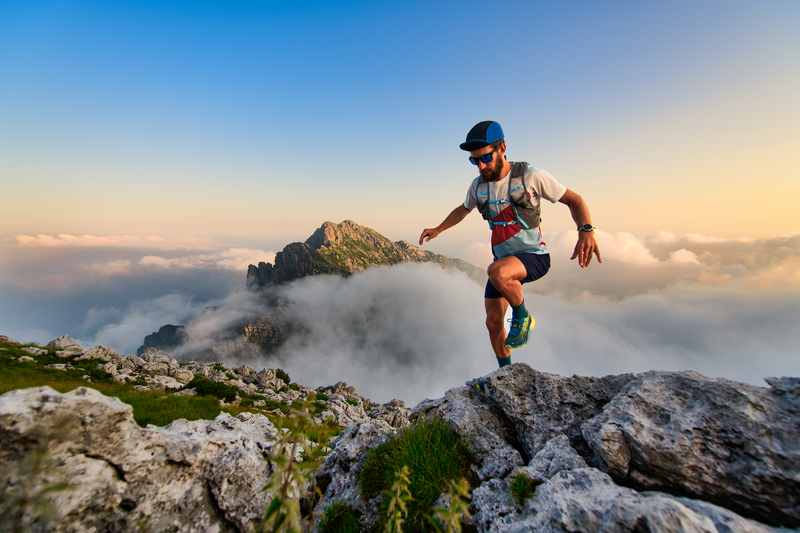
94% of researchers rate our articles as excellent or good
Learn more about the work of our research integrity team to safeguard the quality of each article we publish.
Find out more
ORIGINAL RESEARCH article
Front. Microbiol. , 23 May 2022
Sec. Food Microbiology
Volume 13 - 2022 | https://doi.org/10.3389/fmicb.2022.823581
Lipids are essential energy storage compounds and are the core structural elements of all biological membranes. During wine alcoholic fermentation, the ability of yeasts to adjust the lipid composition of the plasma membrane partly determines their ability to cope with various fermentation-related stresses, including elevated levels of ethanol and the presence of weak acids. In addition, the lipid composition of grape juice also impacts the production of many wine-relevant aromatic compounds. Several studies have evaluated the impact of lipids and of their metabolism on fermentation performance and aroma production in the dominant wine yeast Saccharomyces cerevisiae, but limited information is available on other yeast species. Thus, the aim of this study was to evaluate the influence of specific fatty acid and sterol mixtures on various non-Saccharomyces yeast fermentation rates and the production of primary fermentation metabolites. The data show that the response to different lipid mixtures is species-dependent. For Metschnikowia pulcherrima, a slight increase in carbon dioxide production was observed in media enriched with unsaturated fatty acids whereas Kluyveromyces marxianus fermented significantly better in synthetic media containing a higher concentration of polyunsaturated fatty acids than monounsaturated fatty acids. Torulaspora delbrueckii fermentation rate increased in media supplemented with lipids present at an equimolar concentration. The data indicate that these different responses may be linked to variations in the lipid profile of these yeasts and divergent metabolic activities, in particular the regulation of acetyl-CoA metabolism. Finally, the results suggest that the yeast metabolic footprint and ultimately the wine organoleptic properties could be optimized via species-specific lipid adjustments.
Non-Saccharomyces yeasts have become valuable in the wine industry due to their potential to diversify wine flavor profiles and/or address specific (bio)technological challenges (Capozzi et al., 2015; Padilla et al., 2016; Rossouw and Bauer, 2016; Furlani et al., 2017). Despite these benefits, these yeasts cannot fully replace the common wine yeast Saccharomyces cerevisiae as they fail to ferment to completion. Multi-starter or sequential fermentations with S. cerevisiae are therefore required to ensure the complete utilization of all sugars (Bely et al., 2008; Gobbi et al., 2013; Taillandier et al., 2014). Indeed, a successful fermentation requires a yeast strain that is resistant to osmotic pressure and to the accumulation of ethanol and other toxic molecules (weak acids, antimicrobial peptides, etc.) as well as oxygen limitation (Bauer and Pretorius, 2000; Rossignol et al., 2003; Shima and Takagi, 2009; Tronchoni et al., 2017). The ability of yeast species to respond to these constraints determines the extent of their survival and overall fermentation performance (Mannazzu et al., 2008; Rodríguez-Porrata et al., 2011; Oro et al., 2014; Curiel et al., 2017).
Lipids play a major role in mediating yeast stress response. Indeed, lipids such as sterols, phospholipids and sphingolipids are essential components of the yeast plasma membrane. Adjustments in the lipid composition of this relatively impermeable barrier enables the yeast cell to adjust to various fermentation conditions such as ethanol accumulation, low pH and supports the transport of specific molecules via a vast array of transport proteins (Van der Rest et al., 1995). Adjustments also include changes in the relative concentration of phospholipids, sterols and the saturation of fatty acids. However, the production of ergosterol, the main yeast sterol, and the desaturation of fatty acids require oxygen, an element in limited supply during self-anaerobic fermentations (Valero et al., 2002; Mannazzu et al., 2008; Archana et al., 2015).
During the early stages of wine alcoholic fermentation, yeasts synthesize ergosterol and unsaturated fatty acids when oxygen is present (Stukeys et al., 1990; Belviso et al., 2004; Liu et al., 2019). As fermentation progresses, conditions become rapidly anaerobic, and the lack of oxygen prevents the necessary modifications to the cell lipid composition to ensure yeast survival (Rosenfeld et al., 2003). Indeed, in S. cerevisiae, alcoholic fermentation in the absence of oxygen and exogenous lipids leads to an enrichment in saturated fatty acids, a decrease in the unsaturated fatty acid as well as sterol concentration. Furthermore, enhanced production of medium chain fatty acids is also observed under anaerobiosis, and these impacts may lead to reduced fermentation efficiency and sluggish or stuck fermentation (Bardi et al., 1999). An efficient fermentation therefore requires either the uptake of exogenous lipids or the presence of oxygen (Delfini et al., 1993; Burin et al., 2015; Houtman and Du Plessis, 2017).
Standard wine making practices impact lipid metabolism and composition. Indeed, yeast-derived fermentation additives (referred to as complex nutrients) containing significant lipid concentrations typically used in the rehydration of yeasts directly reinforce membrane integrity and support fermentative activity (Valero et al., 1998, 2001; Belviso et al., 2004; Varela et al., 2012; Ochando et al., 2017). Furthermore, aeration is used in winemaking to enhance or reactivate yeast viability or metabolism (Shekhawat et al., 2017). Indeed, the micro-oxygenation of fermenting grape must has been shown to increase yeast growth and performance during alcoholic fermentation due to the reactivation of lipid biosynthesis in Torulaspora delbrueckii and S. cerevisiae (Mauricio et al., 1998). Other authors showed that the cultivation of Candida stellata, T. delbrueckii, Hanseniaspora uvarum, Hanseniaspora guilliermondii and Debaryomyces hansenii under aerobic conditions impacts ethanol stress resistance (Pina et al., 2004). Oxygen sparging also influences the production of higher alcohols, medium fatty acids and esters during alcoholic fermentation (Fujii et al., 1997; Varela et al., 2012; Ochando et al., 2017). Although oxygen was observed to inhibit acetate ester synthesis in S. cerevisiae via the inhibition of the alcohol acetyl transferase ATF1 and repressed ethyl ester production, an overall increase of ester production has been attributed to improved biomass formation (Mauricio et al., 1997; Varela et al., 2012; Ochando et al., 2017).
