/1) and recipient strains (ITM_Ns_9/1, ITM_Ns_45/1 and ITM_Ns_38/1) were available from a previous study by our group and included in the comparative analysis (de Block et al., 2021). BLAST Ring Image Generator (BRIG) was used for genome comparison (Alikhan et al., 2011). Mauve (Darling et al., 2004) was used to align contigs and MEGAX (Kumar et al., 2018) was used to align DNA fragments. Percent sequence identity of DNA fragments was calculated using Muscle (https://www.ebi.ac.uk/Tools/msa/muscle/, version 3.8.31).
Results
Horizontal Gene Transfer of msr(D) From Commensal Neisseria
Intra-species Transformation of msr(D) on Agar Plates
After 48 h of exposure to each of the three pools of high-level resistance (HLR)-azithromycin DNA (donor) on agar plates, both N. subflava recipient strains (ITM_Ns_9/1 and ITM_Ns_45/1; Table 1) attained an azithromycin MIC >256 mg/L (n = 6; Table 2). These isolates are henceforth referred to as transformants. There was no increase in azithromycin MIC in the control experiments. To confirm if the uptake of the msr(D) was successful in these six transformants, the presence of msr(D) was confirmed using qPCR (Table 2). One transformant strain of each recipient was used for WGS.
Inter-species Transformation of msr(D) on Agar Plates
In the three experiments where N. gonorrhoeae was used as recipient, the azithromycin MIC did not increase following incubation with the three donor DNA pools. qPCRs confirmed that msr(D) was not taken up by N. gonorrhoeae in any of these experiments (ct value >30 or NA).
Transformation of Neisseria gonorrhoeae in the NGmorbidostat
Differences were noted in the azithromycin MIC trajectories in the four flasks (Figure 1). The azithromycin MIC of the N. gonorrhoeae recipient increased by day 5 in the flask containing DNA + azithromycin (condition 1) However, the qPCR of msr(D) remained negative in all samples. WGS of samples from day 7 and day 14 revealed a well-known mutation previously linked to macrolide resistance in N. gonorrhoeae: C2611T (Escherichia coli numbering) in the 23S rRNA gene.
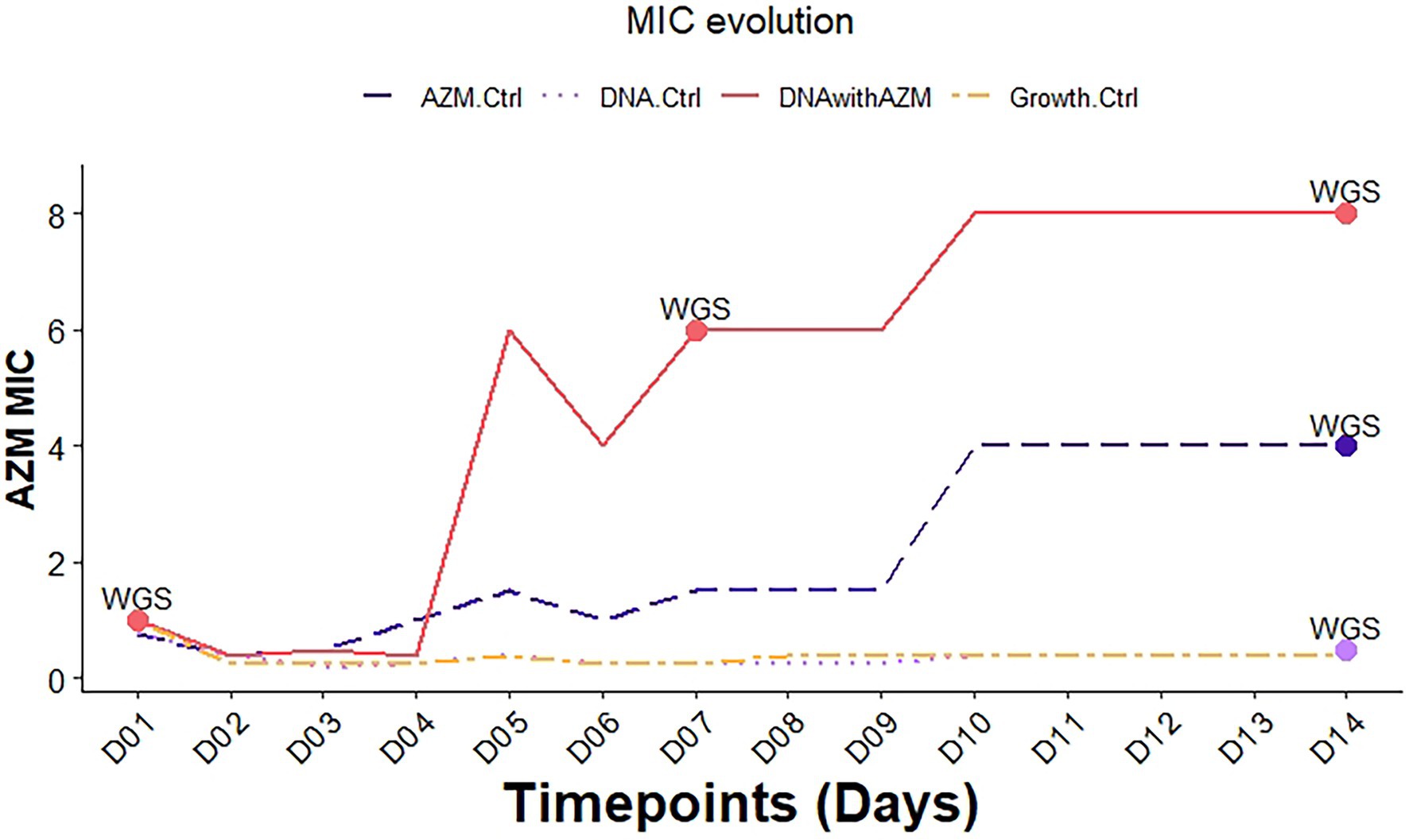
Figure 1. Azithromycin MIC evolution of Neisseria gonorrhoeae in the morbidostat transformation experiment in different conditions. (AZM.Ctrl: azithromycin control (condition 2); DNA.Ctrl: DNA control (condition 3); DNAwithAZM: DNA with azithromycin (condition 1); Growth.Ctrl: Growth control (condition 4); WGS—whole-genome sequencing, D01—day 1, etc., MIC—minimal inhibitory concentrations). The time points when samples were subjected to WGS are indicated with a dot.
