Corrigendum: A Transcriptomic Study Reveals That Fish Vibriosis Due to the Zoonotic Pathogen Vibrio vulnificus Is an Acute Inflammatory Disease in Which Erythrocytes May Play an Important Role
- 1Instituto Universitario de Biotecnología y Biomedicina (BIOTECMED), Universitat de València, Valencia, Spain
- 2Centro de Biotecnología Acuícola, Facultad de Química y Biología, Universidad de Santiago de Chile, Santiago, Chile
- 3Department of Cell Biology, Physiology, and Immunology, Universitat Autònoma de Barcelona, Bellaterra, Spain
- 4Facultad de Medicina Veterinaria y Agronomía, Universidad de Las Américas, Santiago, Chile
Vibrio vulnificus is a marine zoonotic pathogen associated with fish farms that is considered a biomarker of climate change. Zoonotic strains trigger a rapid death of their susceptible hosts (fish or humans) by septicemia that has been linked to a cytokine storm in mice. Therefore, we hypothesize that V. vulnificus also causes fish death by triggering a cytokine storm in which red blood cells (RBCs), as nucleated cells in fish, could play an active role. To do it, we used the eel immersion infection model and then analyzed the transcriptome in RBCs, white BCs, and whole blood using an eel-specific microarray platform. Our results demonstrate that V. vulnificus triggers an acute but atypical inflammatory response that occurs in two main phases. The early phase (3 h post-infection [hpi]) is characterized by the upregulation of several genes for proinflammatory cytokines related to the mucosal immune response (il17a/f1 and il20) along with genes for antiviral cytokines (il12β) and antiviral factors (ifna and ifnc). In contrast, the late phase (12 hpi) is based on the upregulation of genes for typical inflammatory cytokines (il1β), endothelial destruction (mmp9 and hyal2), and, interestingly, genes related to an RNA-based immune response (sidt1). Functional assays revealed significant proteolytic and hemolytic activity in serum at 12 hpi that would explain the hemorrhages characteristic of this septicemia in fish. As expected, we found evidence that RBCs are transcriptionally active and contribute to this atypical immune response, especially in the short term. Based on a selected set of marker genes, we propose here an in vivo RT-qPCR assay that allows detection of early sepsis caused by V. vulnificus. Finally, we develop a model of sepsis that could serve as a basis for understanding sepsis caused by V. vulnificus not only in fish but also in humans.
Introduction
Fish vibriosis encompasses a group of diseases with common clinical signs caused by different genus Vibrio species (Amaro et al., 2020). Among these species, Vibrio vulnificus stands out as the only one linked to zoonotic cases acquired through contact with diseased fish, mainly farmed fish (Amaro et al., 2015; Oliver, 2015). Moreover, it is the only one that can cause rapid death by septicemia in both humans and fish (Ceccarelli et al., 2019; Amaro et al., 2020).
The severity of outbreaks caused by V. vulnificus in fish farms is highly dependent on water temperature since the highest mortality rates occur at temperatures above 25°C (Amaro et al., 1995). This temperature dependence explains why this vibriosis (hereafter Vv-vibriosis) mainly affects fish reared above 22°C, as well as why clinical cases in humans and animals are increasing with global warming (Ceccarelli et al., 2019; Amaro et al., 2020). Part of the reason for this dependence is that an increase in temperature above 22°C significantly increases the transcription of several pathogen genes related to colonization and resistance to innate immunity in fish, thus favoring disease transmission and unbalancing the host-pathogen relationship toward the pathogen (Hernández-Cabanyero et al., 2020). These data correlate with field data and underline the relevance of V. vulnificus as a biological barometer of climate change (Baker-Austin et al., 2012, 2018).
Vv-vibriosis differs from other vibriosis in that death from septicemia occurs very quickly and without the pathogen reaching as high numbers in the blood or tissues as it does in other vibriosis (Valiente and Amaro, 2006; Valiente et al., 2008a). Previous studies in eels infected by immersion showed that the pathogen infects animals through water, colonize the gill and intestinal epithelium and cause local inflammation that favors its entry into the blood (Marco-Noales et al., 2001; Callol et al., 2015a). Furthermore, a series of additional studies demonstrated that once in the bloodstream, the pathogen produces a series of iron-regulated proteins that allow it to resist innate immunity and survive (Pajuelo et al., 2016; Hernández-Cabanyero et al., 2019). However, although toxins and exoenzymes that could cause cell death and/or tissue injury are known (Jeong and Satchell, 2012; Lee et al., 2013), is less clear the mechanism by which such a small number of bacteria triggers rapid death by sepsis. Murciano et al. (2017) shed light on how this bacterium could cause rapid death by sepsis. The authors infected mice by intraperitoneal injection with a zoonotic strain. They showed that the animal’s death was related to an early cytokine storm triggered by the pathogen. However, although the mouse is the animal model used to study human vibriosis, it is neither a natural host for V. vulnificus nor is injection a natural route of infection. Therefore, it would have to be shown that the pathogen triggers a cytokine storm by using one of its natural animal hosts infected by the natural route.
Given the above, the main objective of this study was to demonstrate that V. vulnificus causes an early cytokine storm in fish. To do so, we infected eels by immersion (Amaro et al., 1995) with the same strain used by Murciano et al. (2017). We analyzed the transcriptome in blood using an eel-specific microarray platform containing probes for thousands of immune-related genes (Callol et al., 2015b). Since fish red blood cells (RBCs) are nucleated cells involved in defense against viruses (Workenhe et al., 2008; Morera et al., 2011; Dahle et al., 2015; Nombela et al., 2017; Nombela and Ortega-Villaizan, 2018), we also considered analyzing the RBCs-associated transcriptome. Accordingly, we studied and compared the transcriptome associated with RBCs, white BCs (WBCs), and whole blood (B) at 0-, 3- and 12-h post-infection (hpi). We then validated the results obtained by RT-qPCR and performed a series of functional confirmatory assays. Our results suggest that V. vulnificus triggers an acute but atypical inflammatory response in two main phases. The early phase (detectable at 3 hpi) is characterized by the upregulation of important genes for proinflammatory cytokines related to the mucosal immune response (il17a/f1 [in RBCs] and il20 [in RBCs and WBCs]) along with antiviral cytokine genes (il12β [in both cell types]) and antiviral factors (ifna and ifnc [in WBCs only]). The late phase (detectable at 12 hpi) is characterized by the upregulation of genes for typical inflammatory cytokines (il1β), endothelial destruction (mmp9 and hyal2), and genes related to an RNA-based immune response (sidt1), all of them detected in B samples. Functional assays revealed significant proteolytic and hemolytic activity in serum at 12 hpi that would explain the hemorrhages characteristic of this septicemia. As expected, we found evidence that RBCs are transcriptionally active and may contribute to this atypical immune response, especially in the short term. We also selected a series of marker genes and validated in vivo an RT-qPCR assay for early detection of Vv-vibriosis. Finally, we developed a model of septicemia that could serve as a basis for understanding sepsis caused by V. vulnificus not only in fish but also in humans.
Materials and Methods
Animal Maintenance
Adult European eels (Anguilla anguilla) of around 20 g (50% lethal dose [LD50] determination) or 100 g (sample collection for transcriptomic experiments) of body weight were purchased from a local eel farm (Valenciana de Acuicultura SA, Spain) that does not vaccinate against V. vulnificus. Eel maintenance and all the experiments described above were performed at 28°C in 180-liter tanks containing either 60 (infection experiments) or 120 L (the rest of experiments and animal maintenance) of saline water (SW, 1.5% NaCl, pH 7) with a system of aeration and filtration in the facilities of the Central Service for Experimental Research (SCSIE) of the University of Valencia (Spain).
Bacterial Strain, Growth Media, and Conditions
The V. vulnificus strain CECT 4999 (Spanish Type Culture Collection; hereafter R99), which was isolated from a diseased eel in Spain (Lee et al., 2008), was used in this study. It was routinely grown in Tryptone Soy Agar or Luria-Bertani broth, both supplemented with 1% NaCl (TSA-1 and LB-1, respectively) with gentle agitation (100 rpm), at 28°C for 18 h to reach a concentration of 109 colony forming units (CFU)/ml for bath infection. Bacterial concentration was checked before and after bath infection by drop-plate counting in TSA-1 plates (Hoben and Somasegaran, 1982). The bacterial strain was stored in LB-1 plus glycerol (20%) at −80°C.
In vivo Bacterial Challenge, Sample Collection, and Preparation
Before the transcriptomic experiments, the LD50 of R99 to the eel stock was determined by immersion challenge, according to Amaro et al. (1995). Briefly, groups of 6 eels of 20 g were immersed in tanks containing either seawater (SW; control group) or serial decimal concentrations of R99 strain in SW (from 1 × 108 to 1 × 105 CFU/ml) for 1 h. Eels were then transferred to new tanks containing fresh SW and monitored for 1 week. Moribund animals were microbiologically analyzed to confirm that they were infected with V. vulnificus (liver sampling in TSA-1 and TCBS, followed by serological confirmation), and LD50 was calculated according to Reed and Muench (1938). For transcriptomic experiments, eels of 100 g were distributed into two groups, the tested (n = 24 individuals) and the control group (n = 6 individuals). Individuals were then immersed either in an infective bath containing 2 × 106 CFU of the strain R99 (the previously estimated LD50; tested group) or in sterilized SW (control group). After 1 h of immersion, fish were transferred separately into new tanks and kept under constant conditions until sampling. We selected as sampling points, time zero (0 hpi; used as another control for the analysis), 3 hpi [as the early time at which most V. vulnificus virulence factors are expressed in vivo (Lee et al., 2013; Callol et al., 2015b; Murciano et al., 2017; Hernández-Cabanyero et al., 2019)], and 12 hpi [the average time at which eels start to die (Amaro et al., 2015)]. Six live eels were randomly sampled at the selected times. Prior sampling, eels were anesthetized with MS222 (50 mg/l), and around 2.5 ml of blood per individual was extracted from the caudal vein with heparinized syringes. Bled eels were then sacrificed using an overdose of MS222 (150 mg/l). Next, a volume of 0.5 ml of the sampled blood was used for bacterial drop-plate counting on TSA-1 and blood cell counts (RBCs and WBCs), a volume of 1 ml was used as a whole blood sample (B), and the rest was processed to get RBCs and WBCs samples. To this end, blood was centrifuged at 800 × g for 5 min. Serum was removed from cells and stored at −80°C until use (for fuctional assays, see below). The pelleted cells were washed with 1 ml of Phosphate Buffered Saline (PBS, pH 7), centrifuged again at 800 × g for 5 min, and the final pellet was resuspended in the same volume of PBS. Then, a density gradient separation was carried out by mixing the suspension with Ficoll®-Paque Premium (Sigma-Aldrich; vol:vol) and centrifugation at 720 × g for 30 min. RBCs and WBCs layers were collected and washed in PBS. We assured that the samples were not contaminated with other cellular populations by observation under the microscope. Finally, the different samples were treated with 1 ml of NucleoZOL (Macherey-Nagel) and stored at −80°C until use. All the in vivo experiments were performed in triplicate.
