- 1Food Microbiology, Wageningen University and Research, Wageningen, Netherlands
- 2Laboratory of Biochemistry, Wageningen University and Research, Wageningen, Netherlands
Lactococcus cremoris and L. lactis are well known for their occurrence and applications in dairy fermentations, but their niche extends to a range of natural and food production environments. L. cremoris and L. lactis produce MKs (vitamin K2), mainly as the long-chain forms represented by MK-9 and MK-8, and a detectable number of short-chain forms represented by MK-3. The physiological significance of the different MK forms in the lifestyle of these bacterial species has not been investigated extensively. In this study, we used L. cremoris MG1363 to construct mutants producing different MK profiles by deletion of genes encoding (i) a menaquinone-specific isochorismate synthase, (ii) a geranyltranstransferase, and (iii) a prenyl diphosphate synthase. These gene deletions resulted in (i) a non-MK producer (ΔmenF), (ii) a presumed MK-1 producer (ΔispA), and (iii) an MK-3 producer (Δllmg_0196), respectively. By examining the phenotypes of the MG1363 wildtype strain and respective mutants, including biomass accumulation, stationary phase survival, oxygen consumption, primary metabolites, azo dye/copper reduction, and proteomes, under aerobic, anaerobic, and respiration-permissive conditions, we could infer that short-chain MKs like MK-1 and MK-3 are preferred to mediate extracellular electron transfer and reaction with extracellular oxygen, while the long-chain MKs like MK-9 and MK-8 are more efficient in aerobic respiratory electron transport chain. The different electron transfer routes mediated by short-chain and long-chain MKs likely support growth and survival of L. cremoris in a range of (transiently) anaerobic and aerobic niches including food fermentations, highlighting the physiological significance of diverse MKs in L. cremoris.
Introduction
Lactococcus cremoris [previous known as Lactococcus lactis ssp. cremoris (Li et al., 2021)] and L. lactis are lactic acid bacteria (LAB) that play important roles in food fermentation processes, especially in the manufacturing of fermented dairy products: they are the main constituent of various dairy starter cultures used all over the world for the production of cheese, buttermilk, and sour cream (Cavanagh et al., 2015). The essential involvement of L. cremoris and L. lactis in the fermentation of food raw materials highlights the interest in understanding the physiology and lifestyle of this bacterium.
Although L. cremoris and L. lactis are best known for their application and occurrence in dairy products, they are found in a diverse range of natural niches such as the gastrointestinal tract of particular fish species and various plant materials (Cavanagh et al., 2015). In fact, the dairy isolates are believed to originate from plant isolates which successfully adapted to thrive in the dairy environment (Kleerebezem et al., 2020). It has been suggested that the niche adaptation history is reflected in the lifestyle and adaptability of L. cremoris and L. lactis in different environmental conditions. For instance, although L. cremoris is classified as a facultative anaerobe with a fermentative metabolism, evidence has also been provided that in presence of oxygen and exogenous supplemented of heme, the organism can switch to aerobic respiration, a process enabled by the menaquinones (MKs, also referred to as vitamin K2) produced by L. cremoris as electron carriers (Duwat et al., 2001; Brooijmans et al., 2009c). Notably, many other LAB species commonly applied in food fermentation processes, e.g., Lactiplantibacillus plantarum [previously referred to as Lactobacillus plantarum (Zheng et al., 2020)], have lost the ability to produce MKs (Pedersen et al., 2012). The ecological and physiological significance of MKs and their contribution to the successful applications of L. cremoris in food fermentations is an interesting, but under-explored topic.
Menaquinones accumulate in the cell membrane of producing bacteria (Walther et al., 2013). All MKs share a naphthoquinone structure but the variants differ in the length of sidechains consisting of isoprenyl units (Lenaz and Genova, 2013). In the abbreviation MK-n, the number of isoprenyl units in the side chain is indicated by n. The variants of MKs produced in different bacterial species are distinct and have even been proposed as taxonomic markers (Collins and Jones, 1981). For example, Bacillus subtilis produces MK-7, Escherichia coli produces mainly MK-8, and Propionibacterium freudenreichii produces MK-9 (4H) (Walther et al., 2013). L. cremoris and L. lactis are known to produce a range of MK variants, including MK-3, MK-5 through MK-10, among which MK-8 and MK-9 are the major forms (Brooijmans et al., 2009c; Liu et al., 2019).
Menaquinone is the sole quinone that shuttles electrons in the respiratory electron transport chain (ETC) in Gram-positive bacteria, essential for both aerobic and anaerobic respiration processes (Kurosu and Begari, 2010). For respiring Gram-positive bacteria, MK is essential for growth and survival, and enzymes in the biosynthesis pathway in pathogens have therefore been considered as targets for developing antibacterial agents. As mentioned, functional respiration in response to oxygen and heme supplementation has been observed in L. cremoris strains, where the NADH dehydrogenase complex, MKs, and the bd-type cytochrome complex (where heme is required as a cofactor) together form a functional ETC in the cell membrane (Brooijmans et al., 2009c). L. cremoris is not able to synthesize heme, thus heme has to be supplied exogenously to allow aerobic respiration in L. cremoris (Pedersen et al., 2012). Nevertheless, heme is not considered to be present in the dairy environment, and aerobic respiration is not a common or essential metabolic mode for L. cremoris in food fermentations. In fact, in the production processes of fermented dairy product, L. cremoris is considered to mainly encounter anaerobic conditions during the fermentation. However, L. cremoris may be exposed to oxygen during starter culture production and during early stages in cheese production, i.e., aerobic conditions in the absence of heme (Cretenet et al., 2014).
Under anaerobic conditions, MKs are known to endow some bacteria with the ability to utilize extracellular electron acceptors as alternatives for oxygen, e.g., nitrate or fumarate, allowing anaerobic respiration as reported for E. coli, Enterococcus faecalis, and Lb. plantarum (with exogenously supplied heme and MK) (Huycke et al., 2001; Brooijmans et al., 2009b; Kurosu and Begari, 2010). Moreover, MKs or precursors such as demethylmenaquinones (DMKs) or naphthoquinones were shown to be crucial for extracellular electron transfer (EET) in various Gram-positive bacteria species including L. cremoris, which has been shown to be able to reduce Cu2+ ions, transfer electrons to electrodes, and decolorize azo dyes conceivably via flavin-based EET (Rezaïki et al., 2008; Freguia et al., 2009; Pérez-Díaz and McFeeters, 2009; Light et al., 2018, 2019).
Under aerobic conditions, L. cremoris is known to be oxygen tolerant. (D)MKs were found to mediate reduction of exogenous oxygen to reactive oxygen species (ROS) such as superoxide in L. cremoris and E. faecalis (Huycke et al., 2001; Rezaïki et al., 2008). On the other hand, Liu et al. obtained L. cremoris vitamin K2 (MK) overproducers that showed high resistance to oxidative stress by laboratory evolution under aerobic conditions, although a direct relationship between elevated MK content and enhanced resistance to oxidative stress remains to be established (Liu et al., 2021).
Interestingly, a significant amount of MKs was found in L. cremoris stationary phase cells from different growth conditions: anaerobic/microaerophilic, aerobic, and respiration-permissive (aerobic conditions with the addition of heme) (Brooijmans et al., 2009c; Liu et al., 2019). Notably, L. cremoris produces multiple MK forms, whereas most MK producing bacteria are known to produce one specific form, or one major form with two adjacent forms in very minor amount. L. cremoris produces noticeable amounts of both short-chain MKs represented by MK-3, and long-chain MKs represented by MK-8 and MK-9, and the distribution of the short-chain and long-chain MK forms alters depending on the growth conditions (Brooijmans et al., 2009c; Walther et al., 2013; Liu et al., 2019). It remains to be elucidated whether the short-chain or long-chain forms of MKs are favored for particular functions under the different aeration conditions (anaerobic or aerobic) and metabolic modes (fermentation or respiration) that L. cremoris could be exposed to in a range of natural and food production environments, for example, in dairy fermentations.
To examine the physiological significance of the short-chain and long-chain MKs in L. cremoris, we constructed dedicated mutants using model strain L. cremoris MG1363, based on the annotation of putative genes in the MK biosynthesis pathway in L. cremoris (Figure 1; Wegmann et al., 2007; Brooijmans et al., 2009c): candidate genes encoding a menaquinone-specific isochorismate synthase (menF), a geranyltranstransferase (ispA), and a prenyl diphosphate synthase (llmg_0196) were deleted, with the prediction to create mutants producing no MK (ΔmenF), only MK-1 (ΔispA), and only MK-3 (Δllmg_0196), respectively. These mutants, together with the original strain MG1363 producing mainly MK-9 and MK-8, allowed the study of functionality of short-chain and long-chain MK forms in L. cremoris under anaerobic, aerobic, and respiration-permissive conditions, where involvement of MKs in bacterial metabolism and physiology has been proposed. Under these conditions, relevant phenotypes including MK profile, biomass accumulation, viability/survival, oxygen consumption, and electron transfer to extracellular acceptors (e.g., azo dye, Cu2+), metabolites, and proteomes were examined for strain MG1363 and mutants.
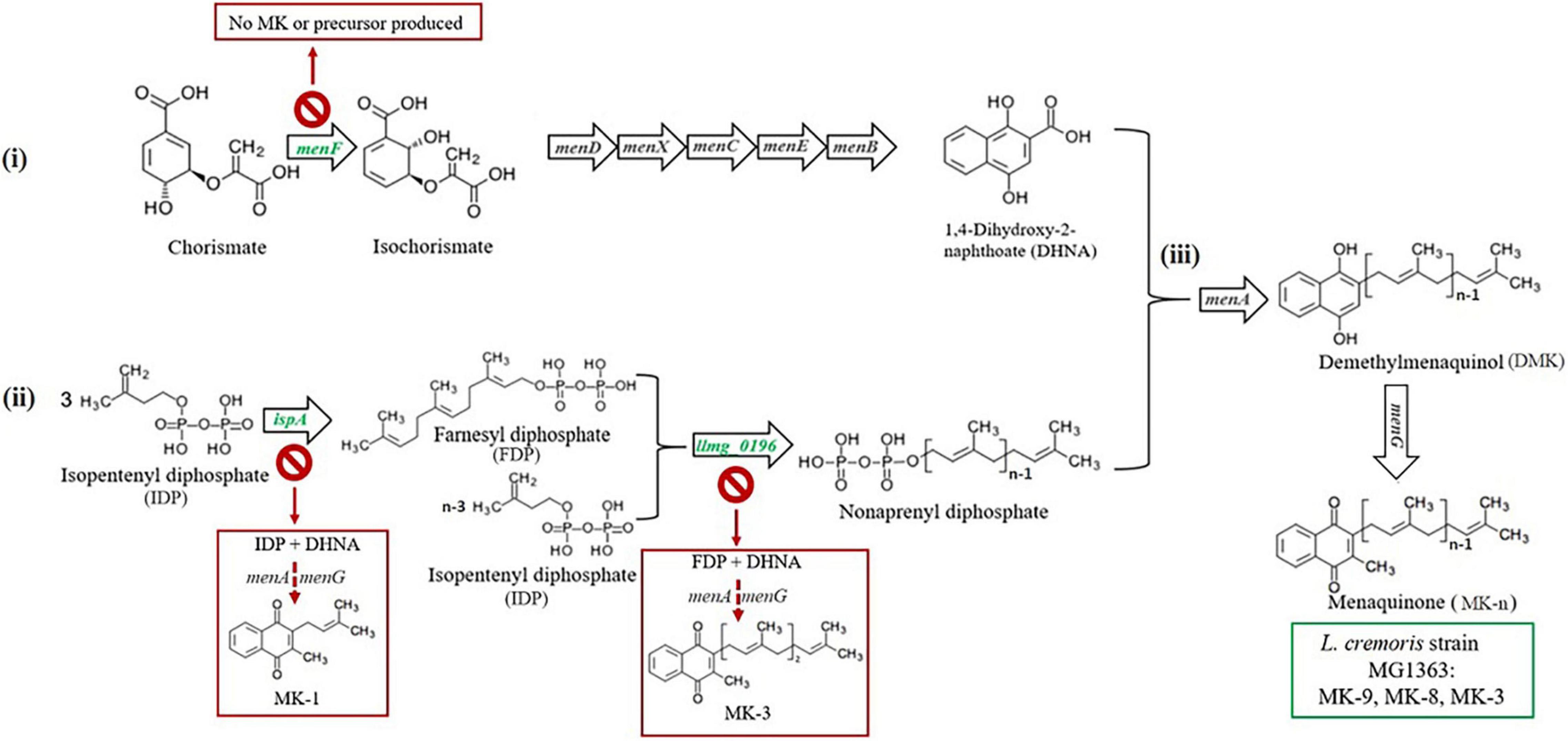
Figure 1. Predicted menaquinone biosynthesis pathway in L. cremoris strain MG1363 and mutants. MK biosynthesis in L. cremoris/L. lactis is predicted to follow a pathway consisting of three parts: (i) synthesis of the naphthoquinone structure (the head group), (ii) synthesis of the isoprenyl side chain (the tail group), and (iii) joining of the head and tail structures giving the MK. The head group synthesis starts with chorismate generated from the Shikimate pathway with the first step catalyzed by a menaquinone-specific isochorismate synthase (predicted encoding gene is menF), and the tail group synthesis starts with isopentenyl diphosphate (IPP), containing a single isoprene unit, generated from mevalonate pathway. For building the side chain, first a geranyltranstransferase (predicted encoding gene is ispA) is required to form farnesyl diphosphate (FPP) containing three isoprene units. More IPP is linked to FPP to form longer isoprene chains, and this step is catalyzed by a prenyl diphosphate synthase (predicted encoding gene is llmg_0196). The latter enzyme also is thought to play a role in determination of the length of the isoprene chain (Nagel et al., 2018; Johnston and Bulloch, 2020). Finally, the naphthoquinone head group – 1.4-dihydroxy-2-naphthoic acid (DHNA), and the polyprenyl tail group are combined by isoprenyl diphosphate:1,4-dihydroxy-2-naphthoate isoprenyltransferase to form demethylmenaquinone (DMK), which is methylated to give MK. Mainly MK-9, MK-8, and MK-3 are formed in original L. cremoris strain MG1363 (green box). Mutants ΔmenF,ΔispA, andΔllmg_0196 are expected to produce no MK, only MK-1, and only MK-3, respectively. Genes targeted in this study are shown in green color. Predicted MK production route and profiles in respective gene deletion mutants are shown in red boxes.
