- 1State Key Laboratory of Microbial Technology, Shandong University, Qingdao, China
- 2Institute of Marine Science and Technology, Shandong University, Qingdao, China
- 3School of Molecular Biosciences, Washington State University, Pullman, WA, United States
Escherichia coli recA− strains are usually used for cloning to prevent insert instability via RecA-dependent recombination. Here, we report that E. coli BW25113 (recA+) competent cells prepared by using a previously reported transformation and storage solution (TSS) had 100-fold or higher transformation efficiency than the commonly used E. coli cloning strains, including XL1-Blue MRF’. The cloning success rates with E. coli BW25113 were 440 to 1,267-fold higher than those with E. coli XL1-Blue MRF’ when several inserts were assembled into four vectors by using a simple DNA assembly method. The difference was in part due to RecA, as the recA deletion in E. coli BW25113 reduced the transformation efficiency by 16 folds and cloning success rate by about 10 folds. However, the transformation efficiency and the cloning success rate of the recA deletion mutant of E. coli BW25113 are still 12- and >48-fold higher than those of E. coli XL1-Blue MRF’, which is a commonly used cloning strain. The cloned inserts with different lengths of homologous sequences were assembled into four vectors and transformed into E. coli BW25113, and they were stably maintained in BW25113. Thus, we recommend using E. coli BW25113 for efficient cloning and DNA assembly.
Introduction
DNA cloning is a common technique used in biological research, such as constructing CRISPR vectors and plasmids encoding metabolic pathways (Ellis et al., 2011; Sanjana et al., 2014). The conventional method relies on DNA digestion by restriction enzymes and re-ligation by T4 DNA ligase (Cohen et al., 1973; Smolke, 2009). Recently, seamless cloning methods have gained adaptation to meet the requirements of higher efficiency, fidelity, and modularity (Kosuri and Church, 2014). DNA fragments with homology ends or ends that can be recognized by a phage recombinase are generated and assembled either in vivo or in vitro (Fu et al., 2008; Chao et al., 2015). For in vivo methods, these DNA fragments are joined in host cells, such as Saccharomyces cerevisiae or Escherichia coli. When E. coli is used, it usually overexpresses a bacteriophage recombination system to join DNA fragments (House et al., 2004; Wang et al., 2016). For in vitro methods, DNA fragments are treated with enzymes to join DNA fragments. The in vitro methods are widely used for routine cloning, and in vivo methods that often require longer homologous ends are more useful in the assembly of large plasmids (Zhu et al., 2007; Jeong et al., 2012; Zhang et al., 2012). We have developed a “T5 exonuclease DNA assembly” (TEDA) method that combines in vitro digestion by 5′-3′ exonuclease with the rest recombination inside E. coli to join homology ends with high efficiency for routine cloning at low cost, and the method works better with chemically prepared competent cells than with electroporation (Xia et al., 2019).
E. coli K-12 derivatives with a defective recA (recA1) that is defective in recombination are often used for cloning (Bullock, 1987; Anton and Raleigh, 2016). DH5α is engineered to enable blue white screening for the β-galactosidase (LacZ) activity (Hanahan, 1983). XL1-Blue MRF’ with all restriction systems being removed is often used to clone methylated DNA especially from eukaryotes (Bullock, 1987). JM109 is considered as a better choice to clone repetitive DNA (Yanischperron et al., 1985; Metcalf and Wanner, 1993). Stbl2 is a derivative of JM109 (Trinh et al., 1994), and Stbl3 is a hybrid strain of E. coli K-12 and E. coli B; both are developed to clone direct repeats, retroviral sequences, and tandem array genes (Al-Allaf et al., 2013). Derivatives from other E. coli strains are also used. Mach1, derived from E. coli W, is a fast-growing recA− strain (Green and Sambrook, 2018). The commonly used E. coli recA+ strain is BL21(DE3) for the expression of recombinant proteins. Although BL21(DE3) is recA+, its transformation efficiency (TE) is much lower than commonly used cloning strains derived from K-12 (Chan et al., 2013). Clearly, E. coli K-12 recA− strains are the common cloning E. coli strains.
The common cloning E. coli strains often contain additional mutations to benefit cloning. The mutation of the hsdR or hsdS gene prevents the unmethylated DNA from being degraded (Sain and Murray, 1980). The endA mutation avoids random degradation of DNA by endonuclease I after cell lysis, improving both the quantity and quality of plasmid extraction (Lin, 1992; Altermark et al., 2007). DeoR regulates cells’ ability to continuously synthesize deoxyribose, whose mutation increases cells’ ability to absorb large plasmid DNA (Hanahan et al., 1991). The galE mutation reduces the interference from lipopolysaccharide for DNA uptake (van Die et al., 1984). FhuA serves as the receptor for phages T5, T1, φ80, and UC-1 (Hantke and Braun, 1975, 1978; Carmel and Coulton, 1991), and its deletion protects cells from phages infection especially during library construction (Bonhivers et al., 1998). The mutation of these genes is commonly employed for constructing cloning strains.
The recA gene in almost all E. coli cloning strains is inactivated to increase plasmid stability (Clark, 1973). RecA is the core component of homologous recombination (Maraboeuf et al., 1995; Kowalczykowski, 2000; Morimatsu and Kowalczykowski, 2014), and it catalyzes intermolecular recombination to generate dimers that lead to plasmid loss without selection pressure (Summers et al., 2010; Standley et al., 2019). Low copy number plasmid is relatively stable in recA+ strains, but high copy number plasmids easily form multimers and generate plasmid-free cells without selection (Herman-Antosiewicz and Wegrzyn, 1999). With selection pressure, the subpopulation with dimers in recA+ cells is confined due to the reduced growth for cells with plasmid dimers (Summers et al., 2010). Other concerns related to RecA are homologous recombination events within a plasmid or between a plasmid and the chromosome (Sambrook et al., 2017). Although these are valid concerns, direct evidence on their effects on cloning has not been reported.
We report here that E. coli BW25113, a K-12 derivative with a recA+ and hsdR− genotype and the parent of the Keio collection of single-gene knockouts (Baba et al., 2006), had >100-fold higher TE than the commonly used cloning E. coli hosts when intact pBluescript SK- (pSK-) was chemically transformed by using a single step method of the transformation and storage solution (TSS). The cloning success rates with BW25113 were 440 to 1,267-fold higher than those with XL1-Blue MRF’ when various inserts were assembled into 4 vectors by the TEDA method. The cloned inserts with different lengths of homologous sequences were stable in BW25113. Our results promote the reconsideration of using the recA+ strain E. coli BW25113 for DNA assembly in synthetic biology with significantly increased success rates.
Materials and Methods
Strains and Plasmids
The strains used in this study were listed in Supplementary Table S1. All mutants in this research were obtained according to the Wanner’s method (Datsenko and Wanner, 2000). All strains were cultured at 37°C in Luria-Bertani (LB) medium with appropriate antibiotics. Ampicillin, spectinomycin, gentamycin (Gm), chloramphenicol, and kanamycin (Kan) were used at 100, 50, 10, 25, and 50 μg/ml, respectively.
Enzymes and Reagents
Phusion DNA polymerase (Thermo Fisher, United States) was used to amply DNA fragments. PrimeSTAR GXL DNA Polymerase (Takara, Japan) was used to amply DNA fragments whose length are larger than 10 kb. Trans 5 K and 1 kb DNA markers (TransGen Biotech, Beijing) were used to measure the size of DNA fragments in agarose gel electrophoresis. Gel extraction kit (Omega, United States), Plasmid extraction mini Kit (Omega, United States), and TIANamp Bacteria DNA Kit (TianGen, China) were used to purify DNA fragments. All oligos were synthesized by Beijing Genomics Institute. Magnesium chloride, manganese chloride, PEG8000, PEG3350, and dimethylsulfoxide (DMSO) were purchased from Sigma-Aldrich (US), and the rest reagents were purchased from Sangon Biotech (China).
Procedures for Competent Cells Preparation and Transformation
To prepare competent cells, a fresh single colony was normally inoculated into 4-mL LB medium and incubated at 37°C overnight. Then, 1% culture was transferred to 50 ml fresh LB medium and cultured at 37°C until OD600 around 0.5 to prepare competent cells for the TSS method and at 20°C to prepare competent cells for the Hanahan and Inoue methods. The growth was stopped by incubating on ice for 10 min. Cells were harvested by centrifuging at 4°C, 4000 g × 10 min, for the preparation of competent cells with different methods.
The TSS method was modified from two references (Chung et al., 1989; Parrish et al., 2004). Briefly, the harvested cells were resuspended in 1 ml of the TSS buffer (LB-HCl pH = 6.1, 10% PEG3350, 5% DMSO, 10% Glycerol, 10 mM MgSO4, and 10 mM MgCl2), and chilled on ice for 10 min. This mixture was aliquoted into 30 μl per tube. The preparation steps of competent cells were mainly adopted from the study of Chung et al. (1989). In the transformation step, 5 μl of 5 × KCM (0.5 M KCl, 150 mM CaCl2, and 250 mM MgCl2) was mixed with DNA and ddH2O to total 25 μl mixture. The mixture was gently mixed with 25 μl competent cells and incubated on ice for 30 min. A total of 250 μl fresh LB medium was added for cell recovery at 37°C for 1 h. Recovered cultures were spread into the LB plate with the indicated antibiotic. KCM was reported by Parrish et al. (2004).
For the Inoue method (Green and Sambrook, 2020), the harvested cells were washed with 20 ml of the Inoue buffer (55 mM MnCl2, 15 mM CaCl2, 250 mM KCl, and 10 mM PIPES pH = 6.7) and finally resuspended in 1 ml of the Inoue buffer. Then, 75 μl DMSO was added, and the mixture was incubated on ice for 10 min. The cell mixture was aliquoted into 100 μl/tube and could be stored at −80°C.
For the Hanahan method (Green and Sambrook, 2018), the harvested cells were washed with the CCMB80 buffer (80 mM CaCl2, 20 mM MnCl2, 10 mM MgCl2, 10% Glycerol, and 10 mM Potassium acetate buffer pH = 7.0) and finally resuspended in 1 ml CCMB80 buffer. The cell mixture was aliquoted into 100 μl/tube and could be stored at −80°C before use.
The transformation procedures used with the Inoue and the Hanahan method were similar. A 100 μl of competent cells was thawed on ice and mixed with DNA solution. The mixture was incubated on ice for 30 min before heat shock at 42°C for 90 s. After heat shock, 900 μl of fresh LB was added for cell recovery at 37°C for 1 h. Recovered cultures were spread into the LB plate with the indicated antibiotic.