WGS of the N. gonorrhoeae recipient in the azithromycin control on day 14 (condition 2) revealed that the recipient acquired the recently described macrolide resistance-associated mutation (RAM) G70D in the 50S ribosomal protein L4 (rplD; Ma et al., 2020; Laumen et al., 2021a).
There was no increase in azithromycin MICs of the N. gonorrhoeae recipient in the DNA control (condition 3) and the growth control (condition 4).
Transformation of Neisseria gonorrhoeae With msr(D) PCR Product
There was no increase in the azithromycin MIC of the N. gonorrhoeae recipient strain after incubation on agar plates for 48 h with a dsDNA fragment containing msr(D) and a DUS. qPCR analysis confirmed that the msr(D) gene was not acquired by the recipient (ct value >30).
Fitness Cost of Transformants
MIC Stability
There was no significant decline in the azithromycin MIC value in the transformant or donor strain during 7 days of subculturing (Table 3).
Growth Rate
There was no statistically significant difference obtained in the growth rate ratios between recipient (ITM_Ns_9/1, mean: 0.64) and transformant strain (ITM_Ns_9/1_P1, mean: 0.67; value of p: 0.3673; Supplementary Figure 2).
Whole-Genome Sequencing of msr(D) Transformants
Two PCR-confirmed msr(D)-transformant N. subflava strains (ITM_Ns_45/1_P1 and ITM_Ns_9/1_P1; Table 2) were subjected to WGS to identify the exact integration site of msr(D). DNA sequences including the upstream (6,725 bp) and downstream (9,134 bp) region of msr(D) with a maximum total length of 17,803 bp were extracted for further analyses. Donor (ITM_Ns_3/2, ITM_Ns_27/1 and ITM_Ns_36/1), recipient (ITM_Ns_45/1 and ITM_Ns_9/1) and transformant (ITM_Ns_9/1_P1 and ITM_Ns_45/1_P1) DNA sequences were aligned. This alignment revealed the acquisition of the msr(D) gene at the same site (GCATA-acquisition of msr(D)-ATTGA) in the chromosome in both recipients, 32 bp downstream of a DUS sequence (Figure 2). Genome comparison of donor, recipient and transformant revealed that the transformants had acquired a new msr(D)-containing DNA fragment, which originated from the donor, and was not present in the recipient (Figure 3).
