- 1Department of Biology, Universidad Autónoma de Madrid, Madrid, Spain
- 2Department of Ecology, Universidad Autónoma de Madrid, Madrid, Spain
- 3Centro de Investigación en Biodiversidad y Cambio Global (CIBC-UAM), Universidad Autónoma de Madrid, Madrid, Spain
- 4UC3M-Santander Big Data Institute (IBiDat), Universidad Carlos III de Madrid, Madrid, Spain
- 5Department of Mathematics, Universidad Autónoma de Madrid, Madrid, Spain
The biological activity of marine vertebrates represents an input of nutrients for Antarctic terrestrial biota, with relevant consequences for the entire ecosystem. Even though microbial mats assemble most of the biological diversity of the non-marine Antarctica, the effects of the local macrofauna on these microecosystems remain understudied. Using 16S rRNA gene sequencing, 13C and 15N stable isotopes, and by characterizing the P and N-derived nutrient levels, we evaluated the effects of penguins and other marine vertebrates on four microbial mats located along the Antarctic Peninsula. Our results show that P concentrations, C/N and N/P ratios, and δ15N values of “penguin-impacted” microbial mats were significantly higher than values obtained for “macrofauna-free” sample. Nutrients derived from penguin colonies and other marine vertebrates altered the trophic interactions of communities within microbial mats, as well as the relative abundance and trophic position of meiofaunal groups. Twenty-nine bacterial families from eight different phyla significantly changed with the presence of penguins, with inorganic nitrogen (NH4+ and NO3–) and δ15N appearing as key factors in driving bacterial community composition. An apparent change in richness, diversity, and dominance of prokaryotes was also related to penguin-derived nutrients, affecting N utilization strategies of microbial mats and relating oligotrophic systems to communities with a higher metabolic versatility. The interdisciplinary approach of this study makes these results advance our understanding of interactions and composition of communities inhabiting microbial mats from Antarctica, revealing how they are deeply associated with marine animals.
Introduction
The Antarctic climatic conditions impose severe restrictions on living organisms. Low temperatures, low availability of liquid water, long periods with continuous irradiance, and months of nearly complete darkness during winter (Thomas et al., 2008) result in an ecosystem dominated by microorganisms. The harsh environmental conditions, together with the prevalence of rocky sites with low nutrient levels and continental geographic isolation, make microscopic organisms the most diverse and abundant components of terrestrial Antarctic communities (Hughes et al., 2015). This provides a unique setup to validate ecological hypotheses on microorganism-based food webs in a more “controlled” scenario (Hansson and Tranvik, 2003). Cyanobacteria become key players at these high-latitude ecosystems (Quesada and Vincent, 2012), because of their extreme resilience resisting desiccation, freeze–thaw cycles, and high levels of UV radiation, which contribute to their dominance in polar aquatic ecosystems (Bonilla et al., 2005). Cyanobacterial microbial mats accommodate high biodiversity and represent one of the highest concentrations of non-marine biomass with a ubiquitous distribution throughout Antarctica (Quesada and Vincent, 2012) and therefore constitute an important carbon (C) reservoir.
Recently, Velázquez et al. (2017) and Almela et al. (2019) described in detail the trophic relationships among the different elements of the community within Antarctic microbial mats. The authors described at least four trophic levels in the microbial mats and a community adapted to a short growing season by virtue of a fine temporal coupling, where fungal and bacterial activities represent the main connectors between consumers and producers. These studies were conducted from communities growing in oligotrophic environments. However, microbial mats are also found in the vicinity of areas with presence of marine vertebrates. The influence of local macrofauna on polar ecosystems is well known (Jakubas et al., 2008; Zawierucha et al., 2016, 2019; Bokhorst et al., 2019; Wang et al., 2020), but the influence of their biological activity on the ecology of microbial mats (diversity and trophic interactions) is understudied. Seal haul-outs and seabird colonies on Antarctic coastal ecosystems are not considered part of the terrestrial biota (Laybourn-Parry, 2009), but they influence the non-marine ecosystem where they remain a considerable portion of their time. The overall seabird population in Antarctica and its sub-Antarctic islands represents 26% of the global population. However, the nesting populations in this regions account for 80% of the total N and P excreted worldwide (Otero et al., 2018). Therefore, marine vertebrates, mainly seabirds, and especially penguins, provide a relevant input of nutrients through their droppings into this ecosystem by transferring nitrogen and phosphorus from sea to land (Bokhorst et al., 2019; Wang et al., 2020), which could deeply modify the ecosystem where they reside (De La Peña-Lastra, 2021).
The Antarctic Peninsula region has shown rapid warming, similar in magnitude to some regions in the Arctic and substantially faster than the global mean (Robinson et al., 2020). This region is considered as one of the most sensitive on Earth to climate warming as average temperatures are close to the freezing point (Rochera et al., 2013), and small temperature shifts can trigger pronounced effects. Climate change in the region is already causing an alteration in marine vertebrates’ communities with shifts in species and abundance in their populations (Lynch et al., 2012; Clucas et al., 2014; Dunn et al., 2016; Morley et al., 2019; Ropert-Coudert et al., 2019), suggesting major implications for local terrestrial biodiversity patterns in Antarctica (Bokhorst et al., 2019).
Here we determined δ15N, phosphorus (P), total nitrogen (TN), C/N, N/P, and inorganic nitrogen (N-NH4 + and NO3) in microbial mats adjacent and isolated from penguin colonies along the Antarctic Peninsula. The aim of this study was to identify the impact of N and P derived from macrofaunal activity on benthic microbial mats and hence its effects on bacterial, fungal, photosynthetic, and meiofaunal communities and the trophic relationships that occur within them. To date, it is unknown how the activity of marine vertebrates affects these polar microecosystems. The results presented here will shed new insights on microbial community function to understand and assess the ecological risks over freshwater microbial communities derived from changes on populations of seabirds and marine mammals.
Materials and Methods
Study Site and Sampling
Samplings were conducted during two different Antarctic campaigns in different ice-free areas of the Antarctic Peninsula (Figure 1A): Byers Peninsula plateau (BP), Cierva Point (CP), and Avian Island (AI) microbial mats were sampled in February 2016 and Lagotellerie Island (LI) microbial mat in February 2019.
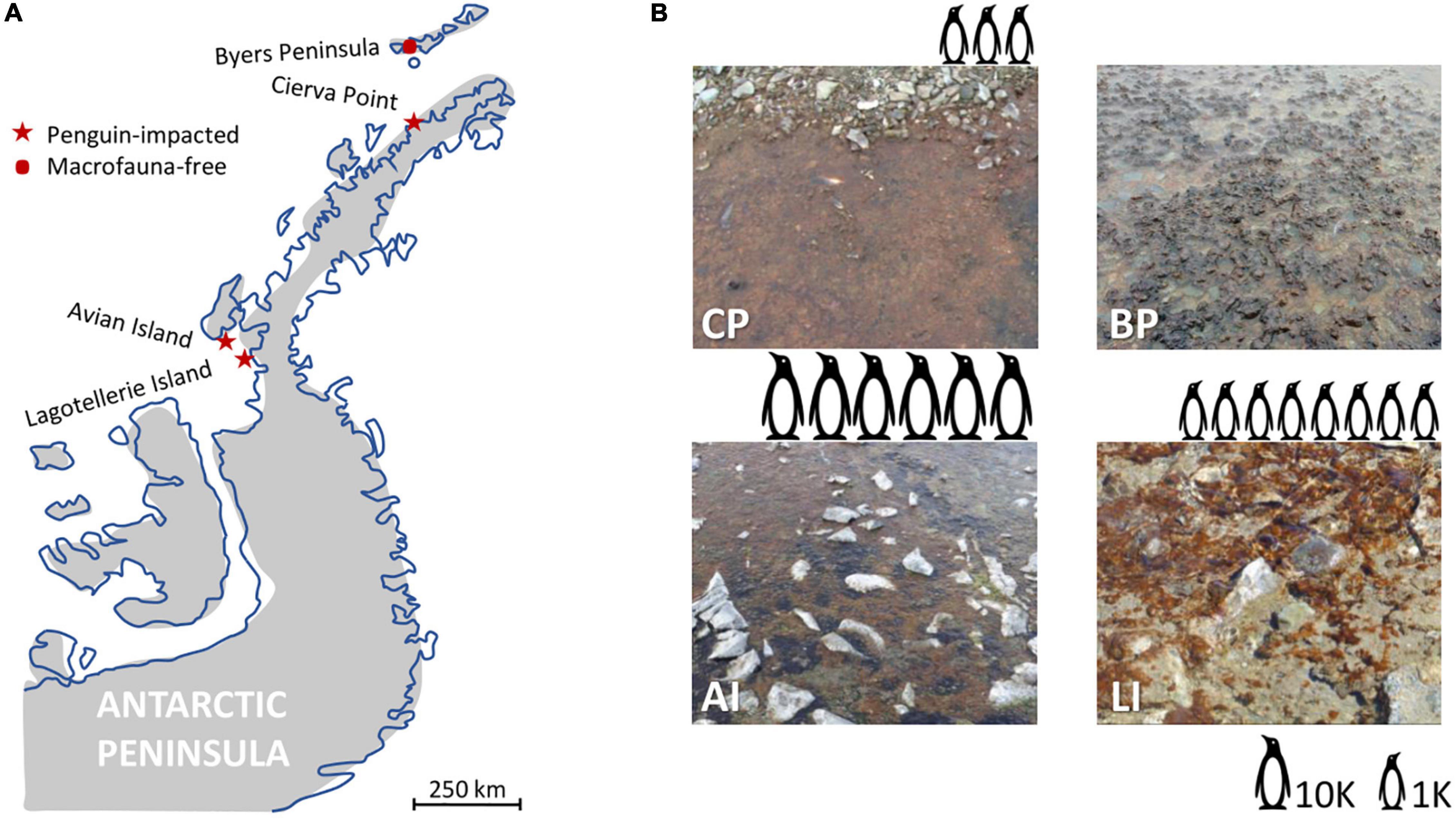
Figure 1. Map indicating the location of the sampling areas along the Antarctic Peninsula. (A) Distribution of the sampled microbial mats along the Antarctic Peninsula. Red stars indicate the sampling areas with penguin colonies in their surroundings, whereas the red dot indicates the sampling area isolated from marine vertebrates. (B) Detailed picture of microbial mats from Byers Peninsula Plateau (BP), Caleta Primavera (CP), Avian Island (AI), and Lagotellerie Island (LI). Images of penguins represent the population (number of nests) of penguin colonies from CP, AI, and LI sampling areas (data from MAPPPD; Humphries et al., 2017).
BP is one of the largest ice-free areas in the Antarctic Peninsula. It is located at the western end of Livingston Island (South Shetland archipelago). It is designated as the Antarctic Specially Protected Area (ASPA) no. 126 to protect its terrestrial and lacustrine habitats, and it is considered one of the main Antarctic hotspots of biodiversity (ATCM, 2011). Here, the sampled microbial mat (BP) was located over the plateau, 1.5 km away from the coast and 60 masl. The microbial mat was multilayered and orange pigmented, with an average thickness of 13 ± 2 mm. Mosses were observed at the edge of the waterbody where the microbial mat was collected, but sporadically, some stems can be observed within the mat structure.
CP, which is also designated as ASPA (no. 134), is located in the northwestern portion of the Antarctic Peninsula. The site comprises the ice-free area between the southwest coast of Cierva Cove and the northeast coast of Santucci Cove. It has great scientific value because of its unusual biodiversity, which includes numerous species of birds, flora, and invertebrates (ATCM, 2013). The sampled microbial mat (CP), orange–green in color and 4 ± 1 mm in thickness, was located within the ASPA, 200 m away from the coast and 34 masl.
AI (ASPA no. 117) is situated 400 m south of Adelaide Island at Marguerite Bay on the western side of the central Antarctic Peninsula. The island comprises 0.49 km2, which usually remains ice-free during the summer seasons. The values described under its ASPA designation aim to protect the abundance and diversity of breeding seabirds on the island (ATCM, 2002). The sampled microbial mat (AI), purple–green in color and 5 ± 2 mm in thickness, was collected in a pond at less than 30 m from the coast and 10 masl.
Finally, we also collected samples from LI, located at Marguerite Bay as well. It has been designated as ASPA no. 115 because of its relatively diverse flora and fauna, which is characteristic of the southern Antarctic Peninsula (ATCM, 2017). The studied microbial mat from this location, orange–brown in color and 5 ± 2 mm in thickness, was collected in an outlet stream to the coast at 2 masl.
The presence of penguins in the surroundings of the studied microbial mats was different among the sampling areas. On the one hand, CP mat was adjacent to an active Gentoo penguin (Pygoscelis papua) colony (ATCM, 2013), and AI and LI mats were adjacent to an Adelie penguin (Pygoscelis adeliae) colony (ATCM, 2002, 2017). Penguin population data were obtained from the online database Mapping Application for Penguin Populations and Projected Dynamics (MAPPPD; Humphries et al., 2017), estimating colonies of 3304 (CP), 59,895 (AI), and 8195 (LI) nests (Figure 1B). These locations have a remarkable influence of different seabirds, such as skuas (Catharacta maccormicki, Catharacta loennbergi), gulls (Larus dominicanus), and imperial shags (Phalacrocorax atriceps). Also, southern elephant seals (Mirounga leonina) and seals (Arctocephalus gazella, Leptonychotes weddellii) are frequently observed in the surroundings (ATCM, 2002, 2013, 2017). On the other hand, the BP sample was collected from an area with no influence of marine vertebrates, far and geographically separate from the coastline, and therefore without nutrient inputs derived from macrofaunal activity. The four sampling areas are not subjected to almost any direct human influence, and visitors can only access the sites for scientific purposes and with permits. Both access and sampling were specifically permitted by Spanish Polar Committee.