Materials and Methods
Strains and Conditions
Lactococcus cremoris MG1363 and derived gene deletion mutants Δllmg_0196, ΔispA, and ΔmenF were cultivated in GM17 medium [M17 broth (Difco, BD Biosciences) supplemented with 0.5% (w/v) glucose] at 30°C. Cultivation times and conditions are specified in the Results section and figure legends for each particular case. For mutants with gene complementation, 5 μg/mL erythromycin was added to the medium.
For general static cultivation, cultures were incubated statically in closed Greiner tubes in maximal volume under normal atmosphere. For specified anaerobic cultivation (AN), cultivation vessels with bacterial cultures were placed in anaerobic jars flushed with a gas mix (10% CO2, 10% H2 and 80% N2) using the anaerobic cycle program by Anoxomat (WS9000, Mart Microbiology, Netherlands) unless specified otherwise. For aerobic cultivation (AE), bacterial cultures filled up to 10% volume of Erlenmeyer flasks and were shaken at 180 rpm. Respiration-permissive cultivation conditions (RES) were the same as those applied for aerobic cultivations but with the addition of 2 μg/mL heme (hemin, Sigma).
Escherichia coli strains used in the genetic modification procedures, harboring plasmids with erythromycin resistance (Emr), were cultivated/selected in LB medium supplemented with 150 μg/mL erythromycin at 37°C. Liquid cultures were shaken at 160 rpm with 90% headspace for no longer than 16 h, agar plates were incubated at normal atmosphere for a maximal 22 h.
An overview of strains used in this study is provided in Table 1.
Genetic Modification
Plasmid Construction
Deletion of genes menF, llmg_0196, and ispA in L. cremoris MG1363 was achieved by homologous recombination. To construct the plasmids for gene deletion, the upstream and downstream homologous regions of 700–800 bp of the target genes were amplified, and desired restriction sites were introduced by PCR using primers listed in Supplementary Table 1. Phusion High-fidelity PCR kit (Thermo Fisher Scientific) was used according to the manufacturer’s instructions. Both upstream and downstream homologous regions of each target gene were inserted in plasmid pCS1966 [gift from Solem et al. (2008)] by restriction digestion and ligation following basic guidelines provided with the enzymes (Thermo Fisher Scientific). In brief, restriction site PstI was used to connect the upstream and downstream homologous regions of each target gene, HindIII and XbaI sites were used to insert the two homologous regions to the backbone of pCS1966. As a result, plasmid pYL005, pYL004, and pYL003 harboring the surrounding homologous regions of menF, llmg_0196, and ispA, respectively, were constructed.
For gene complementation, promoter regions (approximately 50 bp upstream of the start codon) and open reading frames of menF, llmg_0196, and ispA were amplified by PCR using primers listed in Supplementary Table 2. PCR products for each gene were inserted in the backbone (8301 bp fragment obtained by XbaI and BgIII digestion) of plasmid pMSP3545 [gift from Gary Dunny (Bryan et al., 2000), Addgene plasmid # 46888] using NEBuilder HiFi DNA assembly cloning kit (New England Biolabs) according to the manufacturer’s instructions. As a result, p45-menF, p45-0196, and p45-ispA containing the promoter and gene sequences of menF, llmg_0196, and ispA, respectively, were constructed.
Assembled plasmids were first transformed into E. coli competent cells Mix & Go Zymo 5α (Zymo Research) according to product manuals, and transformed candidates were made into liquid culture for plasmid isolation. Assembled plasmids were checked for correctness by restriction digestion analysis after propagation in transformed E. coli. The correctness of PCR amplified sequences was checked by Sanger sequencing (BaseClear, Leiden, Netherlands).
An overview of plasmids used in this study is provided in Table 1.
Transformation and Homologous Recombination in L. cremoris
For gene deletion, plasmid pYL005, pYL004, and pYL003 were separately transformed into MG1363 with the protocol described by Holo and Nes (1989) with the following modifications: MG1363 was cultured in SMGG media (M17, 0.5 M sucrose, 0.5% glucose, and 0.5% glycine) until mid-log phase (OD600 = 0.6–0.9). An additional washing step was performed in between the original two washing steps, with 0.5 volume EDTA washing buffer (0.05 M EDTA, 0.5 M sucrose, 10% glycerol). For transformation, 500–1000 ng plasmid pYL005, pYL004, or pYL003 in volume 1–2 μL was added. Electroporation was done using the Gene Pulser Xcell Electroporation Systems (Bio-Rad) at 2500 V, 25 μF, and 200 Ω. Cells were plated on selection plates (GM17, 1.5% agar, 0.5 M sucrose and 3 μg/mL erythromycin) and were incubated at 30°C for 2–3 d until colonies emerge.
At this stage the plasmids, having no replication origin in L. cremoris, are integrated into the genome of MG1363 at either homologous region of the target genes. To eliminate the plasmid backbone and achieve gene deletion, transformants were inoculated in 2 mL SA medium (Jensen and Hammer, 1993) containing 1% glucose and incubated at 30°C overnight. Then they were diluted 10× in SA (1% glucose) medium and incubated at 30°C for 6 h. The culture was then plated on SA (1% glucose) agar plates supplemented with 10 μg/mL 5-Fluoroorotate (Sigma). Plates were incubated at 30°C until colonies emerge. Due to the oroP gene on the plasmid of pCS1966, the presence of 5-fluoroorotate selects for mutants that have eliminated the plasmid backbone. From these mutants, the ones with gene deletion were selected by PCR (DreamTaq DNA polymerase, Thermo Fisher Scientific) using the primers listed in Supplementary Table 3.
For gene complementation, p45-menF, p45-0196, and p45-ispA were transformed into mutants ΔmenF, ΔispA, and llmg_0196, respectively, with the electroporation protocol described above. Transformants were checked by PCR (DreamTaq DNA polymerase, Thermo Fisher Scientific) using the primers listed in Supplementary Table 2.
Menaquinone Analysis
Menaquinones were extracted from cells cultivated in GM17 media under indicated conditions for 16–18 h at 30°C as described previously (Liu et al., 2019). Briefly, the biomass was first treated with lysozyme and then MKs were extracted with organic solvent n-hexane and eventually dissolved in iso-propanol. All samples were diluted in methanol and subjected to analysis in the ultra-performance liquid chromatography (Thermo Scientific Vanquish) coupled with mass spectrometry (Thermo Q-Exactive hybrid quadrupole-Orbitrap) (UPLC–MS), exactly as described in Liu et al. (2021). Calculations from analytical standards were performed as described previously (Liu et al., 2019).
Biomass Quantification
Strains were cultivated in GM17 medium under indicated conditions for 16–18 h at 30°C before biomass quantification. For cell dry weight (CDW) determination, PBS washed cell pellets from bacterial cultures were kept at 80°C for 48–72 h to evaporate water content. The dried biomass was then weighed. For optical density (OD) determination, OD600 of cell cultures was measured by a spectrophotometer (600 nm; path length 10 mm).
Viable Cell Count Enumeration
All strains were cultivated in GM17 media under indicated conditions at 30°C, culturability was determined at 24, 48, and 72 h. All strains were inoculated at 106 CFU/mL at 0 h. Bacterial cultures were subjected to serial dilution series in 96-well plates using PBS. Each dilution was spotted (10 μL) in triplicate on GM17 agar plates. Agar plates were incubated at 30°C for 24 h under microaerobic conditions before colonies were counted.
Oxygen Consumption Rate Analysis
The measurement and calculation of oxygen consumption rate in L. cremoris were performed exactly as described previously (Liu et al., 2021). For AE test, cells were obtained from overnight culture in GM17 media under anaerobic conditions. For RES test, cells were obtained from overnight culture in GM17 media supplemented with 2 μg/ml heme under anaerobic conditions. PBS-washed cells were suspended in air-saturated PBS with standardized OD values for oxygen consumption test at room temperature. Reaction was initiated by adding 1% glucose. The oxygen concentration in the bacterial suspension was followed by a Microx 4 oxygen meter (PreSens, Germany) every minute. The linear part of oxygen concentration – time correlation was used to derive the oxygen consumption rate (the absolute value of the slope).
Decolorization of Azo Dyes
Most azo dyes are not permeable to the cell membrane, and get decolorized upon reduction (Fang et al., 2019). Therefore, here we use the decolorization of an azo dye as an indicator for EET.
Strains were all cultivated under anaerobic conditions overnight in GM17 to obtain biomass for oxygen consumption analysis. The cells were harvested and washed in PBS once, and OD600 was standardized to 1 in PBS. Azo dye Reactive Black 5 (Sigma) was added to the cell suspension at a final concentration of 0.005%. The infusion bottles were flushed with N2 gas for 2 min to ensure an anaerobic environment. Reaction was at 30°C and was initiated by adding glucose to a final concentration of 1%. OD of cell-free supernatant was followed for 4 h with 1 h interval at 595 nm. The OD595 at time 0 h was regarded as 100% color intensity. The absolute value of the initial slope of the linear correlation between color intensity and time for each measurement was taken as the absolute azo dye decolorization rate. Thereafter, a relative azo dye reduction rate was calculated by comparing the absolute decolorization rate among the strains, setting the highest absolute decolorization rate as 100%.
Reduction of Copper Ions
The reduction of Cu2+ was measured indirectly in L. cremoris. When Cu2+ is reduced to the toxic species Cu+, growth of L. lactis was shown to be inhibited (Abicht et al., 2013). Therefore, inhibition of growth of L. cremoris was used as an indication of Cu2+ reduction.
Strains were cultivated statically in GM17 overnight, and then the cultures were diluted to an OD600 value of 0.1 in fresh GM17. For Cu2+ reduction tests, 300 nM CuCl2 was added to the media, and for growth controls CuCl2 was not added. For anaerobic cultivation, aliquots of 450 μL were brought into 100-well Honeycomb microplates (Thermo Fisher Scientific) and incubated at 30°C for 48 h in Bioscreen C (Thermo Fisher Scientific), where the OD600 was measured at 1-h intervals. For aerobic cultivation, aliquots of 350 μL were transferred to microplates which were subsequently incubated with continuous shaking at medium intensity. For respiration-permissive conditions, cultures were incubated the same way as for aerobic conditions, albeit with the addition of 2 μg/mL heme (hemin, Sigma).
The time to reach (TTR) OD600 value 0.4 was used as a measurement of growth; the factor difference between TTRs in the test of 300 nM CuCl2 and in the growth control without CuCl2 was eventually used as the indicator of Cu2+ reduction-induced growth inhibition/toxicity.
Metabolite Analysis
Metabolites were analyzed from the supernatant of cell cultures incubated in GM17 at 30°C for 16–18 h under indicated conditions. Metabolites including lactate, formate, acetate, acetoin, ethanol, and succinate were measured in cell-free supernatant from overnight cultures by high-performance liquid chromatography (HPLC) analysis exactly as described previously (Liu et al., 2021). The amount of each metabolite measured in uncultured GM17 medium was used as level zero to calculate the production or consumption of metabolites in the bacterial cultures.
Proteomics Analysis
Cells from overnight culture (16–18 h) in GM17 media at 30°C were used for proteomics analysis. For each strain and condition combination, samples were collected from three independent experiments. Sample preparation, LCMS analysis, and data processing were performed exactly as described previously (Liu et al., 2021). The proteome of L. cremoris MG1363 (UniProt ID UP000000364) was used as the protein database. The mass spectrometry proteomics data have been deposited to the ProteomeXchange Consortium via the PRIDE (Vizcaíno et al., 2016) partner repository with the dataset identifier PXD030833.
Data Analysis
Statistical significance analysis was performed in JASP (0.11.1) (Love et al., 2019) using two-way analysis of variance (ANOVA). Post hoc multiple comparisons were conducted using Tukey’s test (2-sided) and in all cases the control group was L. cremoris MG1363 (*p < 0.05).
Results
Selection of Targeted Genes
The MK biosynthesis pathway in L. cremoris MG1363 was predicted based on information retrieved from KEGG (Kanehisa and Goto, 2000) and the genome of L. cremoris MG1363 (Wegmann et al., 2007) and homology searches based on sequences from other bacteria with experimentally confirmed functions (Figure 1). To obtain mutants of L. cremoris MG1363 with different MK profiles, several genes were selected and subsequently targeted for gene deletion procedures. For the mutant producing no MK at all, it was decided that the gene involved in the first step of MK-specific synthesis pathway would be the target, to eliminate possible interference of intermediate products on the mutant phenotype (Figure 1). We used BLAST to identify MenF homologs in the microbial protein database (Joint Genome Institute) (Chen et al., 2019). Gene product of menF (llmg_1828) in L. cremoris MG1363 was identified with 26% identity with MenF protein sequence (Uniprot ID: P38051) from E. coli K12, for which the function has been confirmed (Daruwala et al., 1996), and was chosen as the first target gene to delete in strain MG1363. For the mutant producing short-chain MKs, genes encoding the geranyltranstransferase and prenyl diphosphate synthase were the targets. Combining previous knowledge (Kobayashi et al., 2003), sequences of genes hepT (P31114), hepS (P31112), and yqiD (P54383) from Bacillus subtilis 168 were used to search for homologs in L. cremoris MG1363 using BLAST. No sequences similar to B. subtilis hepS were found in L. cremoris MG1363. Moreover, ispB (llmg_1110), llmg_0196, and ispA (llmg_1689) in L. cremoris MG1363 were identified with 34, 31, and 35% identity to B. subtilis hepT, respectively. Finally, ispB (llmg_1110), llmg_0196, and ispA (llmg_1689) were identified with 30, 28, and 45% identity to B. subtilis yqiD, respectively. Genes encoding ispB (llmg_1110), llmg_0196, and ispA (llmg_1689) were all chosen as the target genes for modifying MK side chains to obtain mutants producing only MK-3 or MK-1 (Figure 1). The ispB (llmg_1110) deletion mutant did not show any alteration in MK profile compared to MG1363 and will not be discussed in further detail in this study.
