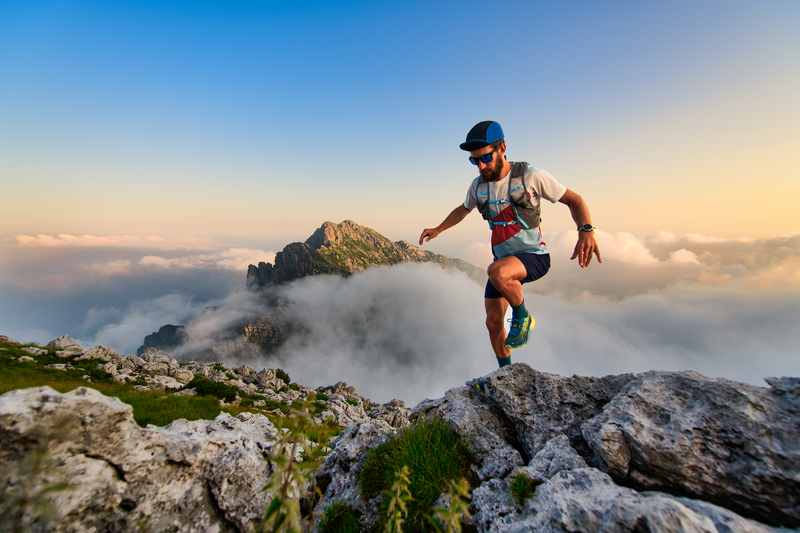
94% of researchers rate our articles as excellent or good
Learn more about the work of our research integrity team to safeguard the quality of each article we publish.
Find out more
ORIGINAL RESEARCH article
Front. Microbiol. , 11 May 2022
Sec. Evolutionary and Genomic Microbiology
Volume 13 - 2022 | https://doi.org/10.3389/fmicb.2022.848536
Bacteria employ small non-coding RNAs (sRNAs) to regulate gene expression. Ms1 is an sRNA that binds to the RNA polymerase (RNAP) core and affects the intracellular level of this essential enzyme. Ms1 is structurally related to 6S RNA that binds to a different form of RNAP, the holoenzyme bearing the primary sigma factor. 6S RNAs are widespread in the bacterial kingdom except for the industrially and medicinally important Actinobacteria. While Ms1 RNA was identified in Mycobacterium, it is not clear whether Ms1 RNA is present also in other Actinobacteria species. Here, using a computational search based on secondary structure similarities combined with a linguistic gene synteny approach, we identified Ms1 RNA in Streptomyces. In S. coelicolor, Ms1 RNA overlaps with the previously annotated scr3559 sRNA with an unknown function. We experimentally confirmed that Ms1 RNA/scr3559 associates with the RNAP core without the primary sigma factor HrdB in vivo. Subsequently, we applied the computational approach to other Actinobacteria and identified Ms1 RNA candidates in 824 Actinobacteria species, revealing Ms1 RNA as a widespread class of RNAP binding sRNAs, and demonstrating the ability of our multifactorial computational approach to identify weakly conserved sRNAs in evolutionarily distant genomes.
Small non-coding RNAs (sRNAs) are important regulators of gene expression in bacteria. A majority of sRNAs act by base-pairing to target mRNAs and change mRNA stability or translation but a minor group of sRNAs directly regulates proteins through RNA-protein interaction (Svensson and Sharma, 2016). The best-known example is 6S RNA that interacts with the RNA polymerase (RNAP) holoenzyme (Wassarman and Storz, 2000; Klocko and Wassarman, 2009; Steuten et al., 2013; Chen et al., 2017; Wassarman, 2018). The RNAP holoenzyme is composed of the catalytic core (E, subunits α2ββ′ω) and the primary σ factor that is required to recognize promoters of housekeeping genes to initiate transcription. All bacteria contain this housekeeping or primary σ factor, termed σ70 in Escherichia coli, σA in Bacillus subtilis and in Mycobacterium smegmatis (Gomez et al., 1998), or HrdB in Streptomyces (Brown et al., 1992). In addition, bacterial species contain different numbers of alternative sigma factors ranging from zero in Mycoplasma genitalium (Fraser et al., 1995) to almost 70 in Streptomyces coelicolor (Bentley et al., 2002).
6S RNA was first described in E. coli (Hindley, 1967) where it interacts with the RNAP-σ70 holoenzyme (Eσ70) (Wassarman and Storz, 2000) and regulates the expression of hundreds of genes (Cavanagh et al., 2008; Neusser et al., 2010). 6S RNA itself also serves as a template for transcription of pRNA, a short RNA of ≤20 nucleotides (Wassarman and Saecker, 2006; Beckmann et al., 2011; Hoch et al., 2016). Transcription of pRNA rearranges the structure of 6S RNA, releasing Eσ from 6S RNA (Wurm et al., 2010; Beckmann et al., 2012; Cavanagh et al., 2012; Panchapakesan and Unrau, 2012; Burenina et al., 2014).
6S RNA has a conserved secondary structure that is critical for the interaction with Eσ70 (Barrick et al., 2005; Trotochaud and Wassarman, 2005; Shephard et al., 2010). 6S RNA forms a double-stranded hairpin like structure with a central, unpaired bubble region which resembles an open promoter (Barrick et al., 2005; Wassarman, 2018). Many 6S RNAs were predicted from the genomic sequences based on secondary structure similarities (Barrick et al., 2005; Trotochaud and Wassarman, 2005; Wehner et al., 2014). Alternatively, 6S RNAs were directly identified as abundant ∼180–200 nt RNAs in B. subtilis, Bordetella pertussis, Pseudomonas aeruginosa or Caulobacter crescentus (Vogel et al., 1987; Barrick et al., 2005; Trotochaud and Wassarman, 2005) or 6S RNAs were discovered after the detection of their complementary pRNAs (Sharma et al., 2010; Köhler et al., 2015).
The intracellular levels of 6S RNAs are high, similar to those of essential non-coding RNAs, such as rRNAs, tRNAs, RNAse P, tmRNA or SRP RNA. Furthermore, 6S RNAs have been found in many bacterial species and are widespread in the bacterial kingdom (Trotochaud and Wassarman, 2005; Faucher et al., 2010; Sharma et al., 2010; Rediger et al., 2012; Wehner et al., 2014; Köhler et al., 2015; Jones et al., 2016; Elkina et al., 2017; Burenina et al., 2020) with one exception—the group of Actinobacteria. This phylum includes serious human pathogens (Mycobacterium tuberculosis, Mycobacterium leprae, and Corynebacterium diphtheria), industrially important producers of amino acids (Corynebacterium glutamicum) and antibiotics (Streptomyces), bacteria involved in symbiotic nitrogen fixation (Frankia), bacteria utilized for bioremediation (Rhodococcus), probiotic bacteria (Bifidobacterium), and numerous other genera (Nocardia, Micrococcus, Gardnerella).
In Actinobacteria, 6S RNAs had been undetected for a long time. In Mycobacterium smegmatis, we identified a putative 6S RNA candidate by the computational suboptimal secondary structure approach (Pánek et al., 2011). However, we discovered that it interacted with the RNAP core (E) without the primary sigma factor (Hnilicova et al., 2014) and, thus, by definition, was not a 6S RNA and we therefore named it Ms1. In Mycobacterium smegmatis, Ms1 accumulates during the stationary phase of growth, regulates the RNAP level, and this facilitates cell outgrowth from stationary phase (Sikova et al., 2019).
Ms1 RNA is longer than a typical 6S RNA (∼300 nt vs. ∼180 nt) and their secondary structures differ as well—in addition to the central bubble, Ms1 has two additional short hairpins at its 3′ and 5′ ends (Hnilicova et al., 2014). An Ms1 homolog was also found in M. tuberculosis (MTS2823 sRNA) (Arnvig et al., 2011). Other Ms1 homologs were identified only in closely related species within the Actinobacteria group (genera Mycobacterium, Rhodococcus and Nocardia) (Hnilicova et al., 2014; Behra et al., 2019). Currently, it is unclear whether Ms1 RNA is present only in the three above-mentioned bacterial genera or is widespread throughout Actinobacteria.
In Streptomyces coelicolor, a putative 6S RNA (the gene was named ssrS) was identified (Panek et al., 2008), but the experimental proof that ssrS encodes 6S RNA was based on an in vitro interaction between the purified RNAP-HrdB holoenzyme and in vitro transcribed 6S RNA (Mikulík et al., 2014). In addition, this interaction was detected only after UV crosslinking (Mikulík et al., 2014). Moreover, in vitro transcribed ssrS gene only partially overlaps with the in vivo detected scr3559 sRNA identified by RNA-seq (Vockenhuber et al., 2011; Moody et al., 2013) which is expressed from the same genomic locus (sco3558-sco3559 intergenic region) and thus it is unclear if the putative 6S RNA sequence is expressed in vivo.
Recently, (Bobek et al., 2021) reported a new 6S RNA in S. coelicolor, which was named “6S-like scr3559 RNA.” It is expressed from the sco3558-sco3559 genomic locus but from the minus strand (the previously published 6S RNA sequence was expressed from the plus strand), with its transcription start site located at position 3,934,888 in the genome (Bobek et al., 2021). This 6S-like RNA is processed and its 5′ end corresponds to position 3,934,820 (Bobek et al., 2021). However, the processed 6S-like RNA was almost undetectable in wild type S. coelicolor by Northern blotting (Bobek et al., 2021). In addition, the 6S-like RNA does not correspond to the scr3559 sRNA that is transcribed from the plus strand with the transcription start site at position 3,934,693 (Moody et al., 2013; Jeong et al., 2016). Both sequences of the putative 6S RNAs (Mikulík et al., 2014; Bobek et al., 2021) differ from scr3559, although scr3559 is the main transcript derived from the sco3558-sco3559 intergenic region as detected by RNA-seq (Vockenhuber et al., 2011; Moody et al., 2013). The function of scr3559 is unknown.
To elucidate whether Ms1 RNA is conserved in the phylogenetic group of Actinobacteria, we combined a homology search based on evolutionary conservation of RNAs with a bioinformatic linguistic search for genomic context (synteny) of RNA genes to identify Ms1 candidate genes. First, we applied this approach to Streptomyces coelicolor. Our search identified a Ms1 candidate—the scr3559 sRNA. We validated this result experimentally, demonstrating that scr3559 RNA binds the RNAP core without the primary σ factor HrdB in vivo and, thus, is an Ms1 but not a 6S RNA homolog. Subsequently, we applied our linguistic search to the phylum of Actinobacteria, revealing that Ms1 is present in most orders of Actinobacteria. Ms1 is thus a new type of regulatory RNA associated with RNA polymerase, in addition to 6S RNA, B2 RNA (regulates RNA polymerase II in humans) (Allen et al., 2004; Espinoza et al., 2004), or svRNAs (regulates the Influenza A virus RNA-dependent RNA polymerase) (Perez et al., 2010; Perez et al., 2012).