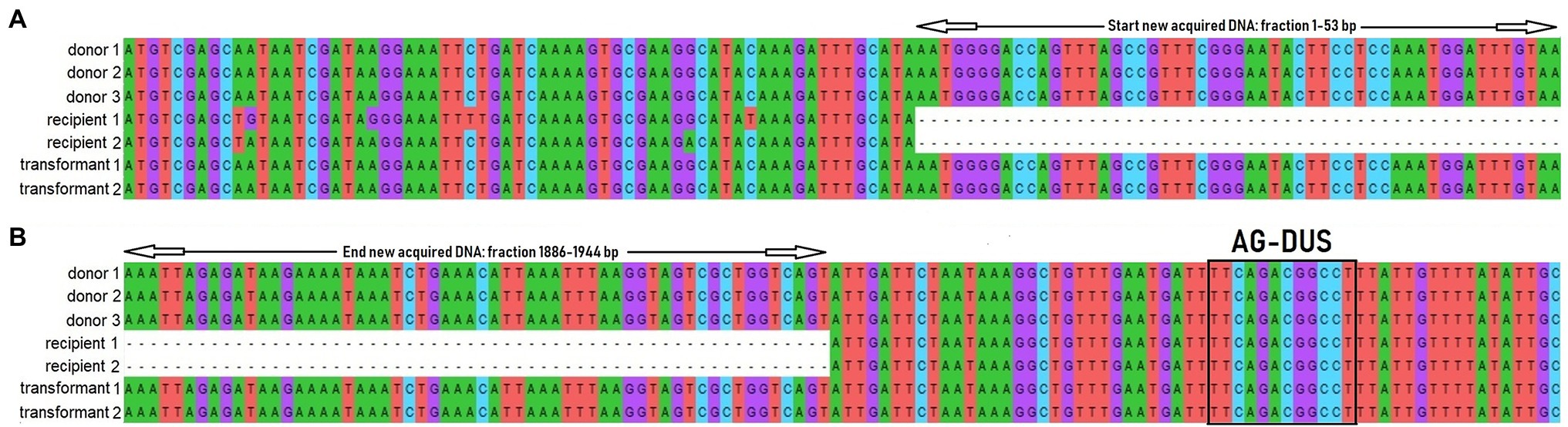
Figure 2. Fragment of DNA sequence alignment of the start (A) and end (B) point (black triangle) of the integration of the new acquired DNA fragment containing msr(D). AG-DUS 31 bp upstream msr(D) is indicated with a black box. Transformation of recipient strains (recipient 1; ITM_Ns_9/1, recipient 2; ITM_Ns_9/1) with donor DNA containing msr(D) (donor 1; ITM_Ns_3/2, donor 2; ITM_Ns_27/1 and donor 3; ITM_Ns_36/1) resulted in transformant 1 (ITM_Ns_9/1_P1) and 2 (ITM_Ns_45/1_P1).
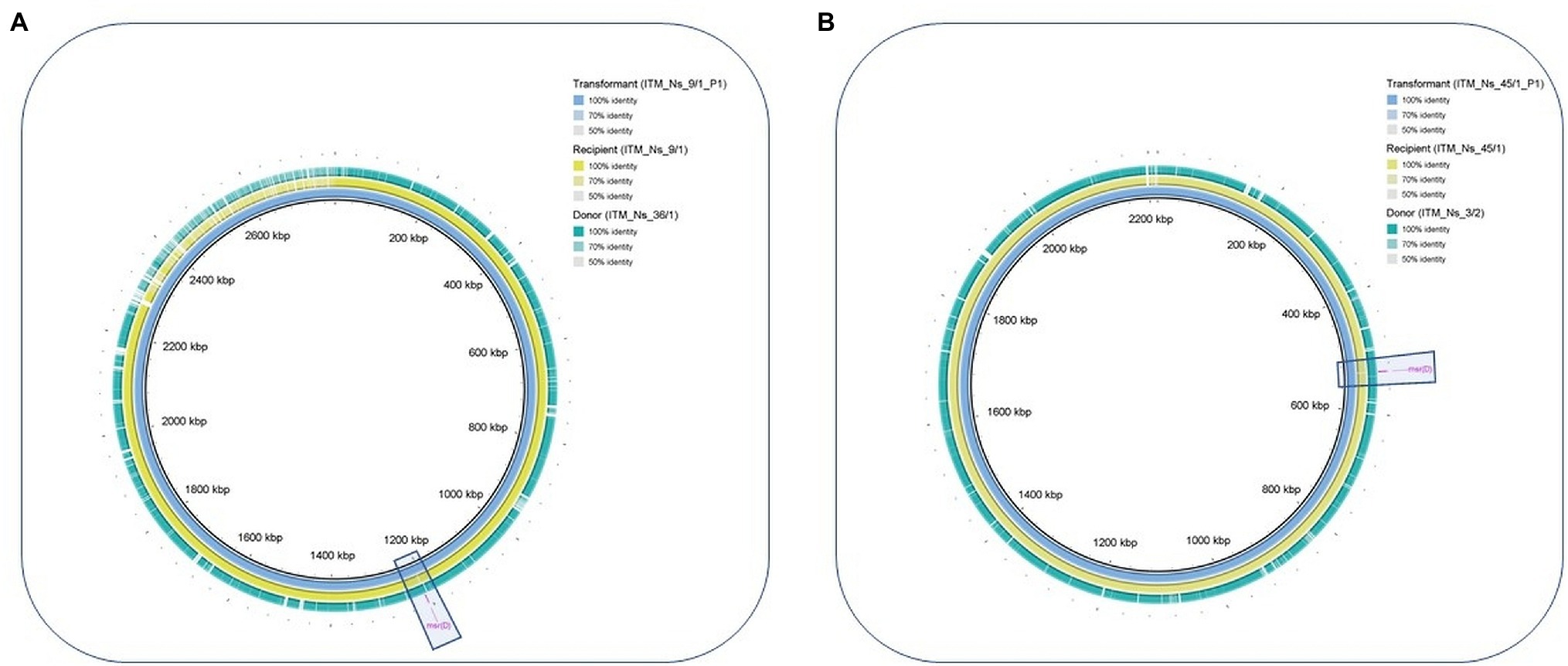
Figure 3. Genome visualisation of msr(D) transformation between two strains of N. subflava in Brig (A; transformant ITM_Ns_9/1_p1 used as reference with recipient ITM_Ns_9/1 and donor ITM_Ns_36/1, B; transformant ITM_Ns_45/1_P1 used as reference with recipient ITM_Ns_45/1 and donor ITM_Ns_3/2). The inner-ring (blue) depicts the transformant genome, where msr(D) (manually labelled in fuchsia) is integrated in the recipient (yellow circle) from donor (green circle).
