- 1Department of Parasitology, University of Agriculture, Faisalabad, Pakistan
- 2Department of Epidemiology and Public Health, University of Agriculture, Faisalabad, Pakistan
- 3Department of Clinical Medicine and Surgery, University of Agriculture, Faisalabad, Pakistan
- 4Institute of Microbiology, University of Agriculture, Faisalabad, Pakistan
- 5Section of Parasitology, Department of Pathobiology, KBCMA College of Veterinary and Animal Sciences Narowal, Lahore, Pakistan
- 6Department of Parasitology, University of Veterinary and Animal Sciences, Lahore, Pakistan
- 7Section of Parasitology, Department of Pathobiology, Riphah College of Veterinary Sciences, Riphah International University, Lahore, Pakistan
- 8Department of Pathobiology, University of the Poonch Rawalakot, Rawalakot, Pakistan
- 9Department of Parasitology, Cholistan University of Veterinary and Animal Sciences, Bahawalpur, Pakistan
- 10Medicine Section, Department of Clinical Sciences, Collège of Veterinary and Animal Sciences, Jhang, Pakistan
- 11University of Veterinary and Animal Sciences, Lahore, Pakistan
- 12Agricultural Linkages Program, Pakistan Agriculture Research Council, Islamabad, Pakistan
- 13Department of Zoology, University of Balochistan, Quetta, Pakistan
Ticks (Acari; Ixodidae) are the second most important vector for transmission of pathogens to humans, livestock, and wildlife. Ticks as vectors for viruses have been reported many times over the last 100 years. Tick-borne viruses (TBVs) belong to two orders (Bunyavirales and Mononegavirales) containing nine families (Bunyaviridae, Rhabdoviridae, Asfarviridae, Orthomyxovirida, Reoviridae, Flaviviridae, Phenuviridae, Nyamiviridae, and Nairoviridae). Among these TBVs, some are very pathogenic, causing huge mortality, and hence, deserve to be covered under the umbrella of one health. About 38 viral species are being transmitted by <10% of the tick species of the families Ixodidae and Argasidae. All TBVs are RNA viruses except for the African swine fever virus from the family Asfarviridae. Tick-borne viral diseases have also been classified as an emerging threat to public health and animals, especially in resource-poor communities of the developing world. Tick-host interaction plays an important role in the successful transmission of pathogens. The ticks' salivary glands are the main cellular machinery involved in the uptake, settlement, and multiplication of viruses, which are required for successful transmission into the final host. Furthermore, tick saliva also participates as an augmenting tool during the physiological process of transmission. Tick saliva is an important key element in the successful transmission of pathogens and contains different antimicrobial proteins, e.g., defensin, serine, proteases, and cement protein, which are key players in tick-virus interaction. While tick-virus interaction is a crucial factor in the propagation of tick-borne viral diseases, other factors (physiological, immunological, and gut flora) are also involved. Some immunological factors, e.g., toll-like receptors, scavenger receptors, Janus-kinase (JAK-STAT) pathway, and immunodeficiency (IMD) pathway are involved in tick-virus interaction by helping in virus assembly and acting to increase transmission. Ticks also harbor some endogenous viruses as internal microbial faunas, which also play a significant role in tick-virus interaction. Studies focusing on tick saliva and its role in pathogen transmission, tick feeding, and control of ticks using functional genomics all point toward solutions to this emerging threat. Information regarding tick-virus interaction is somewhat lacking; however, this information is necessary for a complete understanding of transmission TBVs and their persistence in nature. This review encompasses insight into the ecology and vectorial capacity of tick vectors, as well as our current understanding of the predisposing, enabling, precipitating, and reinforcing factors that influence TBV epidemics. The review explores the cellular, biochemical, and immunological tools which ensure and augment successful evading of the ticks' defense systems and transmission of the viruses to the final hosts at the virus-vector interface. The role of functional genomics, proteomics, and metabolomics in profiling tick-virus interaction is also discussed. This review is an initial attempt to comprehensively elaborate on the epidemiological determinants of TBVs with a focus on intra-vector physiological processes involved in the successful execution of the docking, uptake, settlement, replication, and transmission processes of arboviruses. This adds valuable data to the existing bank of knowledge for global stakeholders, policymakers, and the scientific community working to devise appropriate strategies to control ticks and TBVs.
Introduction
Pests are causing damage to our lives. Several organisms act as vectors and transmit or cause diseases in the agriculture sector, humans, wildlife, and livestock. Ticks (Acari: Ixodida) are blood-sucking ectoparasites and act as vectors of pathogens infecting livestock, wildlife, and humans across the world (Bente et al., 2013; Pfäffle et al., 2013; Guglielmone et al., 2014; Monfared et al., 2015; de la Fuente et al., 2016; Sajid et al., 2017; Ghafar et al., 2020). Ticks and tick-borne diseases (hereafter abbreviated as TBDs) are known to decrease production below the genetic potential of livestock (Sajid et al., 2007). Ticks are ectoparasites of a wide range of mammals, reptiles, and birds (Karim et al., 2017). Even if not infected with tick-borne diseases (TBDs), ticks are responsible for direct damage to the skin and hides, cause allergy, irritation, and toxicosis, and can lead to decreased livestock productivity (Sajid et al., 2007). Over 80% of the world's cattle population is affected by tick-transmitted pathogens that cause diseases called TBDs (De Meneghi et al., 2016; Rodriguez-Vivas et al., 2018). They are one of the major management issues in Africa and the Americas, and are particularly important in Asia because of hot and humid climatic conditions (Moming et al., 2018; Rosà et al., 2018). The typical life cycle involving questing and infesting stages, host diversity, and inappropriate micro and macro-management lead to the successful settlement of these arthropod vectors (Soulsby, 1982). In some countries (e.g., Turkey), ticks have adapted themselves to wild animals, such as spur-thighed tortoises, which can act as reservoirs of infestation for contiguous livestock populations (Uslu et al., 2019).
It is a proven fact that ticks act as biological vectors of a wide range of causative agents of protozoal (e.g., babesiosis and theileriosis), bacterial (ehrlichiosis, borreliosis, Rocky Mountain spotted fever, and Q fever), viral (e.g., Crimean Congo hemorrhagic fever, and Powassan), and rickettsial (e.g., anaplasmosis) diseases and Lyme disease (Dantas-Torres et al., 2012; Mccoy et al., 2013; Pfäffle et al.,2013; Gharbi and Aziz Darghouth, 2014; Ghosh and Nagar, 2014; Guglielmone et al., 2014; Pantchev et al., 2015; Solano-Gallego et al., 2016; Rashid et al., 2019; Siddique et al., 2020). Ticks are known to infest a wide range of hosts, including humans, livestock, pets, and wildlife, and are considered the second most widely used vector for disease transmission among arthropods on the planet (again behind mosquitoes) (Monfared et al., 2015). Almost 898 tick species are recognized, belonging to three different families: Argasidae (soft ticks, 194 species), Nuttalliellidae (intermediate, 1 species), and Ixodidae (hard ticks, 703 species) (Latif et al., 2012). Among these, the Ixodidae is the most diverse, abundant, and dominant tick family from a One Health significance (Tsatsaris et al., 2016). The prevalence of tick-borne diseases (TBDs) has increased recently because of several biotic and abiotic factors (Estrada-Peña and de la Fuente, 2014, 2018; Estrada-Peña et al., 2017; Martina et al., 2017). Thus, ticks are among the major contributing factors to lowered production and mortality, and are the basic reason for economic losses in livestock around the globe (Grisi et al., 2014).
Tick-Borne Viruses
Tick-borne viruses (TBVs), also known as tibo viruses, constitute various viruses that are transmitted successfully between two different environments. These are the host environment, the stable one, while the other is the opposite of stable, i.e., tick internal environment (Hubálek and Rudolf, 2012). Over time, the relationship between ticks and viruses has evolved. Consequently, the tick feeding cycle is synced with the viral life cycle, sculpting the evolution of tibo viruses (Sidorenko et al., 2021; Migné et al., 2022). Recent studies have illuminated the fact that tick cells undergo transcriptional changes while harboring viruses (Mansfield et al., 2017).
The history of TBVs links back to 1929, when the tick transmission of a flavivirus, the louping ill virus, accountable for encephalitis in sheep, was discovered (Bichaud et al., 2014; Shi et al., 2018). Later, in 1945, another TBV, the Crimean Congo virus, was confirmed in Soviet soldiers and local inhabitants of the Crimean Peninsula of the USSR (Zivcec et al., 2016). This led to a path for the subsequent discovery of heterogenous TBVs falling under two orders, Bunyavirales and Mononegavirales. These are divided into one DNA virus family (Asfarviridae) and eight RNA virus families (Bunyaviridae, Rhabdoviridae, Orthomyxoviridae, Reoviridae, Flaviviridae, Phenuviridae, Nyamiviridae, and Nairoviridae) (Nuttall, 2013).
In the last decade, there was an emergence or re-emergence of tick-borne encephalitis virus that jeopardized public and animal health. There have been reports of TBVs in new geographical locations, a rise in several specific diseases, e.g., Possowan virus in America, and the occurrence of novel viruses, such as the Alkhurma virus, (a subtype of Kyasanur forest disease virus) (Burthe et al., 2020; Madani and Abuelzein, 2021; Yang et al., 2022), and deer tick virus (a subtype of POWV) (Hermance and Thangamani, 2018). These new viruses are placed in different families based on the latest molecular diagnostic techniques, resulting in major changes made in the families Bunyaviridae and Rhabdoviridae (Kazimírová et al., 2017) (Figure 1).
Morphology Of Tick Salivary Glands
During tick-host interaction, host tissues (blood) and tick saliva pass through a common buccal canal. Tick saliva originates from branched and paired alveolar salivary glands, which are present anterolaterally and extend into the posterior sides of the body. From each salivary gland, a duct originates that enters a broad shallow tube, i.e., salivarium. This tube lies above the pharynx in the pharyngeal region valve and opens into the food canal at the anterior opening of the pharynx (Kemp and Tatchell, 1971).
Anatomy Of Tick Salivary Glands Of Ixodidae
Complex alveoli are present in the Ixodid ticks' salivary glands, which are variable in numbers in female and male ticks, i.e., three in females and four in males (Balashov, 1972; Krolak et al., 1982; Barker et al., 1984).
Type 1 Alveoli
The anterior region of the main salivary duct is attached with type 1 alveoli and may extend toward the posterior branches of the main duct. Type I alveoli contain granular cells.
Type 2 Alveoli
Morphologically, types 2 and 3 alveoli are similar, and type 2 contains six types of granular cells, i.e., a,b, C-C4, which form reactions during staining procedures (Binnington, 1978). The morphology of an alveolus undergoes remarkable changes after several days of tick feeding but a change in number has not been reported. Enlargement of nuclei and the cytoplasm occurs, which, in turn, causes an increase in the overall mass of the alveolus. Along with this, abluminal interstitial cell enlargement also occurs, which forms a basal labyrinth during the feeding procedure. The granular materials present in the salivary glands of unfed ticks in the early feeding stage are absent during their final feeding stages. In the case of Ixodes (I.) holocyclus, type 2 female alveoli contain only two granular cell types (Šimo et al., 2013).
Type 3 Alveoli
The most abundant type of alveoli in ticks' salivary glands are type 3 alveoli, which contain three granular cell types (d, e, and f), along with some granular cells. Type 3 alveoli are located in the posterior and peripheral regions of the glands (Binnington, 1978). The f cells of type 3 alveoli undergo cell transformation during the feeding process. The proliferation of cell plasma membrane occurs, and hypertrophy of abluminal interstitial cells and an increase in the number of mitochondria result in the formation of the basal labyrinth. Transformed f cells form a complex mass of plasma membranes and mitochondria, which is a common feature for fluid-transporting epithelia (Fawcett et al., 1986). An increase in the size of type 3 alveoli suggests that the transportation of the bulk of excreted fluid has taken place during the feeding process. In male ticks, the development of abluminal cells and f-cells occurs to a lesser degree than in female ticks (Coons and Lamoreaux, 1986). Only one common ad-luminal cell is present in types 2 and 3 cells that line the alveolus lumen in a web-like fashion (Labuda et al., 1993). In type 3 alveoli, the main function of ad-luminal cells is the same as that performed by myoepithelial cells, i.e., expansion of fluid-filled alveolus causing the ejection of fluid from the lumen to the salivary ducts and then outside the ducts (Kim et al., 2014).
Type 4 Alveoli
Type 4 alveoli are only present in males along with abluminal and ad-luminal cells (Binnington, 1978). Only one granular cell type is present in type 4 alveoli, i.e.g., which is filled with secretion granules during the feeding process (Fawcett et al., 1986).
Anatomy Of Tick Salivary Glands Of Argasidae
Argasids or soft ticks' salivary glands are less complex as compared to those of hard ticks and contain only two types of alveolar acini i.e., I and II (El Shoura, 1987). In soft ticks, coxal organs are involved in fluid secretion instead of salivary glands, and the change in salivary gland morphology during feeding is minor as compared to Ixodidae (Kaufman and Sauer, 1982).
Type 1 Alveolar Acini
Type I alveolar acini are connected to the anterior region of the main salivary duct through short alveolar ducts. Cell types and ducts present in argasid type 1 alveoli are like Ixodid type 1 alveoli (El Shoura, 1987).
Type 2 Alveolar Acini
In the case of type 2 alveoli, three granular cells are present, i.e., a, b, and c. The fourth type of cell, d, is also reported in Ornithodoros (O.) savignyi (Mans et al., 2004). The lumen of alveoli leads to a chitinous alveolar duct, which lacks a complex valvular structure compared to ixodid alveoli (Roshdy and Coons, 1975). Canaliculi formation occurs in argasid ticks but not to the extent observed in type III alveoli of Ixodid ticks (El Shoura, 1987).
Development And Degeneration Of Salivary Glands
In newly hatched larvae, extremely small salivary glands are present, and only ducts are distinguishable. Few alveoli begin to develop in older Ixodid larvae, e.g., types 1, 2, and 3 alveoli are observed in larvae of Haemaphysalis (Hae.) spinigera. Type 4 alveoli are not distinguishable in larval stages (Ullah and Kaufman, 2014). The size of salivary glands enlarges during the larval feeding process, and when engorged larvae drop from the host, degeneration of salivary gland alveoli occurs. However, salivary ducts form branch ducts that terminate in small alveoli containing undifferentiated cells (Nodari et al., 2012). Salivary alveoli continue to increase in numbers and differentiate into types 1, 2, and 3 alveoli in nymphal stages, while fully differentiated types of salivary gland alveoli are present in molted adults (Esteves et al., 2017).
During tick feeding, a 25-fold increase in mass, protein content (stimulation of mRNA synthesis and new protein expression), and size of salivary glands takes place (Tirloni et al., 2017). Further development of salivary glands occurs after the mating process, and mRNA and protein synthesis rates become double during this phase. In mated rapid-feeding female ticks, higher ATPase, Na+, K+, and adenylate cyclaseactivities and fluid secretion rate are present (Yu et al., 2017). Different hemolymph-borne factors are involved in controlling these changes in salivary glands during feeding and mating phases, e.g., an applied juvenile hormone partially stimulates protein synthesis and ATPase, Na+- and K+- activity in Amblyomma (A.) americanum (Kim et al., 2016).