For the search, 10 selected Streptomyces well annotated genomes were used (GenBank assembly IDs shown in parenthesis following organism name): S. coelicolor A3(2) (GCA_000203835.1_ASM20383v1), S. ambofaciens ATCC 23877 (GCA_001267885.1_ASM126788v1), S. nodosus (GCA_000819545.1_ASM81954 v1), S. reticuli (GCA_001511815.1_TUE45), S. avermitilis MA 4680 (GCA_000009765.2_ASM976v2), S. griseus NBRC (GCA_000010605.1_ASM1060v1), S. scabies 87 22 (GCA_000091305.1_ ASM9130v1), S. cattleya NRRL8057 (GCA_000237305.1_ASM 23730v1), S. lincolnensis (GCA_001685355.1_ASM168535v1, S. pristinaespiralis ATCC 25486 (GCA_000154945.1_ASM 15494v1).
The search was performed in the following steps:
(i) Intergenic regions (IGRs) were identified in the 10 selected Streptomyces genomes, according to GenBank genomic annotations. IGRs in both DNA strands were searched, hence, an IGR in one strand may overlap with an open reading frame encoded by the other strand.
(ii) The IGR sequences were sampled with approximate nucleotide lengths of 180 and 300 nucleotides that are specific for 6S and Ms1 RNAs, respectively. The sampling was done in a sliding window, moving sequence window by 5 nucleotides a step through IGR sequences.
(iii) 10 suboptimal secondary structures were predicted for each sampled sequence by UNAfold (Markham and Zuker, 2008) with parameters P = 1000, W = 1 and X = 10.
(iv) The suboptimal structures were matched to secondary structures of B. subtilis 6S RNA (Trotochaud and Wassarman, 2005; Beckmann et al., 2012; Burenina et al., 2014) (for 6S RNA-length sequences) and M. smegmatis Ms1 RNA (Hnilicova et al., 2014) (for Ms1 RNA-length sequences) that were used as structural templates to get their pairwise structural similarity scores using RNAdistance (Lorenz et al., 2011).
(v) The average of three best scores for each sampled sequence was compared to the structural similarity thresholds of 80 and 135 for 6S RNA and Ms1 RNA, respectively. The thresholds were chosen such that we were able to identify known 6S and Ms1 RNAs by the search. To be considered as a candidate for one of the searched for sRNAs, the candidate sequence had to have the averaged similarity score of three most similar suboptimal structures better than the thresholds.
To increase reliability of the search, the candidates had to fulfill the following additional criteria derived from genomic properties of known 6S and Ms1 RNAs that were: (1) existence of similar sequences in evolutionarily close species identified by BLAST with BLAST E-values < 10–20, and (2) conserved genomic position in the ten searched Streptomyces genomes.
The full-length sequences were predicted using the approximate length of the transcripts detected by Northern blotting and the genomic positions of oligonucleotide sequences used for sRNA verification by Northern blotting (Figure 1). The sequences of approximate length were constructed using the oligonucleotides positioned at the 5′ end, center, and 3′ end. The three constructed sequences were BLASTed against genomes of the ten Streptomyces species listed in the previous paragraph. As sRNAs in general are conserved in evolutionarily related species, here in Streptomyces species, the constructed sequences had to be identified by BLAST in multiple Streptomyces species to ensure that they were constructed correctly. Using the superposition of constructed sequences according to their conservation identified by BLAST, we reconstructed probable sRNA sequences in Streptomyces coelicolor. The probable sequences are shown in Supplementary Table 1 for expressed Streptomyces sRNAs.
Figure 1. (A–F) Expression of predicted sRNAs. Total RNA was isolated from S. coelicolor at 35 (exponential phase of growth), 55 and 65 h after germination and the expression was detected by Northern blotting. 65 hours of growth represents stationary phase of growth. Orientation and the flanking genes are schematically shown for each sRNA. For as1791 and as1761 RNAs, fragments of sco1791 and sco1761 transcripts detected by Northern blotting are also shown in (A,B). For scr1506, 5′ ends was detected by 5′ RACE (G,H).
The search was based on text phrases in genomic annotations of the genes flanking potential Ms1 RNA genes and sequence similarity search based on BLAST for verification. The search was possible as sRNAs including Ms1 RNA are known to be conserved in evolutionarily close bacteria (Hnilicova et al., 2014). The search has several steps:
(i) Synteny analysis. Specific words in annotations of 5′ and 3′ end flanking genes of known and newly discovered IGR containing Ms1 RNAs are identified. Among them, most repeating specific words are identified. This step is explained in detail in the following section.
(ii) Synteny phrases. Text phrases, comprising of at least one most repeating specific word, that are specific for genomic annotation of genes flanking Ms1 RNAs are generated. Synteny phrases used in this work are shown in Tables 1–3.
(iii) Text search for Ms1 synteny phrases in genomic annotations of Actinobacteria genera which had >4 annotated genomes and in which Ms1 RNA has not yet been discovered. The search is implemented as a sequence of grep LINUX searches, followed by additional text processing implemented in MATLAB computational environment.
(iv) Synteny hit. An IGR with Ms1 synteny containing a putative Ms1 RNA gene.
(v) Evolutionary conservation of the synteny hit. The hit was considered as evolutionarily conserved when there existed IGRs with similar sequence and Ms1 synteny for at least one of the flanking genes.
The IGRs with similar sequences were identified by BLAST of the sequence of the synteny hit in NCBI’s nt database with sensitive setting for cross-species exploration (with parameters -r 1 -q 1 -G 1 -E 2 -W 7 (Korf et al., 2003) with BLAST E-value threshold set to 0.05).
Annotations of flanking genes of the identified IGRs were checked for Ms1 synteny phrases. The annotations contained either Ms1 phrases, or their synonyms, or contained new phrases. When contained new phrases, only one of the flanking genes was allowed to contain them and at the same time they had to repeat in annotations of multiple IGRs. Both new phrases and synonyms were used to update old phrases for the next synteny search iteration (Figure 5).
If the synteny hit was not found to be evolutionarily conserved, it was not used.
(vi) Flanking genes annotations of both synteny hit and IGRs homologous to it in new, related species. They were analyzed to get their specific words [step (ii)] and to update old synteny phrases [step (iii)].
Table 2. Synteny phrases for linguistic search after 1st update using synteny annotations of Streptomyces Ms1 RNA homologs (Supplementary Figure 2). For description of the table, see legend of Table 1.
Table 3. Synteny phrases for linguistic search after 2nd update using synteny annotations of Cellulomonas Ms1 RNA homologs (Supplementary Figure 3).
Figure 2. S. coelicolor Ms1 RNA candidate. (A) Histogram of relative genomic position of a Ms1 candidate, Str13, in 188 Streptomyces species. x-axis shows a relative genomic position with “0” and “1” corresponding to the terminal arms of the linear Streptomyces genome and with “0.5” corresponding to the middle of Streptomyces linear genome. y-axis shows percentage of 188 Streptomyces species in which Str13 homologs were identified using sequence similarity. The figure indicates that the Str13 relative genomic position is conserved in the middle of the linear Streptomyces genomes. (B) Ms1 candidate in S. coelicolor, Ms1 in M. smegmatis and MTS2823 Ms1 homolog in M. tuberculosis and their flanking genes. In Streptomyces coelicolor, positions of the previously published sRNAs are also included. The position of 6S-Like scr3559 was adopted from the 6S-Like scr3559 sequence reported in Figure 2B of Bobek et al. (2021). The position of ssrS/6S RNA was adopted from Mikulík et al. (2014), from the sequences of primers that were used to generate DNA template carrying T7 promoter for in vitro transcription of 6S RNA. Experiments showing 6S RNA—RNAP interaction were performed with this in vitro transcribed RNA. scr3559 position was adopted form available S. coelicolor dRNA-seq data (Romero et al., 2014; Jeong et al., 2016; Kim et al., 2020) and RNA-seq data (Vockenhuber et al., 2011; Moody et al., 2013). (C) ∼230 nt long RNA was detected by Northern blotting with the probe specific to Ms1 candidate/scr3559/(probe 2796). The 5′ end of Ms1 candidate was determined by 5′ RACE (D), 3′ end by 3′ RACE (E) and corresponds to the scr3559 sRNA (F). 6S RNA expression was not detected by Northern blotting (G). (H) Structure of scr3559. (I) Structure of Ms1 RNA from M. smegmatis.
Figure 3. Immunoprecipitation of S. coelicolor RNAP β and HA-HrdB (A–C) and M. smegmatis RNAP β (D). Lysates from S. coelicolor cells carrying HA-tagged HrdB (42 and 66 h after germination, exponential and stationary phase of growth, respectively) were incubated with antibodies against RNAP β, sigma 70 and HA tag and immunoprecipitated proteins were resolved on SDS PAGE and stained with Coomassie (A). RNA that co-immunoprecipitation with RNAP or HrdB was isolated, cDNA was reverse transcribed and the amount of Ms1 and 6S RNA candidates were determined by qRT-PCR (B,C). In S. coelicolor, 16S rRNA and RNAs expressed from sco3352, sco3710, and sco2713 genes were selected as controls that should not bind to RNAP/HrdB. In M. smegmatis, the amount of Ms1 associated with RNAP is shown as a positive control, RpoB and RpoC mRNAs do not co-immunoprecipitate with RNAP (D). The error bars show ±SEM from at least three independent experiments. The amount of RNAP β and HrdB was measured by western blotting in A3(2) hrdB-HA 42 h (exponential phase) and 66 h after germination (stationary phase) (E), the same amount of proteins (15 μg) was loaded.
Figure 4. Total RNA isolated from Mycobacterium smegmatis mc2 155, Corynebacterium glutamicum, Rhodococcus erythropolis CCM2595, Streptomyces coelicolor A3(2), and Bacillus subtilis from exponential (“ex”) and stationary (“st”) phase of growth, resolved on polyacrylamide gel electrophoresis and stained with GelRed. Ms1 RNAs in M. smegmatis and R. erythropolis and a band corresponding to the size of Str13/Ms1 in S. coelicolor are labelled with *. Two forms of B. subtilis 6S-1 RNA are marked by arrow.
Figure 5. Scheme of the linguistic gene synteny search for Ms1 RNA in Actinobacteria. Titles of analyzed data and information are in boxes, while analytic steps are shown as plain text. Arrows indicate the data and information flow. Synteny analysis (step i.) generates Synteny phrases (Tables 1–3, step ii.). For the first synteny analysis, annotations of Ms1 flanking genes from Mycobacterium, Rhodococcus, Nocardia, Gordonia, Mycobacteroides, Hoyosella, and Tsukamurella were used and Table 1 generated. Synteny phrases are used to search for new putative Ms1 candidates (iv. Synteny hits). If the putative Ms1 candidates are evolutionary conserved in related species (step v.), annotations of their flanking genes (step vi.) are added to the Synteny analysis (step i.) to generate updated Synteny phrases (step ii., Tables 2, 3) and the whole procedure is repeated.