RNA Extraction, Microarray Hybridization, and Data Analysis
Total RNA (from eels B, RBCs, and WBCs obtained at 0, 3, and 12 hpi) was extracted with NucleoZOL (Macherey-Nagel) following the manufacturer’s instructions. Possible contaminating DNA was eliminated using TURBO™ DNase (Ambion) and then, RNA was cleaned with RNA Cleanup and Concentration Micro Kit RNA (Thermo Scientific) according to the manufacturer’s instructions. RNA integrity and quality were verified with a 2,100 Bioanalyzer (Agilent), and only high-quality samples (RNA Integrity Number [RIN] ≥ 7.5) were selected and used for hybridization with the microarray.
For hybridization, it was used a custom eel-specific microarray platform that contains 42,403 probes (3 per target) of 60-oligonucleotide in length (accession number GPL16775) corresponding to each one of the ORFs identified in the eel immune-transcriptome determined by Callol et al. (2015b). Since the eel genome was not available at the moment the microarray was designed, the eel immune-transcriptome was annotated by similarity with other genomes, searching sequence homologies against NCBI’s non-redundant protein, and NCBI’s redundant nucleotide database by bestBLAST iterative methodology (Callol et al., 2015b). Therefore, the microarray genes in this work refer to those annotated genes. General procedures to obtain labeled cDNA were performed as previously described by Callol et al. (2015b).
Microarray data were extracted from raw images with Feature Extraction software (Agilent technologies). Quality reports were generated and checked for each array. Extracted raw data were imported and analyzed with Genespring GX 14.5 software (Agilent technologies). The 75% percentile normalization was used to standardize arrays for comparisons. All samples were analyzed at gene level using a relative analysis, comparing each sample against a reference sample (0 hpi sample of each cell type). Supplementary Figure S1 summarizes the experimental design and all the comparisons performed. Statistical analysis available in Genespring software was run. One-way analysis of variance (ANOVA; p < 0.05) followed by Tukey’s pairwise comparisons were performed to describe transcriptomic profile differences along the time for each cell type in response to V. vulnificus infection.
Transcriptomic data are available at Gene Expression Omnibus (GEO) database with accession number GSE196944.
Validation of Microarray Results by RT-qPCR
RT-qPCR was performed in parallel to hybridization to validate the microarray results. Table 1 lists the genes, the conditions in which the samples were taken, and the control sample used in each case to calculate the fold induction. Supplementary Table S1 lists the primers used. cDNA samples were obtained from RNA using Maxima H Minus Reverse Transcriptase (Thermo Scientific). Then, qPCR was performed on cDNA using Power SYBR® green PCR Mastermix on a StepOnePlus™ Real-Time PCR System. The CT values were determined with StepOne Software v2.0 to establish the relative mRNA levels of the tested genes, using eel actin (act) as the gold standard (Paria et al., 2016) and the fold induction (2-ΔΔCt) for each gene was calculated according to Livak and Schmittgen (2001). Statistical analysis was performed using GraphPad Prism 7. Data were analyzed by ANOVA analysis for each gene to determine differences between groups (p < 0.05).
Functional Assays
Proteolytic and Hemolytic Activity
Serum samples from infected and control animals were serially diluted in PBS (dilutions from 1:2 to 1:64 were performed). The enzymatic activity of the serum was evaluated by plating 5 μl of the serum samples, and dilutions on 1% agarose plates supplemented with 5% casein (for proteolysis) or with 1% erythrocytes (bovine erythrocytes from Sigma, for hemolysis). 5 μl of PBS and proteinase K (2.5 mg/ml, for proteolysis) or molecular water (for hemolysis) were plated as a negative and positive control for the assay, respectively. Plates were incubated at 28°C for 24 h. The maximal dilution of eel serum with positive activity on agarose-casein or agarose-erythrocytes (transparent halo) was determined and considered the titter of proteolytic and hemolytic activity (Pajuelo et al., 2016). Three independent technical replicates of proteolytic and hemolytic activity were performed for each biological sample of serum.
Bacteriolytic Activity
R99 strain was grown in a layout at LB-1 plates. Then, plates were inoculated with 5 μl of the serum samples and dilutions (performed as specified in the previous section). 5 μl of PBS and lysozyme (103 μg/ml) were plated as a negative and positive control for the assay, respectively. Plates were incubated at 28°C for 24 h, and the maximal dilution of eel serum with positive bacteriolytic activity measured as inhibition halo of bacterial growth was determined. Three independent technical replicates of bacteriolytic activity were performed for each biological sample of serum.
Design and Validation of a New RT-qPCR Assay to the Early Detection of Vv-Vibriosis
A selection of genes (npsn, cox2, mmp9 and sidt1) have been used to develop a new RT-qPCR assay to the early detection of Vv-vibriosis. The list of primers in Supplementary Table S1. Eel infection, blood sampling, sample processing, and RT-qPCR procedure were performed as described on the previous sections. Statistical analysis was performed using GraphPad Prism 7. Data were analyzed by ANOVA analysis followed by the post-hoc multiple comparison by Bonferroni’s method that was run for each gene to determine differences between groups (p < 0.05).
Results
Cell Analysis
First, we monitored the presence of the pathogen in blood and found bacterial counts (0 hpi; <102 CFU/ml; 3 hpi; 7 ± 0.5 × 102 ± CFU/ml; and 12 hpi; 3 ± 0.7 × 104 CFU/ml) and cell numbers (RBCs; 1.5 ± 0.3 × 109 at 0 hpi and 2.5 ± 0.5 × 109 cells/ml at 12 hpi: WBCs; 1.7 ± 0.9 × 107 at 0 hpi and 2.3 ± 1.1 × 107 cells/ml at 12 hpi). The bacterial counts and cell numbers found in eel blood in our experiments were similar to those previously obtained from eels infected by immersion (Callol et al., 2015a; Pajuelo et al., 2015) but lower than those obtained from intraperitoneally-infected eels (Valiente et al., 2008b). We highlight the high number of RBCs in the eels from the stock analyzed, values that were similar to those found by Valiente et al. (2008b), compared to those found in the eels’ stock analyzed by Callol et al. (2015a). These apparently contradictory results are not surprising, given that eels do not reproduce in captivity and that researchers work with wild populations of different origins (Palstra et al., 2005; Mes et al., 2016; Jehannet et al., 2017).
Transcriptomic Analysis
Figure 1 shows the number of differentially expressed genes (DEGs) per sample and sampling time. The early response of WBCs was greater than that of RBCs, both in terms of the number of DEGs and fold change values (Figure 1; Table 2; Supplementary Table S2). Thus, RBCs and WBCs showed about 1,000 and more than 1,700 upregulated genes, respectively. In contrast, the number of upregulated genes decreased significantly at 12 hpi, especially in the case of WBCs (Figure 1). Previous studies with eel RBCs and WBCs showed that erythrocytes, granulocytes, and macrophages could be destroyed by V. vulnificus in vitro (Lee et al., 2013; Hernández-Cabanyero et al., 2019), which would be compatible with a reduction in cell number that was not found in the present study. Instead of this reduction, we found that RBCs, and especially WBCs, were less transcriptionally active at 12 hpi, which is compatible with a loss of functionality caused directly or indirectly by the pathogen. Interestingly, the number of DEGs detected in the B samples was much lower than that found in the RBCs and WBCs samples (Figure 1). This apparent anomaly could be explained by the cellular heterogeneity of the blood, which could negatively affect the normalization of the data and be the cause of high outlier removal.
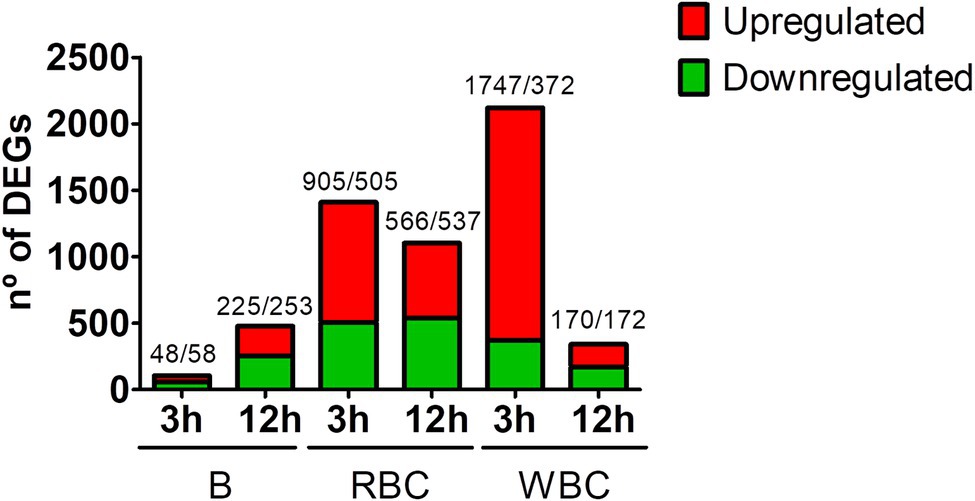
Figure 1. Magnitude of the eel immune response against V. vulnificus represented as the number of differentially expressed genes (DEGs) in blood (B), red blood cells (RBCs) and white blood cells (WBCs) samples. Bars represent total DEGs (sum of upregulated [red] and downregulated [green] DEGs) of each sampling point (3 hpi; 12 hpi) against time zero (0 hpi) of each type of sample. The numbers of up/downregulated DEGs are indicated on the top of each bar.