Menaquinone Profiles in Mutants ΔmenF, ΔispA, and Δllmg_0196
First, we evaluated the gene deletion mutants derived from L. cremoris strain MG1363, by examining whether the MK profiles of the respective mutants were as predicted in silico. Wildtype strain MG1363 is known to produce mainly MK-8 and MK-9, but also MK-3 and a minor amount of other forms. The three gene deletion mutants were predicted to produce either no MK (ΔmenF), only MK-1 (ΔispA), or only MK-3 (Δllmg_0196) based on the putative roles of respective gene products in the MK biosynthesis pathway (Figure 1). The MK profiles as well as other phenotypes of strain MG1363 and the mutants were evaluated under three cultivation conditions (Figure 2A): anaerobic, aerobic, and respiration-permissive (meaning aerobic cultivation with heme supplementation).
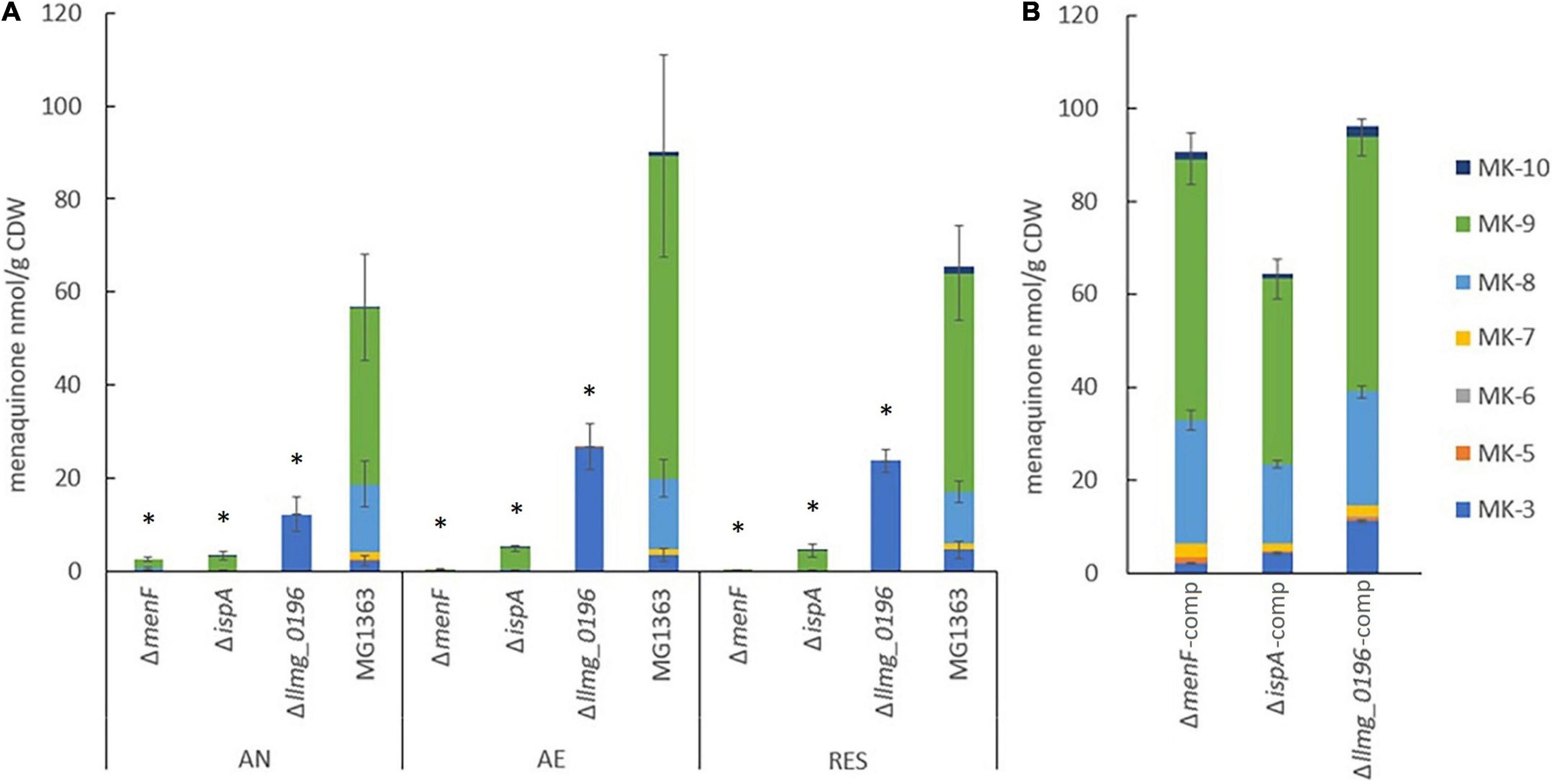
Figure 2. MK content in the biomass of L. cremoris strains. (A) MK content in strain MG1363 and mutants with gene deletions. AN, anaerobic; AE, aerobic; RES, respiration-permissive, i.e., supplement of both heme and aeration. Data from three independent experiments. (B) MK content in mutants (condition AN) with respective gene complementation. Data from four biological replicates. Error bars show SEM for MK-3, MK-8, and MK-9. *Shows significant (p < 0.05) difference to MG1363.
Under all three tested cultivation conditions, substantial amounts of MKs mainly in the forms of MK-8 and MK-9, and noticeable amounts of MK-3 could be found in the biomass of wildtype strain MG1363. In addition, minor amounts of MK7 and MK-10 were also detected (Figure 2A and Supplementary Figure 1A). The average total amount of MKs in MG1363 was 60, 90, and 70 nmol/g CDW under anaerobic, aerobic, and respiration-permissive conditions, respectively.
Δllmg_0196 produced solely MK-3 in the biomass, with the highest amount of about 25 nmol/g CDW was observed under aerobic and respiration-permissive conditions, and 12 nmol/g CDW under anaerobic conditions, confirming the hypothesis that gene llmg_0196 encodes a (nona)prenyl diphosphate synthase. The molar amount of MK-3 produced in Δllmg_0196 was higher than the MK-3 amount found in wildtype MG1363 but counted up to only one-third of the total MK amount in MG1363 (p < 0.05).
It was expected that mutant ΔmenF would not produce MKs at all, and ΔispA would produce only MK-1. Indeed, ΔmenF showed no MK production except for trace amounts of MK-9 under anaerobic conditions (2 nmol/g CDW). ΔispA showed low concentrations (3–5 nmol/g CDW) of MK-9 in all three conditions, while MK-1 was not detected, possibly due to limitations of the extraction method.
Besides examining the MK profiles in the biomass of L. cremoris, the cell-free supernatants of bacterial cultures were also examined for the presence of MKs. In all strains, no MK was detected in the culture supernatant except for Δllmg_0196 culture: a detectable amount of MK-3 was found in the supernatant, counting to 2% of the MK-3 quantity found in the cells obtained from the same culture.
When the mutants were complemented with the respective genes, the MK production levels were restored, and profiles were similar to the original MG1363 (Figure 2B and Supplementary Figure 1B).
Anaerobic Conditions: Mutants Showed Distinct Phenotypes in Azo Dye and Cu2+ Reduction
Under anaerobic conditions, all mutants showed similar biomass accumulation as compared to wildtype MG1363 after overnight cultivation, which was about 1 g/L in cell dry weight (Figure 3A, and OD measurement in Supplementary Figure 2A showed an identical trend). The presumed MK-1 producer ΔispA and MK-3 producer Δllmg_0196 showed 1–2 log higher survival than non-MK producer ΔmenF and wildtype MG1363 (MK-9, 8, 3 producer) after prolonged cultivation for 72 h (Figure 3C).
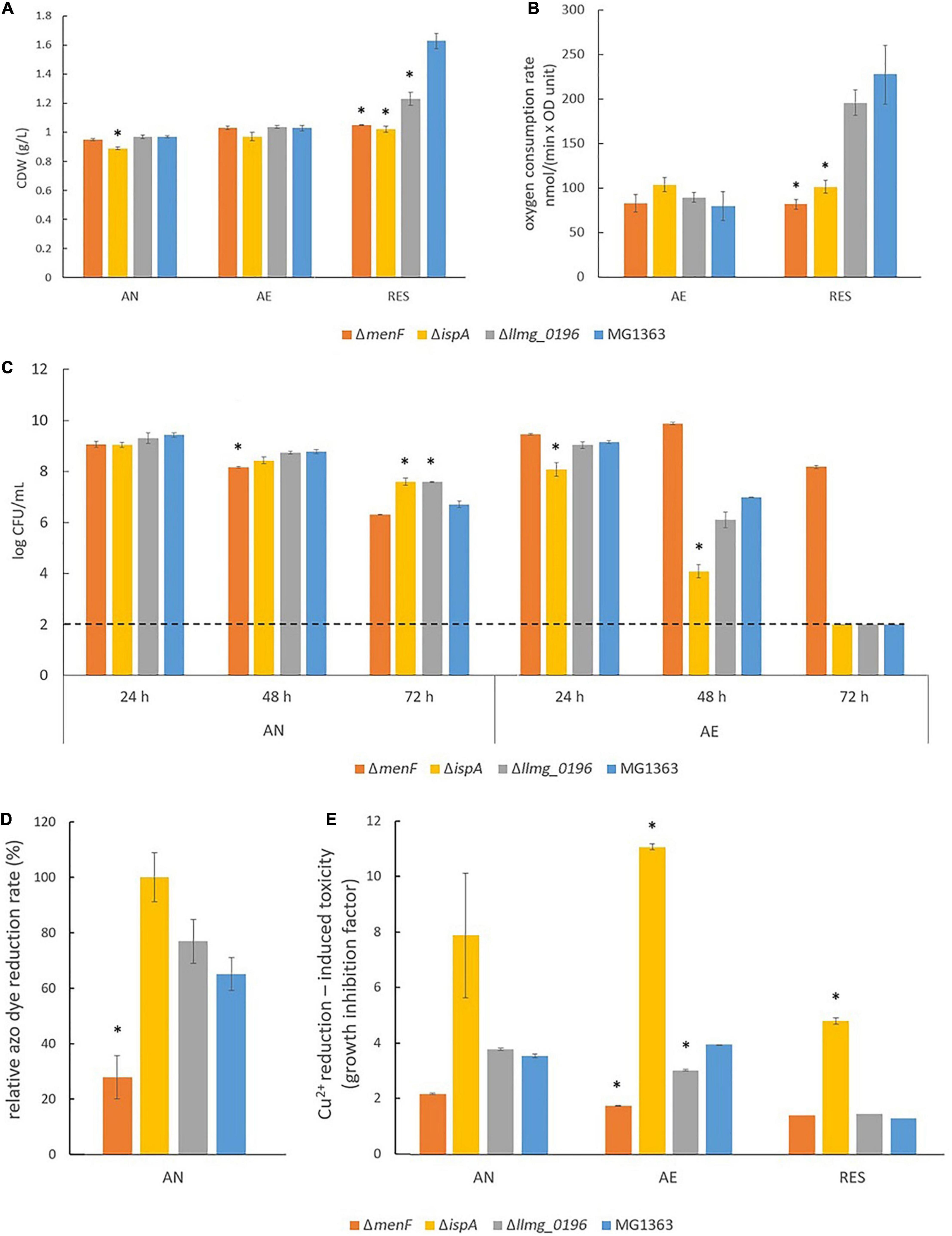
Figure 3. Phenotypical characterization of L. cremoris MG1363 and mutants under anaerobic, aerobic, and respiration-permissive conditions. (A) Biomass accumulation (cell dry weight). (B) Oxygen consumption rate. (C) Viable plate count. The dotted line indicates the detection limit. (D) Relative azo dye reduction rate. The relative azo dye reduction rate was calculated by setting the initial azo dye reduction rate in ΔispA as 100%. (E) Cu2+ reduction-induced toxicity reflected by growth inhibition. The time to reach (TTR) the factor difference between the time to reach (TTR) in the presence and absence of CuCl2 is an indicator of growth inhibition, which reflects Cu2+ reduction. (A–D) Data from three independent experiments, and (E) data from biological triplicates. *Shows significant (p < 0.05) difference to MG1363. Error bars show SEM. AN, anaerobic; AE, aerobic; RES, respiration-permissive, i.e., heme and aeration.
The most distinct phenotypes among the mutants under the anaerobic conditions were observed when subjecting the mutants to an azo dye reduction (decolorization) and a Cu2+ reduction test. In these tests, indicators for the functionality of the different forms of MKs in L. cremoris EET, which are mostly described for anaerobic conditions, were obtained. In the azo dye reduction test, ΔispA showed the highest rate of decolorization among all strains (Figure 3D, and example pictures of azo dye discoloration are provided in Supplementary Figure 4). The reduction rates for strains MG1363, Δllmg_0196, and ΔmenF were 65, 75, and 30% as compared to ΔispA. Observations in line with the azo dye decolorization test were obtained from the Cu2+ reduction test, which was reflected by the degree of growth inhibition in the presence of Cu2+ (Figure 3E). Strain ΔmenF was least inhibited in growth (factor 2) and ΔispA was most inhibited (factor 8), while wildtype strain MG1363 and the MK-3 producer Δllmg_0196 showed an intermediate degree of growth inhibition (factor 4) under anaerobic conditions.
The primary metabolite profiles of MG1363 and mutants were mostly similar among each other under anaerobic conditions: all strains produced mainly lactate (about 55 mM) and hardly any acetate or acetoin (Figure 4A). The analysis of the succinate concentration in culture supernatants revealed that ΔispA produced a noticeable amount of succinate (0.5 mM) while in cultures of the other strains succinate was not detectable indicating consumption of the small amount of succinate present in M17 media, and this effect is most pronounced under anaerobic conditions (Figure 4B).