All samples were collected as follows: three replicates of approximately 6 cm2 were haphazardly collected using sterilized metal spatulas from each sampling spot, covering a sampling area of approximately 2 m2 of the wetland. These samples were stored in sterile Whirl-Pak bags (Nasco Ltd.) at −20°C until further analysis at the laboratory.
Environmental Data Collection and Chemical and Stable Isotope Analyses
The averages of 21 years of number of days per year with temperatures above freezing point (TAF) were considered a good proxy to summarize the climatic characteristics of the sampling sites. This parameter was estimated with the hourly data obtained from the Modern-Era Retrospective Analysis for Research and Applications (Rienecker et al., 2011).
P is an important element of penguin feces, which together with N, and its high δ15N signature, are usually much higher in areas influenced by penguins than the values registered in non-affected sites (Liu et al., 2013; Otero et al., 2018; Bokhorst et al., 2019; Wang et al., 2020). Analyses of P, TN, and δ15N values and C/N and N/P ratios were carried out to assess the microbial mat nutrient levels and whether they are significantly affected by the biological activity of penguins and other marine vertebrates. Briefly, fresh material from each microbial mat was dried out for 48 h at 65°C and homogenized after sieving through a 0.5-mm strainer. Therefore, the nutrient content was jointly determined for the biomass and the contents in the interstitial water present in the samples and expressed as mg ⋅ g–1. Triplicates of 0.3 g of each dried sample were digested with HNO3 and HCl (10 mL) at 105°C for 2 h. After filtration through a 0.45-μm nylon filter membranes, the P concentration was determined by inductively coupled plasma emission spectroscopy (iCAP 6500 DUO; Thermo Scientific, Waltham, MA, United States). The bulk C and N concentrations of samples were measured in triplicate by a LECO CHNS-932 Analyzer (model no. 601-800-500). Briefly, approximately 10 mg of each dried sample was placed in a tin cup, wrapped to compact it, and then analyzed. C/N and N/P ratios were calculated from the C, N, and P concentrations.
The δ15N signature of each microbial mat was quantified by triplicate using an isotope ratio mass spectrometer (MEIR; 20-20 PDZ Europa mass spectrometer, Sandbach, United Kingdom), from fresh samples placed in 175-μL zinc cases. Isotopic values were expressed as δ15N = [(R sample/R standard) − 1] × 1000 (‰), where R represents the 15N/14N ratio, and the R standard value was based on atmospheric N (N2-atm).
NO3– and N-NH4 + concentrations were analyzed to further study the effect of penguin-derived N on microbial mats. NO3– was determined by liquid phase chromatography. Briefly, 2 g of dried and sieved material from each sample was extracted by an aqueous solution (50 mL final volume) while stirring for 30 min. Extracts were filtered through 0.45-μm nylon membranes and then retained in the cartridges (Metrosep A Supp 5-250; Metrohm, Herisau, Switzerland), eluted, and analyzed by ion chromatography (883 Basic IC; Metrohm, United States). The nitrogen content in ammonium (N-NH4+) was determined by ion chromatography following the same previously described protocol (in this case, boiling while stirring for the extraction), but the extracts were retained in cartridges Metrosep C6 250/4.0 (Metrohm, Herisau, Switzerland). Estimations of total biomass were calculated from the ash free dry weight (AFDW) gravimetric approach. Briefly, triplicates of 8-mm-diameter cores per sample of fresh material were dried, weighed, ashed in a muffle furnace at high temperature (450°C for 4 h), and reweighed. The loss is referred to as AFDW and reflects the C content. The biomass values were expressed as total organic C (TOC) per surface (mg ⋅ cm–2).
Phototrophic and Fungal Community Estimations
Phototrophic community abundance (Cyanobacteria, Chlorophyta, Bacillariophyta, and other algae) was estimated by chlorophyll-a (chla) concentration. Chla was extracted in triplicates from 8 mm in diameter core samples, using 90% (vol/vol) acetone (Ritchie, 2008). Extracts were measured spectrophotometrically (Hitachi U-2000 Spectrophotometer; Hitachi Ltd., Tokyo, Japan) at 665 nm.
Ergosterol, a biochemical marker of fungal active biomass, was quantified using high-performance liquid chromatography equipped with a UV detector (282 nm) as described by Gessner (2020). Extraction was performed by triplicate according to Romaní et al. (2009) using samples from the 8-mm-diameter core. The concentration of ergosterol in the eluted product was then transformed to fungal biomass, assuming that 5.5 mg of ergosterol was found in 1 g of fungal biomass (Gessner and Chauvet, 1993).
Microscopic Identification of the Community Composition and 13C and 15N Natural Abundance Analysis
The composition of the trophic community was studied from 8-mm-diameter core samples of fresh material, which were manually disaggregated. Relative abundance of the meiofauna (rotifers, tardigrades, and nematodes) was analyzed by using a stereo-zoom microscope (Leica MZ75) and interpreted per surface units. Photosynthetic organisms were determined using epifluorescence microscopy equipped with an Olympus® blue filter set (EF 400–490 nm, DM 570, FB 590) and an Olympus® green filter set (EF 530–545 nm, DM 570, FB 590) to excite chla and cyanobacterial phycobilin, respectively.
Stable isotope 13C and 15N provide information on the original nutrient source, which makes it one of the most helpful tools for assessing food web functioning. For δ13C‰ and δ15N‰ natural abundance signal analysis, individuals of different trophic levels were manually separated under the microscope by microdissection and encapsulated in triplicates in 175-μL zinc cases and dried at 65°C for 48 h. A minimum of 0.02 mg of dry weight biomass was required (Shaw et al., 2018), which was achieved by collecting approximately 50–100 living individuals per zinc case. Particulate organic matter (POM) smaller than 30 μm was determined in triplicate and per sample, as follows: a piece of mat was pressed gently against a Nytal sieve of 30-μm-mesh-size diameter. From this filtrate, and after standing for 1 h, the POM fraction was obtained with the heavier material that precipitated in 50-mL Corning tubes. Therefore, we obtained the fraction of organic matter that mostly contains exopolysaccharides (EPSs) from cyanobacterial community, but also organisms smaller than 30 μm. From the supernatant, and after filtering through a 0.22-μm hydrophilic membrane and concentrating by evaporation at 40°C under vacuum, dissolved organic matter (DOM) fraction was obtained and encapsulated. The isotopic ratios from all samples were analyzed by mass spectrometry (MEIR; 20-20 PDZ Europa mass spectrometer).
DNA Extraction, Sequencing, and Ribotype Diversity Analysis
Total genomic DNA was extracted in triplicate for the studied microbial mats. The DNAeasy Power Biofilm kit (Qiagen) was used for DNA extraction, using the protocol supplied with the kit. Bacterial 16S rRNA marker gene was amplified from the cellular fractions using the set of primers 8F15B (5′-AGAGTTTGATCCTGG-3′) and 515R14AM (5-TTACCGCGGCTGCT-3′) (Aguirre de Cárcer et al., 2011). The pool of samples with the prepared libraries was sequenced by Illumina MiSeq platform.
Bacterial 16S rRNA gene diversity was assessed with QIIME v2-2019.4 (Bolyen et al., 2019). Briefly, cleaned and trimmed paired reads were filtered and denoised using DADA2 plug-in (Callahan et al., 2016). For chimera identification, 300,000 training sequences were used. Identified amplicon sequence variants (ASVs) were aligned using MAFFT (Katoh et al., 2002) and further processed to construct a phylogeny with fasttree2 (Price et al., 2010). Taxonomy was assigned to ASVs using the q2-feature-classifier (Bokulich et al., 2018) and blasted against the SILVA v132 99% 16S sequence database (Quast et al., 2012). Sequences assigned to chloroplasts were removed from the dataset.
Sequences generated by this study were deposited to GenBank under the BioProject accession number PRJNA795814.
Data Analyses
Statistically significant differences between “penguin-impacted” and “macrofauna-free” microbial mats in terms of nutrient levels, diversity indices, and relative abundance of fungal biomass (ergosterol), primary producers (chla), and meiofaunal groups (tardigrades, nematodes, and rotifers) measurements were determined with a Welch t-test in R v4.1.1 (R Core Team, 2019). To further understand the relationships among environmental and chemical parameters with microbial mat communities, Pearson correlation coefficients among C, N, P, C/N, N/P, and δ15N content of the microbial mats, as well as latitude and the ‘number of days per year with temperatures above freezing’ (TAF) from LI, AI, CP, and BP sites, were calculated using PAST 4.03 software (Hammer et al., 2001). For inference, normal distributions were assumed, and two-tailed t-tests were calculated for the non-correlation null hypothesis, with 0.05 significance level.
To assess the trophic pathways within the microbial mat community, a Bayesian isotopic mixing model, available as an open-source R package, simmr (Parnell and Inger, 2016), was used. Mixing models use organism isotopes to estimate the proportional contribution of sources to the consumer diet composition (Parnell et al., 2010). The model was fitted via Markov Chain Monte Carlo method. After 200,000 iterations of the algorithm, we represented the marginal posterior dietary proportions with boxplots and considered the medians as single summary values. We assumed similar stoichiometry for C and N fractionation through the food web in the model. Trophic enrichment factors (TEFs) were based on mean trophic fractionations with standard deviations (TEF δ13C: −0.4 ± 1.3; TEF δ15N: −3.4 ± 1) (Post, 2002).
Alpha rarefaction analysis was performed using the “qiime diversity alpha-rarefaction” function, to determine if the samples were sequenced to a sufficient depth. Alpha diversity indices (richness and Shannon index) were estimated using the “qiime diversity core-metrics-phylogenetic” function. The lowest sample-specific sequencing depth (24,892) was used to compensate for the variation in read numbers. The Margalef diversity index (Margalef, 1958) was calculated by the formula: , where S is the number of ASV, and N is the number of feature counts in the sample. Shannon and Margalef indices were measured to compare the diversity of bacteria among samples. Beta diversity was assessed using Bray–Curtis dissimilarities among the community compositions of the sampling sites and visualized with non-metric multidimensional scaling (NMDS) using the phyloseq package (v1.14.0) (McMurdie and Holmes, 2013) in R. A UPGMA cluster dendrogram based on Bray–Curtis dissimilarity was used to visualize similarities among the bacterial communities from the studied microbial mats. Differences among bacterial communities were tested with permutational multivariate analysis of variance (PERMANOVA) test using the vegan package (v2.5-3) (Oksanen et al., 2013) in R.
To explore the relationships between microbial communities and environmental variables, a two-distance–based redundancy analysis (db-RDA) was performed (Legendre and Anderson, 1999). The collinear or non-significant predictors were eliminated by using the “ordistep” analysis in the vegan package (Oksanen et al., 2013) in R. All significance testing was assessed by Monte Carlo permutation of full models with 999 unrestricted permutations. To examine the difference in relative abundance of microorganisms (family level) between penguin impacted and non-impacted locations, we calculated log2-fold changes using the DESeq2 package (v.1.34.0) (Love et al., 2014) in R.
Results
Nutrient Levels and Its Origin Differ Between “Penguin-Impacted” and “Macrofauna-Free” Microbial Mats
The lowest concentrations of P, and the highest C/N and N/P ratios were found in BP, the microbial mat far away from any penguin colony and/or macrofaunal activity (Table 1). The highest values of P, N, and the lowest C/N and N/P ratios were present in those microbial mats adjacent to an active penguin colony and considered as “penguin-impacted” samples (LI, AI, and CP). We observed significantly higher values when comparing “penguin-impacted” microbial mats with the sample without macrofaunal influence for P (t = 4.81, p < 0.01), C/N (t = −13.34, p < 0.01), and N/P ratios (t = −8.34, p = 0.01). BP microbial mat also displayed the lowest concentration of N-NH4+ and NO3, compared with “penguin-impacted” microbial mats (Table 1). Despite the high variation shown for δ15N signatures among LI, AI, and CP samples, statistical analysis showed that δ15N values at “penguin-impacted” samples were significantly higher than those in the “macrofauna-free” microbial mat (t = 8.46, p < 0.01).
