- 1Macrophage Lab, Department of Microbiology and Immunology, Institute of Endemic Disease, Seoul National University College of Medicine, Seoul, South Korea
- 2National Primate Research Center, Korea Research Institute of Bioscience and Biotechnology, Cheongju-si, South Korea
- 3KRIBB School of Bioscience, Korea University of Science & Technology (UST), Daejeon, Korea
- 4Transdisciplinary Department of Medicine and Advanced Technology, Seoul National University Hospital, Seoul, South Korea
- 5Department of Biomedical Sciences, Seoul National University College of Medicine, Seoul, South Korea
Re-emerging viral threats have continued to challenge the medical and public health systems. It has become clear that a significant number of severe viral infection cases are due to an overreaction of the immune system, which leads to hyperinflammation. In this study, we aimed to demonstrate the therapeutic efficacy of the dexamethasone nanomedicine in controlling the symptoms of influenza virus infection. We found that the A/Wisconsin/WSLH34939/2009 (H1N1) infection induced severe pneumonia in mice with a death rate of 80%, accompanied by significant epithelial cell damage, infiltration of immune cells, and accumulation of pro-inflammatory cytokines in the airway space. Moreover, the intranasal delivery of liposomal dexamethasone during disease progression reduced the death rate by 20%. It also significantly reduced the protein level of tumor necrosis factor-alpha (TNFα), interleukin-1β (IL-1β), IL-6, and the C-X-C motif chemokine ligand 2 (CXCL2) as well as the number of infiltrated immune cells in the bronchoalveolar lavage fluids as compared to the control and free dexamethasone. The liposomal dexamethasone was mainly distributed into the monocyte/macrophages as a major cell population for inducing the cytokine storm in the lungs. Taken together, the intranasal delivery of liposomal dexamethasone may serve as a novel promising therapeutic strategy for the treatment of influenza A-induced pneumonia.
Introduction
Lower respiratory tract infections account for approximately 7% of infection-related deaths per year worldwide, and viruses are a common cause of community-acquired pneumonia (Li et al., 2004; Russell and Webster, 2005; Shieh et al., 2010; Worobey et al., 2014). The 2009 H1N1 influenza virus caused more than 18,000 confirmed deaths due to lung damage in the United States (Itoh et al., 2009; Walsh et al., 2011). These infections are accompanied by aggressive pro-inflammatory responses along with insufficient control of the anti-inflammatory responses, a combination of events that is referred to as the “cytokine storm (Peiris et al., 2010; To et al., 2010).”
Following the primary exposure of respiratory epithelial cells to the influenza virus, progeny viruses that replicate within these cells can infect other cells, including alveolar macrophages (Bender and SmallJr., 1992; La Gruta et al., 2007; Liu et al., 2016). The inflammatory response begins when the pathogen-associated molecular pattern (PAMP) of the virus is recognized by pattern recognition receptors (PRRs) of innate immune cells (Julkunen et al., 2000; Julkunen et al., 2001; Oslund and Baumgarth, 2011). Specific pro-inflammatory cytokines are expressed and lead to the recruitment of neutrophils, monocytes, macrophages, and T cells into the site of infection (La Gruta et al., 2007; Liu et al., 2016). Hyperinflammation and macrophage activation syndrome (MAS) result in the overproduction of proinflammatory cytokines, such as the IL-1β, IL-6, and TNFα, as well as coagulation abnormalities, which contribute to organ failure and other fatalities (Shinya et al., 2012; Merad and Martin, 2020; Tay et al., 2020). Therefore, the reduction of pro-inflammatory cytokine levels is as important as anti-viral therapies. Since monocytes and macrophages are the main cells that secrete pro-inflammatory cytokines, the efficient control of these cells can be used as a therapeutic target to alleviate inflammation.
Corticosteroids are a class of steroid hormones that exhibit anti-inflammatory activity by binding to the cytoplasmic corticosteroid receptor, which regulates the transcription of anti-inflammatory genes (Rocksen et al., 2000; Darwish et al., 2011). As the outcome of severe influenza is determined by both viral virulence and host resistance, the use of immunomodulatory therapy in combination with conventional antiviral therapy is highly warranted. During the 2009 H1N1 influenza pandemic, nearly 40% of patients in France were treated for ARDS using adjuvant systemic corticosteroids (Brun-Buisson et al., 2011); however, the evidence supporting the use of corticosteroids in severe influenza was unclear. Because immunosuppression resulting from systemic administration of dexamethasone can favor persistent viral replication and further limiting host defenses (Giannella et al., 2011). However, Lammers et al. (2020) recently proposed that targeting alveolar macrophages via the nano-formulation of dexamethasone within liposomes improve the management of respiratory viral infections. Liposomes, microscopic phospholipid bubbles with a bilayered membrane structure, have been successfully applied in clinics as drug carriers to improve the delivery of drugs to target cells and tissues that play a key role in the acute and progressive phases of many diseases (Torchilin, 2005). Although dexamethasone nanomedicine has been used as a therapeutic agent for the coronavirus disease 2019 (COVID-19) (Lammers et al., 2020), it has not yet been tested for the influenza virus infection in pre-clinical studies.
In this study, we demonstrated the therapeutic efficacy of liposomal dexamethasone (DEX/lipo) in a mouse model of influenza virus infection. We manufactured DEX/lipo that can specifically delivery into monocytes and macrophages and found that DEX/lipo outperformed the free dexamethasone in reducing the morbidity and mortality caused by the A/H1N1 influenza virus infection. Therefore, our findings offer valuable insights into the efficiency of the currently available corticosteroids for the treatment of influenza virus infection via the targeted delivery of these drugs.
Materials and Methods
Mice, Virus, and Cell Line
Male C57BL/6J mice of 7-weeks-of-age were purchased from ORIENT BIO (Orient Bio, Gyeonggi-do, South Korea). Animal experiments were conducted at the Institute for Experimental Animals, College of Medicine and cared for according to the Guide for the Care and Use of Laboratory Animals prepared by the Institutional Animal Care and Use Committee of Seoul National University at Seoul (accession number SNU-160307-6-1). Mice were housed in cages with a constant-flow air exchange, supporting specific pathogen-free conditions. Influenza A/Wisconsin/WSLH34939/09 was obtained from the Michael laboratory at the Scripps Research Institute. The adaptation method for influenza A virus in mouse lungs is as follows. The C57BL/6J mice were each anesthetized with isoflurane (HANA PHARM CO., Gyeonggi-do, South Korea), intranasally inoculated with 105 TCID50 of influenza A/Wisconsin/WSLH34939/09 virus in a volume of 20 μl. At day 2 post-infection, the lungs from mice were homogenized in the Dulbecco’s modified Eagle medium (DMEM) medium with 10% fetal bovine serum (FBS) and 1% penicillin-streptomycin (PS), centrifuged at 9,520 × g for 10 min, and the supernatant was stored at −80°C. The first adapted virus was amplified on the MDCK cells and virus titer detected using TCID50. Briefly, the assay was performed by adding a serial dilution of the virus sample to cells in a 96-well plate. After incubation, the percentage of infected wells was observed for each dilution, and the results were used to calculate the TCID50 value. This calculation can generally be performed using the Spearman-Karber method. MDCK cells were cultured in DMEM supplemented with 10% FBS and 1% PS.
Mice under isoflurane anesthesia were infected intranasally with 105 TCID50 of the adapted influenza A virus. One hour after infection, the mice were anesthetized by isoflurane inhalation for the intranasal delivery of vehicle (20 μl of PBS/lipo) or dexamethasone (30 μg/kg dissolved in PBS) or DEX/lipo (30 μg/kg encapsulated in liposomes) for 3 days. On day 3 post-infection, the mice were injected intravenously with 100 μl of PBS/lipo, dexamethasone, or DEX/lipo. The mice monitored for survival.