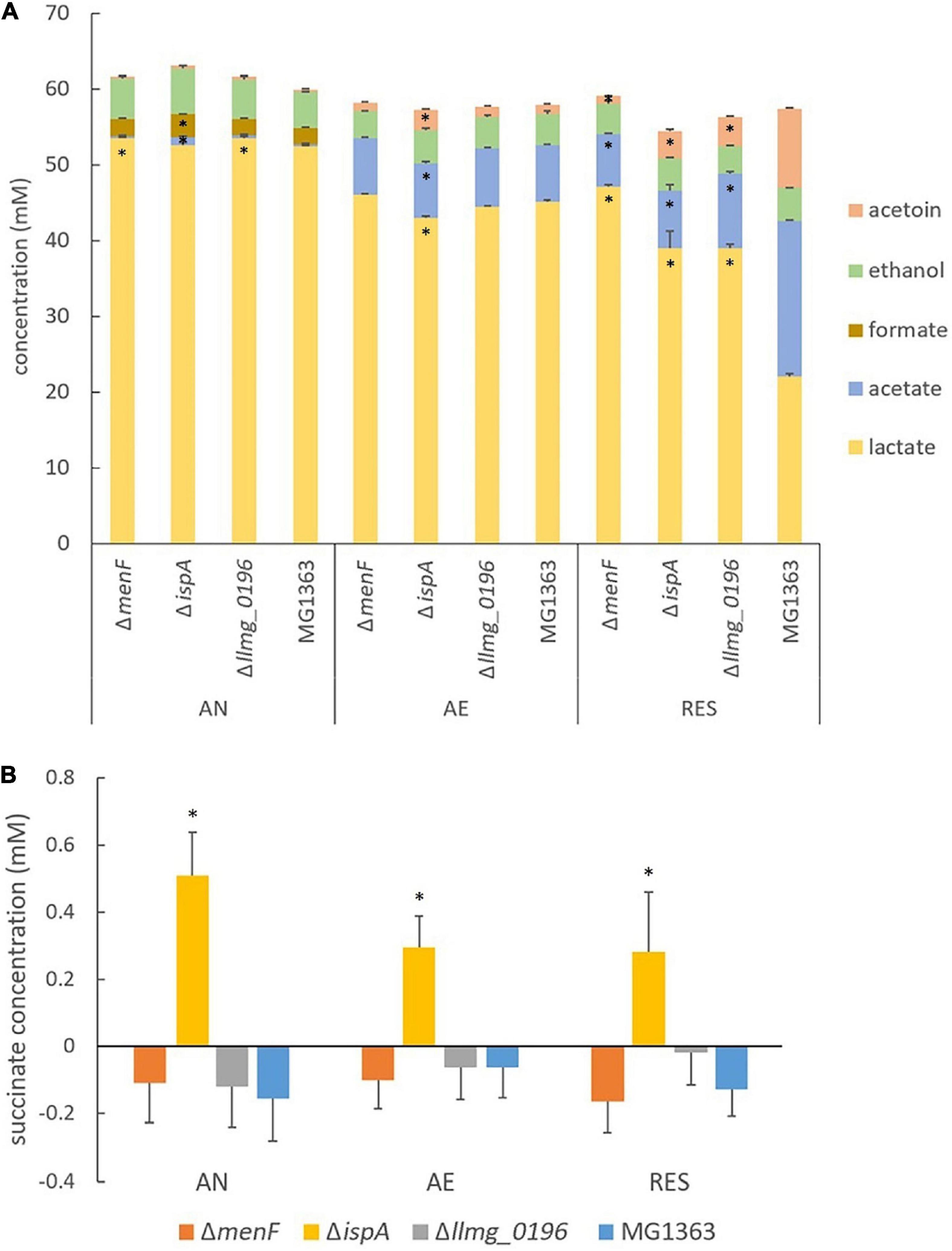
Figure 4. Metabolites in MG1363 and mutants. (A) Concentration of primary metabolites including lactate, acetate, formate, acetoin, and ethanol in the culture supernatant. (B) Concentration of succinate in the culture supernatant. A minor amount of succinate is present in GM17, and negative values from samples indicate consumption of the succinate. AN, anaerobic; AE, aerobic; RES, respiration-permissive, i.e., heme and aeration. Data from three independent experiments, error bars show SEM. *Shows significant (p < 0.05) difference to MG1363, for (A) this comparison is for the specific compound where the * is positioned.
Aerobic Conditions: Mutants Showed Distinct Phenotypes in Long-Term Survival and Cu2+ Reduction
Under aerobic conditions, all mutants showed similar biomass accumulation as compared to the wildtype strain MG1363 after overnight cultivation. The biomass yields were found to be at the same level as those observed under anaerobic conditions (Figure 3A and Supplementary Figure 2A). Also, the oxygen consumption rates for all strains were similar, ranging between 80 and 100 nmol/(min × OD unit) (Figure 3B). The primary metabolite profiles of all strains cultivated under aerobic conditions were also similar to each other, with a small decrease in lactate (by 5 mM) and an increase in acetate (by 5 mM) concentration as compared to the anaerobic conditions (Figure 4A).
The tested strains showed distinct phenotypes in survival when cultivated for a prolonged period of time under aerobic conditions (Figure 3C): the presumed MK-1 producer ΔispA showed 1 log lower viability than the other strains after 24 h cultivation, and the difference even further increased after 48 h. While the culture of the non-MK producer ΔmenF remained at a viable plate count of 10 log CFU/mL, for strain ΔispA this value was lowered to 4 log CFU/mL, followed by the MK-3 producer Δllmg_0196 and MK-9, 8, 3 producer MG1363 (6–7 log CFU/mL). After 72 h, none of the strains showed detectable viability (below 2 log CFU/mL) except strain ΔmenF which stood out with 8 log CFU/mL.
Big differences among the strains were also observed when the reduction of Cu2+ was tested under aerobic conditions (Figure 3E). The growth inhibition, as reflected by the factor difference in the time it took the bacterial culture to grow from OD600 of 0.1 to 0.4 in the absence and the presence of Cu2+, was used to indicate Cu2+ reduction as the reduced product Cu+ poses toxicity to the cells. The Cu2+ reduction induced growth inhibition was most severe with strain ΔispA (factor 11), even more than under anaerobic conditions. While ΔmenF remains the least inhibited (factor 2), Δllmg_0196 showed less inhibition (factor 3) than under anaerobic conditions, followed by MG1363 (factor 4).
Respiration-Permissive Conditions: Mutants Showed Distinct Phenotypes in Biomass Accumulation, Oxygen Consumption, and Metabolite Profiles
Under respiration-permissive conditions, differences were observed among the tested strains in biomass accumulation after overnight cultivation (Figure 3A and Supplementary Figure 2A). The MK-9, 8, 3 producer MG1363 reached about 1.7 g/L in cell dry weight, which is almost a doubling compared to the yield found in anaerobic and aerobic conditions. In the non-MK producer ΔmenF and presumed MK-1 producer ΔispA, the biomass accumulation remained the same level (1 g/L dry weight) as in anaerobic and aerobic conditions. In the MK-3 producer Δllmg_0196, about 1.3 g/L dry weight was obtained under respiration-permissive conditions, which is significantly higher than in ΔmenF and ΔispA but lower than MG1363. When the mutants were complemented with the respective genes, the biomass accumulations became similar to strain MG1363 (Supplementary Figure 3). The stationary phase survival for all strains under the respiration-permissive conditions was not significantly different throughout 72 h, all maintained at about 9 log CFU/mL (not shown).
The oxygen consumption rates in strain MG1363 and mutants under respiration-permissive conditions also showed differences. While ΔmenF and ΔispA remained at the same level as in aerobic conditions [80–100 nmol/(min × OD unit)], MG1363 and Δllmg_0196 showed much higher oxygen consumption rates, reaching values over 200 nmol/(min × OD unit).
Less difference among the strains was observed for the Cu2+ reduction test under the respiration-permissive conditions, all strains showed no growth inhibition caused by Cu2+ reduction (factor 1) except for ΔispA which still showed an inhibition factor of 5.
The metabolite profiles of strain MG1363 and mutants differed the most under respiration-permissive conditions (Figure 4A). Strain MG1363 produced the lowest concentration of lactate (about 20 mM) and the highest acetate (20 mM) and acetoin (10 mM) concentrations among all strains. The metabolite profiles of ΔmenF were similar under the respiration-permissive and aerobic conditions. For ΔispA and Δllmg_0196, about 40 mM lactate, 10 mM acetate, and 5 mM acetoin were observed under respiration-permissive conditions, showing differences in comparison to their own profiles under aerobic conditions. Moreover, the profiles of strains ΔispA and Δllmg_0196 also differed largely from that of strain MG1363 under respiration-permissive conditions.
Additional Insights Provided by Proteomics Analysis
Finally, we examined the proteome profiles of strain MG1363 and mutants under anaerobic, aerobic, and respiration-permissive conditions to further understand the phenotypic differences, which likely result from differences in bacterial metabolism, physiology, and functionality caused by the short-chain and long-chain MK forms. Cells from stationary phase (16 h) were used for this analysis, and 1394 proteins in the sample set were quantified.
We examined changes in the proteome profile between the wildtype strain MG1363 and the MK mutants under the various cultivation conditions (Figure 5). The proteome profiles obtained under anaerobic and aerobic conditions of cultures of the non-MK producer ΔmenF and the MK-3 producer Δllmg_0196 were found to be very similar to that of strain MG1363. Under respiration-permissive conditions, Δllmg_0196 showed a proteome profile similar to that of strain MG1363. In the comparison with strain MG1363, the presumed MK-1 producer ΔispA showed the most different proteome profile in all three conditions.
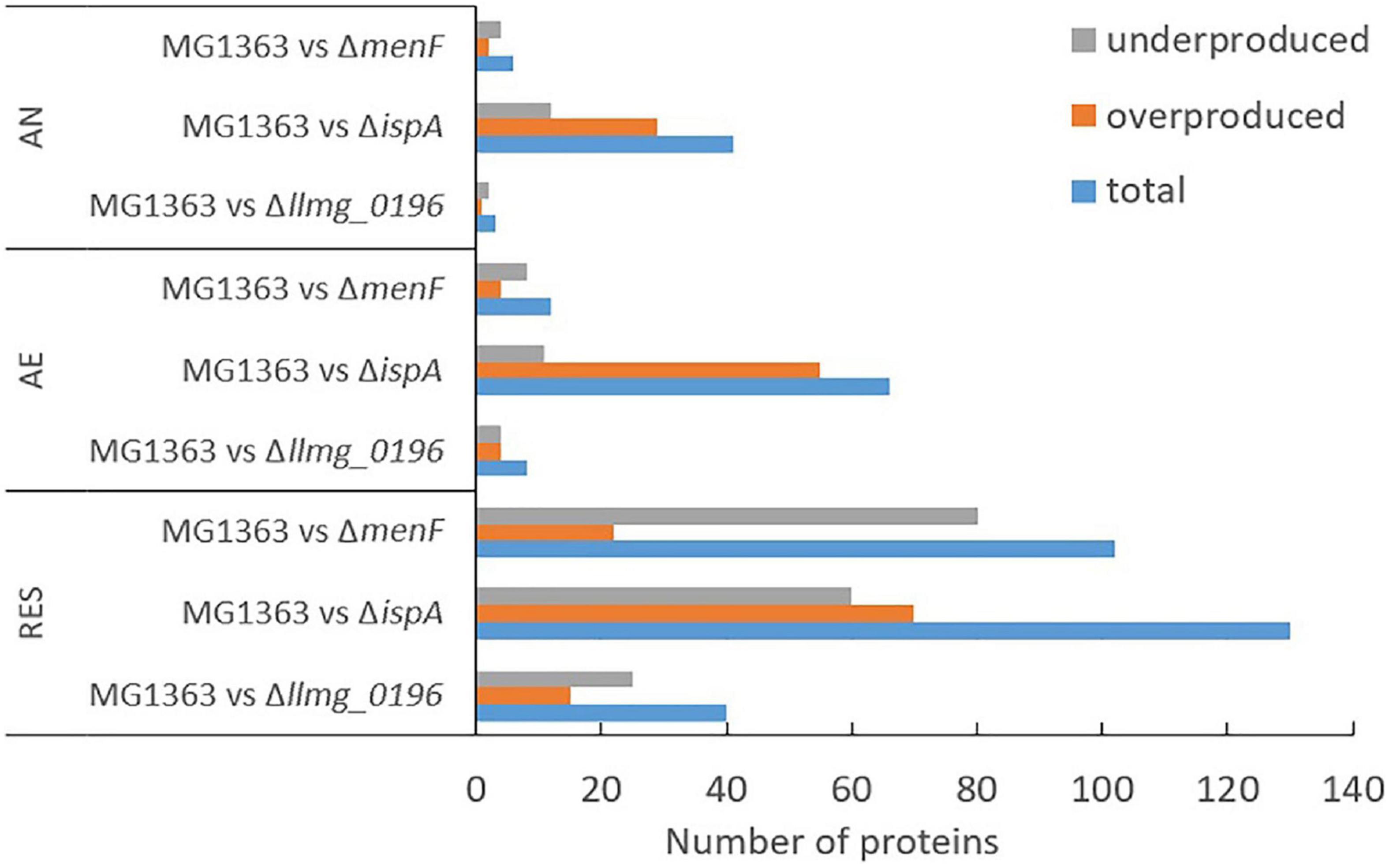
Figure 5. Differential proteomes. Numbers of differentially produced proteins between MG1363 and mutants under each cultivation condition. Samples from three independent experiments. AN, anaerobic; AE, aerobic; RES, respiration-permissive, i.e., heme and aeration. Proteins considered to be differently produced were selected when the LFQ intensity showed fold change ≥2 and p ≤ 0.05.