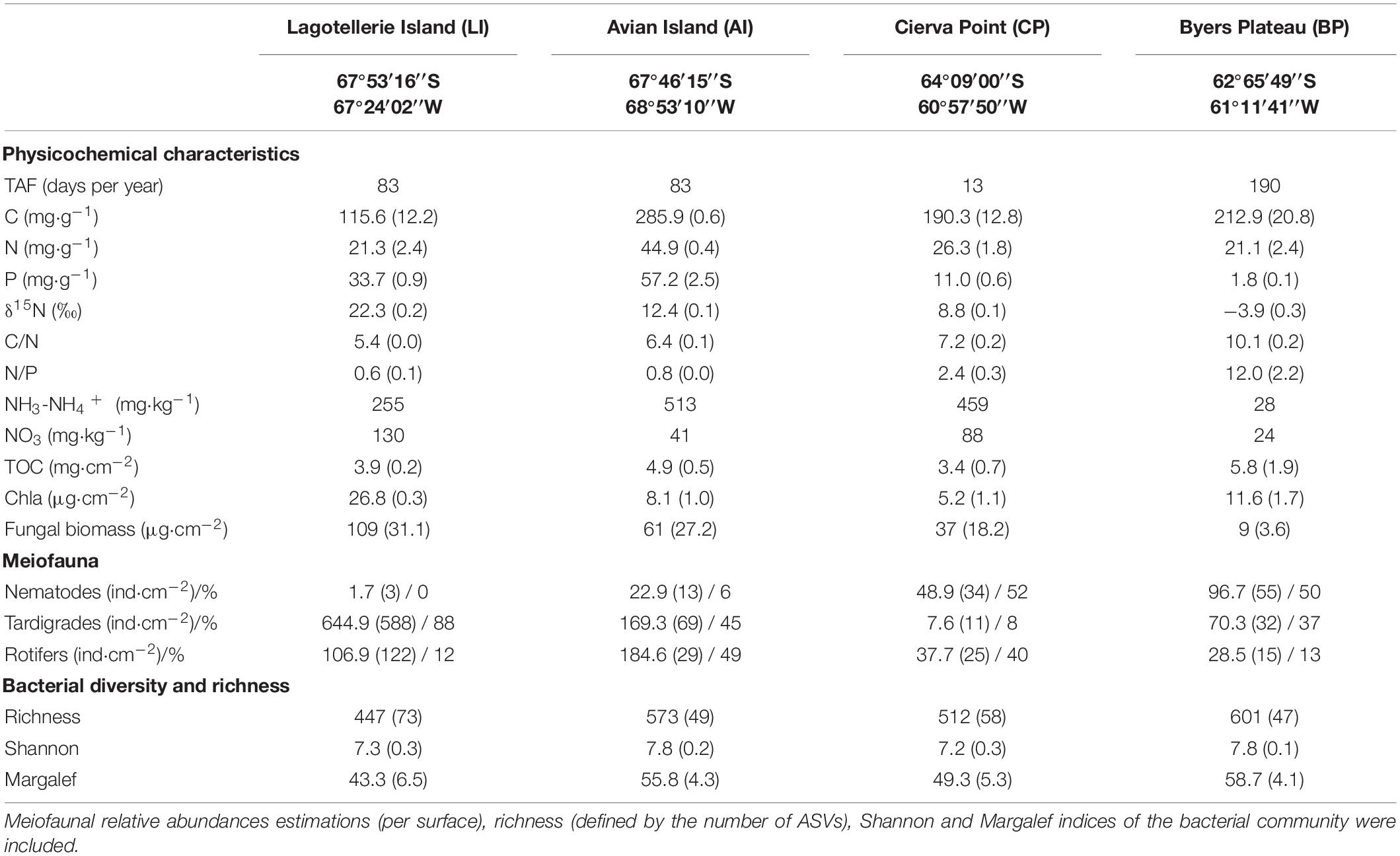
Table 1. Mean (standard deviation in parentheses) values of the physicochemical characteristics of the four microbial mats included in this study.
N and P concentrations in microbial mats were significantly positively correlated with penguin population in the surroundings (0.97 and 0.90, respectively) (Supplementary Figure 1). Therefore, local macrofauna, mainly represented by penguins, indirectly may contribute to a nutrient enrichment of the environment by providing nitrogen and phosphorus compounds to the studied microecosystems. C was also positively correlated with penguins (0.73), whereas TOC was correlated with TAF (0.68).
Fungal Community Shows High Correlation With Penguin-Derived N and P
Fungal biomass, represented by ergosterol concentrations, was significantly higher in “penguin-impacted” microbial mats (t = 5.47, p < 0.01), showing an average 10-fold higher than in BP (Table 1). Moreover, the relative abundance of the fungal community showed a negative correlation with C/N and N/P ratios (−0.86 and −0.78, respectively) and a positive correlation with P concentration, δ15N values, and latitude (0.79, 0.86, and 0.91, respectively) (Supplementary Figure 1). No significant differences were observed for the relative abundance of photosynthetic primary producers, represented by chla concentration, among the samples (data not shown). Although a positive correlation with δ15N (0.61) and a negative correlation with C (−0.75) appeared. TOC, related to total biomass of cyanobacterial microbial mats, showed positive correlation with the climatic parameter of TAF (0.68).
The microscopic analyses indicated that the microbial community matrix was dominated by different Oscillatoriales and Synechococcales (Cyanobacteria) morphotypes (sensu Hoffmann et al., 2005). Wilmottia sp. (5.5–7 μm in diameter), previously classified as Phormidium (Anagnostidis and Komárek, 1988), was common in all the studied microbial mats. Morphotype I sensu Broady and Kibblewhite (1991) (2 μm in diameter, Leptolyngbya cf. antarctica), dominated the cyanobacterial community of BP, but also occurred in all the mats (Supplementary Figure 2). Abundant microcolonies (20–100 μm in diameter) of Nostocalean morphotypes (3 μm in diameter cells) appeared mostly in BP mat, below photosynthetically active layers. Unicellular cyanobacteria (e.g. Synechococcus spp.; 1.5 μm in diameter) were relatively abundant and occurred intermixed within the EPS matrix. The photosynthetic community also included Chlorophyta, although with an apparent relative lower abundance than the cyanobacterial community. Prasiola sp. constituted a top layer in AI and CP microbial mats, where Chlamydomonadaceae were also found. Chlamydomonas sp. (16–20 μm in diameter) was very common within the matrix of LI. Bacillariophyta was not abundant but observed in AI and LI samples. In all microbial mats, Mougeotia sp. (22–27 μm in diameter) occurred.
The Meiofauna Groups Are Heterogeneously Distributed Among the Samples
The meiofauna found in the studied microbial mats consisted of tardigrades Hypsibiidae, bdelloid rotifers, and nematodes (Supplementary Figure 2). None of the groups in any of the samples was clearly associated with a specific layer or portion of the microbial mats. The relative abundance of tardigrades, rotifers, and nematodes (Table 1) was heterogeneous and not correlated with P- and N-related parameters, and no significant correlation was found among groups. Nonetheless, tardigrades were more abundant in LI and AI microbial mats (86 and 45% of total meiofaunal individuals captured per surface, respectively), counting dozens of individuals per analyzed sample (Table 1). Nematodes predominated in CP and BP microbial mats (52 and 50% of total meiofaunal individuals captured per surface, respectively). The relative abundance of bdelloid rotifers was 49 and 40% of total meiofaunal individuals captured per surface in AI and CP, remaining less than 15% in the other microbial mats.
Trophic Relationships Change Among the Studied Microbial Mats
For all microbial mats, Cyanobacteria were the most abundant primary producers, and consequently a potential C source for the trophic web. Also, Chlamydomonadaceae (Chlorophyta) for CP and LI mats, Prasiolaceae (Chlorophyta) for AI mat, and mosses (BP mat) were included in the food web study. In CP and BP, δ13C of Cyanobacteria, POM, and DOM (Supplementary Table 1) showed signals similar to each other, whereas in the other mats, the sources showed different values of both stable isotopes. δ13C and δ15N signals in the BP microbial mat were clearly different from the isotopic signals found in the other mats. While 15N/14N values of BP were negative in all separated groups (with the exception of nematodes where it was slightly positive), in all the other microbial mats, δ15N was always clearly positive averaging from 8.8 to 22.3‰. The same pattern was observed in BP for 13C/12C values, showing less negative values in all the trophic groups studied compared with the other mats.
Consumer’s category consisted for all samples of tardigrades Hypsibiidae, rotifers, and nematodes. Tardigrades in LI microbial mat were classified as top consumers from the marked differences in the 15N signatures, showing δ15N values that exceed those of nematodes by 2.8 (Figure 2A). Nevertheless, nematodes in AI, CP, and BP mats were situated at the top of the food web, as concluded from the observed differences of 4.3, 2.5, and 3.5 δ15N with tardigrades, and differences of 2.5, 2.2, and 2.3 δ15N with rotifers. Enrichments in δ15N found between nematodes and rotifers for LI (0.8) and between rotifers and tardigrades for AI, CP, and BP (1.9, 0.2, and 1.2, respectively) remained below typical 2–4‰ difference among trophic levels.
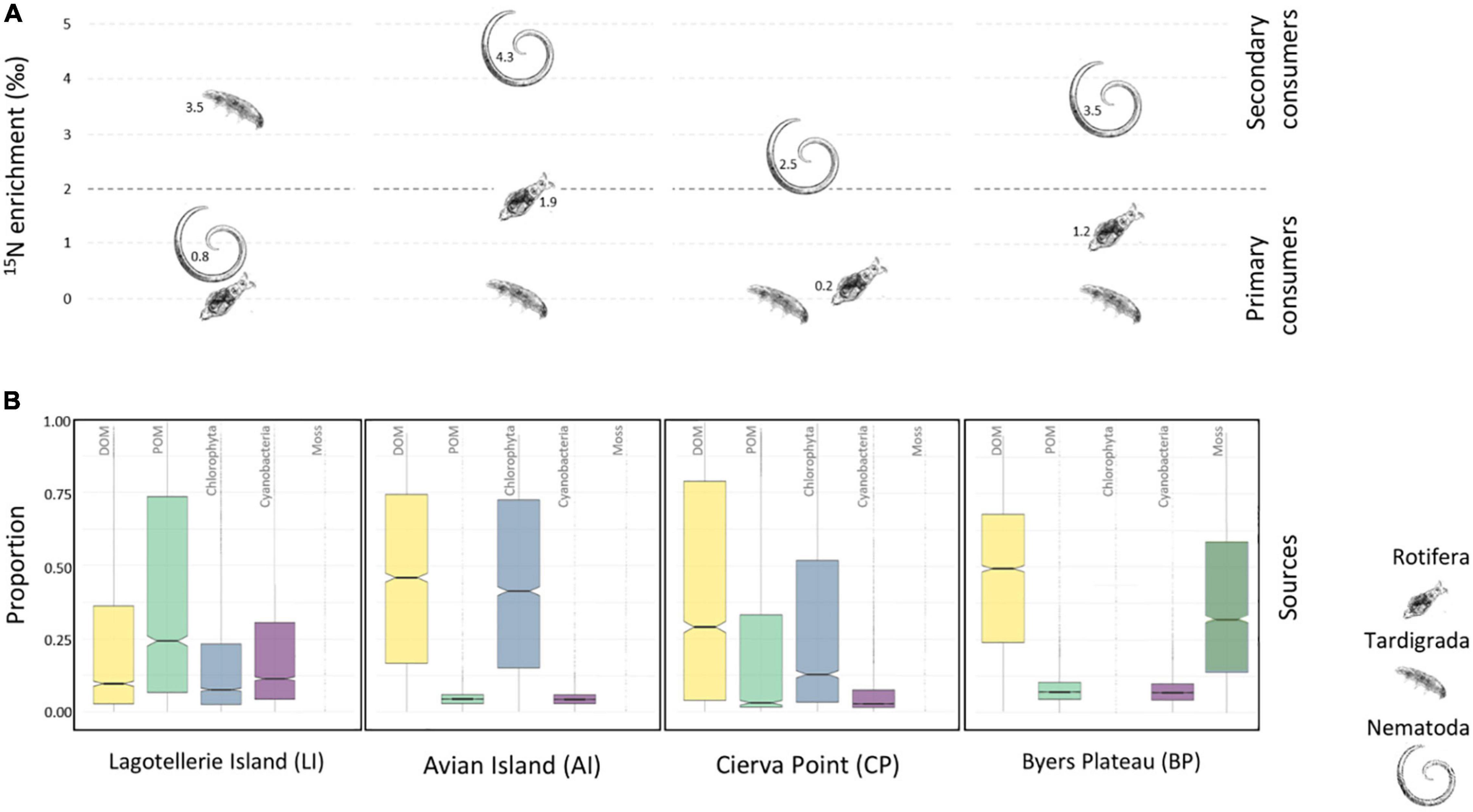
Figure 2. (A) Enrichment in δ15N of rotifers, nematodes, and Hypsibiidae tardigrade. For estimating the differences between “primary consumers” and “secondary consumers,” an enrichment of 2–4‰ was considered. (B) Simmr credibility interval plot of the contribution of potential food sources of the studied microbial mats (boxes enclose the 50% credibility interval; lines within boxes represent median values).
To determine C pathways, we plotted the credibility intervals for the proportions of each potential source to the consumer’s diet contribution in each mat (Figure 2B). The Bayesian isotopic mixing model estimated which were the preferred C sources by consumers, showing DOM as the main food source in most of the microbial mats. Thus, the estimated dietary proportions for DOM in AI, CP, and BP were 46, 29, and 49%, respectively. For LI mat, the POM fraction appeared with the highest dietary proportion (24%) of available sources. Cyanobacterial fractions showed low proportions as a potential source in all microbial mats. Chlorophyta proportions from AI and CP (41 and 12%, respectively) placed them as the second potential food source, as mosses did in BP (29%).