Overall, the transcriptomic results were reliable thanks to the similar fold change values obtained by microarray hybridization and RT-qPCR for a set of genes (Table 1). Venn diagrams showing common DEGs in RBCs and WBCs throughout infection revealed a cell-specific response, as most transcripts that change their transcription level were not shared between both cell types (Figure 2).
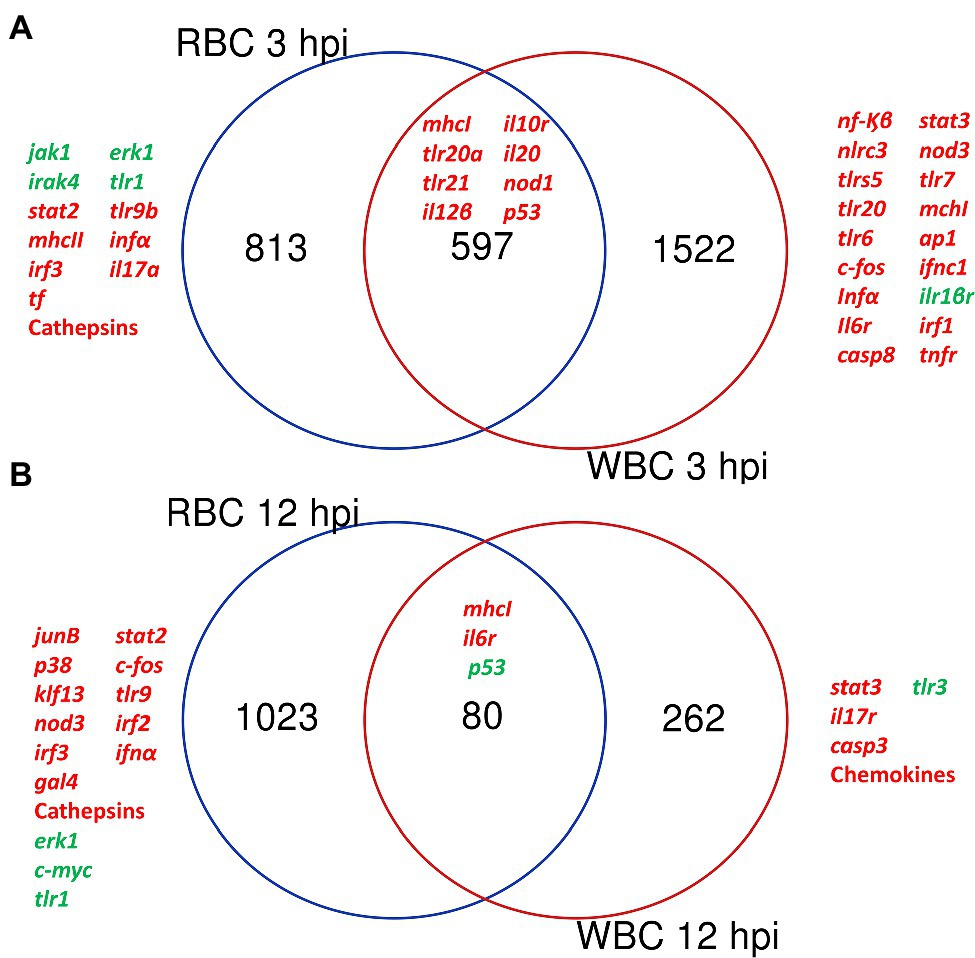
Figure 2. Red blood cells (RBCs) and white blood cells (WBCs) elicit a different immune response against V. vulnificus. Venn diagram depicting the overlap of the differentially expressed genes (DEGs) between RBCs and WBCs at 3 hpi (A) and 12 hpi (B).
The DEGs by the different blood fractions are shown in Supplementary Table S2, and a selection of them by putative function is listed in Table 2. Based on this information, we highlight the following genes and processes that could be related to a harmful defensive response (which could cause self-damage in host tissues and favor its death) of eel against V. vulnificus:
Pathogen Detection and Antigen Presentation Systems
RBCs and WBCs upregulated multiple pattern recognition receptor (Prr) genes as well as major histocompatibility complex (Mhc) genes, the former mainly at 3 hpi and the latter at both 3 and 12 hpi (Table 2; Supplementary Table S2). This result suggested that not only WBCs but also RBCs could act as antigen-presenting cells. Among the Prr genes, we found upregulated tlr genes (Toll-like receptors [Tlrs]), some of which were specifically associated with cell type: i.e., tlr7, tlr6, and tlr5s (encoding the soluble form of Tlr5) with WBCs and tlr9b with RBCs (Figure 2; Table 2). Some of these tlr genes are related to the detection of mainly extracellular antigens (tlr20a, tlr21, tlr6 and tlr5s) and others to the detection of intracellular ones (tlr3, tlr7, and tlr9b). Consistent with this, genes encoding major histocompatibility complex (Mhc) class I (mhcI) and class II (mhcII) were also upregulated, mhcI by RBCs and WBCs, and mhcII only by RBCs. Thus, although V. vulnificus is an extracellular pathogen, our results point to activation of intracellular pathogen recognition and processing mechanisms frequently associated with viral infection (Lund et al., 2004).
In all samples (B, RBCs and WBCs), we also found upregulated genes for cathepsins B, L, and S, a group of lysosomal proteases that play a key role in cellular protein turnover. Cathepsins are associated with Tlr signaling pathways in blood cells to the extent that their inhibition blocks Tlr3-, Tlr7,- and Tlr9-mediated responses (Matsumoto et al., 2008).
Pathogen Control and Destruction
We found evidence of an antibacterial response in blood of infected eels, suggesting that the host immune response tried to eliminate the pathogen after the infection. Our data showed the upregulation of multiple genes related to pathogen growth inhibition (transferrin [RBCs] and hepcidin [WBCs], the hormone that controls iron sequestration, pathogen tagging (i.e., complement factor C3 and lectins), and pathogen destruction (i.e., complement factors C5-C9, Lbp/Bpi protein and genes related to activation of phagocytosis; Table 2; Supplementary Table S2). Complement genes were upregulated by RBCs and WBCs, especially at 3 hpi, although the strongest and most varied response was associated with WBCs at 3 hpi. Similarly, both cell types’ upregulated genes encoding lectins at 3 hpi, especially the galectin and intelectin. Complement/lectin-tagged bacteria can be recognized and phagocytosed more easily by host phagocytes. In accordance, we found several upregulated genes that could be related to phagocytosis and bacterial killing, such as those involved in cytoskeleton rearrangements and nitric oxide synthesis (i.e., inos that was only upregulated by WBCs at 3 hpi), as well as genes related to signal transduction in common with other cellular processes that will be discussed in the following sections (Table 2; Supplementary Table S2).
Regarding antibacterial activity, our results showed the gene encoding Lbp/Bpi, an antibacterial protein produced by different cell types (Inagawa et al., 2002), to be highly upregulated, but only in B samples. Surprisingly, the gene coding for nephrosin (npsn), which has recently been linked to the antibacterial activity of the immune system in fish (Di et al., 2017), was the most strongly upregulated gene in the B samples at both 3 and 12 hpi (Table 2; Supplementary Table S2).
Cell Death
We detected upregulation of multiple genes related to the activation of cell death by apoptosis and/or autophagy, but interestingly only in the WBCs fraction with the sole exception of p53 (RBCs, 3 hpi). Thus, autophagy could be related to the upregulation of autophagy-related proteins (atg9 and atg2a); and apoptosis to p53, caspase-8 (casp8), and genes encoding apoptosis-inducing factors, all upregulated at 3 hpi (Table 2; Supplementary Table S2).
Inflammatory Response
We also found evidence of early regulation of different pathways that trigger a proinflammatory response. At the signal transduction level, this response consisted of upregulation by RBCs and WBCs of nucleotide-binding oligomerization domain containing proteins (nod1 and nod3), as well as the genes for Mapk kinases map2K6, map3K2, and map4K5, whereas mapK6, NF-Κβ (p105 subunit), and signal transducer and activator of transcription 3 (stat3) were only upregulated by WBCs (Table 2; Supplementary Table S2). Indeed, Nod1 and Nod3 activate the Nf-Κβ and Mapk signaling pathways, enhancing the transcription of proinflammatory cytokines (Kim et al., 2016). The rest of the genes mentioned above are part of the Jak/Stat signaling pathway, which is also involved in activating the inflammatory response (Rawlings et al., 2004). Accordingly, we also found upregulated by WBCs at 3 hpi, a gene for an Nlrc3 receptor that acts as a negative regulator of all these processes (Schneider et al., 2013), suggesting an attempt by the immune system to counteract the activation of the inflammatory response against V. vulnificus.