Composition And Function Of Tick Saliva
Saliva is mostly water-derived during the blood meal process and, as in the Ixodid group, most of the blood meal is taken during the last 12–24 h of feeding, so the excess amount of saliva is produced during the final engorgement stage (Šimo et al., 2014). About 1-ml volume of saliva is secreted by large tick species (Koči et al., 2014). During blood feeding, extra water is secreted, so ions are balanced by secreting hypo-stomatic saliva containing 70% water and ions taken up during blood-feeding (Kim et al., 2017).
Cement
The secure attachment of ticks to the host body is due to cement proteins, which result in a prolonged feeding period (Suppan et al., 2018). In the case of soft ticks (fast feeder), adults, and nymphal stags penetrate deep into the skin of the host and make some strong unnecessary attachment, while in hard ticks, all slow Ixodid feeders use cement and enhance protein production to ensure secure attachment by enlarging tick-host association. Types 2 and 3 alveolus cells d and e are involved in cement production (Mans and Neitz, 2004). Cement protein contains some lipid and glycol proteins (Leal et al., 2018). A recent proteomic analysis of a cement cone has provided information regarding the presence of metalloproteases and serine protease inhibitors in A. americanum (Bullard et al., 2016). Cement proteins also have antigenic properties and contain a 90-kDa polypeptide in d and e cells of type 3 alveolus cells, and some of them can be considered potent anti-tick vaccine candidates (Suppan et al., 2018). When a tick attaches to the surface of the host, the cement protein forms a cement cone around tick mouthparts and allows for firm attachment to the host's skin and protection against the host's immune system. A cement cone is also involved in playing an antibacterial role (Suppan et al., 2018). Cement cone production is a specialty of the Ixodid group and differs in composition, size, and shape among the members of this group (Leal et al., 2018). Glycine-rich proteins, such as those found in cement cones, are normally biologically inert and non-immunogenic; however, the use of cement proteins as a vaccine protects mice from lethal tick-borne encephalitis virus. These glycine-rich proteins also play a role in tick embryo development (Suppan et al., 2018).
Enzymes And Enzyme Inhibitors Present In Tick Saliva
Tick saliva is a colorless, hypertonic, alkaline substance. Histochemical analysis of tick saliva has reported the presence of triacylglycerol, aminopeptidase, carboxylic ester hydrolases, and lipases (Bullard et al., 2016). Transcriptomic studies have revealed more than 500 different proteins and peptides further divided into different multigene groups, i.e., metalloproteases, lipocalins, Kunitz-domain proteins, some unique proteins found only in ticks, and some ancestral protein families that are present in Ixodid, argasid, and Nutailella (Mans et al., 2016). Esterases are also found in larvae of B.microplus causing a hypersensitivity reaction in cattle that are previously exposed to ticks; they hydrolyze cholesterol esters in the mast cell membrane, resulting in increased vascular permeability and release of pharmacologically active compounds, i.e., hyaluronidase. The kininase present in tick saliva deactivates the action of bradykinin (a pain-causing mediator) at the feeding site and enhances the tick feeding process (Mulenga et al., 2013).
Members of the Ixodid group contain proteins that inhibit the production of proteolytic enzymes, i.e., plasmin, trypsin, porcine kallikrein, and chymotrypsin (Štibrániová et al., 2013). The metalloprotease proteins of ticks have been identified in saliva, ovary, and midgut, and play an important role in tick blood uptake, vitellogenesis, blood digestion, and innate immunity (Kotál et al., 2015a). Metalloproteases have been identified in diverse tick species, e.g., A. maculatum, Rhipicephalus (R.) samguneus, I. scapularis, R. microplus, I. ricinus, and A. americanum (Ali et al., 2015a,b; Chmelar et al., 2016a). Ticks contain several protease inhibitors that play a role in tick-host interactions (Chmelar et al., 2016b). Four major groups of protease inhibitors are present in ticks: trypsin inhibitors, serpins, and Kazal domain and Kunitz domain cysteine protease inhibitors (Parizi et al., 2018).
Serine protease inhibitors are involved in the production of antimicrobial peptides, digestion of blood, and innate immunity (Meekins et al., 2017). Serine protease inhibitors also bind with different protease and non-protease ligands, including maspin, nexin-1, kallistatin, and anti-chymotrypsin. Different tick species were screened for serine protease inhibitors and reported in I. ricinus, D. variabilis, R. microplus, I. scapularis, A. variegatum, Hae. logicorns, A. americanum, R. appendiculatus, and A. hebraeum (Kim et al., 2014). Characterization of some serine protease inhibitors has been conducted, and they were found to play a role in host defense mechanisms through pro-inflammatory cytokine production (Wikel, 2013; Valdés, 2014).
Another large group of tick protease inhibitors is that of cystatins, which modulate vertebrate biological processes, i.e., immunity, antigen presentation, phagocytosis, apoptosis, and hemoglobin digestion and regulation (Chmelar et al., 2016b). The cystatin group is further divided into four subgroups, i.e., types 1, 2, 3, and 4 cystatins (Kazimírová and Štibrániová, 2013). In ticks, types 1 and 2 cystatins are present, and type 1 was first isolated from R. microplus causing inhibition of vitelline by degrading the cystatin endopeptidase, and plays an immunomodulatory role (Schwarz et al., 2012). Different cystatins are reported on various tick species, i.e., Hae. longicornis, A. americanum, I. ovatus, I. scapularis, and R. microplus, and on a soft tick, Orintodoros moubata (Chmelar et al., 2017). Type 2 cystatins were characterized in I. scapularis and play an important role in the transmission of tick-borne pathogens by interfering with interferon-mediated immune responses and increasing the multiplication of tick-borne viruses in bone marrow dendritic cells (Chen et al., 2014). Various other cystatin forms are characterized by tick species, and interference with their actions results in the impaired feeding process, higher mortality, immunosuppressive effects, and block attachment, and are promising anti-tick vaccine candidates (Kotsyfakis et al., 2008; Chmelar et al., 2017).
Prostaglandins
Prostaglandins have been identified in tick saliva and possess anti-inflammatory, hyperemic, and immunosuppressive activities (Chmelar et al., 2012). Different forms of prostaglandins are identified in ticks' saliva, i.e., F2α, A2/B2, prostacyclin (I2), and D2; among these, A2/B2 is considered to be derived from PGE2 because of the alkaline nature of tick saliva (Carvalho-Costa et al., 2015). Arachidonic acid and its derivatives act as precursors for salivary gland prostaglandins, e.g., endocannabinoids in A. americanum. Arachidonic acid is 8% of total fatty acids in partially fed tick salivary glands compared to the 2% in unfed ticks and increases more than any other fatty acid (Gao et al., 2016). Most vertebrates are capable of synthesizing arachidonic acid from linoleate by desaturation and elongation reactions, but in the case of ticks, females have the capability to synthesize degenerative fatty acids, but desaturation ability is lacking in ticks, and the stearoyl CoA desaturase gene is present in ticks. Phospholipase PLA2 activity is related to arachidonic acid release. Dopamine was also found associated with an increase in free arachidonic acid by stimulating PLA2 by the opening of voltage-dependent Ca2+ channel (Kannangara and Patel, 2018).
Exosomes And Known Unknowns
Tick saliva also contains some exosomes, i.e., microRNA (miRNA) in the Ixodid species, and is involved in change in exosomal origin (Díaz-Martín et al., 2015; Hackenberg et al., 2017; Rodriguez et al., 2018). A transcriptomics analysis revealed the presence of some known unknowns in tick saliva, e.g., in D. andersoni, 677 proteins are identified, and out of this, 80% are of unknown function (Mudenda et al., 2014). It is also probable that tick saliva also contains some unknown unknowns, which are likely to reveal further interesting information regarding the saliva of ticks.
The Function Of Tick Saliva In Controlling Host Response
When a tick bites, it causes activation of coagulation factor XII and bradykinin release, which cause host pain sensation; however Ixodid ticks destroy the bradykinin bymetalloprotease enzyme angiotensin-converting enzyme (ACE) (Chmelar et al., 2012). Two types of ACE have been identified in A. maculatum (Jelinski, 2016). Ticks also use lipid mediators (endocannabinoids) as an analgesic to hide their presence, and, along with this, other analgesic mediators are found: adenosine and miRNA (Hackenberg et al., 2017).
Vasoconstriction is another phenomenon that occurs during tick bite, and Ixodid ticks use prostaglandins and adenosine as counter agents against vasoconstriction (Chmelar et al., 2012). Other proteins involved in vasoconstriction are apyrase, serotonin-binding salivary proteins, histamine-binding proteins, and phenylalanine-rich peptides, which may modulate vascular permeability (Pekáriková et al., 2015).
Platelet aggregation and activation are controlled during tick bite, by the tick releasing, via the saliva, apyrases, thrombin inhibitors, arginine-glycine-aspartate motif, and serotonin binders, which break down the platelet activation agonist ADP, damage the cells, and, ultimately, neutralize the platelet aggregation agonist (Tang et al., 2015; Yun et al., 2016). After platelet plug formation, the secondary phase of hemostasis occurs, i.e., coagulation factor assembly, which leads to fibrin plug formation (Palta et al., 2014). Tick saliva contains serine protease inhibitors as anti-coagulants which target the thrombin and coagulation factor FXa (Blisnick et al., 2017) along with these Kunitz domains also contain thrombin inhibitors e.g., O. moubata contain ornithodorin, variegin in A. variegatum, Salp 14 in I. scapularis saliva, and ixonexin (tail peptide) in I. scapularis (Thompson et al., 2017; Assumpção et al., 2018). This multi-targeted approach by ticks allows for successful control of the coagulation process by interacting with the host coagulation cascade.
Mast cells containing pro-inflammatory compounds are present in abundance in host skin and act as the first line of defense against ticks (Wernersson and Pejler, 2014). When ticks attach to host skin, they activate mast cells, which results in degranulation, and the release of their contents into the extracellular environment, starting bioactive compound de novo synthesis. Ticks use lipocalins, Kunitz-type proteins, to control histamine, serotonin, and tryptase activity, stabilize mast cells, and prevent de novo synthesis (Schuijt et al., 2013). Sialostatin L targets IRF-4-dependent transcription in mast cells, resulting in interleukin-9 suppression (Klein et al., 2015). Pro-inflammatory cytokines (i.e., IL-1, IL-6, IL-8, and TNF) are produced by the host, and ticks inhibit these cytokines by capturing the ligand using cytokine binders called evasions, and about 265 evasions have been identified in different tick genera (Hayward et al., 2018). In A. variegatum, the salivary peptide amphiregulin inhibits cytokine production (Tian et al., 2016). The complement system is the main trigger for inflammation, and about 40 proteins take part in this phenomenon. During tick bite, the complement system is inhibited by the different complement inhibitors present in tick saliva that is produced during feeding and stored in the granular acini (Jore et al., 2016; Perner et al., 2018).
Ticks can control host immune responses by producing immunomodulators that target the host acquired and innate immune system (Kotál et al., 2015a; Wikel, 2018a). In R. appendiculatus, 64 TRP proteins cross-react with epitopes in tick midgut (Kotál et al., 2015b; Chmelar et al., 2016b). Dendritic cells are known as immune sentinels and sense danger and send information to other immune cells and contribute to both adaptive and innate immunities (Heath and Carbone, 2013; Austyn, 2017). In metastriate Ixodid ticks, lipocalin proteins are available that target dendritic cells, e.g., japanin (Preston et al., 2013), while in prostriate ticks, the sialostatin L group, cystatin protease inhibitors, are available for dendritic cell control, e.g., Salp 15 that inhibits CD4+ T cell and dendritic cell activation (Carvalho-Costa et al., 2015; Kotál et al., 2015b; Tomás-Cortázar et al., 2017). Regulatory T cells are also controlled by Salp 15 by the production of immunosuppressants, e.g., adenosine (Tomás-Cortázar et al., 2017). Salp 15 also affects the ability of B cells to produce antigen-specific antibodies, and direct inhibitors of B cells are also found in tick saliva, e.g., B cell inhibitory proteins in I. ricinus and B cell inhibitory factors in Hyalomma (H.) asiaticum (Páleníková et al., 2015).
Dynamic changes in salivary bioactive compound activity are correlated with host responses, cellular and chemical mediators, gluttony, and sex (Heinze et al., 2014). In adult D. andersoni females, it was found that within 2–5 days of the start of the feeding process, a total of 372 proteins can be identified, and among these, almost 140 were identified on day 2 and 165 on day 5 (Mudenda et al., 2014). Expression of saliva genes was recorded to be higher in female ticks than in males, which reflects the goals of feeding females to attain maximum blood meal size and increase egg production (De Castro et al., 2017). Transcriptomics studies have confirmed that salivary gene expression is variable as feeding progresses in I. ricinus females (Perner et al., 2018). For changes in saliva compositions, the term “sialome switching” is used. This change may be attributed to feeding environment changes, i.e., change in host type and host immune system (Karim and Ribeiro, 2015). Studies on saliva time regulation are limited; possibly, epigenetic regulation, chromatin remodeling, and histone modification are involved (Kotsyfakis et al., 2015; Cabezas-Cruz et al., 2016). Salivary gland acini granules are also involved in tick blood-feeding dynamism. Immunoglobulin binding proteins specific for males are stored in unfed R. appendiculatus male type IV acini, cement protein in type 3 acini in D. variabilis, and migration inhibitory factor (MIF) in A. americanum (Perner et al., 2018). This indicates that tick saliva is ready to start its action as soon as ticks attach and start feeding, and the contents of early salivary gland granules help to elucidate tick-host interaction at the feeding site and are early-stage targets for anti-tick vaccine development.
Transmission Dynamics Of Viruses In Ticks
Vector-borne viruses (VBVs) exhibit biological transmission: they enter into the vector, infect, and replicate before reaching the vertebrate host. Following the entry of a virus into the midgut of ticks, it must escape from the midgut and reach the tick salivary glands from which it will be transmitted to the vertebrate host (Šimo et al., 2017). This is described as an extrinsic incubation period as the virus remains inside the vector. Movements of the virus within a vector (midgut to salivary glands) are life-threatening for viruses because of various potential barriers: midgut infection, midgut escape, salivary gland infection, and salivary glands escape (Kazimírová et al., 2017) (Figures 2, 3).
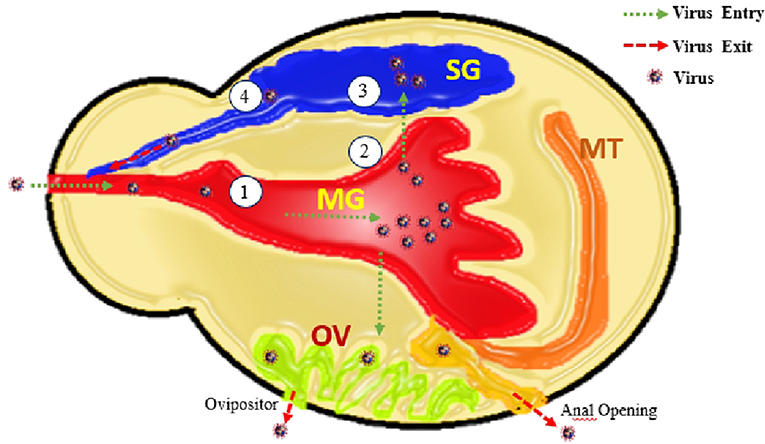
Figure 3. Elaborating the entry of virus through mouth opening along with bleed meal into mid gut (MG), virus multiplication and transfer into ovaries (OV), Salivary glands (SG), and anal opening. From these routes virus shed with saliva, transovarial (with next progeny of ticks), and anus. Along with these four barriers 1. Entry of virus into MG-cell, 2. Exit of virus from midgut cells, 3. Entry of virus into SG-cells (acini), and 4. Exit of virus from MG-cells.