Bacterial Communities Differ Significantly in the Surroundings of Penguin’s Colonies
The total number of 16S rRNA gene sequences obtained was 1,393,079, which were grouped into 3,031 different ASVs. In order to analyze the similarities among bacterial communities inhabiting the microbial mats, the sequencing taxonomic abundance profiles (ASV level) were used to compute a Bray–Curtis dissimilarity matrix, summarized in two dimensions by using NMDS (Figure 3A). Samples were grouped according to location, showing greater dissimilarities among samples than among replicates within the same sample. Hierarchical clustering based on community structure similarity (Figure 3B) revealed four main clusters according to each sampling location. AI and CP mats clustered together, showing a percentage of similarity of 32%. BP showed the community the lowest percentage of similarity (∼7%) to the other microbial mats. A PERMANOVA test corroborated significant evidence of heterogeneity (F = 16.61, p < 0.01) for bacterial communities among microbial mats.
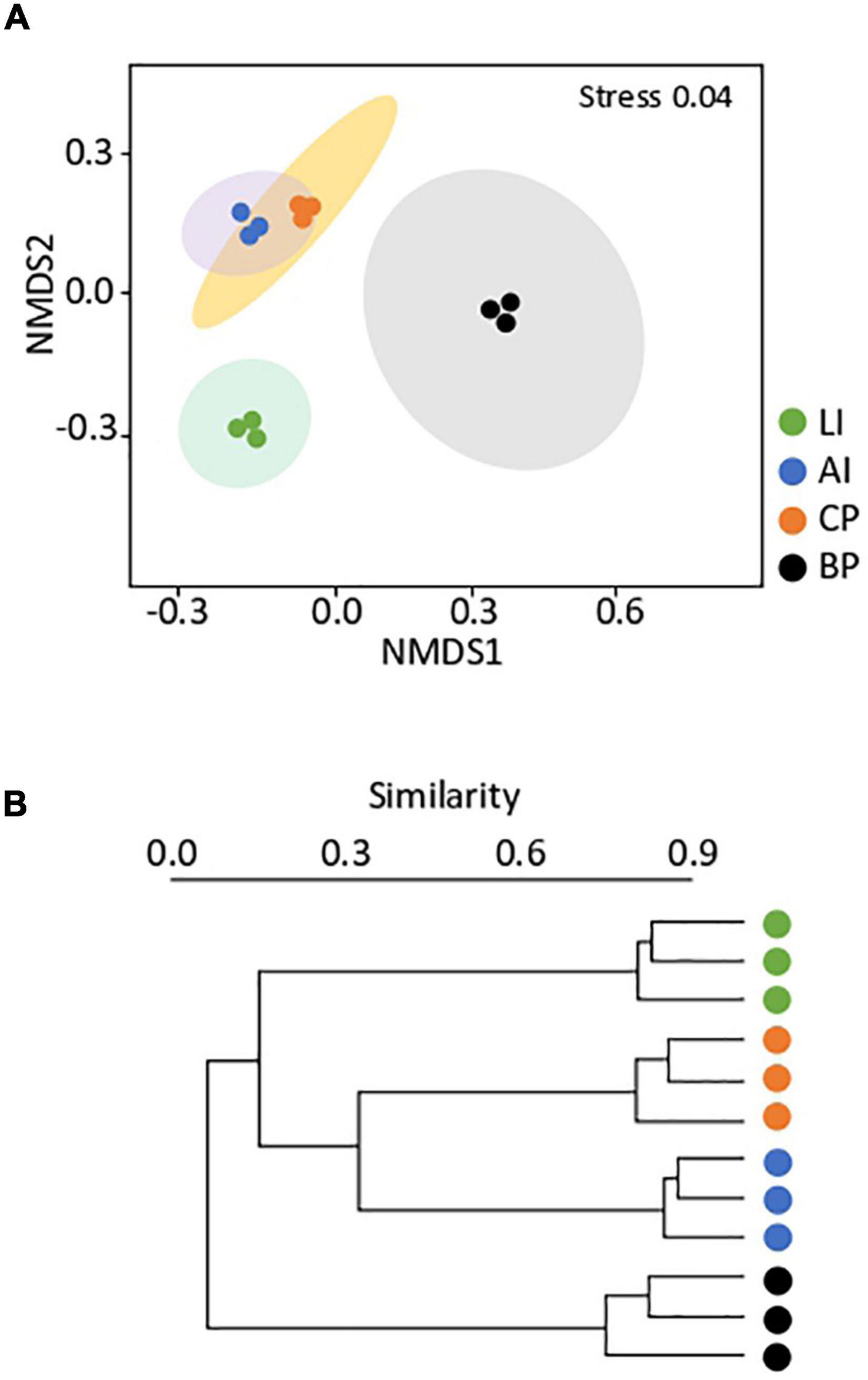
Figure 3. (A) Non-metric multidimensional scaling (NMDS) of the bacterial community composition of Lagotellerie Island (LI), Avian Island (AI), Caleta Primavera (CP), and Byers Peninsula Plateau (BP) microbial mats. The multivariate analysis is based on Bray–Curtis dissimilarity matrices between the microbiome profiles at an ASV level. Distances between symbols on the ordination plot reflect relative dissimilarities in community structures. Ellipses indicate 95% confidence intervals fitted into the spatial ordination. (B) UPGMA cluster dendrogram of the bacterial community within the microbial mats included in this study. The relative abundances were used to evaluate the relationships between bacterial communities, using weighted pair clustering based on Bray–Curtis measurements.
Bacterial communities (Figure 4) were dominated by sequences assigned to Proteobacteria (37–47%) and Bacteroidetes (19–43%). Cyanobacteria sequences ranked as the second phylum in BP with the highest number of assigned sequences (27.8%) and third in the remaining samples (4–21%). When analyzing the sequences at a higher taxonomic resolution, important differences in the composition of the bacterial community appeared. The most abundant orders were Burkholderiales, Leptolyngbyales, and Flavobacteriales, followed by Sphingobacteriales, Chitinophagales, and Sphingomonadales. However, whereas these 6 taxa reached relative abundances of between 68 and 78% in the ‘penguin- affected’ microbial mats, in BP they did not reach 50%. Moreover, those taxa (order level) with relative abundances lower than 1% reached 11% of the total community sequences in BP, whereas in the other microbial mats they represented between 5 and 8% of the total.
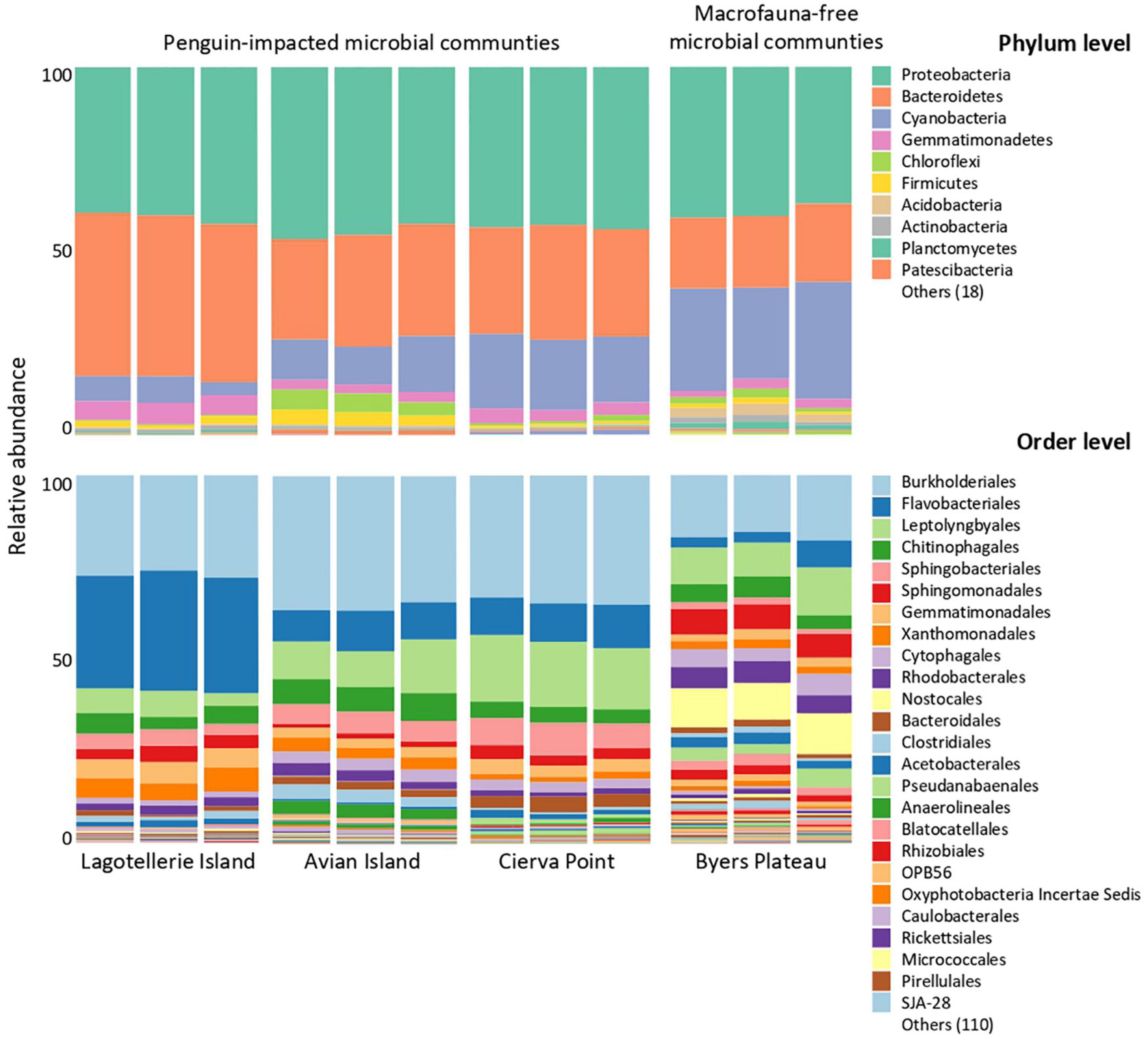
Figure 4. Relative abundance of 16S rRNA genes at different taxonomic resolution (phyla vs. order) from microbial mats included in this study.
The large variation (e.g. standard deviation) in values of the measured bacterial diversity indices among the samples indicated high spatial heterogeneity (Table 1). The lowest number of different ASVs was found in LI mat (367), as well as the lowest values for Shannon and Margalef indices (7.0 and 36.0, respectively). The highest number of different ASVs (655), as well as the highest values for Shannon and Margalef indices (7.9 and 63.4), was shown for BP microbial communities. We observed significantly higher values when comparing the microbial mat without animal influence with microbial mats associated with “penguin-impacted” environments for richness (t = −2.42, p = 0.05), Shannon (t = −2.61, p = 0.03), and Margalef indices (t = −2.67, p = 0.04). In addition, bacterial richness and Margalef index values were positively correlated with C (0.64 and 0.66, respectively) and C/N (0.60 and 0.62, respectively) and negatively correlated with δ15N (−0.67 and −0.69, respectively). Shannon index appeared positively correlated with TAF (0.63).
We identified 29 bacterial families that changed significantly with the presence of penguins using DeSeq2, with 25 that decline and 4 that increase (Supplementary Table 2). Five of the 25 taxa were related to cyanobacterial families (Cyanobacteriaceae, Pseudanabaenaceae, Coleofasciculaceae, Leptolyngbyaceae, and Nostocales incertae sedis), whereas Bacteroidetes and Proteobacteria were the predominant phyla, showing the largest number of families involved (7 and 6, respectively). Flavobacteriaceae, Crocinitomicaceae, and Rhodothermaceae were more abundant in “penguin-affected” microbial mats. The most important factors within the physicochemical environment that directly or indirectly affected the bacterial community composition of microbial mats, based on the distance-based redundancy analysis (db-RDA), were NO3– (F = 10.61, p = 0.01), N-NH4 + (F = 14.76, p = 0.01), and δ15N (F = 12.94, p = 0.00). These factors together contributed significantly to the variance (∼85%, p < 0.01).
Discussion
Effects of Penguin Droppings on Nutrient Levels of the Microbial Mats
In the absence of biological activity, the Antarctic terrestrial ecosystems exhibit an oligotrophic or ultraoligotrophic status (Tanabe et al., 2017), as the ability of non-biological processes to transport nutrients is very limited (Wang et al., 2020). Under these circumstances, any nutrient input into the system will be of significance for the community as most of the ecosystems are usually N (Davey, 1993a,b; Hawes et al., 1993) and P limited (Laybourn-Parry and Wadham, 2014). Seabirds are the main exchangers of nutrients between marine and terrestrial systems (De La Peña-Lastra, 2021), and their presence could deeply modify the ecosystems around their colonies (Otero et al., 2018). According to our results, microbial mats thriving in the vicinity of a penguin colony showed the highest concentrations of P and inorganic N (N-NH4+ and NO3–), which constitute the main nutrients in fresh seabird feces after the decomposition of uric acid (Schmidt et al., 2004). Moreover, a positive correlation was shown between the concentration of TN and P and the presence of penguins. These data suggest that penguins and other seabird droppings represent an input of nutrients for the studied microbial mats, as previously reported for different terrestrial polar ecosystems (Jakubas et al., 2008; Zmudczyñska et al., 2012; Ball et al., 2015; Zawierucha et al., 2016, 2019; Bokhorst et al., 2019; Wang et al., 2020; Ramírez-Fernández et al., 2021) and probably constitute the most important source of nutrients in these microecosystems.