Reagents
Dexamethasone were purchased from Sigma (Sigma, St. Louis, MO, United States).
Preparation of Liposomes
Dexamethasone was encapsulated into liposomes with a diameter of 1,000 nm at a concentration of 1 mg/ml. Briefly, L-alpha-phosphatidylcholine (Sigma, St. Louis, MO, United States) and cholesterol (Sigma, St. Louis, MO, United States) were dissolved in a 2:1 mixture of chloroform and methanol and dried under a nitrogen stream, followed by a vacuum pump. The lipid film was hydrolyzed with PBS buffer and sized to 1,000 nm in a mini-extruder equipped with a 1,000 nm pore polycarbonate membrane (Avanti Polar Lipids, Alabama, AL, United States). The diameter of liposomes was checked by dynamic light scattering (DLS). Liposomes were labeled with DiI (Molecular Probes, Eugene, OR, United States) for visual detection.
Flow Cytometry
The mice lungs were obtained at 24 h post-injection with DiI-stained liposomes and digested with collagenase type I (Sigma, St. Louis, MO, United States) to obtain single cells. Single cells were prepared for flow cytometric analysis. Anti-mouse CD16/32 antibody (clone number 93) was pre-added to block the non-specific binding of immunoglobulin to macrophage Fc receptors. For surface marker analysis, live cells were re-suspended in staining buffer [1% bovine serum albumin (BSA), 5 mM ethylenediaminetetraacetic acid (EDTA), and 0.1% NaN3 in PBS] and stained with anti-mouse CD45 (30-F11), F4/80 (BM8), CD11b (M1/70), CD3 (145-2C11), CD64 (X54-5/7.1; BD Biosciences), Ly6C (HK1.4), and Ly6G (1A8-Ly6g) antibodies at 4°C for 20 min. All antibodies were obtained from eBioscience, unless otherwise indicated. Data were acquired using LSR Fortessa (BD Biosciences) and analyzed using the FlowJo software (FlowJo LCC, Ashland, OR, United States).
Immunofluorescence Analysis
The mice lungs were obtained at 24 h post-injection with DiI-stained liposomes. Frozen tissues were sectioned to a thickness of 5 μm and fixed with 4% paraformaldehyde (Merck, Kenilworth, NJ, United States). Slides were incubated with 1% BSA in PBST for 1 h to block the non-specific antibody binding. Primary antibodies were pre-diluted in blocking buffer to 1:200 for F4/80 (CI-A3-1; Abcam) or EpCAM (G8.8; eBioscience) and applied to tissue sections overnight at 4°C in a humidified chamber. The next day, appropriate secondary antibodies were applied, and nuclei were stained with 4,6-diamidino-2-phenylindole (DAPI; Invitrogen, Waltham, MA, United States) before mounting. Fluorescence signals were detected using a Leica TCS SP8 confocal microscope (Leica, Buffalo Grove, IL, United States).
Cytokine and Chemokine Analyses
On day 2 post-infection, the trachea of euthanized mice was exposed, transected, and intubated with a blunt 18-gage needle that delivered 0.8 ml ice-cold PBS. Infusion of the 0.5 ml volume was repeated twice, and the fluid was recovered. The recovered BALF was centrifuged at 3,000 × g for 3 min at 4°C and stored at −80°C until further use. ELISAs were performed on the bronchoalveolar lavage fluid (BALF) using TNFα, IL-6 ELISA kits (BD Biosciences, Palo Alto, CA, United States), IL-1β, CXCL1, and CXCL2 Duoset ELISA kits (R&D systems, Minneapolis, MN, United States).
Cell Counting
BALF was obtained via cannulation of the trachea and lavaging the airway lumen with 0.8 ml ice-cold PBS for three times on day 2 post-infection. The recovered fluid was centrifuged, and the cell pellets were resuspended in PBS to count the total number of cells. Differential cell counts in the BALF were performed using the Diff-Quik staining reagent (Sysmex, Tokyo, Japan), according to the manufacturer’s instructions. The numbers of macrophages, neutrophils, and T cells were calculated by multiplying the percentages obtained by the total yield. Slides were imaged using the QWin program (Leica, Leider Lane Buffalo Grove, IL, United States).
Histopathological Scoring
Lung tissue samples were fixed in 4% paraformaldehyde neutral buffer solution for 24 h, dehydrated in a graded ethanol series, embedded in paraffin, sliced at 5 μm, and stained with hematoxylin and eosin. Lung histopathological score was assessed using the following parameters: a scale of 0–3 (0 = absent and appeared normal, 1 = light, 2 = moderate, and 3 = severe) according to the histologic features: (1) edema, hyperemia, and congestion; (2) neutrophil margination and tissue infiltration; (3) intra-alveolar hemorrhage and debris. Each parameter was scored from 0 to 3 based on severity. The total score was used to assess lung injury, which was calculated as the sum of all scores for each parameter (0–1, normal to minimal injury; 2–3, mild injury; 4–6, moderate injury; and 7–9, severe injury). The maximum score per animal was 12. Lung section scoring was performed at a low power (×40).
Quantification and Statistical Analyses
GraphPad Prism v.8 (GraphPad Software, La Jolla, CA, United States) was used for the statistical analysis. Results are presented as the mean ± SEM for the experiments, unless otherwise indicated. Unpaired two-tailed Student’s t-tests were used to compare the two groups of independent samples. Sample size (n) is indicated in the figure legends. Statistical significance was set at P-values of less than 0.05.
Results
DEX/Lipo Is Specifically Delivered Into the Macrophages in the Lungs
As monocytes and macrophages seem to be implicated in cytokine storm (Giavridis et al., 2018; Norelli et al., 2018), we encapsulated dexamethasone into liposomes with a particle size of 1,000 nm (DEX/lipo) (Figure 1A) based on many other studies showing that liposomes with relatively larger diameters were more effectively distributed into phagocytes (Chono et al., 2006, 2007; Kelly et al., 2011). After 24 h of intranasal injection in mice, DiI-labeled DEX/lipo was detected in the cytosol of F4/80+ macrophages in the lungs (Figure 1B). We further confirmed the distribution of injected liposomes as up to 70% of DiI+ cells were alveolar macrophages, interstitial macrophages and monocytes (Figure 1C). In contrast, less than 10% of non-leukocytes and lymphocytes can uptake DiI+ liposomes. In particular, up to 15.8% of monocytes, 72.5% of alveolar macrophages, and 42.2% of interstitial macrophages in the lungs were DiI+ (Figure 1D; gating schemes are shown in Supplementary Figure 1). Collectively, these results confirm that locally delivered DEX/lipo is mostly distributed into monocytes/macrophages in the lungs.