More precisely, at the cellular level, the virus may remain unable to cross the cell membrane for entry into the cytoplasm, or the virus may replicate inside the cell following entry but is incompetent to come out of the infected cell (Dou et al., 2018). The intrinsic ability of a tick to become infected, support replication, and ultimately, transmit a tick-borne virus is genetically determined and influenced by environmental factors. Likewise, the ability of a tick-borne virus to infect, replicate, and be dispersed by a tick is both determined genetically and influenced by extrinsic factors. At one level, vector competence is determined through genotype-by-genotype interactions (Althouse and Hanley, 2015). In this sense, the outcome of infection depends on the interplay between the products of 2 genomes, the so-called virus–vector interactome. However, molecular interactions between tick-borne viruses and their tick vectors are yet to be explored. For example, we know little about the role of RNA interference (RNAi) in ticks and, in particular, whether it acts as an innate antivirus immune response modulating virus infection (Kurscheid et al., 2009; Kazimírová et al., 2017). Evidence in mosquitoes indicates that the RNAi pathway modulates arboviral infections, for example, by acting as a gatekeeper to the incoming viruses at the midgut, by minimizing the intensity of the viral infections, and reducing the spread of viruses from the midgut to secondary tissues (Khoo et al., 2010). It seems likely that a similar phenomenon occurs in ticks (Kazimírová et al., 2017).
Midgut Infection Barrier
Evidence of a midgut infection barrier has been reported in experimental studies with Rhipicephalus appendiculatus and Amblyomma variegatum, two tick species that are adept vectors of the Thogoto virus but are not competent for the Dhori virus (Gondard et al., 2020). When larvae and nymphs were fed on virus-infected hamsters, the Thogotovirus settled and replicated within the ticks and was subsequently transmitted when the succeeding adults fed on uninfected hamsters. In contrast, both tick species were refractory to infection by the Dhori virus when they fed on hamsters infected with this virus, with infectivity in the engorged ticks disappearing in 2–6 days. However, when the Dhori virus was inoculated into the hemocoel of engorged nymphs, effectually bypassing the midgut, the virus survived transcardially and was transmitted during the feeding of infected ticks. Thus, the midgut of R. appendiculatus and A. variegatum appear to be a barrier to infection by the Dhori virus but not by the Thogoto virus. As the Thogoto virus and the Dhori virus are members of the same virus genus and have similar infection strategies, the most likely reason for the variation in vector-species specificity lies in the sequence diversity of viral surface glycoproteins (Gondard et al., 2020). If this is the case, specific surface receptors might be existing on the surface of tick midgut cells to which the Thogoto virus binds via its glycoprotein but are not recognized by the Dhori virus. Alternatively, the Thogoto virus might have evolved a mechanism for evading the defense mechanism of R. appendiculatus and A. variegatum that is efficient against the Dhori virus.
Studies on African swine fever virus have also demonstrated the importance of virus replication in the midgut for successful infection of its vector, Ornithodoros porcinus (Nuttall, 2019b). A Malawi strain of the virus failed to replicate successfully in midgut epithelial cells of ticks exposed orally to the virus, although the virus replicated successfully in other cell types. Moreover, a different virus strain was infected and replicated successfully under the same experimental conditions. The results suggest that missing or defective genes in the Malawi strain might account for the failure of the virus to replicate successfully in midgut epithelial cells, although why this should be the case for midgut cells and not appear for other cell types is a conundrum (Lledó et al., 2020). Compared with evidence of a midgut infection barrier based on experimental studies with the Dhori and Thogoto viruses, African swine fever virus data suggest that there might be different types of midgut infection barriers in ticks (Rock, 2021).
One type of midgut barrier might be provided by the unusual way in which ticks digest their blood meal. Unlike insects, in which blood meal digestion is extracellular, ticks are heterophagous: intracellular digestion of blood meal takes place in midgut cells. Several insect-borne viruses depend on proteolytic conditions in the insect gut lumen to cleave a surface protein and expose the virus receptor that initiates infection of the vector (Talactac et al., 2021). The absence of such proteolytic enzymes in tick lumen could provide a highly efficient barrier to infection by viruses that require cleavage of a surface virus protein to initiate infection. If the process of blood meal digestion in ticks is an efficacious barrier to virus infection, arboviruses that can infect ticks are likely to have evolved an outer surface structure that differs significantly from that of their genetic relatives that are not transmitted by ticks. There are some data to support this hypothesis: (i) striking size variations in outer surface proteins of midge-transmitted arboviruses relative to the tick-transmitted arbovirus Broadhaven virus (Nuttall, 2013) and (ii) similarly pronounced differences in surface glycoproteins of influenza viruses relative to their tick-borne relatives (Shi et al., 2018).
However, the three-dimensional structures of the flavivirus that envelope proteins of tick-borne and mosquito-borne flaviviruses appear similar, although this similarity might reflect the common fusion role of this protein after entry into cells (Lemasson et al., 2021). One factor in the infection process that is usually overlooked is the state of a virus within the blood meal of its vector, whether as an extracellular virion (virus particle) or as an infected cell that may potentially be imbibed in the infected blood meal of a feeding tick. If a cellular rather than an extracellular viral inoculum is more effective in establishing infection in the tick vector, this might in part explain the efficiency of non-viremic transmission. Besides the state of a virus in the blood meal (whether “free” or within host cells), the timing of virus uptake also might be a critical factor in determining whether a virus infects a tick. This is because, like hematophagous insects, ticks produce a peritrophic membrane or glycocalyx on the apical surface of the midgut epithelium some hours after the commencement of feeding (Bhowmick and Han, 2020). The chitin-enriched covering potentially presents a formidable barrier to the infection of midgut epithelial cells by viruses. Studies on mosquitoes have shown that virions ingested in the viremic blood meal acquired from chickens infected with western equine encephalitis virus concentrate adjacent to the midgut epithelium. In contrast, when ticks fed on an artificial blood meal containing the virus, the disseminated virus was observed throughout the midgut lumen (Talactac et al., 2021). It waits to be revealed whether such concentration of virions occurs in the tick midgut and/or whether ingestion of infected cells rather than “free” virions helps the virus overcome the barrier presented by the peritrophic membrane. This suggests that viruses are unable to survive if they do not exit the midgut.
Midgut Escape Barrier
The evidence of a midgut escape barrier in ticks is based on comparative studies on infection of R. appendiculatus nymphs infected with the Dhori virus or the Dugbe virus. The Dhori virus can survive for <4 days in R. appendiculatus nymphs following vector feeding on an infected host. The Dugbe virus can survive for at least 21 days after vector meal ingestion but remains unable to survive during molting and has no transmission through adult ticks. Following virus inoculation directly into the hemocoel, just like the Dhori virus, the Dugbe virus replicates and is transmitted by R. appendiculatus; explaining that, there are no barriers for the Dugbe virus to infect the salivary glands of R. appendiculatus as for the Dhori virus (Kazimírová et al., 2017; Nuttall, 2019a). The variation in survival dynamics recommends that R. appendiculatus reveals a midgut infection barrier to the Dhori virus and a midgut escape barrier to the Dugbe virus. However, the nature of the midgut escape barrier is unknown.
Dissemination Barrier
Once a tick-borne virus has escaped from the tick midgut, it presumably passes through the hemocoel, where tissues and organs are immersed in hemolymph, the transport medium for hormones, nutrients, and immune effecter molecules. To migrate to the salivary glands while hiding from the tick's immune system, viruses, such as tick-borne encephalitis virus, African swine fever virus, and Dugbe virus, infect tick hemocytes (Talactac et al., 2021). An alternative route of dissemination is via the nervous system. However, although the Thogoto virus was recognized in the neural cortex of the synganglion, it was not apparent in nerve fibers, suggesting that dissemination through tick vector occurs via the hemolymph rather than a neural route (Grabowski et al., 2018). A dissemination barrier might exist in mosquitoes in which a virus is restricted to abdominal fat body cells, which play a role in insect immune responses (Lee et al., 2019). Their presence in ticks has not been described.
Salivary Gland Infection Barrier
After reaching the salivary glands, a virus faces barriers like those of the midgut: (i) cell infection and replication and (ii) virus release. Although there are some records of virus detection in saliva, little is known about this critical stage of tick-borne virus transmission (Nuttall, 2019a). Experimental studies with the Thogoto virus and Amblyomma variegatum indicate that infection of the salivary glands might not be a precondition for transmission (Gondard et al., 2020); extracellular virus inoculated into the hemocoel was detected shortly afterward in the saliva of ticks. The result was consistent with previous observations that the transmission of Thogoto virus occurred within 24 h of tick attachment to a host, even though virus infection of the salivary glands was not detected until 7 days after feeding commenced. The mechanism of virus transfer from the hemocoel to saliva is unknown. Some proteins found in the hemocoel (e.g., host immunoglobulins) appear to be excreted in tick saliva even though tick salivary glands exclude smaller molecules, such as polyethylene and inulin (Brzezinski, 2019). If tick-borne viruses can pass from the hemocoel into saliva without requiring infection of the salivary glands, salivary gland infection and escape barriers as described for the mosquito-borne transmission of insect-borne arboviruses might not exist in ticks. More importantly, there might be processes by which tick-borne viruses can be transmitted rapidly to the vertebrate host, presenting a greater epidemiological risk to humans.
Interestingly, Thogoto virus-infected ticks secreted less saliva than uninfected ticks or ticks inoculated with the virus into the hemocoel (Nuttall, 2019a). Possibly, virus infection had a deleterious impact on the fluid secretory process. Alternatively, the virus might have stimulated a more vigorous secretion in infected ticks, which would result in lower saliva volumes collected during experimentation. The latter hypothesis is consistent with observations that the tick-borne encephalitis virus stimulates the aggressiveness of its tick vector, Ixodes persulcatus (Morozova et al., 2020).
Salivary Gland Escape Barrier
Once a tick-borne virus has passed into the infected tick's hemocoel and survived molting, there might be a mechanism where the virus can pass into the saliva without having to overcome barriers to infection of the salivary glands. However, evidence of infection of tick salivary glands has been reported for several tick-borne viruses. For example, Dugbe virus infection was detected in discrete cells of type 3 salivary gland acini (Kramer and Tavakoli, 2021). A virus infecting the salivary glands has to be released into the salivary ducts to be transmitted in saliva. Little is known of the mechanisms of release of salivary proteins into saliva, let alone viruses. Similarly, the impact of the physicochemical properties of saliva on tick-borne viruses is unknown. There is no evidence that salivary proteins interact directly with virions, as reported for the tick-borne bacterium Borrelia burgdorferi. However, if tick saliva has a pH value in the range of 9–9.5, as some studies have indicated, the alkalinity of saliva could have a profound effect on the conformation of virions in tick saliva. For example, the icosahedral outer surface of the tick-borne encephalitis virus is steady in a limited pH range and opens when exposed to either acidic or alkaline circumstances (Šimo et al., 2017).
Trans-Stadial Survival
After engorgement, immature ticks undergo ecdysis, and histolytic enzymes and tissue replacement create a potentially hostile environment for viruses. For example, the salivary glands undergo reabsorption and restoration during molting. Thus, an essential feature of a tick-borne virus is its ability to survive the molting period for the virus to be transmitted from its tick vector to a vertebrate host. Virus replication dynamics in ticks might indicate these changing environmental conditions (i.e., a fall in infectious virus titter followed by an increase in titter as a virus infects and replicates in replacement tissues) (Nazar et al., 2013; Yoshii, 2019; Migné et al., 2022). However, the replication of some viruses (e.g., Langat in I. ricinus and Thogoto virus in R. appendiculatus) does not follow these dynamics (Godsey et al., 2021; Hart and Thangamani, 2021). The conflicting results may be explained by various cell and tissue tropisms of tick-borne viruses in their tick vectors. For example, the Thogoto virus establishes infection in the synganglion, where presumably it is safe from the processes of tissue replacement (Nuttall, 2013, 2019b; Morozova et al., 2020).
Because the salivary glands experience reabsorption and rejuvenation during molting, salivary gland infection is expected to be a relatively late event in virus dissemination in tick vectors following the uptake of an infective blood meal. The actual timing of infection of the salivary glands appears to vary. Tick-borne encephalitis virus and Powassan virus infect tick salivary glands before the commencement of feeding; seemingly, they can be transmitted to the vertebrate of the host as soon as fluid secretion occurs (Morozova et al., 2020). In contrast, the Thogoto virus and the Dugbe virus amass in the salivary glands following the commencement of feeding (Nuttall, 2013), although in ticks infected in the earlier stage, the Thogoto virus is found in the salivary glands before blood-feeding (Nuttall, 2019a).
Horizontal Transmission By Ticks
The principal route of transmission for tick-borne viruses is horizontal, from an infected tick to an uninfected definitive host and from an infected host to an uninfected tick. Classically, horizontal transmission from vertebrate to tick was suggested to depend on the level of viremia (virus circulating in the blood). It is now recognized that tick-borne viruses can be transmitted effectively even when an infectious virus is not detectable in the blood (Turell, 2020).
Viremic Transmission
Arboviruses have been defined as “viruses that are maintained in nature principally, or to an important extent, through biological transmission between susceptible vertebrate hosts by hematophagous arthropods or through transovarial and possibly venereal transmission in arthropods: the viruses multiply and produce viremia in the vertebrates, multiply in the tissues of arthropods, and are passed on to new vertebrates by the bites of arthropods after a period of extrinsic incubation.” A crucial point in the WHO definition of an arbovirus is the production of viremia. In animals (including humans) infected by the bite of a tick infected with tick-borne encephalitis virus, the virus replicates first in the skin site of tick feeding and in lymph nodes that drain the site. Neutrophils, monocytes/macrophages, and Langerhans cells attracted to the tick feeding site become infected (Hermance and Thangamani, 2018). Viremia develops when a virus is carried via the lymphatics to the thoracic duct and into the bloodstream. Primary viremia seeds are extraneural tissues that support further virus replication and shedding of the virus into circulation. In studies on mosquito-borne viruses, the threshold level of viremia was defined as the lowest amount of virus capable of causing an infection in around 1 to 5% of the vector population feeding on the viraemic host (Holding et al., 2020). Thus, the lower the infection level, the smaller the infective dose of virus required to infect the vector and, hence, the greater the likelihood of infection of the vector in nature. However, it was assumed that vertebrates in which an arbovirus induced a level of viremia that was below the threshold (or undetectable) were not hosts of the virus and did not contribute to the cycle of transmission (Nuttall, 2019a).