Annotations of flanking genes were first split into single words and non-specific words were removed. The non-specific words were (allowing for grammar errors): ‘hypothetical’, ‘protein’, ‘type’, ‘family’, ‘domain’, ‘putative’, ‘precursor’, ‘component’, ‘subunit’, ‘subfamily’, ‘conserved’, ‘chain’, ‘or’, ‘superfamily’, ‘unknown’, ‘function’, ‘of’, ‘like’, ‘containing’, ‘II’, ‘and’, ‘short’, ‘dependent’, ‘probable’, ‘associated’, ‘to’, ‘that’, ‘the’, ‘predicted’, ‘uncharacterized’, ‘production’, ‘proteases’, ‘fold’, ‘in’, ‘by’, ‘universal’, ‘pathway’, ‘involved’, ‘related’, ‘general’, ‘group’, ‘sequence’, ‘class’, ‘cluster’, ‘accepting’, and ‘determining’.
We also removed the so-called questionable non-specific words whose (non)specificity depended on the analyzed context. They were: ‘box’, ‘enzyme’, ‘factor’, ‘secreted’, ‘release’, ‘neighborhood’, ‘solute’, ‘accessory’, ‘peptide’, ‘biosynthesis’, and ‘substrate’.
Specific words were left. They characterized specific aspects of gene function. An example is the ‘Fic/Doc family protein’ annotation contained two non-specific words, ‘family’ and ‘protein’, and one specific word ‘Fic/Doc’.
The first synteny phrases were obtained from annotations of homologs of M. smegmatis Ms1 RNA identified by sequence similarity by BLAST in 498 species of 8 genera—Mycobacterium, Mycolicibacterium, Rhodococcus, Nocardia, Gordonia, Mycobacteroides, Hoyosella, and Tsukamurella (Supplementary Figure 1). Specific words were selected as those occurring in annotations of flanking genes above the average (Supplementary Figure 1). The specific words were ‘oxidoreductase’, ‘hydrolase’, ‘HAD’, ‘IB’, ‘morphological’, ‘differentiation’, ‘inhibition’, ‘phosphoserine’ and ‘phosphatase’. The occurrence in genera was used rather than the occurrence in species as there were genera with many species with repeating annotations with repeating specific words causing bias of an occurrence of certain words.
Phrases were generated from the most frequent specific words according to their co-occurrence in the annotations from which they were extracted and which defined their semantic binding. For example, specific words ‘hydrolase’, ‘HAD’, ‘IB’, ‘morphological’, ‘differentiation’, ‘inhibition’, ‘phosphoserine’ and ‘phosphatase’ (identified in Supplementary Figure 1 as most occurring) formed the following phrases: ‘IB HAD’, ‘HAD hydrolase’, ‘inhibition morphological differentiation’ and ‘phosphoserine phosphatase’. A complete list of first phrases is in Table 1.
Synteny phrases were repeatedly updated after identification of Ms1-containing IGRs in new species (Supplementary Figures 2–4). New annotations either contained synonyms to old phrases, e.g., oxidoreductase, whose synonym was Fic that also has oxidoreductase activity or contained new annotation phrases. The synonyms were identified using information found on the internet, most often in Wikipedia and/or many various public protein databases/knowledgebases. New annotations were considered as Ms1-syntenous when they repeated for one flanking gene of newly identified Ms1 IGRs in many species, while annotation of the other flanking gene must have contained the known Ms1 phrase. New annotations were analyzed to get new phrases out of them that were merged with old phrases as can be seen in Tables 2, 3.
Mycobacterium smegmatis mc2 155 cells ATCC 700084 (wt, LK865) were grown at 37°C in Middlebrook 7H9 medium with 0.2% glycerol and 0.05% Tween 80, and harvested in exponential (OD600 ∼0.5) or early stationary phase (OD600 ∼2.5–3, 24 h of cultivation). S. coelicolor A3(2) spore stock expressing HA-tagged HrdB was thawed and inoculated to 2YT medium. Germination was carried out at 30°C for 5 h as described previously (Moody et al., 2013; Šmídová et al., 2019). The germinated spores were harvested by centrifugation (3200 × g, 25°C, 5 min), inoculated into Na-glutamate medium supplemented with trace element solution and TMS1 as described previously (Šmídová et al., 2019), cultivated at 30°C and harvested by centrifugation (3200 × g, 4°C, 5 min) at different time points after germination. Rhodococcus erythropolis CCM259 (Strnad et al., 2014) (LK1556) was cultivated at 26°C in 2x TY medium to exponential phase (OD600 ∼2) or stationary phase (OD600 ∼11, 24 h of cultivation), Corynebacterium glutamicum ATCC 13032 (LK1557) was grown in 2xTY at 30°C and harvested in exponential (OD600 ∼1) or stationary phase (OD600 ∼8, 24 h of cultivation). Bacillus subtilis 168 strain was grown in LB medium at 37°C to exponential phase (OD600 ∼0.3) or stationary phase (OD600 ∼4).
Each frozen cell pellet was resuspended in 240 μl TE (pH 8.0) plus 60 μl LETS buffer (50 mM Tris–HCl pH 8.0, 500 mM LiCl, 50 mM EDTA pH 8.0, 5% SDS) and 600 μl acidic phenol (pH∼3):chloroform (1:1). Lysates were sonicated in a fume hood, centrifuged, the aqueous phase extracted three more times with acidic phenol (pH∼3): chloroform and precipitated with ethanol. RNA was dissolved in water, treated with DNase (TURBO DNA-free Kit, Ambion) and visualized on a 7 M urea 7% polyacrylamide gel by staining with GelRed (Labmark).
RNAs were resolved on a 7% polyacrylamide gel and transferred onto an Amersham Hybond-N membrane according to the protocol described in Pánek et al. (2011). 5′ biotinylated oligonucleotide probes were hybridized to the membrane and detected with BrightStar BioDetect Kit (Ambion) according to manufacturer’s instructions. For Northern blot probes sequences, see the Supplementary Material.
The protocol was adopted from Martini et al. (2019). An adapter oligo CTGGAGCACGAGGACACTGACATGGACTG AAGGAGTagaaa (lower case letters are ribonucleotides, upper case letter deoxyribonucleotides) was ligated to RNA 5′ ends. 5 μg DNase-treated RNA was treated with RppH (NEB). Treated and untreated RNA samples (8 μl) were mixed with 1 μl of 1 μg/μl adapter oligo and incubated at 65°C for 10 min, then ligation reaction was set up including 10 μl 50% PEG8000, 3 μl 10X ligase buffer, 3 μl 10 mM ATP, 3 μl DMSO, 1 μl Murine RNase inhibitor (NEB), and 1 μl T4 ligase (NEB). Ligation was performed at 20°C overnight and RNA cleaned using RNA Clean and Concentrator 25 kit (Zymo). RNA was reverse-transcribed into cDNA (SuperScript III, Invitrogen) with random hexamers. PCR was done using a forward primer CTGGAGCACGAGGACACTGA and reverse (gene specific) primers (Str11, 5′-AGCCGCTCCCCTGGTCTGGG-3′, Ms1 candidate 5′-GGTGTCCATGCTCGGTCC-3′). The nucleotide position of the TSS was taken from EMBL/GenBank Accession No. AL645882.
The protocol was adopted from Sedlyarova et al. (2017). 550 pmol of 5′-phosphorylated RNA adaptor (5′P -AAUGGACUCGUAUCACACCCGACAA-3′) was ligated to 6 μg of total DNase treated RNA using T4 RNA Ligase 1 (ssRNA Ligase, New England Biolabs) according to the manufacturer’s protocol overnight at 16°C. RNA was purified with RNA Clean and Concentrator 25 kit (Zymo) and reverse-transcribed into cDNA (SuperScript III, Invitrogen) with 3′ RACE specific primer (5′-TTGTCGGGTGTGATACGAGTCCATT-3′). The same primer was used as reverse primer for PCR together with gene specific forward primer (5′- GATCACCTTAAACACGCATATGG-3′).
Streptomyces coelicolor cells expressing HA-tagged HrdB were pelleted and resuspended in lysis buffer (20 mM Tris–HCl pH 7.9, 150 mM KCl, 1 mM MgCl2, 1 mM dithiothreitol (DTT), 0.5 mM phenylmethylsulfonyl fluoride (PMSF), supplemented with Calbiochem Protease Inhibitor Cocktail Set III protease inhibitors), sonicated 15 × 10 s with 1 min pauses on ice and centrifuged. 500 μg (protein) of lysates were incubated for 16–18 h at 4°C with 20 μl of Protein G plus agarose beads (Santa Cruz) coated with 5 μg mouse monoclonal anti-β subunit of RNAP antibody [clone 8RB13] (BioLegend), 2.5 μg of anti-σ70 antibody [clone 2G10] (BioLegend), 1.25 μg of anti-HA antibody [clone HA-7] (Sigma-Aldrich) or 5 μg of mouse non-specific IgG (Sigma-Aldrich) used as a negative control, respectively. The captured complexes were washed 4 times using 20 mM Tris–HCl pH 7.9, 150 mM KCl, 1 mM MgCl2, finally resuspended in 300 μl 20 mM Tris–HCl pH 7.9, 150 mM KCl, 1 mM MgCl2 and divided into two parts. One third of the beads were incubated in SDS sample buffer for 5 min at 95°C and eluted proteins were detected by Coomassie staining and Western blotting. The remaining two thirds of the beads were resuspended in 200 μl 1% SDS, 150 mM KCl, 20 mM Tris–HCl pH 7.9, 1 mM MgCl2 and incubated on a rotating platform with 200 μl acidic phenol (pH∼3):chloroform (1:1) for 15 min. Eluted RNA was precipitated with ethanol, dissolved in water and DNase treated (TURBO DNA-free Kit, Ambion). RNA was reverse transcribed into cDNA (SuperScript III, Invitrogen) using random hexamers and amplified by quantitative reverse transcription PCR (RT-qPCR) in a LightCycler 480 System (Roche Applied Science) in duplicate reactions containing LightCycler 480 SYBR Green I Master and 0.5 μM primers (each). Primers were designed with Primer3 software and their sequences are in the Supplementary primer list. Negative controls (no template reactions and reactions with RNA as a template to control for contamination with genomic DNA) were run in each experiment, the quality of the PCR products was determined by dissociation curve analysis, and the efficiency of the primers determined by standard curves. The relative amounts of co-immunoprecipitated RNAs were quantified on the basis of the threshold cycle (Ct) for each PCR product that was normalized to input values according to the formula 2∧[Ct(immunoprec)–Ct(input)].