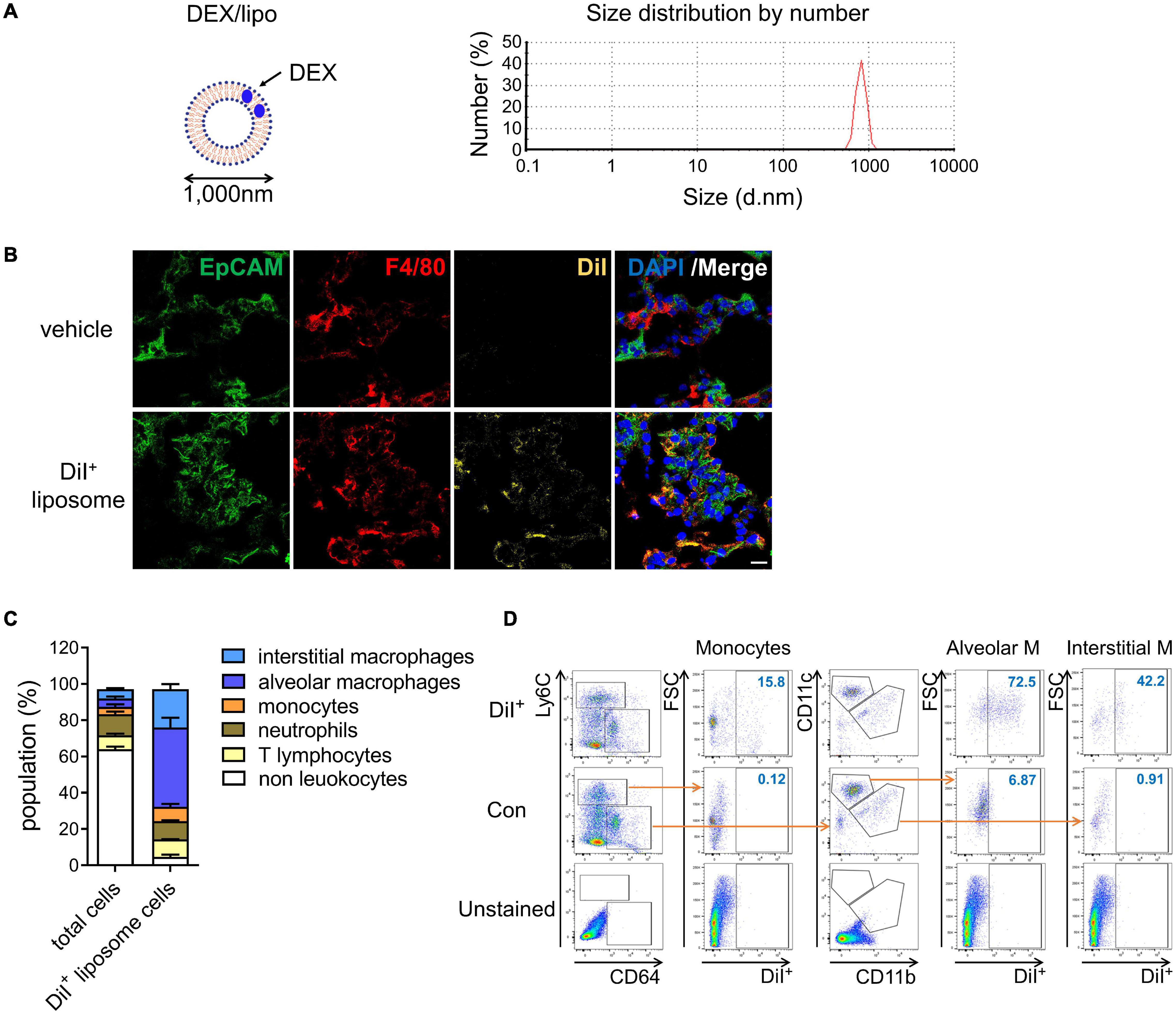
Figure 1. DEX/lipo is specifically delivered into the macrophages in lungs. (A) Schematic of DEX/lipo, left, and size determination of DEX/lipo by dynamic light scattering (DLS), right. The mean diameter is close to 1,000 nm. (B) Co-immunofluorescence (Co-IF) staining was performed to confirm the co-localization of the epithelial cell adhesion molecule (EpCAM) (green) or F4/80 (red) with DiI (yellow). Mice were injected intranally with 20 μl of DiI-labeled liposomes, and lung tissues were dissected at 24 h post-injection. Scale bar; 20 μm. (C) Population percentages in the lungs of mice are shown as total cells compared to DiI+ cells. Representatives of two independent experiments are shown. (D) Flow-cytometry analysis of DiI+ cells. CD45+Ly6C+CD64low monocytes population, CD45+Ly6C–CD64+CD11c+ alveolar macrophages population, and CD45+Ly6C–CD64+CD11b+ interstitial macrophages population are shown in the DiI/forward scatter (FSC) dot plot with gating for the DiI+ population.
DEX/Lipo Effectively Reduces Inflammation in Mice Infected With the Lethal Influenza A Virus
We determined that DEX/lipo has a therapeutic effect on a mouse influenza model infected with influenza A/Wisconsin/WSLH34939/09. Mice under isoflurane anesthesia were infected intranally with 105 50% tissue culture infectious dose (TCID50) of influenza virus. One hour after infection, mice were anesthetized by isoflurane inhalation for intranasal delivery of PBS/lipo, dexamethasone (DEX), or DEX/lipo for three days. On day 3 post-infection, mice were treated with 100 μl of PBS/lipo, DEX, or DEX/lipo intravenously. Mice were monitored for survival for 10 days. While 20% of PBS/lipo-treated mice survived on day 5 post-infection, mice treated with DEX/lipo showed the highest survival rate (40%). However, all mice treated with DEX died on day 5 (Figure 2A). Also, all mice treated with DEX rapidly lost body weight and died by day 5 post-infection. Control group (H1N1 + PBS/lipo) showed significant illness as determined by loss in body weight up to 27–30% whereas the DEX/lipo treated mice showed body weight loss of 15% on day 6 post-infection. From day 7 post-infection, both control group and H1N1 + DEX/lipo group lost body weight, but H1N1 + DEX/lipo group significantly higher than control (Figure 2B). To verify the anti-inflammatory activity of DEX/lipo on the infiltration of inflammatory cells, mice were euthanized at day 10 post-infection to obtain lung tissues for histopathological examination. In mice treated with PBS/lipo, there was a severe inflammatory response characterized by alveolar congestion, thickening of the alveolar wall, and infiltration and aggregation of immune cells in airspaces or vessel walls. However, fewer inflammatory infiltrates were observed in the lungs treated with DEX/lipo than in the lungs of mice treated with PBS/lipo or DEX (Figure 2C). Consistent with these findings, the total histopathological scores also decreased significantly in the H1N1 + DEX/lipo group but not in H1N1 + DEX group (Figure 2D). We also measured the virus titer to determine whether this histopathological score improvement in the H1N1 + DEX/lipo group was due to the difference in viral titers between groups. The viruses isolated from lungs plaqued on Madin-Darby canine kidney (MDCK) cells using TCID50. However, virus isolated from infected mouse lungs did not decrease in the H1N1 + DEX/lipo group (Figure 2E). Collectively, these results support our hypothesis that targeting macrophages using DEX/lipo has a therapeutic effect in reducing inflammation.