Nutrient levels within microbial mats are only partially related to the surrounding waters in streams and/or ponds, given the rapid dynamics of elements within the microbial mats (e.g. interstitial water steep gradients) (Wood et al., 2015). The ecological stoichiometry referred to in ratios (e.g. C/N or N/P) focuses on the relationships between organism, ecosystem structure, and function with the environment (Elser et al., 2000). According to our results, C/N and N/P ratios were significantly lower in LI, AI, and CP samples compared with BP mat, grown in a nutrient-poor area, far from the coast and geographically isolated from marine vertebrates. Therefore, microbial mats from penguin-impacted locations were related to a more eutrophic status (based on the Redfield Ratio C:N:P), compared with a more common oligotrophic status displayed by BP. These differences in the trophic status could be associated with processes of “ornitheutrophication,” previously described for soils and waters where environmental conditions were altered because of the establishment of breeding colonies of seabirds (Otero et al., 2018). Moreover, elemental ratios can be related to important ecological features such as ecosystem-specific composition and diversity (Venterink et al., 2003), processes of organic matter decomposition (Güsewell and Gessner, 2009), and with the ability of organisms to adapt to environmental stresses (Woods et al., 2003). Therefore, the differences observed in nutrient levels would have consequences in the entire biological community that constitute these microecosystems.
Our results also evidenced that 15N values were significantly higher in penguin-impacted microbial mats. These data are consistent with previous studies in other Antarctic and sub-Antarctic regions, where δ15N values of cryptogams (Erskine et al., 1998; Bokhorst and Convey, 2016) and microbial mats (Wang et al., 2020) decreased with increasing distance from penguin colonies. Crittenden et al. (2015) showed that NH3 emitted from a penguin colony at Cape Hallett had positive δ15N values, reflecting 15N enriched in the penguin guano and impacted soils. This may be related to increases in marine N source due to a higher trophic position of seabirds (Cherel et al., 2007) and preferential volatilization of 14N from guano (Mizutani et al., 1985). But higher values of 15N were found throughout the entire food web of LI, AI, and CP mats, as previously reported when studying the impact of penguin guano on terrestrial and aquatic Antarctic ecosystems (Bokhorst and Convey, 2016; Bokhorst et al., 2019; Wang et al., 2020). Therefore, N derived from penguins and other marine vertebrates moves through food webs from primary producers to consumers, and all the organisms would be directly linked to the marine-derived δ15N signature.
Impact of Penguin-Derived Nutrients on the Food Webs and Trophic Relationships of Microbial Mats
The consequences of nutrient load into the terrestrial ecosystem, mainly by penguins but also by other seabirds and marine mammals, may become more evident in primary producers than in consumers, as typically N and P limit primary production in marine and terrestrial environments (Chadwick et al., 1999; Mills et al., 2004). However, synergistic interactions between limited supplies of these nutrients are widespread across aquatic and terrestrial systems (Elser et al., 2007), and nutrient colimitation and other kinds of interactions between limiting resources also need to be considered (Gleeson and Tilman, 1992; Danger et al., 2008; Harpole et al., 2011). Allochthonous nutrient enrichment of Antarctic lakes by local fauna has been documented, reporting increases in chlorophyll concentrations due to these processes (Hawes, 1990; Vinocur and Unrein, 2000; Izaguirre et al., 2001; Rochera, 2012). In the studied cyanobacterial microbial mats, chla concentrations varied in the samples within the range of the values reported in previous studies from Antarctica (Hawes et al., 2019), with more than a two-fold increase between LI and BP. Also, a positive correlation appeared between δ15N and chla concentration. Therefore, nutrient levels may influence the activity and growth of primary producers in these forced ecosystems. However, the length of the season with liquid water available, referred to in this study as the number of days per year with temperatures above the freezing point (TAF), could be relevant by limiting the growth, as suggested by Billi and Potts (2002) even in nutrient-replete ecosystems, thus explaining the positive correlation shown between TOC and TAF.
Understanding the structure of the food web is necessary to determine how organisms and the environment interact and therefore the functioning of the ecosystem (Perkins et al., 2014), but there are few articles published on these interactions in microbial mats. According to our results, the trophic structure of the microbial mats was different among locations, with consumers appearing in different abundances and trophic positions within the studied food webs. Our data indicated that tardigrades and rotifers appeared by dozens in samples taken in the vicinity of penguin colonies and related to more eutrophic conditions, whereas nematodes proportionally dominated BP, considered in more oligotrophic status. These differences shown in their distribution could be partially revealed by the biotic interactions among the meiofaunal groups, as suggested for rotifers and Panagrolaimus sp. on penguin-impacted soils (Porazinska et al., 2002; Sohlenius et al., 2004). However, our results did not show a significant correlation to support the co-occurrence among the studied taxa, although the taxonomic diversity within each group was not considered. It has been also suggested that primary producer populations affect the abundance and diversity of higher trophic levels (Bokhorst et al., 2015; Zawierucha et al., 2019), which in turn represent an important driver of their populations by grazing and nutrient recycling (Zawierucha et al., 2018). The most widespread tardigrade genera in Antarctica (Acutuncus, Hypsibius, and Isohypsibius) are defined as grazers (Nelson et al., 2018). Thus, higher densities of grazers at penguin-impacted microbial mats may be explained by the availability of other resources such as green algae (Vonnahme et al., 2016), as suggested by increases in chla concentration, and probably higher abundances of fungi (Zwolicki et al., 2016; Zawierucha et al., 2019), as suggested by increases in ergosterol concentration. Fungi, with a key role as decomposers within the mat community (Velázquez et al., 2017), also appeared highly positively correlated with P and δ15N and highly negatively correlated with C/N and N/P. These data agree with previous studies, where seabirds-derived nutrients condition the growth rate and diversity of fungal populations (Osono et al., 2006). Therefore, our results suggest that penguin-derived nutrients condition trophic community within microbial mats at two levels: through derived remains (carcasses, pellets, feathers, eggs, etc.) that provide substrates for decomposers and detritivores, and through facilitating the development of populations of herbivores through the fertilizing effects of excrements on primary producers.
Based on the potential food sources provided by the model and the feeding ecological strategies of consumers, our results suggest that the differences in δ15N may be related to an enrichment of δ15N for rotifers intakes caused by preferential consumption of DOM and/or bacteria, and for tardigrades caused by ingesting POM and algae, as previously suggested in microbial mats from Antarctica (Velázquez et al., 2017; Almela et al., 2019). Data on feeding preferences of Antarctic rotifers (bdelloids), which are filter feeders, suggested that the diet consisted of dead organic matter and unicellular algae (Iakovenko et al., 2015), although they could probably feed on bacteria (Jaroměřská et al., 2021). In the studied microbial mats, POM fraction would be composed of EPS, detritus, bacteria, and ciliates, but we also found abundant unicellular cyanobacteria (approximately 1.5 μm in diameter) forming this matrix. As nitrogen-fixing organisms, such as cyanobacteria, have high content of proteins and high 15N values (Kohler et al., 2018), tardigrades from LI mat feeding on them would consequently present higher 15N compared with rotifers, as it has been suggested for tardigrades in Arctic cryoconite holes (Jaroměřská et al., 2021). Fungi should also be considered as a potential food source for these herbivores (Darby and Neher, 2016), although their role within the trophic relationships could not be studied. In BP mat, tardigrades probably feed on POM, which would contain moss fragments, present in the surroundings but rarely within microbial mats. Because cyanobacteria are such a significant portion of the microbial mats, we expected a trophic relationship with nematodes, as shown in different ecosystems (Darby and Neher, 2016; Andriuzzi et al., 2018). However, nematodes did not appear trophically related to cyanobacteria and probably feed on bacteria (Nielsen et al., 2011; Caruso et al., 2019) and other consumers, as suggested by Almela et al. (2019). Therefore, the shift in POM composition could explain the isotopic differences between tardigrades and rotifers, which in turn would be related to the availability of nutrients and the presence of different primary producer densities in the studied microbial mats. These results also suggest that much of the C processed by invertebrate food webs is derived from detrital resources, as described by Koltz et al. (2018) for the Alaskan tundra ecosystem. Furthermore, the differences shown in δ15N among consumers suggest that tardigrades positioned at the top of the trophic web in LI mat, with a typical enrichment of 2–4‰ from diet to consumer (Post, 2002; Vanderklift and Ponsard, 2003; Caut et al., 2009). In the remaining samples, which includes “penguin-impacted” and “macrofauna-free” mats, nematodes were the top consumers, as shown for Antarctic soils (Shaw et al., 2018). The differences in 15N observed in tardigrades could also suggest a different trophic level in LI for Hypsibiidae specimens, or a higher 15N enrichment of this group by feeding directly on marine-derived δ15N enriched primary producers in comparison with the other consumers, which could act mainly as detritivores. We suggest that further research is needed to better understand species diversification and co-occurrence through the different levels of consumers to evaluate in detail the alterations of meiofaunal groups due to penguin-derived nutrients in microbial mats.
Consequences of Penguin-Derived Nutrients on Microbial Community Composition and Bacterial Diversity
Our analysis revealed that inorganic nitrogen (N-NH4+ and NO3–) is a key factor in driving bacterial community composition, which agree with previous studies of polar microbial communities from terrestrial ecosystem (Kleinteich et al., 2017; Valdespino-Castillo et al., 2018; Dillon et al., 2020; Jackson et al., 2021). A similar pattern was determined for Antarctic soils (Smith et al., 2010), where NH3, NO3–, and total N, among other physicochemical parameters, were driving the microbial community composition. While P has been shown as the most limiting nutrient in processes for microbial autotrophs in glacial retreat areas (Darcy et al., 2018), it is well known that N is a key nutrient in ecosystems, and its availability is correlated with the presence of marine vertebrates (Bokhorst et al., 2019; Wang et al., 2020). Our results showed that penguin-derived nutrients influence the community composition of microbial mats with significant differences between “penguin-impacted” and “macrofauna-free” samples. Bacteria belonging to the order Clostridiales and Flavobacteriales had higher relative abundances in those microbial mats influenced by penguins, as was reported in ornithogenic soils (Santamans et al., 2017; Guo et al., 2018) and lakes frequented by different marine vertebrates in Antarctica (Picazo et al., 2019). At family level, Flavobacteriaceae, Crocinitomicaceae, Rhodothermaceae, and Xanthomonadaceae were significantly more abundant in samples affected by penguins. Some of these taxa have been associated with soils influenced by the presence of different macrofaunal groups (Ramírez-Fernández et al., 2021) and are also recognized as initial metabolizers of labile C inputs in soils (Padmanabhan et al., 2003) during initial stages of the microbial loop. Different genera from these families, which were also found in our samples (e.g. Flavobacterium and Gelidibacter), are common members of penguin gastrointestinal tract microbiota (Barbosa et al., 2016). Therefore, these results suggest that macrofaunal groups directly influence the bacterial composition of Antarctic microbial mats. This could determine the internal nutrient cycling processes within microbial mats and potentially explain the relationships between their bacterial communities and the nutrient content.
Cyanobacteria ranked as the second phylum with the highest number of assigned sequences only in BP mat, showing lower relative abundances in penguin-impacted samples. These differences were observed when comparing oligotrophic to nutrient-rich lakes in Antarctica, suggesting that high N concentration associated with enrichment by marine vertebrates would tend to favor non-cyanobacterial taxa (Pearce et al., 2005). Significant differences among penguin-impacted and non-impacted microbial communities were also found for Cyanobacteria at family level. According to our results, sequences affiliated to the nitrogen-related taxa, such as Pseudanabaenaceae and Nostocales, were more abundant in macrofauna-free mat. Pseudanabaena and Geitlerinema potentially fix N2 into NH4+-NH3 (Bergman et al., 1997; Fernández-Valiente et al., 2007; Grim and Dick, 2016), and nitrifying bacteria, such as Nitrospira found in BP, oxidize it into NO2– and NO3–, increasing the availability of nitrogen to the community. This would be the main N input pathway in mats from oligotrophic environments, as assimilating 15N-depleted abiogenic N results in negative δ15N values (Wang et al., 2020), as shown in BP. Marine vertebrates breeding areas, especially penguin rookeries, are considered nutrient hotspots, as a large proportion of the excreted N and P is present in highly bioavailable forms (Otero et al., 2018). The uric acid contained in the feces of these seabirds serves as a substrate for its mineralization in different forms of N through ammonification and nitrification processes. Nostocales activity in non-enrichment environments may constitute up to 23% of the assimilated nitrogen by mats community (Fernández-Valiente et al., 2007). But considering that N would be widely available in areas with local macrofauna, especially when penguins occur (De La Peña-Lastra, 2021), diazotrophs, such as Nostocales, may be less competitive in these communities, and consequently, their relative abundance would decrease. These results suggest that the presence of penguins and other seabirds, through the nutrients derived from its biological activity, has a significant influence on bacterial community composition and a strong influence on the nutrient cycle, affecting N utilization strategies of microbial mats.