Proteins were detected by Western blotting using a rat monoclonal antibody recognizing the HA tag conjugated with HRP [clone BMG-3F10] or a mouse monoclonal antibody recognizing β subunit of RNA polymerase [clone 8RB13] in a combination with secondary antibody conjugated with HRP.
First, we conducted a computational homology search for putative 6S RNA/Ms1 RNA in the Streptomyces genus.
Using this computational search, we identified 12 candidate genes for 6S/Ms1 RNAs in Streptomyces (see Supplementary Material). Interestingly, flanking genes of one of them (Str11, Supplementary Table 1) displayed annotations syntenous to 6S-1 RNA in Firmicutes (Wehner et al., 2014), making it a prime candidate for Streptomyces 6S RNA. Nevertheless, none of the putative identified sRNA genes had the synteny of mycobacterial Ms1 RNAs. We suspected that the search might have not identified all candidate genes (for reasons see section “Discussion”). Therefore, prior to the experimental validation, we extended the homology search with linguistic synteny analysis approach.
The linguistic approach was based on search for text phrases that were specific to Ms1 RNA synteny and could be found in Ms1 flanking genes annotations. Note that meaning of the term ‘synteny’ in this work is ‘conserved genomic context’. Synteny annotations are annotations of conserved flanking genes of Ms1 RNAs. The phrases were used to identify new IGRs containing putative Ms1 RNAs in other species.
Synteny was rarely employed previously for identification of sRNAs (Sridhar and Gunasekaran, 2013), mostly because the sRNAs and their synteny occurred only in certain phyla, e.g., in Enterobacteriaceae (Sridhar and Rafi, 2007) and were not widespread in bacteria. Nevertheless, 6S RNA is an example of a sRNA identified throughout the bacterial kingdom with synteny conserved in specific taxons—for example in Enterobacteriaceae (γ-Proteobacteria) (Wehner et al., 2014). Although no 6S RNA syntenic pattern is valid for all bacteria, some proteins frequently occur in the syntenic regions of the 6S RNA throughout the bacterial kingdom. For example, ygfA, which encodes 5-formyltetrahydrofolate cyclo-ligase, is found adjacent to 6S RNA gene in α-, γ- Proteobacteria, and some species from β-Proteobacteria, δ-Proteobacteria, or Firmicutes (Barrick et al., 2005; Wehner et al., 2014). Therefore, as Ms1 and 6S RNAs are structurally and functionally similar, we assumed that Ms1 flanking genes would be at least partially conserved in Actinobacteria similarly to 6S RNA in γ-Proteobacteria.
Annotations of flanking genes of Ms1 RNAs identified previously in Mycobacterium, Rhodococcus, and Nocardia were conserved (Hnilicova et al., 2014). We speculated that the conservation would also be kept in those Actinobacteria where Ms1 RNA had not been identified. To verify this assumption, we first identified homologs of M. smegmatis Ms1 RNA by sequence similarity using BLAST in 498 species of 8 genera and analyzed annotations of their flanking genes. As expected, we found it conserved (Supplementary Figure 1). Therefore, we extracted synteny text phrases (Table 1) from the annotations and used them to search genomic annotations for their occurrence indicating putative Ms1 RNAs.
Intergenic regions with flanking genes with Ms1 RNA-specific synteny phrases (synteny hits) were found in numerous Streptomyces species for both ‘HAD hydrolase’ and ‘inhibition morphological differentiation’ phrases paired with the ‘oxidoreductase’ phrase. For example, in the first of the Streptomyces species, S. actuosus, a total of six hits of ‘HAD hydrolase’ were obtained. Only one of them fulfilled the other criteria of the synteny search, which was Ms1 synteny phrase (‘oxidoreductase’) in annotation of the other flanking gene and the length of IGR between flanking genes larger than 300 nucleotides. This IGR was considered as an IGR containing a putative Streptomyces Ms1 RNA.
The next criterion was an evolutionary conservation of the candidate Ms1 IGR in related species, i.e., in other Streptomyces species, analogously to Ms1 RNA from M. smegmatis conserved in other Mycobacteria. Therefore, the sequence of the S. actuosus Ms1 IGR was BLASTed against the NCBI nt database, which produced ∼250 BLAST hits in 188 different Streptomyces species (Supplementary Figure 6) with E-values < 1 × 10–24, i.e., strong sequence similarity and with a similar position in the middle of the linear Streptomyces chromosome (Figure 2A). Both sequence similarity and similar genomic loci indicated evolutionary conservation thus suggesting that the IGRs contained Streptomyces Ms1 RNAs.
Among the BLAST hits, a 419 nucleotides long IGR in S. coelicolor A3(2) (ENA ID AL645882.2) were identified at genomic locus 3934559: 3934978. To find out where within this IGR a putative Ms1 RNA was, the 419 nt sequence was sampled with 200–300 nt subsequences in 5 nt steps for which suboptimal secondary structures were predicted using UNAfold (Markham and Zuker, 2008) and compared to the secondary structure of M. smegmatis Ms1 RNA used as a structural template. This way we aimed at identification of a subsequence of the IGR able to adopt a Ms1 RNA-like secondary structure, thus identifying a local position of Ms1 RNA. The Ms1 RNA-like secondary structures were obtained with 220–235 nt subsequences at positions 126–141 downstream of 5′ end of the IGR sequence. Note that this sequence of the putative S. coelicolor Ms1 RNA had no similarity detectable by cross-species exploration BLAST to the sequence Ms1 RNA from M. smegmatis.
To summarize this part, we identified a total of 13 (12 + 1) potential 6S/Ms1 RNA candidate genes in S. coelicolor.
Next, we used Northern analysis to determine expression of these putative sRNA, probing their expression from both strands. The analysis revealed that several of them were expressed, to various degrees, in exponential and stationary phases in S. coelicolor (Figures 1A–F, 2C). Expression of the remaining six sRNAs was not detected. Genomic loci of the new sRNAs are depicted in Figure 1 and their basic characteristics are described in detail in the next two sections and summarized in Supplementary Table 1 (including predicted sequence, position in the S. coelicolor genome, annotations of flanking genes, and location at the chromosome in the Streptomyces genus).
Str1 sRNA is an antisense RNA (as1791, Figure 1A) to the tetratricopeptide repeat protein gene (sco1791) and it was found in two forms (90 and 120 nt). Additionally, for Str1 we detected a short transcript (100 nt) from the opposite strand, a fragment of the sco1791 (1260 nt) mRNA.
Str3 localizes to the sco1761 gene and similarly to Str1, Str3, is also an antisense RNA (130 nt, AS1711, Figure 1B). Furthermore, we also detected two short transcripts (110 and 80 nt, respectively) from the opposite strand, fragments of the 1086 nt long sco1761 mRNA. The antisense nature of both Str1 and Str3 sRNAs suggests that these sRNAs might be cis-acting antisense RNAs and the detected transcripts are fragments of the respective regulated mRNAs.
Str5 (scr5401, Figure 1C), Str8 (scr3567, Figure 1D), and Str10 (scr5145, Figure 1E) were expressed from intergenic regions and their lengths ranged from 120 to 130 nt.
We concluded that the identified genes encode bona fide sRNAs that are expressed in S. coelicolor but their short length (<150 nt) excluded them as potential 6S/Ms1 RNAs. 6S RNA/Ms1 RNA must adopt specific secondary structures and one of the shortest known 6S RNAs is from Aquifex aeolicus, which is ∼160 nt long (Köhler et al., 2015).
Str11 (scr1506), the candidate with the same synteny as 6S-1 RNA in B. subtilis, was long enough (∼220 nt long, Figure 1F) to be considered as a 6S RNA candidate. We identified the exact Str11 5′ end by 5′ RACE (Figures 1G,H). Str11 also had a 6S-like predicted consensus secondary structure (Supplementary Figure 5).
Str13, the Ms1 candidate identified by the linguistics search, partially overlaps with the previously discovered ssrS gene (Panek et al., 2008), which was proposed to encode a 192 nt long 6S RNA (Mikulík et al., 2014). The Str13 sequence also overlaps with the scr3559 sRNA identified by RNA-seq (Vockenhuber et al., 2011; Moody et al., 2013). In S. coelicolor, both scr3559 and ssrS are located between the sco3558 and sco3559 genes (Vockenhuber et al., 2011; Moody et al., 2013). The flanking genes and positions of Str13 in S. coelicolor and Ms1 in M. smegmatis and MTS2823 in M. tuberculosis are shown in Figure 2B; Arnvig et al. (2011), Hnilicova et al. (2014).
To start deciphering whether Str13 (Ms1 candidate) or ssrS (putative 6S RNA) is expressed from the sco3558 - sco3559 intergenic region, we performed Northern blot analysis. We used a probe that could hybridize to both Str13 and ssrS/6S RNA and we detected a signal that corresponded to a ∼230 nt RNA (Figure 2C). This could represent the previously reported 244 nt long ssrS/6S RNA unprocessed transcript (Mikulík et al., 2014). Although we also detected a shorter transcript (Figure 2C), the major isoform was the ∼230 nt long RNA and not the 192 nt ssrS/6S RNA. The size of the RNA detected by the Northern blot (∼230 nt) corresponded to the previously published lengths of scr3559: 235 bp (Vockenhuber et al., 2011) or 227 bp, respectively (Moody et al., 2013).
Next, we mapped the 5′ and 3′ ends of Str13 by 5′ RACE. The 5′ end was identified at position 3,934,693 (Figure 2D) that is 52 nucleotides downstream from the 5′end of the previously annotated ssrS/6S RNA (Mikulík et al., 2014; Figure 2F) and 134 nt downstream from 5′ end of IGR. This position agrees with the computationally predicted genomic locus of the putative S. coelicolor Ms1 (Str13) RNA - predicted Ms1 starts 126–141 nt downstream from 5′ end of IGR. This 5′ RACE result matches the 5′ end of scr3559 as determined by RNA-seq (Vockenhuber et al., 2011; Moody et al., 2013) and dRNA-seq (Romero et al., 2014; Jeong et al., 2016; Kim et al., 2020) in S. coelicolor. We also searched for additional transcription start sites in previously published data and found position 3,933,713 (Kim et al., 2020) that was 20 nucleotides upstream of the 5′ end of scr3559 but also did not correspond to the 5′ end of ssrS/6S RNA that is 52 nucleotides upstream. The 3′ end of Str13 was then determined by 3′ RACE (Figure 2E) at the position 3,934,920, which corresponds to the 3′ end of scr3559 (Vockenhuber et al., 2011; Moody et al., 2013) but not ssrS/6S RNA. The 3′ end in the same position (3,934,920) was identified also by Term-seq (Lee et al., 2020).