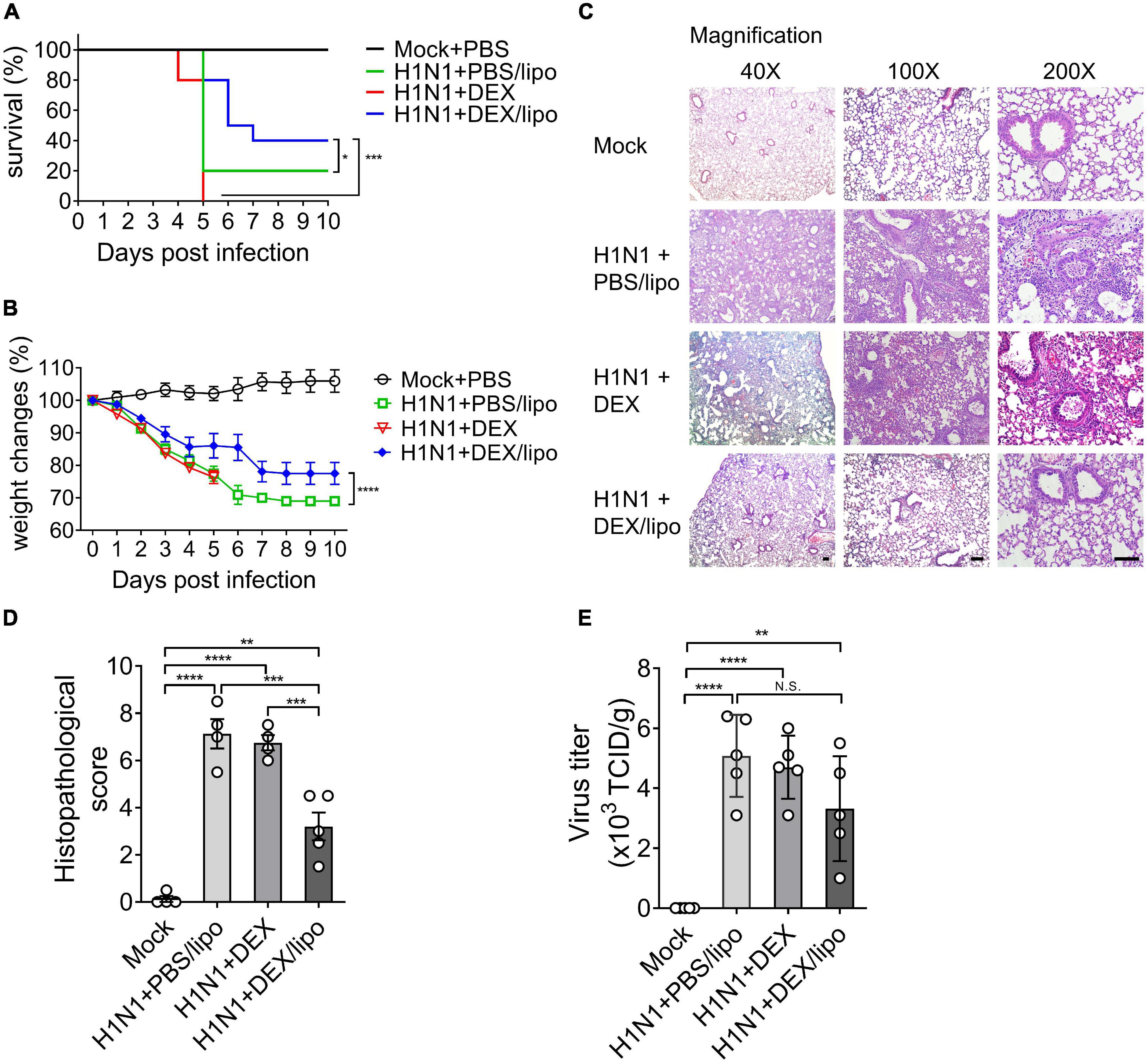
Figure 2. DEX/lipo effectively reduces inflammation in mice infected with the lethal influenza A virus. Mice were infected intranasally with 105 TCID50 of influenza A virus and treated with PBS/lipo, DEX, or DEX/lipo. Mice were monitored daily to record survival rates and body weight changes. (A) Survival rate of infected mice (n = 10 per group) after PBS/lipo, DEX, or DEX/lipo treatment. *P < 0.05, ***P < 0.001 by the log-rank test. (B) Mouse body weight change of infected mice (n = 10 per group) after PBS/lipo, DEX, or DEX/lipo treatment. ***P < 0.001 by the two-way ANOVA test. (C) Hematoxylin and eosin staining of the lungs on day 10 post-infection (n = 5 per group). Scale bar; 100 μm. (D) Comparison of the total histopathological scores of lung injury (n = 4–5 per group). Data are presented as the mean ± standard error of the mean (SEM). **P < 0.005, ***P < 0.001, ****P < 0.0001 by the one-way ANOVA test. (E) Lung viral titers from mice on day 10 post-infection using TCID50 (n = 4–5 per group). Data are presented as the mean ± SEM. **P < 0.01, ****P < 0.0001 the one-way ANOVA test.
DEX/Lipo Decreases Infiltrated Cells in the Bronchoalveolar Lavage Fluid of Mice With Lethal Influenza Virus Infection
We analyzed the infiltrated cells in bronchoalveolar lavage fluid (BALF) collected from the lungs of mice infected with influenza A virus. Many inflammatory cells, such as monocytes, neutrophils, and lymphocytes are recruited in the lungs of mice infected with influenza A virus. The cell infiltrates in the BALF of mice treated with PBS/lipo, DEX, or DEX/lipo were statistically analyzed for cell number and type (Figure 3). DEX/lipo treatment greatly reduced the infiltration of total cells (Figures 3A,B). Among the infiltrating cells, not only macrophages, which are known to cause acute lung injury in influenza (Dukhinova et al., 2020), but also neutrophils and T cells were decreased in the H1N1 + DEX/lipo group. Rather, DEX treatment increased the total number of infiltrated cells, especially macrophages and T cells, as compared to PBS/lipo treatment (Figures 3A,C–E).
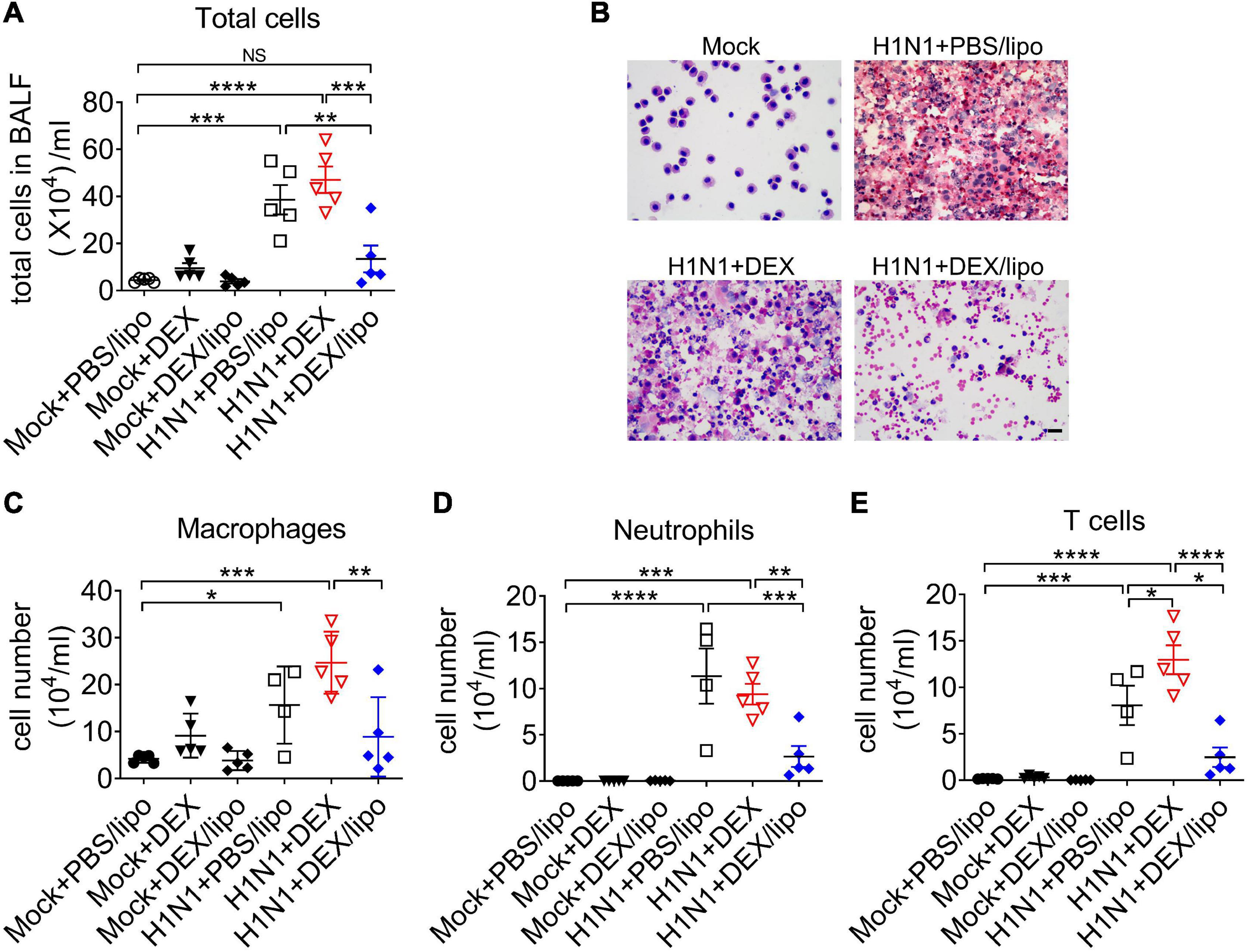
Figure 3. DEX/lipo decreases the total number of cells in the bronchoalveolar lavage fluid of mice with lethal influenza virus infection. (A) Analysis of the total number of infiltrated cells in the BALF collected from the mock or influenza A-virus infected mice treated with PBS/lipo, DEX, or DEX/lipo (n = 4–5 per group) on day 2 post-infection (B). Differential cell counts of BALF cells. Representative pictures of Diff-quick staining of cytospin preparation. Scale bar; 20 μm. Changes in the number of macrophages (C), neutrophils (D), T cells (E). BALF cells counted using the QWin program (Leica Microsystems). Data are presented as the mean ± SEM and are representative of two independent experiments. *P < 0.05, **P < 0.01, ***P < 0.001, ****P < 0.0001 by the one-way ANOVA test.