Experiments designed to evaluate host susceptibility to arbovirus infection routinely involved needle-and-syringe inoculation with the virus and subsequent assays of blood or other tissues for infectivity by intracerebral inoculation of suckling mice or plaque titration in cell cultures. For example, investigations of the infection threshold of I. ricinus for louping ill virus in which ticks were fed on domestic chicks inoculated with the virus indicated that viraemic titters of 4.7 and 3.7 log10 infectious units/ml blood were required to establish infections in larvae and nymphs, respectively. This agreed with the threshold of 3.9 log10 infectious units/ml blood for nymphs fed on viraemic sheep. Based on this experimental approach of needle-and-syringe inoculation with virus and sampling for threshold levels of viremia, mountain hares (Lepus timidus) were considered not to play a significant role in the transmission cycle of louping ill virus (Reid, 2019; Clark et al., 2020). However, subsequent studies have shown that mountain hares play a critical role in the maintenance of the louping ill virus in nature (Holding et al., 2020).
Non-Viremic (Co-Feeding) Transmission
The original concept of an arbovirus requiring threshold levels of virus infectivity in the blood for infection to be transmitted to an arthropod vector feeding on an viremic host has now been updated. The first challenges to the role of viremiain arbovirus transmission were reported in experimental studies involving the Thogoto virus and tick-borne encephalitis virus (Morozova et al., 2020). Following the feeding of infected and uninfected ticks (adults and nymphs) on susceptible hosts, most uninfected nymphs were infected during co-feeding without viremia (Brault et al., 2018). Another study was performed on non-viremic transmission of tick-borne encephalitis virus during co-feeding of virus-infected and non-virus-infected ticks on a non-viraemic host. The virus-free ticks were found positive while co-feeding (Morozova et al., 2020).
The original demonstration of non-viremic transmission using unnatural laboratory hosts has been corroborated by studies using natural host species. For example, infected and uninfected I. ricinus co-feeding on field mice (Apodemusflavicollis and A. agrarius) and bank voles (Myodesglareolus) demonstrated efficient transmission in the absence of viremia or at comparatively low viremia levels. In contrast, pine voles (Pitymyssubterraneus), which developed high levels of viremia, produced only a few infected ticks (Nuttall, 2019b). Similar results were observed for louping ill virus and uninfected wild-caught hares (Lepus timidus). Uninfected I. ricinus nymphs became infected with the virus when co-feeding with infected ticks, while the hares showed only low or undetectable levels of viremia (Brault et al., 2018). Evidence of non-viremic or efficient co-feeding transmission has now been recorded for at least 8 different tick-borne viruses. Further evidence of non-viremic transmission has been provided by studies using hosts' immunity to the virus. For example, natural rodent hosts (bank voles and field mice) of tick-borne encephalitis virus were immunized with the virus, either via subcutaneous syringe inoculation with a virus or by an infective tick bite.
Considering host immune status, it was found that there is a significant reduction in transmission efficiency in virus-immune relative to non-immune hosts, the evidence indicates that immunity to a tick-borne virus does not necessarily mean that an immune host is a dead-end for the virus, as is generally assumed. A 5-year survey on small mammals trapped in western Slovakia revealed a 15% neutralizing antibody prevalence for tick-borne encephalitis virus. The antibody prevalence varied seasonally and according to species (Bournez et al., 2020).
In addition to acquired immunity to tick-borne viruses, vertebrate hosts may also develop resistance to tick infestation, which can impair virus transmission (Nuttall, 2013). Immunity to ticks might explain field mice's greater efficiency than bank voles in supporting tick-borne virus transmission among co-feeding ticks (Brault et al., 2018). Further studies are considered necessary to illustrate the effect of host immune status to ticks on the transmission of tick-borne viruses. In non-viremic transmission, a virus is more likely to be ingested in the blood meal as infected cells than as extracellular virions (virus particles). An infected cell provides a bolus inoculum that might contain tens or even thousands of virions, depending on virus genotype (and its cell tropism), cell type, and stage of virus replication in the infected cell. Infected cells should be a more successful means of infection than extracellular virions in the blood meal, not only because they are likely to provide a larger dose of an infective virus but also because of the heterophagic way ticks digest their blood meal. Thus, the uptake of infected cells during co-feeding transmission might contribute to the efficiency of non-viremic relative to viremic transmission, in which the blood meal contains extracellular virions.
Saliva-Assisted Transmission
Non-viremic transmission between infected and uninfected ticks during co-feeding on the same host can be replicated empirically via needle-and-syringe inoculation if tick saliva or salivary gland extracts are included in the virus inoculum. This phenomenon has been named “saliva-assisted transmission” (Šimo et al., 2017). The first evidence that salivary gland constituents promote virus transmission was reported for the Thogoto virus and tick-borne encephalitis virus (Nuttall, 2019a). For example, syringe inoculation experiments using the Thogoto virus mixed with salivary gland extract generated from uninfected ticks resulted in a 10-times higher number of infected nymphs as compared to the numbers infected while feeding on a host inoculated with the virus. As with non-viremic virus transmission between co-feeding infected and uninfected ticks, none of the inoculated animals showed detectable viremia (Brault et al., 2018).
Augmentation of virus transmission was observed only with an inoculum mixed with salivary gland extract of infected ticks and was not observed with salivary glands from unfed ticks or with extracts from any other tick organ. Similar direct evidence of saliva-assisted transmission has been reported for the Lyme disease spirochetes Borrelia afzelii, B. burgdorferi sensu stricto, and B. lusitaniae, and for Francisellatularensis (Sprygin et al., 2019). The recognition of a saliva protein of I. scapularis, Salp15, that promotes the transmission of B. burgdorferi sensu stricto enabled the direct demonstration of saliva-assisted transmission via co-inoculation of mice with the recombinant Salp15 protein and the spirochete and by use of the RNAi technique. Comparable evidence of saliva components that promote virus transmission is lacking, although many candidates have been considered (Nuttall, 2019b). One of the most promising attribute of the tick-borne encephalitis virus is that it is the dendritic cell modulator (Fialová et al., 2010).
Vertical Transmission By Ticks
Various tick-borne viruses are transferred vertically from parents to offspring. This ability is found in all virus families and occurs in a range of both Argasid and Ixodid tick species. However, the percentage of infection in offspring (larvae) from mothers as transovarial transmission was <5 percent (Raney et al., 2022). Therefore, the prevalence rate via vertical transmission is considered too low to maintain tick-borne viruses without the amplifying effect of horizontal transmission (Turell, 2020). However, larvae show a highly non-random distribution on their hosts, and individuals from an egg batch quests together. Even if only a few larvae from an egg batch are infected transovarially, the infection rate might be enhanced as a result of non-viremic transmission among co-feeding larvae (Nuttall, 2019a). By this means, the low prevalence of transovarial infections may be augmented to yield much higher numbers of nymphal infections and, therefore, make a substantial contribution to virus survival. Opportunities for such augmentation of vertically transmitted infections take place in the field, where a low prevalence of tick-borne encephalitis virus infection in I. ricinus larvae has been recorded. Comparable results have been documented for the Colorado tick fever virus and the Crimean-Congo hemorrhagic fever virus, whereas higher filial infection prevalence was reported for the African swine fever virus (Yadav et al., 2019; Hughes et al., 2021).
Microclimatic Conditions At Tick-Pathogen Interface
The distribution and abundance of ticks are influenced by macro and microclimatic changes, travel, land use, human behavior, and habitat modification. These factors also influence the demography of tick-borne pathogens around the globe. Resurgence, the emergence of new diseases, is also influenced by population growth, shifting, grazing, and transboundary transportation of animals for the economy and politics. Intrinsic changes and extrinsic factors both are enabling factors for tick-borne diseases (Pfäffle et al., 2013; Baneth, 2014; Dantas-Torres, 2015). Ticks are very susceptible to climate. They spend most of their lifetime in the environment and all life cycle stages are dependent on climate variability. Although vegetation and host availability modulate the dynamics of their population, the climate is the major driver for the absence or presence of ticks (Esser et al., 2019). Ticks adapt to vegetation or microclimatic conditions for their survival and development. Host availability concerning time and space is very important for bionomics. Environmental characteristics (rainfall, humidity, and temperature), host characteristics (age, sex, and bodily condition), and management strategies (animal husbandry and land use) all influence tick loads (Kemal et al., 2016).
Shelter and protection under harsh climatic conditions are other drivers for questing ticks because questing ticks are more vulnerable to these conditions. Poor tick management tactics and large-scale transhumance migration of cattle in search of water and pasture during the dry season are causes of excessive tick infestations in several regions (Mirkena et al., 2018). The wet season is found favorable for the progression of ticks and tick-borne diseases, as they require a humidity level of 85–90%. Both the poor health of animals and the wet season are enabling factors for tick burden and illnesses. In tropical dry lands, the wet season is marked by moderate to heavy rainfall, increased humidity, increased plant cover, and an increase in the availability of appropriate hosts (Medlock et al., 2013; Vander Waal et al., 2017). The rainy season, as compared to the dry season, provides more promising micro-climatic conditions for tick mass reproduction and dissemination in hosts (Esser et al., 2019). After a few months of drought, cow mortality owing to tick-borne illnesses (East Coast fever or anaplasmosis) was found to be greater than that seen in the rainy season (Chepkwony et al., 2020).
Drought conditions verily enhance the abundance of ticks as the animals' body condition deteriorates and results in mortalities (Brown et al., 2014). Vander Waal et al. (2017) discovered that during the dry season, parasites, such as ticks, fleas, and mites, were more commonly exchanged in watering locations than during the rainy season. Temperature, with relatively low humidity, leads to the desiccation of eggs and interrupts the life cycle of ticks. Low water in the environment also leads to water stress in adult ticks.
Tick Management
Tick burden was found significantly lower in intensively managed ranches than in ranches managed with the transhumance management scheme. Nonetheless, tick loads on cattle are found to be reduced under intensive management systems with the utilization of acaricides and typically limited host mobility. In contrast, transhumance, which is a key adaptation for pastoralist societies, has been demonstrated to have a favorable impact on parasite distribution and disease dynamics, since animals from nearby areas are likely to bring ticks with them (Mutavi et al., 2018). The epidemiology of ticks and tick-borne diseases is being influenced by dynamic interactions between the abiotic and biotic factors (Wikel, 2018b). Seminal studies have given the concept that zoonotic pathogens and vectors related to them live in distinct habitats that provide the concept of landscape epidemiology or natural nidality of vectored transmissible diseases. Diving into the cellular and molecular level of interaction of tick-host-pathogen, studies provide seminal knowledge of the establishment, pathogenesis, and characterization of the pathogens' transmission and novel clues for control of pathogens and their vectors.
Effect Of Heat Shock Proteins On Ticks
Heat shock and other stress-related responses are helpful for the modulation of ticks and pathogen infections (Espinosa et al., 2017). Stress response proteins (SRPs) and heat shock proteins (HSPs) provide cells with a higher level of tolerance against harsh environments and protect organisms from damage. Glutathione-S-transferase, metallothioneins, ferritin, and selenoproteins have been reported to be involved in various stress situations, such as blood-feeding, pathogen infections, tick attachment, oxidative stress, and heat shock (Busby et al., 2012; Galay et al., 2014; Siddiqi et al., 2016; Hernandez et al., 2019).
Various studies reported that stress response is induced by pathogen infection and heat shock (Rosche et al., 2021; Neelakanta and Sultana, 2022). However, under the natural pathogen-vector relationship, there is no significant interaction between HSPs and SRPs and reflection of the mechanism of co-evaluation. High temperatures and blood-feeding mainly affect the questing speed of ticks under the overexpression of subleasing, HSP 20, and HSP 70. Ticks acquire pathogens from reservoirs while feeding on them and transmit them to a host after multiplication in the gut wall. At the tick-pathogen interface, a virus has to overcome salivary gland barriers and midgut in the body of a tick (Benelli, 2020).
Role Of Immunity In Tick-Pathogen Interface
Ticks' immunity is only dependent on innate immunity, and there is no adaptive immunity, so viruses invade ticks and evade the host immune system, and keep themselves safe from the phagocytosis, nodulation, encapsulation, and secretions of hemolymph (innate immunity) of ticks (McNally and Bloom, 2013). Additional antivirus innate response is dependent on RNAi, which limits virus replications (Migné et al., 2022). Metagenomic studies elucidated that endosymbiont and other pathogens are also present in ticks at the same time (Papa et al., 2017). Tick saliva is the key factor for the increasing pace of tick fauna. Ticks are capable of modulating their saliva, which is a predisposing factor for bloodsucking. As the modulation results in the successful acquisition of feed, this makes ticks successful in the environment and increases pathogen transmission (Wikel, 2013, 2018a; Kotál et al., 2015b; Chmelar et al., 2016a,b). Molecular technologies, such as genomics, metagenomics, functional genomics, proteomics, transcriptomics, and metabolomics, are advanced tools for the rapid detection of pathogens and understanding their complex pathways at the tick-pathogen interface. Saliva makes the cutaneous environment of a host favorable for blood-feeding, transmission, and establishment of infections and infectious agents by deviation or suppression of host pain, inflammation, hemostasis, adaptive and immune defenses, and wound healing (Wikel, 2013, 2018b; Kotál et al., 2015b; Chmelar et al., 2016a,b; Kazimírová et al., 2017).
The first complex study on tick saliva was carried out by analyzing cDNA libraries on bases of expression (Karim and Ribeiro, 2015). Initial transcriptome characterization of salivary glands was under protein constituent complexity and conducted by applying high throughput sequencing technology (Wikel, 2018b). Combined proteomics and transcriptomics analyses provide deep knowledge to understand functional genomics. Pathogens are not entirely silent in ticks but may also affect vector survival, gene expression, and behavior. These are some factors that cause variations in the tick-pathogen relationship. New generation sequencing will help provide more insights into the tick-pathogen interface.
Mitigation Strategies For Tick-Borne Viruses
Ticks have emerged as a vector for virus transmission with unique features, including lengthened life span and multiplex development, prolonged feeding periods, characteristic digestion of blood in the midgut, and hematophagy throughout life stages, making them a successful vector for virus transmission while contributing to the failure to control tick-borne diseases. Tick control methods can be clumped into chemical, non-chemical, and genetic manipulation, biological control, herbal acaricide, use of biopesticides, and vaccination using tick antigens (Manjunathachar et al., 2014).
Acaricidal Control
Until now, tick control is still mainly based on the use of acaricides to encounter tick-related issues and economic losses. Unfortunately, unjustified and extensive use of acaricides has led to some serious concerns comprising the development of acaricidal resistance in ticks, residual effects in milk and meat, and eco-unfriendliness. Moreover, mutations in genes associated with drug susceptibility have also been reported to be leading to the development of resistance. Furthermore, it is resulting in a rise in the lethal dose of drugs for a particular determined species. Currently, a combination of various acaricidal drugs by combining potent active ingredients is widely being used to make the mechanisms of action diverse and minimize the emergence of tick resistance (Domingos et al., 2013). This is a strong signal for the future use of chemical-based acaricide for tick control, as it is still the backbone of tick control strategies. However, the fact cannot be ignored that wide dependence on acaricide usage is not expendable and demands the attention of researchers to avoid the spread of tick-borne viral infections.