Our study showed that richness and diversity of bacterial communities from Antarctic microbial mats are significantly influenced by the presence of nutrients derived from penguin activity. Thus, a lower number of bacterial species appeared in those mats from “penguin-impacted” locations and related to environments associated with more eutrophic conditions. These results are consistent with Pearce et al. (2005), where nutrient-enriched lake, due to seal activity, showed reduced species richness in comparison to more oligotrophic lakes. This reduction in bacterial species richness was also accompanied by an increase in evenness among key groups. In proportion to the overall population size, Burkholderiales, Flavobacteriales, and Leptolyngbyales sequences explained 31% of the bacterial community in BP, whereas in LI and CP, mats explained 55–65% of total sequences. Also, while in “penguin-impacted” mats, the taxa (order level) with relative abundances lower than 1% represented between 5 and 8% of the total, in BP it reached 11%. Nutrient recycling by bacteria might be extremely relevant for microbial mat survival and regeneration, in an ecosystem where the input of allochthonous organic C is limited due the predominant unproductive surrounding catchment (Lyons et al., 2013). A greater number of families mainly associated with Proteobacteria and Bacteroidetes, but also with Firmicutes and Acidobacteria, appeared significantly associated with BP. These groups have been classified as heterotrophic microbes and are major players in microbial communities around the Globe (Rasuk et al., 2016; Echenique-Subiabre et al., 2018; Valdespino-Castillo et al., 2018), with a key role in organic matter decomposition processes in Arctic soils (Tao et al., 2020). Also, the presence of Cyanobacteria and Chloroflexi families significantly associated with these nutrient-poor ecosystems, which can utilize different portions of the radiation spectrum for photosynthesis (Ley et al., 2006), suggests the importance of the autotrophic within this communities. Therefore, there is an apparent change in dominance related to penguin-derived nutrients, which, together with species richness, induces oligotrophic systems into communities previously associated with low resistance to environmental change, but related to a higher metabolic versatility.
Conclusion
We conducted a comprehensive study on the impact of penguins on microbial mat communities. Using the penguins as an example on polar microbial mat communities, this study illustrates how nutrients derived from marine vertebrates can change community composition and trophic structure of the microbial mats in Antarctica. Our results showed that P concentrations, C/N and N/P ratios, and δ15N values of “penguin-impacted” microbial mats were significantly higher than values obtained for “macrofauna-free” sample, indicating a strong influence of their presence in these communities. Nutrients derived from penguin colonies and other marine vertebrates influenced the diversity and structure of bacterial communities, even affecting N utilization strategies of microbial mats. Furthermore, trophic interactions of communities within microbial mats resulted altered, as well as the relative abundance and trophic position of meiofaunal groups. This study contributes to the understanding of the biocomplexity of microbial mats, one of the most diverse ecosystems in non-marine Antarctica, by focusing not only on microbial communities but also on meiofauna. Our results are also relevant in the panorama of global change as nutrient availability in the ecosystem could change and modify the ecological relationships within the ecosystem, throughout alterations in the local fauna. Studies considering the taxonomy of meiofaunal groups would help to better understand the trophic relationships that take place in Antarctic cyanobacterial-based microbial mats.
Data Availability Statement
The datasets presented in this study can be found in online repositories. The names of the repository/repositories and accession number(s) can be found below: https://www.ncbi.nlm.nih.gov/genbank/, PRJNA678484.
Author Contributions
PA, AJ, and AQ collected the samples. PA analyzed the experimental data and wrote the initial manuscript. All authors designed the study and contributed to elaborate the final manuscript.
Funding
This work was supported by the Spanish Agencia Estatal de Investigación (AEI) and Fondo Europeo de Desarrollo Regional (FEDER), Grants PID2020-116520RB-I00, CTM2016-79741-R, and PCIN-2016-001. PA was supported by a FPI-contract fellowship (BES-2017-080558) from MINECO.
Conflict of Interest
The authors declare that the research was conducted in the absence of any commercial or financial relationships that could be construed as a potential conflict of interest.
Publisher’s Note
All claims expressed in this article are solely those of the authors and do not necessarily represent those of their affiliated organizations, or those of the publisher, the editors and the reviewers. Any product that may be evaluated in this article, or claim that may be made by its manufacturer, is not guaranteed or endorsed by the publisher.
Acknowledgments
We are grateful to Vanessa Peiro and Ramon Redondo from SIdI (UAM), Rosana Torremocha and Ricardo Ramos from FPCM, Noemi Guil, member of field team from MICROAIRPOLAR project Sergi González, Unidad Técnica Marina (UTM), Navy crew of BIO Hespérides and B/O Sarmiento de Gamboa. We acknowledge the computer resources, technical expertise and assistance provided by the Centro de Computación Científica at the Universidad Autónoma de Madrid (CCC-UAM). The penguin image from Figure 1 is courtesy of Vecteezy. We are grateful to the reviewers and the editors for their assistance in improving the manuscript.
Supplementary Material
The Supplementary Material for this article can be found online at: https://www.frontiersin.org/articles/10.3389/fmicb.2022.841175/full#supplementary-material
Supplementary Figure 1 | Correlogram for the independent variables identified as relevant to explain the differences in the microbial mats. Colors of circles indicate the strength and direction of correlation. Statistical significance was inferred for p < 0.05.
Supplementary Figure 2 | Light microscopy and fluorescence micrographs of the main primary produces and consumers within the food webs. (A) Chlamydomonadaceae (green algae) visualized in LI. (B) Nostocalean morphotypes (cyanobacteria) from BP mat. Oscillatoria filaments (cyanobacteria) visualized in BP with light microscopy (C) and fluorescence microscopy (D). Black and white triangles indicate the presence of Leptolyngbya sp. filaments (cyanobacteria). (E) Leptolyngbya sp. filaments (cyanobacteria) in BP. (F) Prasiola sp. (green algae) visualized in AI. (G) Hypsibiidae tardigrades, (H) rotifers, and (I) nematode found in CP.
Supplementary Table 1 | Description of samples and isotopic values (δ13C and δ15N) of sources and consumers included in trophic web analysis. Isotopic values are presented as per mille (‰) and related to the international standards of the Pee Dee Belemnite for carbon and atmospheric N2 for nitrogen. Standard deviation (SD) is shown between parentheses.
Supplementary Table 2 | DESeq analysis of ASVs (family level) comparing the different microbial mats. Families significantly different in abundance between “penguin-affected” and “macrofauna-free” microbial mats are shown.
References
Aguirre de Cárcer, D., Denman, S. E., McSweeney, C., and Morrison, M. (2011). Strategy for modular tagged high-throughput amplicon sequencing. Appl. Environ. Microbiol. 77, 6310–6312. doi: 10.1128/AEM.05146-11
Almela, P., Velázquez, D., Rico, E., Justel, A., and Quesada, A. (2019). Carbon pathways through the food web of a microbial mat from Byers Peninsula. Antarctica. Front. Microbiol. 10:628. doi: 10.3389/fmicb.2019.00628
Anagnostidis, K., and Komárek, J. (1988). Modern approach to the classification system of cyanophytes. 3-Oscillatoriales. Arch. Hydrobiol. Suppl. Algol. Stud. 1988, 327–472.
Andriuzzi, W. S., Stanish, L. F., Simmons, B. L., Jaros, C., Adams, B. J., Wall, D. H., et al. (2018). Spatial and temporal patterns of microbial mats and associated invertebrates along an Antarctic stream. Polar Biol. 41, 1911–1921. doi: 10.1007/s00300-018-2331-4
ATCM (2002). Management Plan for Antarctic Specially Protected Area (ASPA) No. 117 Avian Island, Marguerite Bay, Antarctic Peninsula. Berlin: ATCM.
ATCM (2011). Management Plan for Antarctic Specially Protected Area (ASPA) No. 126 Byers Peninsula, Livingston Island, South Shetland Islands. Berlin: ATCM.
ATCM (2013). Management Plan for Antarctic Specially Protected Area (ASPA) No. 134 Cierva Point and Offshore Islands, Danco Coast, Antarctic Peninsula. Berlin: ATCM.
ATCM (2017). Management Plan for Antarctic Specially Protected Area (ASPA) No. 115 Lagotellerie Island, Marguerite Bay, Graham Land. Berlin: ATCM.
Ball, B. A., Tellez, C. R., and Virginia, R. A. (2015). Penguin activity influences soil biogeochemistry and soil respiration in rookeries on Ross Island, Antarctica. Polar Biol. 38, 1357–1368. doi: 10.1007/s00300-015-1699-7
Barbosa, A., Balagué, V., Valera, F., Martínez, A., Benzal, J., Motas, M., et al. (2016). Age-related differences in the gastrointestinal microbiota of chinstrap penguins (Pygoscelis antarctica). PLoS One 11:e0153215. doi: 10.1371/journal.pone.0153215
Bergman, B., Gallon, J., Rai, A., and Stal, L. (1997). N2 fixation by non-heterocystous cyanobacteria. FEMS Microbiol. Rev. 19, 139–185. doi: 10.1016/s0168-6445(96)00028-9
Billi, D., and Potts, M. (2002). Life and death of dried prokaryotes. Res. Microbiol. 153, 7–12. doi: 10.1016/s0923-2508(01)01279-7
Bokhorst, S., Asplund, J., Kardol, P., and Wardle, D. A. (2015). Lichen physiological traits and growth forms affect communities of associated invertebrates. Ecology 96, 2394–2407. doi: 10.1890/14-1030.1
Bokhorst, S., and Convey, P. (2016). Impact of marine vertebrates on Antarctic terrestrial micro-arthropods. Antarct. Sci. 28, 175–186. doi: 10.1017/s0954102015000607
Bokhorst, S., Convey, P., and Aerts, R. (2019). Nitrogen inputs by marine vertebrates drive abundance and richness in Antarctic terrestrial ecosystems. Curr. Biol. 29, 1721–1727. doi: 10.1016/j.cub.2019.04.038
Bokulich, N. A., Kaehler, B. D., Rideout, J. R., Dillon, M., Bolyen, E., Knight, R., et al. (2018). Optimizing taxonomic classification of marker-gene amplicon sequences with QIIME 2’s q2-feature-classifier plugin. Microbiome 6, 1–17. doi: 10.1186/s40168-018-0470-z
Bolyen, E., Rideout, J. R., Dillon, M. R., Bokulich, N. A., Abnet, C. C., Al-Ghalith, G. A., et al. (2019). Reproducible, interactive, scalable and extensible microbiome data science using QIIME 2. Nat. Biotechnol. 37, 852–857.
Bonilla, S., Villeneuve, V., and Vincent, W. F. (2005). Benthic and planktonic algal communities in a high arctic lake: pigment structure and contrasting responses to nutrient enrichment 1. J. Phycol. 41, 1120–1130. doi: 10.1111/j.1529-8817.2005.00154.x
Broady, P. A., and Kibblewhite, A. L. (1991). Morphological characterization of Oscillatoriales (Cyanobacteria) from Ross Island and southern Victoria Land, Antarctica. Antarct. Sci. 3, 35–45. doi: 10.1017/s095410209100007x
Callahan, B. J., McMurdie, P. J., Rosen, M. J., Han, A. W., Johnson, A. J. A., and Holmes, S. P. (2016). DADA2: high-resolution sample inference from Illumina amplicon data. Nat. Methods 13, 581–583. doi: 10.1038/nmeth.3869
Caruso, T., Hogg, I. D., Nielsen, U. N., Bottos, E. M., Lee, C. K., Hopkins, D. W., et al. (2019). Nematodes in a polar desert reveal the relative role of biotic interactions in the coexistence of soil animals. Commun. Biol. 2, 1–9. doi: 10.1038/s42003-018-0260-y
Caut, S., Angulo, E., and Courchamp, F. (2009). Variation in discrimination factors (δ15N and δ13C): the effect of diet isotopic values and applications for diet reconstruction. J. Appl. Ecol. 46, 443–453. doi: 10.1111/j.1365-2664.2009.01620.x
Chadwick, O. A., Derry, L. A., Vitousek, P. M., Huebert, B. J., and Hedin, L. O. (1999). Changing sources of nutrients during four million years of ecosystem development. Nature 397, 491–497. doi: 10.1038/17276
Cherel, Y., Hobson, K. A., Guinet, C., and Vanpe, C. (2007). Stable isotopes document seasonal changes in trophic niches and winter foraging individual specialization in diving predators from the Southern Ocean. J. Anim. Ecol. 76, 826–836. doi: 10.1111/j.1365-2656.2007.01238.x
Clucas, G. V., Dunn, M. J., Dyke, G., Emslie, S. D., Levy, H., Naveen, R., et al. (2014). A reversal of fortunes: climate change ‘winners’ and ‘losers’ in Antarctic Peninsula penguins. Sci. Rep. 4, 1–7. doi: 10.1038/srep05024
Crittenden, P. D., Scrimgeour, C. M., Minnullina, G., Sutton, M. A., Tang, Y. S., and Theobald, M. R. (2015). Lichen response to ammonia deposition defines the footprint of a penguin rookery. Biogeochemistry 122, 295–311. doi: 10.1007/s10533-014-0042-7
Danger, M., Daufresne, T., Lucas, F., Pissard, S., and Lacroix, G. (2008). Does Liebig’s law of the minimum scale up from species to communities? Oikos 117, 1741–1751. doi: 10.1111/j.1600-0706.2008.16793.x
Darby, B. J., and Neher, D. A. (2016). Microfauna within biological soil crusts in Biological soil crusts: an organizing principle in drylands. New York, NY: Springer, 139–157.