DEX/Lipo Significantly Reduces the Pro-inflammatory Cytokines and Chemokines in Mice With Lethal Influenza Virus Infection
Given that inflammatory cytokines and chemokines are linked to lung damage in severe influenza pneumonia, we determined the role of DEX/lipo in mice with influenza virus infection. The protein levels of pro-inflammatory cytokines and chemokines, TNFα, IL-1β, IL-6, CXCL1, and CXCL2 increased significantly in the H1N1 + PBS/lipo and H1N1 + DEX group (Figures 4A–E). However, cytokines and chemokines were decreased significantly in the H1N1 + DEX/lipo group, except for CXCL1 (Figures 4A–E). In particular, TNFα and IL-1β was significantly increased in H1N1 + DEX compared to that in the H1N1 + PBS/lipo group. These results correlated with the infiltrated total cell number.
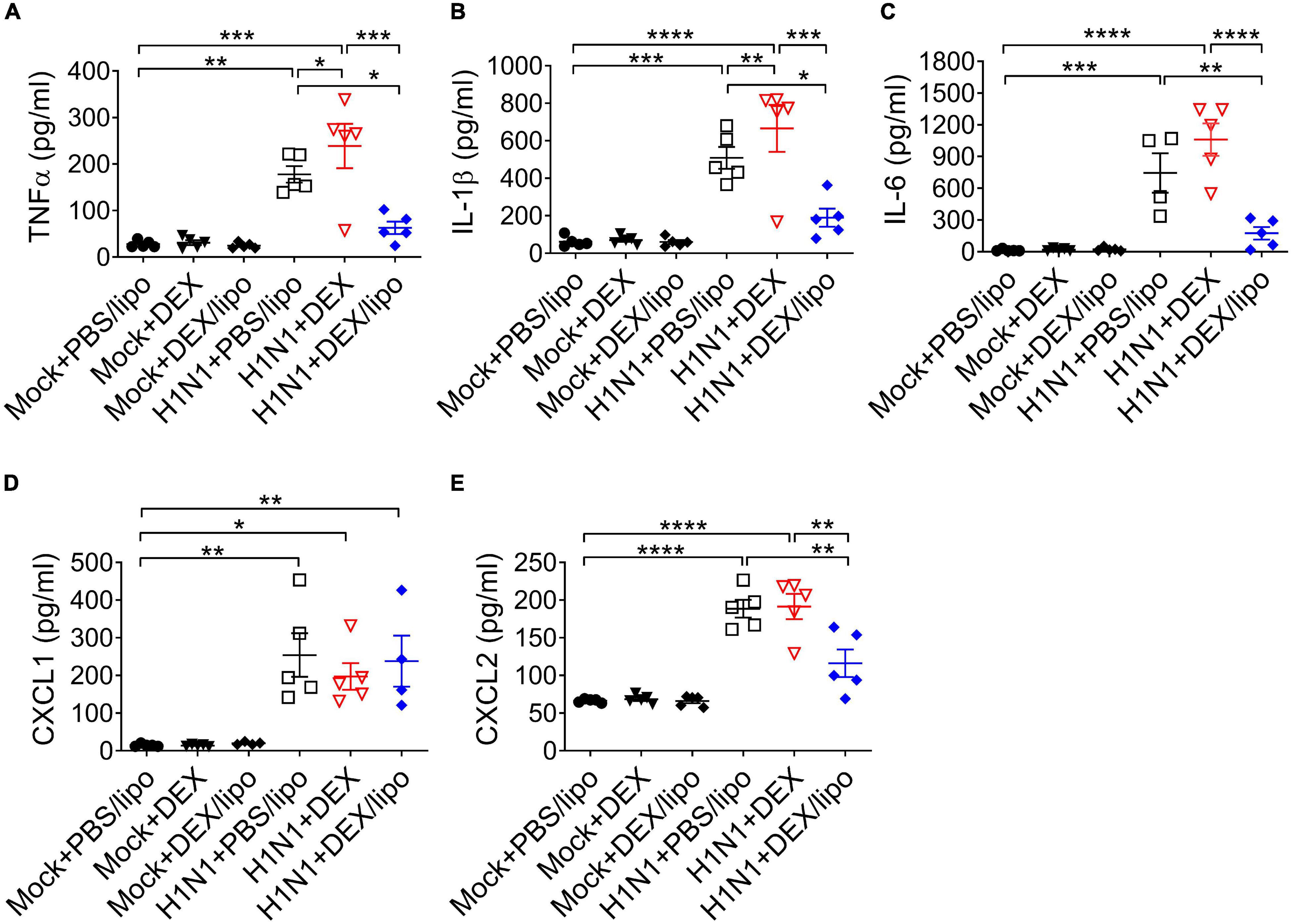
Figure 4. DEX/lipo significantly reduces the pro-inflammatory cytokines and chemokines in mice with lethal influenza virus infection (A–E). Analysis of the inflammatory cytokines and chemokines collected from BALF of mock or influenza A virus infected mice treated with PBS/lipo, DEX, or DEX/lipo (n = 4–5 per group) on day 2 post-infection. TNFα (A), IL-1β (B), IL-6 (C), CXCL-1 (D), and CXCL2 (E) were measured by the enzyme-linked immunosorbent assay (ELISA). Data are presented as the means ± SEM and are representative of two independent experiments. *P < 0.05, **P < 0.01, ***P < 0.001, ****P < 0.0001 by the one-way ANOVA test.
Discussion
Many patients with severe influenza virus die from overwhelming viral pneumonia and serious complications caused by cytokine storms. In this study, we revealed that DEX/lipo effectively reduces pro-inflammatory responses in hosts with pathogenic A/H1N1 influenza virus infection, demonstrating the possible availability of DEX/lipo in controlling disease symptoms of cytokine storm in respiratory infections.