All solutions were autoclaved and used under an ice-cold condition. All operations were performed on ice. The cells were gently resuspended in different buffers. The supernatant after centrifugation was cleanly and quickly pipetted off. The prepared competent cells could be stored at −80°C before use.
The TEs were tested by transforming 0.2 ng intact pBluescript SK- into the testing competent cells. The number of recovery colonies was counted and normalized to 1 μg of the plasmid DNA.
The Preparation of Vectors and Inserts for DNA Assembly
The plasmids and primers used in this study are listed in Supplementary Tables S1 and S2, respectively. The TEDA method was used for plasmid constructions (Xia et al., 2019). Competent cells of defined strains prepared with different methods were used to transform either intact plasmids or DNA cloning mixtures.
Plasmids with artificial homologous characteristics were generated to check for their stability in E. coli recA+ strains. To construct plasmids with homology to E. coli genome, a 3,000-bp fragment was amplified from BW25113 genomic DNA near the rapA gene. The DNA fragment was assembled into plasmids with different replicons.
Direct repeats (DR) were introduced into plasmids through DNA assembly of four DNA fragments. The 5′ end of Kan-resistant gene (5’Kan), the intact Gm resistant gene (Gm), and the 3′ end of Kan-resistant gene (3’Kan) were assembled in pCL1920. Gm was inserted into the open reading frame (ORF) of Kan-resistant gene between 135 and 136 bp. A defined length of 300 bp from 136 to 435 bp of Kan ORF was amplified and inserted before Gm, so that a homologous recombination with the DRs will restore Kan resistance.
Inserts of short and long highly tandem repeats were introduced into plasmids through DNA assembly. For short repeats, 41-bp Tac promoter repeat (TR41) was used. The Tac promoter repeats have been assembled together to promote eGFP expression in a list of plasmids (Li et al., 2012). Fragments harboring 1, 4, 6, or 8 TR41 with an eGFP gene were amplified from corresponding plasmids and cloned into pCL1920. For medium-sized repeats, 300-bp from the C-terminal of eGFP was amplified and inserted after the gene in pCL1920::Pkat-eGFP to generate pCL1920::2TR300 with two repeats and pCL1920::3TR300 with three repeats. The interspace between the three TR regions was 104 and 67 bp. For long repeats, a defined 1-kb fragment containing Pkat-eGFP (TR1000) from pCL1920::Pkat-eGFP was amplified and inserted next to the TR1000 region to produce pCL1920::2TR1000 with two tandem repeats and pCL1920::3TR1000 with three tandem repeats. The interspace between the three tandem repeats regions was 65 and 67 bp. For testing, the inserts harboring tandem repeats were amplified and cloned back into the original pCL1920 plasmid by transforming indicated competent cells.
The TEDA method was used to assemble DNA fragments into various vectors (Xia et al., 2019). The egfp gene under the control of Pkat promoter (Pkat-eGFP) with 20-bp homology ends to SmaI-pSK was used, as previously described (Xia et al., 2019).
The Screening for Positive Colonies and the Stability of Plasmid in Cells
The phenotype was used for initial screening to obtain the positive cloning rates (positive colonies/total colonies). Colonies producing eGFP were green. Further, 20 colonies were checked by using colony PCR. Ten plasmids from the positive colonies were extracted and checked through agarose gel electrophoresis and DNA sequencing (TsingKe BioTech, China).
The stability of plasmids with homology characteristics was initially checked for positive cloning rates. The stability of these plasmids was then tested. Five colonies were randomly picked and cultured in LB medium with appropriate antibiotics. The cultures were continuously transferred into fresh LB medium once reached the stationary phase. After three transfers, the plasmids were extracted and checked by using agarose gel electrophoresis and DNA sequencing.
Results
BW25113 Has a Significantly Higher TE Than Commonly Used E. coli Strains
The E. coli BW25113 cells were made into competent cells by using three commonly used chemical methods: the Hanahan, Inoue, and TSS methods (Chung et al., 1989; Parrish et al., 2004; Green and Sambrook, 2018, 2020). The TSS method gave the highest TE when pSK- was transformed, and its TE was 21- and 71-fold higher than those of the Hanahan and Inoue methods, respectively (Figure 1A). When the E. coli XL1-Blue MRF’ competent cells were prepared by the three methods, the TEs were similar with overlapping error bars (Figure 1A). The TE values of E. coli BW25113 were higher than those of E. coli XL1-Blue MRF’ by 217, 8.1, and 4.6 folds when prepared using the TSS, Hanahan, and Inoue methods, respectively (Figure 1A). The TSS method is a simple method, in which the harvested E. coli cells are resuspended in TSS, stored at −80°C, and used for transformation (Chung et al., 1989; Parrish et al., 2004). The TSS method was then selected to prepare competent cells of BW25113 and several commonly used cloning E. coli strains. The TE of BW25113 was 102-, 202-, 164-, 263-, 279-, and 370-fold higher than that of Omnimax, XL1-Blue MRF’, Stbl3, DH5α, Mach1, and Stbl2, respectively (Figure 1B). The commonly used hosts had similar TEs with differences being <4 folds (Figure 1B).
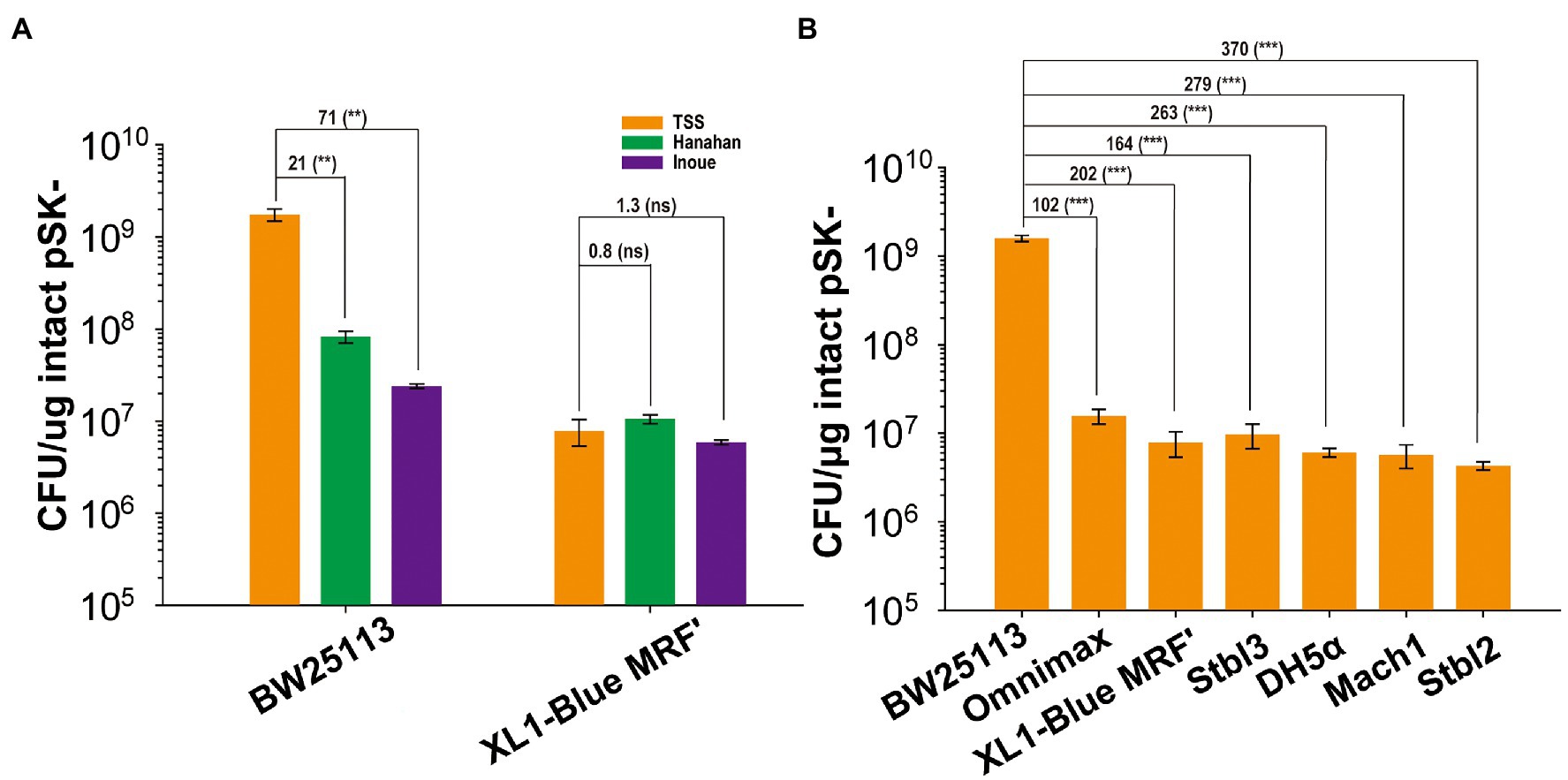
Figure 1. The TEs of E. coli strains. (A) The TEs of competent cells prepared with three different methods for two E. coli strains. (B) The TEs of competent cells prepared with TSS for seven E. coli strains. BW25113, Omnimax, XL1-Blue MRF’, Stbl3, DH5α, Mach1, and Stbl2. Data are averages of three samples with standard deviations (error bars). Unpaired t-tests were performed (** = p < 0.01; *** = p < 0.001; and “ns” = p > 0.05). “ns” means “not significant.” The numbers before (***) represent the difference by folds.
E. coli recA+ Strains Have Much Higher TEs and Viable Cell Counts on Agar Plates Than the Corresponding recA− Strains
Since E. coli BW25113 is a recA+ strain, the effect of RecA was tested. The recA deletion mutant of E. coli BW25113, BWΔrecA, had a TE of 17-fold less than that of BW25113 (Figure 2A). For comparison, hsdR was firstly deleted in MG1655 (MGΔhsdR), as BW25113 is hsdR−. Further deletion of recA in MG1655 ΔhsdR (MGΔhsdR-recA) reduced TE by 15 folds (Figure 2A). The recA deletion mutants grew slightly slower than their parental strains (Supplementary Figures S1A–C). The reductions in TE were recovered by complementing RecA in BWΔrecA (Figure 2B). When E. coli XL1-Blue MRF’ was complemented with recA (XL1-Blue MRF’/recA), its TE increased by 3 folds (Figure 2B), but the growth was not increased (Supplementary Figure S1D).