The activated proinflammatory response was also evidenced by the early (3 hpi) upregulation of genes for several tumor necrosis alpha (Tnfα) receptors, interleukins (Ils), and their receptors and interferon (Ifn) and related proteins by RBCs and WBCs with a common (il12β, il10 receptor β, and il20) and cell-type-specific pattern (RBCs: il17a/f1; WBCs: several Tnf receptors, Il1 receptor-like, ifnc1, ifna2, and irf1) followed by a strong upregulation of genes for Il1β, two receptors for Il1β, Il8 precursor, progranulin and granulin in B samples at 12 hpi (Table 2). Granulins are multifunctional proteins produced after proteolytic processing of progranulin (Bateman et al., 1990) that enhance the production of proinflammatory cytokines such as Tnfα and Il8 (Park et al., 2011). Related to these results, we highlight that Il1β is the main proinflammatory cytokine in both humans and fish (Zou and Secombes, 2016) and that Ifna has been related to the immune response against virus (Zou and Secombes, 2016). Multiple genes for Tnfα- and interferon-induced proteins were also detected in all the samples (Table 2; Supplementary Table S2). In parallel, a few genes encoding for anti-inflammatory cytokine receptors (e.g., il10r) were found early upregulated by RBCs and WBCs, which could be interpreted as an attempt by the organism to control the cytokine storm and restore homeostasis.
Since we detected upregulation of the inflammatory gene markers il1β and caspase-3 (casp3) in B samples and not in WBCs samples, we performed RT-qPCR with the same samples and found both to be upregulated in WBCs (il1β at 3 and 12 hpi; caps3 only at 12 hpi; Table 2).
Sepsis Markers
Cells present in B samples upregulated multiple markers of sepsis at 12 hpi. For example, marker genes for disseminated intravascular coagulation, such as those encoding coagulation factor VIII, and genes encoding leukotrienes, prostaglandins, and cyclooxygenase (i.e., cox2), all of which are considered markers of the acute phase of the disease (Peters-Golden et al., 2005; Gómez-Abellán and Sepulcre, 2016; Wang et al., 2016). Leukotrienes increase leukocyte accumulation, phagocytic capacity for microbial ingestion and elimination, and the generation of other proinflammatory mediators (Peters-Golden et al., 2005). Cyclooxygenases enhance prostaglandin production (Smith et al., 2000), leading to the induction of the immune response (Gómez-Abellán and Sepulcre, 2016). More importantly, genes for matrix metalloproteinases that are implicated in endothelial damage (i.e., mmp9; Pedersen et al., 2015) were among the most overexpressed genes in B samples at 12 hpi (Table 2; Supplementary Table S2). Related to this damage, genes related to endothelial regeneration (angiogenesis) were also upregulated (angiopoietins angpt2 and angpt1 in B and RBCs samples, respectively; Table 2; Supplementary Table S2).
Epigenetic Response
Several histone-related genes (acetylases, deacetylases, and methyltransferases, among others) were found to be DEGs (both up- and downregulated) mainly by RBCs (Table 2; Supplementary Table S2). This effect could be associated with an epigenetic response probably related to modulation of the immune response by gene silencing through methylation (Medzhitov and Horng, 2009; Shakespear et al., 2011). In parallel, we also detected a strongly upregulated gene for an anti-silencing protein in RBCs and WBCs samples at 3 hpi (Table 3; Supplementary Table S2). Anti-silencing proteins are evolutionarily conserved proteins that act as histone chaperones and are required for various chromatin-mediated cellular processes. Recently, it has been demonstrated that all these proteins are involved in antiviral mechanisms promoting Ifnb production (Liu et al., 2016).
Relationship Between Systemic and Mucosal Immunity
It was not surprising to find DEGs that evidenced a link between systemic and mucosal immunity that our group had previously shown to occur in eels vaccinated against V. vulnificus (Esteve-Gassent et al., 2003). Among them, it should be highlighted muc2A, a gene for a mucolipin secreted by mucosal cells that is involved in binding to bacteria for killing (McGuckin et al., 2011; Brinchmann, 2016) and that was upregulated at 3 hpi by both RBCs and WBCs (Table 2; Supplementary Table S2).
RNA-Based Response
One of the most striking results of the present study was the strong upregulation by B samples of a gene for a specific transporter of a systemic interference RNA, sidt1 (systemic RNAi deficient-1; Li et al., 2015), which was detected at 12 hpi (Table 2). This result strongly suggested that a systemic RNAi may be acting during the immune response against V. vulnificus. It is well known that systemic RNAi, common to all vertebrates, is involved in ancestral innate defense mechanisms against viral infections (Li et al., 2015). Since we detected upregulation of this gene in the B samples and not in WBCs samples, we performed RT-qPCR on the same samples and found the gene to be upregulated in WBCs at 3 and 12 hpi (Table 2).
Functional Assays
The transcriptomic results were confirmed by evaluating different enzymatic and lytic activities in eel serum samples. We detected proteolytic, hemolytic, and bacteriolytic activities in serum that were significantly increased at 3 hpi and 12 hpi compared to those found in serum samples at 0 hpi and those found in serum samples from non infected animals (Table 3).
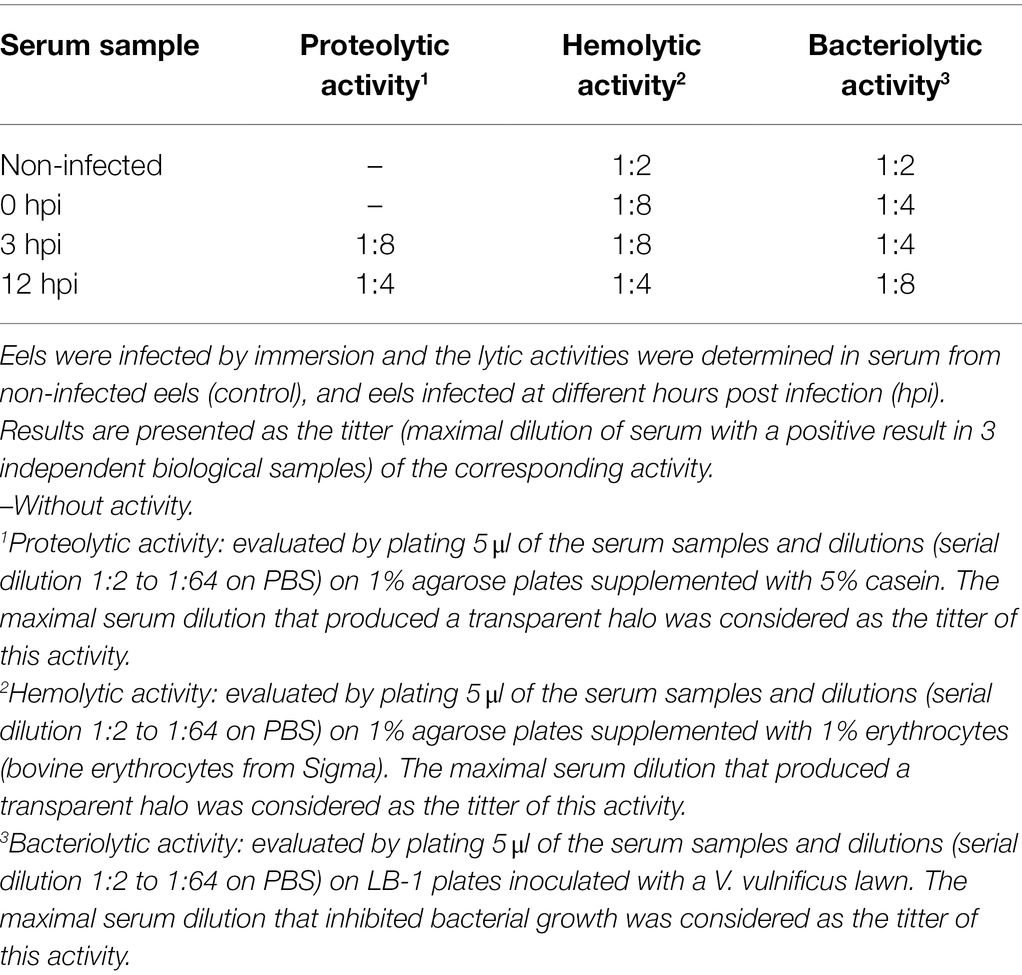
Table 3. Proteolytic, hemolytic and bacteriolytic activity of eel serum before and after V. vulnificus infection.
Early Diagnosis of Fish Septicemia by RT-qPCR
The use of selected gene markers for the early detection of fish septicemia was evaluated by RT-qPCR from eels infected by immersion with V. vulnificus. To do so, we selected the most upregulated genes related with antibacterial activity (npsn), endothelial damage and acute phase of infection (cox2 and mmp9) and the transporter of a systemic interference RNA (sidt1; Table 2). We infected eels with V. vulnificus and analyzed the expression of the selected genes in blood of the infected animals at 3 and 12 hpi compared to non-infected eels. All the selected genes were easily detected upregulated in blood of the infected animals, especially cox2 and sidt1 at 3 hpi (Table 4). Therefore, we propose that this easy and fast methodology could be used to the early diagnose of Vv-vibriosis.
Discussion
V. vulnificus is an emerging zoonotic pathogen associated with fish farms as all clonal groups defined in the species have emerged from outbreaks of fish vibriosis in farms and contain clinical isolates from fish and humans (Roig et al., 2018; Carmona-Salido et al., 2021). Interestingly, this species uses both generalist and host-specific virulence mechanisms, the former mainly related to its toxins and exoenzymes, and the latter to resistance to innate immunity (Hernández-Cabanyero et al., 2019). Using both, V. vulnificus can survive and cause rapid death by septicemia in hosts as evolutionarily distant as humans and eels. Previous studies using mice as an animal model suggested that sepsis death of their original hosts may be due to an early cytokine storm triggered by the pathogen during its interaction with the immune system (Murciano et al., 2017). In this work, we set out to demonstrate this hypothesis using one of the natural hosts of the disease, the eel, and reproducing the natural conditions of infection with a representative strain of the most studied zoonotic group. For the study, a microarray platform was used that was designed from the transcriptome of the hematopoietic organs of eels stimulated with viral/bacterial PAMPs and was consequently enriched in immune genes (Callol et al., 2015b).