A more global alignment conducted in Mauve illustrates the chromosomal organisation around the acquired msr(D) in the transformant ITM_Ns_45/1_P1 compared to the recipient strain (Figure 4). SNP analysis revealed that transformant ITM_Ns_9/1_P1 had taken up a larger DNA fragment than transformant ITM_Ns_45/1_P1 ((Figure 2; Table 4; Supplementary Table 1). The length between the first and last SNP of transformants compared to the recipient strains was 12,033 bp and 5,113 bp for transformants ITM_Ns_9/1_P1 and ITM_Ns_45/1_P1, respectively. The acquired DNA extended from upstream of msr(D) (7,234 bp in ITM_Ns_9/1_P1; 2,883 bp in ITM_Ns_45/1_P1) to downstream of msr(D) (3,335 bp in ITM_Ns_9/1_P1, 766 bp in ITM_Ns_45/1_P1; Supplementary Table 1).
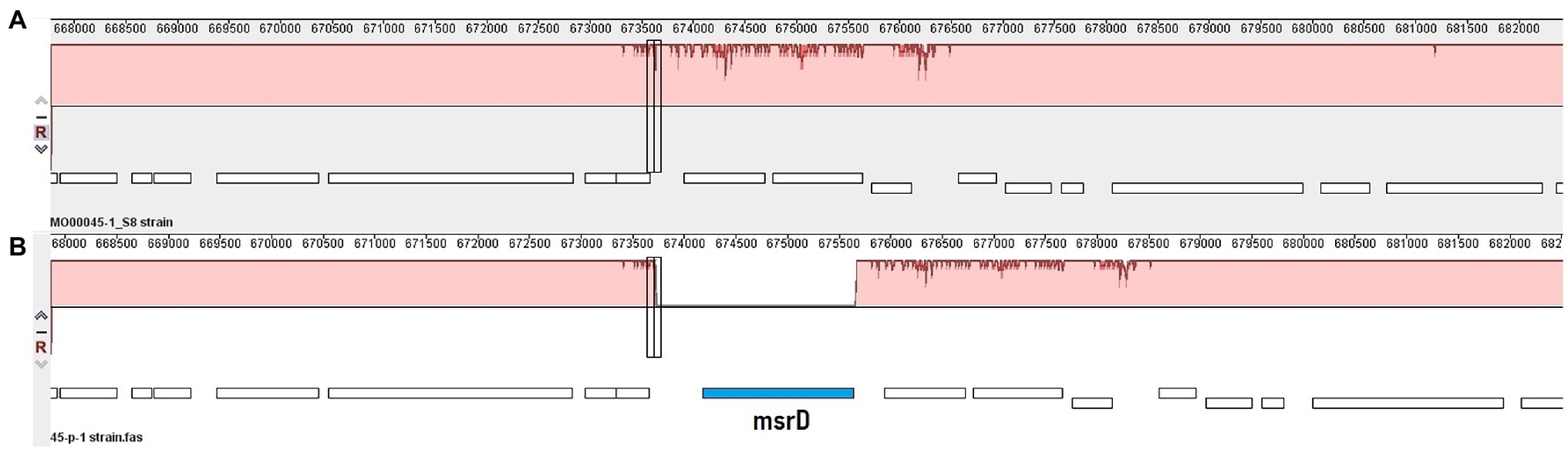
Figure 4. Mauve alignment of recipient (A; ITM_Ns_45/1) with transformant (B; ITM_Ns_45/1_P1) strain. Red color indicates similar DNA sequence in both strains, the blue box indicates the msr(D) gene and the vertical bar indicates the integration site in the recipient strain where the DNA fragment containing msr(D) was inserted. The rough lane in the red box indicates differences in DNA sequence between the two isolates and thus depicts the complete fragment size in the transformant strain (ITM_Ns_45/1_P1) which originated from the donor strain.

Table 4. Characteristics of integrated DNA fraction in transformant ITM_Ns_9/1_P1 and ITM_Ns_45/1_P1.
The uptake-fragment of transformant ITM_Ns_9/1_P1 showed high similarity to ITM_Ns_36/1 (97.57% identical to ITM_Ns_3/2, 92.78% to ITM_Ns_27/1 and 99.96% to ITM_Ns_36/1) and the uptake-fragment of transformant ITM_Ns_45/1_P1 showed high similarity to ITM_Ns_3/2 (99.92% identical to ITM_Ns_3/2, 92.41% to donor ITM_Ns_27/1 and 98.43% to donor ITM_Ns_36/1; Table 4). These data suggest that for both transformants, the complete msr(D) containing fragment was taken up from a single (but different) donor in a single transformation event (ITM_Ns_3/2 as the donor for ITM_Ns_45/1 and ITM_Ns_36/1 for ITM_Ns_9/1_P1).