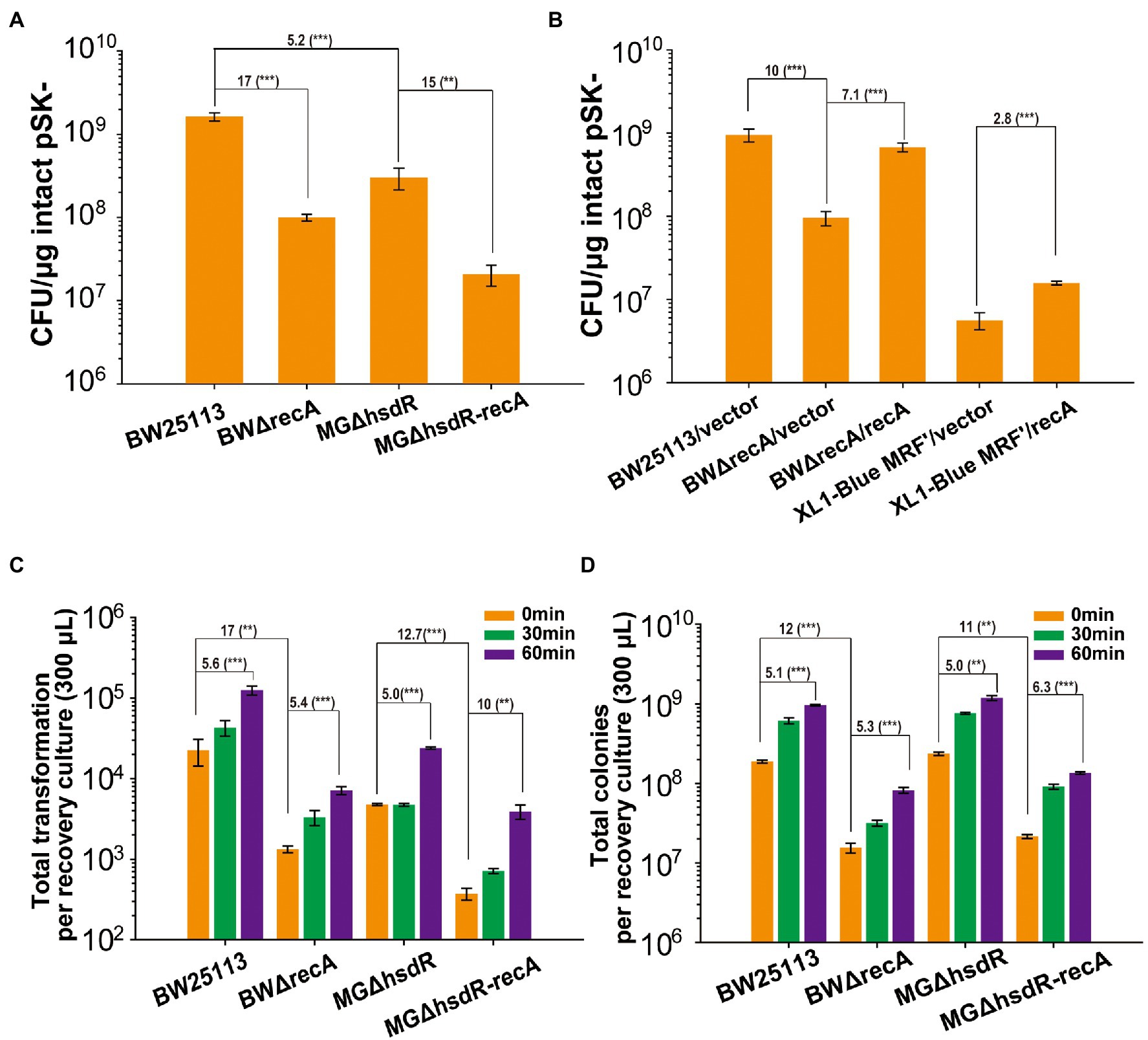
Figure 2. The deletion of recA affects TE and cell viability. (A) The TEs of competent cells prepared with TSS for three strains and their recA mutants. (B) The TEs was recovered by complementing the RecA function in the recA mutant of BW25113 and XL1-Blue MRF’. (C) The transformant colonies of recA mutants and their parent wild-type strains formed on LB plates with the Amp antibiotic after recovering in LB medium for 0 to 60 min. (D) Total colonies of recA mutants and their parent wild-type strains formed on LB plates without antibiotic after recovering in LB medium for 0 to 60 min. Intact pSK- was used for transformation. Unpaired t-tests were performed (** = p < 0.01 and *** = p < 0.001). The numbers before (***) represent the difference by folds. Data are averages of three samples with standard deviations (error bars).
After transformation, the transformants and total live cells in the recovery cultures were determined via counting colonies on agar plates with or without the selective antibiotic. At 0 min of recovery, the transformant colonies and total colonies were 17-fold and 12-fold lower for BWΔrecA than those of BW25113, and 13-fold and 11-fold lower for MG1655ΔhsdR-ΔrecA than those of MG1655ΔhsdR (Figures 2C,D). After 60 min of recovery, the number of colonies increased but the trend was the same (Figures 2C,D). Although all cells were collected at the same OD600 for competent cells preparation, the wild type with recA had significantly more recovered colonies than their recA mutants on agar plates.
We further tested the number of live cells that formed colonies on LB plates when E. coli BW25113, BWΔrecA, XL1-Blue MRF’/vector, and XL1-Blue MRF’/recA grew in LB to OD600 of 0.5. BWΔrecA had 5-fold less live cell counts than BW25113, and XL1-Blue MRF’/vector had 4.6-fold less live cell counts than XL1-Blue MRF’/recA (Figure 3A). Further, the total live cell counts in the recovery culture after transformation had an 11-fold difference between BW25113 and BWΔrecA and only a 4-fold difference between XL1-Blue MRF’/vector and XL1-Blue MRF’/recA (Figure 3B). The results indicate that the number of BWΔrecA cells that can form colonies is further reduced after competent cells preparation, storage, and transformation; however, the number of XL1-Blue MRF’ cells is not further reduced. Our results show that the reduced live cells in the recA− strains competent cells contribute to the reduced TE. Further, the change of G160 into Asp160 of RecA (the recA1 mutant) in XL1-Blue MRF’ reduced its viability and TE, but the effect was less severe than the recA deletion.
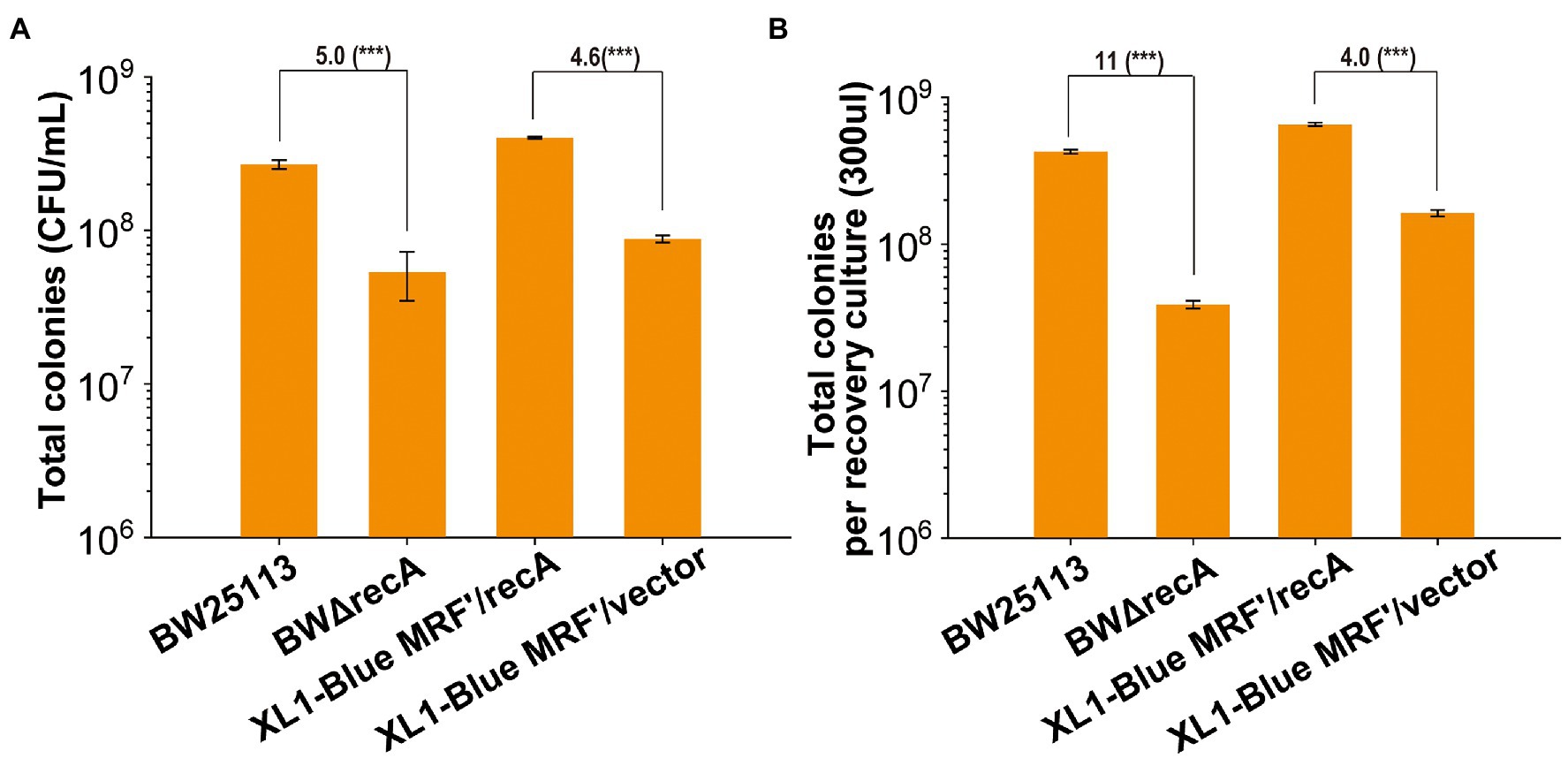
Figure 3. The changes of recovered total colonies for four strains before competent cells preparation and after transformation. (A) Total colonies of recA mutants and their parent wild-type strains formed on LB plates without antibiotics after they were cultured until their OD600nm reached at 0.5. (B) Total colonies of recA mutants and their parent wild-type strains formed on LB plates without antibiotics after recovering in LB medium for 60 min. Unpaired t-tests were performed (*** = p < 0.001). The numbers before (***) represent the difference by folds. Data are averages of three samples with standard deviations (error bars).