To determine whether scr3559, along with ssrS/6S RNA is perhaps expressed, we used three different probes for Northern blot analysis. Probe “A” should specifically hybridize to ssrS/6S RNA, probe “B” to both sr3559 and ssrS/6S RNA, and probe “C” only to the sr3559 (Figure 2F). We detected the ∼230 nt band only with probes B and C that hybridized to sr3559 (Figure 2G). No signal specific for ssrS/6S RNA was detected at 35 and 65 h after germination, indicating that the putative 6S RNA (ssrS gene) is not expressed in detectable amounts in these growth phases.
We cannot exclude that ssrS/6S RNA is expressed under unknown conditions but the main sRNA transcript derived from the sco3558-sco3559 genomic locus starts at position 3,934,693 and differs from the ssrS/6S RNA sequence that was used to experimentally test the interaction of the putative 6S RNA with the RNAP-HrdB holoenzyme in vitro (Mikulík et al., 2014).
As we identified the full sequence of the Ms1 candidate by 5′ and 3′ RACE, we used suboptimal structure folding to search for Ms1-like secondary structure motifs. We folded the Ms1 candidate sequence by UNAfold (with parameters P = 5000, W = 2 and X = 100) that predicted 78 suboptimal structures. The structures were clustered into 5 clusters based on their mutual structure similarity to find structurally representative folds. The clusters represented structural variations of a typical Ms1 fold. The most representative fold was identified in a cluster with most mutually similar suboptimal structures that contained typical Ms1-like structures which resembled Ms1 from M. smegmatis (Figure 2I), revealing its potential to interact with RNAP. An example of the secondary structure from that cluster is shown in Figure 2H.
We concluded that both Str11 (scr1506) and Str13 (scr3559) satisfied the criteria for potential 6S/Ms1 candidates and we selected them for further analysis.
To answer whether Str11 (scr1505) and/or Str13 (scr3559) are homologs of 6S RNAs or Ms1, we wanted to immunoprecipitate the primary σ factor, HrdB, and RNAP from S. coelicolor. As the commercially available antibody against the primary σ70 (clone 2G10) interacted with HrdB only weakly (Figure 3A, lanes 2 and 6), we used a strain with an HA-tagged S. coelicolor hrdB gene (Šmídová et al., 2019) and immunoprecipitated HA-HrdB with an anti-HA antibody from exponentially growing (42 h post-germination) and stationary (66 h post-germination) cells. The anti-HA antibody pulled down HA-HrdB and α, β, β′ subunits of RNAP, especially at 42 h post-germination (Figure 3A, lane 3, protein band identities were verified by mass spectrometry). Thus, the anti-HA antibody interacted both with HA-HrdB alone and also with the RNAP-HrdB complex, which binds 6S RNA in many bacterial species. Then, we immunoprecipitated RNAP with the antibody against the RNAP β subunit (Figure 3A lanes 1 and 5). This antibody preferentially recognizes the RNAP core without the primary σ factor (Figure 3A). We subsequently isolated co-immunoprecipitated RNAs and measured their relative amounts by RT-qPCR (Figures 3B,C).
Str11 (scr1505) associated neither with the RNAP core nor with the RNAP-HrdB holoenzyme, similar to four control RNAs that also did not interact with RNAP: 16S rRNA, sco3552, sco3710 encoding membrane proteins, and sco2013 encoding response regulator PdtaR.
Importantly, ∼2% of Str13 (scr3559) was bound to the RNAP core at 42 h post-germination in S. coelicolor (Figure 3B, the input represents the total amount of scr3559 isolated from the cell lysates), and it increased to ∼5% at 66 h post-germination (Figure 3C). scr3559 bound neither HrdB alone nor the HrdB-RNAP complex. As a control, we performed immunoprecipitation with the same antibody from stationary phase M. smegmatis cells and ∼6% of Ms1 co-immunoprecipitated with the RNAP core (Figure 3D). As we noticed that only a low amount of HrdB-HA was immunoprecipitated at 66 h post-germination (Figure 3A, lane 7) compared to 42 h (Figure 3A, lane 3), we compared the relative levels of HrdB-HA and RNAP in S. coelicolor. The amount of HrdB-HA significantly decreased at 66 h post-germination compared to 42 h (Figure 3E) while the level of the RNAP β subunit was almost unchanged. This suggests that the level of HrdB and subsequently, the level of the RNAP-HrdB complex is low in the late phase of growth in S. coelicolor, similar to previous observations in M. smegmatis (Hnilicova et al., 2014). Str13 (scr3559) was associated in vivo with the RNAP core but not with the HrdB-RNAP holoenzyme.
Therefore, we concluded that Str11 (scr1505) is neither 6S RNA nor Ms1 but a sRNA of unknown function. To the contrary, Str13 (scr3559) is a bona fide homolog of Ms1 in S. coelicolor and we propose to rename Str13 (scr3559) as Ms1.
In Mycobacterium smegmatis and Rhodococcus erythropolis, Ms1 is an abundant RNA in stationary phase, prominently visible in the gel, similarly to 6S RNA in Bacillus subtilis (Figure 4). In Streptomyces coelicolor, we detected a weak ∼230 nt long RNA visible in stationary phase RNA, which might be Ms1 RNA identified in this study. However, in Corynebacterium glutamicum, a species that is relatively evolutionarily close to Mycobacteria (both are in one order - Corynebacteriales), there are no prominent bands in the ∼200–300 nt range, suggesting that it might not contain an Ms1 RNA or its expression is below the detection limit of the staining. Therefore, we decided to extend the linguistic search to the whole group of Actinobacteria to reveal how widespread Ms1 RNA is within other Actinobacteria species.
After we identified S. coelicolor Ms1 RNA using the linguistic search, we applied it to other Actinobacteria. A flowchart of the search procedure is shown in Figure 5.
We started with the synteny phrase identified in Mycobacterium, Rhodococcus, Nocardia, Gordonia, Mycobacteroides, Hoyosella, and Tsukamurella (Table 1, step ii. in Figure 5) which had been used to discover Str13 (Ms1 homolog) in Streptomyces coelicolor.
For the search, Actinobacteria genera with more than four annotated species available in GenBank, according to NCBI Taxonomy (Schoch et al., 2020), were used. In total, there were 40 including Streptomyces. Synteny hits (step iv. in Figure 5) were obtained in five of them, namely in Cellulomonas, Williamsia, Actinospicaceae, Actinopolyspora, and Streptomyces (Str13). Note that sequences of the synteny hits, i.e., IGRs containing putative Ms1 RNAs, may be dissimilar to each other and therefore could not be identified by sequence similarity searches.
Based on sequence similarity to synteny hit from Streptomyces actuosus, we identified IGRs containing Ms1 in 158 species from 46 Actinobacteria genera other than Streptomyces (step v. in Figure 5). Based on occurrence of specific words from the annotations of the identified IGRs (Supplementary Figure 2 step vi. in Figure 5), we generated the new synteny phrases (step i. in Figure 5) and added them to the original phrases (this returned us to the step ii. in Figure 5 and new Table 2 was generated with updated synteny phrases).
Using the updated synteny phrases and the second iterative synteny search, a synteny hit in Cellulomonas gilvus was found. Based on sequence similarity, Ms1 IGRs in 708 species from 109 Actinobacteria genera were identified. Specific words and a histogram of their occurrence in synteny annotations of the 708 Ms1 IGRs are shown in Supplementary Figure 3. The subsequently updated synteny phrases are shown in Table 3.
The phrases in Table 3 yielded synteny hits into another three genera: Williamsia, Actinopolyspora, and Actinospica whose synteny annotations did not produce any new synteny phrases. Sequence similarity of the Williamsia synteny hit was limited to the Williamsia genus and sequence similarity of the both Actinopolyspora and Actinospicaceae synteny hits identified with mostly already known Ms1 RNA candidates in species of evolutionarily closed genera.
Interestingly, in Catenulispora (Catenulisporales), Brevibacterium (Micrococcales), Corynebacterium (Corynebacteriales), Actinomyces (Actinomycetales), and Bifidobacterium (Bifidobacteriales) we found no IGRs that could contain Ms1 RNA (Figure 5A, labeled in gray). We found IGRs with the Ms1 RNA synteny in the species of these taxonomic groups but they were too short to accommodate Ms1 RNA. For example, in Corynebacterium bovis, the Ms1-syntenous IGR was only 6 bps long and in species of the other groups there were Ms1 RNA-syntenous IGRs between only 20 and 100 nucleotides long.
In summary, the linguistics gene synteny search identified Ms1 RNA homologs in 824 Actinobacteria species (Supplementary Table 2 and Supplementary Figure 5 for specific words in their synteny annotations) belonging to 146 genera and 14 Actinobacteria orders (Figure 6A, labeled in red and Table 4). Thus, Ms1 RNA is widespread among Actinobacteria and Ms1 RNA interaction with the RNA polymerase core is conserved both in Mycobacterium smegmatis and Streptomyces coelicolor.
Figure 6. (A) Ms1 homologs were identified in Actinobacteria orders (in italics) or families (narrow italics) labeled by red. Actinobacteria groups with no identified Ms1 homologs and Ms1 flanking genes are in black, groups with identified Ms1 flanking genes but no Ms1 homologs in grey. The tree was adopted from Nouioui et al. (2018). (B) Genome sizes of the Corynebacteriales and (C) Actinobacteria. Only NCBI reference genomes are shown, number of genomes is indicated in brackets.
The presented study reveals the ubiquitous presence of Ms1 RNA in Actinobacteria (exceptions might exist, see below), identifying this sRNA as a major class of protein-interacting RNAs. Ms1 RNA associates with the RNAP core as previously demonstrated in Mycobacteria (Hnilicova et al., 2014) and here in Streptomyces (Figure 3). In addition, our linguistic gene synteny search proved to be a potent tool to identify sequentially unrelated RNAs in evolutionarily distant species.
We bioinformatically identified Str13 as the Ms1 RNA candidate in S. coelicolor. Str13 overlaps with scr3559 sRNA (Figure 2B), an sRNA with unknown function. We showed that Str13/scr3559 is an Ms1 homolog in S. coelicolor. Str13/scr3559 had a similar predicted secondary structure with the mycobacterial Ms1 (cf. Figures 2H,I) and both RNAs bind the RNAP core (Figure 3). Ms1 RNA is thus conserved in evolutionarily distant and morphologically divergent Mycobacterium and Streptomyces. Mycobacteria are unicellular rod-shaped bacteria, while Streptomyces have a complex life cycle, which starts with the germination of a spore that prolongs into filamentous tubes of highly branched vegetative (primary) mycelium, then secondary mycelium is formed and eventually spores (Trotochaud and Wassarman, 2004, 2006; Faucher et al., 2010; Cavanagh and Wassarman, 2013; Hoch et al., 2015). Despite the completely different life cycles, both bacterial species have maintained Ms1 sRNA.