Pathogenesis in human influenza virus infection is accompanied by diffuse epithelial sloughing in tracheal, bronchial, and bronchiole biopsy, predominant presence of mononuclear cells, and bloody exudate in the airway lumen with interstitial swelling (Walsh et al., 1961; Taubenberger and Morens, 2008). In line with this, a mouse model of human pathogenic pandemic A/Wisconsin/WSLH34939/09 influenza virus infection previously showed 80% of death within 12 days post-infection with marked tissue injury, including hemorrhage, pulmonary edema, and mononuclear cell accumulation (Walsh et al., 2011). The mouse adapted influenza virus infection model established in this study showed a faster pathogenesis than the previous study, showing 80% death on day 5 post-infection, but the characteristic histopathological findings, including alveolar congestion and infiltration of macrophages in airway spaces, showed comparable results (Figures 2, 3). Of note, cytokines, such as TNFα and IL-6, all of which are known to be directly correlated with host morbidity and pulmonary injury (Kozak et al., 1997; Hayden et al., 1998; Kaiser et al., 2001), were significantly elevated in bronchoalveolar lavage fluids on day 2 post-infection (Figures 4A,C), implying the importance of immune responses in the disease pathogenesis of our influenza virus infection model.
The most notable results in this study were the differences in the therapeutic responses of DEX/lipo compared to the free dexamethasone. Intranasal delivery of free dexamethasone did not alleviate tissue injury in the infected mice (Figure 2C). DEX/lipo could be delivered specifically to monocyte/macrophages, whereas DEX could be delivered to other cells, such as lung epithelial cells. Based on the previous study that corticosteroid induce apoptosis of airway epithelial cells (Dorscheid et al., 2001), it could be speculated that DEX treatment induced robust damages such as epithelial cells death resulted more severe inflammatory responses in lungs. In our study, we treated DEX immediately within 1 h after influenza virus infection. If DEX treatment is delayed by 24–48 h after influenza virus infection, it is getting difficult to suppress the initial excessive inflammatory responses even most DEX treatments would be delivered after symptom onset. This was further supported by the results shown in Figures 3, 4, because free dexamethasone did not block the accumulation of macrophages and T cells or decrease of TNFα, IL-1β, IL-6, and CXCL2 in bronchoalveolar fluids. In fact, free dexamethasone accelerated the fatality rate of infected mice from 80 to 100% (Figure 2B), possibly due to increased T cells, TNFα, and IL-1β in airway spaces. In contrast, DEX/lipo treatment rescued infected mice from death compared to free dexamethasone, with a significant decrease in tissue damage, infiltration of macrophages and T cells, and accumulation of TNFα, IL-1β, IL-6, and CXCL2 in the lungs. These results are consistent with concerns from previous observations of dexamethasone toxicities in the clinic (Belgaumi et al., 2003; Palladini et al., 2004; Palladini et al., 2005), and further demonstrate the usability of liposomes as delivery cargo in improving the safety and effectiveness of dexamethasone in controlling influenza virus infection.
There have been many cases in which DEX/lipo, mainly targeting macrophages, was delivered to reduce inflammation in various diseases. Polyethylene glycol (PEG)-free formulation of macrophage-targeting DEX/lipo reduces the dose and/or frequency required to treat adjuvant arthritis, with the potential to enhance or prolong therapeutic efficacy and limit side effects in the treatment of rheumatoid arthritis (Anderson et al., 2010). Additionally, administration of tumor-associated macrophage (TAM)-targeting DEX/lipo resulted in a significant inhibition of tumor growth and metastasis in a model of prostate cancer bone metastases (Kroon et al., 2015). In particular, macrophages are a major producer of inflammatory cytokines in influenza virus infection, and we showed that DEX/lipo were effectively distributed into monocytes/macrophages (Figure 2C). The fact that DEX/lipo did not reduce CXCL1, which is mostly produced by epithelial cells, and not by macrophages (Figure 4D), further supports our hypothesis that liposomal encapsulation specifically delivered dexamethasone into macrophages, but not epithelial cells.
Antiviral therapy should be accompanied by an immunomodulatory agent because antiviral drugs do not directly inhibit inflammation in pulmonary injury. In our study, even with the single treatment of DEX/lipo decreased inflammatory cytokines and increased the survival rate.
In conclusion, our data demonstrate that the macrophage-targeting DEX/lipo plays crucial roles in the prevention of pneumonia induced by influenza virus as well as the reduction of pro-inflammatory cytokines/chemokines and infiltration of inflammatory cells (Figure 5). Therefore, targeting macrophages using DEX/lipo may be used as a promising therapeutic approach for the treatment of cytokine storm-induced influenza virus infection with antiviral drugs.
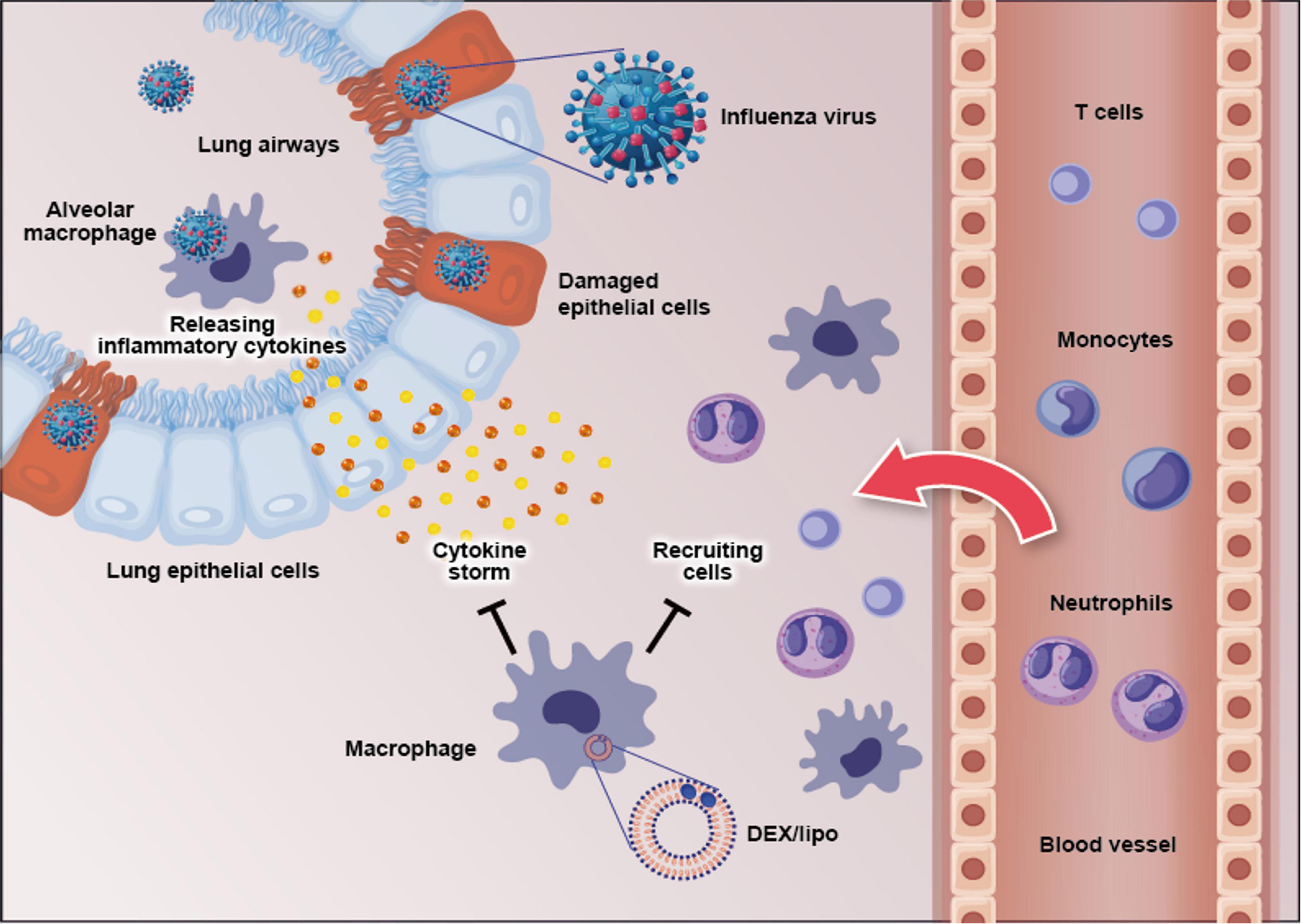
Figure 5. Schematic figure of the effects of DEX/lipo on the pathogenesis of the influenza virus infection. Influenza virus infects the lung epithelial cells and subsequently alveolar macrophages. This results in pro-inflammatory cytokine, and chemokine secretion by mainly macrophages. Specific pro-inflammatory cytokines lead to the recruitment of neutrophils, monocytes, macrophages, and T cells into the site of infection. DEX/lipo delivery targeting macrophages reduces cytokines/chemokines secretion and recruitment of leukocytes to the lung of mice with lethal influenza virus infection.