The Effects of Other Gene Deletions on TE and Growth of E. coli BW25113
The common cloning E. coli hosts also contain other inactivated genes, including endA, fhuA, deoR, and galE, to benefit cloning (van Die et al., 1984; Hanahan et al., 1991; Lin, 1992; Bonhivers et al., 1998; Altermark et al., 2007). Mutants with multiple deletions in E. coli BW25113, including BW3KD (ΔendA, ΔfhuA, and ΔdeoR), BW3KG (ΔendA, ΔfhuA, and ΔgalE) and BW4K (ΔendA, ΔfhuA, ΔgalE, and ΔdeoR), were generated. These gene deletions did not affect cell growth (Figure 4A). BW3KD had a similar TE to that of BW25113, and other two mutants with galE deletion had TE reduction by about 2 folds (Figure 4B). Thus, the inactivation of these genes is not the reason for the significantly increased TE in BW25113 over the commonly used E. coli hosts (Figure 1B).
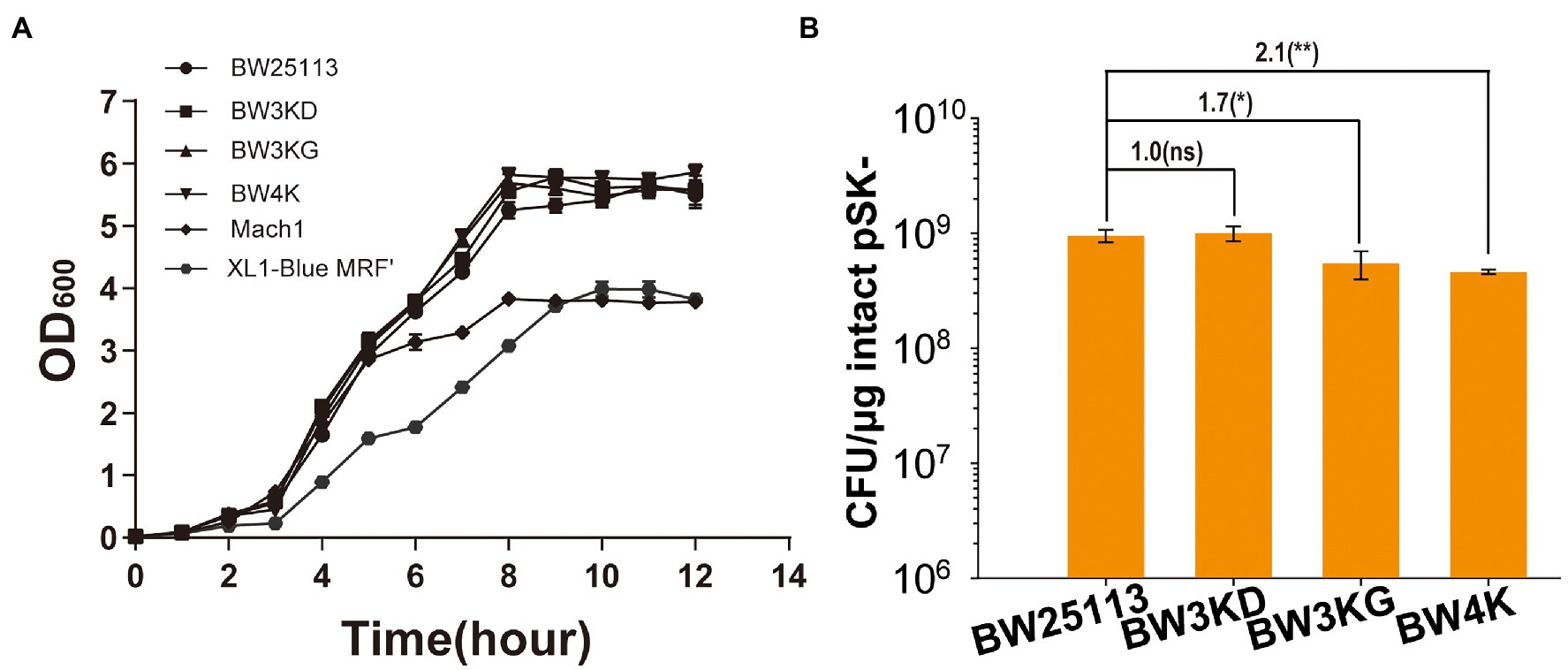
Figure 4. The effect of gene deletion of the growth and TE of E. coli BW25113. (A) The growth curves of BW25113, BW3KD, BW3KG, and BW4K. The latter two are commercially available cloning strains. (B) TEs of these strains. Unpaired t-tests were performed (*** = p < 0.001). The numbers before (***) represent the difference by folds. The “ns” shown in the figure means the p value is higher than 0.05. The numbers before (**) represent the difference by folds. Data are averages of three samples with standard deviations (error bars).
E. coli BW25113 Has a Significantly Higher Cloning Efficiency Than BWΔrecA and XL1-Blue MRF’
A 2.4-kb fragment carrying the gentamicin resistance gene (Gmr) in the middle of the kanamycin resistant gene (Kanr) with a 300-bp direct repeat and a 3-kb fragment from BW25113 chromosome were separately ensembled into four plasmids: pBluescript SK- (pSK-), pCL1920, pBR332, and pBBR1MCS-5 (pMCS5; Figures 5A,B). The assembled constructs were transformed into E. coli BW25113, BWΔrecA, and XL1-Blue MRF’. The cloning efficiency was defined as the total number of colonies (transformants) recovered on selective agar plates. The cloning efficiency with BW25113 was about 7- to 13-fold higher than those with BWΔrecA and 440- to 1,267-fold higher than those with XL1-Blue MRF’ (Figures 5A,B). When 20 or more colonies were checked by PCR, positive cloning rates were all higher than 90% (Supplementary Table S3). Thus, the cloning efficiencies are positively correlated with the TEs.
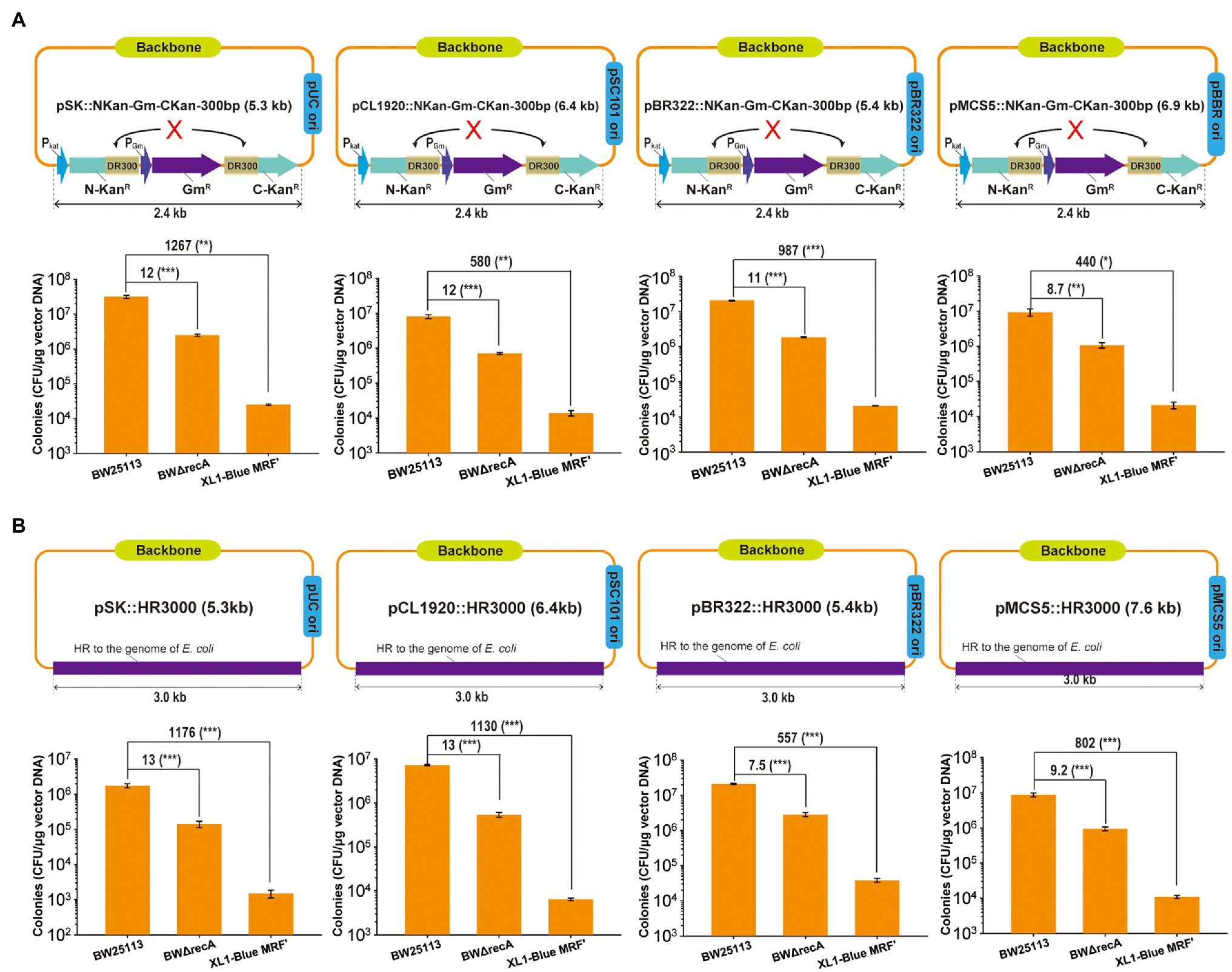
Figure 5. The cloning efficiency by using E. coli BW25113, BWΔrecA, and XL1-Blue MRF’ as competent cells. (A) Artificial DNA fragment containing 300-bp direct repeats was assembled into plasmids with different replicons. (B) A 3 kb-DNA fragment homologous to E. coli chromosome was cloned into plasmids with different replicons. The assembled DNA constructs by using TEDA were transformed into the competent cells of three E. coli strains prepared with TSS and plated with antibiotic selection. The total colonies (transformants) were counted. The correct constructs were tested by PCR and the positive rates were all higher than 90% (Supplementary Table S3). Data are averages of three samples with standard deviations (error bars). Unpaired t-tests were performed (* = p < 0.05; ** = p < 0.01; and *** = p < 0.001). Data are averages of three samples with standard deviations (error bars). The positive rates were reported in Supplementary Table S3.