In circular genomes of Mycobacterium, Nocardia, and Rhodococcus, Ms1 RNA is located close to the ori (replication start site) with the direction of transcription oriented toward it (Hnilicova et al., 2014). This is similar to Ms1 RNAs in Streptomyces that is positioned in the middle of the linear genome, close to ori (Figure 2A). The Streptomyces genomes have a core region of about 4.9 Mb containing essential genes and left and right arms with 1.5 Mb and 2.3 Mb, respectively (Hopwood, 2006), carrying mostly non-essential and species-specific genes. The position of Ms1 RNA genes in the core region indicates that this sRNA belongs among conserved genes in Streptomyces, consistent with our findings that identified Ms1 RNA candidates in 188 Streptomyces species (see Supplementary Figure 6). We further found Ms1 RNA in 145 other Actinobacteria genera (Table 4). Note that the number of newly identified Ms1 RNAs was limited by the availability of annotated Actinobacteria genomes as there were relatively many genera with only one or two genomes available or/and with a single species classified per genus.
In some genera, such as Corynebacterium, Bifidobacterium, and Actinomyces, we detected the Ms1 synteny but the IGR was too short to accommodate Ms1. The missing Ms1 RNA-containing IGR in Corynebacterium was consistent with the absence of a prominent band in the 200–300 nt range in RNA gels from Corynebacterium (Figure 4). The Corynebacterium genus belongs to the Corynebacteriales order, which also includes Mycobacterium, Nocardia, Rhodococcus [where Ms1 RNA has been already described (Hnilicova et al., 2014)], Williamsia, Gordonia, and Tsukamurella, where we identified Ms1 RNAs using the linguistic gene synteny search (Figure 6A). Within the Corynebacteriales order, Corynebacteria have the smallest genome (Figure 6B). Ms1 thus might have been lost from Corynebacterium due to the evolutionary pressure to maintain a reduced genome. Alternatively, Ms1 RNA could be essential for Actinobacteria with the larger genomes.
A comparison of genome sizes of the main Actinobacteria orders with the occurrence of predicted Ms1 RNAs (Figures 6A,C) reveals a trend where Ms1 is lost in bacteria with smaller genomes while the Ms1 synteny is still present. Examples are Bifidobacteriales and Actinomycetales where the respective IGRs were too short to accommodate Ms1 RNA; these orders have the smallest genomes within Actinobacteria (mostly <3.0 Mbp).
However, in Frankiales, Geodermatophilales, and Glycomycetales, where neither the Ms1 synteny nor Ms1 RNA were detected, genome sizes were comparable to Actinobacteria orders with identified Ms1 RNAs. Frankia (Frankiales) genome sizes vary between 4.3 and 10 Mb and this variability is due to the degree of host dependence. Frankia are N2-fixing filamentous plant symbiotic bacteria that can either survive independently in the soil or be entirely dependent on their host plants (Benson et al., 2011). The high diversity of Frankia genomes might be a reason why Ms1 RNA was not detected in our search (the gene synteny on which our search depends, might be too low in Frankia). Glycomycetales are aerobic bacteria that produce branched vegetative mycelia and aerial mycelia with chains of square-ended conidia (Labeda and Kroppenstedt, 2004). Glycomyces were isolated from soil, hypersaline habitats, and seawater (Han et al., 2014; Xing et al., 2014; Nikitina et al., 2020). Geodermatophilales create pigmented (very often black) colonies with the individual cubic cells and extracellular matrix forming cauliflower-like aggregates and have been reported to be highly resistant to stresses such as gamma-radiation, UV, and desiccation (Hezbri et al., 2016). Species from both Glycomycetales and Geodermatophilales can adapt to extreme stress conditions and thus might have evolved specific regulatory pathways independent of Ms1 RNA.
To summarize this section, the genome size is not the only indicator of the Ms1 RNA presence. Alternatively, Ms1 RNA may be present in these species but the Ms1 RNA gene synteny was lost and therefore we were unable to detect Ms1 RNA using our gene-synteny based approach.
The Ms1 RNA synteny itself, especially the “HAD hydrolase” gene is of interest. HAD hydrolase is annotated also as “inhibition of morphological differentiation protein” or “phosphoserine phosphatase” (see Tables 1–3) and it was found in the Ms1 RNA synteny of most species. In Mycobacterium smegmatis, HAD hydrolase is MSMEG_6173, a 293 amino acid long protein with a predicted transmembrane domain at its C-terminus. SCO3558, a 5′ end flanking gene of scr3559, that shares 60% identity with MSMEG_6173, is homologous to CicA (Bellier et al., 2006), which encodes a phosphotransferase in Caulobacter crescentus. An increased concentration of CicA in Caulobacter crescentus causes a loss of the normal rod shape, an almost 10-fold increase of the bacteria’s cell volume and a cell division block (Fuchs et al., 2001). As Ms1 regulates the RNAP amount in stationary phase (Sikova et al., 2019) it is tempting to speculate that the conserved association of the HAD hydrolase gene with the Ms1 RNA gene indicates a link between transcription regulation and cell size and shape.
From the perspective of computational biology, our work demonstrated limits of the use of secondary structure in computational homology searches for homologs of known sRNAs in bacterial genomes. Homology searches use similarity of secondary structure between potential homologs and known sRNAs either solely [e.g., (Pánek et al., 2011)] or in combination with sequence similarity [e.g., (Barquist et al., 2016)]. Structure similarity increases the efficiency and capability of these searches to find homologs as the sRNA secondary structures are more evolutionarily conserved than sRNA sequences.
Nevertheless, still the efficiency of homology searches decreases substantially with the increasing evolutionary distance. This was demonstrated here in the extremely diverse group of Actinobacteria by the search for Ms1 RNA homologs, which was not successful. Also Rfam (Kalvari et al., 2017), an RNA database that employs the infernal software (Barquist et al., 2016) for computational search for homologs of known RNAs, provides Ms1 RNA candidates only from species closely related to Mycobacteria.
A weak point of homology searches is the limited reliability of secondary RNA structure prediction, which decreases especially for sequences >100 nt. Furthermore, a correct RNA sequence, i.e., a sequence with both a correct position in the genome and a correct length, is required for the prediction. But it is not always available in the homology search as (1) the length of the sequence can vary substantially between species or genera, and (2) genomic position of the sequence can be only approximated. In the presented work, the length difference between the known M. smegmatis Ms1 RNA and the identified S. coelicolor Ms1 RNA homolog was 70 nucleotides and therefore predicted secondary structures of potential S. coelicolor Ms1 RNA homologs were wrong and structure similarity to known Ms1 RNA could not be detected. Thus, the unavailability of correct sequences of potential homologs could be another reason why homology search was not successful in the presented work.
To overcome this problem, we employed a genomic synteny search. We adopted a linguistics approach based on similarity of genome annotations rather than similarity of nucleotide or amino acid sequences of flanking genes. Ms1 RNA has conserved synteny with a highly specific phrase in one of its flanking genes, the ‘HAD IB hydrolase’. This phrase is relatively rare and occurs only a few times in well annotated genomes (e.g., 5 × in S. coelicolor). The annotation helped us to identify the IGRs that might contain Ms1 RNA even in extremely distant species as represented here by the Actinobacteria genera with predicted Ms1 RNA, in which flanking nucleotide/amino acid sequences might be dissimilar.
We designed the most parsimonious form of a linguistic search for conserved synteny based on text searches for exact words or phrases. Once the search had identified the first possible Ms1 homolog in a genus (synteny hit), it then proceeded in an iteratively progressive manner within the genus and also in evolutionarily close genera using sequence similarity of the synteny hits. The obtained information was subsequently applied to other genera, expanding the list of identified candidates. Even in this simple form, the computational text search was able to identify Ms1 homologs in Streptomyces and other distantly related Actinobacteria genera. Its versatility and ease of use make it a convenient tool that can be, in principle, applied to searches for other RNAs/genes, allowing their fast identification across a wide range of organisms.
The original contributions presented in the study are included in the article/Supplementary Material, further inquiries can be directed to the corresponding authors.
OM, MK, DK, and MJ validated sRNAs expressions. MŠ and JH performed 5′ and 3′ RACE. VVH and MŠ performed immunoprecipitation experiments. PH identified proteins by mass spectrometry. JP and MSc performed the computations. MSc, LK, JHn, and JP wrote the manuscript. JHn and JP designed the study. All authors contributed to the article and approved the submitted version.
This research was funded by the grants (20-07473S) to JH and (20-12109S) to LK from Czech Science Foundation (www.gacr.cz), ELIXIR CZ research infrastructure project (MEYS Grant No. LM2018131) including access to computing and storage facilities, and European Regional Development Fund (project BIOCEV CZ.1.05/1.1.00/02.0109).
The authors declare that the research was conducted in the absence of any commercial or financial relationships that could be construed as a potential conflict of interest.
All claims expressed in this article are solely those of the authors and do not necessarily represent those of their affiliated organizations, or those of the publisher, the editors and the reviewers. Any product that may be evaluated in this article, or claim that may be made by its manufacturer, is not guaranteed or endorsed by the publisher.
The Supplementary Material for this article can be found online at: https://www.frontiersin.org/articles/10.3389/fmicb.2022.848536/full#supplementary-material
Allen, T. A., Von Kaenel, S., Goodrich, J. A., and Kugel, J. F. (2004). The SINE-encoded mouse B2 RNA represses mRNA transcription in response to heat shock. Nat. Struct. Mol. Biol. 11, 816–821. doi: 10.1038/nsmb813
Arnvig, K. B., Comas, I., Thomson, N. R., Houghton, J., Boshoff, H. I., Croucher, N. J., et al. (2011). Sequence-based analysis uncovers an abundance of non-coding RNA in the total transcriptome of Mycobacterium tuberculosis. PLoS Pathog. 7:e1002342. doi: 10.1371/journal.ppat.1002342
Barquist, L., Burge, S. W., and Gardner, P. P. (2016). Studying RNA homology and conservation with infernal: from single sequences to RNA families. Curr. Protoc. Bioinformatics 54, 12.13.1–12.13.25. doi: 10.1002/cpbi.4.