First, we highlight the critical role that eel RBCs appear to play in the defense against V. vulnificus and, probably, against bacterial pathogens in general. We suspected that RBCs were immunologically active cells because we had observed that bacteria agglutinated in the presence of eel erythrocytes in vitro (Lee et al., 2013). In this work, we found that RBCs do indeed activate multiple lectin genes in response to V. vulnificus infection that could exert this antibacterial function. We also found that RBCs are genetically primed to act as antigen-presenting cells, as they also activate the transcription of extracellular and intracellular Prrs (Tlrs and Nods), as well as Mhc classes I and II. Similar results were previously found in rainbow trout RBCs which express mhcII in response to virus (Nombela et al., 2019). In addition, they are genetically prepared to produce proinflammatory cytokines such as Il17 and Il20 as well as Il12β, whose hypothetic function will be commented on later (Rutz et al., 2014; Zou and Secombes, 2016). Although demonstrating that RBCs act as antigen-presenting cells or produce these cytokines are beyond the scope of this work, these results are consistent with what we know about eel vibriosis, and with the results we have obtained when analyzing the other blood fractions.
Thus, eel WBCs also appear to be very active during the first hours of infection, upregulating the transcription of Prr genes for extracellular and intracellular antigens and, interestingly, only MhcI, the form of Mhc associated with intracellular antigen presentation. In this regard, our results suggest that both RBCs and WBCs may overexpress Tlrs that in fish detect double-stranded RNA (Tlr3 and Tlr13) and DNA (Tlr9 and Tlr21) both extracellularly (Tlr21) and intracellularly (Tlr3, Tlr9, and Tlr13), again suggesting that V. vulnificus could be recognized and processed as if it was an intracellular pathogen. We also observed that eel WBCs could produce an orthologue of mammalian Tlr6 whose function is unknown, as it has not been previously described in any fish species. As expected, we also found considerable evidence that eel WBCs could produce numerous antibacterial compounds and act as phagocytic cells, especially in the short term after infection.
Our transcriptomic results also suggest that signaling pathways would converge in RBCs and WBCs on Iraq4 and Traf3, consistent with activation at 3 hpi of an atypical proinflammatory response typically antiviral (Table 2; Supplementary Table S2). Thus, RBCs and WBCs activated the transcription of genes for Il12β, Il17, and Il20 and several genes for type 1 interferons. These interleukins have been linked to mucosal inflammation in mice and humans, especially in inflammatory bowel diseases (Rutz et al., 2014; Zou and Secombes, 2016; Moschen et al., 2019), while Il12β has been linked to antiviral response in both fish and humans (Sakai et al., 2021). This result is very interesting. Firstly, because links systemic and mucosal immunity, which correlates with previous results showing that eels vaccinated via mucosal route, produce both mucosal and systemic antibodies against V. vulnificus that protect them against Vv-vibriosis (Fouz et al., 2001; Esteve-Gassent et al., 2003). Secondly, the production of Il12β, interferon type 1, and their regulators together with the activation of genes for intracellular antigen recognition and processing mentioned above strongly evidence that this pathogen could be recognized as if it was an intracellular pathogen. Finally, we also found strong evidence that this atypical early immune response leads to a typical inflammatory response at 12 hpi, with upregulation of il1β, il8, and the il1βr that were detected in B and WBCs samples.
In parallel to all these processes, cell death mechanisms by autophagy and apoptosis are probably activated, especially in WBCs. At the same time, RBCs mainly would suffer a stressful situation, as indicated by the strong upregulation of stress markers (Table 2; Supplementary Table S2). Although an increase in the number of WBCs occurs as a natural response in bacterial infections, we did not observe this proliferation in response to V. vulnificus, which would be compatible with death by apoptosis or autophagy of a fraction of WBCs. Related to this, we also observed a drastic reduction in the transcription of most of the genes that had been upregulated at 3 hpi. In contrast, RBCs changed their transcriptional pattern by stopping to transcribe genes for proinflammatory cytokines and chemokines and transcribing genes for MhcI, c-Fos, JunB, Irf2A, Irf2B, and cathepsin B. An overproduction of c-Fos, JunB, and cathepsin B has been linked in fish to tissue repair and the over-activation of irf2A and irf2B with inhibition of interferons alpha and beta (Sato et al., 2009; Botwright et al., 2021), both processes probably related with an attempt to control the strong immune response that was activated at 3 hpi.
Thus, the pathogen would activate an atypical cytokine storm at 3 hpi, followed by a strong inflammation at 12 hpi and blood cell stress and death. This strong inflammation could also lead to endothelial destruction, evidenced by a significant strong activation of sepsis markers related to this destruction; a result compatible with natural disease given that this disease is known as hemorrhagic septicemia (Ince et al., 2016). Beneath this inflammatory response, we found evidence for the activation of a systemic RNAi. Systemic RNAi are part of the conserved biological response mechanisms to double-stranded RNA and are involved in resistance to endogenous and exogenous pathogenic nucleic acids (Abubaker et al., 2014). Its function in fish innate immunity is entirely unknown. Taking all the above mentioned results into account, we hypothesized that V. vulnificus could activate a response against endogenous RNA that, in turn, would trigger the cytokine storm. In fact, it has been recently published the activation of this kind of response in patients with sepsis (Chousterman et al., 2017). Further, we hypothesized that the toxin RtxA1 would be one of the responsible virulence factors.
Previous studies demonstrated that mutants deficient in this toxin kept the ability to infect and invade the bloodstream but were unable to cause death by sepsis in fish while were attenuated in virulence and unable to activate the early cytokine storm in mice (Lee et al., 2013; Murciano et al., 2017). Similarly, in human immune cells, the RtxA1 toxin enhances inflammatory pathways (Kim et al., 2020). In addition, this toxin has an intracellular existence as it is secreted after contact with the eukaryotic cell, associates with the cell membrane for its terminal ends, and forms a pore that allows the central module to enter, self-process, and release the functional domains that attack the cell (Satchell, 2015). Studies are in progress to demonstrate this hypothesis.
Our concluding remarks are summarized in Figures 3 and 4, which present a model of the immune response against V. vulnificus that sheds light on the comprehension of the disease caused by this zoonotic pathogen in its hosts. It should be noticed that although mammalian RBCs are not nucleated and thus considered not active during the immune response, a recent study has demonstrated that human and murine RBCs are involved in the innate immune response to virus (Lam et al., 2021). Therefore, the proposed model could potentially be extended to all V. vulnificus hosts, including humans. According to our model, V. vulnificus indeed triggers an acute but atypical inflammatory response that occurs in two main phases. In the early phase (3 hpi; Figure 3), the pathogen triggers the upregulation of a series of proinflammatory cytokine genes related to the mucosal immune response (il17a/f1 and il20) along with antiviral cytokine genes (il12β) and antiviral factors (ifna and ifnc), while in the late phase (12 hpi; Figure 4) the upregulation of genes for typical inflammatory cytokines (il1β), endothelial destruction (mmp9 and hyal2) and, interestingly, genes related to an RNA-based immune response. Remarkably, some of these genes, especially the gene for systemic RNAi transporter (sidt1), could be used for the early detection of septicemia caused by V. vulnificus infection, as we could diagnose it from blood samples from artificially infected eels by using an RT-qPCR targeting this gene. However, this proposal should be validated with other fish species and by reproducing the vibriosis caused by other Vibrios to determine whether this gene marker is exclusive of Vv-vibriosis. Functional assays also highlighted that the serum from infected animals is proteolytic, hemolytic, and bacteriolytic, partially confirming the transcriptomic results. Finally, we found considerable evidence that RBCs are transcriptionally active and that they may contribute significantly to this atypical immune response, especially in the short term.
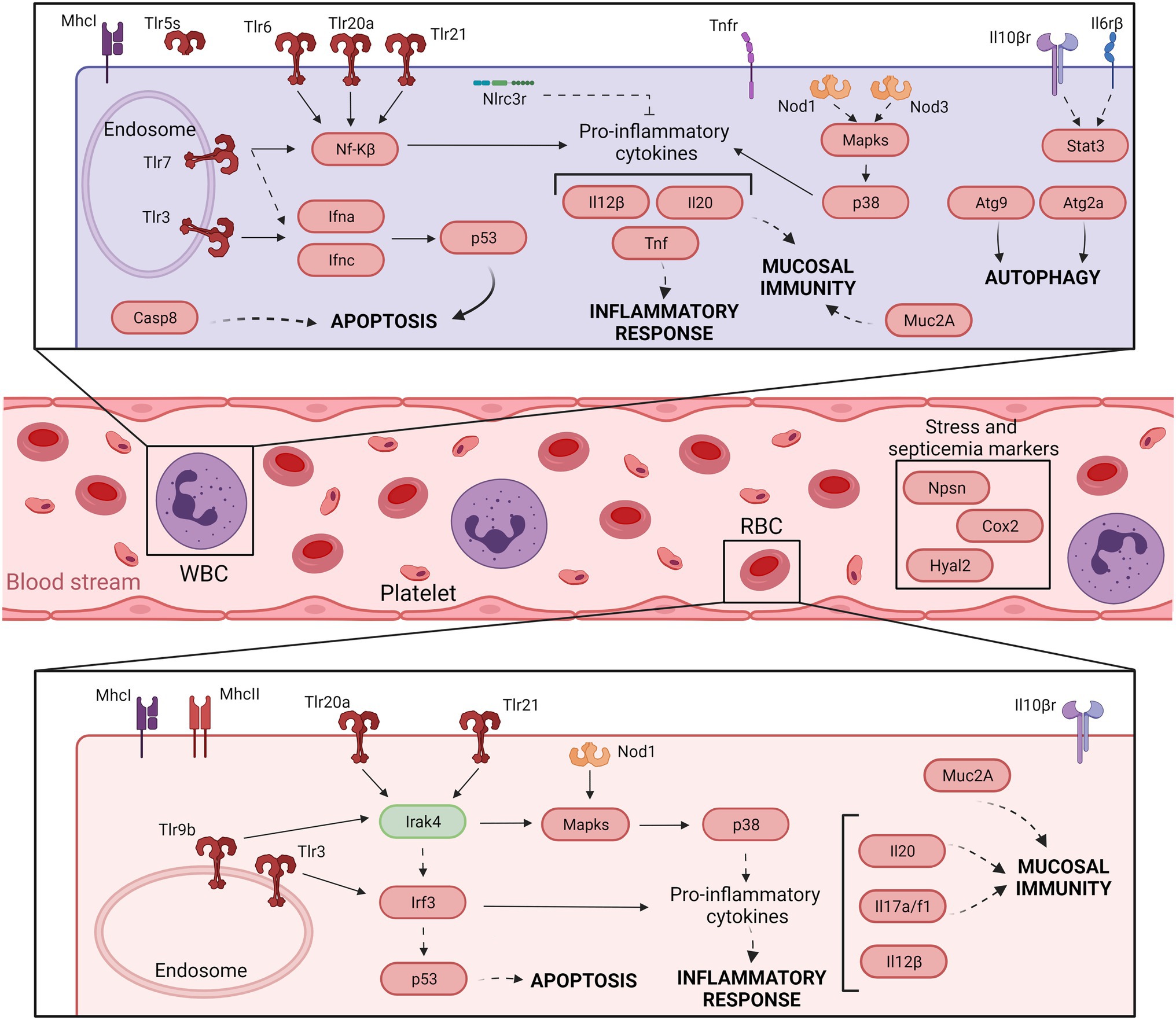
Figure 3. Model of the immune response in eel blood against V. vulnificus infection: early phase of vibriosis (3 hpi). The model shows the resultant proteins produced by the main transcripts differentially expressed by eels’ blood cells during the early phase of Vv-vibriosis (at 3 hpi with V. vulnificus R99 strain) infection. The putative translated proteins from the major immune-related pathways are represented in a code color depending on the gene modulation: upregulated (red) and downregulated (green).