The Effect of RecA on Insert Stability Is Not Observed
Intermolecular recombination between copies of the plasmid renders dimerization (Boe and Tolker-Nielsen, 1997). When pSK- was extracted from BW25113 and BWΔrecA and analyzed through gel electrophoresis, the two bright bands were the supercoiled and relaxed forms of pSK- isolated from BW25113 and BWΔrecA (Figure 6, lanes 3 and 4), and the faint bands on top were the dimer form of pSK− from BW25113 (Figure 6, lane 3). The results indicate that a small fraction of the high copy number plasmid pSK- is in the dimer form in BW25113 but not in BWΔrecA. Dimerization was not observed with the medium copy number plasmids pBR322 and pMCS5 and the low copy number plasmid pCL1920 in both BW25113 and BWΔrecA (Figure 6).
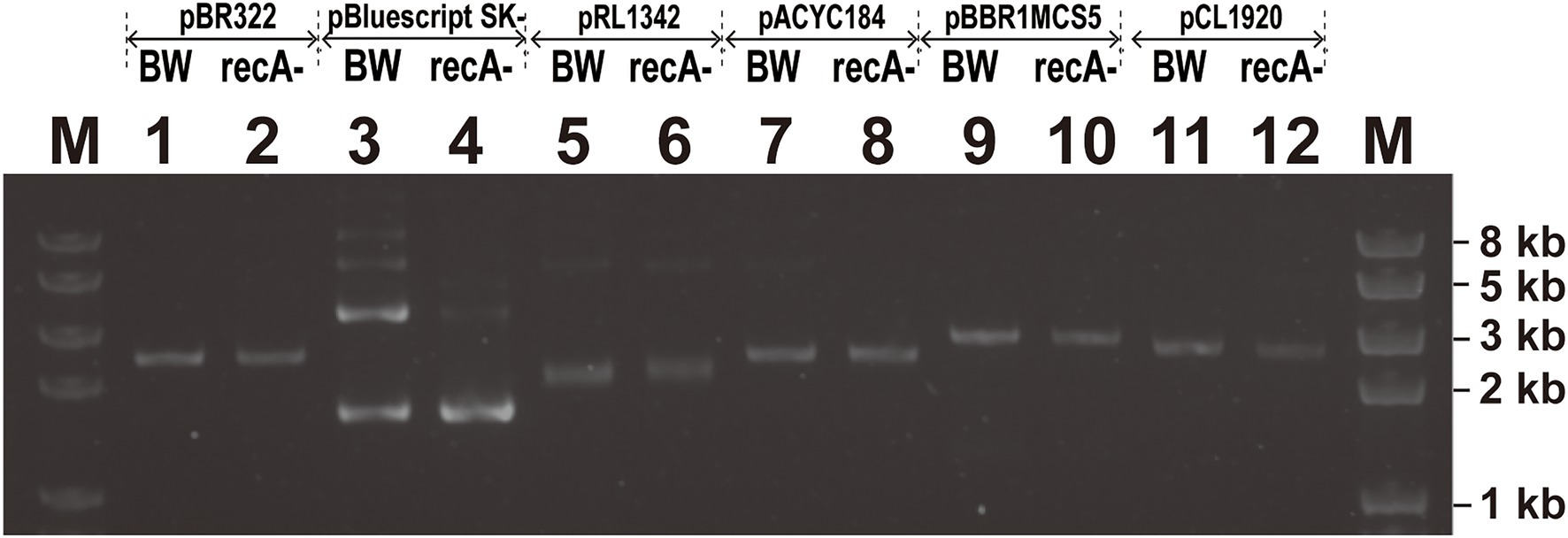
Figure 6. The screen of plasmids multimerization with different replicons in E. coli BW25113 and E. coli BWΔrecA. The top two lines on the gel showed the plasmids that were transformed into either BW25113 (BW) or BWΔrecA (recA−). Note: The two lower bands in Lanes 3 and 4 are the relaxed form and supercoiled form of pBluescipt SK- monomer; the faint bands on top of Lane 3 are the dimer forms.
To test whether recombination occurred between direct repeats on plasmids, the four plasmids carrying Gmr inserted in the middle of Kanr with the 300-bp direct repeats in BW25113 and BWΔrecA were analyzed (Figure 5A). When recombination occurred between the direct repeats, the antibiotic resistance would be altered from Kan−/Gm+ into Kan+/Gm− (Figure 5A). Three transformant colonies were randomly selected and cultured in LB without antibiotics. After 3 transfers (>60 generations), no Kan+ colonies were obtained, and the plasmids were the same via gel electrophoresis analysis (Figure 7A); the plasmid yields from 3 ml of overnight cultures were also similar (Supplementary Table S4).
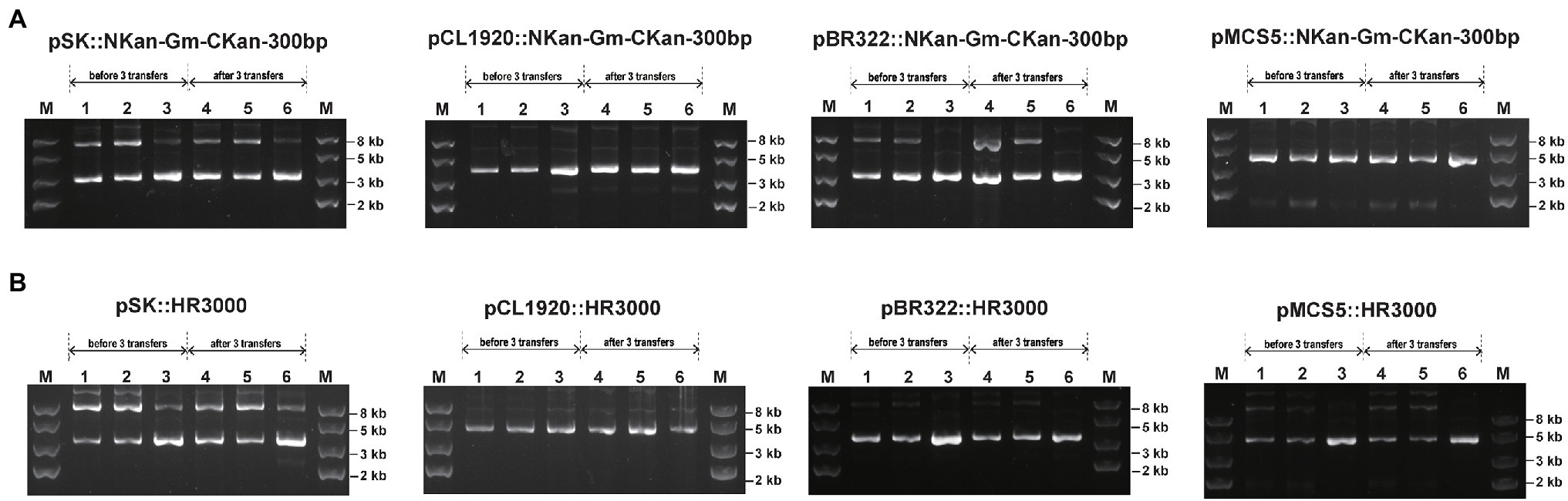
Figure 7. The stability of plasmids with homology features after extended culturing for 3 transfers. Three transformants were randomly selected from plates and cultured in LB medium for 3 transfers, representing about 60 generations. The plasmids were then extracted and checked via gel electrophoresis. (A) NKan-Gm-CKan-300 bp was assembled into four vectors. (B) HR300 was assembled into four vectors. Lanes 1–3 in each figure represent plasmids extracted from three cultures before 3 transfers, and lanes 4–6 in each figure represent plasmids extracted from three cultures after 3 transfers. Lane 1–2 and 4–5 represent plasmids extracted from strain BW25113, and Lane 3 and 6 represent plasmids extracted from strain XL1-Blue MRF’.
To check whether recombination occurred between the identical fragments on plasmids and the chromosome, a 3,000-bp DNA sequence from the genome of BW25113 was amplified and assembled into the four plasmids (Figure 5B). Three transformant colonies were randomly selected and cultured in LB with the proper antibiotics. After 3 transfers, the plasmids were extracted and analyzed by electrophoresis, no plasmid loss was observed (Figure 7B; Supplementary Table S4).
To check whether homologous recombination occurred among tandem repeats on plasmids, the repeats with unit length at 41-, 300-, and 1,000-bp were assayed. First, the 4, 6, and 8 tandem repeats of the 41-bp tac promoter, Ptac (TR41), with the eGFP gene were amplified and assembled into pCL1920, and the assembly of a single Ptac before eGFP was assembled into pCL1920 (pCL1920::1tac-eGFP) as the control (Figure 8A). The 300-bp tandem repeats and 1,000 bp tandem repeats were generated by using the plasmid pCL1920::Pkat-eGFP as the backbone (Figures 8B,C). The 2 and 3 tandem repeats at length of 300 bp and 1,000 bp were reassembled with pCL1920, and the assembly of single unit of the repeats was used as the control (Figures 8B,C). The presence of tandem repeats with all three lengths did not change the assembly efficiency for both BW25113 and BWΔrecA (Figures 8A–C). The positive rates for the assembly of all tandem repeats were higher than 90% for both strains, except the assembly of three units of TR1000. The positive rates were 80% in BWΔrecA and 50% in BW25113; however, 11-fold more transformant colonies were obtained with BW25113. All these constructed plasmids with highly tandem repeats including the one with 3 units of TR1000 repeats could be stably maintained in cells of BW25113 without apparent recombination or plasmid loss after three transfers in LB with antibiotic selection (Figure 8D; Supplementary Table S4). Collectively, these results suggest that these plasmids with homologous features were successfully maintained in the recA+ BW25113.
