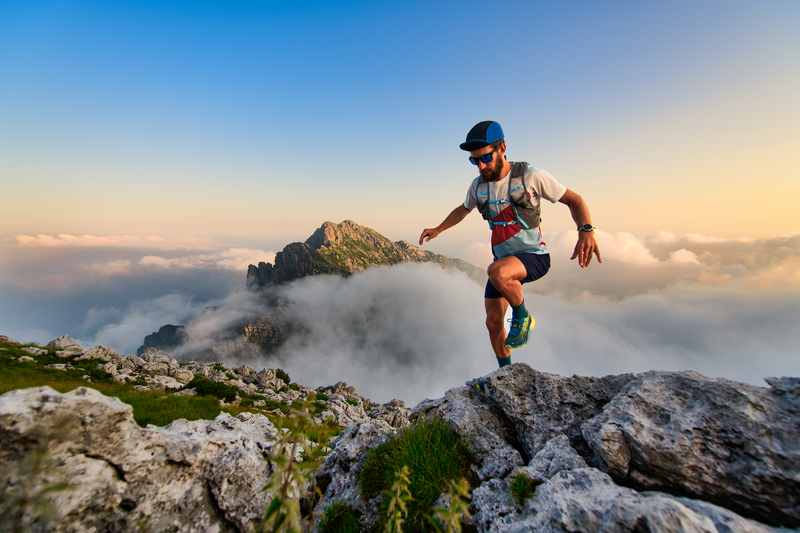
95% of researchers rate our articles as excellent or good
Learn more about the work of our research integrity team to safeguard the quality of each article we publish.
Find out more
REVIEW article
Front. Microbiol. , 07 March 2022
Sec. Microbe and Virus Interactions with Plants
Volume 13 - 2022 | https://doi.org/10.3389/fmicb.2022.833566
This article is part of the Research Topic Insights in Microbe and Virus Interactions with Plants: 2021 View all 14 articles
Microbial symbionts can mediate plant stress responses by enhancing thermal tolerance, but less attention has been paid to measuring these effects across plant-microbe studies. We performed a meta-analysis of published studies as well as discussed with relevant literature to determine how the symbionts influence plant responses under non-stressed versus thermal-stressed conditions. As compared to non-inoculated plants, inoculated plants had significantly higher biomass and photosynthesis under heat stress conditions. A significantly decreased accumulation of malondialdehyde (MDA) and hydrogen peroxide (H2O2) indicated a lower oxidation level in the colonized plants, which was also correlated with the higher activity of catalase, peroxidase, glutathione reductase enzymes due to microbial colonization under heat stress. However, the activity of superoxide dismutase, ascorbate oxidase, ascorbate peroxidase, and proline were variable. Our meta-analysis revealed that microbial colonization influenced plant growth and physiology, but their effects were more noticeable when their host plants were exposed to high-temperature stress than when they grew under ambient temperature conditions. We discussed the mechanisms of microbial conferred plant thermotolerance, including at the molecular level based on the available literature. Further, we highlighted and proposed future directions toward exploring the effects of symbionts on the heat tolerances of plants for their implications in sustainable agricultural production.
Due to climate change, the constant rise of ambient temperature is one of the major global issues and has devastating impacts on crop growth and productivity, consequently, in food security. Results from various climate model simulations predict that the global average temperature could be between 1.1 to 5.4°C warmer in 2100 than it is today (IPCC, 2019). Heat stress causes a manifold adverse impact on the growth, development, physiological processes of plants (Wahid et al., 2007; Hasanuzzaman et al., 2013; Hassan et al., 2021; Jagadish et al., 2021). The plant employs varying levels of adaptation, avoidance, acclimation, and tolerance mechanisms to cope with heat stress via physical, physiological, biochemical, and molecular strategies, including the use of ion transporters, proteins, osmolytes, antioxidants, and other factors involved in signaling and transcriptional regulation (Hasanuzzaman et al., 2013; Hassan et al., 2021; Jagadish et al., 2021; Zhao et al., 2021). Plant responses to abiotic stress have been studied widely for the last few years, and the role of microbes on plant stress responses has also been given attention in recent years. In particular, plant species commonly associated with microbial symbionts, known as plant microbiome, may influence responses of the host plant to environmental stimuli, including heat stress.
Microorganisms are ubiquitous, and all plants are colonized by various types of microbes which play important roles in plant ecology and physiology (Rodriguez et al., 2009; Turner et al., 2013; Compant et al., 2019; Dastogeer et al., 2020a). For example, AMF (arbuscular mycorrhizal fungi) present in the roots of 80% of terrestrial plants (Brachmann and Parniske, 2006) and microbial endophytes (both fungal and bacterial) are highly diverse and live asymptomatically in every plant studied so far. It is increasingly recognized that these microbes, as well as others including, e.g., epiphytes, viruses, ectomycorrhiza, N-fixers, etc., exert multiple effects on the plants particularly under adverse environmental conditions (Porras-Alfaro and Bayman, 2011; Hill et al., 2019; Dastogeer et al., 2020b; Kumar et al., 2020). These beneficial effects, which are strongly dependent on environmental conditions (Rodriguez et al., 2009; Hoeksema et al., 2010; Dastogeer, 2018), include priming against pests or herbivores, the acquisition of growth-limiting nutrients from the soil, tolerance to drought, salinity and thermal stress, etc. (Rodriguez et al., 2009; Goh et al., 2013; Hardoim et al., 2015; Azad and Kaminskyj, 2016; González-Teuber, 2016; Molina-Montenegro et al., 2016; Dastogeer and Wylie, 2017; Rho et al., 2018; Hill et al., 2019). Microbes produce a wide range of compounds to impact the responses of plants at the molecular level, triggering the biosynthesis of pigments, secondary metabolites, hormones, antioxidants, and alkaloids (Meena et al., 2017; Dastogeer et al., 2018; Basit et al., 2021; Naamala and Smith, 2021). Just as for other stresses, it is becoming increasingly evident that the heat stress responses of plants may be influenced by their interactions with microbes (Eerens et al., 1998; Ali et al., 2011; Khan A. L. et al., 2015; Ismail et al., 2020a; Li X. et al., 2021).
Despite the ubiquity and high diversity of microbial symbionts in plant tissues and the widespread effects and economic relevance of heat as a stress factor for plants, when compared with other abiotic factors such as drought, salinity, or nutrient limitation, the involvement of symbionts in plant host responses to heat stress have been received less attention (Meena et al., 2017; Kumar and Verma, 2018). The handful of studies available indicate that the magnitudes of the effect of microbes on plant heat stress tolerance and associated mechanisms such as accumulation of osmolytes, antioxidants, phytohormones, etc., vary among various studies (Meena et al., 2017; Kumar and Verma, 2018). We assume these differences could be associated with multiple factors such as level of stress, types of host and symbiont partners, environmental conditions, and their complex interactions. In order to gain insight into and harness the benefit of symbiont in agricultural management practice, it is imperative to elucidate the detailed mechanism of microbe-plant interaction under heat stress conditions (Harman and Uphoff, 2019). It is indeed tenuous and often erroneous to deduce the findings in general context from the individual investigation (Dastogeer, 2018). Therefore, to ascertain the central tendency and pinpoint the patterns of microbial influence on plants under stress and compare with them under control, it is paramount to integrate results across studies in order to determine if the factors can be known (Borenstein et al., 2009; Dastogeer, 2018). Here, we performed a meta-analysis to measure the overall strength and direction of effect (beneficial, harmful or neutral) of symbiosis on important plant characteristics associated with stress tolerance mechanisms. To the best of our knowledge, this aspect has not previously been discussed in a review of the published article, and thus present article fills a substantial gap in our understanding of interactions between plants and their symbiotic microbial partners.
A meta-analysis is a mathematical and statistical approach that pools the results of various investigations to estimate a mean effect size for treatment across a range of studies (Rosenberg et al., 2000). We can evaluate the findings of a study with respect to all other comparable studies to assess if the effect of a treatment is consistent across studies or if it differs substantially among studies and which factor might be accounted for the differences (Borenstein et al., 2009). The categorical variables or “moderators” are often included in meta-analyses to pinpoint which and how various features modulate the treatment effect of interest. This form of analysis has been, for example, used to determine the impact of microbial symbionts on plant response to salinity stress (Chandrasekaran et al., 2014, 2016; Rho et al., 2018), drought stress (Dastogeer, 2018; Rho et al., 2018), and cold stress (Acuna-Rodriguez et al., 2020). In the current study, we accumulated data from all studies to date and measured the effects of endophytes on 24 plant response parameters that encompass plant growth, photosynthesis, metabolites, and enzymatic activities that are subjected to change under thermal stress conditions. Our purpose was to answer the questions, broadly; what is the overall impact of microbial colonization on various plant growth and photosynthetic parameters of plants exposed to heat stress? Does the plant-symbiont relationship differ under heat-stressed conditions compared to unstressed conditions? What is the role of symbiosis in osmotic balance and antioxidant enzymes regulation in plants exposed to thermal stress? We supplemented our meta-analysis with a review of relevant literature for a deeper insight into this subject.
The meta-data were collected by following the general guidelines of Field and Gillett (2010). We performed a literature search in Web of Science (Clarivate Analysis) and PubMed database through September 2020. Our search terms were: endophyte or AMF or mycorrhiza or bacteria or fungi AND either “heat stress*” or “hot stress*” or “high temperature.” The Boolean truncation (“*”) was used so as to include the variations of the word such as fungi, fungus, and fungal. The search produced 4,562 unduplicated papers, and 90 peer-reviewed articles were considered likely to contain relevant information by reading the title and abstract. To finally select the papers for data extraction, we had preset criteria such as so that these studies can be included in our meta-analysis and extreme heterogeneity minimized (Cooper, 2015; Ahn and Kang, 2018; Cooper et al., 2019; Seidler et al., 2019):
(i) The article must describe the findings of original research, and as such, review papers, opinion articles, editorials, book chapters, and systematic reviews were discarded for inclusion into our meta-analysis.
(ii) The investigation had to use at least one microbial strain regardless of inoculation protocol or rate,
(iii) The inoculum was used singly, and we did not consider any mixed-inoculation for this meta-analysis,
(iv) Both microbe-inoculated and non-inoculated plants were grown under normal stressed and non-stressed conditions, and data provided,
(v) Any of the growth and other parameters, e.g., biomass, enzymes, hormones, etc., were measured
(vi) The papers must have provided sample size (i.e., replication), means, standard deviations/errors, and other relevant statistical data that could be converted to measure an effect size.
Based on the above criteria, most articles were excluded for meta-analysis, and only 39 peer-reviewed original research articles were retained for the final analysis (Supplementary Table 1). We accepted studies to differ in the levels of fertilizer applied, growth conditions (greenhouse, growth chamber, or field), duration of stress application, the magnitude of stress, and growth media into our meta-analysis. Papers spanned 23 years (1998–2021) and were in English. We apologize for not including the potential paper that contains data written in other languages or those that we might have unintentionally missed during our search or due to stipulated selection criteria.
When a publication provided results of more than one study system/group, those systems were taken as independent data points (Hedges et al., 1999). From these articles, we extracted information on host identity (plant species, genus, family), symbionts’ identity (genus, species), plant biomass (shoot and root), length (shoot and root), relative water content, photosynthetic parameters, enzymes parameters, and other relevant data from the studies (Supplementary Table 2). The means, sample sizes (replications), standard deviation were recorded from each study. Standard deviation was calculated from standard errors (SE) using the equation: SE = SD (n–1/2). Frequently the results were presented in a graph, and we used WebPlotDigitizer (Rohatgi, 2021) to digitize the values. If any paper reported multiple treatments or host/AMF combinations, these were included as an independent data unit in the analysis. However, it might increase the dependence of a particular study when considered them as an independent report (Gurevitch and Hedges, 1999). Nonetheless, this practice is assumed to increase the statistical power of meta-analysis (Lajeunesse and Forbes, 2003) and several biological meta-analyses papers also used the same (Holmgren et al., 2012; Veresoglou et al., 2012; Mayerhofer et al., 2013; McGrath and Lobell, 2013; Dastogeer, 2018).