The addition of individual or defined mixtures of lipids including ergosterol and unsaturated fatty acids such as palmitoleic acid, oleic acid, linoleic and linolenic acid improves yeast performance during alcoholic fermentation (Ohta and Hayashida, 1983; Casu et al., 2016; Ochando et al., 2017; Fairbairn et al., 2019). However, the impact of these compounds on yeast survival and performance is concentration- and condition-dependent (You et al., 2003; Fairbairn et al., 2019; Liu et al., 2019). Furthermore, adjusting the lipid content via direct supplementation impacts the production of certain volatile compounds during alcoholic fermentation (Varela et al., 2012; Duan et al., 2015; Liu et al., 2018, 2020; Fairbairn et al., 2019). For instance, acetic acid production was observed to decrease after the addition of phytosterols and unsaturated fatty acids in S. cerevisiae, possibly due to a reduced need for acetyl-CoA, the main substrate of lipid metabolism. However, an increase in acetic acid was observed when higher amounts of phytosterols and unsaturated fatty acids were used and this was attributed to an increase in yeast biomass (Luparia et al., 2004; Ochando et al., 2017; Deroite et al., 2018). Moreover, certain species-specific responses have also been reported. Indeed, a previous study showed that the low levels of acetic acid observed in M. pulcherrima fermentations may result from a limitation of acetyl-CoA which also led to a reduction in medium chain fatty acids and their corresponding ethyl esters (Seguinot et al., 2020). However, the lipid composition of this yeast was not investigated. In this context, more studies are necessary to evaluate the utilization of lipids and their impact on yeast performance during alcoholic fermentation in non-Saccharomyces yeasts.
The aim of this study was to evaluate the impact of fatty acids and sterols on yeast fermentation performance and to determine whether the impact of these compounds on yeast performance is species-dependent. The impact of different lipid ratios and concentrations on yeast performance was evaluated in selected yeast strains belonging to three yeast species, namely M. pulcherrima, T. delbrueckii and K. marxianus.
The following yeast strains were used in the study: a Metschnikowia pulcherrima yeast strain (Strain A), a Torulaspora delbrueckii yeast strain (Strain B) and a Saccharomyces cerevisiae strain (Lalvin EC1118®) from Lallemand SA (Montreal, Canada) and Kluyveromyces marxianus (IWBT Y885). The latter yeast belongs to the yeast collection of the South African Grape and Wine Research Institute, Stellenbosch University, South Africa.
Fermentations were conducted in synthetic grape juice-like medium containing 100 g/l glucose, 100 g/l fructose, 2.5 g/l tartaric acid, 3 g/l malic acid, 0.2 g/l citric acid, 1.14 g/l potassium phosphate dibasic, 1.23 g/l magnesium sulfate heptahydrate, 0.44 g/l calcium chloride dihydrate, supplemented with vitamins, trace elements and 300 mg/l Yeast Assimilable Nitrogen (with amino acids and ammonium chloride) as shown in Table 1 (Bely et al., 1990; Henschke and Jiranek, 1993).
Table 1. Trace elements, vitamins and nitrogen stock (with amino acids and ammonium chloride) used to prepare grape juice-like synthetic medium.
Freeze cultures were streaked onto Yeast Peptone Dextrose (YPD) Agar (Merck, Fontenay-sous-Bois, France) plates and incubated at 30°C for 48 h. A single colony was inoculated into 5 ml Yeast Nutrient Base (YNB) medium with 20 g/l sugar for 24 h. A 1 ml culture was then transferred into 100 ml YNB and incubated until the yeast populations reached late exponential growth under semi-aerobic conditions in an Erlenmeyer flask with 70% headspace. Yeast cultures were harvested, centrifuged at 4,193 g for 5 min, washed with saline solution (0.9% NaCl), inoculated into 250 ml synthetic medium containing myristic, palmitic, palmitoleic, stearic, oleic, linoleic, linolenic acid and ergosterol (Sigma, Saint-Quentin-Fallavier, France) in different combinations as shown in Table 2 and incubated at 25°C with agitation at 120 rpm. The fatty acids used in this study are commonly found in grape juice and the cell pellet. Ergosterol was used because it is the main sterol found in yeasts. Medium A contained equimolar concentrations of lipids, Medium B an equimolar lipid mixture with double the amount of unsaturated fatty acids and Medium C a grape juice-like lipid mixture based on previous studies (Yunoki et al., 2005; Tumanov et al., 2015). Fermentations were conducted in a 250-ml bottle with 10% headspace under anaerobic conditions. Dissolved oxygen was removed by sparging argon for 25 min prior to the addition of lipid mixtures. In order to evaluate the fatty acid and sterol composition of yeasts, self-evolving fermentations were performed in synthetic grape juice-like media without the addition of lipids. Yeasts were pre-cultured and inoculated as previously described and the fermentations were also incubated at 25°C.
Yeast fermentation rate (carbon dioxide production) was monitored throughout fermentation via weight loss. Samples were harvested when S. cerevisiae fermentations became complete (this will be termed “end of alcoholic fermentation” for all strains in this study). The yeast population at the end of fermentation was determined using a Counter ZB-2 (Beckman Coulter, Villepinte, France). Residual sugars, ethanol, glycerol, and fermentation derived acids were also analyzed when fermentations were terminated using High Performance Liquid Chromatography or HPLC (HPLC 1290 Infinity, Agilent Technologies, California, United States) with a Phenomenex Rezex ROA column (Agilent Technologies California, United States). Sulphuric acid or H2SO4 (0.005 mol/l, Merck, France) was used as a mobile phase with a flow rate of 0.6 ml/min at 60°C. The EZChrom software package was used for data analysis (Seguinot et al., 2020).
Similar to the previous fermentation, yeast performance was also monitored via weight loss. Samples were harvested when S. cerevisiae completed fermentation (also termed “end of alcoholic fermentation” for all strains in this study) and centrifuged at 4,193 g. The supernatant was discarded and the yeast pellet was stored at −80°C. Fatty acids and sterols were extracted from the yeast pellet using the protocol described by Williams et al. (2021).
Fermentations were performed in triplicate (with the exception of K. marxianus in Medium A) and the impact of lipid mixtures on fermentation parameters, primary metabolite production and yield was evaluated by using ANOVA in XLSTAT (p < 0.05). A Bonferroni corrected test with a modified significance level of 0.017 showed differences in some lipid treatments. The cellular fatty acid and sterol profile of yeasts was also analyzed using ANOVA and the significance was also corrected via a Bonferroni test. Furthermore, the cellular lipid profile of yeasts was analyzed using Principal Component Analysis (XLSTAT). A hierarchical cluster analysis was performed to analyze different groups of primary fermentation metabolite yields in XLSTAT.