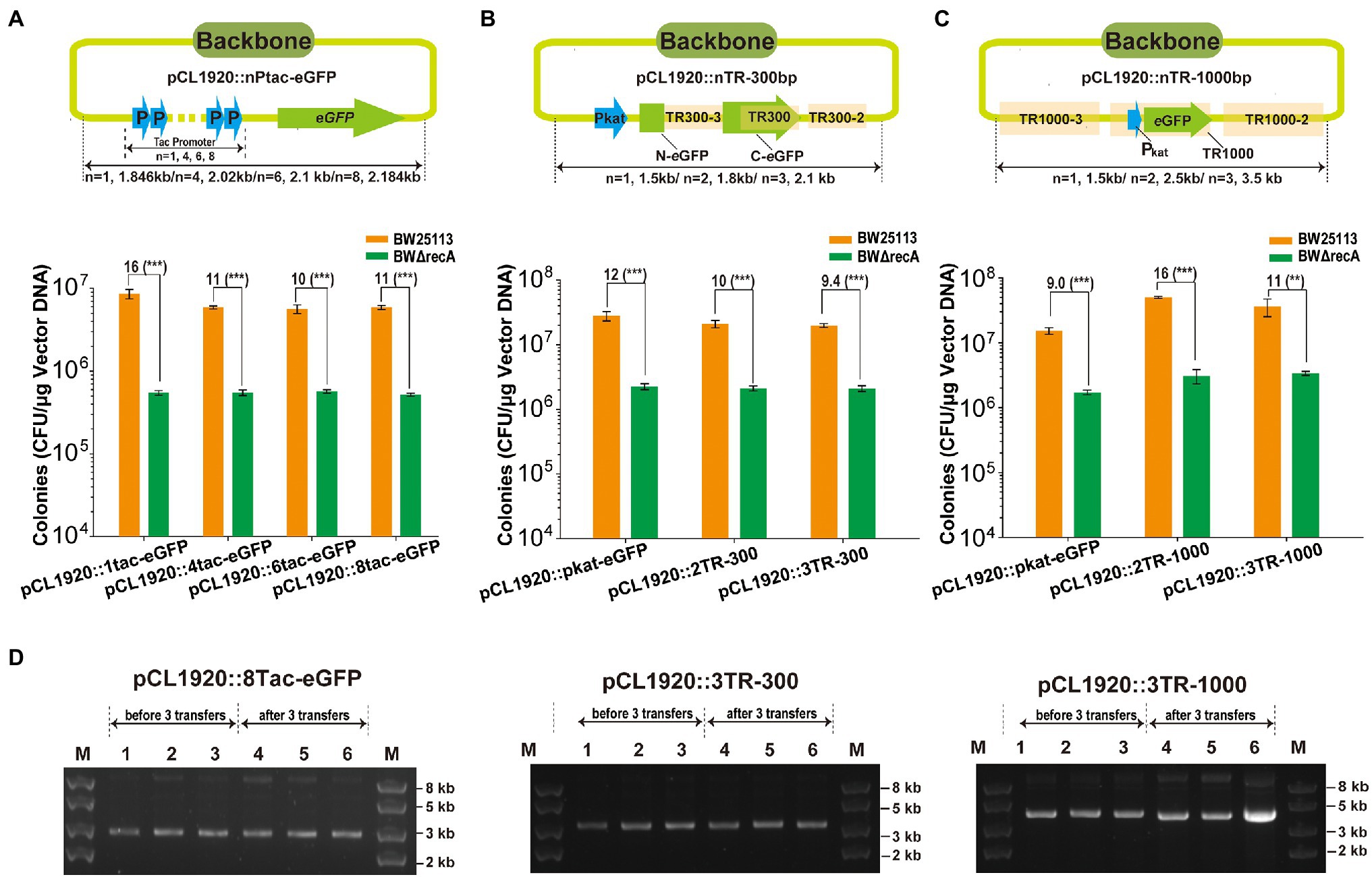
Figure 8. The cloning of plasmids with TRs at different lengths in E. coli BW25113. (A) DNA fragments containing an eGFP gene under the control of 1, 4, 6, and 8 units of the 41-bp Ptac was assembled into pCL1920. (B) DNA fragments containing 1, 2, or 3 units of the 300-bp TRs (TR300), derived from the C-terminal eGFP gene, were assembled into pCL1920. (C) DNA fragments containing 1, 2, or 3 units of the 1,000-bp TRs (TR1000), included the Pkat and eGFP, were assembled into pCL1920. The constructs were transformed into E. coli BW25113 and BW25113ΔrecA, and the total transformant colonies on LB plates with antibiotic selection were counted. Unpaired t-tests were performed (*** = p < 0.001). The numbers before (***) represent the difference by folds. Data are averages of three samples with standard deviations (error bars). (D) Gel electrophoresis of pCL1920::8Tac-eGFP, 3TR-300 bp, and 3TR-of 1,000 bp. Lanes 1–3 in each figure represent plasmids extracted from the three cultures before 3 transfers, and lanes 4–6 in each figure represent plasmids extracted from the three cultures after 3 transfers. Lane 1–2 and 4–5 represent plasmids extracted from BW25113, and Lane 3 and 6 represent plasmids extracted from the strain BWΔrecA.
Discussion
Our results support the use of the TSS method to prepare E. coli BW25113 and its recA deletion mutant BWΔrecA cells for cloning and DNA assembly (Xia et al., 2015, 2019). The choice of host strains is heavily relying on their availability. During our investigation on the complete pathway of the recombination of short-homologous ends in E. coli (Yang et al., 2021), we noticed E. coli BW25113 may be efficient in cloning and DNA assembly. The significantly higher TE and cloning efficiency of E. coli BW25113 over commonly used E. coli strains are beyond our expectations (Figures 1, 5). Even its recA deletion mutant BWΔrecA is significantly better, with its TE and cloning efficiency being 12- and >44-fold higher than those of E. coli XL1-Blue MRF’ (Figures 2, 5). The difference is enhanced by using the TSS method over the Hanahan and Inoue methods (Figure 1). However, the Hanahan and Inoue methods are usually better in preparing competent cells than the TSS methods for commonly used E. coli hosts, such as E. coli XL1-Blue MRF’ (Figure 1; Hanahan et al., 1991; Xia et al., 2019). Competent cells prepared with the Hanahan and Inoue methods may reach the TEs of 109 CFU/μg DNA with supercoiled plasmids (Inoue et al., 1990; Hengen, 1996; Xia et al., 2019), but both methods are normally kept the TEs at 108 CFU/μg DNA (Green and Sambrook, 2018, 2020). The TSS method is also commonly used because of its simplicity, in which the harvested cells are resuspended in TSS, stored at −80°C, and used for transformation, but its TS is around 107 CFU/μg DNA (Chung et al., 1989; Parrish et al., 2004; Xia et al., 2019). In our hands, the commonly used E. coli cloning strain XL1-Blue MRF’ had TEs around 107 CFU/μg DNA by using the three methods (Figure 1A), and E. coli BW25113 had TE higher than 109 CFU/μg DNA (Figure 1A). Although electroporation can reach up to 1010 CFU/μg DNA with supercoiled plasmids (Dower et al., 1988), it is not frequently used with the in vitro methods. The limitation is related to arching when the assembled DNA is directly used in large volumes (Sambrook et al., 2017). To prevent arching, either the assembled DNA is purified or a small volume is directly used in electroporation. The DNA loss during purification or small volumes may lead to low cloning efficiency (Tan and Yiap, 2009). Since both E. coli BW25113 and BWΔrecA are widely available (Baba et al., 2006), their chemically prepared competent cells by using the TSS method may be the better choices to be used with the in vitro methods for efficient cloning or DNA assembly.
E. coli BW25113 displays at least 100-fold higher TE than commonly used E. coli cloning strains (Figure 1B). RecA is a major factor contributing to the high TE and cloning efficiency in E. coli BW25113 (Figures 1–3, 5, 8), as its deletion reduced TE of BWΔrecA by 16 folds (Figure 2A), which is still 6-, 12-, 10-, 16-, 17-, and 23-fold higher than that of Omnimax, XL1-Blue MRF’, Stbl3, DH5α, Mach1, and Stbl2, respectively (Figures 1B, 2A). Therefore, RecA accounts for about half of the increased TE in E. coli BW25113. The recA− has been reported to reduce the mutant’s growth (Cox et al., 2008), but the decreases for both BW25113 and MG1655 were minor (Supplementary Figures S1A,B). The reduced TE in recA− strains is likely due to the reduced abilities of the recA− strains to form colonies on LB plates. The recA− strains had about 5-fold fewer colonies on LB plates than the recA+ strains when they grew to the same OD600nm (Figure 3A). The colonies of the recA− strains were further reduced to 17-fold and 13-fold fewer than that of the corresponding recA+ strains (BW25113 and MG1655) after competent cell preparation, storage, and transformation (Figure 2C). E. coli XL1-Blue MRF’ contains recA1 that has a point mutation changing Gly160 to Asp160 (Bullock, 1987). E. coli recA1 mutants are defective in recombination, as the RecA1 protein binds to single-stranded DNA but cannot carry out the ATP-dependent strand exchange reaction (Clark and Margulies, 1965; Muench and Bryant, 1990). E. coli XL1-Blue MRF’/recA only increased TE and the total colony counts by about 3–4 folds over XL1-Blue MRF’ (Figures 2B, 3B). The reduced increase is likely due to the interference of RecA1 in E. coli XL1-Blue MRF’/recA. Our results show a positive correlation between the TEs and the total live cells that are able to form colonies on LB plates after transformation. The reduced colony numbers of the recA− strains could be due to the presence of H2O2 in agar plates from autoclaving (Tanaka et al., 2014), which damages DNA (Hong et al., 2019). RecA is the core component of bacterial DNA homologous recombination (Kowalczykowski, 2000; Morimatsu and Kowalczykowski, 2014), participating in DNA damage repair (Li and Heyer, 2008). Hence, RecA enhances cell survival in the presence of H2O2. The mechanism warrants further investigation.
Other intentionally inactivated genes, such as galE, may contribute to the reduced TE but have little effect (Figure 4B). E. coli BW25113 may represent a good host for cloning for its intrinsic high TE when prepared with the TSS method (Figures 1, 2). E. coli BW25113 had 5-fold higher TE than MGΔhsdR, which is the hsdR deletion strain of E. coli MG1655 (Figure 2A). Only few differences exist on their genomes (Grenier et al., 2014). MGΔhsdR contains mutations in some phosphotransferase systems (Grenier et al., 2014), and BW25113 contains a mutation in the YjjP membrane protein (Grenier et al., 2014; Price et al., 2016), which is related to the transport of succinate (Fukui et al., 2017). There are also some differences in mobile elements between MGΔhsdR and BW25113, which may affect the stability of DNA (Darmon and Leach, 2014). These mutations do not affect the growth of the two strains in LB (Supplementary Figures S1A,B). It is unclear which difference determines their TEs. Further study is needed to reveal the mutations that affect the TEs of these strains.