Meta-analyses were conducted using the “metacont” function of the package “meta” (Balduzzi et al., 2019) implemented in R version 4.0.4 (R Core Team, 2021). Although the DerSimonian and Laird (DL) method is commonly used by default to estimate the between-study variance, it was suggested that for continuous data, the restricted maximum likelihood estimator (REML) are better alternatives to estimate the between-study variance (DerSimonian and Laird, 1986; Veroniki et al., 2016; Cooper et al., 2019). We used the Restricted maximum-likelihood estimator (REML) to make statistical inferences considering the effects as random. Standardized mean difference (SMD), which is defined as the ratio of the difference in mean performance between treatments to the pooled standard deviation, was calculated. This effect size measurement is done in meta-analyses that involve studies reporting continuous outcomes, similar to our study (Faraone, 2008). Generally, the SMD values of 0.3, 0.5, and 0.8 are considered as a small, medium, and large effect sizes, respectively (Cohen, 1988). Quantification of heterogeneity as well as testing the significance were estimated with Higgin’s I2 and Cochran’s Q statistics. “I2” is defined as the ratio of true heterogeneity to total heterogeneity across the effect sizes, whereas “Q” is the weighted deviations of the summary effect size that is due to true heterogeneity rather than sampling error (Higgins and Thompson, 2002; Higgins et al., 2003; Huedo-Medina et al., 2006). The I2 values < 25%, 25–75%, and > 75% are regarded as representing low, moderate, and high heterogeneity (Higgins et al., 2003) by convention. The coefficient of interval estimate of effect sizes was set to 95%, and the effect size (SMD) was considered significant when 95% CIs (confidence interval) did not cross zero. SMD of zero suggests that the two treatments (microbial inoculated or non-inoculated) have equivalent effects; SMDs greater than zero indicate the degree to which the microbial inoculated outperforms the non-inoculated samples and vice-versa.
Publication bias for each dataset of different parameters was tested by inspecting funnel plots and Egger regression test for funnel plot asymmetry (Begg and Mazumdar, 1994; Egger et al., 1997; Simonsohn et al., 2014). We found that for most of the parameters, there were no substantial publication biases in these datasets (Supplementary Table 2). For some parameters which showed some biases, then we took the effect sizes (SMD), CIs, and heterogeneity statistics after applying the trim-and-fill method to correct the biases (Duval and Tweedie, 2000). However, for the majority of the cases, the trim and fill did not substantially alter (decreased) the SMD values than the untrimmed SMD values (Supplementary Table 2). Therefore, we used the untrimmed SMD values (i.e., did not adjust for publication bias) for the sake of consistency for all the parameters for creating forest plots. After carefully observing the heterogeneity statistics as well as significance level for SMDs, we performed subgroup analyses for root length parameters to determine the influence of the factors such as plant or endophyte identity, type, etc., because sufficient data were available. For a factor to be included in the analysis as a subgroup variable, it had to be reported from at least four studies.
The articles we reviewed presented findings of experiments involving various host-microbe systems. These investigations considered various parameters in evaluating the influences of microbial colonization in providing heat stress tolerances of plants such as plant biomass and response at the physiological, molecular, metabolic as well as the hormonal level (Figure 1). Effect of fungi was encountered more often, which accounted for 69% of the 42 articles used in our meta-analysis. In general, both fungal and bacterial symbionts were found to increase plant fitness under thermal stress by augmenting photosynthesis, improving antioxidant responses of plants, triggering earlier hormonal signaling, enhancing osmolyte and nutritional balance (Table 1). In the next section, we outlined the influence of microbial symbionts on plant thermal stress tolerance as obtained from our meta-analysis and discussed in light of available literature.
Figure 1. Number of papers out of the total 42 papers reviewed here grouped by response variables. Broad categories of variable are shown in panel (A), while physiological responses are shown in panel (B).
Table 1. The 39 investigations that were included in the review here reporting the effects of microbial symbionts on plant responses to thermal stresses.
The microbial symbiosis significantly stimulated plant shoot dry biomass as well as increased shoot length both under normal and high-temperature stress (Figure 2). Although root dry biomass was found to be significantly augmented (SMD: 0.4728, p: 0.037) by microbial colonization under heat stress, its effect on the length of the root was variable and less prominent (SMD: 0.6739, p: 0.098) even though under non-stressed conditions microbial colonization resulted in longer roots in host plants (Figure 2).
Figure 2. Growth responses of microbe-inoculated plants compared with those of non-inoculated plants under heat stress and non-stressed conditions. Error bars are effect size (SMD) means ± 95% CIs. Where the CIs do not overlap the vertical dashed lines, the effect size for a parameter is significant, i.e., the growth responses of inoculated plants were significantly different from those of non-inoculated plants. n = number of studies included in the meta-analysis, p = significance level of SMD.
Leaf relative water content (RWC) did not show any significant changes due to microbial colonization either under stress or non-stressed conditions. However, the wider CI values propose that the influence on plant RWC varies by some factors (Figure 2).
Since there was significant heterogeneity in microbial impact on plant root length, we attempted to identify the potential moderators by categorical analysis. We noticed that microbes had a substantial impact on root length regardless of whether the host belonged to the legume with non-legume or whether the symbiont belonged to fungi or bacteria under thermal stress (Figures 3A,D). However, under normal conditions, bacterial symbiont had significantly increased root length, but fungi did not demonstrate this effect. The roots of dicotyledonous plants increased significantly in the presence of microbial symbionts regardless of stress impact, but those of monocotyledons did not show this response (Figure 3B). When considering the plant family as a moderator, Poaceae and Asteraceae had an overall neutral influence in root length, but Fabaceae and those belong the other family had significantly positive responses to microbial colonization under both stressed and normal temperature (Figure 3C).
Figure 3. Effects of microbial inoculation on plant root length under normal and high temperature stress conditions for various categorical variables such as (A) Plant nodulation, (B) Plant clade, (C) Plant Family, and (D) microbes’ types (fungi or bacteria). Error bars are the effect size means ± 95% CIs. Where the CIs do not overlap the vertical dashed lines, the effect size for a parameter is significant, i.e., the growth responses of AMF plants were significantly different from those of non-AMF plants. n = number of studies included in the meta-analysis, p = significance level of SMD.
All photosynthetic parameters considered, however, tended to be influenced more under stress than under non-stress conditions, as evident from their larger effect sizes (Figure 4). Microbial colonization significantly increased net photosynthesis, maximum photochemical efficiency (Fv/Fm), and photosynthetic pigments such as chlorophyll, carotenoids, flavonoids, and phenolics in plants under high-temperature stress as compared to non-colonized plants. However, under normal temperature, these effects were not as strong as under stressed conditions (Figure 4). For example, symbiotic plants had 25% (1.37, p = 0.0002) and 51% (2.80, p = 0.004) higher total chlorophyll and net photosynthesis, respectively, over non-symbiotic plants under stress but these were only 11% (0.94, p = 0.004) and 16% (1.79, p = 0.035) higher under ambient conditions (Figure 4). There was no significant effect on stomatal conductance in general, but the wider CI-values, as well as heterogeneity statistics, showed that it varies due to different plant-microbe systems.
Figure 4. Effect of microbe inoculation on photosynthetic parameters compared with those of non-inoculated plants under heat stress and non-stressed conditions. Error bars are effect size (SMD) means ± 95% CIs. Where the CIs do not overlap the vertical dashed lines, the effect size for a parameter is significant, i.e., the growth responses of inoculated plants were significantly different from those of non-inoculated plants. n = number of studies included in the meta-analysis, p = significance level of SMD.
Improvement in the photosynthetic ability of symbiotic plants is correlated clearly with the increased plant biomass of inoculated plants. Thermal stress exerts a negative impact on photosynthetic machinery and gas exchange in plants causing altered physiological and biochemical processes. Similar to heat stress, early reaction to water deficit could result in accelerated stomatal closure and reduced water loss (Malinowski and Belesky, 2000) in microbial colonized plants. Also, an augmented chlorophyll pigment in symbiotic plants under stress reflects their better photosynthesis which corelates with their stress tolerance (Harman, 2000; Harman et al., 2004; Bae et al., 2009). Carotenoids and flavonoids are efficient quenchers of ROS, such as peroxide radicals and singlet oxygen molecules, and thus abate oxidative damage (Wahid et al., 2007; Agati et al., 2012). Increased carotenoids content in microbe-treated plants compared to untreated under heat stress provides evidence of involvement of this antioxidant in the microbe mediated ROS scavenging pathway for increasing plant heat stress tolerance (Figure 4). It has been shown that carotenoids of the xanthophyll family and some other terpenoids bring about a decreased fluidity (thermostability) of the lipid membrane, resulting in reduced susceptibility to lipid peroxidation under high temperatures (Havaux, 1998; Velikova et al., 2004; Sharkey, 2005). It is also worthwhile mentioning that in a recent paper it was demonstrated that plants grown under warmer conditions are able to better control oxidative stress and lipid peroxidation thanks to the activation of higher antioxidant defenses (Bertini et al., 2021). Phenolics, flavonoids, anthocyanins, lignins, etc., are important secondary metabolites in plants and are involved in stress tolerance in the plant (Chalker-Scott, 2002; Wahid, 2007; Wahid et al., 2007). We noted that microbial colonization significantly increased phenolics and flavonoids in plants under heat stress (Figure 4). Increased accumulation of phenolics under heat stress was shown to be associated with higher phenyl ammonia-lyase (PAL) and lower peroxidase and polyphenol lyase activities (Rivero et al., 2001).
One of the important mechanisms plants employ to withstand various stresses, including extreme temperatures stress, is the accumulation of certain organic compounds known as compatible osmolytes (Hare et al., 1998; Sakamoto and Murata, 2002). Depending on the plant species and stress type and severity, various compounds such as sugars, sugar alcohols, proline, glycinebetaine (GB), and tertiary sulfonium compounds are accumulated (Sairam and Tyagi, 2004). In plants, GB plays a vital role as an osmolyte under various stresses, such as drought, salinity, or high temperature (Giri, 2011). Different plants showed varying capacity to synthesize GB under stress (Ashraf and Foolad, 2007; Wahid et al., 2007). However, we did not come across any study that investigated the involvement of GB in microbial-mediated plant heat stress tolerance; there is room for future researchers to find out the role of this compound.
Similar to GB, proline also accumulates in plants under stresses and take part in plant abiotic stress tolerances. Proline accumulation helps in osmotic adjustment and plays a protective role as a ROS scavenger under thermal stress (Szabados and Savouré, 2010; Kaur and Asthir, 2015). However, it is still controversial if its presence is an adaptive response that provides greater stress tolerance or if its increase is a symptom of stress injury (Ashraf and Foolad, 2007). Apparently, no significant difference in proline accumulation in colonized plants versus non-colonized plants (Figure 5) suggests that the effect of proline is not independent but relies on many factors, one among them being phytohormones (Iqbal et al., 2019). Similar findings were reported on proline accumulation and its association in microbe-mediated plant drought tolerance (Dastogeer, 2018). It was hypothesized that relatively higher levels of proline in microbial colonized plants indicate a reduced extent of damage in a stressed plant (Dastogeer and Wylie, 2017; Dastogeer et al., 2018). Proline functions as low-molecular weight chaperones in plants and helps avoid the stress effect (Gupta et al., 2013). Because of its zwitter ion nature, proline accumulates to high-concentration in cell cytoplasm during stress. Yet, over-accumulation of proline could show toxicity to plant cells (Rizhsky et al., 2004). Genotypic variations for varying levels of proline due to stress have been known in earlier studies with sunflower (Amutha et al., 2007; Harsh et al., 2016). Therefore, the status of proline level is not a reliable indicator of stress tolerance of plants (Silvente et al., 2012).
Figure 5. Effect of microbe inoculation on osmplytes and hormones compared with those of non-inoculated plants under heat stress and non-stressed conditions. Error bars are effect size (SMD) means ± 95% CIs. Where the CIs do not overlap the vertical dashed lines, the effect size for a parameter is significant, i.e., the growth responses of inoculated plants were significantly different from those of non-inoculated plants. n = number of studies included in the meta-analysis, p = significance level of SMD.