When closely examining the protein list, we identified proteins that are predicted to be involved in MK biosynthesis, aerobic respiration, aerobic growth, and EET/anaerobic respiration (Tables 2–5). Among proteins predicted to be part of the MK biosynthesis pathway (Table 2), we could first confirm that in respective mutants, the protein products of the deleted genes were indeed absent/only showed signals at exceptionally low levels possibly due to cross-contaminations in sample preparations and quantification errors. Most other MK biosynthesis proteins in this list showed constitutive presence in all strains and conditions, and for the protein product (A2RM75) of menB, for example, a slightly higher level was observed under the aerobic conditions, which could contribute to the higher MK content in L. cremoris under aerobic conditions observed in this study (Figure 2). The protein product (A2RHR6) of the gene annotated as menA was not detected in all cases, but the homologous protein (A2RJB1) encoded by ubiA was present constitutively, suggesting that the gene annotated as ubiA is in fact functional in merging the head and tail group of MKs in strain MG1363 instead of the gene annotated as menA. The absence of the protein product of gerCA (gene deletion mutant of gerCA) and ispB showed no influence on the MK profile and inconsistent detection of menX product also highlighted the necessity to experimentally confirm the functionality of the genes predicted in the MK biosynthesis pathway.
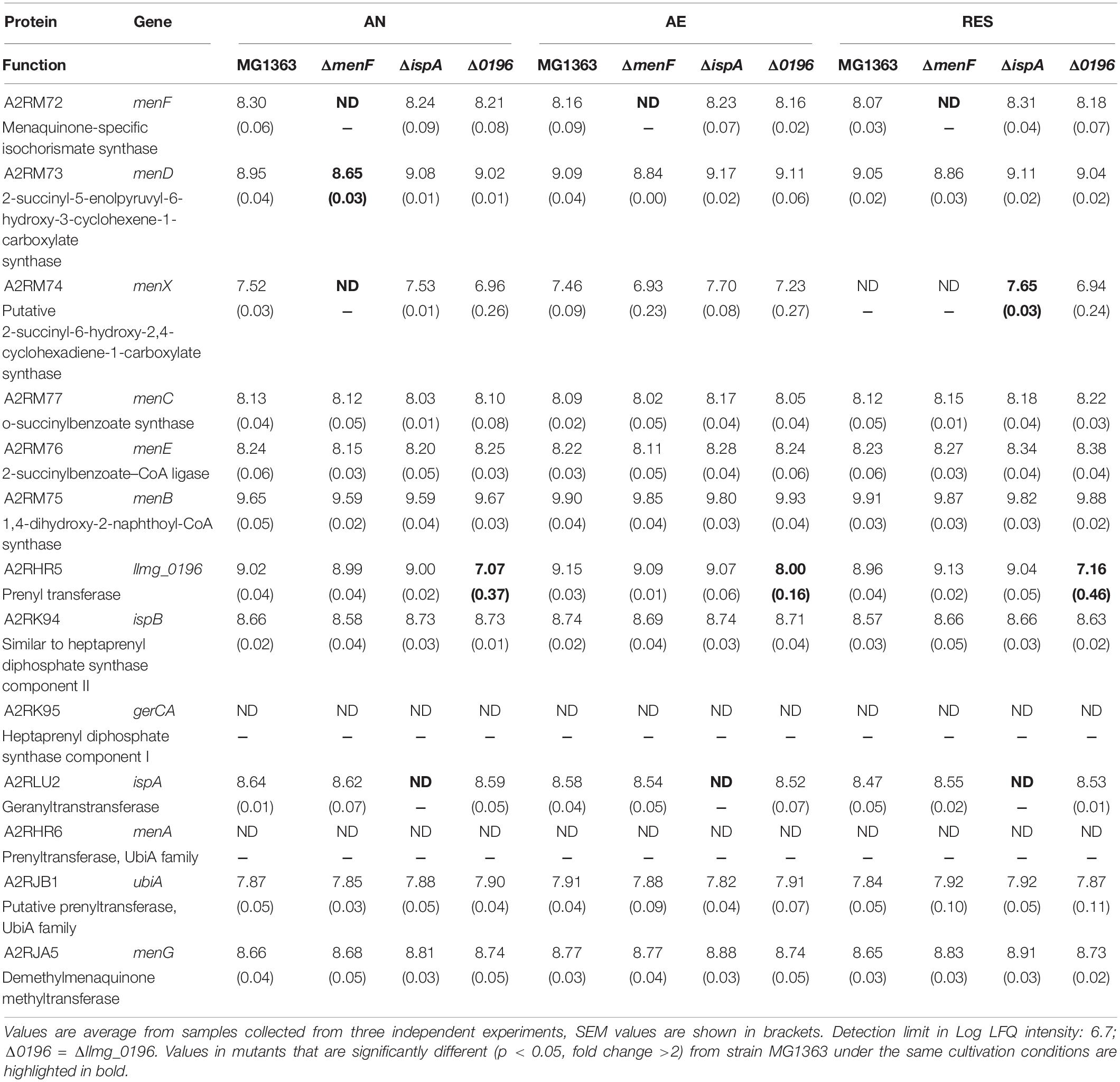
Table 2. Quantity (Log LFQ intensity) of proteins predicted in the MK biosynthesis pathway in MG1363 and mutant under different cultivation conditions.
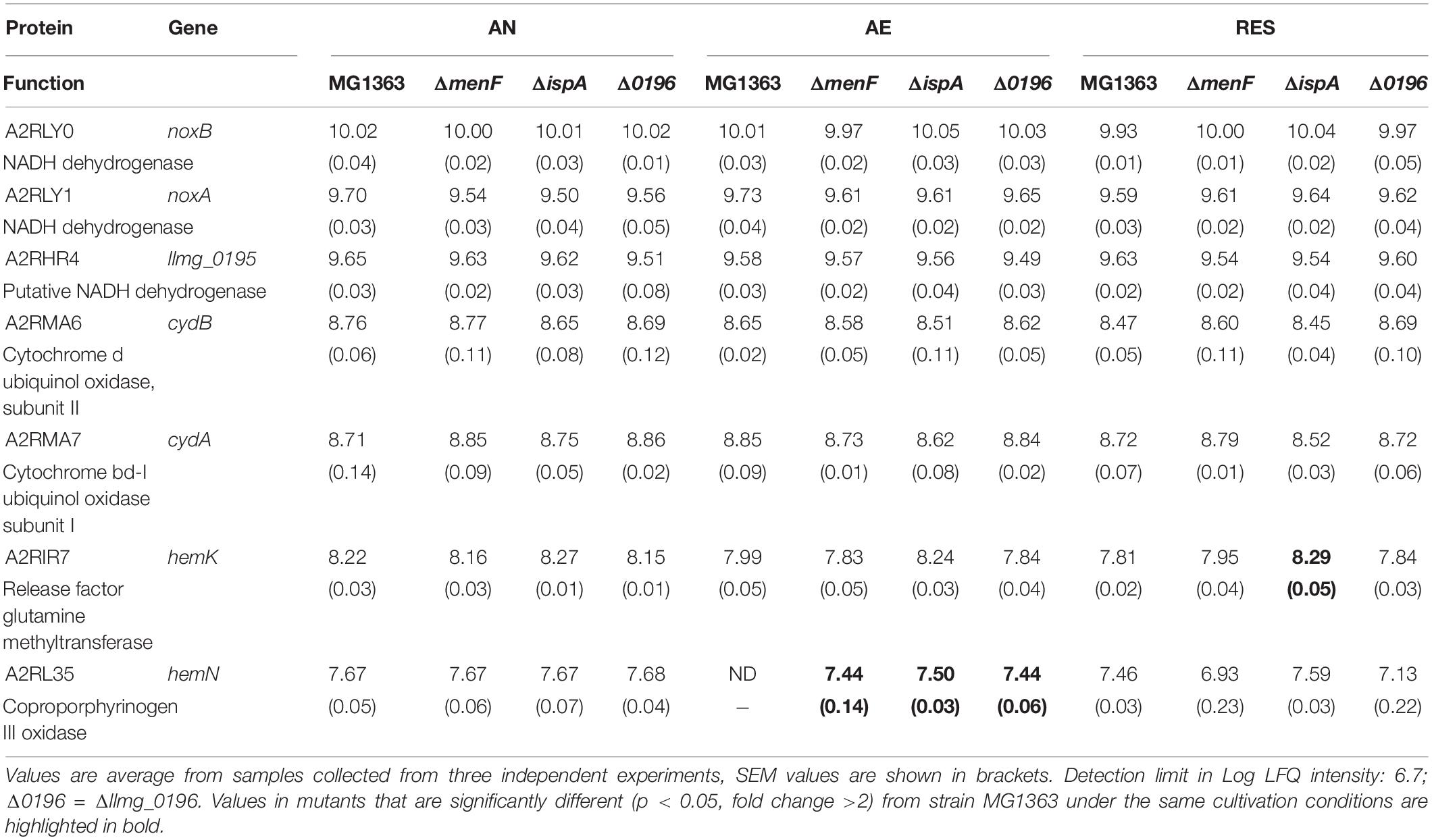
Table 3. Quantity (Log LFQ intensity) of proteins involved in aerobic respiratory ETC in MG1363 and mutant under different cultivation conditions.
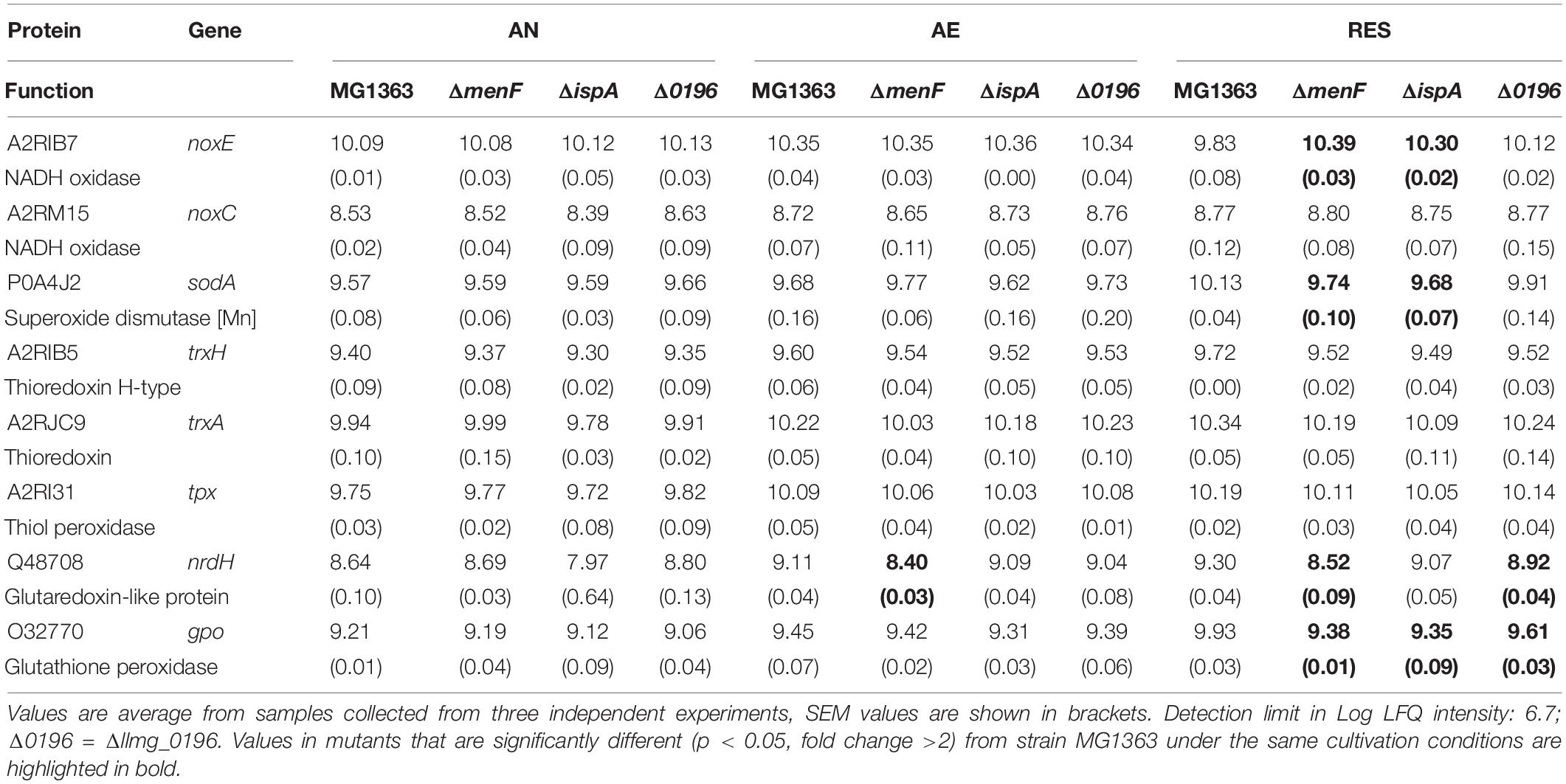
Table 4. Quantity (Log LFQ intensity) of proteins relevant for aerobic growth in general in MG1363 and mutant under different cultivation conditions.