Discussion
We studied the intra- and inter-species transformability of the resistance conferring msr(D) gene in Neisseria spp. We found that intraspecies transformation in commensals under azithromycin pressure in N. subflava was very efficient. Azithromycin triggered the integration of msr(D) into strains of N. subflava with low level azithromycin resistance (3–6 mg/l). The msr(D) gene could be acquired without any apparent fitness cost and was universally associated with an elevation of azithromycin MICs to >256 mg/L. We did not conclusively establish that msr(D) is responsible for macrolide resistance in N. subflava. This was, however, not one of the study aims as this has been clearly established for a range of gram negative and positive bacterial species (Daly et al., 2004; Nunez-Samudio and Chesneau, 2013; Zhang et al., 2016; Iannelli et al., 2018; Fostier et al., 2020).
In a previous study, we found that nine out of 11 clinical N. subflava strains had the msr(D) gene integrated in the same place in the genome (de Block et al., 2021). The complete integrated DNA sequence originates from the MEGA element in S. pneumoniae. The integration in N. subflava was located 32 bp downstream of a DUS sequence, suggesting that this DUS enhances the transformation efficiency. WGS of transformant N. subflava strains in the current study revealed that the chromosomal integration of the msr(D) gene was integrated into the same position in the genome as the donor strains. The complete fragment size in the recipients included up- and downstream regions of msr(D) with a total length of 5 and 12 kb, respectively. Thus, a DNA insert up to 12 kb can be transformed into the cell and integrated into the chromosome in a single event. Other studies have found similar sized transformation events in Neisseria spp. (Chen et al., 2020). A previous core genome MLST analysis revealed that the msr(D) gene was present in different clusters of clinical isolates of N. subflava (de Block et al., 2021). This implies that horizontal gene transfers such as transformation either took place on more than one occasion, or that the msr(D) has been taken up and lost in sub-lineages. This suggests that single transformation events of msr(D) could also take place in vivo.
The MEGA element in S. pneumoniae contains both the msr(D) gene (which is responsible for displacing bound macrolides) and mef(A) which codes an efflux pump that pumps the displaced macrolide out of the cell. Together, these genes belong to the two-gene efflux transport system of the ATP-Binding Cassette (ABC) superfamily and are responsible for type M resistance to macrolides (Iannelli et al., 2018). In N. subflava, the mef(A) is truncated and likely non-functional (de Block et al., 2021). This suggests that another efflux pump may be used by N. subflava to expel the dissociated macrolide. This function is may be carried out by the mtrCDE efflux pump. Interestingly, the N. subflava’s used in this study all contained the K823E mtrD mutant which is known to enhance the ability of the mtrCDE pump to export macrolides (Lyu et al., 2020).
Although it has been proven that interspecies recombination is successful between commensals and pathogenic Neisseria in vitro, we were unable to transform msr(D) into N. gonorrhoeae (Chen et al., 2020). There are a number of possible explanations for this finding. Firstly, the chromosomal organisation around msr(D) is very similar in the N. subflava donor and recipient strains but is divergent to N. gonorrhoeae strains (Supplementary Figures 3, 4). We have previously established that the core genome of the strains used in this study varies considerably between N. subflava and N. gonorrhoeae (De Block et al., 2021). This could affect efficient chromosomal integration of msr(D) and explain why interspecies transformation between N. subflava and N. gonorrhoeae was not successful (Qvarnstrom and Swedberg, 2006). Secondly, the relative frequency of the 12-bp DUS sequences varies considerably between N. subflava and N. gonorrhoeae. The 5’-ATGCCGTCTGAA-3’ DUS is more prevalent in N. gonorrhoeae, whereas the 5’-AGGCCGTCTGAA-3’ DUS is more prevalent in N. subflava (Supplementary Figure 4; Berry et al., 2013). These differences in the relative frequency of DUS-subtypes have been shown to influence the probability of transformation (Duffin and Seifert, 2010). This provided the rational for using dsDNA fragments containing msr(D) combined with the predominant N. gonorrhoeae DUS for the transformation experiments. However, this approach did not result in transformation. Thirdly, the differential DNA methylation pattern between species of Neisseria may result in the uptake of msr(D) containing DNA from N. subflava being toxic to N. gonorrhoeae but not N. subflava (Kim et al., 2020). Finally, the failure to transform msr(D) into N. gonorrhoeae may be due to limitations in our experimental approach. Although we used three different experimental approaches to transform msr(D) into N. gonorrhoeae, we did so in a limited number of strains. Furthermore, while we have previously been able to conduct successful transformation experiments with two of these strains of N. gonorrhoeae using the same experimental protocol, we did not include positive controls in the current experiments (Abdellati et al., 2019). These limitations mean that we cannot conclude that the msr(D) gene could not be transformed into N. gonorrhoeae. A further limitation of our study is the crude methods we used to measure the fitness cost associated with the acquisition of the msr(D) gene.