Similarly, the accumulation of soluble sugars under heat stress has been reported in sugarcane, which entails great implications for heat tolerance (Wahid and Close, 2007). Under high temperatures, fruit set in tomato plants failed due to the disruption of sugar metabolism and proline transport during the narrow window of male reproductive development (Sato et al., 2006). Among other osmolytes, 4-aminobutyric acid (GABA), a non-protein amino acid, is widely distributed throughout the biological world to act as a compatible solute. Several studies showed that various environmental stresses increase GABA accumulation through metabolic or mechanical disruptions, thus leading to cytosolic acidification. Rapid accumulation of GABA in stressed tissues may provide a critical link in the chain of events stemming from perception of environmental stresses to timely physiological responses (Kinnersley and Turano, 2000).
Phytohormones, like abscisic acid (ABA), salicylic acid (SA), and jasmonic acid (JA), serve as signaling compounds during stress (Shinozaki and Yamaguchi-Shinozaki, 2007). ABA, an isoprenoid phytohormone, is involved in the regulation of many physiological processes in plants, including stomatal opening and closing, protein storage, and adaptation to stress. In nature, where heat and drought stress usually coincide, induction of ABA is an important element of thermotolerance, pointing to its role in biochemical pathways essential for survival under heat stress (Maestri et al., 2002; Sah et al., 2016). Several studies highlighted that induction of various heat shock proteins (HSPs) (e.g., HSP70) by ABA may be one mechanism whereby it confers thermotolerance (Pareek et al., 1998). Interestingly, however, in our meta-analysis, ABA level was found significantly lower in colonized plants as compared to the non-colonized plants (Figure 5). Some microbes produce biologically active gibberellins (GAs), which might be associated with plant growth promotion through reducing stress hormones like ABA (Zhang et al., 2014; Verma et al., 2016; Bashar et al., 2019). Under stressful conditions, the plant regulates stress hormones like ABA through active chemical signals, which induce extreme sensitivity to stomatal conductance (de Ollas and Dodd, 2016; Verma et al., 2016).
The salicyclic acid (SA) is an important signaling component in response to systemic acquired resistance (SAR) and the hypersensitive response (HR) (Kawano et al., 1998) and modulates plant growth, development, flowering, stomatal response, ethylene synthesis, and respiration (Waqas et al., 2012; Khan M. I. R. et al., 2015; Wani et al., 2017). Various studies highlighted the roles of SA in improving plant tolerance to heat stress (Larkindale and Knight, 2002; Wang L.-J. et al., 2010; Khan M. I. R. et al., 2015). The SA stabilizes the trimers of heat shock transcription factors and helps them bind heat shock elements to the promoter of heat shock-related genes. SA-induced thermotolerance involves Ca2+ homeostasis and antioxidant systems (Wang and Li, 2006). Our meta-analysis showed that microbial inoculation greatly enhanced SA levels in plants under heat stress conditions (Figure 5).
The Jasmonic acid (JA) has roles in the biosynthesis of defensive secondary metabolites and proteins (Balbi and Devoto, 2008) as well as it is involved in many physiological processes such as resistance to insects and pathogen, pollen development, senescence, and root growth (Lorenzo et al., 2004). Khan et al. (2012b) and Waqas et al. (2015) reported a substantially low induction of this hormone in microbial colonized plants under stress suggesting its involvement in heat tolerance. The JA level was increased in Exophiala sp.-inoculated plants under growth conditions significantly reduced as compared to non-inoculated plants (Waqas et al., 2015). Further studies are needed to observe if reduction of JA is common in all microbe-mediated thermo-tolerance and what are the underlying mechanisms involved.
Another hormone, ethylene is involved in almost all growth and developmental processes in plants, including stress tolerance (Iqbal et al., 2017). Variable responses in ethylene production (i.e., increase or decrease) in plants have been reported under heat stress conditions (Antunes and Sfakiotakis, 2000; Arshad and Frankenberger, 2002; Larkindale and Huang, 2005). We did not come across any study that looked into ethylene production for microbial colonized versus non-colonized plants under normal or stress conditions, and this is an area of future research.
The hormones gibberellins and cytokinins show contrasting roles on plant heat tolerance to ABA (Liu and Hou, 2018; Li N. et al., 2021). However, there are insufficient studies on the effect of gibberellins and cytokinins on microbial conferred heat tolerance for inclusion into our meta-analysis. In a study, an endophyte Penicillium resedanum LK6 produces gibberellins and improves growth, and provides resilience to environmental stresses (Khan A. L. et al., 2015). Further investigation is necessary for revealing the involvement of gibberellins and cytokinins in this regard.
Reactive oxygen species (ROS) are produced in the plant as by-products of aerobic metabolism and exist in several forms such as hydroxyl radicals (•OH) and superoxide anions (O2–), hydrogen peroxide (H2O2), or singlet oxygen (1O2) (Blokhina et al., 2003; Apel and Hirt, 2004; Mittler et al., 2004). The ROS functions as signaling molecules, at low levels, they are necessary for basic biological processes of the plant, but higher levels are detrimental and may cause DNA damage and incorrect timing of programmed cell death (PCD) directly (Tsukagoshi et al., 2010; Zafra et al., 2010; Xie et al., 2014). The plant has an efficient well-coordinated, and rapidly responsive antioxidant system, including both non-enzymatic and enzymatic processes to mitigate the effect of overproduction of ROS. However, the equilibrium between synthesis and removal of ROS may be perplexed under various biotic and abiotic stresses (Quan et al., 2008; Huang et al., 2019). For example, at higher temperatures, there is an increased production of ROS causing a disparity between ROS production and the ability of the scavenging process to detoxify and remove the reactive intermediates (Saidi et al., 2011; Hemantaranjan et al., 2014). The association of microbial symbiosis has been described for higher tolerance to different environmental stresses (Hernandez et al., 2000; Larkindale and Knight, 2002; Halliwell, 2006). Thus, the involvement of the microbiome in mitigating oxidative stress in the plant has recently attracted research interest.
It has been shown in several studies that microbial-mediated plant heat stress tolerance is associated with heightened antioxidant defense in inoculated plants. The contributions of the ascorbate-glutathione (AsA-GSH) cycle on plant tolerance under various abiotic stresses have been reported in many plant species (Anjum et al., 2010; Bartoli et al., 2017; Kuźniak et al., 2017). The four enzymes that steer the cycle are ascorbate peroxidase (APX), dehydroascorbate reductase (DHAR), mono-dehydroascorbate reductase (MDHAR), and glutathione reductase (GR) (Quan et al., 2008; Noctor et al., 2014; Mohi-Ud-Din et al., 2021). In our analysis, we found that there were no overall changes in APX activity in colonized plants under heat stress (Figure 6). However, the wider CI values indicated that the APX accumulation is variable depending on host-microbe types or other factors. For example, it was reported that treatment with bacteria (Bacillus amyloliquefaciens or Azospirillum brasilense) decreased the activity of this enzyme as well as significantly lower expression of the APX1 gene was observed in heat exposed wheat seedlings (Abd El-Daim et al., 2014). A strikingly different finding was reported in several other studies where microbial treatment increased the level of APX accumulation over non-treated plants under high heat conditions (Maya and Matsubara, 2013; Yeasmin et al., 2019; Khan et al., 2020b). The other two enzymes: DHAR and MDHAR, could not be included in our meta-analysis because of the insufficient number of reports, but both of them were found to increase significantly in plants under elevated temperature, and microbial colonization results in lower accumulation as compared to non-colonized plants (Abd El-Daim et al., 2014, 2019). In general, a significantly higher accumulation of GR in the microbial colonized plants over non-colonized plants was observed through our meta-analysis (Figure 6). The GR plays a crucial role in controlling the levels of reduced glutathione in the plant (Huang et al., 2019). Glutathione modulates ROS levels in cells through both auxin/PLETHORA (PLT) dependent and independent pathways, thereby participating in and maintaining ROS homeostasis in the plant (Yu et al., 2013).
Figure 6. Effects of microbial inoculation on plant ROS production and antioxidant enzymatic activity under non-stressed, and heat stress. Error bars are means ± 95% CIs. Where the CIs do not overlap the vertical dashed lines, the effect size for a parameter is significant. n = number of studies included in the meta-analysis, p = significance level of SMD.
Apart from the AsA-GSH cycle, SOD (superoxide dismutase), CAT (catalase), POD (peroxidase), glutathione peroxidases (GPX), and glutathione-S-transferases (GST) are important antioxidant enzymes in plants taking part in ROS homeostasis under stress. In the plant cells, SOD provides primary protection against O2•–, which is then converted to H2O2 for subsequent metabolism to H2O by CAT and peroxidases (POD, APX, and GPX), thus protecting the cell damage (Mittler et al., 2004; Gill and Tuteja, 2010; Wang G. et al., 2010; Mohi-Ud-Din et al., 2021). The current meta-analysis showed no apparent disparity in SOD measurement in colonized over non-colonized plants, but the higher CI values indicated that SOD accumulation varied significantly among studies. For example, in a study, Li X. et al. (2021) did not notice any changes in SOD accumulation in Aspergillus aculeatus inoculated Lolium perenne, although this fungus improved the heat tolerance of this plant. Similar results have been reported for Septoglomus deserticola and Septoglomus constrictum (AM fungi) mediated heat stress tolerance in the tomato plant with no apparent changes in SOD activity (Duc et al., 2018). In contrast, a few studies reported a significant increase in SOD in microbial colonized plants under high-temperature stress (Maya and Matsubara, 2013; Yeasmin et al., 2019; Bruno et al., 2020; Khan et al., 2020a). Regarding CAT and POD, they showed consistently higher accumulation (CAT, p = 0.021; POD, p = 0.006) in colonized plants as compared to non-colonized controls under heat stress across various studies (Figure 6).
Also, a significantly decreased level of hydrogen peroxide (H2O2) and Malondialdehyde (MDA) (p < 0.001) in microbial-treated plants adds further support to a lower level of heat stress injury due to the increased induction of the antioxidant defense systems compared to the non-treated plants (Figure 6). The MDA is the principal and extensively studied product of polyunsaturated fatty acid (PUFAs) peroxidation. The MDA levels are used as a measure of membrane lipid peroxidation and oxidative stress and the impact it has on membrane fluidity, leakiness, and damage of membrane proteins, enzymes, and ion channels (Garg and Manchanda, 2009; de Dios Alché, 2019).
There is a huge lack of understanding of the mechanisms of microbe-mediated plant heat stress tolerance at the molecular level. The heat shock protein (HSPs) act as “molecular chaperones,” and their upregulation is well known to increase thermotolerance in plants. The expression of heat stress-dependent regulatory proteins such as heat shock transcription factors (HSFs) and heat shock proteins (HSPs) have been described. The HSPs are crucial in regulating and restoring protein structures and maintaining the condition of plants under heat stress (Queitsch et al., 2000; Nakamoto and Vigh, 2007; Xu et al., 2012; Wang et al., 2014). Several studies reported an increased level of expression of HSPs in the microbial colonized plant compared to non-colonized plants under heat stress (Abd El-Daim et al., 2019; Bruno et al., 2020; Li X. et al., 2021). To reveal the mechanisms through which Enterobacter sp. SA187 mediates plant thermotolerance in Arabidopsis Shekhawat et al. (2020) carried out RNA-seq and targeted transcriptomic analyses. They described that the bacteria-mediated heat tolerance is associated with the gene networks for heat protection of plants similar to that of the heat priming (pre-exposure of plants to heat that enables plants to be better prepared to response to heat stress). For example, heat tolerance is associated with the upregulation of genes related to the auxin and gibberellin hormones and the down-regulation of the genes related to jasmonic and abscisic acid. It was also found to be related to hyper-induction of heat-responsive and heat memory genes, which is proven in heat primed plants (Lämke et al., 2016; Ling et al., 2018; Liu et al., 2018). The bacteria inoculated plants resulted in higher expression of several heat-responsive genes such as HSFA2, HSP101, HSP70, HSP70B, GA3OX1, HSP90.1, and ATERDJ3A as well as heat memory genes; APX2 (ascorbate peroxidase 2), HSP18.2, H3K4me3 (epigenetic modification to the DNA packaging protein Histone H3) (Lämke et al., 2016; Sedaghatmehr et al., 2016). In a study, increased expression of heat shock protein gene HSP17.8 and Dehydration-responsive gene DREB1 and decreased expression of POD47 and FeSOD have been reported for fungal (Aspergillus aculeatus) conferred heat stress tolerance in Lolium perenne grass (Li X. et al., 2021). Khan et al. (2020b) reported that GmLAX3 and GmAKT2, the key genes involved in the regulation of the ABA-dependent pathway, were downregulated initially but upregulated after 5 and 10 days in the inoculated soybean plant under thermal stress. The expression of these genes may be linked with plant stress response by modulating auxin and ABA signaling, decreasing ROS production and enhancing K+ gradients, which are critical in plant tissues under various stresses (Zhou et al., 2014; Chai et al., 2016). The B. velezensis inoculation induced an increased abiotic stress tolerance, and the pattern of metabolic modulation and abundance of several proteins were found to be similar in wheat challenged with different abiotic stress factors such as heat, cold, and drought (Abd El-Daim et al., 2019).