Barrick, J. E., Sudarsan, N., Weinberg, Z., Ruzzo, W. L., and Breaker, R. R. (2005). 6S RNA is a widespread regulator of eubacterial RNA polymerase that resembles an open promoter. RNA 11, 774–784. doi: 10.1261/rna.7286705
Beckmann, B. M., Burenina, O. Y., Hoch, P. G., Kubareva, E. A., Sharma, C. M., and Hartmann, R. K. (2011). In vivo and in vitro analysis of 6S RNA-templated short transcripts in Bacillus subtilis. RNA Biol. 8, 839–849. doi: 10.4161/rna.8.5.16151
Beckmann, B. M., Hoch, P. G., Marz, M., Willkomm, D. K., Salas, M., and Hartmann, R. K. (2012). A pRNA-induced structural rearrangement triggers 6S-1 RNA release from RNA polymerase in Bacillus subtilis. EMBO J. 31, 1727–1738. doi: 10.1038/emboj.2012.23
Behra, P. R. K., Pettersson, B. M. F., Das, S., Dasgupta, S., and Kirsebom, L. A. (2019). Comparative genomics of Mycobacterium mucogenicum and Mycobacterium neoaurum clade members emphasizing tRNA and non-coding RNA. BMC Evol. Biol. 19:124. doi: 10.1186/s12862-019-1447-7
Bellier, A., Gominet, M., and Mazodier, P. (2006). Post-translational control of the Streptomyces lividans ClgR regulon by ClpP. Microbiology 152(Pt 4), 1021–1027. doi: 10.1099/mic.0.28564-0
Benson, D. R., Brooks, J. M., Huang, Y., Bickhart, D. M., and Mastronunzio, J. E. (2011). The biology of Frankia sp. strains in the post-genome era. Mol. Plant Microbe Interact. 24, 1310–1316. doi: 10.1094/MPMI-06-11-0150
Bentley, S. D., Chater, K. F., Cerdeno-Tarraga, A. M., Challis, G. L., Thomson, N. R., James, K. D., et al. (2002). Complete genome sequence of the model actinomycete Streptomyces coelicolor A3(2). Nature 417, 141–147. doi: 10.1038/417141a
Bobek, J., Mikulová, A., Šetinová, D., Elliot, M., and Çihák, M. (2021). 6S-Like scr3559 RNA affects development and antibiotic production in Streptomyces coelicolor. Microorganisms 9:2004. doi: 10.3390/microorganisms9102004
Brown, K. L., Wood, S., and Buttner, M. J. (1992). Isolation and characterization of the major vegetative RNA polymerase of Streptomyces coelicolor A3(2); renaturation of a sigma subunit using GroEL. Mol. Microbiol. 6, 1133–1139. doi: 10.1111/j.1365-2958.1992.tb01551.x
Burenina, O. Y., Elkina, D. A., Migur, A. Y., Oretskaya, T. S., Evguenieva-Hackenberg, E., Hartmann, R. K., et al. (2020). Similarities and differences between 6S RNAs from Bradyrhizobium japonicum and Sinorhizobium meliloti. J. Microbiol. 58, 945–956. doi: 10.1007/s12275-020-0283-1
Burenina, O. Y., Hoch, P. G., Damm, K., Salas, M., Zatsepin, T. S., Lechner, M., et al. (2014). Mechanistic comparison of Bacillus subtilis 6S-1 and 6S-2 RNAs–commonalities and differences. RNA 20, 348–359. doi: 10.1261/rna.042077.113
Cavanagh, A. T., Klocko, A. D., Liu, X., and Wassarman, K. M. (2008). Promoter specificity for 6S RNA regulation of transcription is determined by core promoter sequences and competition for region 4.2 of sigma70. Mol. Microbiol. 67, 1242–1256. doi: 10.1111/j.1365-2958.2008.06117.x
Cavanagh, A. T., Sperger, J. M., and Wassarman, K. M. (2012). Regulation of 6S RNA by pRNA synthesis is required for efficient recovery from stationary phase in E. coli and B. subtilis. Nucleic Acids Res 40, 2234–2246. doi: 10.1093/nar/gkr1003
Cavanagh, A. T., and Wassarman, K. M. (2013). 6S-1 RNA function leads to a delay in sporulation in Bacillus subtilis. J. Bacteriol. 195, 2079–2086. doi: 10.1128/JB.00050-13
Chen, J., Wassarman, K. M., Feng, S., Leon, K., Feklistov, A., Winkelman, J. T., et al. (2017). 6S RNA mimics B-form DNA to regulate Escherichia coli RNA polymerase. Mol. Cell 68, 388–397.e6. doi: 10.1016/j.molcel.2017.09.006
Elkina, D., Weber, L., Lechner, M., Burenina, O., Weisert, A., Kubareva, E., et al. (2017). 6S RNA in Rhodobacter sphaeroides: 6S RNA and pRNA transcript levels peak in late exponential phase and gene deletion causes a high salt stress phenotype. RNA Biol. 14, 1627–1637. doi: 10.1080/15476286.2017.1342933
Espinoza, C. A., Allen, T. A., Hieb, A. R., Kugel, J. F., and Goodrich, J. A. (2004). B2 RNA binds directly to RNA polymerase II to repress transcript synthesis. Nat. Struct. Mol. Biol. 11, 822–829. doi: 10.1038/nsmb812
Faucher, S. P., Friedlander, G., Livny, J., Margalit, H., and Shuman, H. A. (2010). Legionella pneumophila 6S RNA optimizes intracellular multiplication. Proc. Natl. Acad. Sci. U. S. A. 107, 7533–7538. doi: 10.1073/pnas.0911764107
Fraser, C. M., Gocayne, J. D., White, O., Adams, M. D., Clayton, R. A., Fleischmann, R. D., et al. (1995). The minimal gene complement of Mycoplasma genitalium. Science 270, 397–403. doi: 10.1126/science.270.5235.397
Fuchs, T., Wiget, P., Osterås, M., and Jenal, U. (2001). Precise amounts of a novel member of a phosphotransferase superfamily are essential for growth and normal morphology in Caulobacter crescentus. Mol. Microbiol. 39, 679–692. doi: 10.1046/j.1365-2958.2001.02238.x
Gomez, M., Doukhan, L., Nair, G., and Smith, I. (1998). sigA is an essential gene in Mycobacterium smegmatis. Mol. Microbiol. 29, 617–628. doi: 10.1046/j.1365-2958.1998.00960.x
Han, X. X., Luo, X. X., and Zhang, L. L. (2014). Glycomyces fuscus sp. nov. and Glycomyces albus sp. nov., actinomycetes isolated from a hypersaline habitat. Int. J. Syst. Evol. Microbiol. 64(Pt 7), 2437–2441. doi: 10.1099/ijs.0.061788-0
Hezbri, K., Ghodhbane-Gtari, F., Montero-Calasanz, M. D. C., Nouioui, I., Rohde, M., Spröer, C., et al. (2016). Geodermatophilus pulveris sp. nov., a gamma-radiation-resistant actinobacterium isolated from the Sahara desert. Int. J. Syst. Evol. Microbiol. 66, 3828–3834. doi: 10.1099/ijsem.0.001272
Hindley, J. (1967). Fractionation of 32P-labelled ribonucleic acids on polyacrylamide gels and their characterization by fingerprinting. J. Mol. Biol. 30, 125–136. doi: 10.1016/0022-2836(67)90248-3
Hnilicova, J., Matejckova, J. J., Sikova, M., Pospisil, J., Halada, P., Panek, J., et al. (2014). Ms1, a novel sRNA interacting with the RNA polymerase core in mycobacteria. Nucleic Acids Res. 42, 11763–11776. doi: 10.1093/nar/gku793
Hoch, P. G., Burenina, O. Y., Weber, M. H., Elkina, D. A., Nesterchuk, M. V., Sergiev, P. V., et al. (2015). Phenotypic characterization and complementation analysis of Bacillus subtilis 6S RNA single and double deletion mutants. Biochimie 117, 87–99. doi: 10.1016/j.biochi.2014.12.019
Hoch, P. G., Schlereth, J., Lechner, M., and Hartmann, R. K. (2016). Bacillus subtilis 6S-2 RNA serves as a template for short transcripts in vivo. RNA 22, 614–622. doi: 10.1261/rna.055616.115
Hopwood, D. A. (2006). Soil to genomics: the Streptomyces chromosome. Annu. Rev. Genet. 40, 1–23. doi: 10.1146/annurev.genet.40.110405.090639
Jeong, Y., Kim, J. N., Kim, M. W., Bucca, G., Cho, S., Yoon, Y. J., et al. (2016). The dynamic transcriptional and translational landscape of the model antibiotic producer Streptomyces coelicolor A3(2). Nat. Commun. 7:11605. doi: 10.1038/ncomms11605
Jones, A. J., Venkataramanan, K. P., and Papoutsakis, T. (2016). Overexpression of two stress-responsive, small, non-coding RNAs, 6S and tmRNA, imparts butanol tolerance in Clostridium acetobutylicum. FEMS Microbiol. Lett. 363:fnw063. doi: 10.1093/femsle/fnw063
Kalvari, I., Argasinska, J., Quinones-Olvera, N., Nawrocki, E. P., Rivas, E., Eddy, S. R., et al. (2017). Rfam 13.0: shifting to a genome-centric resource for non-coding RNA families. Nucleic Acids Res. 46, D335–D342. doi: 10.1093/nar/gkx1038
Kim, W., Hwang, S., Lee, N., Lee, Y., Cho, S., Palsson, B., et al. (2020). Transcriptome and translatome profiles of Streptomyces species in different growth phases. Sci. Data 7:138. doi: 10.1038/s41597-020-0476-9
Klocko, A. D., and Wassarman, K. M. (2009). 6S RNA binding to Esigma(70) requires a positively charged surface of sigma(70) region 4.2. Mol. Microbiol. 73, 152–164. doi: 10.1111/j.1365-2958.2009.06758.x
Köhler, K., Duchardt-Ferner, E., Lechner, M., Damm, K., Hoch, P. G., Salas, M., et al. (2015). Structural and mechanistic characterization of 6S RNA from the hyperthermophilic bacterium Aquifex aeolicus. Biochimie 117, 72–86. doi: 10.1016/j.biochi.2015.03.004
Labeda, D. P., and Kroppenstedt, R. M. (2004). Emended description of the genus Glycomyces and description of Glycomyces algeriensis sp. nov., Glycomyces arizonensis sp. nov. and Glycomyces lechevalierae sp. nov. Int. J. Syst. Evol. Microbiol. 54(Pt 6), 2343–2346. doi: 10.1099/ijs.0.63089-0
Lee, Y., Lee, N., Hwang, S., Kim, W., Jeong, Y., Cho, S., et al. (2020). Genome-scale determination of 5′ and 3′ boundaries of RNA transcripts in Streptomyces genomes. Sci. Data 7:436. doi: 10.1038/s41597-020-00775-w
Lorenz, R., Bernhart, S. H., Honer Zu Siederdissen, C., Tafer, H., Flamm, C., Stadler, P. F., et al. (2011). ViennaRNA Package 2.0. Algorithms Mol. Biol. 6:26. doi: 10.1186/1748-7188-6-26
Markham, N. R., and Zuker, M. (2008). UNAFold: software for nucleic acid folding and hybridization. Methods Mol. Biol. 453, 3–31. doi: 10.1007/978-1-60327-429-6_1