Although it is believed that the RecA-dependent recombination affects plasmid stability, clear evidence is only related to the dimer formation with high copy number plasmids, which leads to plasmid loss without selection (Herman-Antosiewicz and Wegrzyn, 1999; Summers et al., 2010; Standley et al., 2019). We also observed dimers with plasmids harbor pUC18 ori, but the plasmids were stably maintained with selection (Figures 6, 7; Supplementary Table S4). When the monomer and dimer mixture isolated from BW25113 was transformed into BWΔrecA or XL1-Blue MRF’, only monomers were recovered, possibly due to RecF that is known to resolve dimers back to monomers (Summers and Sherratt, 1984). Further, recA+ significantly increased the cloning efficiency (Figures 5, 8) but did not affect the stability of inserts with tandem repeats of various size and a 3-kb host DNA (Figures 5, 7, 8). Considering the rate of RecA-dependent homologous recombination in E. coli is very low, occurring at approximately 10−5 per cell generation (Lovett et al., 2002), the effect of RecA on cloned inserts may be tolerated. Indeed, the recA+ E. coli BL21(DE3) is routinely used in protein overexpression (Chan et al., 2013), and recA+ K-12 derivatives are widely used in metabolic engineering (Cui et al., 2018; Badri et al., 2021). Hence, the effect of RecA on insert stability will not affect the cloned inserts in most cases, especially when homologous regions are small or not present (Lovett et al., 2002).
In summary, E. coli BW25113, a recA+ strain, is >100-fold more efficient for transformation than the commonly used E. coli recA− cloning strains when the competent cells are prepared with the TSS method (Figure 1B). The difference in cloning efficiency between BW25113 and XL1-Blue MRF’ is more dramatic, higher than 440 folds (Figure 5). RecA plays a major role in the increased TE and cloning efficiency (Figures 2, 5). The deletion of recA significantly reduces the fitness of the recA− strains, as they form less colonies than their corresponding recA+ strains on LB plates at the same OD600nm. Since we did not observe apparent insert instability with several homologous features in different vectors (Figures 5, 7, 8), E. coli BW25113 and other recA+ strains may be reconsidered for use in cloning for significantly increased success rates, especially when the inserts have none or minimal homologous features. When recA becomes a real concern, the recA mutant E. coli BWΔrecA can be used, as it is still significantly more efficient in cloning and DNA assembly than the commonly used cloning strains. BW25113 and its single-gene deletion mutants are readily available (Baba et al., 2006). They may be used to increase the cloning success rates in DNA assembly.
Data Availability Statement
The original contributions presented in the study are included in the article/Supplementary Material, further inquiries can be directed to the corresponding authors.
Author Contributions
YY acquired and analyzed the data. QY contributed to the preparation of competent cells. YY and MW contributed to the gene deletion and plasmid construction. YY and RZ contributed to the test of plasmid instability in RecA+ cells. YX, LX, and HL conceptualized this project and supervised the overall experiments. YY, YX, and HL prepared the manuscript. All authors contributed to the article and approved the submitted version.
Funding
We appreciated supports by grants from the Natural Science Foundation of China (31870085, 91951202, and 31961133015), the funding by the State Key Laboratory of Microbial Technology, and Qilu Youth Scholar Startup Funding of SDU (to YX).
Conflict of Interest
The authors declare that the research was conducted in the absence of any commercial or financial relationships that could be construed as a potential conflict of interest.
Publisher’s Note
All claims expressed in this article are solely those of the authors and do not necessarily represent those of their affiliated organizations, or those of the publisher, the editors and the reviewers. Any product that may be evaluated in this article, or claim that may be made by its manufacturer, is not guaranteed or endorsed by the publisher.
Supplementary Material
The Supplementary Material for this article can be found online at: https://www.frontiersin.org/articles/10.3389/fmicb.2022.838698/full#supplementary-material
References
Al-Allaf, F. A., Tolmachov, O. E., Zambetti, L. P., Tchetchelnitski, V., and Mehmet, H. (2013). Remarkable stability of an instability-prone lentiviral vector plasmid in Escherichia coli Stbl3. 3. Biotech 3, 61–70. doi: 10.1007/s13205-012-0070-8
Altermark, B., Niiranen, L., Willassen, N. P., Smalas, A. O., and Moe, E. (2007). Comparative studies of endonuclease I from cold-adapted Vibrio salmonicida and Mesophilic vibrio cholerae. FEBS J. 274, 252–263. doi: 10.1111/j.1742-4658.2006.05580.x
Anton, B. P., and Raleigh, E. A. (2016). Complete genome sequence of NEB 5-alpha, a derivative of Escherichia coli K-12 DH5 alpha. Microbiol. Resour. Announc. 4, e01245–e01216. doi: 10.1128/genomeA.01245-16
Baba, T., Ara, T., Hasegawa, M., Takai, Y., Okumura, Y., Baba, M., et al. (2006). Construction of Escherichia coli K-12 in-frame, single-gene knockout mutants: the Keio collection. Mol. Syst. Biol. 2, 2006.0008. doi: 10.1038/msb4100050
Badri, A., Williams, A., Awofiranye, A., Datta, P., Xia, K., He, W. Q., et al. (2021). Complete biosynthesis of a sulfated chondroitin in Escherichia coli. Nat. Commun. 12, 1389. doi: 10.1038/s41467-021-21692-5
Boe, L., and Tolker-Nielsen, T. (1997). Plasmid stability: comments on the dimer catastrophe hypothesis. Mol. Microbiol. 23, 247–253. doi: 10.1046/j.1365-2958.1997.2151579.x
Bonhivers, M., Plançon, L., Ghazi, A., Boulanger, P., le Maire, M., Lambert, O., et al. (1998). FhuA, an Escherichia coli outer membrane protein with a dual function of transporter and channel which mediates the transport of phage DNA. Biochimie 80, 363–369. doi: 10.1016/s0300-9084(00)80004-8
Bullock, W. (1987). XL1-blue: a high efficiency plasmid transforming recA Escherichia coli strain with beta-galactosidase selection. BioTecchniques 5, 376–379.
Carmel, G., and Coulton, J. W. (1991). Internal deletions in the FhuA receptor of Escherichia coli K-12 define domains of ligand interactions. J. Bacteriol. 173, 4394–4403. doi: 10.1128/jb.173.14.4394-4403.1991
Chan, W. T., Verma, C. S., Lane, D. P., and Gan, S. K. (2013). A comparison and optimization of methods and factors affecting the transformation of Escherichia coli. Biosci. Rep. 33:e00086. doi: 10.1042/BSR20130098
Chao, R., Yuan, Y. B., and Zhao, H. M. (2015). Recent advances in DNA assembly technologies. FEMS Yeast Res. 15, 1–9. doi: 10.1111/1567-1364.12171
Chung, C. T., Niemela, S. L., and Miller, R. H. (1989). One-step preparation of competent Escherichia-coli - transformation and storage of bacterial-cells in the same solution. Proc. Natl. Acad. Sci. U. S. A. 86, 2172–2175. doi: 10.1073/pnas.86.7.2172
Clark, A. J. (1973). Recombination deficient mutants of E. coli and other bacteria. Annu. Rev. Genet. 7, 67–86. doi: 10.1146/annurev.ge.07.120173.000435
Clark, A. J., and Margulies, A. D. (1965). Isolation and characterization of recombination-deficient mutants of Escherichia coli K12. Proc. Natl. Acad. Sci. U. S. A. 53, 451–459. doi: 10.1073/pnas.53.2.451
Cohen, S. N., Chang, A. C. Y., Boyer, H. W., and Helling, R. B. (1973). Construction of biologically functional bacterial plasmids in vitro. Proc. Natl. Acad. Sci. U. S. A. 70, 3240–3244. doi: 10.1073/pnas.70.11.3240
Cox, J. M., Li, H., Wood, E. A., Chitteni-Pattu, S., Inman, R. B., and Cox, M. M. (2008). Defective dissociation of a "slow" recA mutant proteinimparts an Escherichia coli growth defect. J. Biol. Chem. 283, 24909–24921. doi: 10.1074/jbc.M803934200
Cui, L., Vigouroux, A., Rousset, F., Varet, H., Khanna, V., and Bikard, D. (2018). A CRISPRi screen in E. coli reveals sequence-specific toxicity of dCas9. Nat. Commun. 9, 1912. doi: 10.1038/s41467-018-04209-5
Darmon, E., and Leach, D. R. (2014). Bacterial genome instability. Microbiol. Mol. Biol. Rev. 78, 1–39. doi: 10.1128/MMBR.00035-13
Datsenko, K. A., and Wanner, B. L. (2000). One-step inactivation of chromosomal genes in Escherichia coli K-12 using PCR products. Proc. Natl. Acad. Sci. U. S. A. 97, 6640–6645. doi: 10.1073/pnas.120163297
Dower, W. J., Miller, J. F., and Ragsdale, C. W. (1988). High efficiency transformation of E. coli by high voltage electroporation. Nucleic Acids Res. 16, 6127–6145. doi: 10.1093/nar/16.13.6127
Ellis, T., Adie, T., and Baldwin, G. S. (2011). DNA assembly for synthetic biology: from parts to pathways and beyond. Integr. Biol. 3, 109–118. doi: 10.1039/c0ib00070a
Fu, C. L., Wehr, D. R., Edwards, J., and Hauge, B. (2008). Rapid one-step recombinational cloning. Nucleic Acids Res. 36:e54. doi: 10.1093/nar/gkn167
Fukui, K., Nanatani, K., Hara, Y., Yamakami, S., Yahagi, D., Chinen, A., et al. (2017). Escherichia coli yjjPB genes encode a succinate transporter important for succinate production. Biosci. Biotechnol. Biochem. 81, 1837–1844. doi: 10.1080/09168451.2017.1345612
Green, M. R., and Sambrook, J. (2018). The hanahan method for preparation and transformation of competent Escherichia coli: high-efficiency transformation. Cold Spring Harb Protoc 2018:pdb.prot101188. doi: 10.1101/pdb.prot101188
Green, M. R., and Sambrook, J. (2020). The Inoue method for preparation and transformation of competent Escherichia coli: "Ultracompetent" cells. Cold Spring Harb Protoc 2020:101196. doi: 10.1101/pdb.prot101196
Grenier, F., Matteau, D., Baby, V., and Rodrigue, S. (2014). Complete genome sequence of Escherichia coli BW25113. Genome Announc. 2, 1038–1401038. doi: 10.1128/genomeA.01038-14
Hanahan, D. (1983). Studies on transformation of Escherichia coli with plasmids. J. Mol. Biol. 166, 557–580. doi: 10.1016/s0022-2836(83)80284-8
Hanahan, D., Jessee, J., and Bloom, F. R. (1991). Plasmid transformation of Escherichia-coli and other bacteria. Methods Enzymol. 204, 63–113. doi: 10.1016/0076-6879(91)04006-A
Hantke, K., and Braun, V. (1975). Membrane receptor dependent iron transport in Escherichia coli. FEBS Lett. 49, 301–305. doi: 10.1016/0014-5793(75)80771-x
Hantke, K., and Braun, V. (1978). Functional interaction of the tonA/tonB receptor system in Escherichia coli. J. Bacteriol. 135, 190–197. doi: 10.1128/JB.135.1.190-197.1978
Hengen, P. N. (1996). Methods and reagents - preparing ultra-competent Escherichia coli. Trends Biochem. Sci. 21, 75–76. doi: 10.1016/S0968-0004(96)80187-3
Herman-Antosiewicz, A., and Wegrzyn, G. (1999). Regulation of copy number and stability of phage lambda derived pTC lambda 1 plasmid in the light of the dimer/multimer catastrophe hypothesis. FEMS Microbiol. Lett. 176, 489–493. doi: 10.1016/S0378-1097(99)00278-5
Hong, Y. Z., Zeng, J., Wang, X. H., Drlica, K., and Zhao, X. L. (2019). Post-stress bacterial cell death mediated by reactive oxygen species. Proc. Natl. Acad. Sci. U. S. A. 116, 10064–10071. doi: 10.1073/pnas.1901730116
House, B. L., Mortimer, M. W., and Kahn, M. L. (2004). New recombination methods for Sinorhizobium meliloti genetics. Appl. Environ. Microbiol. 70, 2806–2815. doi: 10.1128/Aem.70.5.2806-2815.2004
Inoue, H., Nojima, H., and Okayama, H. (1990). High-efficiency transformation of Escherichia-coli with plasmids. Gene 96, 23–28. doi: 10.1016/0378-1119(90)90336-P
Jeong, J. Y., Yim, H. S., Ryu, J. Y., Lee, H. S., Lee, J. H., Seen, D. S., et al. (2012). One-step sequence- and ligation-independent cloning as a rapid and versatile cloning method for functional genomics studies. Appl. Environ. Microbiol. 78, 5440–5443. doi: 10.1128/Aem.00844-12
Kosuri, S., and Church, G. M. (2014). Large-scale de novo DNA synthesis: technologies and applications. Nat. Methods 11, 499–507. doi: 10.1038/Nmeth.2918
Kowalczykowski, S. C. (2000). Initiation of genetic recombination and recombination-dependent replication. Trends Biochem. Sci. 25, 156–165. doi: 10.1016/s0968-0004(00)01569-3
Li, X., and Heyer, W. D. (2008). Homologous recombination in DNA repair and DNA damage tolerance. Cell Res. 18, 99–113. doi: 10.1038/cr.2008.1
Li, M. J., Wang, J. S., Geng, Y. P., Li, Y. K., Wang, Q., Liang, Q. F., et al. (2012). A strategy of gene overexpression based on tandem repetitive promoters in Escherichia coli. Microb. Cell Factories 11, 19. doi: 10.1186/1475-2859-11-19
Lin, J. J. (1992). Endonuclease A degrades chromosomal and plasmid DNA of Escherichia coli present in most preparations of single stranded DNA from phagemids. Proc. Natl. Sci. Counc. Repub. China B Life Sci. 16, 1–5.