Taken together, high-temperature influences plant growth by affecting photosynthesis, osmotic balance, enzymatic activities, and metabolic activities. Microbial symbionts consistently help plants reduce the impact of thermal stress by manipulating physiological processes (Figure 7). Microbe-induced plant heat stress tolerance has broad ecological and agricultural implications. In some regions, increased stress tolerance can result in higher crop yield under higher temperature environments. Continued interest in the microbiome and their functional roles in uncovering the underlying mechanisms of plant-microbe interaction appears well-justified.
Figure 7. Illustration of the effects of microbial inoculation on plant physiological activity and stress tolerance under high temperature. The upward arrow (↑) indicates increase of the value for microbial-treated plants compared to non-treated plants whereas, downward arrow (↓) indicates decease, wavy line (∼) indicates the effect is variable and the question mark (?) unavailable information.
Despite the evidence of global warming and the importance of heat stress on plant ecology and productivity (Wahid et al., 2007; Teixeira et al., 2013; Jagadish et al., 2021) and the increasing evidence of microbial functions of plant stress tolerance (Table 1 and Figure 1), the current review here identified a significant lack in our knowledge on microbial conferred plant performance and productivity under high-temperature conditions. Apparently, the most crucial of these is the lack of experiments that demonstrate how do microbes actually influence plant responses in the natural environment. Most of the investigations reviewed here were carried out under controlled environmental conditions and on cultivated host species. Therefore, experiments focusing on the impacts of symbionts on wild and crop plants exposed to heat stresses in the natural environment are required. In a recent paper, Ballesteros et al. (2020) analyzed metatranscriptomic data from the Antarctic plant Colobanthus quitensis, highlighting that the plant-associated fungal community provides beneficial effects, such as increased plant growth, tolerance to abiotic stress, and higher leaf carbon gain, when plants are grown under a simulated global warming scenario. The reports from these investigations should include mean values of response variables, measures of variance, and replication and preferably provide the actual dataset as the Supplementary Data so that these data can be used in future meta-analyses. Although it was shown that increasing temperatures due to climate change might negatively impact the outcomes of symbioses (Kiers et al., 2010), the current meta-analyses indicated that microbial symbionts have more positive effects on plant performance under heat stress than under normal conditions. It is not clear why microbial inoculation exerts its effects in more stressed conditions than under normal temperatures conditions, but putatively, this is associated with plant stress acclimation at the molecular or cellular levels. Tolerance of non-colonized plants to heat stress is well studied, and the reports suggested that the mechanisms are associated with enhanced expression of a variety of heat shock proteins, other related proteins, production of ROS, and induction of mitogen-activated protein kinase (MAPK) and calcium-dependent protein kinase (CDPK) cascades, and, most importantly, chaperone signaling and transcriptional activation (Wahid et al., 2007). As previously shown for abiotic stresses such as freezing and drought and assumed for microbial conferred cold adaptation (Knight and Knight, 2001; Acuna-Rodriguez et al., 2020), some kind of cross-talk between the proteins common to signaling pathways are present for responses to heat and microbial colonization leading to enhanced plant metabolism. The involvement of microbial elicitors at the plant cell surface resulting in increases in cytosolic Ca2+ concentrations could be another possibility (Sanders et al., 1999; Acuna-Rodriguez et al., 2020). However, more research is needed to establish whether these or similar mechanisms might explain the beneficial effects of microbial symbionts on plants at higher temperatures. As discussed by Acuna-Rodriguez et al. (2020), less attention has been paid toward understanding the differences in the optimum growth temperatures of microbial symbionts and their plant hosts. For instance, in the Epichloe symbiosis, the seemingly decreased plant performance at low temperatures in the natural conditions could be that the minimum temperatures requirement of the endophytes being higher than those of their hosts (Casler and Van Santen, 2008; Wäli et al., 2008). Extensive surveys to identify novel microbial symbionts capable of improving plant performance under stress are advocated. Isolation of naturally occurring plant and soil microbiomes and screening them for potential symbiotic benefits is a continuous process. Developing a rapid but robust screening method is important so that a large collection of microbes can be tested for their effects, and the results should be supported by field experiments in order to translate the research into the field. A few surveys focused on endophytes collected from hot environments, and some of the endophytes tested were found to grow at high temperatures (Zhou et al., 2015; Dastogeer et al., 2019). Studies on other components of the plant microbiome such as algae, protists, and viruses revealed that they are also present in the tissues or in the rhizosphere of many plant species and provide some fitness benefits (Roossinck, 2015; Gao et al., 2019; Dastogeer et al., 2020a; Dumack and Bonkowski, 2021; Lee and Ryu, 2021). For example, rhizosphere protists altered the metabolites of the maize plant, particularly, polyols. This alteration is presumably to be associated with reduction of the impact of stress caused due to grazing (Kuppardt et al., 2018). A plant virus, yellowtail flower mild mottle virus (YTMMV, genus Tobamovirus), enhanced drought stress tolerance in Nicotiana benthamiana plants by modulating its metabolic compounds (Dastogeer et al., 2018). However, the effect of many of the microbiome components has received less attention. We also suggest studies that consider microbial symbionts on plant heat tolerance should take into account the involvement of secondary metabolites because many microbes utilize these compounds in the interaction with plants and with environments (Zaynab et al., 2018; Ullah et al., 2020). Another important area of research, but has so far been neglected, is to study the multi-partite interaction of microbes and their impact on stress tolerance, such as thermal tolerance. It was found that a fungus can increase the heat tolerance of a plant only in the presence of a virus within it, whereas neither virus nor the plant separately could confer this benefit. Therefore, a three-way symbiosis is required for thermal tolerance. In nature, plants do not remain in isolation but in continuous interaction with a wide variety of microbes (Marquez et al., 2007). In addition to microbe-plant interaction, microbe-microbe and microbe-environment interaction should be taken into account to understand the stable functions of a particular microbe in its potential application in agricultural settings, which is a dynamic environment and subject to continuous changes (Dastogeer et al., 2020a).
KD developed the concept, performed the database search, partially collected the data, analyzed and interpreted the results, and wrote the draft. MZ, MR, and MS extracted the data, produced the graphs, discussed the results, and revised the manuscript. AC produced the table and discussed the results. All authors approved the final version of the manuscript.
The authors declare that the research was conducted in the absence of any commercial or financial relationships that could be construed as a potential conflict of interest.
All claims expressed in this article are solely those of the authors and do not necessarily represent those of their affiliated organizations, or those of the publisher, the editors and the reviewers. Any product that may be evaluated in this article, or claim that may be made by its manufacturer, is not guaranteed or endorsed by the publisher.
The Supplementary Material for this article can be found online at: https://www.frontiersin.org/articles/10.3389/fmicb.2022.833566/full#supplementary-material
Abd El-Daim, I. A., Bejai, S., Fridborg, I., and Meijer, J. (2018). Identifying potential molecular factors involved in Bacillus amyloliquefaciens 5113 mediated abiotic stress tolerance in wheat. Plant Biol. 20, 271–279. doi: 10.1111/plb.12680
Abd El-Daim, I. A., Bejai, S., and Meijer, J. (2014). Improved heat stress tolerance of wheat seedlings by bacterial seed treatment. Plant Soil 379, 337–350. doi: 10.1007/s11104-014-2063-3
Abd El-Daim, I. A., Bejai, S., and Meijer, J. (2019). Bacillus velezensis 5113 induced metabolic and molecular reprogramming during abiotic stress tolerance in wheat. Sci. Rep. 9, 1–18. doi: 10.1038/s41598-019-52567-x
Acuna-Rodriguez, I. S., Newsham, K. K., Gundel, P. E., Torres-Diaz, C., and Molina-Montenegro, M. A. (2020). Functional roles of microbial symbionts in plant cold tolerance. Ecol. Lett. 23, 1034–1048. doi: 10.1111/ele.13502
Agati, G., Azzarello, E., Pollastri, S., and Tattini, M. (2012). Flavonoids as antioxidants in plants: location and functional significance. Plant Sci. 196, 67–76. doi: 10.1016/j.plantsci.2012.07.014
Ahn, E., and Kang, H. (2018). Introduction to systematic review and meta-analysis. Korean J. Anesthesiol. 71:103.
Ali, A. H., Abdelrahman, M., Radwan, U., El-Zayat, S., and El-Sayed, M. A. (2018). Effect of Thermomyces fungal endophyte isolated from extreme hot desert-adapted plant on heat stress tolerance of cucumber. Appl. Soil Ecol. 124, 155–162. doi: 10.1016/j.apsoil.2017.11.004
Ali, A. H., Radwan, U., El-Zayat, S., and El-Sayed, M. A. (2019). The role of the endophytic fungus, Thermomyces lanuginosus, on mitigation of heat stress to its host desert plant Cullen plicata. Biol. Futura 70, 1–7. doi: 10.1556/019.70.2019.01
Ali, S. Z., Sandhya, V., Grover, M., Kishore, N., Rao, L. V., and Venkateswarlu, B. (2009). Pseudomonas sp. strain AKM-P6 enhances tolerance of sorghum seedlings to elevated temperatures. Biol. Fertil. Soils 46, 45–55. doi: 10.1007/s00374-009-0404-9
Ali, S. Z., Sandhya, V., Grover, M., Linga, V. R., and Bandi, V. (2011). Effect of inoculation with a thermotolerant plant growth promoting Pseudomonas putida strain AKMP7 on growth of wheat (Triticum spp.) under heat stress. J. Plant Interact. 6, 239–246. doi: 10.1080/17429145.2010.545147
Amutha, R., Muthulaksmi, S., Baby Rani, W., Indira, K., and Mareeswari, P. (2007). Studies on biochemical basis of heat tolerance in sunflower (Helianthus annus L.). Res. J. Agric. Biol. Sci. 3, 234–238.
Anjum, N. A., Umar, S., and Chan, M.-T. (2010). Ascorbate-Glutathione Pathway and Stress Tolerance in Plants. Berlin: Springer Science & Business Media.
Antunes, M., and Sfakiotakis, E. (2000). Effect of high temperature stress on ethylene biosynthesis, respiration and ripening of ‘Hayward’kiwifruit. Postharvest Biol. Technol. 20, 251–259. doi: 10.1016/s0925-5214(00)00136-8
Apel, K., and Hirt, H. (2004). Reactive oxygen species: metabolism, oxidative stress, and signal transduction. Annu. Rev. Plant Biol. 55, 373–399. doi: 10.1146/annurev.arplant.55.031903.141701
Arshad, M., and Frankenberger, W. T. Jr. (2002). Ethylene: Agricultural Resources and Applications. Berlin: Springer science & Business media.
Ashraf, M., and Foolad, M. R. (2007). Roles of glycine betaine and proline in improving plant abiotic stress resistance. Environ. Exp. Bot. 59, 206–216.