Vaccines And Genetic Manipulation
One reason for the availability of comparatively fewer vaccines to control TBV is that infectious diseases have a worse epidemiological impact than tick-borne viral diseases. The development of a vaccine that particularly interferes with the transmission of tick-borne viruses can aid to overcome the challenge (Kazimírová et al., 2017). Tick feeding exerts certain effects on the host's immune system expressing the complex aspects of host-tick interaction. The slow feeding habit of ticks accompanied by immunomodulatory and immunosuppressive components of their saliva made ticks survive longer on a host (Tirloni et al., 2014). Besides this protective mechanism, the salivary components also act as antigens to trigger immune responses resulting in acquired resistance of a host. However, this form of resistance by the host is transitory, suggesting that ticks have eluded the host's immune system (Kitsou et al., 2021).
The effectiveness of a vaccine greatly depends on the magnitude and persistence of an antibody, although repeated booster is essential for maximum efficacy. The commercially available anti-Boophilus vaccine containing BM86 antigen has been found effective. However, DNA anti-tick vaccines are in inception. It is believed that plasmid-injected DNA molecules directly enter the nucleus and remain as episomal DNA inside the nucleus, generating protective antigens if a cell lives. The uninterrupted in vivo formation, processing, and presentation of antigens to T cells in DNA vaccinated animals help to maintain maximum antibody titer resultantly avoiding the need for the repated boosters (Rego et al., 2019).
A tick control strategy comprising a vaccine based on a recombinant tick gene exhibits promising results. It demonstrates several advantages, as it is cost-effective, reduces acaricidal application, and minimizes the prevalence of tick-borne diseases by reducing the exposure of animals to infected ticks. However, the efficacy of such a vaccine widely depends on geographical distribution and tick species (de la Fuente et al., 2007). Tick cell lines have played a significant role in the identification of tick protective antigens to produce a wide range of vaccines for controlling tick-borne pathogens. Cell lines obtained from susceptible and resistant ticks, gene manipulated cell lines, and cell lines promoting the growth of intracellular tick-borne pathogens generated in vitro can assist to decrease the prevalence of tick-borne diseases (Al-Rofaai and Bell-Sakyi, 2020).
Use Of Endosymbionts
Endosymbionts can be a potential tick control strategy, but unfortunately, it is still unexplored. Few studies in the past have reflected on the identification and characterization of endosymbionts of ticks (Azagi et al., 2017). Ticks depend on the host's blood, the only source of nutrition providing all the essential nutrients for their growth and development. Ticks do have primary endosymbionts that are transmitted maternally or vertically through progenies. This association between ticks and endosymbionts can be beneficial for an arthropod host. Since endosymbionts are necessary for an arthropod host, removal of these organisms would make the survival of the arthropod host difficult. Studies on physiology and genetics would be required for the manipulation of this symbiotic interface. Along with that, microbiological, chemotherapeutic, and immunological approaches will also be required (Budachetri et al., 2018).
Biological Control
Natural enemies of ticks include parasitoid wasps, insectivorous birds, nematodes, Bacillus thuringiensis bacteria, and deuteromycete fungi (Bassiana, Beauveria, and Metarhizium). The biocontrol potential of entomopathogenic fungi for tick control has been examined in various laboratory bioassays (Ebani and Mancianti, 2021). Conidia have been found effective when applied on an animal host under field and semi-field conditions but greatly depend on the behavior of the tick species infesting and the animal host involved.
Various species of fungi were reported as pathogenic to a wide range of tick species and cause high mortality in susceptible species, such as R microplus. Similarly, M. anisoplae can also aid in the management of the tick population with relatively fewer adverse effects on the environment. Moreover, it has been reported as potentially effective against a wide range of arthropods and, thus, can cause the death of non-target species (Azagi et al., 2017). Some parasitoid Ixodiphagus hookeri wasps can parasitize various forms of ticks, such as larvae and nymphs. It has been suggested that the odor from tick host animals attracts parasitoid wasps (Sormunen et al., 2019). Moreover, 42 nematode strains have shown an anti-tick activity with varying degrees of virulence. At higher concentrations and under optimal conditions, nematodes can kill engorged female ticks before they lay eggs. It is also said that nematodes normally do not attack ticks, but using a tick as bait can help to detect some aggressive strains of tick pathogenic nematodes (Singh et al., 2018).
Some entomopathogenic bacteria, such as B. thuringiensis, exhibit mortality in ticks, specifically the larval form of ticks, and higher mortality rates were recorded with an increase in spore concentration. This control strategy offers potential for the control of ectoparasites (Ebani and Mancianti, 2021).
Genetic Manipulation Using Rna Interference
RNA interference is an extensively used gene silencing technique for the genetic manipulation of ticks. It has been proved as an effective tool to identify and characterize tick-pathogen interference, tick protective antigens, and screening. It is a nucleic acid-based reverse genetic method used to determine gene function and its possible effects on the metabolic pathway by disrupting gene expression. Four methods, namely, injection, soaking, feeding, and virus production, of dsRNA have been implied to deliver dsRNA for RNA interference in ticks (Niu et al., 2018).
Through this technique, a large number of genes can be recognized as potent candidates for a vaccine. This approach is relatively cheap and requires minimum use of laboratory animals. Selected antigens, after characterization and evaluation, can be produced as recombinant proteins that can be used for vaccine trials (Sudhakar et al., 2013). To understand better and utilize this approach, dsRNA-induced RNAi mechanism should be clarified and refined, since it can elaborate the tick-virus interface and can play a role in vaccine development and control of transmission of tick-borne viruses.
Conclusion
Based on the above-mentioned discussion, it can be concluded that tick-borne viruses are a major threat to public health, and tick-virus interaction is the key point of the spread of these infections. Different factors both from the side of ticks and viruses are involved in virus replication and blockage in tick saliva and midgut. By controlling/ modifying the proteomics of tick saliva, transmission routes, and vector control strategies, the damage caused by tick-borne viruses can be minimized. We are hopeful that this review will enhance the public perception regarding these viruses and tick-virus interaction and will provide insight into future investigations regarding their control using the factors involved at the tick-virus interaction level.
Author Contributions
All authors equally contributed in manuscript writing and editing. All authors contributed to the article and approved the submitted version.
Conflict of Interest
The authors declare that the research was conducted in the absence of any commercial or financial relationships that could be construed as a potential conflict of interest.
Publisher's Note
All claims expressed in this article are solely those of the authors and do not necessarily represent those of their affiliated organizations, or those of the publisher, the editors and the reviewers. Any product that may be evaluated in this article, or claim that may be made by its manufacturer, is not guaranteed or endorsed by the publisher.
Acknowledgments
We are thankful to Professor Emeritus Thomas J Nolan of Penn State University Pennsylvania, United States for assisting with English grammar review and improving the manuscript significantly.
References
Ali, A., Fernando Parizi, L., Garcia Guizzo, M., Tirloni, L., Seixas, A., Silva Vaz, I., et al. (2015a). Immunoprotective potential of a rhipicephalus (boophilus) microplus metalloprotease. Vet. Parasitol. 207, 107–114. doi: 10.1016/j.vetpar.2014.11.007
Ali, A., Khan, S., Ali, I., Karim, S., da Silva Vaz, I., and Termignoni, C. (2015b). Probing the functional role of tick metalloproteases. Physiol. Entomol. 40, 177–188. doi: 10.1111/phen.12104
Al-Rofaai, A., and Bell-Sakyi, L. (2020). Tick cell lines in research on tick control. Front. Physiol. 11, 152. doi: 10.3389/fphys.2020.00152
Althouse, B. M., and Hanley, K. A. (2015). The tortoise or the hare? Impacts of within-host dynamics on transmission success of arthropod-borne viruses. Philos. Trans. R. Soc. B Biol. Sci. 370, 20140299. doi: 10.1098/rstb.2014.0299
Assumpção, T. C., Mizurini, D. M., Ma, D., Monteiro, R. Q., Ahlstedt, S., Reyes, M., et al. (2018). Ixonnexin from tick saliva promotes fibrinolysis by interacting with plasminogen and tissue-type plasminogen activator, and prevents arterial thrombosis. Sci. Rep. 8, 4806. doi: 10.1038/s41598-018-22780-1
Austyn, J. M. (2017). Dendritic cells in the immune system-history, lineages, tissues, tolerance, and immunity. Microbiol. Spectr. 4, 4–6. doi: 10.1128/9781555819194.ch10
Azagi, T., Klement, E., Perlman, G., Lustig, Y., Mumcuoglu, K. Y., Apanaskevich, D. A., et al. (2017). Francisella-like endosymbionts and Rickettsia species in local and imported Hyalomma ticks. Appl. Environ. Microbiol. 83, e01302–e01317. doi: 10.1128/AEM.01302-17
Balashov, Y. S. (1972). Blood-sucking ticks (ixodidae)-vectors of disease of man and animals. Misc. Pib. Entomol. Soc. Am. 8, 161–376.
Baneth, G. (2014). Tick-borne infections of animals and humans: a common ground. Int. J. Parasitol. 44, 591–596. doi: 10.1016/j.ijpara.2014.03.011
Barker, D. M., Ownby, C. L., and Krolak, J. M. (1984). The effects of attachment, feeding, and mating on the morphology of the type I alveolus of salivary glands of the lone star tick, Amblyommaamericanum (L.). J. Parasitol. 70, 99–113. doi: 10.2307/3281931
Benelli, G. (2020). Pathogens manipulating tick behavior—through a glass, darkly. Pathogens 9, 664. doi: 10.3390/pathogens9080664
Bente, D. A., Forrester, N. L., Watts, D. M., McAuley, A. J., Whitehouse, C. A., and Bray, M. (2013). Crimean-Congo hemorrhagic fever: history, epidemiology, pathogenesis, clinical syndrome and genetic diversity. Antiviral Res. 100, 159–189. doi: 10.1016/j.antiviral.2013.07.006
Bhowmick, B., and Han, Q. (2020). Understanding tick biology and its implications in anti-tick and transmission blocking vaccines against tick-borne pathogens. Front. Vet. Sci. 7, 319. doi: 10.3389/fvets.2020.00319
Bichaud, L., de Lamballerie, X., Alkan, C., Izri, A., Gould, E. A., and Charrel, R. N. (2014). Arthropods as a source of new RNA viruses. Microb. Pathog. 77, 136–141. doi: 10.1016/j.micpath.2014.09.002
Binnington, K. C. (1978). Sequential changes in salivary gland structure during attachment and feeding of the cattle tick, Boophilus microplus. Int. J. Parasitol. 8, 97–115. doi: 10.1016/0020-7519(78)90004-8
Blisnick, A. A., Foulon, T., and Bonnet, S. I. (2017). Serine protease inhibitors in ticks: an overview of their role in tick biology and tick-borne pathogen transmission. Front. Cell. Infect. Microbiol. 7, 1–24. doi: 10.3389/fcimb.2017.00199
Bournez, L., Umhang, G., Moinet, M., Richomme, C., Demerson, J.-M., Caillot, C., et al. (2020). Tick-borne encephalitis virus: seasonal and annual variation of epidemiological parameters related to nymph-to-larva transmission and exposure of small mammals. Pathogens 9, 518. doi: 10.3390/pathogens9070518
Brault, A. C., Savage, H. M., Duggal, N. K., Eisen, R. J., and Staples, J. E. (2018). Heartland virus epidemiology, vector association, and disease potential. Viruses 10, 498. doi: 10.3390/v10090498
Brown, L., Medlock, J., and Murray, V. (2014). Impact of drought on vector-borne diseases-how does one manage the risk?. Public Health. 128, 29-37. doi: 10.1016/j.puhe.2013.09.006
Brzezinski, K. (2019). Examining the effects of cold on gut epithelial permeability in locusta migratoria (Master's dissertation). Carleton University, Ottawa, ON, Canada.
Budachetri, K., Kumar, D., Crispell, G., Beck, C., Dasch, G., and Karim, S. (2018). The tick endosymbiont candidatus Midichloria mitochondrii and selenoproteins are essential for the growth of rickettsia parkeri in the Gulf Coast tick vector. Microbiome 6, 1–15. doi: 10.1186/s40168-018-0524-2
Bullard, R., Allen, P., Chao, C. C., Douglas, J., Das, P., Morgan, S. E., et al. (2016). Structural characterization of tick cement cones collected from in vivo and artificial membrane blood-fed lone star ticks (Amblyomma americanum). Ticks Tick. Borne. Dis. 7, 880–892. doi: 10.1016/j.ttbdis.2016.04.006
Burthe, S. J., Schäfer, S. M., Asaaga, F. A., Balakrishnan, N., Chanda, M. M., Darshan, N., et al. (2020). Reviewing the ecological evidence-base for management of emerging tropical zoonoses: kyasanur forest disease in india as a case study. PLoS Negl. Trop. Dis. 15, e0009243. doi: 10.21203/rs.3.rs-35351/v1
Busby, A. T., Ayllón, N., Kocan, K. M., Blouin, E. F., De La Fuente, G., Galindo, R. C., et al. (2012). Expression of heat shock proteins and subolesin affects stress responses, Anaplasma phagocytophilum infection and questing behaviour in the tick, Ixodes scapularis. Med. Vet. Entomol. 26, 92–102. doi: 10.1111/j.1365-2915.2011.00973.x
Cabezas-Cruz, A., Alberdi, P., Ayllón, N., Valdés, J. J., Pierce, R., Villar, M., et al. (2016). Anaplasmaphagocytophilum increases the levels of histone modifying enzymes to inhibit cell apoptosis and facilitate pathogen infection in the tick vector Ixodes scapularis. Epigenetics 11, 303–319. doi: 10.1080/15592294.2016.1163460
Carvalho-Costa, T. M., Mendes, M. T., Da Silva, M. V., Da Costa, T. A., Tiburcio, M. G. S., Anhê, A. C. B. M., et al. (2015). Immunosuppressive effects of Amblyomma cajennense tick saliva on murine bone marrow-derived dendritic cells. Parasit. Vect. 8, 22. doi: 10.1186/s13071-015-0634-7
Chen, Z., Liu, Q., Liu, J. Q., Xu, B. L., Lv, S., Xia, S., et al. (2014). Tick-borne pathogens and associated co-infections in ticks collected from domestic animals in central China. Parasit.Vect. 7, 237. doi: 10.1186/1756-3305-7-237
Chepkwony, R., Castagna, C., Heitkönig, I., Van Bommel, S., and Van Langevelde, F. (2020). Associations between monthly rainfall and mortality in cattle due to East Coast fever, anaplasmosis and babesiosis. Parasitology 147, 1743–1751. doi: 10.1017/S0031182020001638
Chmelar, J., Calvo, E., Pedra, J. H. F., Francischetti, I. M. B., and Kotsyfakis, M. (2012). Tick salivary secretion as a source of antihemostatics. J. Proteomics 75, 3842–3854. doi: 10.1016/j.jprot.2012.04.026
Chmelar, J., Kotál, J., Karim, S., Kopacek, P., Francischetti, I. M. B., Pedra, J. H. F., et al. (2016b). Sialomes and mialomes: a systems-biology view of tick tissues and tick-host interactions. Trends Parasitol. 32, 242–254. doi: 10.1016/j.pt.2015.10.002
Chmelar, J., Kotál, J., Kopecký, J., Pedra, J. H. F., and Kotsyfakis, M. (2016a). All for one and one for all on the tick-host battlefield. Trends Parasitol. 32, 368–377. doi: 10.1016/j.pt.2016.01.004
Chmelar, J., Kotál, J., Langhansová, H., and Kotsyfakis, M. (2017). Protease inhibitors in tick saliva: the role of serpins and cystatins in tick-host-pathogen interaction. Front. Cell. Infect. Microbiol. 7, 216. doi: 10.3389/fcimb.2017.00216
Clark, J. J., Gilray, J., Orton, R. J., Baird, M., Wilkie, G., Filipe, A., et al. (2020). Population genomics of louping ill virus provide new insights into the evolution of tick-borne flaviviruses. PLoS Negl. Trop. Dis. 14, e0008133. doi: 10.1371/journal.pntd.0008133
Coons, L. B., and Lamoreaux, W. J. (1986). Developmental Changes in The Salivary Glands of Male and Female Dermacentor Variabilis (Say) During Feeding. University Forida-IFAS. Florida Medical Entomology Lab.