Another transformation pathway to evaluate in a future study is the transformability between different commensal strains. It may be possible to transform msr(D) from N. subflava to another commensal Neisseria species, such as N. lactamica, which is then able to transform the msr(D) in N. gonorrhoeae or N. meningitidis. For example, N. lactamica is known to be an efficient AMR donor to N. meningitidis (Chen et al., 2020).
Our study showed that intraspecies transformation of msr(D) under azithromycin pressure is very efficient within N. subflava. We were unable to transform msr(D) into N. gonorrhoeae. The limitations noted above mean that we cannot exclude the possibility of this occurring in the future.
Data Availability Statement
The datasets presented in this study can be found in online repositories. The names of the repository/repositories and accession number(s) can be found at: https://www.ncbi.nlm.nih.gov/, PRJNA794044.
Author Contributions
SA conducted the wet laboratory experiments. TB and NG conducted the bioinformatic analysis and wrote the first draft. CK, SA and SM-B conceptualized the study. SA, CD, JL, SM-B, IB, DB and CK reviewed and edited the manuscript. All authors contributed to the article and approved the submitted version.
Funding
The study was funded by SOFI 2021 grant–‘‘PReventing the Emergence of untreatable STIs via radical Prevention, (PRESTIP).
Conflict of Interest
The authors declare that the research was conducted in the absence of any commercial or financial relationships that could be construed as a potential conflict of interest.
Publisher’s Note
All claims expressed in this article are solely those of the authors and do not necessarily represent those of their affiliated organizations, or those of the publisher, the editors and the reviewers. Any product that may be evaluated in this article, or claim that may be made by its manufacturer, is not guaranteed or endorsed by the publisher.
Supplementary Material
The Supplementary Material for this article can be found online at: https://www.frontiersin.org/articles/10.3389/fmicb.2022.855482/full#supplementary-material
References
Abdellati, S., Verhoeven, E., Irith, D. B., Crucitti, T., and Kenyon, C. (2019). Transfer of High-Level Macrolide Resistance in Neisseria Gonorrhoeae. London: BMJ Publishing Group Ltd. P668.
Alikhan, N. F., Petty, N. K., Ben Zakour, N. L., and Beatson, S. A. (2011). BLAST ring image generator (BRIG): simple prokaryote genome comparisons. BMC Genomics 12:402. doi: 10.1186/1471-2164-12-402
Berry, J. L., Cehovin, A., McDowell, M. A., Lea, S. M., and Pelicic, V. (2013). Functional analysis of the interdependence between DNA uptake sequence and its cognate ComP receptor during natural transformation in Neisseria species. PLoS Genet. 9:1004014. doi: 10.1371/journal.pgen.1004014
Bowler, L. D., Zhang, Q.-Y., Riou, J.-Y., and Sprattf, B. G. (1994). Interspecies recombination between the penA genes of Neisseria meningitidis and commensal Neisseria species during the emergence of penicillin resistance in N. meningitidis: natural events and laboratory simulation. Journal of Bacteriology 176, 333–337. doi: 10.1128/jb.176.2.333-337.1994
Chen, M., Zhang, C., Zhang, X., and Chen, M. (2020). Meningococcal quinolone resistance originated from several commensal Neisseria species. Antimicrob Agents Chemother 64, e01494–19. doi: 10.1128/AAC.01494-19
Daly, M. M., Doktor, S., Flamm, R., and Shortridge, D. (2004). Characterization and prevalence of MefA, MefE, and the associated msr (D) Gene in Streptococcus pneumoniae Clinical Isolates. J. Clin. Microbiol. 42, 3570–3574. doi: 10.1128/JCM.42.8.3570
Darling, A. C. E., Mau, B., Blattner, F. R., and Perna, N. T. (2004). Mauve: multiple alignment of conserved genomic sequence with rearrangements. Genome Res. 14, 1394–1403. doi: 10.1101/gr.2289704.tion
de Block, T., Laumen, J. G. E., Van Dijck, C., Abdellati, S., Baetselier, I. De, Manoharan-Basil, S. S., et al. (2021). Wgs of commensal Neisseria reveals acquisition of a new ribosomal protection protein (Msrd) as a possible explanation for high level azithromycin resistance in Belgium. Pathogens 10:384. doi: 10.3390/pathogens10030384
Dinos, G. P. (2017). The macrolide antibiotic renaissance. Br. J. Pharmacol. 174, 2967–2983. doi: 10.1111/bph.13936
Dong, H. V., Pham, L. Q., Nguyen, H. T., Nguyen, M. X. B., Nguyen, T. V., May, F., et al. (2020). Decreased cephalosporin susceptibility of Oropharyngeal Neisseria species in antibiotic-using men who have sex with men in Hanoi, Vietnam. Clin. Infect. Dis. 70, 1169–1175. doi: 10.1093/cid/ciz365
Duffin, P. M., and Seifert, H. S. (2010). DNA uptake sequence-mediated enhancement of transformation in Neisseria gonorrhoeae is strain dependent. J. Bacteriol. 192, 4436–4444. doi: 10.1128/JB.00442-10
Fostier, C. R., Singh, S., and Hunt, J. F. (2020). ABC-F translation factors: from antibiotic resistance to immune response. 1–32. doi: 10.1002/1873-3468.13984