Yeasts were pre-cultured in YNB medium for 18 h under semi-aerobic conditions (with 70% headspace) and harvested at the late exponential growth phase for inoculation into three synthetic grape juice-like media differing only in lipid composition (Table 2): Medium A contained equimolar concentrations of lipids, Medium B had double the amount of unsaturated fatty acids and Medium C contained a lipid mixture reflecting averages reported for natural grape juice (Yunoki et al., 2005; Tumanov et al., 2015). Medium B was used to determine whether the presence of higher amounts of unsaturated fatty acids improves fermentation performance compared to Medium A. On the other hand, Medium C was used to determine how the different yeast species perform in grape juice-like conditions based on averages reported for natural grape juice (Yunoki et al., 2005; Tumanov et al., 2015) when compared to the other two media. Fermentations were terminated when S. cerevisiae completed alcoholic fermentation (termed “end of alcoholic fermentation” for all strains in this study). The influence of the lipid treatments on yeast fermentation rate and yeast population dynamics are shown in Figures 1, 2. Overall and regardless of the lipid treatments, the lowest yeast population and carbon dioxide production was observed in M. pulcherrima followed by K. marxianus, T. delbrueckii and S. cerevisiae (Figures 1, 2). However, lipid ratios impacted yeast performances in a strain-specific manner. Fermentations in Medium C led to a significantly higher carbon dioxide production in K. marxianus at the end of alcoholic fermentation whereas maximal carbon dioxide production was significantly higher in Medium A when compared to Medium C in T. delbrueckii. Although not significant, M. pulcherrima displayed a slightly higher maximal carbon dioxide production in Medium B. The maximal fermentation rate was also higher in Medium C and A for K. marxianus and T. delbrueckii, respectively (although not significantly for K. marxianus). Interestingly, the yeast cell count was significantly higher in Medium A when compared to Medium C in K. marxianus and T. delbrueckii whereas no significant differences were observed in M. pulcherrima. In this study, minimal differences were observed in the yeast cell count, carbon dioxide production and fermentation rate between lipid treatments in S. cerevisiae.
Figure 1. Carbon dioxide production (weight loss) in M. pulcherrima (A), K. marxianus (B), T. delbrueckii (C) and S. cerevisiae (D) in Medium A (green), Medium B (blue) and Medium C (red).
Figure 2. Maximal carbon dioxide or CO2 max production (A), maximal fermentation rate (B) and yeast cell count (C) in M. pulcherrima, K. marxianus, T. delbrueckii and S. cerevisiae in Medium A, Medium B and Medium C. Blue bars-M. pulcherrima in Medium A (Mp A), B (Mp B) and C (Mp C). Pink bars—K. marxianus in Medium A (Km A), B (Km B) and C (Km C). Green bars—T. delbrueckii in Medium A (Td A), B (Td B) and C (Td C). Orange bars—S. cerevisiae in Medium A (Sc A), B (Sc B) and C (Sc C). Results obtained for treatments with the same letters are not statistically significant (p < 0.05).
At the end of fermentation, residual sugars and primary metabolites were quantified (Table 3). Overall and regardless of lipid treatments, the highest residual sugar concentrations were observed in M. pulcherrima, followed by K. marxianus, T. delbrueckii and S. cerevisiae (which was the only strain to ferment to dryness) whereas the opposite trend was observed with ethanol. The highest glycerol concentrations were also observed in S. cerevisiae, followed by K. marxianus, T. delbrueckii and M. pulcherrima. Interestingly, T. delbrueckii produced the lowest amount of acetic acid followed by M. pulcherrima, K. marxianus and S. cerevisiae. Although M. pulcherrima fermented poorly, the highest alpha-ketoglutarate levels were observed in this yeast regardless of lipid treatments followed by S. cerevisiae, K. marxianus and T. delbrueckii. The highest pyruvate concentrations were observed in K. marxianus followed by M. pulcherrima, T. delbrueckii and S. cerevisiae. The overall highest succinic acid concentration was also observed in K. marxianus followed by T. delbrueckii, S. cerevisiae and M. pulcherrima. In S. cerevisiae, the presence of different lipid mixtures did not significantly impact sugar consumption and metabolite production. On the other hand, differences were observed in the residual sugar and metabolite concentrations for non-Saccharomyces yeasts due to lipid additions. Indeed, in T. delbrueckii, the lowest residual sugar concentration was observed in Medium A (96 g/l) when compared to Medium B and C (with residual sugar concentrations of 117 and 114 g/l, respectively). The lower residual sugar concentration in Medium A resulted in significantly higher amounts of ethanol, glycerol, α-ketoglutarate and acetic acid when compared to Medium C for T. delbrueckii. In K. marxianus, the lowest residual sugar concentration was observed in Medium C (96 g/l) when compared to Medium A and B (with residual sugar concentrations of 129 and 128 g/l, respectively). Consequently, ethanol, glycerol, acetic acid and succinic acid production was significantly higher in Medium C for this yeast. Compared to the other two lipid treatments, the residual sugar concentration was significantly lower in Medium B (with 132 g/l) for M. pulcherrima when compared to Medium A and C (with a residual sugar concentration of 155 and 151 g/l, respectively) resulting in a significantly higher ethanol concentration. Although the glycerol, acetic acid and succinate concentration was higher in Medium B for M. pulcherrima, it was not significant. On the other hand, the α-ketoglutarate concentration and pyruvate concentrations were significantly higher in Medium B for this yeast. Since fermentations were terminated at different stages, the concentration of metabolites deriving from central carbon metabolism and the Krebs cycle were normalized against sugar consumption (Figure 3). The pyruvate yield was significantly lower in media that resulted in the highest carbon dioxide production in M. pulcherrima and K. marxianus (Mp A and Km C) and the low pyruvate yields were linked to a significantly higher ethanol yield in these yeasts. Although acetate and succinate yields were highest in K. marxianus no significant differences were observed between lipid treatments. Furthermore, the lipid mixtures used in this study did not significantly impact acetic and succinic acid yields in the other yeasts. The highest α-ketoglutarate yield was observed in M. pulcherrima and some differences were observed between lipid treatments. In particular, the α-ketoglutarate yield was significantly higher in Medium A for M. pulcherrima when compared to Medium C. On the other hand, fermentations in Medium B resulted in a significantly higher α-ketoglutarate yield for K. marxianus.
Table 3. Residual sugars and fermentation derived metabolite production in Medium A containing an equimolar lipid composition, Medium B with double the amount of unsaturated fatty acids and Medium C with a grape juice-like lipid composition when fermentations were terminated (g/l).
Figure 3. Fermentation derived metabolites yields in media containing different lipid combinations. Samples were harvested when fermentations were terminated and yields were calculated by normalizing the metabolites produced by sugar consumption (mM/M sugar consumed). Blue bars-M. pulcherrima in Medium A (Mp A), B (Mp B) and C (Mp C). Pink bars—K. marxianus in Medium A (Km A), B (Km B) and C (Km C). Green bars—T. delbrueckii in Medium A (Td A), B (Td B) and C (Td C). Orange bars—S. cerevisiae in Medium A (Sc A), B (Sc B) and C (Sc C). Results obtained for treatments with the same letters are not statistically significant (p < 0.05).
Fermentation-derived metabolite yields in synthetic grape-juice with different lipid mixtures were visually evaluated using Hierarchical Cluster Analysis (HCA) as shown in Figure 4. The relative abundance of metabolites was group dependent. Regardless of lipid mixtures, M. pulcherrima fermentations were characterized by a higher abundance of alpha-ketoglutarate and pyruvate. An increase in the relative abundance of acetic acid and succinic acid was observed for K. marxianus fermentations. T. delbrueckii fermentations were characterized by a strong reduction in the relative abundance of acetic acid whereas the relative abundance of ethanol was strongly enhanced in S. cerevisiae. For non-Saccharomyces yeast in this study, the relative abundance of ethanol and glycerol increased in media that resulted in the highest carbon dioxide production (Medium B for M. pulcherrima, Medium C for K. marxianus and Medium A for T. delbrueckii) but the enhancement in the relative abundance of these metabolites varied between strains.