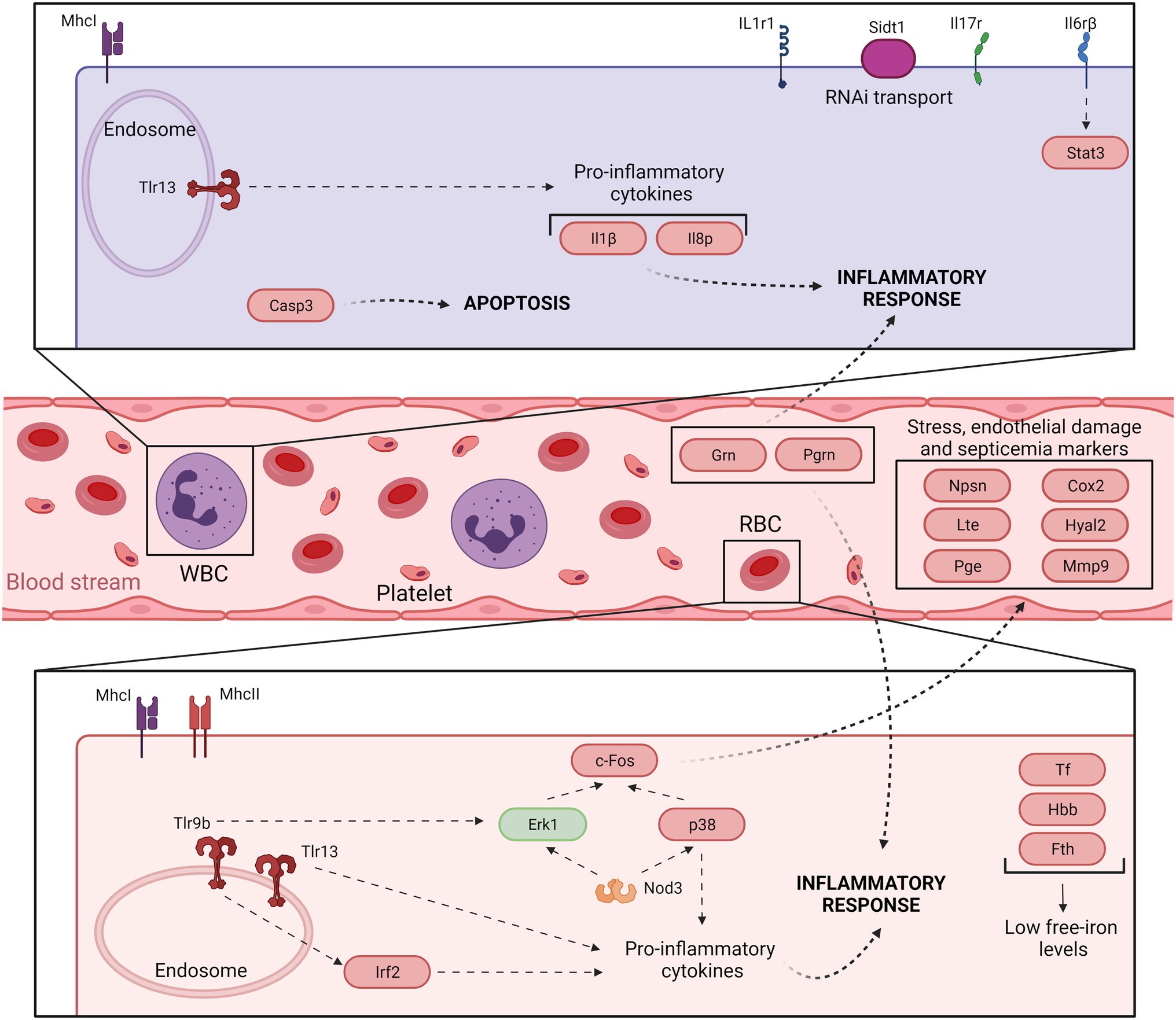
Figure 4. Model of the immune response in eel blood against V. vulnificus infection: late phase of vibriosis (12 hpi). The model shows the resultant proteins produced by the main transcripts differentially expressed by eels’ blood cells during the late phase of Vv-vibriosis (at 12 hpi with V. vulnificus R99 strain) infection. The putative translated proteins from the major immune-related pathways are represented in a code color depending on the gene modulation: upregulated (red) and downregulated (green).
Data Availability Statement
The datasets presented in this study can be found in online repositories. The names of the repository/repositories and accession number(s) can be found at: Gene Expression Omnibus - GSE196944.
Ethics Statement
All assays involving animals were approved by the Institutional Animal Care and Use Committee and the local authority (Conselleria de Agricultura, Medio Ambiente, Cambio Climático y Desarrollo Rural. Generalitat Valenciana) to use eel for scientific research purposes under the protocol 2016-USC-PEA-00033 type 2. The experiments were carried out following the European Directive 2010/63/EU and the Spanish law “Real Decreto” 53/2013.
Author Contributions
CA conceived the study and performed the initial design that CH-C improved. CH-C, ES, and FER-L performed the laboratory experiments. CH-C and EV-V analyzed the data. CH-C wrote the first draft of the manuscript that was corrected and improved by CA. CA and CH-C built the final version taking into account all the corrections and suggestions of the other authors. All authors read and approved the submitted version.
Funding
This work has been financed by grants AGL2017-87723-P co-funded with FEDER funds) from the Ministry of Science, Innovation, and Universities (Spain) and AICO/2018/123 and AICO/2020/076 from Generalitat Valenciana (Spain). CH-C has been financed by grant BES-2015-073117, an FPI grant from the Ministry of Science, Innovation and Universities (Spain). EV-V and FER-L thank the support of Fondecyt iniciación (project number 11221308) and Fondecyt regular (project number: 1211841) (Agencia Nacional de Investigación y Desarrollo (ANID), Government of Chile) grants, respectively. This work was also supported by Ministerio de Ciencia e Innovación (MICIN/AEI) (DOI ID: 10.13039/501100011033), PID2020-120619RB-I00 to CA.
Conflict of Interest
The authors declare that the research was conducted in the absence of any commercial or financial relationships that could be construed as a potential conflict of interest.
Publisher’s Note
All claims expressed in this article are solely those of the authors and do not necessarily represent those of their affiliated organizations, or those of the publisher, the editors and the reviewers. Any product that may be evaluated in this article, or claim that may be made by its manufacturer, is not guaranteed or endorsed by the publisher.
Supplementary Material
The Supplementary Material for this article can be found online at: https://www.frontiersin.org/articles/10.3389/fmicb.2022.852677/full#supplementary-material
Supplementary Figure S1 | Experimental design used in this study and comparisons performed in the transcriptomic analysis. For specific information about procedures see Materials and methods section.
References
Abubaker, S., Abdalla, S., Mahmud, S., and Wilkie, B. (2014). Antiviral innate immune response of RNA interference. J. Inf. Dev. Ctries. 8, 804–810. doi: 10.3855/jidc.4187
Amaro, C., Biosca, E. G., Fouz, B., Alcaide, E., and Esteve, C. (1995). Evidence that water transmits Vibrio vulnificus biotype 2 infections to eels. Appl. Environ. Microbiol. 61, 1133–1137. doi: 10.1128/aem.61.3.1133-1137.1995
Amaro, C., Fouz, B., Sanjuán, E., and Romalde, J. (2020). “Climate change and infectious fish diseases,” in Vibriosis. eds. P. T. K. Woo, J. A. Leong, and K. Buchmann (UK: CABI).