Dantas-Torres, F. (2015). Climate change, biodiversity, ticks and tick-borne diseases: the butterfly effect. Int. J. Parasitol. Parasites Wildl. 4, 452–461. doi: 10.1016/j.ijppaw.2015.07.001
Dantas-Torres, F., Chomel, B. B., and Otranto, D. (2012). Ticks and tick-borne diseases: a one health perspective. Trends Parasitol. 28, 437–446. doi: 10.1016/j.pt.2012.07.003
De Castro, M. H., De Klerk, D., Pienaar, R., Rees, D. J. G., and Mans, B. J. (2017). Sialotranscriptomics of Rhipicephalus zambeziensis reveals intricate expression profiles of secretory proteins and suggests tight temporal transcriptional regulation during blood-feeding. Parasit. Vect. 10, 384. doi: 10.1186/s13071-017-2312-4
de la Fuente, J., Kocan, K. M., Almazán, C., and Blouin, E. F. (2007). RNA interference for the study and genetic manipulation of ticks. Trends Parasitol. 23, 427–433. doi: 10.1016/j.pt.2007.07.002
de la Fuente, J., Villar, M., Cabezas-Cruz, A., Estrada-Peña, A., Ayllón, N., and Alberdi, P. (2016). Tick–Host–Pathogen interactions: conflict and cooperation. PLoS Pathog. 12, e1005488. doi: 10.1371/journal.ppat.1005488
De Meneghi, D., Stachurski, F., and Adakal, H. (2016). Experiences in tick control by acaricide in the traditional cattle sector in Zambia and Burkina Faso: possible environmental and public health implications. Front. Public Health 4, 239. doi: 10.3389/fpubh.2016.00239
Díaz-Martín, V., Manzano-Román, R., Oleaga, A., and Pérez-Sánchez, R. (2015). New salivary anti-haemostatics containing protective epitopes from ornithodoros moubata ticks: assessment of their individual and combined vaccine efficacy. Vet. Parasitol. 212, 336–349. doi: 10.1016/j.vetpar.2015.08.005
Domingos, A., Antunes, S., Borges, L., and Rosario, V. E. (2013). Approaches towards tick and tick-borne diseases control. Rev. Soc. Bras. Med. Trop. 46, 265–269. doi: 10.1590/0037-8682-0014-2012
Dou, D., Revol, R., Östbye, H., Wang, H., and Daniels, R. (2018). Influenza A virus cell entry, replication, virion assembly and movement. Front. Immunol. 9, 1581. doi: 10.3389/fimmu.2018.01581
Ebani, V. V., and Mancianti, F. (2021). Entomopathogenic fungi and bacteria in a veterinary perspective. Biology 10, 479. doi: 10.3390/biology10060479
El Shoura, S. M. (1987). Spermiogenesis ultrastructure in the tick argas (persicargas) arboreus (Acari: Ixodoidea: Argasidae). J. Med. Entomol. 24, 532–535. doi: 10.1093/jmedent/24.5.532
Espinosa, P. J., Alberdi, P., Villar, M., and Cabezas-Cruz, A. (2017). “Heat shock proteins in vector-pathogen interactions: The anaplasma phagocytophilum model,” in Heat Shock Proteins in Veterinary Medicine and Sciences, eds A. Alexzander, A. Asea, and P. Kaur (Cham; New York, NY: Springer), 375–398.
Esser, H. J., Mögling, R., Cleton, N. B., Van Der Jeugd, H., Sprong, H., Stroo, A., et al. (2019). Risk factors associated with sustained circulation of six zoonotic arboviruses: a systematic review for selection of surveillance sites in non-endemic areas. Parasit. Vect. 12, 265. doi: 10.1186/s13071-019-3515-7
Esteves, E., Maruyama, S. R., Kawahara, R., Fujita, A., Martins, L. A., Righi, A. A., et al. (2017). Analysis of the salivary gland transcriptome of unfed and partially fed Amblyomma sculptum ticks and descriptive proteome of the saliva. Front. Cell. Infect. Microbiol. 7, 476. doi: 10.3389/fcimb.2017.00476
Estrada-Peña, A., and de la Fuente, J. (2014). The ecology of ticks and epidemiology of tick-borne viral diseases. Antiviral Res. 108, 104–128. doi: 10.1016/j.antiviral.2014.05.016
Estrada-Peña, A., and de la Fuente, J. (2018). The fossil record and the origin of ticks revisited. Exp. Appl. Acarol. 75, 255–261. doi: 10.1007/s10493-018-0261-z
Estrada-Peña, A., Roura, X., Sainz, A., Miró, G., Solano-Gallego, L. (2017). Species of ticks and carried pathogens in owned dogs in Spain: results of a one-year national survey. Ticks tick-Borne Dis. 8, 43–52. doi: 10.1016/j.ttbdis.2017.02.001
Fawcett, D. W., Binnington, K., and Voigt, W. P. (1986). The cell biology of the ixodid tick salivary gland. Morphol. Physiol. Behav. Biol. Ticks 22–45.
Fialová, A., Cimburek, Z., Iezzi, G., and Kopecký, J. (2010). Ixodes ricinus tick saliva modulates tick-borne encephalitis virus infection of dendritic cells. Microbes Infect. 12, 580–585. doi: 10.1016/j.micinf.2010.03.015
Galay, R. L., Miyata, T., Umemiya-Shirafuji, R., Maeda, H., Kusakisako, K., Tsuji, N., et al. (2014). Evaluation and comparison of the potential of two ferritins as anti-tick vaccines against Haemaphysalis longicornis. Parasit. Vectors 7, 1-10. doi: 10.1186/s13071-014-0482-x
Gao, Y., Zhao, C., Wang, W., Jin, R., Li, Q., Ge, Q., et al. (2016). Prostaglandins E2 signal mediated by receptor subtype EP2 promotes IgE production in vivo and contributes to asthma development. Sci. Rep. 6, 20505. doi: 10.1038/srep20505
Ghafar, A., Gasser, R. B., Rashid, I., Ghafoor, A., and Jabbar, A. (2020). Exploring the prevalence and diversity of bovine ticks in five agro-ecological zones of Pakistan using phenetic and genetic tools. Ticks Tick. Borne. Dis. 6, 483–488. doi: 10.1016/j.ttbdis.2020.101472
Gharbi, M., and Aziz Darghouth, M. (2014). A review of hyalommascupense (Acari, Ixodidae) in the maghreb region: from biology to control. Parasite 21, 2. doi: 10.1051/parasite/2014002
Ghosh, S., and Nagar, G. (2014). Problem of ticks and tick-borne diseases in India with special emphasis on progress in tick control research: a review. J. Vector Borne Dis. 51, 259–270.
Godsey, M. S. Jr., Rose, D., Burkhalter, K. L., Breuner, N., Bosco-Lauth, A. M., Kosoy, O. I., et al. (2021). Experimental infection of Amblyomma americanum (Acari: Ixodidae) with bourbon virus (Orthomyxoviridae: Thogotovirus). J. Med. Entomol. 58, 873–879. doi: 10.1093/jme/tjaa191
Gondard, M., Temmam, S., Devillers, E., Pinarello, V., Bigot, T., Chrétien, D., et al. (2020). RNA viruses of amblyomma variegatum and rhipicephalus microplus and cattle susceptibility in the french antilles. Viruses 12, 144. doi: 10.3390/v12020144
Grabowski, J. M., Offerdahl, D. K., and Bloom, M. E. (2018). The use of ex vivo organ cultures in tick-borne virus research. ACS Infect. Dis. 4, 247–256. doi: 10.1021/acsinfecdis.7b00274
Grisi, L., Leite, R. C., Martins, J. R. S., Barros, A. T. M., Andreotti, R., Cançado, P. H. D., et al. (2014). Reassessment of the potential economic impact of cattle parasites in Brazil. Rev. Bras. Parasitol. Vet. 23, 150–156. doi: 10.1590/S1984-29612014042
Guglielmone, A. A., Robbins, R. G., Apanaskevich, D. A., Petney, T. N., Estrada-Peña, A., and Horak, I. G. (2014). The Hard Ticks of the World. Dordrecht: Springer. doi: 10.1007/978-94-007-7497-1
Hackenberg, M., Langenberger, D., Schwarz, A., Erhart, J., and Kotsyfakis, M. (2017). In silico target network analysis of de novo-discovered, tick saliva-specific microRNAs reveals important combinatorial effects in their interference with vertebrate host physiology. RNA 23, 1259–1269. doi: 10.1261/rna.061168.117
Hart, C. E., and Thangamani, S. (2021). Tick-virus interactions: current understanding and future perspectives. Parasit. Immunol. 43, e12815. doi: 10.1111/pim.12815
Hayward, J., Sanchez, J., Perry, A., Huang, C., Valle, M. R., Canals, M., et al. (2018). Erratum: ticks from diverse genera encode chemokine-inhibitory evasin proteins. J. Biol. Chem. 292, 15670–15680. doi: 10.1074/jbc.M117.807255
Heath, W. R., and Carbone, F. R. (2013). The skin-resident and migratory immune system in steady state and memory: innate lymphocytes, dendritic cells and T cells. Nat. Immunol. 14, 978–985. doi: 10.1038/ni.2680
Heinze, D. M., Carmical, J. R., Aronson, J. F., Alarcon-Chaidez, F., Wikel, S., and Thangamani, S. (2014). Murine cutaneous responses to the rocky mountain spotted fever vector, Dermacentor andersoni, feeding. Front. Microbiol. 5, 198. doi: 10.3389/fmicb.2014.00198
Hermance, M. E., and Thangamani, S. (2018). Tick–virus–host interactions at the cutaneous interface: the nidus of flavivirus transmission. Viruses 10, 362. doi: 10.3390/v10070362
Hernandez, E. P., Talactac, M. R., Fujisaki, K., and Tanaka, T. (2019). The case for oxidative stress molecule involvement in the tick-pathogen interactions-an omics approach. Dev. Comp. Immunol. 100, 103409. doi: 10.1016/j.dci.2019.103409
Holding, M., Dowall, S. D., Medlock, J. M., Carter, D. P., Pullan, S. T., Lewis, J., et al. (2020). Tick-borne encephalitis virus, United Kingdom. Emerg. Infect. Dis. 26, 90. doi: 10.3201/eid2601.191085
Hubálek, Z., and Rudolf, I. (2012). Tick-borne viruses in Europe. Parasitol. Res. 111, 9–36. doi: 10.1007/s00436-012-2910-1
Hughes, H. R., Velez, J. O., Fitzpatrick, K., Davis, E. H., Russell, B. J., Lambert, A. J., et al. (2021). Genomic evaluation of the genus coltivirus indicates genetic diversity among colorado tick fever virus strains and demarcation of a new species. Diseases 9, 92. doi: 10.3390/diseases9040092
Jelinski, J. W. (2016). Painless Hematophagy: The Functional Role of Novel Tick Metalloproteases in Pain Suppression.
Jore, M. M., Johnson, S., Sheppard, D., Barber, N. M., Li, Y. I., Nunn, M. A., et al. (2016). Structural basis for therapeutic inhibition of complement C5. Nat. Struct. Mol. Biol. 23, 378–386. doi: 10.1038/nsmb.3196
Kannangara, D. W., and Patel, P. (2018). Report of non-lyme, erythema migrans rashes from New Jersey with a review of possible role of tick salivary toxins. Vect. Borne Zoo. Dis. 18, 641–652. doi: 10.1089/vbz.2018.2278
Karim, S., Budachetri, K., Mukherjee, N., Williams, J., Kausar, A., Hassan, M. J., et al. (2017). A study of ticks and tick-borne livestock pathogens in Pakistan. PLoS Negl. Trop. Dis. 11, e0005681. doi: 10.1371/journal.pntd.0005681
Karim, S., and Ribeiro, J. M. C. (2015). An insight into the sialome of the lone star tick, Amblyommaamericanum, with a glimpse on its time dependent gene expression. PLoS ONE 10, e0131292. doi: 10.1371/journal.pone.0131292
Kaufman, W. R., and Sauer, J. R. (1982). “Ion and water balance in feeding ticks: mechanisms of tick excretion,” in Physiology of Ticks, eds F. D. Obenchain and R. Galun (London: Elsevier), 213–244.