Figure 4. Hierarchical cluster analysis of fermentation derived metabolites yields (mM/M sugar consumed) grouped based on chemical properties and yeasts.
In order to better understand the impact of the three lipid mixtures reported above, the lipid composition of the four yeast strains was determined. Yeasts were inoculated into synthetic grape juice-like medium with no exogenous lipids and self-anaerobic fermentations were performed. Following the trends displayed above, the highest carbon dioxide production was observed in S. cerevisiae followed by T. delbrueckii, K. marxianus and M. pulcherrima (Figure 5A). Samples were harvested at the end of fermentation for cellular lipid analysis as shown in Figure 5B. Overall, stearic and palmitic acid were the main fatty acids present in all yeasts at the end of alcoholic fermentation, with very little differences between species. However, some differences were observed for the other lipids. For instance, M. pulcherrima produced significantly higher levels of linolenic, linoleic and oleic acids. On the other hand, palmitoleic acid level was significantly higher in K. marxianus while T. delbrueckii and S. cerevisiae produced significantly higher amounts of squalene when fermentations were terminated. The fatty acid and sterol profile of yeasts was visually evaluated using Principal Component Analysis (PCA) as shown in Figure 5C. Factor 1, representing 55.7% of the total variance, showed positive loadings for stearic acid, ergosterol, oleic, linoleic and linolenic acid whereas the opposite was observed for squalene, palmitoleic, palmitic and myristic acid. Factor 2, representing 34.1% of the variance, showed a positive loading for squalene, stearic and linolenic acid. S. cerevisiae fermentations clustered separately from the non-Saccharomyces yeast fermentations due to high amounts of squalene whereas M. pulcherrima clustered separately from the other yeasts due to the high levels of linoleic and linolenic acid. K. marxianus and T. delbrueckii clustered closely together due to palmitoleic, palmitic and myristic acid.
Figure 5. The carbon dioxide production (A), cellular lipid profile (B) and principal component analysis biplot (C) of M. pulcherrima, K. marxianus, T. delbrueckii and S. cerevisiae at the end of alcoholic fermentation in synthetic grape juice-like media with no lipids. Blue bars-M. pulcherrima, pink bars—K. marxianus, green bars—T. delbrueckii and orange bars—S. cerevisiae. Results obtained for treatments with the same letters are not statistically significant (p < 0.05).
This study evaluated the impact of lipid mixtures on yeast performance during alcoholic fermentation. Regardless of the lipid treatment, the lowest carbon dioxide production was observed in M. pulcherrima followed by K. marxianus, T. delbrueckii and S. cerevisiae when fermentations were terminated. Similar to previous studies, M. pulcherrima fermented poorly (Shekhawat et al., 2017; Puškaš and Miljić, 2020) whereas S. cerevisiae fermented to completion (i.e., consumed all the sugars) leading to the highest ethanol, glycerol and acetic acid levels. On the other hand, T. delbrueckii produced moderate amounts of glycerol and the lowest acetic acid concentration, as previously reported (Renault et al., 2009; Mbuyane et al., 2018; Zhang et al., 2018) and the addition of different lipid mixtures did not alter the low acetic acid production trait of this yeast. This may indicate that acetic acid production in this yeast is impacted by other regulatory mechanisms that are not related to lipid metabolism or that the lipid concentrations used in this study did not significantly impact acetyl-CoA metabolism in T. delbrueckii. No significant differences were observed in S. cerevisiae following the addition of different lipid treatments. Interestingly, in a previous study, S. cerevisiae EC1118 was observed to be less sensitive to changes in the concentration of palmitic, oleic, linoleic and linolenic acid mixtures when compared to another strain (Liu et al., 2020). Similarly, this yeast may be less sensitive to the lipid concentrations and mixtures used in this study but may respond to higher lipid concentrations under more stringent fermentation conditions (i.e., higher sugar concentrations). So, the results obtained in this study for strain EC1118 may not be generalized to all strains of the species S. cerevisiae. Furthermore, fermentation conditions (such as the sugar concentration, oxygen conditions and type of lipid/s used) may cause discrepancies as previous studies have reported differences in EC1118 fermentation rate and metabolite production in response to different lipid mixtures (Duan et al., 2015; Liu et al., 2019).
This study shows for the first time that the addition of lipid mixtures impacts non-Saccharomyces yeast fermentation performance in a species-dependent manner, to a much greater extent than S. cerevisiae. Indeed, the fermentation of the three non-Saccharomyces yeasts investigated were improved when exposed to specific lipid mixtures. Although still fermenting poorly compared to the other species, M. pulcherrima performed somewhat better in the medium containing the highest level of unsaturated fatty acids and the data in this study also showed M. pulcherrima accumulated higher levels of unsaturated fatty acids in synthetic grape juice media with no lipids when compared to other yeasts. This indicates that this strain may have a higher demand for lipids innately found in the plasma membrane (i.e., unsaturated fatty acids) in an attempt to maintain membrane balance under conditions of stress. Indeed, since the accumulation of ethanol affects plasma membrane integrity, denatures important enzymes responsible for glycolysis and may affect nutrient uptake (Santos et al., 2008; Ma and Liu, 2010), the presence of unsaturated fatty acids in Medium B may have contributed to lowering ethanol sensitivity in this particularly sensitive species. Further studies are required to determine if M. pulcherrima has transport mechanisms different from S. cerevisiae which favor the uptake and/or utilization of unsaturated fatty acids. In K. marxianus, the highest fermentation rate was observed in Medium C with the highest concentration of palmitic acid and contained a higher total polyunsaturated fatty acid content (linoleic and linolenic) than the total monounsaturated fatty acid content (palmitoleic and oleic acid). Interestingly, K. marxianus produced the highest amount of palmitic acid and accumulated higher levels of monounsaturated fatty acids than polyunsaturated fatty acids when cultured in medium with no lipids. The data therefore suggests that the yeast lipid composition may determine the impact of exogenous fatty acids and sterols on yeast fermentation performance. However, further studies are required to confirm this observation in different strains. The highest carbon dioxide production in T. delbrueckii was observed in Medium A which contained an equimolar lipid mixture. Since T. delbrueckii accumulated intermediate concentrations of unsaturated fatty acids, this yeast may prefer media with a balanced lipid mixture. While the addition of unsaturated fatty acids was observed to increase ethanol shock resistance in T. delbrueckii in a previous study, (Pina et al., 2004) the polyunsaturated fatty acid cell content of T. delbrueckii was linked to ethanol sensitivity whereas the high ergosterol and monounsaturated fatty acid content of S. cerevisiae strains was linked to ethanol resistance (Aguilera et al., 2006) in another study. T. delbrueckii may therefore require media that contains all the necessary lipids required for anaerobic growth at an equimolar concentration in order to maintain plasma membrane structure/balance but further investigations are necessary to confirm this. Recently, it was observed that genes homologous to the sterol transporters AUS1 and PDR11 are absent in T. delbrueckii, M. pulcherrima, K. marxianus and other non-Saccharomyces yeasts (Dekker et al., 2021; Tesnière et al., 2021). Therefore, these yeasts may be more sensitive to changes in the fatty acid profile of the cell and may require specific fatty acid combinations for an improvement in fermentation performance especially under anaerobiosis.