Azad, K., and Kaminskyj, S. (2016). A fungal endophyte strategy for mitigating the effect of salt and drought stress on plant growth. Symbiosis 68, 73–78. doi: 10.1371/journal.pone.0014823
Bae, H., Sicher, R. C., Kim, M. S., Kim, S. H., Strem, M. D., Melnick, R. L., et al. (2009). The beneficial endophyte Trichoderma hamatum isolate DIS 219b promotes growth and delays the onset of the drought response in Theobroma cacao. J. Exp. Bot. 60, 3279–3295. doi: 10.1093/jxb/erp165
Balbi, V., and Devoto, A. (2008). Jasmonate signalling network in Arabidopsis thaliana: crucial regulatory nodes and new physiological scenarios. New Phytol. 177, 301–318. doi: 10.1111/j.1469-8137.2007.02292.x
Balduzzi, S., Rücker, G., and Schwarzer, G. (2019). How to perform a meta-analysis with R: a practical tutorial. Evidence Based Ment. Health 22, 153–160. doi: 10.1136/ebmental-2019-300117
Ballesteros, G. I., Torres-Diaz, C., Bravo, L. A., Balboa, K., Caruso, C., Bertini, L., et al. (2020). In silico analysis of metatranscriptomic data from the Antarctic vascular plant Colobanthus quitensis: responses to a global warming scenario through changes in fungal gene expression levels. Fung. Ecol. 43:100873. doi: 10.1016/j.funeco.2019.100873
Bartoli, C. G., Buet, A., Grozeff, G. G., Galatro, A., and Simontacchi, M. (2017). “Ascorbate-glutathione cycle and abiotic stress tolerance in plants,” in Ascorbic Acid in Plant Growth, Development and Stress Tolerance, eds M. A. Hossain, S. Munné-Bosch, D. J. Burritt, P. Diaz-Vivancos, M. Fujita, and A. Lorence (Berlin: Springer), 177–200. doi: 10.1007/978-3-319-74057-7_7
Bashar, K. K., Tareq, M., Amin, M., Honi, U., Tahjib-Ul-Arif, M., Sadat, M., et al. (2019). Phytohormone-mediated stomatal response, escape and quiescence strategies in plants under flooding stress. Agronomy 9:43.
Basit, A., Shah, S. T., Ullah, I., Ullah, I., and Mohamed, H. I. (2021). “Microbial bioactive compounds produced by endophytes (bacteria and fungi) and their uses in plant health,” in Plant Growth-Promoting Microbes for Sustainable Biotic and Abiotic Stress Management, eds H. I. Mohamed, H. E. D. Saad El-Beltagi, and K. A. Abd-Elsalam (Berlin: Springer), 285–318.
Begg, C., and Mazumdar, M. (1994). Operating characteristics of a rank correlation test for publication bias. Biometrics 50, 1088–1101. doi: 10.2307/2533446
Bertini, L., Cozzolino, F., Proietti, S., Falconieri, G. S., Iacobucci, I., Salvia, R., et al. (2021). What antarctic plants can tell us about climate changes: temperature as a driver for metabolic reprogramming. Biomolecules 11:1094. doi: 10.3390/biom11081094
Blokhina, O., Virolainen, E., and Fagerstedt, K. V. (2003). Antioxidants, oxidative damage and oxygen deprivation stress: a review. Ann. Bot. 91, 179–194. doi: 10.1093/aob/mcf118
Borenstein, M., Hedges, L. V., Higgins, J. P. T., and Rothstein, H. R. (2009). Introduction to Meta-Analysis. Chichester: Wiley.
Brachmann, A., and Parniske, M. (2006). The most widespread symbiosis on earth. PLoS Biol. 4:e239. doi: 10.1371/journal.pbio.0040239
Bruno, L. B., Karthik, C., Ma, Y., Kadirvelu, K., Freitas, H., and Rajkumar, M. (2020). Amelioration of chromium and heat stresses in Sorghum bicolor by Cr6+ reducing-thermotolerant plant growth promoting bacteria. Chemosphere 244:125521. doi: 10.1016/j.chemosphere.2019.125521
Bunn, R., Lekberg, Y., and Zabinski, C. (2009). Arbuscular mycorrhizal fungi ameliorate temperature stress in thermophilic plants. Ecology 90, 1378–1388. doi: 10.1890/07-2080.1
Cabral, C., Ravnskov, S., Tringovska, I., and Wollenweber, B. (2016). Arbuscular mycorrhizal fungi modify nutrient allocation and composition in wheat (Triticum aestivum L.) subjected to heat-stress. Plant Soil 408, 385–399. doi: 10.1007/s11104-016-2942-x
Casler, M. D., and Van Santen, E. (2008). Fungal endophyte removal does not reduce cold tolerance of tall fescue. Crop Sci. 48, 2033–2039. doi: 10.2135/cropsci2007.11.0615
Chai, C., Wang, Y., Valliyodan, B., and Nguyen, H. T. (2016). Comprehensive analysis of the soybean (Glycine max) GmLAX auxin transporter gene family. Front. Plant Sci. 7:282. doi: 10.3389/fpls.2016.00282
Chalker-Scott, L. (2002). Do anthocyanins function as osmoregulators in leaf tissues? Adv. Bot. Res. 37, 103–127. doi: 10.1007/s00709-015-0771-z
Chandrasekaran, M., Boughattas, S., Hu, S., Oh, S.-H., and Sa, T. (2014). A meta-analysis of arbuscular mycorrhizal effects on plants grown under salt stress. Mycorrhiza 24, 611–625. doi: 10.1007/s00572-014-0582-7
Chandrasekaran, M., Kim, K., Krishnamoorthy, R., Walitang, D., Sundaram, S., Joe, M. M., et al. (2016). Mycorrhizal symbiotic efficiency on C3 and C4 plants under salinity stress–a meta-analysis. Front. Microbiol. 7:1246. doi: 10.3389/fmicb.2016.01246
Cohen, J. (1988). Statistical Power Analysis for the Behavioral Sciences. Hillsdale, NJ: L. Erlbaum Associates.
Compant, S., Samad, A., Faist, H., and Sessitsch, A. (2019). A review on the plant microbiome: ecology, functions, and emerging trends in microbial application. J. Adv. Res. 19, 29–37. doi: 10.1016/j.jare.2019.03.004
Cooper, H. (2015). Research Synthesis and Meta-Analysis: A Step-By-Step Approach. Thousand Oaks, CA: Sage publications.
Cooper, H., Hedges, L. V., and Valentine, J. C. (2019). The Handbook of Research Synthesis and Meta-Analysis. New York, NY: Russell Sage Foundation.
Dastogeer, K. M. (2018). Influence of fungal endophytes on plant physiology is more pronounced under stress than well-watered conditions: a meta-analysis. Planta 248, 1403–1416. doi: 10.1007/s00425-018-2982-y
Dastogeer, K. M., Tumpa, F. H., Sultana, A., Akter, M. A., and Chakraborty, A. (2020a). Plant microbiome–an account of the factors that shape community composition and diversity. Curr. Plant Biol. 23:100161. doi: 10.1016/j.cpb.2020.100161
Dastogeer, K. M., Zahan, M. I., Tahjib-Ul-Arif, M., Akter, M. A., and Okazaki, S. (2020b). Plant salinity tolerance conferred by arbuscular mycorrhizal fungi and associated mechanisms: a meta-analysis. Front. Plant Sci. 11:588550. doi: 10.3389/fpls.2020.588550
Dastogeer, K. M. G., Li, H., Sivasithamparam, K., Jones, M. G. K., and Wylie, S. J. (2018). Fungal endophytes and a virus confer drought tolerance to Nicotiana benthamiana plants through modulating osmolytes, antioxidant enzymes and expression of host drought responsive genes. Environ. Exp. Bot. 149, 95–108. doi: 10.1016/j.envexpbot.2018.02.009
Dastogeer, K. M. G., Li, H., Sivasithamparam, K., and Wylie, S. J. (2019). In vitro salt and thermal tolerance of fungal endophytes of Nicotiana spp. growing in arid regions of north-western Australia. Arch. Phytopathol. Plant Protect. 52, 602–616. doi: 10.1080/03235408.2018.1503762
Dastogeer, K. M. G., and Wylie, S. J. (2017). “Plant–fungi association: role of fungal endophytes in improving plant tolerance to water stress,” in Plant-Microbe Interactions in Agro-Ecological Perspectives, eds D. P. Singh, H. B. Singh, and R. Prabha (Cham: Springer Nature Singapore Pte Ltd.).
de Dios Alché, J. (2019). A concise appraisal of lipid oxidation and lipoxidation in higher plants. Redox Biol. 23:101136. doi: 10.1016/j.redox.2019.101136
de Ollas, C., and Dodd, I. C. (2016). Physiological impacts of ABA–JA interactions under water-limitation. Plant Mol. Biol. 91, 641–650. doi: 10.1007/s11103-016-0503-6
DerSimonian, R., and Laird, N. (1986). Meta-analysis in clinical trials. Control. Clin. Trials 7, 177–188.
Duc, N. H., Csintalan, Z., and Posta, K. (2018). Arbuscular mycorrhizal fungi mitigate negative effects of combined drought and heat stress on tomato plants. Plant Physiol. Biochem. 132, 297–307. doi: 10.1016/j.plaphy.2018.09.011
Dumack, K., and Bonkowski, M. (2021). “Protists in the plant microbiome: an untapped field of research,” in The Plant Microbiome, eds L. C. Carvalhais and P. G. Dennis (Cham: Springer), 77–84. doi: 10.1007/978-1-0716-1040-4_8
Duval, S., and Tweedie, R. (2000). Trim and fill: a simple funnel-plot–based method of testing and adjusting for publication bias in meta-analysis. Biometrics 56, 455–463. doi: 10.1111/j.0006-341x.2000.00455.x
Eerens, J. P. J., Lucas, R. J., Easton, S., and White, J. G. H. (1998). Influence of the endophyte (Neotyphodium lolii) on morphology, physiology, and alkaloid synthesis of perennial ryegrass during high temperature and water stress. N. Zeal. J. Agric. Res. 41, 219–226. doi: 10.1080/00288233.1998.9513305
Egger, M., Smith, G. D., Schneider, M., and Minder, C. (1997). Bias in meta-analysis detected by a simple, graphical test. Increase in studies of publication bias coincided with increasing use of meta-analysis. Br. Med. J. 316, 629–634.
Faraone, S. V. (2008). Interpreting estimates of treatment effects: implications for managed care. Pharm. Ther. 33:700.
Field, A. P., and Gillett, R. (2010). How to do a meta-analysis. Br. J. Math. Stat. Psychol. 63, 665–694.
Gao, Z., Karlsson, I., Geisen, S., Kowalchuk, G., and Jousset, A. (2019). Protists: puppet masters of the rhizosphere microbiome. Trends Plant Sci. 24, 165–176. doi: 10.1016/j.tplants.2018.10.011
Garg, N., and Manchanda, G. (2009). ROS generation in plants: boon or bane? Plant Biosyst. 143, 81–96.
Gill, S. S., and Tuteja, N. (2010). Reactive oxygen species and antioxidant machinery in abiotic stress tolerance in crop plants. Plant Physiol. Biochem. 48, 909–930. doi: 10.1016/j.plaphy.2010.08.016
Giri, J. (2011). Glycinebetaine and abiotic stress tolerance in plants. Plant Signal. Behav. 6, 1746–1751.
Goh, C.-H., Vallejos, D. F. V., Nicotra, A. B., and Mathesius, U. (2013). The impact of beneficial plant-associated microbes on plant phenotypic plasticity. J. Chem. Ecol. 39, 826–839. doi: 10.1007/s10886-013-0326-8
González-Teuber, M. (2016). The defensive role of foliar endophytic fungi for a South American tree. AoB Plants 8:lw050. doi: 10.1093/aobpla/plw050
Gupta, N., Agarwal, S., Agarwal, V., Nathawat, N., Gupta, S., and Singh, G. (2013). Effect of short-term heat stress on growth, physiology and antioxidative defence system in wheat seedlings. Acta Physiol. Plant. 35, 1837–1842.