Amaro, C., Sanjuán, E., Fouz, B., Pajuelo, D., Lee, C. T., Hor, L., et al. (2015). The fish pathogen Vibrio vulnificus biotype 2: epidemiology, phylogeny and virulence factors involved in warm-water vibriosis. Microbiol. Spect. 3:5-2014. doi: 10.1128/microbiolspec.VE-0005-2014
Baker-Austin, C., Oliver, J. D., Alam, M., Ali, A., Waldor, M. K., Qadri, F., et al. (2018). Vibrio spp. Infect. Nat. Rev. Dis. Prim. 4, 1–19. doi: 10.1038/s41572-018-0005-8
Baker-Austin, C., Trinanes, J. A., Taylor, N. G. H., Hartnell, R., Siitonen, A., and Martinez-Urtaza, J. (2012). Emerging vibrio risk at high latitudes in response to ocean warming. Nat. Clim. Chang. 3, 73–77. doi: 10.1038/nclimate1628
Bateman, A., Belcourt, D., Bennett, H., Lazure, C., and Solomon, S. (1990). Granulins, a novel class of peptide from leukocytes. Biochem. Biophys. Res. Commun. 173, 1161–1168. doi: 10.1016/S0006-291X(05)80908-8
Botwright, N. A., Mohamed, A. R., Slinger, J., Lima, P. C., and Wynne, J. W. (2021). Host-parasite interaction of Atlantic salmon (Salmo salar) and the Ectoparasite Neoparamoeba perurans in amoebic gill disease. Front. Immunol. 12:672700. doi: 10.3389/fimmu.2021.672700
Brinchmann, M. F. (2016). Immune relevant molecules identified in the skin mucus of fish using -omics technologies. Mol. BioSyst. 12, 2056–2063. doi: 10.1039/c5mb00890e
Callol, A., Pajuelo, D., Ebbesson, L., Teles, M., MacKenzie, S., and Amaro, C. (2015a). Early steps in the European eel (Anguilla anguilla)-Vibrio vulnificus interaction in the gills: role of the RtxA13 toxin. Fish Shellfish Immun. 43, 502–509. doi: 10.1016/j.fsi.2015.01.009
Callol, A., Reyes-López, F. E., Roig, F. J., Goetz, G., Goetz, F. W., Amaro, C., et al. (2015b). An enriched European eel Transcriptome sheds light upon host-pathogen interactions with Vibrio vulnificus. PLoS One 10:e0133328. doi: 10.1371/journal.pone.0133328
Carmona-Salido, H., Fouz, B., Sanjuán, E., Carda-Diéguez, M., Delannoy, C. M. J., García-González, N., et al. (2021). Draft genome sequences of Vibrio vulnificus strains recovered from moribund tilapia. Microbiol. Resour. Announc. 10, e0009421–e0009425. doi: 10.1128/mra.00094-21
Ceccarelli, D., Amaro, C., Romalde, J., Suffredini, E., and Vezzulli, L. (2019). “Vibrio species,” in Food Microbiology: Fundamentals and Frontiers. 5th Edn. eds. M. Doyle, F. Diez-González, and C. Hill (Washington. DC: ASM Press).
Chousterman, B. G., Swirski, F. K., and Weber, G. F. (2017). Cytokine storm and sepsis disease pathogenesis. Semin. Immunopathol. 39, 517–528. doi: 10.1007/s00281-017-0639-8
Dahle, M. K., Wessel, Ø., Timmerhaus, G., Nyman, I. B., Jørgensen, S. M., Rimstad, E., et al. (2015). Transcriptome analyses of Atlantic salmon (Salmo salar L.) erythrocytes infected with piscine orthoreovirus (PRV). Fish Shellfish Immun. 45, 780–790. doi: 10.1016/j.fsi.2015.05.049
Di, Q., Lin, Q., Huang, Z., Chi, Y., Chen, X., Zhang, W., et al. (2017). Zebrafish nephrosin helps host defence against Escherichia coli infection. Open Biol. 7:40. doi: 10.1098/rsob.170040
Esteve-Gassent, M. D., Nielsen, M. E., and Amaro, C. (2003). The kinetics of antibody production in mucus and serum of European eel (Anguilla anguilla) after vaccination against Vibrio vulnificus: development of a new method for antibody quantification in skin mucus. Fish Shellfish Immun. 15, 51–61. doi: 10.1016/S1050-4648(02)00138-9
Fouz, B., Esteve-Gassent, M. D., Barrera, R., Larsen, J. L., and Nielsen, M. E. (2001). Field testing of a vaccine against eel diseases caused by Vibrio vulnificus. Dis. Aquat. Org. 45, 183–189. doi: 10.3354/dao045183
Gómez-Abellán, V., and Sepulcre, M. P. (2016). The role of prostaglandins in the regulation of fish immunity. Mol. Immunol. 69, 139–145. doi: 10.1016/j.molimm.2015.09.022
Hernández-Cabanyero, C., Lee, C. T., Tolosa-Enguis, V., Sanjuán, E., Pajuelo, D., Reyes-López, F., et al. (2019). Adaptation to host in Vibrio vulnificus, a zoonotic pathogen that causes septicemia in fish and humans. Environ. Microbiol. 21, 3118–3139. doi: 10.1111/1462-2920.14714
Hernández-Cabanyero, C., Sanjuán, E., Fouz, B., Pajuelo, D., Vallejos-Vidal, E., Reyes-López, F. E., et al. (2020). The effect of the environmental temperature on the adaptation to host in the zoonotic pathogen Vibrio vulnificus. Front. Microbiol. 11:489. doi: 10.3389/fmicb.2020.00489
Hoben, H. J., and Somasegaran, P. (1982). Comparison of the pour, spread, and drop plate methods for enumeration of rhizobium spp. in inoculants made from presterilized peat. Appl. Environ. Microbiol. 44, 1246–1247. doi: 10.1128/aem.44.5.1246-1247.1982
Inagawa, H., Honda, T., Kohchi, C., Nishizawa, T., Yoshiura, Y., Nakanishi, T., et al. (2002). Cloning and characterization of the homolog of mammalian lipopolysaccharide-binding protein and bactericidal permeability-increasing protein in rainbow trout Oncorhynchus mykiss. J. Immunol. 168, 5638–5644. doi: 10.4049/jimmunol.168.11.5638
Ince, C., Mayeux, P. R., Nguyen, T., Gomez, H., Kellum, J. A., Ospina-Tascón, G. A., et al. (2016). The endothelium in sepsis. Shock 45, 259–270. doi: 10.1097/SHK.0000000000000473
Jehannet, P., Heinsbroek, L. T. N., and Palstra, A. P. (2017). Ultrasonography to assist with timing of spawning in European eel. Theriogenology 101, 73–80. doi: 10.1016/j.theriogenology.2017.06.016
Jeong, H. G., and Satchell, K. J. F. (2012). Additive function of Vibrio vulnificus MARTXVv and VvhA cytolysins promotes rapid growth and epithelial tissue necrosis during intestinal infection. PLoS Pathog. 8:e1002581. doi: 10.1371/journal.ppat.1002581
Kim, B. S., Kim, J. H., Choi, S., Park, S., Lee, E. Y., Koh, S., et al. (2020). MARTX toxin-stimulated interplay between human cells and Vibrio vulnificus. mSphere. 5:20. doi: 10.1128/mSphere.00659-20
Kim, Y. K., Shin, J. S., and Nahm, M. H. (2016). NOD-like receptors in infection, immunity, and diseases. Yonsei Med. J. 57, 5–14. doi: 10.3349/ymj.2016.57.1.5
Lam, L. K. M., Murphy, S., Kokkinaki, D., Venosa, A., Sherrill-Mix, S., Casu, C., et al. (2021). DNA binding to TLR9 expressed by red blood cells promotes innate immune activation and anemia. Sci. Transl. Med. 13:eabj1008. doi: 10.1126/scitranslmed.abj1008
Lee, C. T., Amaro, C., Wu, K. M., Valiente, E., Chang, Y. F., Tsai, S. F., et al. (2008). A common virulence plasmid in biotype 2 Vibrio vulnificus and its dissemination aided by a conjugal plasmid. J. Bacteriol. 190, 1638–1648. doi: 10.1128/JB.01484-07
Lee, C. T., Pajuelo, D., Llorens, A., Chen, Y. H., Leiro, J. M., Padrós, F., et al. (2013). MARTX of Vibrio vulnificus biotype 2 is a virulence and survival factor. Environ. Microbiol. 15, 419–432. doi: 10.1111/j.1462-2920.2012.02854.x
Li, W., Koutmou, K. S., Leahy, D. J., and Li, M. (2015). Systemic RNA interference Deficiency-1 (SID-1) extracellular domain selectively binds long double-stranded RNA and is required for RNA transport by SID-1. J. Biol. Chem. 290, 18904–18913. doi: 10.1074/jbc.M115.658864
Liu, Z., Yang, L., Sun, Y., Xie, X., and Huang, J. (2016). ASF1a enhances antiviral immune response by associating with CBP to mediate acetylation of H3K56 at the Ifnb promoter. Mol. Immunol. 78, 57–64. doi: 10.1016/j.molimm.2016.08.008
Livak, K. J., and Schmittgen, T. D. (2001). Analysis of relative gene expression data using real-time quantitative PCR and. Methods 25, 402–408. doi: 10.1006/meth.2001.1262
Lund, J. M., Alexopoulou, L., Sato, A., Karow, M., Adams, N. C., Gale, N. W., et al. (2004). Recognition of single-stranded RNA viruses by toll-like receptor 7. PNAS 101, 5598–5603. doi: 10.1073/pnas.0400937101
Marco-Noales, E., Milán, M., Fouz, B., Sanjuán, E., and Amaro, C. (2001). Transmission to eels, portals of entry, and putative reservoirs of Vibrio vulnificus Serovar E (Bioype 2). Appl. Environ. Microbiol. 67, 4717–4725. doi: 10.1128/AEM.67.10.4717
Matsumoto, F., Saitoh, S. I., Fukui, R., Kobayashi, T., Tanimura, N., Konno, K., et al. (2008). Cathepsins are required for toll-like receptor 9 responses. Biochem. Biophys. Res. Commun. 367, 693–699. doi: 10.1016/j.bbrc.2007.12.130
McGuckin, M. A., Lindén, S. K., Sutton, P., and Florin, T. H. (2011). Mucin dynamics and enteric pathogens. Nat. Rev. Microbiol. 9, 265–278. doi: 10.1038/nrmicro2538