Kazimírová, M., and Štibrániová, I. (2013). Tick salivary compounds: their role in modulation of host defences and pathogen transmission. Front. Cell. Infect. Microbiol. 4, 43. doi: 10.3389/fcimb.2013.00043
Kazimírová, M., Thangamani, S., Bartíková, P., Hermance, M., Holíková, V., Štibrániová, I., et al. (2017). Tick-borne viruses and biological processes at the tick-host-virus interface. Front. Cell. Infect. Microbiol. 7, 339. doi: 10.3389/fcimb.2017.00339
Kemal, J., Muktar, Y., and Alemu, S. (2016). Distribution and prevalence of tick infestation in cattle in Babille district, eastern Ethiopia. Livest. Res. Rural Dev. 28, 1–9. doi: 10.1155/2016/9618291
Kemp, D. H., and Tatchell, R. J. (1971). The mechanism of feeding and salivation in Boophilus microplus (Canestrini, 1887). Zeitschrift für Parasit. 37, 55–69. doi: 10.1007/BF00259545
Khoo, C. C. H., Piper, J., Sanchez-Vargas, I., Olson, K. E., and Franz, A. W. E. (2010). The RNA interference pathway affects midgut infection- and escape barriers for Sindbis virus in Aedes aegypti. BMC Microbiol. 10, 130. doi: 10.1186/1471-2180-10-130
Kim, D., Maldonado-Ruiz, P., Zurek, L., and Park, Y. (2017). Water absorption through salivary gland type I acini in the blacklegged tick, Ixodes scapularis. PeerJ. 5, e3984. doi: 10.7717/peerj.3984
Kim, D., Šimo, L., and Park, Y. (2014). Orchestration of salivary secretion mediated by two different dopamine receptors in the blacklegged tick Ixodes scapularis. J. Exp. Biol. 217, 3656–3663. doi: 10.1242/jeb.109462
Kim, T. K., Tirloni, L., Pinto, A. F. M., Moresco, J., Yates, J. R., da Silva Vaz, I., et al. (2016). Ixodes scapularis tick saliva proteins sequentially secreted every 24 h during blood feeding. PLoS Negl. Trop. Dis. 10, 1–35. doi: 10.1371/journal.pntd.0004323
Kitsou, C., Fikrig, E., and Pal, U. (2021). Tick host immunity: vector immunomodulation and acquired tick resistance. Trends Immunol. 42, 554–574. doi: 10.1016/j.it.2021.05.005
Klein, M., Brühl, T.-J., Staudt, V., Reuter, S., Grebe, N., Gerlitzki, B., et al. (2015). Tick salivary sialostatin L represses the initiation of immune responses by targeting IRF4-dependent transcription in murine mast cells. J. Immunol. 195, 621–631. doi: 10.4049/jimmunol.1401823
Koči, J., Šimo, L., and Park, Y. (2014). Autocrine/paracrine dopamine in the salivary glands of the blacklegged tick Ixodes scapularis. J. Insect. Physiol. 62, 39–45. doi: 10.1016/j.jinsphys.2014.01.007
Kotál, J., Langhansová, H., Lieskovská, J., Andersen, J. F., Francischetti, I. M. B., Chavakis, T., et al. (2015a). Modulation of host immunity by tick saliva. J. Proteomics 128, 58–68. doi: 10.116/j.jprot.2015.07.005
Kotál, J., Langhansová, H., Lieskovská, J., Andersen, J. F., Francischetti, I. M. B., Chavakis, T., et al. (2015b). Modulation of host immunity by tick saliva. J. Proteomics 128, 58–68. doi: 10.1016/j.jprot.2015.07.005
Kotsyfakis, M., Anderson, J. M., Andersen, J. F., Calvo, E., Francischetti, I. M. B., Mather, T. N., et al. (2008). Cutting edge: immunity against a “silent” salivary antigen of the lyme vector Ixodes scapularis impairs its ability to feed. J. Immunol. 181, 5209–5212. doi: 10.4049/jimmunol.181.8.5209
Kotsyfakis, M., Schwarz, A., Erhart, J., and Ribeiro, J. M. C. (2015). Tissue- and time-dependent transcription in ixodes ricinus salivary glands and midguts when blood feeding on the vertebrate host. Sci. Rep. 5, 9103. doi: 10.1038/srep09103
Kramer, L. D., and Tavakoli, N. P. (2021). Viruses of terrestrial mammals in Studies in Viral Ecology, ed S. J. Hurst (John Wiley and Sons Ltd), 541–583.
Krolak, J. M., Ownby, C. L., and Sauer, J. R. (1982). Alveolar structure of salivary glands of the lone star tick, Amblyommaamericanum (L.): unfed females. J. Parasitol. 68, 61–82. doi: 10.2307/3281326
Kurscheid, S., Lew-Tabor, A. E., Valle, M. R., Bruyeres, A. G., Doogan, V. J., Munderloh, U. G., et al. (2009). Evidence of a tick RNAi pathway by comparative genomics and reverse genetics screen of targets with known loss-of-function phenotypes in Drosophila. BMC Mol. Biol. 10, 26. doi: 10.1186/1471-2199-10-26
Labuda, M., Jones, L. D., Williams, T., and Nuttall, P. A. (1993). Enhancement of tick-borne encephalitis virus transmission by tick salivary gland extracts. Med. Vet. Entomol. 7, 193–196. doi: 10.1111/j.1365-2915.1993.tb00674.x
Latif, A. A., Putterill, J. F., de Klerk, D. G., Pienaar, R., and Mans, B. J. (2012). Nuttalliellanamaqua (Ixodoidea: Nuttalliellidae): first description of the male, immature stages and re-description of the female. PLoS ONE 7, e0041651. doi: 10.1371/journal.pone.0041651
Leal, B. F., Alzugaray, M. F., Seixas, A., Da Silva Vaz, I., and Ferreira, C. A. S. (2018). Characterization of a glycine-rich protein from rhipicephalus microplus: tissue expression, gene silencing and immune recognition. Parasitology 145, 927–938. doi: 10.1017/S0031182017001998
Lee, W.-S., Webster, J. A., Madzokere, E. T., Stephenson, E. B., and Herrero, L. J. (2019). Mosquito antiviral defense mechanisms: a delicate balance between innate immunity and persistent viral infection. Parasit. Vect. 12, 165. doi: 10.1186/s13071-019-3433-8
Lemasson, M., Caignard, G., Unterfinger, Y., Attoui, H., Bell-Sakyi, L., Hirchaud, E., et al. (2021). Exploration of binary protein–protein interactions between tick-borne flaviviruses and Ixodes ricinus. Parasit. Vect. 14, 1–17. doi: 10.1186/s13071-021-04651-3
Lledó, L., Giménez-Pardo, C., and Gegúndez, M. I. (2020). Epidemiological study of thogoto and dhori virus infection in people bitten by ticks, and in sheep, in an area of Northern Spain. Int. J. Environ. Res. Public Health 17, 2254. doi: 10.3390/ijerph17072254
Madani, T. A., and Abuelzein, E. T. M. E. (2021). Alkhumra hemorrhagic fever virus infection. Arch. Virol. 166, 2357–2367. doi: 10.1007/s00705-021-05083-1
Manjunathachar, H. V., Saravanan, B. C., Kesavan, M., Karthik, K., Rathod, P., Gopi, M., et al. (2014). Economic importance of ticks and their effective control strategies. Asian Pac. J. Trop. Dis. 4, S770–S779. doi: 10.1016/S2222-1808(14)60725-8
Mans, B. J., De Castro, M. H., Pienaar, R., De Klerk, D., Gaven, P., Genu, S., et al. (2016). Ancestral reconstruction of tick lineages. Ticks Tick. Borne. Dis. 7, 509–535. doi: 10.1016/j.ttbdis.2016.02.002
Mans, B. J., Gothe, R., and Neitz, A. W. H. (2004). Biochemical perspectives on paralysis and other forms of toxicoses caused by ticks. Parasitol. 129, 95-111. doi: 10.1017/S0031182003004670
Mans, B. J., and Neitz, A. W. H. (2004). Adaptation of ticks to a blood-feeding environment: evolution from a functional perspective. Insect Biochem. Mol. Biol. 34, 1–17. doi: 10.1016/j.ibmb.2003.09.002
Mansfield, K. L., Jizhou, L., Phipps, L. P., and Johnson, N. (2017). Emerging tick-borne viruses in the twenty-first century. Front. Cell. Infect. Microbiol. 7, 298. doi: 10.3389/fcimb.2017.00298
Martina, B. E., Barzon, L., Pijlman, G. P., de la Fuente, J., Rizzoli, A., Wammes, L. J., et al. (2017). Human to human transmission of arthropod-borne pathogens. Curr. Opin. Virol. 22, 13–21. doi: 10.1016/j.coviro.2016.11.005
Mccoy, K. D., Léger, E., and Dietrich, M. (2013). Host specialization in ticks and transmission of tick-borne diseases: a review. Front. Cell. Infect. Microbiol. 3, 57. doi: 10.3389/fcimb.2013.00057
McNally, K. L., and Bloom, M. E. (2013). “The Tick-Virus Interface” in Viral Infection Global Change, ed S. K. Singh (John Wiley and Sons Ltd), 603–616.
Medlock, J. M., Hansford, K. M., Bormane, A., Derdakova, M., Estrada-peña, A., George, J., et al. (2013). Artikel ixodes ricinus Europa, 2013. Parasit. Vect. 6, 1. doi: 10.1186/1756-3305-6-1
Meekins, D. A., Kanost, M. R., and Michel, K. (2017). Serpins in arthropod biology. Semi. Cell.Dev. Biol. 62, 105–119. doi: 10.1016/j.semcdb.2016.09.001
Migné, C. V., Hönig, V., Bonnet, S. I., Palus, M., Rakotobe, S., Galon, C., et al. (2022). Evaluation of two artificial infection methods of live ticks as tools for studying interactions between tick-borne viruses and their tick vectors. Sci. Rep. 12, 491. doi: 10.1038/s41598-021-04498-9
Mirkena, T., Walelign, E., Tewolde, N., Gari, G., Abebe, G., and Newman, S. (2018). Camel production systems in Ethiopia: a review of literature with notes on MERS-CoV risk factors. Pastoralism 8, 30. doi: 10.1186/s13570-018-0135-3
Moming, A., Yue, X., Shen, S., Chang, C., Wang, C., Luo, T., et al. (2018). Prevalence and phylogenetic analysis of crimean-congo hemorrhagic fever virus in ticks from different ecosystems in Xinjiang, China. Virol. Sin. 33, 67–73. doi: 10.1007/s12250-018-0016-3
Monfared, A. L., Mahmoodi, M., and Fattahi, R. (2015). Prevalence of ixodid ticks on cattle, sheep and goats in Ilam County, Ilam Province, Iran. J. Parasit. Dis. 39, 37–40. doi: 10.1007/s12639-013-0267-8
Morozova, O. V., Panov, V. V., and Bakhvalova, V. N. (2020). Innate and adaptive immunity in wild rodents spontaneously and experimentally infected with the tick-borne encephalitis virus. Infect. Genet. Evol. 80, 104187. doi: 10.1016/j.meegid.2020.104187
Mudenda, L., Pierlé, S. A., Turse, J. E., Scoles, G. A., Purvine, S. O., Nicora, C. D., et al. (2014). Proteomics informed by transcriptomics identifies novel secreted proteins in Dermacentor andersoni saliva. Int. J. Parasitol. 44, 1029–1037. doi: 10.1016/j.ijpara.2014.07.003
Mulenga, A., Kim, T., and Ibelli, A. M. G. (2013). A mblyomma americanum tick saliva serine protease inhibitor 6 is a cross-class inhibitor of serine proteases and papain-like cysteine proteases that delays plasma clotting and inhibits platelet aggregation. Insect Mol. Biol. 22, 306–319. doi: 10.1111/imb.12024
Mutavi, F., Aarts, N., Van Paassen, A., Heitkönig, I., and Wieland, B. (2018). Techne meets Metis: Knowledge and practices for tick control in Laikipia County, Kenya. NJAS Wageningen J. Life Sci. 86–87, 136–145. doi: 10.1016/j.njas.2018.08.001
Nazar, M., Khan, M. N. M. A. M. A. Q. S. N. Q. S. A., Shah, A. A., Rahman, S. U., Khan, I. I. U. I. I., Ullah, A., et al. (2013). Prevalence and risk factors of anaplasmosis in cattle and buffalo populations of district Khanewal, Punjab, Pakistan. Glob. Vet. 22, 146–153. doi: 10.1590/s1984-29612013000200038
Neelakanta, G., and Sultana, H. (2022). Tick saliva and salivary glands: what do we know so far on their role in arthropod blood feeding and pathogen transmission. Front. Cell. Infect. Microbiol. 11, 816547. doi: 10.3389/fcimb.2021.816547
Niu, J., Taning, C. N. T., Christiaens, O., Smagghe, G., and Wang, J.-J. (2018). “Rethink RNAi in insect pest control: challenges and perspectives.” Adv. Ins. Physiol. 55, 1–17. doi: 10.1016/bs.aiip.2018.07.003
Nodari, E. F., Roma, G. C., Furquim, K. C. S., De Oliveira, P. R., Bechara, G. H., and Camargo-Mathias, M. I. (2012). Degenerative process and cell death in salivary glands of Rhipicephalus sanguineus (Latreille, 1806) (Acari: Ixodidae) semi-engorged female exposed to the acaricide permethrin. Microsc. Res. Tech. 75, 1012–1018. doi: 10.1002/jemt.22025
Nuttall, P. A. (2019a). Tick saliva and its role in pathogen transmission. Wien. Klin. Wochenschr. 131, 1–12. doi: 10.1007/s00508-019-1500-y
Nuttall, P. A. (2019b). Wonders of tick saliva. Ticks Tick. Borne. Dis. 10, 470–481. doi: 10.1016/j.ttbdis.2018.11.005
Páleníková, J., Lieskovská, J., Langhansová, H., Kotsyfakis, M., Chmelar, J., and Kopecký, J. (2015). Ixodes ricinus salivary serpin IRS-2 affects Th17 differentiation via inhibition of the interleukin-6/STAT-3 signaling pathway. Infect. Immun. 83, 1949–1956. doi: 10.1128/IAI.03065-14
Palta, S., Saroa, R., and Palta, A. (2014). Overview of the coagulation system. Indian. J. Anaesth. 58, 515–523. doi: 10.4103/0019-5049.144643
Pantchev, N., Pluta, S., Huisinga, E., Nather, S., Scheufelen, M., Vrhovec, M. G., et al. (2015). Tick-borne diseases (borreliosis, anaplasmosis, babesiosis) in German and Austrian dogs: status quo and review of distribution, transmission, clinical findings, diagnostics and prophylaxis. Parasitol. Res. 114, 19–54. doi: 10.1007/s00436-015-4513-0
Papa, A., Tsioka, K., Kontana, A., Papadopoulos, C., and Giadinis, N. (2017). Bacterial pathogens and endosymbionts in ticks. Ticks Tick. Borne. Dis. 8, 31–35. doi: 10.1016/j.ttbdis.2016.09.011
Parizi, L. F., Ali, A., Tirloni, L., Oldiges, D. P., Sabadin, G. A., Coutinho, M. L., et al. (2018). Peptidase inhibitors in tick physiology. Med. Vet. Entomol. 32, 129–144. doi: 10.1111/mve.12276
Pekáriková, D., Rajská, P., Kazimírová, M., Pechánová, O., Takáč, P., and Nuttall, P. A. (2015). Vasoconstriction induced by salivary gland extracts from ixodid ticks. Int. J. Parasitol. 45, 879–883. doi: 10.1016/j.ijpara.2015.08.006
Perner, J., Kropáčková, S., Kopáček, P., and Ribeiro, J. M. C. (2018). Sialome diversity of ticks revealed by RNAseq of single tick salivary glands. PLoS Negl. Trop. Dis. 12, e0006410. doi: 10.1371/journal.pntd.0006410
Pfäffle, M., Littwin, N., Muders, S. V., and Petney, T. N. (2013). The ecology of tick-borne diseases. Int. J. Parasitol. 43, 1059–1077. doi: 10.1016/j.ijpara.2013.06.009
Preston, S. G., Majtán, J., Kouremenou, C., Rysnik, O., Burger, L. F., Cabezas Cruz, A., et al. (2013). Novel immunomodulators from hard ticks selectively reprogramme human dendritic cell responses. PLoSPathog. 9, e1003450. doi: 10.1371/journal.ppat.1003450
Raney, W. R., Perry, J. B., and Hermance, M. E. (2022). Transovarial transmission of heartland virus by invasive asian longhorned ticks under laboratory conditions. Emerg. Infect. Dis. 28, 726. doi: 10.3201/eid2803.210973
Rashid, M., Rashid, M. I., Akbar, H., Ahmad, L., Hassan, M. A., Ashraf, K., et al. (2019). A systematic review on modelling approaches for economic losses studies caused by parasites and their associated diseases in cattle. Parasitology 146, 129–141. doi: 10.1017/S0031182018001282
Rego, R. O. M., Trentelman, J. J. A., Anguita, J., Nijhof, A. M., Sprong, H., Klempa, B., et al. (2019). Counterattacking the tick bite: towards a rational design of anti-tick vaccines targeting pathogen transmission. Parasit. Vect. 12, 229. doi: 10.1186/s13071-019-3468-x
Reid, H. W. (2019). “Louping-ill,” in The Arboviruses: Epidemiology and Ecology, ed T. P. Monath (London: CRC Press), 117–136.