Gurevitch, J., and Hedges, L. (1999). Statistical issues in ecological metaanalyses. Ecology 80, 1142–1149. doi: 10.1890/0012-9658(1999)080[1142:siiema]2.0.co;2
Halliwell, B. (2006). Reactive species and antioxidants. Redox biology is a fundamental theme of aerobic life. Plant Physiol. 141, 312–322. doi: 10.1104/pp.106.077073
Hardoim, P. R., Van Overbeek, L. S., Berg, G., Pirttilä, A. M., Compant, S., Campisano, A., et al. (2015). The hidden world within plants: ecological and evolutionary considerations for defining functioning of microbial endophytes. Microbiol. Mol. Biol. Rev. 79, 293–320. doi: 10.1128/MMBR.00050-14
Hare, P. D., Cress, W. A., and Van Staden, J. (1998). Dissecting the roles of osmolyte accumulation during stress. Plant Cell Environ. 21, 535–553. doi: 10.1046/j.1365-3040.1998.00309.x
Harman, G. E. (2000). Myths and dogmas of biocontrol. Changes in perceptions derived from research on Trichoderma harzianum T-22. Plant Dis. 84, 377–393. doi: 10.1094/PDIS.2000.84.4.377
Harman, G. E., Howell, C. R., Viterbo, A., Chet, I., and Lorito, M. (2004). Trichoderma species – opportunistic, avirulent plant symbionts. Nat. Rev. Microbiol. 2, 43–56. doi: 10.1038/nrmicro797
Harman, G. E., and Uphoff, N. (2019). Symbiotic root-endophytic soil microbes improve crop productivity and provide environmental benefits. Scientifica 2019:9106395. doi: 10.1155/2019/9106395
Harsh, A., Sharma, Y., Joshi, U., Rampuria, S., Singh, G., Kumar, S., et al. (2016). Effect of short-term heat stress on total sugars, proline and some antioxidant enzymes in moth bean (Vigna aconitifolia). Ann. Agric. Sci. 61, 57–64. doi: 10.1016/j.aoas.2016.02.001
Hasanuzzaman, M., Nahar, K., Alam, M., Roychowdhury, R., and Fujita, M. (2013). Physiological, biochemical, and molecular mechanisms of heat stress tolerance in plants. Int. J. Mol. Sci. 14, 9643–9684. doi: 10.3390/ijms14059643
Hassan, M. U., Chattha, M. U., Khan, I., Chattha, M. B., Barbanti, L., Aamer, M., et al. (2021). Heat stress in cultivated plants: nature, impact, mechanisms, and mitigation strategies—A review. Plant Biosyst. Int. J. Deal. Aspects Plant Biol. 155, 211–234. doi: 10.1080/11263504.2020.1727987
Havaux, M. (1998). Carotenoids as membrane stabilizers in chloroplasts. Trends Plant Sci. 3, 147–151. doi: 10.1016/s1360-1385(98)01200-x
Hedges, L. V., Gurevitch, J., and Curtis, P. S. (1999). The meta-analysis of response ratios in experimental ecology. Ecology 80, 1150–1156.
Hemantaranjan, A., Bhanu, A. N., Singh, M., Yadav, D., Patel, P., Singh, R., et al. (2014). Heat stress responses and thermotolerance. Adv. Plants Agric. Res. 1, 1–10.
Hennessy, L. M., Popay, A. J., Finch, S. C., Clearwater, M. J., and Cave, V. M. (2016). Temperature and plant genotype alter alkaloid concentrations in ryegrass infected with an Epichloë endophyte and this affects an insect herbivore. Front. Plant Sci. 7:1097. doi: 10.3389/fpls.2016.01097
Hernandez, J. A., Jiménez, A., Mullineaux, P., and Sevilia, F. (2000). Tolerance of pea (Pisum sativum L.) to long-term salt stress is associated with induction of antioxidant defences. Plant Cell Environ. 23, 853–862.
Higgins, J. P., Thompson, S. G., Deeks, J. J., and Altman, D. G. (2003). Measuring inconsistency in meta-analyses. Br. Med. J. 327, 557–560. doi: 10.1136/bmj.327.7414.557
Higgins, J. P. T., and Thompson, S. G. (2002). Quantifying heterogeneity in a meta- analysis. Stat. Med. 21, 1539–1558. doi: 10.1002/sim.1186
Hill, P. W., Broughton, R., Bougoure, J., Havelange, W., Newsham, K. K., Grant, H., et al. (2019). Angiosperm symbioses with non-mycorrhizal fungal partners enhance N acquisition from ancient organic matter in a warming maritime Antarctic. Ecol. Lett. 22, 2111–2119. doi: 10.1111/ele.13399
Hoeksema, J. D., Chaudhary, V. B., Gehring, C. A., Johnson, N. C., Karst, J., Koide, R. T., et al. (2010). A meta-analysis of context-dependency in plant response to inoculation with mycorrhizal fungi. Ecol. Lett. 13, 394–407. doi: 10.1111/j.1461-0248.2009.01430.x
Holmgren, M., Gómez-Aparicio, L., Quero, J. L., and Valladares, F. (2012). Non-linear effects of drought under shade-reconciling physiological and ecological models in plant communities. Oecologia 169, 293–305. doi: 10.1007/s00442-011-2196-5
Huang, H., Ullah, F., Zhou, D.-X., Yi, M., and Zhao, Y. (2019). Mechanisms of ROS regulation of plant development and stress responses. Front. Plant Sci. 10:800. doi: 10.3389/fpls.2019.00800
Hubbard, M., Germida, J., and Vujanovic, V. (2012). Fungal endophytes improve wheat seed germination under heat and drought stress. Bot. Botanique 90, 137–149. doi: 10.1139/B11-091
Hubbard, M., Germida, J. J., and Vujanovic, V. (2014). Fungal endophytes enhance wheat heat and drought tolerance in terms of grain yield and second-generation seed viability. J. Appl. Microbiol. 116, 109–122. doi: 10.1111/jam.12311
Huedo-Medina, T. B., Sánchez-Meca, J., Marín-Martínez, F., and Botella, J. (2006). Assessing heterogeneity in meta-analysis: Q statistic or I2 index? Psychol. Methods 11, 193–206. doi: 10.1037/1082-989X.11.2.193
IPCC (2019). An IPCC Special Report on the Impacts of Global Warming of 1.5° C above Pre-Industrial Levels and Related Global Greenhouse Gas Emission Pathways. Geneva: IPCC.
Iqbal, N., Fatma, M., Khan, N. A., and Umar, S. (2019). “Regulatory role of proline in heat stress tolerance: modulation by salicylic acid,” in Plant Signaling Molecules, eds M. I. Khan, P. Sudhakar Reddy, A. Ferrante, and N. A. Khan (Amsterdam: Elsevier), 437–448.
Iqbal, N., Khan, N. A., Ferrante, A., Trivellini, A., Francini, A., and Khan, M. (2017). Ethylene role in plant growth, development and senescence: interaction with other phytohormones. Front. Plant Sci. 8:475. doi: 10.3389/fpls.2017.00475
Ismail, I., Hamayun, M., Hussain, A., Afzal Khan, S., Iqbal, A., and Lee, I.-J. (2019). Aspergillus flavus promoted the growth of soybean and sunflower seedlings at elevated temperature. BioMed Res. Int. 2019:1295457. doi: 10.1155/2019/1295457
Ismail, I., Hamayun, M., Hussain, A., Iqbal, A., Khan, S. A., and Lee, I. J. (2018). Endophytic fungus aspergillus japonicus mediates host plant growth under normal and heat stress conditions. Biomed Res. Int. 2018:7696831. doi: 10.1155/2018/7696831
Ismail, I., Hamayun, M., Hussain, A., Iqbal, A., Khan, S. A., and Lee, I.-J. (2020a). Aspergillus niger boosted heat stress tolerance in sunflower and soybean via regulating their metabolic and antioxidant system. J. Plant Interact. 15, 223–232.
Ismail, I., Hamayun, M., Hussain, A., Khan, S. A., Iqbal, A., and Lee, I. J. (2020b). An endophytic fungus Aspergillus violaceofuscus can be used as heat stress adaptive tool for Glycine max L. and Helianthus annuus L. J. Appl. Bot. Food Qual. 93, 112–125. doi: 10.5073/Jabfq.2020.093.014
Ismail, I., Hussain, A., Mehmood, A., Qadir, M., Husna, H., Iqbal, A., et al. (2020c). Thermal stress alleviating potential of endophytic fungus rhizopus oryzae inoculated to sunflower (Helianthus annuus L.) and Soybean (Glycine max L.). Pak. J. Bot. 52, 1857–1865. doi: 10.30848/Pjb2020-5(10)
Issa, A., Esmaeel, Q., Sanchez, L., Courteaux, B., Guise, J.-F., Gibon, Y., et al. (2018). Impacts of Paraburkholderia phytofirmans strain PsJN on tomato (Lycopersicon esculentum L.) under high temperature. Front. Plant Sci. 9:1397. doi: 10.3389/fpls.2018.01397
Jagadish, S. K., Way, D. A., and Sharkey, T. D. (2021). Plant heat stress: concepts directing future research. Plant Cell Environ. 44, 1992–2005. doi: 10.1111/pce.14050
Kaur, G., and Asthir, B. (2015). Proline: a key player in plant abiotic stress tolerance. Biol. Plant. 59, 609–619.
Kawano, T., Sahashi, N., Takahashi, K., Uozumi, N., and Muto, S. (1998). Salicylic acid induces extracellular superoxide generation followed by an increase in cytosolic calcium ion in tobacco suspension culture: the earliest events in salicylic acid signal transduction. Plant Cell Physiol. 39, 721–730.
Khan, A. L., Hamayun, M., Radhakrishnan, R., Waqas, M., Kang, S.-M., Kim, Y.-H., et al. (2012a). Mutualistic association of Paecilomyces formosus LHL10 offers thermotolerance to Cucumis sativus. Antonie van Leeuwenhoek 101, 267–279. doi: 10.1007/s10482-011-9630-x
Khan, A. L., Hamayun, M., Waqas, M., Kang, S. M., Kim, Y. H., Kim, D. H., et al. (2012b). Exophiala sp.LHL08 association gives heat stress tolerance by avoiding oxidative damage to cucumber plants. Biol. Fertil. Soils 48, 519–529. doi: 10.1007/s00374-011-0649-y
Khan, A. L., Kang, S. M., Dhakal, K. H., Hussain, J., Adnan, M., Kim, J. G., et al. (2013). Flavonoids and amino acid regulation in Capsicum annuum L. by endophytic fungi under different heat stress regimes. Sci. Hortic. 155, 1–7. doi: 10.1016/j.scienta.2013.02.028
Khan, A. L., Waqas, M., and Lee, I.-J. (2015). Resilience of Penicillium resedanum LK6 and exogenous gibberellin in improving Capsicum annuum growth under abiotic stresses. J. Plant Res. 128, 259–268. doi: 10.1007/s10265-014-0688-1
Khan, M. A., Asaf, S., Khan, A. L., Jan, R., Kang, S.-M., Kim, K.-M., et al. (2020a). Extending thermotolerance to tomato seedlings by inoculation with SA1 isolate of Bacillus cereus and comparison with exogenous humic acid application. PLoS One 15:e0232228. doi: 10.1371/journal.pone.0232228
Khan, M. A., Asaf, S., Khan, A. L., Jan, R., Kang, S. M., Kim, K. M., et al. (2020b). Thermotolerance effect of plant growth-promoting Bacillus cereus SA1 on soybean during heat stress. BMC Microbiol. 20:175. doi: 10.1186/s12866-020-01822-7
Khan, M. I. R., Fatma, M., Per, T. S., Anjum, N. A., and Khan, N. A. (2015). Salicylic acid-induced abiotic stress tolerance and underlying mechanisms in plants. Front. Plant Sci. 6:462. doi: 10.3389/fpls.2015.00462
Kiers, E. T., Palmer, T. M., Ives, A. R., Bruno, J. F., and Bronstein, J. L. (2010). Mutualisms in a changing world: an evolutionary perspective. Ecol. Lett. 13, 1459–1474. doi: 10.1111/j.1461-0248.2010.01538.x
Kinnersley, A. M., and Turano, F. J. (2000). Gamma aminobutyric acid (GABA) and plant responses to stress. Crit. Rev. Plant Sci. 19, 479–509.