Darcy, J. L., Schmidt, S. K., Knelman, J. E., Cleveland, C. C., Castle, S. C., and Nemergut, D. R. (2018). Phosphorus, not nitrogen, limits plants and microbial primary producers following glacial retreat. Sci. Adv. 4:eaaq0942. doi: 10.1126/sciadv.aaq0942
Davey, M. C. (1993a). Carbon and nitrogen dynamics in a maritime Antarctic stream. Freshw. Biol. 30, 319–330. doi: 10.1111/j.1365-2427.1993.tb00812.x
Davey, M. C. (1993b). Carbon and nitrogen dynamics in a small pond in the maritime Antarctic. Hydrobiologia 257, 165–175. doi: 10.1007/bf00765009
De La Peña-Lastra, S. (2021). Seabird droppings: Effects on a global and local level. Sci. Total Environ. 754:142148. doi: 10.1016/j.scitotenv.2020.142148
Dillon, M. L., Hawes, I., Jungblut, A. D., Mackey, T. J., Eisen, J. A., Doran, P. T., et al. (2020). Environmental control on the distribution of metabolic strategies of benthic microbial mats in Lake Fryxell. Antarctica. PLoS One 15:e0231053. doi: 10.1371/journal.pone.0231053
Dunn, M. J., Jackson, J. A., Adlard, S., Lynnes, A. S., Briggs, D. R., Fox, D., et al. (2016). Population size and decadal trends of three penguin species nesting at Signy Island, South Orkney Islands. PLoS One 11:e0164025. doi: 10.1371/journal.pone.0164025
Echenique-Subiabre, I., Zancarini, A., Heath, M. W., Wood, S. A., Quiblier, C., and Humbert, J. F. (2018). Multiple processes acting from local to large geographical scales shape bacterial communities associated with Phormidium (cyanobacteria) biofilms in French and New Zealand rivers. Sci. Rep. 8, 1–12. doi: 10.1038/s41598-018-32772-w
Elser, J. J., Bracken, M. E., Cleland, E. E., Gruner, D. S., Harpole, W. S., Hillebrand, H., et al. (2007). Global analysis of nitrogen and phosphorus limitation of primary producers in freshwater, marine and terrestrial ecosystems. Ecol. Lett. 10, 1135–1142. doi: 10.1111/j.1461-0248.2007.01113.x
Elser, J. J., Fagan, W. F., Denno, R. F., Dobberfuhl, D. R., Folarin, A., Huberty, A., et al. (2000). Nutritional constraints in terrestrial and freshwater food webs. Nature 408, 578–580. doi: 10.1038/35046058
Erskine, P. D., Bergstrom, D. M., Schmidt, S., Stewart, G. R., Tweedie, C. E., and Shaw, J. D. (1998). Subantarctic Macquarie Island-a model ecosystem for studying animal-derived nitrogen sources using 15N natural abundance. Oecologia 117, 187–193. doi: 10.1007/s004420050647
Fernández-Valiente, E., Camacho, A., Rochera, C., Rico, E., Vincent, W. F., and Quesada, A. (2007). Community structure and physiological characterization of microbial mats in byers peninsula, Livingston Island (South Shetland Islands, Antarctica). FEMS Microbiol. Ecol. 59, 377–385. doi: 10.1111/j.1574-6941.2006.00221.x
Gessner, M. O., and Chauvet, E. (1993). Ergosterol-to-biomass conversion factors for aquatic hyphomycetes. Appl. Environ. Microbiol. 59, 502–507. doi: 10.1128/aem.59.2.502-507.1993
Gessner, M. O. (2020). Ergosterol as a measure of fungal biomass, in Methods to study litter decomposition. New York, NY: Springer, 247–255.
Gleeson, S. K., and Tilman, D. (1992). Plant allocation and the multiple limitation hypothesis. Amer. Naturalist 139, 1322–1343. doi: 10.1086/285389
Grim, S. L., and Dick, G. J. (2016). Photosynthetic versatility in the genome of Geitlerinema sp. PCC 9228 (formerly Oscillatoria limnetica ‘Solar Lake’), a model anoxygenic photosynthetic cyanobacterium. Front. Microbiol. 7:1546. doi: 10.3389/fmicb.2016.01546
Guo, Y., Wang, N., Li, G., Rosas, G., Zang, J., Ma, Y., et al. (2018). Direct and indirect effects of penguin feces on microbiomes in Antarctic ornithogenic soils. Front. Microbiol. 9:552. doi: 10.3389/fmicb.2018.00552
Güsewell, S., and Gessner, M. O. (2009). N: P ratios influence litter decomposition and colonization by fungi and bacteria in microcosms. Funct. Ecol. 23, 211–219. doi: 10.1111/j.1365-2435.2008.01478.x
Hammer, O., Harper, D. A., and Ryan, P. D. (2001). PAST: Paleontological statistics software package for education and data analysis. Palaeontol. Electron 4:9.
Hansson, L.-A., and Tranvik, L. J. (2003). Food webs in sub-Antarctic lakes: a stable isotope approach. Polar Biol. 26, 783–788. doi: 10.1007/s00300-003-0553-5
Harpole, W. S., Ngai, J. T., Cleland, E. E., Seabloom, E. W., Borer, E. T., Bracken, M. E., et al. (2011). Nutrient co-limitation of primary producer communities. Ecol. Lett. 14, 852–862. doi: 10.1111/j.1461-0248.2011.01651.x
Hawes, I. (1990). Eutrophication and vegetation development in maritime Antarctic lakes in Antarctic ecosystems. New York, NY: Springer, 83–90.
Hawes, I., Howard-Williams, C., and Pridmore, R. (1993). Environmental control of microbial biomass in the ponds of the McMurdo Ice Shelf, Antarctica. Arch. Hydrobiol. 1993, 271–287. doi: 10.1127/archiv-hydrobiol/127/1993/271
Hawes, I., Sumner, D., and Jungblut, A. D. (2019). Complex structure but simple function in microbial mats from Antarctic Lakes in The Structure and Function of Aquatic Microbial Communities. New York, NY: Springer, 91–120.
Hoffmann, L., Komárek, J., and Kaštovskı, J. (2005). System of cyanoprokaryotes (cyanobacteria) state in 2004. Arch. Hydrobiol. Suppl. Algol. Stud. 2005, 95–115. doi: 10.1127/1864-1318/2005/0117-0095
Hughes, K. A., Cowan, D. A., and Wilmotte, A. (2015). Protection of Antarctic microbial communities-‘out of sight, out of mind.’. Front. Microbiol. 6:151. doi: 10.3389/fmicb.2015.00151
Humphries, G., Naveen, R., Schwaller, M., Che-Castaldo, C., McDowall, P., Schrimpf, M., et al. (2017). Mapping application for penguin populations and projected dynamics (MAPPPD): data and tools for dynamic management and decision support. Polar Rec. 53, 160–166. doi: 10.1017/s0032247417000055
Iakovenko, N., Smykla, J., Convey, P., Kašparová, E., Kozeretska, I., Trokhymets, V., et al. (2015). Antarctic bdelloid rotifers: diversity, endemism and evolution. Hydrobiologia 761, 5–43. doi: 10.1007/s10750-015-2463-2
Izaguirre, I., Mataloni, G., Allende, L., and Vinocur, A. (2001). Summer fluctuations of microbial planktonic communities in a eutrophic lake—Cierva Point, Antarctica. J. Plankton Res. 23, 1095–1109. doi: 10.1093/plankt/23.10.1095
Jackson, E. E., Hawes, I., and Jungblut, A. D. (2021). 16S rRNA gene and 18S rRNA gene diversity in microbial mat communities in meltwater ponds on the McMurdo Ice Shelf, Antarctica. Polar Biol. 44, 823–836. doi: 10.1007/s00300-021-02843-2
Jakubas, D., Zmudczyñska, K., Wojczulanis-Jakubas, K., and Stempniewicz, L. (2008). Faeces deposition and numbers of vertebrate herbivores in the vicinity of planktivorous and piscivorous seabird colonies in Hornsund, Spitsbergen. Pol. Polar Res. 2008, 45–58.
Katoh, K., Misawa, K., Kuma, K., and Miyata, T. (2002). MAFFT: a novel method for rapid multiple sequence alignment based on fast Fourier transform. Nucleic Acids Res. 30, 3059–3066. doi: 10.1093/nar/gkf436
Kleinteich, J., Hildebrand, F., Bahram, M., Voigt, A. Y., Wood, S. A., Jungblut, A. D., et al. (2017). Pole-to-pole connections: similarities between Arctic and Antarctic microbiomes and their vulnerability to environmental change. Front. Ecol. Evol. 5:137.
Kohler, T. J., Stanish, L. F., Liptzin, D., Barrett, J. E., and McKnight, D. M. (2018). Catch and release: Hyporheic retention and mineralization of N-fixing Nostoc sustains downstream microbial mat biomass in two polar desert streams. Limnol. Oceanogr. Lett. 3, 357–364. doi: 10.1002/lol2.10087
Koltz, A. M., Classen, A. T., and Wright, J. P. (2018). Warming reverses top-down effects of predators on belowground ecosystem function in Arctic tundra. Proc. Natl. Acad. Sci. U.S.A. 115, E7541–E7549. doi: 10.1073/pnas.1808754115
Legendre, P., and Anderson, M. J. (1999). Distance-based redundancy analysis: testing multispecies responses in multifactorial ecological experiments. Ecol. Monogr. 69, 1–24.
Ley, R. E., Harris, J. K., Wilcox, J., Spear, J. R., Miller, S. R., Bebout, B. M., et al. (2006). Unexpected diversity and complexity of the Guerrero Negro hypersaline microbial mat. Appl. Environ. Microbiol. 72, 3685–3695. doi: 10.1128/AEM.72.5.3685-3695.2006
Liu, X., Nie, Y., Sun, L., and Emslie, S. D. (2013). Eco-environmental implications of elemental and carbon isotope distributions in ornithogenic sediments from the Ross Sea region, Antarctica. Geochim. Cosmochim. Ac. 117, 99–114. doi: 10.1016/j.gca.2013.04.013
Love, M. I., Huber, W., and Anders, S. (2014). Moderated estimation of fold change and dispersion for RNA-seq data with DESeq2. Genome Biol. 15, 1–21. doi: 10.1186/s13059-014-0550-8
Lynch, H. J., Naveen, R., Trathan, P. N., and Fagan, W. F. (2012). Spatially integrated assessment reveals widespread changes in penguin populations on the Antarctic Peninsula. Ecology 93, 1367–1377. doi: 10.1890/11-1588.1
Lyons, W. B., Welch, K., Welch, S., Camacho, A., Rochera, C., Michaud, L., et al. (2013). Geochemistry of streams from byers peninsula, Livingston Island. Antarct. Sci. 25, 181–190. doi: 10.1017/s0954102012000776
McMurdie, P. J., and Holmes, S. (2013). phyloseq: an R package for reproducible interactive analysis and graphics of microbiome census data. PLoS One 8:e61217. doi: 10.1371/journal.pone.0061217
Mills, M. M., Ridame, C., Davey, M., La Roche, J., and Geider, R. J. (2004). Iron and phosphorus co-limit nitrogen fixation in the eastern tropical North Atlantic. Nature 429, 292–294. doi: 10.1038/nature02550
Mizutani, H., Kabaya, Y., and Wada, E. (1985). Ammonia volatilization and high 15N/14N ratio in a penguin rookery in Antarctica. Geochem. J. 19, 323–327. doi: 10.2343/geochemj.19.323
Morley, S. A., Barnes, D. K., and Dunn, M. J. (2019). Predicting which species succeed in climate-forced polar seas. Front. Mar. Sci. 5:507.
Nelson, D. R., Bartels, P. J., and Guil, N. (2018). “Tardigrade ecology,” in Water bears: the biology of tardigrades. New York, NY: Springer, 163–210.
Nielsen, U. N., Wall, D. H., Adams, B. J., and Virginia, R. A. (2011). Antarctic nematode communities: observed and predicted responses to climate change. Polar Biol. 34, 1701–1711. doi: 10.1002/ecy.2090
Jaroměřská, T., Trubač, J., Zawierucha, K., Vondrovicová, L., Devetter, M., and Žárský, J. D. (2021). Stable isotopic composition of top consumers in Arctic cryoconite holes: revealing divergent roles in a supraglacial trophic network. Biogeosciences 18, 1543–1557. doi: 10.5194/bg-18-1543-2021
Oksanen, J., Blanchet, F. G., Kindt, R., Legendre, P., Minchin, P. R., O’hara, R., et al. (2013). Package ‘vegan.’ Community ecology package, version, Vol. 2. 1–295.
Venterink, H., Wassen, M. J., Verkroost, A., and De Ruiter, P. (2003). Species richness-productivity patterns differ between N-, P-, and K-limited wetlands. Ecology 84, 2191–2199.