Fox, V., Santoro, F., Pozzi, G., and Iannelli, F. (2021). Predicted transmembrane proteins with homology to Mef (A) are not responsible for complementing mef (A) deletion in the mef (A)–msr (D) macrolide efflux system in Streptococcus pneumoniae. BMC. Res. Notes 14, 1–7. doi: 10.1186/s13104-021-05856-6
Gurevich, A., Saveliev, V., Vyahhi, N., and Tesler, G. (2013). QUAST: quality assessment tool for genome assemblies. Bioinformatics 29, 1072–1075. doi: 10.1093/bioinformatics/btt086
Hamilton, H. L., and Dillard, J. P. (2006). Natural transformation of Neisseria gonorrhoeae: from DNA donation to homologous recombination. Mol. Microbiol. 59, 376–385. doi: 10.1111/j.1365-2958.2005.04964.x
Iannelli, F., Santoro, F., Santagati, M., Docquier, J. D., Lazzeri, E., Pastore, G., et al. (2018). Type M resistance to macrolides is due to a two-gene efflux transport system of the ATP-binding cassette (ABC) superfamily. Front. Microbiol. 9, 1–9. doi: 10.3389/fmicb.2018.01670
Ito, M., Deguchi, T., Mizutani, K.-S., Yasuda, M., Yokoi, S., Ito, S.-I., et al. (2005). Emergence and spread of Neisseria gonorrhoeae clinical isolates harboring mosaic-Like structure of penicillin-binding protein 2 in Central Japan. Antimicrob. Agents Chemother. 49, 137–143. doi: 10.1128/AAC.49.1.137-143.2005
Kenyon, C., Laumen, J., and Manoharan-Basil, S. (2021). Choosing new therapies for gonorrhoea: we need to consider the impact on the pan-neisseria genome. A viewpoint. Antibiotics 10:515. doi: 10.3390/antibiotics10050515
Kim, W. J., Higashi, D., Goytia, M., Rendón, M. A., Pilligua-, M., Bronnimann, M., et al. (2020). Commensal Neisseria kill Neisseria gonorrhoeae through a DNA-dependent mechanism in mice. Cell Host Microbe 26, 228–239. doi: 10.1016/j.chom.2019.07.003.Commensal
Kumar, S., Stecher, G., Li, M., Knyaz, C., and Tamura, K. (2018). MEGA X: molecular evolutionary genetics analysis across computing platforms. Mol. Biol. Evol. 35, 1547–1549. doi: 10.1093/molbev/msy096
Laumen, J. G. E., Manoharan-Basil, S. S., Verhoeven, E., Abdellati, S., De Baetselier, I., Crucitti, T., et al. (2021a). Molecular pathways to high-level azithromycin resistance in Neisseria gonorrhoeae. J. Antimicrob. Chemother. 76, 1752–1758. doi: 10.1093/jac/dkab084
Laumen, J. G. E., Van Dijck, C., Abdellati, S., Manoharan-Basil, S. S., De Baetselier, I., Martiny, D., et al. (2021b). Markedly reduced azithromycin and ceftriaxone susceptibility in commensal neisseria species in clinical Samples from Belgian men who have sex with men. Clin. Infect. Dis. 72, 363–364. doi: 10.1093/cid/ciaa565
Liu, G., Tang, C. M., and Exley, R. M. (2015). Non-pathogenic Neisseria: members of an abundant, multi-habitat, diverse genus. Microbiology 161, 1297–1312. doi: 10.1099/mic.0.000086
Lyu, M., Moseng, M. A., Reimche, J. L., Holley, C. L., Dhulipala, V., Su, C. C., et al. (2020). Cryo-EM structures of a gonococcal multidrug efflux pump illuminate a mechanism of drug recognition and resistance. MBio 11, e00996–e00920. doi: 10.1128/mBio.00996-20
Ma, K. C., Mortimer, T. D., Duckett, M. A., Hicks, A. L., Wheeler, N. E., Sánchez-Busó, L., et al. (2020). Increased power from conditional bacterial genome-wide association identifies macrolide resistance mutations in Neisseria gonorrhoeae. Nat. Commun. 11, 5374. doi: 10.1038/s41467-020-19250-6
Manoharan-Basil, S. S., Laumen, J. G. E., Van Dijck, C., De Block, T., De Baetselier, I., and Kenyon, C. (2021). Evidence of horizontal gene transfer of 50S ribosomal genes rplB, rplD, and rplY in Neisseria gonorrhoeae. Front. Microbiol. 12, 1–17. doi: 10.3389/fmicb.2021.683901
Nakayama, S. I., Shimuta, K., Furubayashi, K. I., Kawahata, T., Unemo, M., and Ohnishi, M. (2016). New ceftriaxone- and multidrug-resistant Neisseria gonorrhoeae strain with a novel mosaic penA gene isolated in Japan. Antimicrob. Agents Chemother. 60, 4339–4341. doi: 10.1128/AAC.00504-16
Nunez-Samudio, V., and Chesneau, O. (2013). Functional interplay between the ATP binding cassette Msr(D) protein and the membrane facilitator superfamily Mef(E) transporter for macrolide resistance in Escherichia coli. Res. Microbiol. 164, 226–235. doi: 10.1016/j.resmic.2012.12.003
O'Regan, E., Quinn, T., Frye, J. G., Pagès, J. M., Porwollik, S., Fedorka-Cray, P. J., et al. (2010). Fitness costs and stability of a high-level ciprofloxacin resistance phenotype in Salmonella enterica serotype enteritidis: reduced infectivity associated with decreased expression of Salmonella pathogenicity island 1 genes. Antimicrobial agents and chemotherapy 54, 367–374. doi: 10.1128/AAC.00801-09
Prjibelski, A., Antipov, D., Meleshko, D., Lapidus, A., and Korobeynikov, A. (2020). Using SPAdes de novo assembler. Curr. Protoc. Bioinform. 70:e102. doi: 10.1002/cpbi.102
Qvarnstrom, Y., and Swedberg, G. (2006). Variations in gene organization and DNA uptake signal sequence in the folP region between commensal and pathogenic Neisseria species. BMC microbiology 6:11. doi: 10.1186/1471-2180-6-11
R Core Team (2019). R: A Language and Environment for Statistical Computing. R Foundation for Statistical Computing, Vienna, Austria.