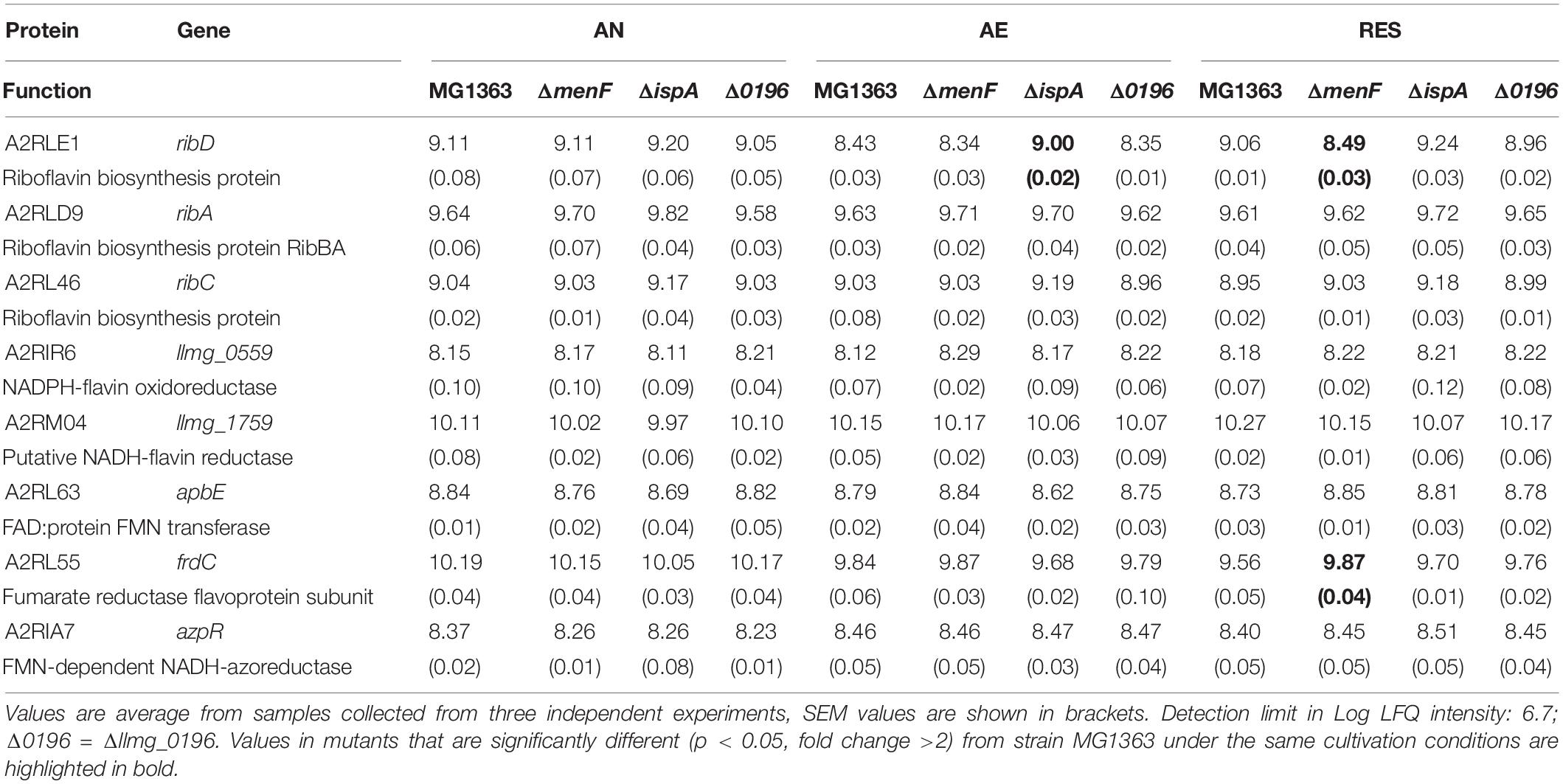
Table 5. Quantity (Log LFQ intensity) of proteins relevant for EET/anaerobic respiration in MG1363 and mutant under different cultivation conditions.
When examining the proteins predicted to be involved in aerobic respiration (Table 3), including the NADH dehydrogenase and cytochrome bd oxidase in the respiratory ETC, we see that these proteins are present in all strains under all cultivation conditions at similar levels. Protein products of two heme traffic/synthesis genes hemN and hemK were constantly present in most cases. Two type II NADH dehydrogenases have been identified in the proteome, encoded by genes noxA and noxB.
We also examined other proteins relevant for the aerobic cultivation conditions (Table 4), including NADH oxidase and several oxidoreductases. In general, proteins in this category were produced at higher levels under the aerobic conditions than anaerobic, as expected. The NADH oxidase (A2RIB7) in MG1363 and Δllmg_0196 was produced at a lower level in respiration-permissive conditions compared to aerobic conditions, which could be a result of the activated respiratory ETC taking over the reduction of oxygen in these two strains. Antioxidation proteins like thioredoxins (A2RIB5, A2RJC9, and A2RI31) were, in general, more abundant under the aerobic conditions than anaerobic conditions. Moreover, the low level of glutaredoxin-like protein (Q48708) in the ΔmenF under the aerobic conditions indeed reflects particular roles of MKs under oxidative/aerobic conditions.
Furthermore, we examined proteins predicted to be relevant for EET/anaerobic respiration (Table 5). While the NADH dehydrogenase encoded by noxB and most flavin synthesis or oxidoreduction related proteins were constantly present at similar levels in all strains and conditions, one riboflavin biosynthesis protein (A2RLE1) was more abundant in the MK-1 producer ΔispA than other strains under aerobic and respiration-permissive conditions. A fumarate reductase flavoprotein subunit protein (A2RL55) showed the highest level under anaerobic conditions in all strains, pointing to a potential role in anaerobic respiration.
Besides the proteins that are expected to be highly relevant for the several cultivation conditions and electron transfer pathways, much more information could be derived from the proteomics analysis. For example, when examining the proteins involved in primary metabolism (Supplementary Table 4), we observed protein level changes across the three cultivation conditions in the tested strains, which corresponds to the production of primary metabolites (Figure 4A). When examining the remainder of the proteins that showed significant changes across the strains or cultivation conditions, membrane proteins, oxidoreductases, stress proteins, metal ion (copper, iron, etc.) transporters, ATP-binding cassette (ABC) transporters were among the most often observed categories. A non-exhaustive list of these proteins is provided in Supplementary Table 5.
Discussion
Lactococcus cremoris and L. lactis are best-known for their occurrence and applications in dairy fermentations, but their niche extends to a range of natural and food production environments, where the bacteria encounters different growth conditions including anaerobic, aerobic, and respiration-permissive conditions. L. cremoris and L. lactis are among the few LAB species that maintained the ability to produce MKs (vitamin K2), in both short-chain (MK-3) and long-chain forms (MK-9 and MK-8). The physiological significance of the short-chain and long-chain MK forms in the lifestyle of L. cremoris and L. lactis has not been investigated extensively. In this study, we constructed L. cremoris mutants producing short-chain and long-chain MKs: deletion of genes menF, ispA, and llmg_0196 resulted in a non-MK producer, a presumed MK-1 producer, and an MK-3 producer, respectively. Together with the wildtype MG1363 producing mainly MK-9 and MK-8, these mutants with gene deletions served to further elucidate the functionality of MKs in L. cremoris. By exposing this set of strains to anaerobic, aerobic, and respiration-permissive conditions, functionalities of long-chain and short-chain MKs in L. cremoris under different environmental conditions could be inferred (Figure 6).
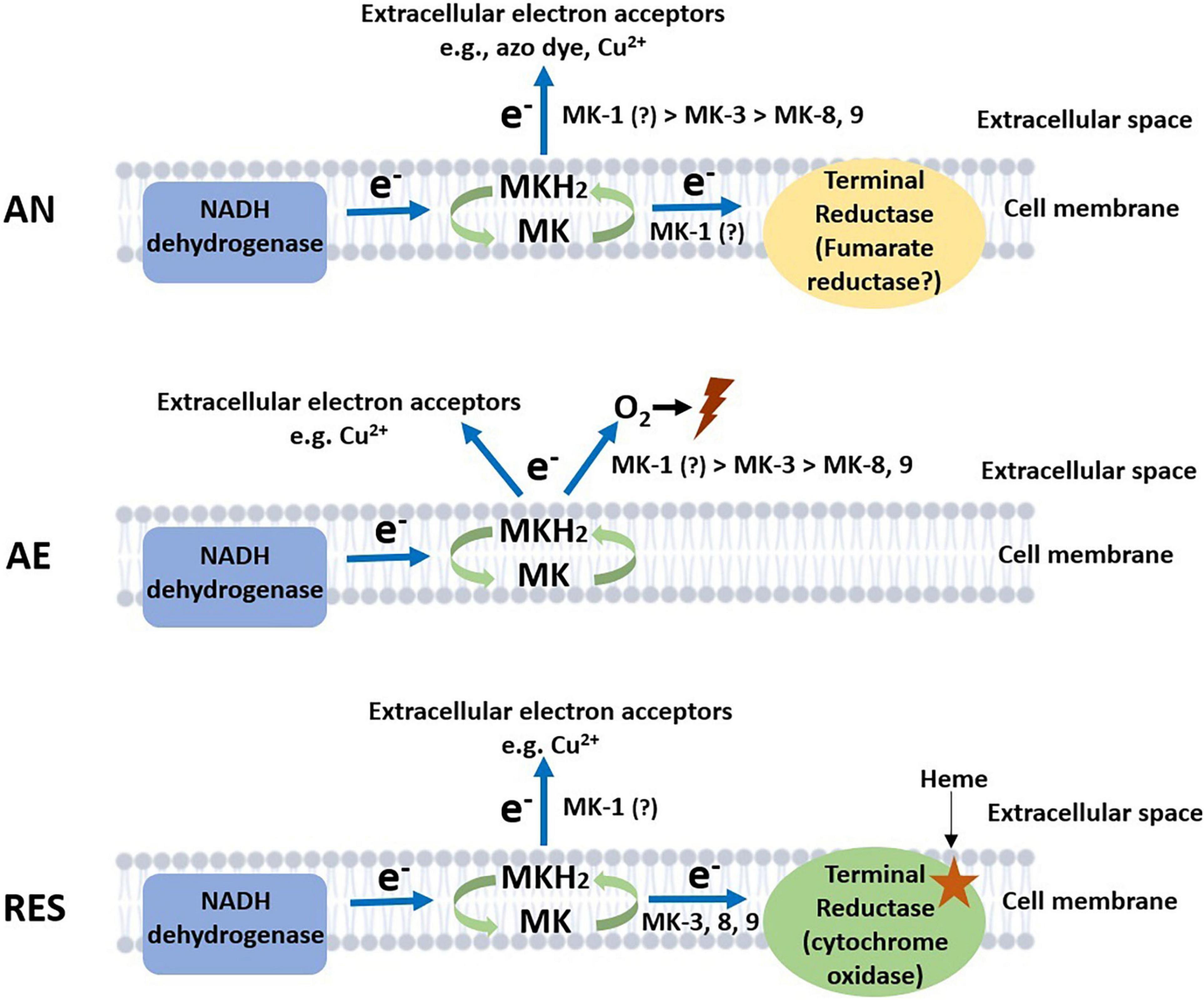
Figure 6. Proposed model of electron flows facilitated by MKs under anaerobic, aerobic, and respiration-permissive conditions. The predominantly involved MK forms are annotated next to the arrows indicating electron flows, with “>” symbols indicating preferences. Lightning symbol represents loss of viability in the cells. AN, anaerobic; AE, aerobic; RES, respiration-permissive, i.e., heme and aeration. Note that the illustration is only a schematic presentation of proposed electron flow routes, the location of enzymes is not confirmed in this study.
We could confirm that llmg_0196 indeed encodes a (nona)prenyl diphosphate synthase as the corresponding gene deletion mutant only produced MK-3. The level of MK-3 in Δllmg_0196 was lower than the total MK level in MG1363, indicating that there are feedback mechanisms influenced by the intermediate compounds of the MK biosynthetic pathway. Another possible explanation to this is a lower efficiency of the DHNA polyprenyltransferase (MenA) in incorporating the farnesyl diphosphate group (containing 3 isoprene units) to the head group.
Based on the observed MK profiles alone, it can be concluded that strains ΔmenF and ΔispA hardly produce MKs. The remaining low amount of MK-9 produced in strain ΔispA could be a result of additional activity of the prenyl diphosphate synthase encoded by llmg_0196. Combining other analyses that demonstrated the role of MKs, we saw distinct phenotypes of ΔmenF and ΔispA: in ΔmenF, phenotypes from all processes including aerobic respiration or copper reduction suggested absence of MK-related functions. In ΔispA, clear activities of, e.g., azo dye/copper reduction, could be observed, suggesting the presence of (a) MK form(s) that was not detected in the MK profile analysis. It is most plausible that MK-1 was produced by ΔispA but this MK was not quantified in the analysis because the method used in this study is based on hexane extraction of hydrophobic/lipophilic molecules. This method works well for long-chain MKs and lipids, but it is likely that MK-1 is too low in hydrophobicity to be extracted by hexane from the aqueous environment. This assumption is supported by a recent study from Van Cleave et al. (2021), where MK-1 was demonstrated to show distinct physiochemical features including reduced interactions with model membranes as compared to MK-2 to MK-4. Notably, the current MK extraction method is commonly adopted for MK analysis in scientific research, which could mean that the presence and role of MK-1, if any, may have been missed thus far in MK related studies. It is desired that in future studies, MK profile analysis includes methodology that allows extraction and detection of MK-1, and confirms the location of MK-1 (cell-associated or released into the aqueous environment). This could provide additional insights to explain the distinct features of ΔispA. Whether the reduced and oxidized MK forms have different physiochemical properties is also an interesting but thus far underexplored subject, due to instability of the reduced MK forms (Van Cleave et al., 2021).
Nevertheless, we could infer the function of MK-1 in the current study from the different phenotypes of the mutants. In this context it is worth mentioning that we did not analyze the presence of any MK precursors such as demethylmenaquinones DMKs, previously studied in L. cremoris (Rezaïki et al., 2008) and Listeria monocytogenes (Light et al., 2018). Here we focused on the MK forms with various sidechain length, and will therefore not discuss the effect of the methylation (DMK to MK) separately. Moreover, deletion of gene ispA as reported for other bacteria is expected to display pleiotropic effects including impaired growth (Krute et al., 2015). This was also observed for strain ΔispA in this study since ΔispA shows a slightly lower growth rate in comparison to the other strains (Supplementary Figure 2B).
Based on existing knowledge on MK roles in bacteria (Duwat et al., 2001; Koebmann et al., 2008; Rezaïki et al., 2008; Light et al., 2018), we examined the phenotypes of mutants in anaerobic, aerobic, and respiration-permissive conditions. The common or different functional features of short-chain MKs and long-chain MKs were revealed in the three conditions (Figure 6).