Martini, M. C., Zhou, Y., Sun, H., and Shell, S. S. (2019). Defining the transcriptional and post-transcriptional landscapes of. Front. Microbiol. 10:591. doi: 10.3389/fmicb.2019.00591
Mikulík, K., Bobek, J., Zídková, J., and Felsberg, J. (2014). 6S RNA modulates growth and antibiotic production in Streptomyces coelicolor. Appl. Microbiol. Biotechnol. 98, 7185–7197. doi: 10.1007/s00253-014-5806-4
Moody, M. J., Young, R. A., Jones, S. E., and Elliot, M. A. (2013). Comparative analysis of non-coding RNAs in the antibiotic-producing Streptomyces bacteria. BMC Genomics 14:558. doi: 10.1186/1471-2164-14-558
Neusser, T., Polen, T., Geissen, R., and Wagner, R. (2010). Depletion of the non-coding regulatory 6S RNA in E. coli causes a surprising reduction in the expression of the translation machinery. BMC Genomics 11:165. doi: 10.1186/1471-2164-11-165
Nikitina, E., Liu, S. W., Li, F. N., Buyantueva, L., Abidueva, E., and Sun, C. H. (2020). sp. nov., an actinobacterium isolated from steppe soil. Int. J. Syst. Evol. Microbiol. 70, 1356–1363. doi: 10.1099/ijsem.0.003923
Nouioui, I., Carro, L., García-López, M., Meier-Kolthoff, J. P., Woyke, T., Kyrpides, N. C., et al. (2018). Genome-based taxonomic classification of the phylum. Front. Microbiol. 9:2007. doi: 10.3389/fmicb.2018.02007
Panchapakesan, S. S., and Unrau, P. J. (2012). E. coli 6S RNA release from RNA polymerase requires σ70 ejection by scrunching and is orchestrated by a conserved RNA hairpin. RNA 18, 2251–2259. doi: 10.1261/rna.034785.112
Panek, J., Bobek, J., Mikulik, K., Basler, M., and Vohradsky, J. (2008). Biocomputational prediction of small non-coding RNAs in Streptomyces. BMC Genomics 9:217. doi: 10.1186/1471-2164-9-217
Pánek, J., Krásny, L., Bobek, J., Jezková, E., Korelusová, J., and Vohradsky, J. (2011). The suboptimal structures find the optimal RNAs: homology search for bacterial non-coding RNAs using suboptimal RNA structures. Nucleic Acids Res. 39, 3418–3426. doi: 10.1093/nar/gkq1186
Perez, J. T., Varble, A., Sachidanandam, R., Zlatev, I., Manoharan, M., García-Sastre, A., et al. (2010). tenOever: Influenza a virus-generated small RNAs regulate the switch from transcription to replication. Proc. Natl. Acad. Sci. U. S. A. 107, 11525–11530. doi: 10.1073/pnas.1001984107
Perez, J. T., Zlatev, I., Aggarwal, S., Subramanian, S., Sachidanandam, R., Kim, B., et al. (2012). tenOever: A small-RNA enhancer of viral polymerase activity. J. Virol. 86, 13475-13485. doi: 10.1128/JVI.02295-12
Rediger, A., Geissen, R., Steuten, B., Heilmann, B., Wagner, R., and Axmann, I. M. (2012). 6S RNA - an old issue became blue-green. Microbiology 158(Pt 10), 2480–2491. doi: 10.1099/mic.0.058958-0
Romero, D. A., Hasan, A. H., Lin, Y. F., Kime, L., Ruiz-Larrabeiti, O., Urem, M., et al. (2014). A comparison of key aspects of gene regulation in Streptomyces coelicolor and Escherichia coli using nucleotide-resolution transcription maps produced in parallel by global and differential RNA sequencing. Mol. Microbiol. 94, 963–987. doi: 10.1111/mmi.12810
Schoch, C. L., Ciufo, S., Domrachev, M., Hotton, C. L., Kannan, S., Khovanskaya, R., et al. (2020). NCBI Taxonomy: a comprehensive update on curation, resources and tools. Database 2020:baaa062. doi: 10.1093/database/baaa062
Sedlyarova, N., Rescheneder, P., Magán, A., Popitsch, N., Rziha, N., Bilusic, I., et al. (2017). Natural RNA polymerase aptamers regulate transcription in E. coli. Mol. Cell 67, 30–43.e6. doi: 10.1016/j.molcel.2017.05.025
Sharma, C. M., Hoffmann, S., Darfeuille, F., Reignier, J., Findeiss, S., Sittka, A., et al. (2010). The primary transcriptome of the major human pathogen Helicobacter pylori. Nature 464, 250–255. doi: 10.1038/nature08756
Shephard, L., Dobson, N., and Unrau, P. J. (2010). Binding and release of the 6S transcriptional control RNA. RNA 16, 885–892. doi: 10.1261/rna.2036210
Sikova, M., Janouskova, M., Ramaniuk, O., Palenikova, P., Pospisil, J., Bartl, P., et al. (2019). Ms1 RNA increases the amount of RNA polymerase in Mycobacterium smegmatis. Mol. Microbiol. 111, 354–372. doi: 10.1111/mmi.14159
Šmídová, K., Ziková, A., Pospíšil, J., Schwarz, M., Bobek, J., and Vohradsky, J. (2019). DNA mapping and kinetic modeling of the HrdB regulon in Streptomyces coelicolor. Nucleic Acids Res. 47, 621–633. doi: 10.1093/nar/gky1018
Sridhar, J., and Gunasekaran, P. (2013). Computational small RNA prediction in bacteria. Bioinform. Biol. Insights 7, 83–95. doi: 10.4137/BBI.S11213
Sridhar, J., and Rafi, Z. A. (2007). Small RNA identification in Enterobacteriaceae using synteny and genomic backbone retention. OMICS 11, 74–99. doi: 10.1089/omi.2006.0006
Steuten, B., Setny, P., Zacharias, M., and Wagner, R. (2013). Mapping the spatial neighborhood of the regulatory 6S RNA bound to Escherichia coli RNA polymerase holoenzyme. J. Mol. Biol. 425, 3649–3661. doi: 10.1016/j.jmb.2013.07.008
Strnad, H., Patek, M., Fousek, J., Szokol, J., Ulbrich, P., Nesvera, J., et al. (2014). Genome sequence of Rhodococcus erythropolis strain CCM2595, a phenol derivative-degrading bacterium. Genome Announc. 2:e00208-14. doi: 10.1128/genomeA.00208-14
Svensson, S. L., and Sharma, C. M. (2016). Small RNAs in bacterial virulence and communication. Microbiol. Spectr. 4, 169–212. doi: 10.1128/microbiolspec.VMBF-0028-2015
Trotochaud, A. E., and Wassarman, K. M. (2004). 6S RNA function enhances long-term cell survival. J. Bacteriol. 186, 4978–4985. doi: 10.1128/JB.186.15.4978-4985.2004
Trotochaud, A. E., and Wassarman, K. M. (2005). A highly conserved 6S RNA structure is required for regulation of transcription. Nat. Struct. Mol. Biol. 12, 313–319. doi: 10.1038/nsmb917
Trotochaud, A. E., and Wassarman, K. M. (2006). 6S RNA regulation of pspF transcription leads to altered cell survival at high pH. J. Bacteriol. 188, 3936–3943. doi: 10.1128/JB.00079-06
Vockenhuber, M. P., Sharma, C. M., Statt, M. G., Schmidt, D., Xu, Z., Dietrich, S., et al. (2011). Deep sequencing-based identification of small non-coding RNAs in Streptomyces coelicolor. RNA Biol. 8, 468–477. doi: 10.4161/rna.8.3.14421
Vogel, D. W., Hartmann, R. K., Struck, J. C., Ulbrich, N., and Erdmann, V. A. (1987). The sequence of the 6S RNA gene of Pseudomonas aeruginosa. Nucleic Acids Res. 15, 4583–4591. doi: 10.1093/nar/15.11.4583
Wassarman, K. M. (2018). 6S RNA, a global regulator of transcription. Microbiol. Spectr. 6: RWR-0019-2018 doi: 10.1128/microbiolspec.RWR-0019-2018
Wassarman, K. M., and Saecker, R. M. (2006). Synthesis-mediated release of a small RNA inhibitor of RNA polymerase. Science 314, 1601–1603. doi: 10.1126/science.1134830
Wassarman, K. M., and Storz, G. (2000). 6S RNA regulates E. coli RNA polymerase activity. Cell 101, 613–623. doi: 10.1016/s0092-8674(00)80873-9
Wehner, S., Damm, K., Hartmann, R. K., and Marz, M. (2014). Dissemination of 6S RNA among bacteria. RNA Biol. 11, 1467–1478. doi: 10.4161/rna.29894
Wurm, R., Neusser, T., and Wagner, R. (2010). 6S RNA-dependent inhibition of RNA polymerase is released by RNA-dependent synthesis of small de novo products. Biol. Chem. 391, 187–196. doi: 10.1515/BC.2010.018
Keywords: sRNA, Actinobacteria, Ms1 RNA, Streptomyces, gene synteny, Mycobacterium, 6S RNA
Citation: Vaňková Hausnerová V, Marvalová O, Šiková M, Shoman M, Havelková J, Kambová M, Janoušková M, Kumar D, Halada P, Schwarz M, Krásný L, Hnilicová J and Pánek J (2022) Ms1 RNA Interacts With the RNA Polymerase Core in Streptomyces coelicolor and Was Identified in Majority of Actinobacteria Using a Linguistic Gene Synteny Search. Front. Microbiol. 13:848536. doi: 10.3389/fmicb.2022.848536
Received: 04 January 2022; Accepted: 22 February 2022;
Published: 11 May 2022.
Edited by:
Damien Paul Devos, Andalusian Center for Development Biology (CSIC), SpainReviewed by:
Dagmara Jakimowicz, University of Wrocław, PolandCopyright © 2022 Vaňková Hausnerová, Marvalová, Šiková, Shoman, Havelková, Kambová, Janoušková, Kumar, Halada, Schwarz, Krásný, Hnilicová and Pánek. This is an open-access article distributed under the terms of the Creative Commons Attribution License (CC BY). The use, distribution or reproduction in other forums is permitted, provided the original author(s) and the copyright owner(s) are credited and that the original publication in this journal is cited, in accordance with accepted academic practice. No use, distribution or reproduction is permitted which does not comply with these terms.
*Correspondence: Jarmila Hnilicová, aG5pbGljb3ZhQGJpb21lZC5jYXMuY3o=; Josef Pánek, cGFuZWtAYmlvbWVkLmNhcy5jeg==
Disclaimer: All claims expressed in this article are solely those of the authors and do not necessarily represent those of their affiliated organizations, or those of the publisher, the editors and the reviewers. Any product that may be evaluated in this article or claim that may be made by its manufacturer is not guaranteed or endorsed by the publisher.
Research integrity at Frontiers
Learn more about the work of our research integrity team to safeguard the quality of each article we publish.