Osono, T., Hobara, S., Koba, K., and Kameda, K. (2006). Reduction of fungal growth and lignin decomposition in needle litter by avian excreta. Soil Biol. Biochem. 38, 1623–1630. doi: 10.1016/j.soilbio.2005.12.001
Otero, X. L., De La Peña-Lastra, S., Pérez-Alberti, A., Ferreira, T. O., and Huerta-Diaz, M. A. (2018). Seabird colonies as important global drivers in the nitrogen and phosphorus cycles. Nat. Commun. 9, 1–8. doi: 10.1038/s41467-017-02446-8
Padmanabhan, P., Padmanabhan, S., DeRito, C., Gray, A., Gannon, D., Snape, J., et al. (2003). Respiration of 13C-labeled substrates added to soil in the field and subsequent 16S rRNA gene analysis of 13C-labeled soil DNA. Appl. Environ. Microbiol. 69, 1614–1622. doi: 10.1128/AEM.69.3.1614-1622.2003
Parnell, A. C., Inger, R., Bearhop, S., and Jackson, A. L. (2010). Source partitioning using stable isotopes: coping with too much variation. PLoS One 5:e9672. doi: 10.1371/journal.pone.0009672
Pearce, D. A., Van Der Gast, C. J., Woodward, K., and Newsham, K. K. (2005). Significant changes in the bacterioplankton community structure of a maritime Antarctic freshwater lake following nutrient enrichment. Microbiology 151, 3237–3248. doi: 10.1099/mic.0.27258-0
Perkins, M. J., McDonald, R. A., van Veen, F. F., Kelly, S. D., Rees, G., and Bearhop, S. (2014). Application of nitrogen and carbon stable isotopes (δ15N and δ13C) to quantify food chain length and trophic structure. PLoS One 9:e93281. doi: 10.1371/journal.pone.0093281
Picazo, A., Rochera, C., Villaescusa, J. A., Miralles-Lorenzo, J., Velázquez, D., Quesada, A., et al. (2019). Bacterioplankton community composition along environmental gradients in lakes from Byers peninsula (Maritime Antarctica) as determined by next-generation sequencing. Front. Microbiol. 10:908. doi: 10.3389/fmicb.2019.00908
Porazinska, D. L., Wall, D. H., and Virginia, R. A. (2002). Invertebrates in ornithogenic soils on Ross Island, Antarctica. Polar Biol. 25, 569–574. doi: 10.1007/s00300-002-0386-7
Post, D. M. (2002). Using stable isotopes to estimate trophic position: models, methods, and assumptions. Ecology 83, 703–718. doi: 10.1111/j.1095-8649.2012.03251.x
Price, M. N., Dehal, P. S., and Arkin, A. P. (2010). FastTree 2-approximately maximum-likelihood trees for large alignments. PLoS One 5:e9490. doi: 10.1371/journal.pone.0009490
Quast, C., Pruesse, E., Yilmaz, P., Gerken, J., Schweer, T., Yarza, P., et al. (2012). The SILVA ribosomal RNA gene database project: improved data processing and web-based tools. Nucleic Acids Res. 41, D590–D596. doi: 10.1093/nar/gks1219
Quesada, A., and Vincent, W. F. (2012). Cyanobacteria in the cryosphere: snow, ice and extreme cold in Ecology of cyanobacteria II. New York, NY: Springer, 387–399.
Ramírez-Fernández, L., Orellana, L. H., Johnston, E. R., Konstantinidis, K. T., and Orlando, J. (2021). Diversity of microbial communities and genes involved in nitrous oxide emissions in Antarctic soils impacted by marine animals as revealed by metagenomics and 100 metagenome-assembled genomes. Sci. Total Environ. 788:147693. doi: 10.1016/j.scitotenv.2021.147693
Rasuk, M. C., Fernández, A. B., Kurth, D., Contreras, M., Novoa, F., Poiré, D., et al. (2016). Bacterial diversity in microbial mats and sediments from the Atacama Desert. Microb. Ecol. 71, 44–56. doi: 10.1007/s00248-015-0649-9
R Core Team (2019). R: A Language and Environment for Statistical Computing. Vienna: R Foundation for Statistical Computing.
Rienecker, M. M., Suarez, M. J., Gelaro, R., Todling, R., Bacmeister, J., Liu, E., et al. (2011). MERRA: NASA’s modern-era retrospective analysis for research and applications. J. Clim. 24, 3624–3648.
Ritchie, R. (2008). Universal chlorophyll equations for estimating chlorophylls a, b, c, and d and total chlorophylls in natural assemblages of photosynthetic organisms using acetone, methanol, or ethanol solvents. Photosynthetica 46, 115–126. doi: 10.1007/s11099-008-0019-7
Robinson, S. A., Klekociuk, A. R., King, D. H., Pizarro Rojas, M., Zúñiga, G. E., and Bergstrom, D. M. (2020). The 2019/2020 summer of Antarctic heatwaves. Glob. Chang. Biol. 26, 3178–3180. doi: 10.1111/gcb.15083
Rochera, C. (2012). Functional Ecology of Microbial Freshwater Communities from Byers Peninsula (Livingston Island, Antarctica). Doctoral dissertation. Valencia: Universitat de València.
Rochera, C., Villaescusa, J. A., Velázquez, D., Fernández-Valiente, E., Quesada, A., and Camacho, A. (2013). Vertical structure of bi-layered microbial mats from Byers Peninsula, Maritime Antarctica. Antarct. Sci. 25, 270–276. doi: 10.1017/s0954102012000983
Romaní, A. M., Artigas, J., Camacho, A., Graça, M. A., and Pascoal, C. (2009). “La biota de los ríos: los microorganismos heterotróficos,” in Conceptos y Técnicas en Ecología Fluvial, eds A. Elosegi and S. Sabater (Bilbao: Fundación BBVA), 169–218.
Ropert-Coudert, Y., Chiaradia, A., Ainley, D., Barbosa, A., Boersma, P. D., Brasso, R., et al. (2019). Happy feet in a hostile world? The future of penguins depends on proactive management of current and expected threats. Front. Mar. Sci. 6:248.
Santamans, A. C., Boluda, R., Picazo, A., Gil, C., Ramos-Miras, J., Tejedo, P., et al. (2017). Soil features in rookeries of Antarctic penguins reveal sea to land biotransport of chemical pollutants. PLoS One 12:e0181901. doi: 10.1371/journal.pone.0181901
Schmidt, S., Dennison, W. C., Moss, G. J., and Stewart, G. R. (2004). Nitrogen ecophysiology of Heron Island, a subtropical coral cay of the Great Barrier Reef, Australia. Funct. Plant. Biol. 31, 517–528. doi: 10.1071/FP04024
Shaw, E. A., Adams, B. J., Barrett, J. E., Lyons, W. B., Virginia, R. A., and Wall, D. H. (2018). Stable C and N isotope ratios reveal soil food web structure and identify the nematode Eudorylaimus antarcticus as an omnivore-predator in Taylor Valley, Antarctica. Polar Biol. 41, 1013–1018. doi: 10.1007/s00300-017-2243-8
Smith, J. L., Barrett, J. E., Tusnády, G., Rejtö, L., and Cary, S. C. (2010). Resolving environmental drivers of microbial community structure in Antarctic soils. Antarct. Sci. 22, 673–680. doi: 10.1017/s0954102010000763
Sohlenius, B., Boström, S., and Jönsson, K. I. (2004). Occurrence of nematodes, tardigrades and rotifers on ice-free areas in East Antarctica. Pedobiologia 48, 395–408. doi: 10.1016/j.pedobi.2004.06.001
Tanabe, Y., Yasui, S., Osono, T., Uchida, M., Kudoh, S., and Yamamuro, M. (2017). Abundant deposits of nutrients inside lakebeds of Antarctic oligotrophic lakes. Polar Biol. 40, 603–613. doi: 10.1007/s00300-016-1983-1
Tao, X., Feng, J., Yang, Y., Wang, G., Tian, R., Fan, F., et al. (2020). Winter warming in Alaska accelerates lignin decomposition contributed by Proteobacteria. Microbiome 8, 1–12. doi: 10.1186/s40168-020-00838-5
Thomas, D. N., Fogg, G. E., Convey, P., Fritsen, C. H., Gili, J.-M., Gradinger, R., et al. (2008). The biology of polar regions. Oxford: OUP Oxford.
Valdespino-Castillo, P. M., Cerqueda-García, D., Espinosa, A. C., Batista, S., Merino-Ibarra, M., Taş, N., et al. (2018). Microbial distribution and turnover in Antarctic microbial mats highlight the relevance of heterotrophic bacteria in low-nutrient environments. FEMS Microbiol. Ecol. 94:fiy129. doi: 10.1093/femsec/fiy129
Vanderklift, M. A., and Ponsard, S. (2003). Sources of variation in consumer-diet δ 15 N enrichment: a meta-analysis. Oecologia 136, 169–182. doi: 10.1007/s00442-003-1270-z
Velázquez, D., Jungblut, A. D., Rochera, C., Rico, E., Camacho, A., and Quesada, A. (2017). Trophic interactions in microbial mats on Byers Peninsula, maritime Antarctica. Polar Biol. 40, 1115–1126. doi: 10.1007/s00300-016-2039-2
Vinocur, A., and Unrein, F. (2000). Typology of lentic water bodies at Potter Peninsula (King George Island, Antarctica) based on physical-chemical characteristics and phytoplankton communities. Polar Biol. 23, 858–870. doi: 10.1007/s003000000165
Vonnahme, T., Devetter, M., Žárský, J., Šabacká, M., and Elster, J. (2016). Controls on microalgal community structures in cryoconite holes upon high-Arctic glaciers, Svalbard. Biogeosciences 13, 659–674. doi: 10.5194/bg-13-659-2016
Wang, X., Liu, X., Fang, Y., Jin, J., Wu, L., Fu, P., et al. (2020). Application of δ15N to trace the impact of penguin guano on terrestrial and aquatic nitrogen cycles in Victoria Land, Ross Sea region, Antarctica. Sci. Total Environ. 709:134496. doi: 10.1016/j.scitotenv.2019.134496
Wood, S. A., Depree, C., Brown, L., McAllister, T., and Hawes, I. (2015). Entrapped sediments as a source of phosphorus in epilithic cyanobacterial proliferations in low nutrient rivers. PLoS One 10:e0141063. doi: 10.1371/journal.pone.0141063
Woods, H., Makino, W., Cotner, J., Hobbie, S., Harrison, J., Acharya, K., et al. (2003). Temperature and the chemical composition of poikilothermic organisms. Funct. Ecol. 17, 237–245. doi: 10.1046/j.1365-2435.2003.00724.x
Zawierucha, K., Zmudczyñska-Skarbek, K., Kaczmarek, K., and Wojczulanis-Jakubas, K. (2016). The influence of a seabird colony on abundance and species composition of water bears (Tardigrada) in Hornsund (Spitsbergen, Arctic). Polar Biol. 39, 713–723. doi: 10.1007/s00300-015-1827-4
Zawierucha, K., Buda, J., Pietryka, M., Richter, D., Łokas, E., Lehmann-Konera, S., et al. (2018). Snapshot of micro-animals and associated biotic and abiotic environmental variables on the edge of the south-west Greenland ice sheet. Limnology 19, 141–150. doi: 10.1007/s10201-017-0528-9
Zawierucha, K., Zmudczyñska-Skarbek, K., Guil, N., and Bogdziewicz, M. (2019). Seabirds modify trophic groups, while altitude promotes xeric-tolerant species of Tardigrada in the high Arctic tundra (Svalbard archipelago). Acta Oecol. 98, 50–58. doi: 10.1016/j.actao.2019.05.007
Zmudczyñska, K., Olejniczak, I., Zwolicki, A., Iliszko, L., Convey, P., and Stempniewicz, L. (2012). Influence of allochtonous nutrients delivered by colonial seabirds on soil collembolan communities on Spitsbergen. Polar Biol. 35, 1233–1245. doi: 10.1007/s00300-012-1169-4
Keywords: penguins, nitrogen, phosphorus, microbial mat, trophic relationships, bacterial community, Antarctica
Citation: Almela P, Velázquez D, Rico E, Justel A and Quesada A (2022) Marine Vertebrates Impact the Bacterial Community Composition and Food Webs of Antarctic Microbial Mats. Front. Microbiol. 13:841175. doi: 10.3389/fmicb.2022.841175
Received: 22 December 2021; Accepted: 28 February 2022;
Published: 08 April 2022.
Edited by:
Jesse G. Dillon, California State University, Long Beach, United StatesReviewed by:
Céline Lavergne, Universidad de Playa Ancha, ChileBeatriz Estela Modenutti, National University of Comahue, Argentina
Luisa I. Falcon, National Autonomous University of Mexico, Mexico
Copyright © 2022 Almela, Velázquez, Rico, Justel and Quesada. This is an open-access article distributed under the terms of the Creative Commons Attribution License (CC BY). The use, distribution or reproduction in other forums is permitted, provided the original author(s) and the copyright owner(s) are credited and that the original publication in this journal is cited, in accordance with accepted academic practice. No use, distribution or reproduction is permitted which does not comply with these terms.
*Correspondence: Antonio Quesada, YW50b25pby5xdWVzYWRhQHVhbS5lcw==