Under anaerobic conditions, the most distinct phenotypes among the strains were the ability to reduce azo dye and copper ions, which are indications of EET. The EET efficiency appeared to be higher in the presumed MK-1 producer ΔispA than the MK-3 and long-chain MKs producers, indicating a preference in anaerobic EET for short-chain MKs over long-chain MKs (Figure 6). The same preference was suggested by the metabolite analysis where succinate was formed by ΔispA, plausibly because of anaerobic electron transport chain with fumarate serving as the electron acceptor. The role of MKs in anaerobic respiration via reduction of fumarate to succinate has been reported for E. coli and E. faecalis (Huycke et al., 2001; Brooijmans et al., 2009a). Genome annotation of L. cremoris strain MG1363 indeed reveals the gene frdC encoding a fumarate reductase, homologous to the fumarate reductases reported for E. coli and E. faecalis. In fact, activity of a fumarate reductase was indeed reported in L. lactis a long time ago (Hillier et al., 1979). In agreement with the theory, a fumarate reductase flavoprotein subunit protein (A2RL55) was also identified in this study in the proteome of all strains, with high abundance under anaerobic conditions. In addition, the higher stationary phase survival of ΔispA and Δllmg_0196 under anaerobic conditions supports the hypothesis that short-chain MKs offer an advantage for L. cremoris in anaerobic respiration/EET, allowing additional energy generation for maintenance in the stationary phase cells.
Under aerobic conditions, significant differences were observed in stationary phase survival of the strains, where non-MK producer ΔmenF maintained high viability throughout the incubation period while the presumed MK-1 producer ΔispA lost viability the fastest followed by the MK-3 producer Δllmg_0196. Under aerobic conditions where the cytochrome oxidase is not functional (no heme supplementation), MKs have been suggested to mediate the reduction of oxygen, promoting ROS formation in L. cremoris (Rezaïki et al., 2008). The MK-mediated ROS formation could reduce the viability of cells, explaining the observed differences in the tested strains. In the proposed model, we assume MK-mediated ROS formation as an extracellular activity. This model is supported by measurement of extracellular superoxide formation mediated by (D)MKs in L. cremoris (Rezaïki et al., 2008) and E. faecalis (Huycke et al., 2001) under aerobic conditions. However, we do not exclude the possibility that MK-mediated ROS formation also takes place in the cytoplasm, but this intracellular ROS fraction is not expected to pose major damage to the cells due to the activity of an intracellular superoxide dismutase (Huycke et al., 2001; Ballal et al., 2015), which has also been identified in the proteome determined in our study (Table 4, protein ID P0A4J2). Therefore, the difference in cell viability observed after prolonged cultivation under aerobic conditions is considered to be mainly a result of the extracellular ROS formation mediated by MKs. Here a preference for short-chain MKs seemed to exist for the reaction with oxygen/ROS formation too (Figure 6).
Furthermore, the copper reduction still took place under aerobic conditions, but to a lesser extent in Δllmg_0196 as compared to anaerobic conditions. This could mean that, in the absence of a functional cytochrome oxidase, the electron flow via MKs toward oxygen and EET can both be active, with possible competition between the two pathways of the electrons (Figure 6).
Under respiration-permissive conditions, it was consistently shown that MG1363 demonstrated all aerobic respiration-related phenotypes: increased biomass yield in comparison to fermentative growth due to more efficient ATP generation, higher oxygen consumption rate than under aerobic conditions, large decrease in lactate production and increase in acetate and acetoin as compared to cultures in the fermentative growth mode as a result of redox cofactor (NAD/NADH) changes caused by the membrane embedded ETC, etc. (Duwat et al., 2001; Brooijmans et al., 2009c). The MK-3 producer Δllmg_0196 demonstrated these phenotypical changes, but to a lesser extent than MG1363, which could be explained by the lower content of MKs. The non-MK producer ΔmenF as well as presumed MK-1 producer ΔispA did not show these changes. The proteome profiles reflected the same change/difference among the strains. It could be collectively deduced that long-chain MKs (MK-9 and MK-8) and MK-3, but not MK-1, complement the respiratory ETC in L. cremoris.
As a functional cytochrome oxidase was provided, the copper reduction activity was attenuated in MG1363 and Δllmg_0196. This observation agrees with the previous findings: heme-induced respiration of L. lactis suppressed copper reduction (Abicht et al., 2013); L. cremoris and L. lactis could decolorize azo dyes only under anaerobic conditions (Pérez-Díaz and McFeeters, 2009); and the EET in E. faecalis was attenuated by cytochrome bd oxidase activity (Pankratova et al., 2018). The remaining copper reduction activity in ΔispA suggests that MK-1 has the highest affinity for EET among all studied electron transfer routes.
The different efficiency of long-chain and short-chain MKs in the various electron transfer pathways is a result of the hydrophobicity of the MK forms in relation to the location where electron transfer takes place. The respiratory ETC has all components located in the lipid bilayer of the cell membrane, and long-chain MKs are well-embedded in the membrane due to the strong hydrophobicity. In contrast, pathways like EET direct electrons toward the extracellular electron acceptors, and thus the short-chain MKs with higher hydrophilicity demonstrate advantages in transferring electrons to the free flavin shuttles (Light et al., 2018), or even act as soluble electron shuttles themselves (Freguia et al., 2009).
This study examined the functions of short-chain and long-chain MKs in L. cremoris in lab conditions, but the significance can be inferred for L. cremoris and L. lactis in natural settings, too. For example, the role of MKs in EET allows more options for bacteria to utilize carbon sources that are otherwise not fermentable (Light et al., 2018). Although not investigated extensively in this study, we did identify a putative fumarate reductase flavoprotein (Table 5, protein ID A2RL55) that is overproduced under anaerobic conditions in L. cremoris. This fumarate reductase shows homology to a cell surface-associated fumarate reductase described in Ls. monocytogenes (Light et al., 2019). The fumarate reductase in Ls. monocytogenes was found to rely on EET to reduce fumarate to succinate, supporting growth of the bacterium via an anaerobic respiration mechanism; an EET mutant showed competitive disadvantage in the gut of mouse (Light et al., 2018). Similarly, competitive advantages offered by EET mediated by MKs can be inferred for L. cremoris and L. lactis: for the application in cheese production, the ripening process takes place when these bacteria face nutrient limitation/carbon starvation, but metabolic activity is still desired for flavor development in cheese (Smid and Kleerebezem, 2014). EET mediated by MKs, especially the short-chain MKs, are expected to offer advantages to L. cremoris and L. lactis for additional nutrient source utilization and maintaining longer survival/metabolic activity period, ideal for successful or even accelerated cheese ripening.
The possible role of MKs in ROS formation and the consequence for stationary phase survival of L. cremoris suggest disadvantages of MK production in this bacterium, in particular under aerobic conditions when heme is not supplied. This knowledge can be applied for selecting natural variants of L. cremoris and L. lactis that are extra resistant to the oxidative conditions during starter culture production and initial stages in cheese production, given the improved robustness of the non-MK producer under aerobic conditions. Interestingly, a previous study demonstrated that adaptive laboratory evolution of L. cremoris under highly aerated conditions resulted in evolved mutants showing elevated vitamin K2 content and enhanced resistance to oxidative stress (Liu et al., 2021). This supports that natural selection methods, based on the roles of MKs in L. cremoris, allow us to obtain several types of variants that are of interest for applications.
Nevertheless, as the effect of MKs in L. cremoris survival is only displayed after prolonged exposure to oxygen (48–72 h), MKs can be beneficial for L. cremoris in dairy fermentation, where the bacterium is more exposed to anaerobic conditions. In other natural settings, such as the original niche of L. cremoris and L. lactis, i.e., plant surfaces, heme, and oxygen are often available (Pedersen et al., 2012; Cavanagh et al., 2015). Under such circumstances, maintaining the MK producing ability indeed offers advantage to L. cremoris and L. lactis as the aerobic respiration promotes the growth and survival of these bacteria (Duwat et al., 2001; Gaudu et al., 2002). The heme-induced respiration has been applied in starter production to obtain higher yield and more robust L. cremoris and L. lactis for the dairy industry (Pedersen et al., 2005; Garrigues et al., 2006).
Notably, MKs are also known as vitamin K2, a fat-soluble vitamin essential to human health (Vermeer and Schurgers, 2000; Beulens et al., 2013). In the human body, vitamin K2 acts as the co-factor for γ-glutamyl carboxylase catalyzing the carboxylation of the glutamate residues in Gla-proteins, thereby ensuring biological functions of Gla-proteins in essential human physiological processes including hemostasis, calcium metabolism, and cell growth regulation. Dietary intake of vitamin K2 has been associated with reduced risk of coronary heart disease and improved bone health (Geleijnse et al., 2004; Fujita et al., 2012). In terms of benefits for human health, the interest often goes to the long-chain MKs for the higher bioavailability they demonstrated compared to the short-chain MKs; the association with cardiovascular health was also demonstrated prominently with intake of long-chain MKs (Geleijnse et al., 2004; Sato et al., 2012). The insight in the physiological functions of MKs in L. cremoris also provides opportunities for vitamin K2 enrichment of fermented food products: suitable conditions can be designed accordingly for natural selection procedures to obtain variants with desired vitamin K2 production profiles.
Taken together, the set of both long-chain and short-chain MKs produced by L. cremoris (and L. lactis), in combination with the different functionality of MK variants in anaerobic, aerobic, and respiration-permissive conditions, points to an efficient strategy to adapt to diverse aeration and nutritional conditions that L. cremoris and L. lactis strains may encounter in their natural niche or during food fermentation processes. It is highly desirable to extend the inferred significance of MKs in L. cremoris from lab settings to natural ecological systems with further investigations. As the short-chain MKs are more water soluble and allow possibilities for cross-feeding (Rezaïki et al., 2008), their relevance for interacting not only with the environment, but also with hosts or cohabitating microbes extends the significance to diverse habitats.
Data Availability Statement
The proteome dataset presented in this study can be found in online repository ProteomeXchange (PXD030833).
Author Contributions
YL, ES, and TA conceived the study and wrote the manuscript. YL designed the experiments, executed the experiments, and carried out the data analysis and interpretation. NC contributed to the phenotypical characterization of the mutants. SB contributed to obtaining the proteomics data. NC, JB, and AL contributed to mutant construction. All authors read and approved the final manuscript.
Funding
This work was subsidized by the Netherlands Organization for Scientific Research (NWO) through the Graduate Program on Food Structures, Digestion and Health.
Conflict of Interest
The authors declare that the research was conducted in the absence of any commercial or financial relationships that could be construed as a potential conflict of interest.
Publisher’s Note
All claims expressed in this article are solely those of the authors and do not necessarily represent those of their affiliated organizations, or those of the publisher, the editors and the reviewers. Any product that may be evaluated in this article, or claim that may be made by its manufacturer, is not guaranteed or endorsed by the publisher.
Acknowledgments
We would like to thank Jeroen Koomen (Food Microbiology, Wageningen University and Research) for his kind help in analyzing proteomics data. We also thank Mark Sanders (Food Chemistry, Wageningen University and Research) for his assistance in vitamin K2 analysis.