Knight, H., and Knight, M. R. (2001). Abiotic stress signalling pathways: specificity and cross-talk. Trends Plant Sci. 6, 262–267. doi: 10.1016/s1360-1385(01)01946-x
Kumar, A., Singh, S., Gaurav, A. K., Srivastava, S., and Verma, J. P. (2020). Plant growth-promoting bacteria: biological tools for the mitigation of salinity stress in plants. Front. Microbiol. 11:1216. doi: 10.3389/fmicb.2020.01216
Kumar, A., and Verma, J. P. (2018). Does plant—microbe interaction confer stress tolerance in plants: a review? Microbiol. Res. 207, 41–52. doi: 10.1016/j.micres.2017.11.004
Kuppardt, A., Fester, T., Härtig, C., and Chatzinotas, A. (2018). Rhizosphere protists change metabolite profiles in Zea mays. Front. Microbiol. 9:857. doi: 10.3389/fmicb.2018.00857
Kuźniak, E., Kopczewski, T., and Chojak-Koźniewska, J. (2017). “Ascorbate-glutathione cycle and biotic stress tolerance in plants,” in Ascorbic Acid in Plant Growth, Development and Stress Tolerance, eds M. A. Hossain, S. Munné-Bosch, D. J. Burritt, P. Diaz-Vivancos, M. Fujita, and A. L. Springer (Berlin: Springer), 201–231.
Lajeunesse, M. J., and Forbes, M. R. (2003). Variable reporting and quantitative reviews: acomparison of three meta-analytical techniques. Ecol. Lett. 6, 448–454. doi: 10.1046/j.1461-0248.2003.00448.x
Lämke, J., Brzezinka, K., Altmann, S., and Bäurle, I. (2016). A hit-and-run heat shock factor governs sustained histone methylation and transcriptional stress memory. EMBO J. 35, 162–175. doi: 10.15252/embj.201592593
Larkindale, J., and Huang, B. (2005). Effects of abscisic acid, salicylic acid, ethylene and hydrogen peroxide in thermotolerance and recovery for creeping bentgrass. Plant Growth Regul. 47, 17–28.
Larkindale, J., and Knight, M. R. (2002). Protection against heat stress-induced oxidative damage in Arabidopsis involves calcium, abscisic acid, ethylene, and salicylic acid. Plant Physiol. 128, 682–695. doi: 10.1104/pp.010320
Lee, S.-M., and Ryu, C.-M. (2021). Algae as new kids in the beneficial plant microbiome. Front. Plant Sci. 12:91. doi: 10.3389/fpls.2021.599742
Li, N., Euring, D., Cha, J. Y., Lin, Z., Lu, M., Huang, L.-J., et al. (2021). Plant hormone-mediated regulation of heat tolerance in response to global climate change. Front. Plant Sci. 11:627969. doi: 10.3389/fpls.2020.627969
Li, X., Zhao, C., Zhang, T., Wang, G., Amombo, E., Xie, Y., et al. (2021). Exogenous Aspergillus aculeatus enhances drought and heat tolerance of perennial ryegrass. Front. Microbiol. 12:593722. doi: 10.3389/fmicb.2021.593722
Ling, Y., Serrano, N., Gao, G., Atia, M., Mokhtar, M., Woo, Y. H., et al. (2018). Thermopriming triggers splicing memory in Arabidopsis. J. Exp. Bot. 69, 2659–2675. doi: 10.1093/jxb/ery062
Liu, H. C., Lämke, J., Lin, S. Y., Hung, M. J., Liu, K. M., Charng, Y. Y., et al. (2018). Distinct heat shock factors and chromatin modifications mediate the organ-autonomous transcriptional memory of heat stress. Plant J. 95, 401–413. doi: 10.1111/tpj.13958
Liu, X., and Hou, X. (2018). Antagonistic regulation of ABA and GA in metabolism and signaling pathways. Front. Plant Sci. 9:251. doi: 10.3389/fpls.2018.00251
Lorenzo, O., Chico, J. M., Saénchez-Serrano, J. J., and Solano, R. (2004). JASMONATE-INSENSITIVE1 encodes a MYC transcription factor essential to discriminate between different jasmonate-regulated defense responses in Arabidopsis. Plant Cell 16, 1938–1950. doi: 10.1105/tpc.022319
Maestri, E., Klueva, N., Perrotta, C., Gulli, M., Nguyen, H. T., and Marmiroli, N. (2002). Molecular genetics of heat tolerance and heat shock proteins in cereals. Plant Mol. Biol. 48, 667–681. doi: 10.1023/a:1014826730024
Malinowski, D. P., and Belesky, D. P. (2000). Adaptation of endophyte-infected cool-season grasses to environmental stresses: mechanisms of drought and mineral stress tolerance. Crop Sci. 40, 923–940. doi: 10.2135/cropsci2000.404923x
Marquez, L. M., Redman, R. S., Rodriguez, R. J., and Roossinck, M. J. (2007). A virus in a fungus in a plant: three-way symbiosis required for thermal tolerance. Science 315, 513–515. doi: 10.1126/science.1136237
Martin, C. A., and Stutz, J. C. (2004). Interactive effects of temperature and arbuscular mycorrhizal fungi on growth, P uptake and root respiration of Capsicum annuum L. Mycorrhiza 14, 241–244. doi: 10.1007/s00572-003-0261-6
Mathur, S., and Jajoo, A. (2020). Arbuscular mycorrhizal fungi protects maize plants from high temperature stress by regulating photosystem II heterogeneity. Ind. Crops Product. 143:111934. doi: 10.1016/j.indcrop.2019.111934
Maya, M. A., and Matsubara, Y. (2013). Influence of arbuscular mycorrhiza on the growth and antioxidative activity in cyclamen under heat stress. Mycorrhiza 23, 381–390. doi: 10.1007/s00572-013-0477-z
Mayerhofer, M. S., Kernaghan, G., and Harper, K. A. (2013). The effects of fungal root endophytes on plant growth. Mycorrhiza 23, 199–128.
McGrath, J. M., and Lobell, D. B. (2013). Reduction of transpiration and altered nutrient allocation contribute to nutrient decline of crops grown in elevated CO2 concentrations. Plant Cell Environ. 36, 697–705. doi: 10.1111/pce.12007
Meena, K. K., Sorty, A. M., Bitla, U. M., Choudhary, K., Gupta, P., Pareek, A., et al. (2017). Abiotic stress responses and microbe-mediated mitigation in plants: the omics strategies. Front. Plant Sci. 8:172. doi: 10.3389/fpls.2017.00172
Mittler, R., Vanderauwera, S., Gollery, M., and Van Breusegem, F. (2004). Reactive oxygen gene network of plants. Trends Plant Sci. 9, 490–498. doi: 10.1016/j.tplants.2004.08.009
Mohi-Ud-Din, M., Siddiqui, M., Rohman, M., Jagadish, S., Ahmed, J. U., Hassan, M. M., et al. (2021). Physiological and biochemical dissection reveals a trade-off between antioxidant capacity and heat tolerance in bread wheat (Triticum aestivum L.). Antioxidants 10:351. doi: 10.3390/antiox10030351
Molina-Montenegro, M. A., Oses, R., Torres-Díaz, C., Atala, C., Zurita-Silva, A., and Ruiz-Lara, S. (2016). Root-endophytes improve the ecophysiological performance and production of an agricultural species under drought condition. AoB Plants 8:lw062. doi: 10.1093/aobpla/plw062
Mukhtar, T., Rehman, S. U., Smith, D., Sultan, T., Seleiman, M. F., Alsadon, A. A., et al. (2020). Mitigation of heat stress in Solanum lycopersicum L. by ACC-deaminase and exopolysaccharide producing Bacillus cereus: effects on biochemical profiling. Sustainability 12:2159. doi: 10.3390/su12062159
Naamala, J., and Smith, D. L. (2021). Microbial derived compounds, a step toward enhancing microbial inoculants technology for sustainable agriculture. Front. Microbiol. 12:634807. doi: 10.3389/fmicb.2021.634807
Nakamoto, H., and Vigh, L. (2007). The small heat shock proteins and their clients. Cell. Mol. Life Sci. 64, 294–306. doi: 10.1007/s00018-006-6321-2
Noctor, G., Mhamdi, A., and Foyer, C. H. (2014). The roles of reactive oxygen metabolism in drought: not so cut and dried. Plant Physiol. 164, 1636–1648. doi: 10.1104/pp.113.233478
Pareek, A., Singla, S. L., and Grover, A. (1998). Proteins alterations associated with salinity, desiccation, high and low temperature stresses and abscisic acid application in seedlings of Pusa 169, a high-yielding rice (Oryza sativa L.) cultivar. Curr. Sci. 75, 1023–1035.
Porras-Alfaro, A., and Bayman, P. (2011). Hidden fungi, emergent properties: endophytes and microbiomes. Annu. Rev. Phytopathol. 49, 291–315. doi: 10.1146/annurev-phyto-080508-081831
Quan, L. J., Zhang, B., Shi, W. W., and Li, H. Y. (2008). Hydrogen peroxide in plants: a versatile molecule of the reactive oxygen species network. J. Integr. Plant Biol. 50, 2–18. doi: 10.1111/j.1744-7909.2007.00599.x
Queitsch, C., Hong, S.-W., Vierling, E., and Lindquist, S. (2000). Heat shock protein 101 plays a crucial role in thermotolerance in Arabidopsis. Plant Cell 12, 479–492. doi: 10.1105/tpc.12.4.479
R Core Team (2021). R: A Language and Environment for Statistical Computing. Vienna: R Foundation for Statistical Computing.
Rho, H., Hsieh, M., Kandel, S. L., Cantillo, J., Doty, S. L., and Kim, S.-H. (2018). Do endophytes promote growth of host plants under stress? A meta-analysis on plant stress mitigation by endophytes. Microb. Ecol. 75, 407–418. doi: 10.1007/s00248-017-1054-3
Rivero, R. M., Ruiz, J. M., Garcıa, P. C., Lopez-Lefebre, L. R., Sánchez, E., and Romero, L. (2001). Resistance to cold and heat stress: accumulation of phenolic compounds in tomato and watermelon plants. Plant Sci. 160, 315–321. doi: 10.1016/s0168-9452(00)00395-2
Rizhsky, L., Liang, H., Shuman, J., Shulaev, V., Davletova, S., and Mittler, R. (2004). When defense pathways collide. The response of Arabidopsis to a combination of drought and heat stress. Plant Physiol. 134, 1683–1696. doi: 10.1104/pp.103.033431
Rodriguez, R., White, J. Jr., Arnold, A., and Redman, A. R. A. (2009). Fungal endophytes: diversity and functional roles. New Phytol. 182, 314–330. doi: 10.1111/j.1469-8137.2009.02773.x
Roossinck, M. J. (2015). A new look at plant viruses and their potential beneficial roles in crops. Mol. Plant Pathol. 16:331. doi: 10.1111/mpp.12241
Rosenberg, M. S., Adams, D. C., and Gurevitch, J. (2000). Metawin: Statistical Software for Meta-Analysis. Version 2. Sunderland, MA: Sinauer Associates.
Sah, S. K., Reddy, K. R., and Li, J. (2016). Abscisic acid and abiotic stress tolerance in crop plants. Front. Plant Sci. 7:571. doi: 10.3389/fpls.2016.00571
Saidi, Y., Finka, A., and Goloubinoff, P. (2011). Heat perception and signalling in plants: a tortuous path to thermotolerance. New Phytol. 190, 556–565. doi: 10.1111/j.1469-8137.2010.03571.x
Sairam, R., and Tyagi, A. (2004). Physiology and molecular biology of salinity stress tolerance in plants. Curr. Sci. 86, 407–421.