This study also shows that the addition of specific lipid mixtures impacts the production of fermentation derived metabolites in non-Saccharomyces yeasts. An increase in the yeast fermentation rate led may lead to a higher utilization of pyruvate, an increase in ethanol and glycerol production (Erasmus et al., 2004; Varela et al., 2012; Goold et al., 2017; Casu et al., 2018). Although this was observed in all non-Saccharomyces yeasts, the increase in ethanol and/or glycerol was not always significant indicating that yeasts may require higher lipid concentrations or the presence of outliers (caused by oxygen ingress which affects metabolite production) may have minimized the effects of lipid mixtures.
Acetic acid is produced via the pyruvate dehydrogenase bypass whereby pyruvate is converted into acetaldehyde by a decarboxylase followed by the formation of this acid in a reaction catalyzed by an aldehyde dehydrogenase (Remize and Andrieu, 2000; Pigeau and Inglis, 2005, 2007). The pyruvate dehydrogenase bypass is closely linked to lipid metabolism because the resulting acetic acid produced in this pathway can be converted into acetyl-CoA which is an important building block for lipid biosynthesis (Van den Berg and Steensma, 1995; Kozak et al., 2014; Seguinot et al., 2020). Indeed, the presence of lipids was observed to decrease acetic acid production due to a reduced need for the carbon flux to be directed toward acetyl-CoA for lipid production (Luparia et al., 2004; Ochando et al., 2017; Deroite et al., 2018; Liu et al., 2020). On the other hand, excess unsaturated fatty acid concentrations were observed to increase acetic acid production in S. cerevisiae (Fairbairn et al., 2019; Liu et al., 2019). In this study, the presence of exogenous fatty acids and ergosterol did not significantly impact the acetate yield potentially due to inadequate concentrations being utilized, poor growth (in the case of M. pulcherrima) or the conditions not being stringent enough to elicit a reaction (in the case of S. cerevisiae). The highest acetic acid yield was observed in K. marxianus regardless of lipid treatments, probably as a result of this yeast’s higher carbon flux toward acetyl-CoA biosynthesis (Sakihama et al., 2019). This further highlights that the concentration of lipid mixtures utilized in this study was perhaps not enough to fulfill the acetyl-CoA demand of K. marxianus necessary for biosynthetic reactions. K. marxianus also produced the highest succinate levels in this study possibly due to the high flux toward acetyl-CoA synthesis which may enter into the TCA cycle (Sakihama et al., 2019).
Following the formation of acetyl-CoA, the pyruvate dehydrogenase bypass ends with shuffling this building block into the mitochondria via a carnitine acetyl transferase for use in the TCA cycle (Van Rossum et al., 2016). The TCA pathway operates as two branches during alcoholic fermentation instead of one complete cycle. While both branches ultimately lead to succinate accumulation, the oxidative branch occurs via the formation of α -ketoglutarate whereas the reductive branch is active via fumarate formation. In S. cerevisiae, the enzymatic activity of the oxidative branch was reported to be dysfunctional or minimal under anaerobic conditions and functional when glutamate was used as the main source of nitrogen (Pronk et al., 1996; Rodrigues et al., 2012). On the other hand, the reductive branch is known to be active during alcoholic fermentation as indicated by the activity of fumarate reductase (Camarasa et al., 2003). Furthermore, the biosynthetic reactions of the TCA cycle are also known to recycle reducing equivalents whereby NAD+ is converted to NADH (Chidi et al., 2018). Alpha-ketoglutarate is also integral in yeast nitrogen metabolism as it is utilized in the first step of the Ehrlich pathway to produce glutamate using NH3 in an NADP+-dependent reaction catalyzed by glutamate dehydrogenase. Thereafter, glutamate is converted into glutamine using NH3 in a reaction catalyzed by glutamine synthase (Hirst and Richter, 2016). On the other hand, glutamine can also be converted back into glutamate and finally α -ketoglutarate for utilization in the TCA cycle in a series of reactions that require glutaminases, NAD+-dependent glutamate dehydrogenases and yield NH3 (Guillamo and Verrips, 2001). In this study, an inverse relationship between α -ketoglutarate and succinate biosynthesis was observed potentially due to a redox balance regulation in the three non-Saccharomyces yeasts. Furthermore, M. pulcherrima produced higher amounts of α -ketoglutarate and lower levels of succinate whereas the opposite was observed in the other yeast strains. This may indicate that this yeast regulates its biosynthetic reactions and redox balance using mechanisms different from the other yeasts. Alternatively, M. pulcherrima may require less amino acids (such as glutamate which is produced from α -ketoglutarate) than other yeasts in this study due to low growth. Thus, excess α -ketoglutarate would be excreted into the environment. Further investigations are necessary to determine α -ketoglutarate antioxidant properties in yeasts as this metabolite was reported to protect against lipid peroxidation (Bayliak et al., 2017).
This study shows that while minimal differences were observed in the fermentation rate of the specific strain of S. cerevisiae investigated, significant differences were observed in the production of carbon dioxide in response to different lipid ratios in selected non-Saccharomyces yeasts (in particular, K. marxiianus). However, the impact of different lipid ratios on the biosynthesis of fermentation-derived products was not always clear. This study shows that the yeast lipid profile is species-dependent and that the impact of exogenous lipids on yeast fermentation performance may be dependent on the yeast lipid composition. Moreover, the species-specific response to the different lipid mixtures could be linked to acetyl-CoA availability and the regulation of redox balance via the TCA cycle as well as acetyl-CoA metabolism. However, further investigations are required to evaluate the impact of exogenous lipids on a broader range of fermentation derived metabolites (such as medium chain fatty acids, ethyl and acetate esters) in order to understand non-Saccharomyces yeast lipid metabolism during winemaking. Although the addition of lipids may not result in complete fermentations in non-Saccharomyces yeasts, these compounds may increase the fermentation performance and ultimately their metabolic footprint in wine.
The original contributions presented in the study are included in the article/supplementary material, further inquiries can be directed to the corresponding author/s.
LM performed the experiments, analyzed the data, and wrote the manuscript. AB, CC, FB, and BD conceived the experiments, supervised the experimental work, assisted in the interpretation of the data, and edited the manuscript. AO-J provided inputs in the design of the experiments and discussion of the data. All authors have contributed and agreed to the submitted version of the manuscript.
This work was based on the research supported by the National Research Foundation of South Africa (Grant Nos. 118110, 130518, and 83471) and by Lallemand Oenology.
AO-J was employed by Lallemand SAS.
The remaining authors declare that the research was conducted in the absence of any commercial or financial relationships that could be construed as a potential conflict of interest.
All claims expressed in this article are solely those of the authors and do not necessarily represent those of their affiliated organizations, or those of the publisher, the editors and the reviewers. Any product that may be evaluated in this article, or claim that may be made by its manufacturer, is not guaranteed or endorsed by the publisher.
We would like to thank Montpellier SupAgro for providing the infrastructure and equipment for performing experiments. We would also like to thank the National Research Foundation of South Africa and Lallemand ænologie for providing funding.