Supplementary Material
The Supplementary Material for this article can be found online at: https://www.frontiersin.org/articles/10.3389/fmicb.2022.823623/full#supplementary-material
References
Abicht, H. K., Gonskikh, Y., Gerber, S. D., and Solioz, M. (2013). Non-enzymic copper reduction by menaquinone enhances copper toxicity in Lactococcus lactis IL1403. Microbiology 159, 1190–1197. doi: 10.1099/mic.0.066928-0
Ballal, S. A., Veiga, P., Fenn, K., Michaud, M., Kim, J. H., Gallini, C. A., et al. (2015). Host lysozyme-mediated lysis of Lactococcus lactis facilitates delivery of colitis-attenuating superoxide dismutase to inflamed colons. Proc. Natl. Acad. Sci. U. S. A. 112, 7803–7808. doi: 10.1073/pnas.1501897112
Beulens, J. W. J., Booth, S. L., van Den Heuvel, E. G. H. M., Stoecklin, E., Baka, A., and Vermeer, C. (2013). The role of menaquinones (vitamin K2) in human health. Br. J. Nutr. 110, 1357–1368. doi: 10.1017/S0007114513001013
Brooijmans, R., Smit, B., Santos, F., van Riel, J., de Vos, W. M., and Hugenholtz, J. (2009c). Heme and menaquinone induced electron transport in lactic acid bacteria. Microb. Cell Fact. 8:28. doi: 10.1186/1475-2859-8-28
Brooijmans, R. J. W., de Vos, W. M., and Hugenholtz, J. (2009b). Lactobacillus plantarum WCFS1 electron transport chains. Appl. Environ. Microbiol. 75, 3580–3585. doi: 10.1128/AEM.00147-09
Brooijmans, R., de Vos, W. M., and Hugenholtz, J. (2009a). Electron transport chains of lactic acid bacteria - walking on crutches is part of their lifestyle. F1000 Biol. Rep. 1:34. doi: 10.3410/b1-34
Bryan, E. M., Bae, T., Kleerebezem, M., and Dunny, G. M. (2000). Improved vectors for nisin-controlled expression in Gram-positive bacteria. Plasmid 44, 183–190. doi: 10.1006/plas.2000.1484
Cavanagh, D., Fitzgerald, G. F., and McAuliffe, O. (2015). From field to fermentation: the origins of Lactococcus lactis and its domestication to the dairy environment. Food Microbiol. 47, 45–61. doi: 10.1016/j.fm.2014.11.001
Chen, I. M. A., Chu, K., Palaniappan, K., Pillay, M., Ratner, A., Huang, J., et al. (2019). IMG/M v.5.0: an integrated data management and comparative analysis system for microbial genomes and microbiomes. Nucleic Acids Res. 47, D666–D677. doi: 10.1093/nar/gky901
Collins, M. D., and Jones, D. (1981). Distribution of isoprenoid quinone structural types in bacteria and their taxonomic implications. Microbiol. Rev. 45, 316–354. doi: 10.1128/mmbr.45.2.316-354.1981
Cretenet, M., Le Gall, G., Wegmann, U., Even, S., Shearman, C., Stentz, R., et al. (2014). Early adaptation to oxygen is key to the industrially important traits of Lactococcus lactis ssp. cremoris during milk fermentation. BMC Genomics 15:1054. doi: 10.1186/1471-2164-15-1054
Daruwala, R., Kwon, O., Meganathan, R., and Hudspeth, M. E. S. (1996). A new isochorismate synthase specifically involved in menaquinone (vitamin K2) biosynthesis encoded by the menF gene. FEMS Microbiol. Lett. 140, 159–163. doi: 10.1111/j.1574-6968.1996.tb08330.x
Duwat, P., Sourice, S., Cesselin, B., Lamberet, G., Vido, K., Gaudu, P., et al. (2001). Respiration capacity of the fermenting bacterium Lactococcus lactis and its positive effects on growth and survival. J. Bacteriol. 183, 4509–4516. doi: 10.1128/JB.183.15.4509-4516.2001
Fang, Y., Liu, J., Kong, G., Liu, X., Yang, Y., Li, E., et al. (2019). Adaptive responses of Shewanella decolorationis to toxic organic extracellular electron acceptor azo dyes in anaerobic respiration. Appl. Environ. Microbiol. 85, e00550–19. doi: 10.1128/AEM.00550-19
Freguia, S., Masuda, M., Tsujimura, S., and Kano, K. (2009). Lactococcus lactis catalyses electricity generation at microbial fuel cell anodes via excretion of a soluble quinone. Bioelectrochemistry 76, 14–18. doi: 10.1016/j.bioelechem.2009.04.001
Fujita, Y., Iki, M., Tamaki, J., Kouda, K., Yura, A., Kadowaki, E., et al. (2012). Association between vitamin K intake from fermented soybeans, natto, and bone mineral density in elderly Japanese men: the Fujiwara-kyo Osteoporosis Risk in Men (FORMEN) study. Osteoporos. Int. 23, 705–714. doi: 10.1007/s00198-011-1594-1
Garrigues, C., Johansen, E., Pedersen, M. B. Møllgaard, H. Sørensen, K. I., Gaudu, P., et al. (2006). Getting high (OD) on heme. Nat. Rev. Microbiol. 4:318. doi: 10.1038/nrmicro1403-c1
Gasson, M. J. (1983). Plasmid complements of Streptococcus lactis NCDO 712 and other lactic streptococci after protoplast-induced curing. J. Bacteriol. 154, 1–9. doi: 10.1128/jb.154.1.1-9.1983
Gaudu, P., Vido, K., Cesselin, B., Kulakauskas, S., Tremblay, J., Rezaïki, L., et al. (2002). Respiration capacity and consequences in Lactococcus lactis. Antonie Van Leeuwenhoek 82, 263–269. doi: 10.1007/978-94-017-2029-8_16
Geleijnse, J. M., Vermeer, C., Grobbee, D. E., Schurgers, L. J., Knapen, M. H. J., van Der Meer, I. M., et al. (2004). Dietary intake of menaquinone is associated with a reduced risk of coronary heart disease: the Rotterdam Study. J. Nutr. 134, 3100–3105. doi: 10.1093/jn/134.11.3100
Hillier, A. J., Jericho, R. E., Green, S. M., and Jago, G. R. (1979). Properties and Function of Fumarate Reductase (n.d.) in Streptococcus Lactis. Aust. J. Biol. Sci. 32, 625–635. doi: 10.1071/BI9790625
Holo, H., and Nes, I. F. (1989). High-Frequency Transformation, by Electroporation, of Lactococcus lactis subsp. cremoris Grown with Glycine in Osmotically Stabilized Media. Appl. Environ. Microbiol. 55, 3119–3123. doi: 10.1128/aem.55.12.3119-3123.1989
Huycke, M. M., Moore, D., Joyce, W., Wise, P., Shepard, L., Kotake, Y., et al. (2001). Extracellular superoxide production by Enterococcus faecalis requires demethylmenaquinone and is attenuated by functional terminal quinol oxidases. Mol. Microbiol. 42, 729–740. doi: 10.1046/j.1365-2958.2001.02638.x
Jensen, P. R., and Hammer, K. (1993). Minimal Requirements for Exponential Growth of Lactococcus lactis. Appl. Environ. Microbiol. 59, 4363–4366. doi: 10.1128/aem.59.12.4363-4366.1993
Johnston, J. M., and Bulloch, E. M. (2020). Advances in menaquinone biosynthesis: sublocalisation and allosteric regulation. Curr. Opin. Struct. Biol. 65, 33–41. doi: 10.1016/j.sbi.2020.05.005
Kanehisa, M., and Goto, S. (2000). KEGG: Kyoto Encyclopedia of Genes and Genomes. Nucleic Acids Res. 28, 27–30. doi: 10.1093/nar/28.1.27
Kleerebezem, M., Bachmann, H., van Pelt-KleinJan, E., Douwenga, S., Smid, E. J., Teusink, B., et al. (2020). Lifestyle, metabolism and environmental adaptation in Lactococcus lactis. FEMS Microbiol. Rev. 44, 804–820. doi: 10.1093/femsre/fuaa033
Kobayashi, K., Ehrlich, S. D., Albertini, A., Amati, G., Andersen, K. K., Arnaud, M., et al. (2003). Essential Bacillus subtilis genes. Proc. Natl. Acad. Sci. U. S. A. 100, 4678–4683. doi: 10.1073/pnas.0730515100
Koebmann, B., Blank, L. M., Solem, C., Petranovic, D., Nielsen, L. K., and Jensen, P. R. (2008). Increased biomass yield of Lactococcus lactis during energetically limited growth and respiratory conditions. Biotechnol. Appl. Biochem. 50, 25–33. doi: 10.1042/ba20070132
Krute, C. N., Carroll, R. K., Rivera, F. E., Weiss, A., Young, R. M., Shilling, A., et al. (2015). The disruption of prenylation leads to pleiotropic rearrangements in cellular behavior in Staphylococcus aureus. Mol. Microbiol. 95, 819–832. doi: 10.1111/mmi.12900
Kurosu, M., and Begari, E. (2010). Vitamin K2 in electron transport system: are enzymes involved in vitamin K2 biosynthesis promising drug targets? Molecules 15, 1531–1553. doi: 10.3390/molecules15031531
Lenaz, G., and Genova, M. L. (2013). “Quinones,” in Encyclopedia of Biological Chemistry, eds W. Lennarz and M. Lane (Cambridge: Academic Press), 722–729. doi: 10.1016/B978-0-12-378630-2.00204-8
Li, T. T., Wen, L. T., and Chun, T. G. (2021). Elevation of Lactococcus lactis subsp. cremoris to the species level as Lactococcus cremoris sp. nov. and transfer of Lactococcus lactis subsp. tructae to Lactococcus cremoris as Lactococcus cremoris subsp. tructae comb. nov. Int. J. Syst. Evol. 71:004727.
Light, S. H., Méheust, R., Ferrell, J. L., Cho, J., Deng, D., Agostoni, M., et al. (2019). Extracellular electron transfer powers flavinylated extracellular reductases in Gram-positive bacteria. Proc. Natl. Acad. Sci. U. S. A. 116, 26892–26899. doi: 10.1073/pnas.1915678116
Light, S. H., Su, L., Rivera-Lugo, R., Cornejo, J. A., Louie, A., Iavarone, A. T., et al. (2018). A flavin-based extracellular electron transfer mechanism in diverse Gram-positive bacteria. Nature 562, 140–144. doi: 10.1038/s41586-018-0498-z
Liu, Y., de Groot, A., Boeren, S., Abee, T., and Smid, E. J. (2021). Lactococcus lactis Mutants Obtained From Laboratory Evolution Showed Elevated Vitamin K2 Content and Enhanced Resistance to Oxidative Stress. Front. Microbiol. 12:746770. doi: 10.3389/FMICB.2021.746770
Liu, Y., van Bennekom, E. O., Zhang, Y., Abee, T., and Smid, E. J. (2019). Long-chain vitamin K2 production in Lactococcus lactis is influenced by temperature, carbon source, aeration and mode of energy metabolism. Microb. Cell Fact. 18:129. doi: 10.1186/s12934-019-1179-9
Love, J., Selker, R., Marsman, M., Jamil, T., Dropmann, D., Verhagen, J., et al. (2019). JASP: graphical statistical software for common statistical designs. J. Stat. Softw. 88:2. doi: 10.18637/jss.v088.i02
Nagel, R., Thomas, J. A., Adekunle, F. A., Mann, F. M., and Peters, R. J. (2018). Arginine in the FARM and SARM: a role in chain-length determination for arginine in the aspartate-rich motifs of isoprenyl diphosphate synthases from Mycobacterium tuberculosis. Molecules 23:2546. doi: 10.3390/molecules23102546
Pankratova, G., Leech, D., Gorton, L., and Hederstedt, L. (2018). Extracellular electron transfer by the Gram-positive bacterium Enterococcus faecalis. Biochemistry 57, 4597–4603. doi: 10.1021/acs.biochem.8b00600
Pedersen, M. B., Gaudu, P., Lechardeur, D., Petit, M. A., and Gruss, A. (2012). Aerobic respiration metabolism in lactic acid bacteria and uses in biotechnology. Annu. Rev. Food Sci. Technol. 3, 37–58. doi: 10.1146/annurev-food-022811-101255
Pedersen, M. B., Iversen, S. L. Sørensen, K. I., and Johansen, E. (2005). The long and winding road from the research laboratory to industrial applications of lactic acid bacteria. FEMS Microbiol. Rev. 29, 611–624. doi: 10.1016/j.fmrre.2005.04.001
Pérez-Díaz, I. M., and McFeeters, R. F. (2009). Modification of azo dyes by lactic acid bacteria. J. Appl. Microbiol. 107, 584–589. doi: 10.1111/j.1365-2672.2009.04227.x
Rezaïki, L., Lamberet, G., Derré, A., Gruss, A., and Gaudu, P. (2008). Lactococcus lactis produces short-chain quinones that cross-feed Group B Streptococcus to activate respiration growth. Mol. Microbiol. 67, 947–957. doi: 10.1111/j.1365-2958.2007.06083.x
Sato, T., Schurgers, L. J., and Uenishi, K. (2012). Comparison of menaquinone-4 and menaquinone-7 bioavailability in healthy women. Nutr. J. 11:93. doi: 10.1186/1475-2891-11-93
Smid, E. J., and Kleerebezem, M. (2014). Production of aroma compounds in lactic fermentations. Annu. Rev. Food Sci. Technol. 5, 313–326. doi: 10.1146/annurev-food-030713-092339
Solem, C., Defoor, E., Jensen, P. R., and Martinussen, J. (2008). Plasmid pCS1966, a new selection/counterselection tool for lactic acid bacterium strain construction based on the oroP gene, encoding an orotate transporter from Lactococcus lactis. Appl. Environ. Microbiol. 74, 4772–4775. doi: 10.1128/AEM.00134-08
Van Cleave, C., Koehn, J. T., Pereira, C. S., Haase, A. A., Peters, B. J., Croslow, S. W., et al. (2021). Interactions of truncated menaquinones in lipid monolayers and bilayers. Int. J. Mol. Sci. 22:9755. doi: 10.3390/ijms22189755
Vermeer, C., and Schurgers, L. J. (2000). A comprehensive review of vitamin K and vitamin K antagonists. Hematol. Oncol. Clin. North Am. 14, 339–353. doi: 10.1016/S0889-8588(05)70137-4
Vizcaíno, J. A., Csordas, A., Del-Toro, N., Dianes, J. A., Griss, J., Lavidas, I., et al. (2016). 2016 update of the PRIDE database and related tools. Nucleic Acids Res. 44, D447–D456. doi: 10.1093/nar/gkv1145
Walther, B., Karl, J. P., Booth, S. L., and Boyaval, P. (2013). Menaquinones, bacteria, and the food supply: the relevance of dairy and fermented food products to vitamin K requirements. Adv. Nutr. 4, 463–473. doi: 10.3945/an.113.003855
Wegmann, U., O’Connell-Motherway, M., Zomer, A., Buist, G., Shearman, C., Canchaya, C., et al. (2007). Complete genome sequence of the prototype lactic acid bacterium Lactococcus lactis subsp. cremoris MG1363. J. Bacteriol. 189, 3256–3270. doi: 10.1128/JB.01768-06
Zheng, J., Wittouck, S., Salvetti, E., Franz, C. M. A. P., Harris, H. M. B., Mattarelli, P., et al. (2020). A taxonomic note on the genus Lactobacillus: description of 23 novel genera, emended description of the genus Lactobacillus Beijerinck 1901, and union of Lactobacillaceae and Leuconostocaceae. Int. J. Syst. Evol. Microbiol. 70, 2782–2858. doi: 10.1099/IJSEM.0.004107
Keywords: lactic acid bacteria, Lactococcus lactis, respiration, electron transport chain (ETC), comparative proteomics, food fermentation
Citation: Liu Y, Charamis N, Boeren S, Blok J, Lewis AG, Smid EJ and Abee T (2022) Physiological Roles of Short-Chain and Long-Chain Menaquinones (Vitamin K2) in Lactococcus cremoris. Front. Microbiol. 13:823623. doi: 10.3389/fmicb.2022.823623
Received: 27 November 2021; Accepted: 17 January 2022;
Published: 15 March 2022.
Edited by:
Vittorio Capozzi, Italian National Research Council, ItalyReviewed by:
Beatriz del Rio, Spanish National Research Council (CSIC), SpainMogens Kilstrup, Technical University of Denmark, Denmark
Copyright © 2022 Liu, Charamis, Boeren, Blok, Lewis, Smid and Abee. This is an open-access article distributed under the terms of the Creative Commons Attribution License (CC BY). The use, distribution or reproduction in other forums is permitted, provided the original author(s) and the copyright owner(s) are credited and that the original publication in this journal is cited, in accordance with accepted academic practice. No use, distribution or reproduction is permitted which does not comply with these terms.
*Correspondence: Eddy J. Smid, ZWRkeS5zbWlkQHd1ci5ubA==; Tjakko Abee, dGpha2tvLmFiZWVAd3VyLm5s