Medzhitov, R., and Horng, T. (2009). Transcriptional control of the inflammatory response. Nat. Rev. Immunol. 9, 692–703. doi: 10.1038/nri2634
Mes, D., Dirks, R. P., and Palstra, A. P. (2016). Simulated migration under mimicked photothermal conditions enhances sexual maturation of farmed European eel (Anguilla anguilla). Aquaculture 452, 367–372. doi: 10.1016/j.aquaculture.2015.11.020
Morera, D., Roher, N., Ribas, L., Balasch, J. C., Doñate, C., Callol, A., et al. (2011). Rna-seq reveals an integrated immune response in nucleated erythrocytes. PLoS One 6:e26998. doi: 10.1371/journal.pone.0026998
Moschen, A. R., Tilg, H., and Raine, T. (2019). IL-12, IL-23 and IL-17 in IBD: immunobiology and therapeutic targeting. Nat. Rev. Gastroenterol. Hepatol. 16, 185–196. doi: 10.1038/s41575-018-0084-8
Murciano, C., Lee, C. T., Fernández-Bravo, A., Hsieh, T. H., Fouz, B., Hor, L. I., et al. (2017). MARTX toxin in the zoonotic serovar of Vibrio vulnificus triggers an early cytokine storm in mice. Front. Cell. Infect. Microbiol. 7:332. doi: 10.3389/fcimb.2017.00332
Nombela, I., Carrion, A., Puente-Marin, S., Chico, V., Mercado, L., Perez, L., et al. (2017). Infectious pancreatic necrosis virus triggers antiviral immune response in rainbow trout red blood cells, despite not being infective. F1000Res. 6:1968. doi: 10.12688/f1000research.12994.2
Nombela, I., and Ortega-Villaizan, M. M. (2018). Nucleated red blood cells: immune cell mediators of the antiviral response. PLoS Pathog. 14:e1006910. doi: 10.1371/journal.ppat.1006910
Nombela, I., Requena-Platek, R., Morales-Lange, B., Chico, V., Puente-Marin, S., Ciordia, S., et al. (2019). Rainbow trout red blood cells exposed to viral hemorrhagic septicemia virus up-regulate antigen-processing mechanisms and MHC I&II, CD86, and CD83 antigen-presenting cell markers. Cell 8:386. doi: 10.3390/cells8050386
Oliver, J. D. (2015). The biology of Vibrio vulnificus. Microbiol. Spect. 3, 1–10. doi: 10.1128/microbiolspec.VE-0001-2014
Pajuelo, D., Hernández-Cabanyero, C., Sanjuan, E., Lee, C. T., Silva-Hernández, F. X., Hor, L. I., et al. (2016). Iron and fur in the life cycle of the zoonotic pathogen vibrio vulnificus. Environ. Microbiol. 18, 4005–4022. doi: 10.1111/1462-2920.13424
Pajuelo, D., Lee, C. T., Roig, F. J., Hor, L. I., and Amaro, C. (2015). Novel host-specific iron acquisition system in the zoonotic pathogen Vibrio vulnificus. Environ. Microbiol. 17, 2076–2089. doi: 10.1111/1462-2920.12782
Palstra, A. P., Cohen, E. G. H., Niemantsverdriet, P. R. W., Van Ginneken, V. J. T., and Van Den Thillart, G. E. E. J. M. (2005). Artificial maturation and reproduction of European silver eel: development of oocytes during final maturation. Aquaculture 249, 533–547. doi: 10.1016/j.aquaculture.2005.04.031
Paria, A., Dong, J., Suresh Babu, P. P., Makesh, M., Chaudhari, A., Thirunavukkarasu, A. R., et al. (2016). Evaluation of candidate reference genes for quantitative expression studies in asian seabass (Lates calcarifer) during ontogenesis and in tissues of healthy and infected fishes. Indian J. Exp. Biol. 54, 597–605.
Park, B., Buti, L., Lee, S., Matsuwaki, T., Spooner, E., Brinkmann, M. M., et al. (2011). Granulin is a soluble cofactor for toll-like receptor 9 signaling. Immunity 34, 505–513. doi: 10.1016/j.immuni.2011.01.018
Pedersen, M. E., Vuong, T. T., Rønning, S. B., and Kolset, S. O. (2015). Matrix metalloproteinases in fish biology and matrix turnover. Matrix Biol. 44, 86–93. doi: 10.1016/j.matbio.2015.01.009
Peters-Golden, M., Canetti, C., Mancuso, P., and Coffey, M. J. (2005). Leukotrienes: underappreciated mediators of innate immune responses. J. Immunol. 174, 589–594. doi: 10.4049/jimmunol.174.2.589
Rawlings, J. S., Rosler, K. M., and Harrison, D. A. (2004). The JAK/STAT signaling pathway. J. Cell Sci. 117, 1281–1283. doi: 10.1242/jcs.00963
Reed, L. J., and Muench, H. (1938). A simple method of esti- mating fifty percent endpoints. Am. J. Epidemiol. 27, 493–497. doi: 10.1093/oxfordjournals.aje.a118408
Roig, F. J., González-Candelas, F., Sanjuán, E., Fouz, B., Feil, E. J., Llorens, C., et al. (2018). Phylogeny of Vibrio vulnificus from the analysis of the core-genome: implications for intra-species taxonomy. Front. Microbiol. 8:2613. doi: 10.3389/fmicb.2017.02613
Rutz, S., Wang, X., and Ouyang, W. (2014). The IL-20 subfamily of cytokines — from host defence to tissue homeostasis. Nat. Rev. Immunol. 14, 783–795. doi: 10.1038/nri3766
Sakai, M., Hikima, J., and Kono, T. (2021). Fish cytokines: current research and applications. Fish. Sci. 87, 1–9. doi: 10.1007/s12562-020-01476-4
Satchell, K. J. F. (2015). Multifunctional-autoprocessing repeats-in-toxin (MARTX) toxins of Vibrios. Microbiol. Spect. 3:2014. doi: 10.1128/microbiolspec.VE-0002-2014.f1
Sato, T., Onai, N., Yoshihara, H., Arai, F., Suda, T., and Ohteki, T. (2009). Interferon regulatory factor-2 protects quiescent hematopoietic stem cells from type i interferon-dependent exhaustion. Nat. Med. 15, 696–700. doi: 10.1038/nm.1973
Schneider, M., Zimmermann, A. G., Roberts, R. A., Zhang, L., Karen, V., Rahman, A. H., et al. (2013). The innate immune sensor NLRC3 attenuates toll-like receptr signaling via modification of the signaling adaptor TRAF6 and ttranscription factor NF-κB. Nat. Immunol. 13, 823–831. doi: 10.1038/ni.2378
Shakespear, M. R., Halili, M. A., Irvine, K. M., Fairlie, D. P., and Sweet, M. J. (2011). Histone deacetylases as regulators of inflammation and immunity. Trends Immunol. 32, 335–343. doi: 10.1016/j.it.2011.04.001
Smith, W. L., Dewitt, D. L., and Garavito, R. M. (2000). Cyclooxigenases: structural, cellular, and molecular biology. Annu. Rev. Biochem. 69, 145–182. doi: 10.1146/annurev.biochem.69.1.145
Valiente, E., and Amaro, C. (2006). A method to diagnose the carrier state of Vibrio vulnificus serovar E in eels: development and field studies. Aquaculture 258, 173–179. doi: 10.1016/j.aquaculture.2006.05.002
Valiente, E., Lee, C. T., Hor, L. I., Fouz, B., and Amaro, C. (2008a). Role of the metalloprotease Vvp and the virulence plasmid pR99 of Vibrio vulnificus serovar E in surface colonization and fish virulence. Environ. Microbiol. 10, 328–338. doi: 10.1111/j.1462-2920.2007.01454.x
Valiente, E., Padrós, F., Lamas, J., Llorens, A., and Amaro, C. (2008b). Microbial and histopathological study of the vibriosis caused by Vibrio vulnificus serovar E in eels: The metalloprotease Vvp is not an essential lesional factor. Microb. Pathog. 45, 386–393. doi: 10.1016/j.micpath.2008.09.001
Wang, T., Yan, J., Xu, W., Ai, Q., and Mai, K. (2016). Characterization of Cyclooxygenase-2 and its induction pathways in response to high lipid diet-induced inflammation in Larmichthys crocea. Sci. Rep. 6:19921. doi: 10.1038/srep19921
Workenhe, S. T., Kibenge, M. J. T., Wright, G. M., Wadowska, D. W., Groman, D. B., and Kibenge, F. S. B. (2008). Infectious salmon anaemia virus replication and induction of alpha interferon in Atlantic salmon erythrocytes. Virol. J. 5:36. doi: 10.1186/1743-422X-5-36
Keywords: Vibrio vulnificus, zoonotic pathogen, blood, erythrocytes, European eel, host-pathogen relationship, immune response
Citation: Hernández-Cabanyero C, Sanjuán E, Reyes-López FE, Vallejos-Vidal E, Tort L and Amaro C (2022) A Transcriptomic Study Reveals That Fish Vibriosis Due to the Zoonotic Pathogen Vibrio vulnificus Is an Acute Inflammatory Disease in Which Erythrocytes May Play an Important Role. Front. Microbiol. 13:852677. doi: 10.3389/fmicb.2022.852677
Edited by:
David Kornspan, Kimron Veterinary Institute, IsraelReviewed by:
Keun Hwa Lee, Hanyang University, South KoreaMaria Del Mar Ortega-Villaizan, Miguel Hernández University of Elche, Spain
Panpan Zhao, Jilin University, China
Copyright © 2022 Hernández-Cabanyero, Sanjuán, Reyes-López, Vallejos-Vidal, Tort and Amaro. This is an open-access article distributed under the terms of the Creative Commons Attribution License (CC BY). The use, distribution or reproduction in other forums is permitted, provided the original author(s) and the copyright owner(s) are credited and that the original publication in this journal is cited, in accordance with accepted academic practice. No use, distribution or reproduction is permitted which does not comply with these terms.
*Correspondence: Carmen Amaro, Y2FybWVuLmFtYXJvQHV2LmVz