Sakamoto, A., and Murata, N. (2002). The role of glycine betaine in the protection of plants from stress: clues from transgenic plants. Plant Cell Environ. 25, 163–171. doi: 10.1046/j.0016-8025.2001.00790.x
Sanders, D., Brownlee, C., and Harper, J. F. (1999). Communicating with calcium. Plant Cell 11, 691–706. doi: 10.2307/3870893
Sangamesh, M., Jambagi, S., Vasanthakumari, M., Shetty, N. J., Kolte, H., Ravikanth, G., et al. (2018). Thermotolerance of fungal endophytes isolated from plants adapted to the Thar Desert, India. Symbiosis 75, 135–147. doi: 10.1007/s13199-017-0527-y
Sarkar, J., Chakraborty, B., and Chakraborty, U. (2018). Plant growth promoting rhizobacteria protect wheat plants against temperature stress through antioxidant signalling and reducing chloroplast and membrane injury. J. Plant Growth Regul. 37, 1396–1412. doi: 10.1007/s00344-018-9789-8
Sato, S., Kamiyama, M., Iwata, T., Makita, N., Furukawa, H., and Ikeda, H. (2006). Moderate increase of mean daily temperature adversely affects fruit set of Lycopersicon esculentum by disrupting specific physiological processes in male reproductive development. Ann. Bot. 97, 731–738. doi: 10.1093/aob/mcl037
Sedaghatmehr, M., Mueller-Roeber, B., and Balazadeh, S. (2016). The plastid metalloprotease FtsH6 and small heat shock protein HSP21 jointly regulate thermomemory in Arabidopsis. Nat. Commun. 7, 1–14. doi: 10.1038/ncomms12439
Seidler, A. L., Hunter, K. E., Cheyne, S., Ghersi, D., Berlin, J. A., and Askie, L. (2019). A guide to prospective meta-analysis. BMJ 367:l5342. doi: 10.1136/bmj.l5342
Sharkey, T. D. (2005). Effects of moderate heat stress on photosynthesis: importance of thylakoid reactions, rubisco deactivation, reactive oxygen species, and thermotolerance provided by isoprene. Plant Cell Environ. 28, 269–277. doi: 10.1111/j.1365-3040.2005.01324.x
Shekhawat, K., Sheikh, A., Mariappan, K., Jalal, R., and Hirt, H. (2020). Enterobacter sp. SA187 mediates plant thermotolerance by chromatin modification of heat stress genes. bioRxiv [Preprint]. doi: 10.1101/2020.01.16.908756
Shinozaki, K., and Yamaguchi-Shinozaki, K. (2007). Gene networks involved in drought stress response and tolerance. J. Exp. Bot. 58, 221–227. doi: 10.1093/jxb/erl164
Silvente, S., Sobolev, A. P., and Lara, M. (2012). Metabolite adjustments in drought tolerant and sensitive soybean genotypes in response to water stress. PLoS One 7:e38554. doi: 10.1371/journal.pone.0038554
Simonsohn, U., Nelson, L. D., and Simmons, J. P. (2014). P-curve: a key to the file-drawer. J. Exp. Psychol. Gen. 143:534. doi: 10.1037/a0033242
Szabados, L., and Savouré, A. (2010). Proline: a multifunctional amino acid. Trends Plant Sci. 15, 89–97. doi: 10.1016/j.tplants.2009.11.009
Teixeira, E. I., Fischer, G., Van Velthuizen, H., Walter, C., and Ewert, F. (2013). Global hot-spots of heat stress on agricultural crops due to climate change. Agric. For. Meteorol. 170, 206–215. doi: 10.1016/j.agrformet.2011.09.002
Tian, Z. P., Huang, B. R., and Belanger, F. C. (2015). Effects of epichloe festucae fungal endophyte infection on drought and heat stress responses of strong creeping red fescue. J. Am. Soc. Hortic. Sci. 140, 257–264. doi: 10.21273/Jashs.140.3.257
Tsukagoshi, H., Busch, W., and Benfey, P. N. (2010). Transcriptional regulation of ROS controls transition from proliferation to differentiation in the root. Cell 143, 606–616. doi: 10.1016/j.cell.2010.10.020
Turner, T. R., James, E. K., and Poole, P. S. (2013). The plant microbiome. Genome Biol. 14, 1–10. doi: 10.3390/microorganisms9010188
Ullah, A., Bano, A., and Janjua, H. T. (2020). “Microbial secondary metabolites and defense of plant stress,” in Microbial Services in Restoration Ecology, eds J. Shankar Singh and S. R. Vimal (Amsterdam: Elsevier), 37–46. doi: 10.1016/b978-0-12-819978-7.00003-8
Velikova, V., Edreva, A., and Loreto, F. (2004). Endogenous isoprene protects Phragmites australis leaves against singlet oxygen. Physiol. Plant. 122, 219–225. doi: 10.1111/j.0031-9317.2004.00392.x
Veresoglou, S. D., Menexes, G., and Rillig, M. C. (2012). Do arbuscular mycor- rhizal fungi affect the allometric partition of host plant biomass to shoots and roots? A meta-analysis of studies from1990 to 2010. Mycorrhiza 22, 227–235. doi: 10.1007/s00572-011-0398-7
Verma, V., Ravindran, P., and Kumar, P. P. (2016). Plant hormone-mediated regulation of stress responses. BMC Plant Biol. 16:86. doi: 10.1186/s12870-016-0771-y
Veroniki, A. A., Jackson, D., Viechtbauer, W., Bender, R., Bowden, J., Knapp, G., et al. (2016). Methods to estimate the between-study variance and its uncertainty in meta-analysis. Res. Synthesis Methods 7, 55–79. doi: 10.1002/jrsm.1164
Wahid, A. (2007). Physiological implications of metabolite biosynthesis for net assimilation and heat-stress tolerance of sugarcane (Saccharum officinarum) sprouts. J. Plant Res. 120, 219–228. doi: 10.1007/s10265-006-0040-5
Wahid, A., and Close, T. (2007). Expression of dehydrins under heat stress and their relationship with water relations of sugarcane leaves. Biol. Plant. 51, 104–109. doi: 10.1007/s10535-007-0021-0
Wahid, A., Gelani, S., Ashraf, M., and Foolad, M. R. (2007). Heat tolerance in plants: an overview. Environ. Exp. Bot. 61, 199–223. doi: 10.1016/j.envexpbot.2007.05.011
Wäli, P., Helander, M., Nissinen, O., Lehtonen, P., and Saikkonen, K. (2008). Endophyte infection, nutrient status of the soil and duration of snow cover influence the performance of meadow fescue in sub-artic conditions. Grass Forage Sci. 63, 324–330. doi: 10.1111/j.1365-2494.2008.00639.x
Wang, G., Zhang, X., Li, F., Luo, Y., and Wang, W. (2010). Overaccumulation of glycine betaine enhances tolerance to drought and heat stress in wheat leaves in the protection of photosynthesis. Photosynthetica 48, 117–126.
Wang, K., Zhang, X., Goatley, M., and Ervin, E. (2014). Heat shock proteins in relation to heat stress tolerance of creeping bentgrass at different N levels. PLoS One 9:e102914. doi: 10.1371/journal.pone.0102914
Wang, L.-J., Fan, L., Loescher, W., Duan, W., Liu, G.-J., Cheng, J.-S., et al. (2010). Salicylic acid alleviates decreases in photosynthesis under heat stress and accelerates recovery in grapevine leaves. BMC Plant Biol. 10:34. doi: 10.1186/1471-2229-10-34
Wang, L.-J., and Li, S.-H. (2006). Salicylic acid-induced heat or cold tolerance in relation to Ca2+ homeostasis and antioxidant systems in young grape plants. Plant Sci. 170, 685–694.
Wani, A. B., Chadar, H., Wani, A. H., Singh, S., and Upadhyay, N. (2017). Salicylic acid to decrease plant stress. Environ. Chem. Lett. 15, 101–123.
Waqas, M., Khan, A. L., Kamran, M., Hamayun, M., Kang, S.-M., Kim, Y.-H., et al. (2012). Endophytic fungi produce gibberellins and indoleacetic acid and promotes host-plant growth during stress. Molecules 17, 10754–10773. doi: 10.3390/molecules170910754
Waqas, M., Khan, A. L., Shahzad, R., Ullah, I., Khan, A. R., and Lee, I. J. (2015). Mutualistic fungal endophytes produce phytohormones and organic acids that promote japonica rice plant growth under prolonged heat stress. J. Zhejiang Univ. Sci. B 16, 1011–1018. doi: 10.1631/jzus.B1500081
Xie, H.-T., Wan, Z.-Y., Li, S., and Zhang, Y. (2014). Spatiotemporal production of reactive oxygen species by NADPH oxidase is critical for tapetal programmed cell death and pollen development in Arabidopsis. Plant Cell 26, 2007–2023. doi: 10.1105/tpc.114.125427
Xu, Z.-S., Li, Z.-Y., Chen, Y., Chen, M., Li, L.-C., and Ma, Y.-Z. (2012). Heat shock protein 90 in plants: molecular mechanisms and roles in stress responses. Int. J. Mol. Sci. 13, 15706–15723. doi: 10.3390/ijms131215706
Yeasmin, R., Bonser, S. P., Motoki, S., and Nishihara, E. (2019). Arbuscular mycorrhiza influences growth and nutrient uptake of Asparagus (Asparagus officinalis L.) under heat stress. Hortscience 54, 846–850. doi: 10.21273/Hortsci13587-18
Yu, X., Pasternak, T., Eiblmeier, M., Ditengou, F., Kochersperger, P., Sun, J., et al. (2013). Plastid-localized glutathione reductase2–regulated glutathione redox status is essential for Arabidopsis root apical meristem maintenance. Plant Cell 25, 4451–4468. doi: 10.1105/tpc.113.117028
Zafra, A., Rodríguez-García, M. I., and de Dios Alché, J. (2010). Cellular localization of ROS and NO in olive reproductive tissues during flower development. BMC Plant Biol. 10:36. doi: 10.1186/1471-2229-10-36
Zaynab, M., Fatima, M., Abbas, S., Sharif, Y., Umair, M., Zafar, M. H., et al. (2018). Role of secondary metabolites in plant defense against pathogens. Microb. Pathog. 124, 198–202. doi: 10.1016/j.micpath.2018.08.034
Zhang, H. J., Zhang, N., Yang, R. C., Wang, L., Sun, Q. Q., Li, D. B., et al. (2014). Melatonin promotes seed germination under high salinity by regulating antioxidant systems, ABA and GA 4 interaction in cucumber (Cucumis sativus L.). J. Pineal Res. 57, 269–279. doi: 10.1111/jpi.12167
Zhao, J., Lu, Z., Wang, L., and Jin, B. (2021). Plant responses to heat stress: physiology, transcription, noncoding RNAs, and epigenetics. Int. J. Mol. Sci. 22:117. doi: 10.3390/ijms22010117
Zhou, L., He, H., Liu, R., Han, Q., Shou, H., and Liu, B. (2014). Overexpression of GmAKT2 potassium channel enhances resistance to soybean mosaic virus. BMC Plant Biol. 14:154. doi: 10.1186/1471-2229-14-154
Zhou, W.-N., White, J. F., Soares, M. A., Torres, M. S., Zhou, Z.-P., and Li, H.-Y. (2015). Diversity of fungi associated with plants growing in geothermal ecosystems and evaluation of their capacities to enhance thermotolerance of host plants. J. Plant Interact. 10, 305–314.
Keywords: antioxidant, heat stress, meta-analysis, plant-microbe interaction, plant physiology, symbiosis
Citation: Dastogeer KMG, Zahan MI, Rhaman MS, Sarker MSA and Chakraborty A (2022) Microbe-Mediated Thermotolerance in Plants and Pertinent Mechanisms- A Meta-Analysis and Review. Front. Microbiol. 13:833566. doi: 10.3389/fmicb.2022.833566
Received: 11 December 2021; Accepted: 04 February 2022;
Published: 07 March 2022.
Edited by:
Marco Scortichini, Council for Agricultural and Economics Research (CREA), ItalyReviewed by:
Laura Bertini, University of Tuscia, ItalyCopyright © 2022 Dastogeer, Zahan, Rhaman, Sarker and Chakraborty. This is an open-access article distributed under the terms of the Creative Commons Attribution License (CC BY). The use, distribution or reproduction in other forums is permitted, provided the original author(s) and the copyright owner(s) are credited and that the original publication in this journal is cited, in accordance with accepted academic practice. No use, distribution or reproduction is permitted which does not comply with these terms.
*Correspondence: Khondoker M. G. Dastogeer, ZGFzdG9nZWVyLnBwYXRoQGJhdS5lZHUuYmQ=
Disclaimer: All claims expressed in this article are solely those of the authors and do not necessarily represent those of their affiliated organizations, or those of the publisher, the editors and the reviewers. Any product that may be evaluated in this article or claim that may be made by its manufacturer is not guaranteed or endorsed by the publisher.
Research integrity at Frontiers
Learn more about the work of our research integrity team to safeguard the quality of each article we publish.