Lovett, S. T., Hurley, R. L., Sutera, V. A., Aubuchon, R. H., and Lebedeva, M. A. (2002). Crossing over between regions of limited homology in Escherichia coli: RecA-dependent and RecA-independent pathways. Genetics 160, 851–859. doi: 10.1093/genetics/160.3.851
Maraboeuf, F., Voloshin, O., CameriniOtero, R. D., and Takahashi, M. (1995). The central aromatic residue in loop L2 of RecA interacts with DNA. Quenching of the fluorescence of a tryptophan reporter inserted in L2 upon binding to DNA. J. Biol. Chem. 270, 30927–30932. doi: 10.1074/jbc.270.52.30927
Metcalf, W. W., and Wanner, B. L. (1993). Construction of new beta-glucuronidase cassettes for making transcriptional fusions and their use with new methods for allele replacement. Gene 129, 17–25. doi: 10.1016/0378-1119(93)90691-u
Morimatsu, K., and Kowalczykowski, S. C. (2014). RecQ helicase and RecJ nuclease provide complementary functions to resect DNA for homologous recombination. Proc. Natl. Acad. Sci. U. S. A. 111, E5133–E5142. doi: 10.1073/pnas.1420009111
Muench, K. A., and Bryant, F. R. (1990). An obligatory pH-mediated isomerization on the [Asn-160]recA protein-promoted DNA strand exchange reaction pathway. J. Biol. Chem. 265, 11560–11566. doi: 10.1016/S0021-9258(19)38434-0
Parrish, J. R., Limjindaporn, T., Hines, J. A., Liu, J. Y., Liu, G. Z., and Finley, R. L. (2004). High-throughput cloning of Campylobacter jejuni ORFs by in vivo recombination in Escherichia coli. J. Proteome Res. 3, 582–586. doi: 10.1021/pr0341134
Price, M. N., Wetmore, K. M., Deutschbauer, A. M., and Arkin, A. P. (2016). A comparison of the costs and benefits of bacterial gene expression. PLoS One 11:e00086, e0164314. doi: 10.1371/journal.pone.0164314
Sain, B., and Murray, N. E. (1980). The hsd (host specificity) genes of E. coli K12. Mol. Gen. Genet. 180, 35–46. doi: 10.1007/BF00267350
Sambrook, J., Fritsch, E. F., and Maniatis, T. (2017). Molecular Cloning: A Laboratory Manual. New York, USA: Cold Spring Harbor Laboratory Press.
Sanjana, N. E., Shalem, O., and Zhang, F. (2014). Improved vectors and genome-wide libraries for CRISPR screening. Nat. Methods 11, 783–784. doi: 10.1038/nmeth.3047
Smolke, C. D. (2009). Building outside of the box: iGEM and the BioBricks foundation. Nat. Biotechnol. 27, 1099–1102. doi: 10.1038/nbt1209-1099
Standley, M. S., Million-Weaver, S., Alexander, D. L., Shuai, H., and Camps, M. J. O. (2019). Genetic control of ColE1 plasmid stability that is independent of plasmid copy number regulation. Curr. Genet. 65, 179–192. doi: 10.1007/s00294-018-0858-0
Summers, D. K., Beton, C. W. H., and Withers, H. L. (2010). Multicopy plasmid instability: the dimer catastrophe hypothesis. Mol. Microbiol. 8, 1031–1038. doi: 10.1111/j.1365-2958.1993.tb01648.x
Summers, D. K., and Sherratt, D. J. (1984). Multimerization of high copy number plasmids causes instability: ColE 1 encodes a determinant essential for plasmid monomerization and stability. Cell 36, 1097–1103. doi: 10.1016/0092-8674(84)90060-6
Tan, S. C., and Yiap, B. C. (2009). DNA, RNA, and protein extraction: the past and the present. J. Biomed. Biotechnol. 2009:574398. doi: 10.1155/2009/574398
Tanaka, T., Kawasaki, K., Daimon, S., Kitagawa, W., Yamamoto, K., Tamaki, H., et al. (2014). A hidden pitfall in the preparation of agar media undermines microorganism cultivability. Appl. Environ. Microbiol. 80, 7659–7666. doi: 10.1128/Aem.02741-14
Trinh, T., Jessee, J., Bloom, F., and Hirsch, V. (1994). STBL2: an Escherichia coli strain for the stable propagation of retroviral clones and direct repeat sequences. Focus 16, 78–80.
van Die, I. M., Zuidweg, E. M., Bergmans, J. E., and Hoekstra, W. P. (1984). Transformability of galE variants derived from uropathogenic Escherichia coli strains. J. Bacteriol. 158, 760–761. doi: 10.1128/JB.158.2.760-761.1984
Wang, H. L., Li, Z., Jia, R. N., Hou, Y., Yin, J., Bian, X. Y., et al. (2016). RecET direct cloning and red alpha beta recombineering of biosynthetic gene clusters, large operons or single genes for heterologous expression. Nat. Protoc. 11, 1175–1190. doi: 10.1038/nprot.2016.054
Xia, Y., Chu, W., Qi, Q., and Xun, L. (2015). New insights into the QuikChange process guide the use of Phusion DNA polymerase for site-directed mutagenesis. Nucleic Acids Res. 43:e12. doi: 10.1093/nar/gku1189
Xia, Y., Li, K., Li, J., Wang, T., Gu, L., and Xun, L. (2019). T5 exonuclease-dependent assembly offers a low-cost method for efficient cloning and site-directed mutagenesis. Nucleic Acids Res. 47:e15. doi: 10.1093/nar/gky1169
Yang, Y. Q., Wang, T. Q., Yu, Q. L., Liu, H. W., Xun, L. Y., and Xia, Y. Z. (2021). The pathway of recombining short homologous ends in Escherichia coli revealed by the genetic study. Mol. Microbiol. 115, 1309–1322. doi: 10.1111/mmi.14677
Yanischperron, C., Vieira, J., and Messing, J. (1985). Improved M13 phage cloning vectors and host strains: nucleotide sequences of the M13mp18 and pUC19 vectors. Gene 33, 103–119. doi: 10.1016/0378-1119(85)90120-9
Zhang, Y. W., Werling, U., and Edelmann, W. (2012). SLiCE: a novel bacterial cell extract-based DNA cloning method. Nucleic Acids Res. 40:e55. doi: 10.1093/nar/gkr1288
Keywords: transformation, competent cells, Escherichia coli, recA, cloning, recombination
Citation: Yang Y, Yu Q, Wang M, Zhao R, Liu H, Xun L and Xia Y (2022) Escherichia coli BW25113 Competent Cells Prepared Using a Simple Chemical Method Have Unmatched Transformation and Cloning Efficiencies. Front. Microbiol. 13:838698. doi: 10.3389/fmicb.2022.838698
Edited by:
Ning-Yi Zhou, Shanghai Jiao Tong University, ChinaReviewed by:
Qing Hong, Nanjing Agricultural University, ChinaXinqing Zhao, Shanghai Jiao Tong University, China
Copyright © 2022 Yang, Yu, Wang, Zhao, Liu, Xun and Xia. This is an open-access article distributed under the terms of the Creative Commons Attribution License (CC BY). The use, distribution or reproduction in other forums is permitted, provided the original author(s) and the copyright owner(s) are credited and that the original publication in this journal is cited, in accordance with accepted academic practice. No use, distribution or reproduction is permitted which does not comply with these terms.
*Correspondence: Luying Xun, bHV5aW5nX3h1bkB2ZXRtZWQuZWR1LmVkdQ== Yongzhen Xia, eGlheW9uZ3poZW4yMDAyQHNkdS5lZHUuY24=