Data Availability Statement
The original contributions presented in the study are included in the article/Supplementary Material, further inquiries can be directed to the corresponding author/s.
Ethics Statement
The studies involving human participants were reviewed and approved by Seoul National University Hospital. The patients/participants provided their written informed consent to participate in this study.
Author Contributions
JK, YN, and SS conceived, designed, and supervised the experiments. JK and YN performed most experiments, analyzed the data, and wrote the original draft. HQ analyzed the data. JS, HC, DJ, and JH conducted some experiments. All authors reviewed and approved the manuscript.
Funding
This study was supported by Basic Science Research Program through the National Research Foundation of Korea (NRF) funded by the Ministry of Science, ICT and Future Planning (2020R1A2C2010202 and 2020R1A4A2002903), the Korea Research Institute of Bioscience and Biotechnology Research Initiative Program (KGM4572121), the NRF (NRF-2021R1A2C2010219), and SUNH Research Fund (0320210190).
Conflict of Interest
The authors declare that the research was conducted in the absence of any commercial or financial relationships that could be construed as a potential conflict of interest.
Publisher’s Note
All claims expressed in this article are solely those of the authors and do not necessarily represent those of their affiliated organizations, or those of the publisher, the editors and the reviewers. Any product that may be evaluated in this article, or claim that may be made by its manufacturer, is not guaranteed or endorsed by the publisher.
Supplementary Material
The Supplementary Material for this article can be found online at: https://www.frontiersin.org/articles/10.3389/fmicb.2022.845795/full#supplementary-material
Supplementary Figure 1 | Gating strategies to determine the cellular compositions of the lungs of mice (Related to Figure 1). Single cells were isolated from the lungs of DiI+ injected mice and analyzed by flow cytometry. Sequentially gated cells were (A) CD-45– non-leukocytes, (B) CD45+ CD11b–CD3+ T cells, (C) CD45+ CD11b+Ly6G+ neutrophils, (D) CD45+ Ly6G–CD64low Ly6C+ monocytes, (E) CD45+ Ly6G–CD64+ CD11c+ alveolar macrophages, (F) CD45+ Ly6G–CD64+ CD11b+ interstitial macrophages. Representative dot plots are shown.
References
Anderson, R., Franch, A., Castell, M., Perez-Cano, F. J., Brauer, R., Pohlers, D., et al. (2010). Liposomal encapsulation enhances and prolongs the anti-inflammatory effects of water-soluble dexamethasone phosphate in experimental adjuvant arthritis. Arthr. Res. Ther. 12:R147. doi: 10.1186/ar3089
Belgaumi, A. F., Al-Bakrah, M., Al-Mahr, M., Al-Jefri, A., Al-Musa, A., Saleh, M., et al. (2003). Dexamethasone-associated toxicity during induction chemotherapy for childhood acute lymphoblastic leukemia is augmented by concurrent use of daunomycin. Cancer 97, 2898–2903. doi: 10.1002/cncr.11390
Bender, B. S., and Small, P. A. Jr. (1992). Influenza: pathogenesis and host defense. Semin. Respir. Infect. 7, 38–45.
Brun-Buisson, C., Richard, J. C., Mercat, A., Thiebaut, A. C., and Brochard, L., and REVA-SRLF A/H1N1v 2009 Registry Group (2011). Early corticosteroids in severe influenza A/H1N1 pneumonia and acute respiratory distress syndrome. Am. J. Respir. Crit. Care Med. 183, 1200–1206. doi: 10.1164/rccm.201101-0135OC
Chono, S., Tanino, T., Seki, T., and Morimoto, K. (2006). Influence of particle size on drug delivery to rat alveolar macrophages following pulmonary administration of ciprofloxacin incorporated into liposomes. J. Drug Target. 14, 557–566. doi: 10.1080/10611860600834375
Chono, S., Tanino, T., Seki, T., and Morimoto, K. (2007). Uptake characteristics of liposomes by rat alveolar macrophages: influence of particle size and surface mannose modification. J. Pharm. Pharmacol. 59, 75–80. doi: 10.1211/jpp.59.1.0010
Darwish, I., Mubareka, S., and Liles, W. C. (2011). Immunomodulatory therapy for severe influenza. Expert Rev. Anti Infect. Ther. 9, 807–822.
Dorscheid, D. R., Wojcik, K. R., Sun, S., Marroquin, B., and White, S. R. (2001). Apoptosis of airway epithelial cells induced by corticosteroids. Am. J. Respir. Crit. Care Med. 164, 1939–1947. doi: 10.1164/ajrccm.164.10.2103013
Dukhinova, M., Kokinos, E., Kuchur, P., Komissarov, A., and Shtro, A. (2020). Macrophage-derived cytokines in pneumonia: linking cellular immunology and genetics. Cytokine Growth Factor Rev. 59, 46–61. doi: 10.1016/j.cytogfr.2020.11.003
Giannella, M., Alonso, M., Garcia De Viedma, D., Lopez Roa, P., Catalan, P., Padilla, B., et al. (2011). Prolonged viral shedding in pandemic influenza A(H1N1): clinical significance and viral load analysis in hospitalized patients. Clin. Microbiol. Infect. 17, 1160–1165. doi: 10.1111/j.1469-0691.2010.03399.x
Giavridis, T., Van Der Stegen, S. J. C., Eyquem, J., Hamieh, M., Piersigilli, A., and Sadelain, M. (2018). CAR T cell-induced cytokine release syndrome is mediated by macrophages and abated by IL-1 blockade. Nat. Med. 24, 731–738. doi: 10.1038/s41591-018-0041-7
Hayden, F. G., Fritz, R., Lobo, M. C., Alvord, W., Strober, W., and Straus, S. E. (1998). Local and systemic cytokine responses during experimental human influenza A virus infection. Relation to symptom formation and host defense. J. Clin. Invest. 101, 643–649. doi: 10.1172/JCI1355
Itoh, Y., Shinya, K., Kiso, M., Watanabe, T., Sakoda, Y., Hatta, M., et al. (2009). In vitro and in vivo characterization of new swine-origin H1N1 influenza viruses. Nature 460, 1021–1025. doi: 10.1038/nature08260
Julkunen, I., Melen, K., Nyqvist, M., Pirhonen, J., Sareneva, T., and Matikainen, S. (2000). Inflammatory responses in influenza A virus infection. Vaccine 19(Suppl. 1), S32–S37.