Aguilera, F., Peinado, R. A., Millán, C., Ortega, J. M., and Mauricio J. C. (2006). Relationship between ethanol tolerance, H+-ATPase activity and the lipid composition of the plasma membrane in different wine yeast strains. Int. J. Food Microbiol. 110, 34–42. doi: 10.1016/j.ijfoodmicro.2006.02.002
Archana, K. M., Ravi, R., and Anu-Appaiah, K. A. (2015). Correlation between ethanol stress and cellular fatty acid composition of alcohol producing non-Saccharomyces in comparison with Saccharomyces cerevisiae by multivariate techniques. J. Food Sci. Technol. 52, 6770–6776. doi: 10.1007/s13197-015-1762-y
Bardi, L., Cocito, C., and Marzona, M. (1999). Saccharomyces cerevisiae cell fatty acid composition and release during fermentation without aeration and in absence of exogenous lipids. Int. J. Food Microbiol. 47, 133–140. doi: 10.1016/s0168-1605(98)00203-7
Bauer, F. F., and Pretorius, I. S. (2000). Yeast stress response and fermentation efficiency: How to survive the making of wine - A review. S. Afr. J. Enol. Vitic. 21, 27–51. doi: 10.21548/21-1-3557
Bayliak, M., Burdyliuk, N., and Lushchak, V. (2017). Growth on alpha-ketoglutarate increases oxidative stress resistance in the yeast Saccharomyces cerevisiae. Int. J. Microbiol. 2017:5792192. doi: 10.1155/2017/5792192
Belviso, S., Bardi, L., Bartolini, A. B., and Marzona, M. (2004). Lipid nutrition of Saccharomyces cerevisiae in winemaking. Can. J. Microbiol. 50, 669–674. doi: 10.1139/w04-051
Bely, M., Sablayrolles, J.-M., and Barre, P. (1990). Automatic detection of assimilable nitogen deficiencies during alcoholic fermentation in enological conditions. J. Ferment. Bioeng. 70, 246–252. doi: 10.1016/0922-338X(90)90057-4
Bely, M., Stoeckle, P., Masneuf-Pomarede, I., and Dubourdieu, D. (2008). Impact of mixed Torulaspora delbrueckii-Saccharomyces cerevisiae culture on high-sugar fermentation. Int. J. Food Microbiol. 122, 312–320. doi: 10.1016/j.ijfoodmicro.2007.12.023
Burin, V. M., Gomes, T. M., Caliari, V., Rosier, J. P., and Bordignon Luiz, M. T. (2015). Establishment of influence the nitrogen content in musts and volatile profile of white wines associated to chemometric tools. Microchem. J. 122, 20–28. doi: 10.1016/j.microc.2015.03.011
Camarasa, C., Grivet, J., and Dequin, S. (2003). Investigation by 13C-NMR and tricarboxylic acid (TCA) deletion mutant analysis of pathways of succinate formation in Saccharomyces cerevisiae during anaerobic fermentation. Microbiology 149, 2669–2678. doi: 10.1099/mic.0.26007-0
Capozzi, V., Garofalo, C., Chiriatti, M. A., Grieco, F., and Spano, G. (2015). Microbial terroir and food innovation: the case of yeast biodiversity in wine. Microbiol. Res. 181, 75–83. doi: 10.1016/j.micres.2015.10.005
Casu, F., Pinu, F. R., Fedrizzi, B., Greenwood, D. R., and Villas-boas, S. G. (2016). The effect of linoleic acid on the Sauvignon blanc fermentation by different wine yeast strains. FEMS Yeast Res. 16:foy050. doi: 10.1093/femsyr/fow050
Casu, F., Pinu, F. R., Stefanello, E., Greenwood, D. R., and Villas-Bôas, S. G. (2018). The fate of linoleic acid on Saccharomyces cerevisiae metabolism under aerobic and anaerobic conditions. Metabolomics 14:103. doi: 10.1007/s11306-018-1399-8
Chidi, B. S., Bauer, F. F., and Rossouw, D. (2018). Organic acid metabolism and the impact of fermentation practices on wine acidity: a review. S. Afr. J. Enol. Vitic. 39, 315–329. doi: 10.21548/39-2-3172
Curiel, J. A., Morales, P., Gonzalez, R., and Tronchoni, J. (2017). Different non-Saccharomyces yeast species stimulate nutrient consumption in S. cerevisiae mixed cultures. Front. Microbiol. 8:2121. doi: 10.3389/fmicb.2017.02121
Dekker, W. J. C., Ortiz-Merino, R. A., Kaljouw, A., Battjes, J., Wiering, F. W., Mooiman, C., et al. (2021). Engineering the thermotolerant industrial yeast Kluyveromyces marxianus for anaerobic growth. Metab. Eng. 67, 347–364. doi: 10.1016/j.ymben.2021.07.006
Delfini, C., Cocito, C., Ravaglia, S., and Conterno, L. (1993). Influence of clarification and suspended grape solid materials on sterol content of free run and pressed grape musts in the presence of growing yeast cells. Am. J. Enol. Vitic. 44:452–458.
Deroite, A., Legras, J.-L., Rigou, P., Ortiz-Julien, A., and Dequin, S. (2018). Lipids modulate acetic acid and thiol final concentrations in wine during fermentation by Saccharomyces cerevisiae × Saccharomyces kudriavzevii hybrids. AMB Expr. 8:130. doi: 10.1186/s13568-018-0657-5
Duan, L. L., Shi, Y., Jiang, R., Yang, Q., Wang, Y. Q., Liu, P.T., Duan, C. Q., and Yan, G. L. (2015). Effects of adding unsaturated fatty acids on fatty acid composition of Saccharomyces cerevisiae and major volatile compounds in wine. S. Afr. J. Enol. Vitic. 36, 285–295. doi: 10.21548/36-2-962
Erasmus, D. J., Cliff, M., and Van Vuuren, H. J. J. (2004). Impact of yeast strain on the production of acetic acid, glycerol, and the sensory attributes of icewine. Am. J. Enol. Vitic. 55, 371–378.
Fairbairn, S., Ferreira, A. C. S., and Bauer, F. F. (2019). Modulation of yeast-derived volatile aromas by oleic acid and sterols. S. Afr. J. Enol. Vitic. 40, 210–220. doi: 10.21548/40-2-3264
Fujii, T., Kobayashi, O., Yoshimoto, H., Furukawa, S., and Tamai, Y. (1997). Effect of aeration and unsaturated fatty acids on expression of the Saccharomyces cerevisiae alcohol acetyltransferase gene. Appl. Environ. Microbiol. 63:910–915. doi: 10.1128/aem.63.3.910-915.1997
Furlani, M. V. M., Maturano, Y. P., Combina, M., Mercado, L. A., Toro, M. E., and Vazquez, F. (2017). Selection of non-Saccharomyces yeasts to be used in grape musts with high alcoholic potential: a strategy to obtain wines with reduced ethanol content. FEMS Yeast Res. 17:fox010. doi: 10.1093/femsyr/fox010
Gobbi, M., Comitini, F., Domizio, P., Romani, C., Lencioni, L., Mannazzu, I., and Ciani, M. (2013). Lachancea thermotolerans and Saccharomyces cerevisiae in simultaneous and sequential co-fermentation: a strategy to enhance acidity and improve the overall quality of wine. Food Microbiol. 33, 271–281. doi: 10.1016/j.fm.2012.10.004
Goold, H. D., Kroukamp, H., Williams, T. C., Paulsen, I. T., Varela, C., and Pretorius, I. S. (2017). Yeast’s balancing act between ethanol and glycerol production in low-alcohol wines. Microb. Biotechnol. 10, 264–278. doi: 10.1111/1751-7915.12488
Guillamo, M., and Verrips, C. T. (2001). The glutamate synthase (GOGAT) of Saccharomyces cerevisiae plays an important role in central nitrogen metabolism. FEMS Yeast Res. 1, 169–175. doi: 10.1111/j.1567-1364.2001.tb00031.x
Henschke, P. A., and Jiranek, V. (1993). “Yeast: metabolism of nitrogen compounds” in Wine Microbiology and Biotechnology, ed. G.H. Fleet. (Lausanne: Harwood Academie), 77–164.