Rock, D. L. (2021). Thoughts on African swine fever vaccines. Viruses 13, 943. doi: 10.3390/v13050943
Rodriguez, S. E., McAuley, A. J., Gargili, A., and Bente, D. A. (2018). Interactions of human dermal dendritic cells and langerhans cells treated with hyalomma tick saliva with crimean-congo hemorrhagic fever virus. Viruses 10, 381. doi: 10.3390/v10070381
Rodriguez-Vivas, R. I., Jonsson, N. N., and Bhushan, C. (2018). Strategies for the control of Rhipicephalus microplus ticks in a world of conventional acaricide and macrocyclic lactone resistance. Parasitol. Res. 117, 3–29. doi: 10.1007/s00436-017-5677-6
Rosà, R., Andreo, V., Tagliapietra, V., Baráková, I., Arnoldi, D., Hauffe, H. C., et al. (2018). Effect of climate and land use on the spatio-temporal variability of tick-borne bacteria in Europe. Int. J. Environ. Res. Public Health 15, 732. doi: 10.3390/ijerph15040732
Rosche, K. L., Sidak-Loftis, L. C., Hurtado, J., Fisk, E. A., and Shaw, D. K. (2021). Arthropods under pressure: Stress responses and immunity at the pathogen-vector interface. Front. Immunol. 11, 629777. doi: 10.3389/fimmu.2020.629777
Roshdy, M. A., and Coons, L. B. (1975). The subgenus persicargas (Ixodoidea: Argasidae: Argas). 23. Fine structure of the salivary glands of unfed A. (P.) arboreus Kaiser, Hoogstraal, and Kohls. J. Parasitol. 61, 743–752. doi: 10.2307/3279478
Sajid, M. S., Iqbal, Z., Khan, M. N., Muhamamd, G., and Iqbal, M. U. (2007). Effect of hyalomma ticks (Acari: Ixodidae) on milk production of dairy buffaloes (Bos bubalus bubalis) of Punjab (Pakistan). Ital. J. Anim. Sci. 6, 939–941. doi: 10.4081/ijas.2007.s2.939
Sajid, M. S., Iqbal, Z., Shamim, A., Siddique, R. M., Hassan, M. J., and Rizwan, H. M. (2017). Distribution and abundance of ticks infesting livestock population along Karakorum highway from Mansehra to Gilgit, Pakistan. J. Hell. Vet. Med. Soc. 68, 51–58. doi: 10.12681/jhvms.15556
Schuijt, T. J., Bakhtiari, K., Daffre, S., Deponte, K., Wielders, S. J. H., Marquart, J. A., et al. (2013). Factor XA activation of factor v is of paramount importance in initiating the coagulation system: lessons from a tick salivary protein. Circulation 128, 254–266. doi: 10.1161/CIRCULATIONAHA.113.003191
Schwarz, A., Valdés, J. J., and Kotsyfakis, M. (2012). The role of cystatins in tick physiology and blood feeding. Ticks Tick. Borne. Dis. 3, 117–127. doi: 10.1016/j.ttbdis.2012.03.004
Shi, J., Hu, Z., Deng, F., and Shen, S. (2018). Tick-borne viruses. Virol. Sin. 33, 21–43. doi: 10.1007/s12250-018-0019-0
Siddiqi, M. K., Shahein, Y. E., Hussein, N., and Khan, R. H. (2016). Effect of surfactants on Ra-sHSPI–A small heat shock protein from the cattle tick Rhipicephalus annulatus. J. Mol. Struct. 1119, 12–17. doi: 10.1016/j.molstruc.2016.04.002
Siddique, R. M., Sajid, M. S., Iqbal, Z., and Saqib, M. (2020). Association of different risk factors with the prevalence of babesiosis in cattle and buffalos. Pakistan J. Agric. Sci. 57, 517–524. doi: 10.21162/PAKJAS/19.8626
Sidorenko, M., Radzijevskaja, J., Mickevičius, S., Bratčikoviene, N., and Paulauskas, A. (2021). Prevalence of tick-borne encephalitis virus in questing Dermacentor reticulatus and Ixodes ricinus ticks in Lithuania. Ticks Tick. Borne. Dis. 12, 101594. doi: 10.1016/j.ttbdis.2020.101594
Šimo, L., Kazimirova, M., Richardson, J., and Bonnet, S. I. (2017). The essential role of tick salivary glands and saliva in tick feeding and pathogen transmission. Front. Cell. Infect. Microbiol. 7, 281. doi: 10.3389/fcimb.2017.00281
Šimo, L., Koči, J., Kim, D., and Park, Y. (2014). Invertebrate specific D1-like dopamine receptor in control of salivary glands in the black-legged tick Ixodes scapularis. J. Comp. Neurol. 522, 2038–2052. doi: 10.1002/cne.23515
Šimo, L., Koči, J., and Park, Y. (2013). Receptors for the neuropeptides, myoinhibitory peptide and SIFamide, in control of the salivary glands of the blacklegged tick Ixodes scapularis. Insect Biochem. Mol. Biol. 43, 376–387. doi: 10.1016/j.ibmb.2013.01.002
Singh, N. K., Goolsby, J. A., Shapiro-Ilan, D. I., Miller, R. J., Setamou, M., and de Leon, A. A. P. (2018). Effect of immersion time on efficacy of entomopathogenic nematodes against engorged females of cattle fever tick, rhipicephalus (= boophilus) microplus. Southwest. Entomol. 43, 19–28. doi: 10.3958/059.043.0120
Solano-Gallego, L., Sainz, Á., Roura, X., Estrada-Peña, A., and Miró, G. (2016). A review of canine babesiosis: the European perspective. Parasit. Vect. 9, 336. doi: 10.1186/s13071-016-1596-0
Sormunen, J. J., Sippola, E., Kaunisto, K. M., Vesterinen, E. J., and Sääksjärvi, I. E. (2019). First evidence of ixodiphagushookeri (Hymenoptera: Encyrtidae) parasitization in finnish castor bean ticks (Ixodes ricinus). Exp. Appl. Acarol. 79, 395–404. doi: 10.1007/s10493-019-00437-6
Soulsby, E. J. L. (1982). Helminths, Arthropods and Protozoa of Domesticated Animals. 7th Edn. London: Bailliere Tindall.
Sprygin, A., Pestova, Y., Wallace, D. B., Tuppurainen, E., and Kononov, A. V. (2019). Transmission of lumpy skin disease virus: a short review. Virus Res. 269, 197637. doi: 10.1016/j.virusres.2019.05.015
Štibrániová, I., Lahová, M., and Bartíková, P. (2013). Immunomodulators in tick saliva and their benefits. Acta Virol. 57, 200–216. doi: 10.4149/av_2013_02_200
Sudhakar, N. R., Manjunathachar, H. V., Karthik, K., Sahu, S., Gopi, M., Shanthaveer, S. B., et al. (2013). RNA interference in parasites: prospects and pitfalls. Adv Anim Vet Sci 1, 1–6.
Suppan, J., Engel, B., Marchetti-Deschmann, M., and Nürnberger, S. (2018). Tick attachment cement–reviewing the mysteries of a biological skin plug system. Biol. Rev. 93, 1056–1076. doi: 10.1111/brv.12384
Talactac, M. R., Hernandez, E. P., Hatta, T., Yoshii, K., Kusakisako, K., Tsuji, N., et al. (2021). The antiviral immunity of ticks against transmitted viral pathogens. Dev. Comp. Immunol. 119, 104012. doi: 10.1016/j.dci.2021.104012
Tang, J., Fang, Y., Han, Y., Bai, X., Yan, X., Zhang, Y., et al. (2015). YY-39, a tick anti-thrombosis peptide containing RGD domain. Peptides 68, 99–104. doi: 10.1016/j.peptides.2014.08.008
Thompson, R. E., Liu, X., Ripoll-Rozada, J., Alonso-García, N., Parker, B. L., Pereira, P. J. B., et al. (2017). Tyrosine sulfation modulates activity of tick-derived thrombin inhibitors. Nat. Chem. 9, 909–917. doi: 10.1038/nchem.2744
Tian, Y., Chen, W., Mo, G., Chen, R., Fang, M., Yedid, G., et al. (2016). An immunosuppressant peptide from the hard tick Amblyomma variegatum. Toxins 8, 133. doi: 10.3390/toxins8050133
Tirloni, L., Kim, T. K., Pinto, A. F. M., Yates, J. R., da Silva Vaz, I., and Mulenga, A. (2017). Tick-host range adaptation: changes in protein profiles in unfed adult ixodes scapularis and Amblyomma americanum saliva stimulated to feed on different hosts. Front. Cell. Infect. Microbiol. 7, 517. doi: 10.3389/fcimb.2017.00517
Tirloni, L., Reck, J., Terra, R. M. S., Martins, J. R., Mulenga, A., Sherman, N. E., et al. (2014). Proteomic analysis of cattle tick rhipicephalus (boophilus) microplus saliva: a comparison between partially and fully engorged females. PLoS ONE 9, e94831. doi: 10.1371/journal.pone.0094831
Tomás-Cortázar, J., Martín-Ruiz, I., Barriales, D., Pascual-Itoiz, M. Á., De Juan, V. G., Caro-Maldonado, A., et al. (2017). The immunosuppressive effect of the tick protein, Salp15, is long-lasting and persists in a murine model of hematopoietic transplant. Sci. Rep. 7, 10740. doi: 10.1038/s41598-017-11354-2
Tsatsaris, A., Chochlakis, D., Papadopoulos, B., Petsa, A., Georgalis, L., Angelakis, E., et al. (2016). Species composition, distribution, ecological preference and host association of ticks in Cyprus. Exp. Appl. Acarol. 70, 523–542. doi: 10.1007/s10493-016-0091-9
Turell, M. J. (2020). “Horizontal and vertical transmission of viruses by insect and tick vectors,” in The Arboviruses: Epidemiology and Ecology, ed T. P. Monath (London: CRC Press), 127–152.
Ullah, S. A. K. M., and Kaufman, W. R. (2014). Salivary gland degeneration and ovarian development in the Rocky Mountain wood tick, Dermacentor andersoni stiles (Acari: Ixodidae): I. Post-engorgement events. Ticks Tick. Borne. Dis. 5, 569–574. doi: 10.1016/j.ttbdis.2014.03.012
Uslu, U., Sajid, M. S., Ceylan, O., and Ejaz, A. (2019). Prevalence of hard ticks (Acari: Ixodidae) in spur-thighed tortoise (Testudo graecaibera) population of Konya. Eurasian J. Vet. Sci. 35, 158–164. doi: 10.15312/EurasianJVetSci.2019.239
Valdés, J. J. (2014). Antihistamine response: a dynamically refined function at the host-tick interface. Parasit. Vect. 7, 491. doi: 10.1186/s13071-014-0491-9
Vander Waal, K., Gilbertson, M., Okanga, S., Allan, B. F., and Craft, M. E. (2017). Seasonality and pathogen transmission in pastoral cattle contact networks. R. Soc. Open Sci. 4, 170808. doi: 10.1098/rsos.170808
Wernersson, S., and Pejler, G. (2014). Mast cell secretory granules: armed for battle. Nat. Rev. Immunol. 14, 478–494. doi: 10.1038/nri3690c
Wikel, S. (2013). Ticks and tick-borne pathogens at the cutaneous interface: Host defenses, tick countermeasures, and a suitable environment for pathogen establishment. Front. Microbiol. 4, 337. doi: 10.3389/fmicb.2013.00337
Wikel, S. K. (2018a). Tick-host-pathogen systems immunobiology: an interactive trio. Front. Biosci. Landmark 23, 265–283. doi: 10.2741/4590
Wikel, S. K. (2018b). Ticks and tick-borne infections: complex ecology, agents, and host interactions. Vet. Sci. 5, 60. doi: 10.3390/vetsci5020060
Yadav, P. D., Whitmer, S. L. M., Sarkale, P., Fei Fan Ng, T., Goldsmith, C. S., Nyayanit, D. A., et al. (2019). Characterization of novel reoviruses wad medani virus (orbivirus) and kundal virus (coltivirus) collected from hyalomma anatolicum ticks in india during surveillance for crimean congo hemorrhagic fever. J. Virol. 93, e00106–e00119. doi: 10.1128/JVI.00106-19
Yang, X., Gao, G. F., and Liu, W. J. (2022). Powassan virus: a tick borne flavivirus infecting humans. Biosaf. Heal. 4, 30–37. doi: 10.1016/j.bsheal.2021.12.007
Yoshii, K. (2019). Epidemiology and pathological mechanisms of tick-borne encephalitis. J. Vet. Med. Sci. 81, 343–347. doi: 10.1292/jvms.18-0373
Yu, X., Zhou, Y., Cao, J., Zhang, H., Gong, H., and Zhou, J. (2017). Caspase-1 participates in apoptosis of salivary glands in Rhipicephalus haemaphysaloides. Parasit. Vect. 10, 225. doi: 10.1186/s13071-017-2161-1
Yun, S. M., Song, B. G., Choi, W., Roh, J. Y., Lee, Y. J., Park, W., et al. (2016). First Isolation of severe fever with thrombocytopenia syndrome virus from haemaphysalislongicornis ticks collected in severe fever with thrombocytopenia syndrome outbreak areas in the Republic of Korea. Vect. Borne Zoo. Dis. 16, 66–70. doi: 10.1089/vbz.2015.1832
Keywords: ticks, immunity, tick-virus interaction, tick microbes, salivary glands
Citation: Maqbool M, Sajid MS, Saqib M, Anjum FR, Tayyab MH, Rizwan HM, Rashid MI, Rashid I, Iqbal A, Siddique RM, Shamim A, Hassan MA, Atif FA, Razzaq A, Zeeshan M, Hussain K, Nisar RHA, Tanveer A, Younas S, Kamran K and Rahman Su (2022) Potential Mechanisms of Transmission of Tick-Borne Viruses at the Virus-Tick Interface. Front. Microbiol. 13:846884. doi: 10.3389/fmicb.2022.846884
Received: 31 December 2021; Accepted: 18 March 2022;
Published: 05 May 2022.
Edited by:
Ali Zohaib, Islamia University of Bahawalpur, PakistanReviewed by:
Khalid Mehmood, Islamia University of Bahawalpur, PakistanCopyright © 2022 Maqbool, Sajid, Saqib, Anjum, Tayyab, Rizwan, Rashid, Rashid, Iqbal, Siddique, Shamim, Hassan, Atif, Razzaq, Zeeshan, Hussain, Nisar, Tanveer, Younas, Kamran and Rahman. This is an open-access article distributed under the terms of the Creative Commons Attribution License (CC BY). The use, distribution or reproduction in other forums is permitted, provided the original author(s) and the copyright owner(s) are credited and that the original publication in this journal is cited, in accordance with accepted academic practice. No use, distribution or reproduction is permitted which does not comply with these terms.
*Correspondence: Muhammad Sohail Sajid, ZHJzb2hhaWx1YWYmI3gwMDA0MDtob3RtYWlsLmNvbQ==