Roberts, M. C., Chung, W. O., Roe, D., Xia, M., Marquez, C., Borthagaray, G., et al. (1999). Erythromycin-resistant Neisseria gonorrhoeae and oral commensal Neisseria spp. carry known rRNA methylase genes. Antimicrob. Agents Chemother. 43, 1367–1372. doi: 10.1128/aac.43.6.1367
Rotman, E., and Seifert, H. S. (2014). The genetics of Neisseria species. Annu. Rev. Genet. 48, 405–431. doi: 10.1146/annurev-genet-120213-092007
Seemann, T. (2014). Prokka: rapid prokaryotic genome annotation. Bioinformatics 30, 2068–2069. doi: 10.1093/bioinformatics/btu153
Sharkey, L. K. R., Edwards, T. A., and O’Neill, A. J. (2016). ABC-F proteins mediate antibiotic resistance through ribosomal protection. MBio 7, 1–10. doi: 10.1128/mBio.01975-15
Sprouffske, K., and Wagner, A. (2016). Growthcurver: an R package for obtaining interpretable metrics from microbial growth curves. BMC Bioinform. 17, 17–20. doi: 10.1186/s12859-016-1016-7
Tedijanto, C., Olesen, S. W., Grad, Y. H., and Lipsitch, M. (2018). Estimating the proportion of bystander selection for antibiotic resistance among potentially pathogenic bacterial flora. Proc. Natl. Acad. Sci. U. S. A. 115, E11988–E11995. doi: 10.1073/pnas.1810840115
Unemo, M., and Shafer, W. M. (2014). Antimicrobial resistance in Neisseria gonorrhoeae in the 21st century: past, evolution, and future. Clin. Microbiol. Rev. 27, 587–613. doi: 10.1128/CMR.00010-14
Verhoeven, E., Abdellati, S., Nys, P., Laumen, J., De Baetselier, I., Crucitti, T., et al. (2019). Construction and optimization of a 'NG Morbidostat' - An automated continuous-culture device for studying the pathways towards antibiotic resistance in Neisseria gonorrhoeae. F1000Research 8:1343. doi: 10.12688/f1000research.18861.2
Wadsworth, C. B., Arnold, B. J., Sater, M. R. A., and Grad, Y. H. (2018). Azithromycin resistance through interspecific acquisition of an epistasis-dependent efflux pump component and transcriptional regulator in Neisseria gonorrhoeae. mBio 9, e01419–e01418. doi: 10.1128/mBio.01419-18
Keywords: horizontal gene transfer, msr(D), transformation, Neisseria subflava, Neisseria gonorrhoeae, macrolide resistance
Citation: de Block T, González N, Abdellati S, Laumen JGE, Van Dijck C, De Baetselier I, Van den Bossche D, Manoharan-Basil SS and Kenyon C (2022) Successful Intra- but Not Inter-species Recombination of msr(D) in Neisseria subflava. Front. Microbiol. 13:855482. doi: 10.3389/fmicb.2022.855482
Edited by:
Alberto Antonelli, University of Florence, ItalyReviewed by:
Gianluca Morroni, Marche Polytechnic University, ItalyWilliam Shafer, Emory University, United States
Copyright © 2022 de Block, González, Abdellati, Laumen, Van Dijck, De Baetselier, Van den Bossche, Manoharan-Basil and Kenyon. This is an open-access article distributed under the terms of the Creative Commons Attribution License (CC BY). The use, distribution or reproduction in other forums is permitted, provided the original author(s) and the copyright owner(s) are credited and that the original publication in this journal is cited, in accordance with accepted academic practice. No use, distribution or reproduction is permitted which does not comply with these terms.
*Correspondence: Tessa de Block, dGRlYmxvY2tAaXRnLmJl
†These authors have contributed equally to this work