Hirst, M. B., and Richter, C. L. (2016). Review of aroma formation through metabolic pathways of Saccharomyces cerevisiae in beverage fermentations. Am. J. Enol. Vitic. 67, 361–370. doi: 10.5344/ajev.2016.15098
Houtman, A., and Du Plessis C. (2017). Nutritional deficiencies of clarified white grape juices and their correction in relation to fermentation. S. Afr. J. Enol. Vitic. 7, 39–46. doi: 10.21548/7-1-2345
Kozak, B. U., van Rossum, H. M., Benjamin, K. R., Wu, L., Daran, J. M. G., Pronk, J. T., and Van Maris, A. J. A. (2014). Replacement of the Saccharomyces cerevisiae acetyl-CoA synthetases by alternative pathways for cytosolic acetyl-CoA synthesis. Metab. Eng. 21, 46–59. doi: 10.1016/j.ymben.2013.11.005
Liu, P., Zhang, B., Duan, C., and Yan, G. (2020). Pre-fermentative supplementation of unsaturated fatty acids alters the effect of overexpressing ATF1 and EEB1 on esters biosynthesis in red wine. LWT 120:108925. doi: 10.1016/j.lwt.2019.108925
Liu, P. T., Duan, C. Q., and Yan, G. L. (2019). Comparing the effects of different unsaturated fatty acids on fermentation performance of Saccharomyces cerevisiae and aroma compounds during red wine fermentation. Molecules 24:538. doi: 10.3390/molecules24030538
Liu, P. T., Yu, K. J., Li, Y. T., Duan, C. Q., and Yan, G. L. (2018). The content of linoleic acid in grape must influences the aromatic effect of branched-chain amino acids addition on red wine. Food Res. Int. 114, 214–222. doi: 10.1016/j.foodres.2018.08.016
Luparia, V., Soubeyrand, V., Berges, T., Julien, A., and Salmon, J.-M. (2004). Assimilation of grape phytosterols by Saccharomyces cerevisiae and their impact on enological fermentations. Appl. Microbiol. Biotechnol. 65, 25–32. doi: 10.1007/s00253-003-1549-3
Ma, M., and Liu, Z. L. (2010). Mechanisms of ethanol tolerance in Saccharomyces cerevisiae. Appl. Microbiol. Biotechnol. 87, 829–845. doi: 10.1007/s00253-010-2594-3
Mannazzu, I., Angelozzi, D., Belviso, S., Budroni, M., Farris, G. A., Goffrini, P., Lodi, T., Marzona, M., and Bardi, L. (2008). Behaviour of Saccharomyces cerevisiae wine strains during adaptation to unfavourable conditions of fermentation on synthetic medium: cell lipid composition, membrane integrity, viability and fermentative activity. Int. J. Food Microbiol. 121, 84–91. doi: 10.1016/j.ijfoodmicro.2007.11.003
Mauricio, J. C., Millán, C., and Ortega, J. M. (1998). Influence of oxygen on the biosynthesis of cellular fatty acids, sterols and phospholipids during alcoholic fermentation by Saccharomyces cerevisiae and Torulaspora delbrueckii. World J. Microbiol. Biotechnol. 14, 405–410. doi: 10.1023/A:1008873430077
Mauricio, J. C., Moreno, J. J., Zea, L., Ortega, M., and Medina, M. (1997). The effect of grape must fermentation conditions on volatile alcohols and esters formed by Saccharomyces cerevisiae. J. Sci. Food Agric. 75, 155–160. doi: 10.1002/(SICI)1097-0010(199710)75:2<155::AID-JSFA853>3.0.CO;2-S
Mbuyane, L. L., de Kock, M., Bauer, F. F., and Divol, B. (2018). Torulaspora delbrueckii produces high levels of C5 and C6 polyols during wine fermentations. FEMS Yeast Res. 18:foy084. doi: 10.1093/femsyr/foy084
Ochando, T., Mouret, J. R., Humbert-Goffard, A., Sablayrolles, J. M., and Farines V. (2017). Impact of initial lipid content and oxygen supply on alcoholic fermentation in Champagne-like musts. Food Res. Int. 98, 87–94. doi: 10.1016/j.foodres.2016.11.010
Ohta, K., and Hayashida, S. (1983). Role of tween 80 and monoolein in a lipid-sterol-protein complex which enhances ethanol tolerance of sake yeasts. Appl. Environ. Microbiol. 46, 821–825. doi: 10.1128/aem.46.4.821-825.1983
Oro, L., Ciani, M., and Comitini, F. (2014). Antimicrobial activity of Metschnikowia pulcherrima on wine yeasts. J. Appl. Microbiol. 116, 1209–1217. doi: 10.1111/jam.12446
Padilla, B., Gil, J. V., and Manzanares, P. (2016). Past and future of non-Saccharomyces yeasts: from spoilage microorganisms to biotechnological tools for improving wine aroma complexity. Front. Microbiol. 7:411. doi: 10.3389/fmicb.2016.00411
Pigeau, G. M., and Inglis, D. L. (2005). Upregulation of ALD3 and GPD1 in Saccharomyces cerevisiae during Icewine fermentation. J. Appl. Microbiol. 99, 112–125. doi: 10.1111/j.1365-2672.2005.02577.x
Pigeau, G. M., and Inglis, D. L. (2007). Response of wine yeast (Saccharomyces cerevisiae) aldehyde dehydrogenases to acetaldehyde stress during Icewine fermentation. J. Appl. Microbiol. 103, 1576–1586. doi: 10.1111/j.1365-2672.2007.03381.x
Pina, C., Santos, C., Couto, J. A., and Hogg, T. (2004). Ethanol tolerance of five non-Saccharomyces wine yeasts in comparison with a strain of Saccharomyces cerevisiae - Influence of different culture conditions. Food Microbiol. 21, 439–447. doi: 10.1016/j.fm.2003.10.009
Pronk, J. T., Steensma, H. Y., and Van Dijken, J. P. (1996). Pyruvate metabolism in Saccharomyces cerevisiae. Yeast 12, 1607–1633.