Julkunen, I., Sareneva, T., Pirhonen, J., Ronni, T., Melen, K., and Matikainen, S. (2001). Molecular pathogenesis of influenza A virus infection and virus-induced regulation of cytokine gene expression. Cytokine Growth Factor Rev. 12, 171–180. doi: 10.1016/s1359-6101(00)00026-5
Kaiser, L., Fritz, R. S., Straus, S. E., Gubareva, L., and Hayden, F. G. (2001). Symptom pathogenesis during acute influenza: interleukin-6 and other cytokine responses. J. Med. Virol. 64, 262–268. doi: 10.1002/jmv.1045
Kelly, C., Jefferies, C., and Cryan, S. A. (2011). Targeted liposomal drug delivery to monocytes and macrophages. J. Drug Deliv. 2011, 727241. doi: 10.1155/2011/727241
Kozak, W., Poli, V., Soszynski, D., Conn, C. A., Leon, L. R., and Kluger, M. J. (1997). Sickness behavior in mice deficient in interleukin-6 during turpentine abscess and influenza pneumonitis. Am. J. Physiol. 272, R621–R630. doi: 10.1152/ajpregu.1997.272.2.R621
Kroon, J., Buijs, J. T., Van Der Horst, G., Cheung, H., Van Der Mark, M., Van Bloois, L., et al. (2015). Liposomal delivery of dexamethasone attenuates prostate cancer bone metastatic tumor growth in vivo. Prostate 75, 815–824. doi: 10.1002/pros.22963
La Gruta, N. L., Kedzierska, K., Stambas, J., and Doherty, P. C. (2007). A question of self-preservation: immunopathology in influenza virus infection. Immunol. Cell Biol. 85, 85–92. doi: 10.1038/sj.icb.7100026
Lammers, T., Sofias, A. M., Van Der Meel, R., Schiffelers, R., Storm, G., Tacke, F., et al. (2020). Dexamethasone nanomedicines for COVID-19. Nat. Nanotechnol. 15, 622–624. doi: 10.1038/s41565-020-0752-z
Li, K. S., Guan, Y., Wang, J., Smith, G. J., Xu, K. M., Duan, L., et al. (2004). Genesis of a highly pathogenic and potentially pandemic H5N1 influenza virus in eastern Asia. Nature 430, 209–213. doi: 10.1038/nature02746
Liu, Q., Zhou, Y. H., and Yang, Z. Q. (2016). The cytokine storm of severe influenza and development of immunomodulatory therapy. Cell. Mol. Immunol. 13, 3–10. doi: 10.1038/cmi.2015.74
Merad, M., and Martin, J. C. (2020). Pathological inflammation in patients with COVID-19: a key role for monocytes and macrophages. Nat. Rev. Immunol. 20, 355–362.
Norelli, M., Camisa, B., Barbiera, G., Falcone, L., Purevdorj, A., Genua, M., et al. (2018). Monocyte-derived IL-1 and IL-6 are differentially required for cytokine-release syndrome and neurotoxicity due to CAR T cells. Nat. Med. 24, 739–748. doi: 10.1038/s41591-018-0036-4
Oslund, K. L., and Baumgarth, N. (2011). Influenza-induced innate immunity: regulators of viral replication, respiratory tract pathology & adaptive immunity. Future Virol. 6, 951–962. doi: 10.2217/fvl.11.63
Palladini, G., Perfetti, V., Obici, L., Caccialanza, R., Semino, A., Adami, F., et al. (2004). Association of melphalan and high-dose dexamethasone is effective and well tolerated in patients with AL (primary) amyloidosis who are ineligible for stem cell transplantation. Blood 103, 2936–2938. doi: 10.1182/blood-2003-08-2788
Palladini, G., Perfetti, V., Perlini, S., Obici, L., Lavatelli, F., Caccialanza, R., et al. (2005). The combination of thalidomide and intermediate-dose dexamethasone is an effective but toxic treatment for patients with primary amyloidosis (AL). Blood 105, 2949–2951. doi: 10.1182/blood-2004-08-3231
Peiris, J. S., Hui, K. P., and Yen, H. L. (2010). Host response to influenza virus: protection versus immunopathology. Curr. Opin. Immunol. 22, 475–481. doi: 10.1016/j.coi.2010.06.003
Rocksen, D., Lilliehook, B., Larsson, R., Johansson, T., and Bucht, A. (2000). Differential anti-inflammatory and anti-oxidative effects of dexamethasone and N-acetylcysteine in endotoxin-induced lung inflammation. Clin. Exp. Immunol. 122, 249–256. doi: 10.1046/j.1365-2249.2000.01373.x
Russell, C. J., and Webster, R. G. (2005). The genesis of a pandemic influenza virus. Cell 123, 368–371. doi: 10.1016/j.cell.2005.10.019
Shieh, W. J., Blau, D. M., Denison, A. M., Deleon-Carnes, M., Adem, P., Bhatnagar, J., et al. (2010). 2009 pandemic influenza A (H1N1): pathology and pathogenesis of 100 fatal cases in the United States. Am. J. Pathol. 177, 166–175. doi: 10.2353/ajpath.2010.100115
Shinya, K., Gao, Y., Cilloniz, C., Suzuki, Y., Fujie, M., Deng, G., et al. (2012). Integrated clinical, pathologic, virologic, and transcriptomic analysis of H5N1 influenza virus-induced viral pneumonia in the rhesus macaque. J. Virol. 86, 6055–6066. doi: 10.1128/JVI.00365-12
Taubenberger, J. K., and Morens, D. M. (2008). The pathology of influenza virus infections. Annu. Rev. Pathol. 3, 499–522.
Tay, M. Z., Poh, C. M., Renia, L., Macary, P. A., and Ng, L. F. P. (2020). The trinity of COVID-19: immunity, inflammation and intervention. Nat. Rev. Immunol. 20, 363–374. doi: 10.1038/s41577-020-0311-8
To, K. K., Hung, I. F., Li, I. W., Lee, K. L., Koo, C. K., Yan, W. W., et al. (2010). Delayed clearance of viral load and marked cytokine activation in severe cases of pandemic H1N1 2009 influenza virus infection. Clin. Infect. Dis. 50, 850–859. doi: 10.1086/650581
Torchilin, V. P. (2005). Recent advances with liposomes as pharmaceutical carriers. Nat. Rev. Drug Discov. 4, 145–160. doi: 10.1038/nrd1632
Walsh, J. J., Dietlein, L. F., Low, F. N., Burch, G. E., and Mogabgab, W. J. (1961). Bronchotracheal response in human influenza. Type A, Asian strain, as studied by light and electron microscopic examination of bronchoscopic biopsies. Arch. Intern. Med. 108, 376–388. doi: 10.1001/archinte.1961.03620090048006
Walsh, K. B., Teijaro, J. R., Wilker, P. R., Jatzek, A., Fremgen, D. M., Das, S. C., et al. (2011). Suppression of cytokine storm with a sphingosine analog provides protection against pathogenic influenza virus. Proc. Natl. Acad. Sci. U.S.A. 108, 12018–12023. doi: 10.1073/pnas.1107024108
Keywords: H1N1 influenza, dexamethasone, liposome, macrophage, cytokine
Citation: Kwon JW, Quan H, Song J, Chung H, Jung D, Hong JJ, Na YR and Seok SH (2022) Liposomal Dexamethasone Reduces A/H1N1 Influenza-Associated Morbidity in Mice. Front. Microbiol. 13:845795. doi: 10.3389/fmicb.2022.845795
Received: 30 December 2021; Accepted: 23 February 2022;
Published: 12 April 2022.
Edited by:
Marcelo J. Kuroda, University of California, Davis, United StatesReviewed by:
Timothy Carroll, University of California, Davis, United StatesOliver Planz, University of Tübingen, Germany
Copyright © 2022 Kwon, Quan, Song, Chung, Jung, Hong, Na and Seok. This is an open-access article distributed under the terms of the Creative Commons Attribution License (CC BY). The use, distribution or reproduction in other forums is permitted, provided the original author(s) and the copyright owner(s) are credited and that the original publication in this journal is cited, in accordance with accepted academic practice. No use, distribution or reproduction is permitted which does not comply with these terms.
*Correspondence: Yi Rang Na, eWlyYW5nbmFAc251LmFjLmty; Seung Hyeok Seok, bGFtc2Vva0BzbnUuYWMua3I=