Puškaš, V. S., and Miljić, U. D. (2020). The aptitude of commercial yeast strains for lowering the ethanol content of wine. Food Sci. Nutr. 8, 1489–1498. doi: 10.1002/fsn3.1433
Remize, F., and Andrieu, E. (2000). Engineering of the pyruvate dehydrogenase bypass in Saccharomyces cerevisiae: role of the cytosolic Mg2+ and mitochondrial K+ acetaldehyde dehydrogenases Ald6p and Ald4p in acetate formation during alcoholic Fermentation. Appl. Environ. Microbiol. 66, 3151–3159. doi: 10.1128/AEM.66.8.3151-3159.2000
Renault, P., Miot-Sertier, C., Marullo, P., Hernández-Orte, P., Lagarrigue, L., Lonvaud-Funel, A., and Bely, M. (2009). Genetic characterization and phenotypic variability in Torulaspora delbrueckii species: potential applications in the wine industry. Int. J. Food Microbiol. 134, 201–210. doi: 10.1016/j.ijfoodmicro.2009.06.008
Rodrigues, F., Sousa, M. J., Ludovico, P., Santos, H., Côrte-Real, M., and Leão, C. (2012). The fate of acetic acid during glucose co-metabolism by the spoilage yeast Zygosaccharomyces bailii. PLoS One 7:e52402. doi: 10.1371/journal.pone.0052402
Rodríguez-Porrata, B., Lopez-Martinez, G., Redón, M., Sancho, M., Mas, A., Rozès, N., and Cordero-Otero, R. (2011). Enhancing yeast cell viability after dehydration by modification of the lipid profile. World J. Microbiol. Biotechnol. 27, 75–83. doi: 10.1007/s11274-010-0428-1
Rosenfeld, E., Beauvoit, B., Blondin, B., and Salmon, J.-M. (2003). Oxygen consumption by anaerobic Saccharomyces cerevisiae under enological conditions: effect on fermentation kinetics. Appl. Environ. Microbiol. 69, 113-121. doi: 10.1128/AEM.69.1.113
Rossignol, T., Dulau, L., Julien, A., and Blondin, B. (2003). Genome-wide monitoring of wine yeast gene expression during alcoholic fermentation. Yeast 20, 1369–1385. doi: 10.1002/yea.1046
Rossouw, D., and Bauer, F. F. (2016). Exploring the phenotypic space of non-Saccharomyces wine yeast biodiversity. Food Microbiol. 55, 32–46. doi: 10.1016/j.fm.2015.11.017
Sakihama, Y., Hid, R., Hasunuma, T., and Kondo, A. (2019). Increased flux in acetyl-CoA synthetic pathway and TCA cycle of Kluyveromyces marxianus under respiratory conditions. Sci. Rep. 9:5319. doi: 10.1038/s41598-019-41863-1
Santos, J., Sousa, M. J., Cardoso, H., Inácio, J., Silva, S., Spencer-Martins, I.,, et al. (2008). Ethanol tolerance of sugar transport, and the rectification of stuck wine fermentations. Microbiology 154, 422–430. doi: 10.1099/mic.0.2007/011445-0
Seguinot, P., Ortiz-Julien, A., and Camarasa, C. (2020). Impact of nutrient availability on the fermentation and production of aroma compounds under sequential inoculation with M. pulcherrima and S. cerevisiae. Front. Microbiol. 11:305. doi: 10.3389/fmicb.2020.00305
Shekhawat, K., Bauer, F. F., and Setati, M. E. (2017). Impact of oxygenation on the performance of three non-Saccharomyces yeasts in co-fermentation with Saccharomyces cerevisiae. Appl. Microbiol. Biotechnol. 101, 2479–2491. doi: 10.1007/s00253-016-8001-y
Shima, J., and Takagi, H. (2009). Stress-tolerance of baker’s-yeast (Saccharomyces cerevisiae) cells: stress-protective molecules and genes involved in stress tolerance. Biotechnol. Appl. Biochem. 53, 155–164. doi: 10.1042/BA20090029
Stukeys, J. E., McDonough, V. M., and Martin, C. E. (1990). The OLE1 gene of Saccharomyces cerevisiae encodes the delta 9 fatty acid desaturase and can be functionally replaced by the rat stearoyl-CoA desaturase gene. J. Biol. Chem. 265, 20144–20149. doi: 10.1016/s0021-9258(17)30481-7
Taillandier, P., Lai, Q. P., Julien-Ortiz, A., and Brandam, C. (2014). Interactions between Torulaspora delbrueckii and Saccharomyces cerevisiae in wine fermentation: influence of inoculation and nitrogen content. World J. Microbiol. Biotechnol. 30, 1959–1967. doi: 10.1007/s11274-014-1618-z
Tesnière, C., Pradal, M., and Legras, J. L. (2021). Sterol uptake analysis in Saccharomyces and non-Saccharomyces wine yeast species. FEMS Yeast Res. 21:foab020. doi: 10.1093/femsyr/foab020
Tronchoni, J., Curiel, J. A., Morales, P., Torres-Pérez, R., and Gonzalez, R. (2017). Early transcriptional response to biotic stress in mixed starter fermentations involving Saccharomyces cerevisiae and Torulaspora delbrueckii. Int. J. Food Microbiol. 16, 60-68. doi: 10.1016/j.ijfoodmicro.2016.10.017
Tumanov, S., Zubenko, Y., Greven, M., Greenwood, D. R., Shmanai, V., and Villas-boas, S. G. (2015). Comprehensive lipidome profiling of Sauvignon blanc grape juice. Food Chem. 180, 249–256. doi: 10.1016/j.foodchem.2015.01.134
Valero, E., Millán, C., and Ortega, J. M. (2001). Influence of oxygen addition during growth phase on the biosynthesis of lipids in Saccharomyces cerevisiae (M,30-9) in enological fermentations. J. Biosci. Bioeng. 92, 33–38. doi: 10.1263/jbb.92.33
Valero, E., Millán, C., and Ortega, J. M. (2002). Changes in the lipid composition of Saccharomyces cerevisiae race Capensis (G-1) during alcoholic fermentation and flor film formation. LWT 35, 593–599. doi: 10.1016/S0023-6438(02)90916-3
Valero, E., Millan, M., Mauricio, J., and Ortega, J. (1998). Effect of grape skin maceration on sterol, phospholipid, and fatty acid contents of Saccharomyces cerevisiae during alcoholic fermentation. Am. J. Enol. Vitic. 49, 119–124.
Van den Berg, M. A., and Steensma, H. Y. (1995). ACS2, a Saccharomyces cerevisiae gene encoding acetyl-coenzyme A synthetase, essential for growth on glucose. Eur. J. Biochem. 231, 704–713. doi: 10.1111/j.1432-1033.1995.tb20751.x
Van der Rest, M. E., Kamminga, A. H., Nakano, A., Anraku, Y., Poolman, B., and Konings, W. N. (1995). The plasma membrane of Saccharomyces cerevisiae: structure, function, and biogenesis. Microbiol. Rev. 59, 304–322. doi: 10.1128/mr.59.2.304-322.1995
Van Rossum, H. M., Kozak, B. U., Niemeijer, M. S., Dykstra, J. C., and Luttik, M. A. H. (2016). Requirements for carnitine shuttle-mediated translocation of mitochondrial acetyl moieties to the yeast cytosol. mBio 7:e00520-16. doi: 10.1128/mBio.00520-16
Varela, C., Torrea, D., Schmidt, S. A., Ancin-Azpilicueta, C., and Henschke, P. A. (2012). Effect of oxygen and lipid supplementation on the volatile composition of chemically defined medium and Chardonnay wine fermented with Saccharomyces cerevisiae. Food Chem. 135, 2863–2871. doi: 10.1016/j.foodchem.2012.06.127
Williams, C., Mbuyane, L. L., Bauer, F. F., Mokwena, L., Divol, B., and Buica, A. (2021). A gas chromatography-mass spectrometry method for the determination of fatty acids and sterols in yeast and grape juice. Appl. Sci. 11:5152. doi: 10.3390/app11115152
You, K. M., Rosenfield, C., and Knipple, D. C. (2003). Ethanol tolerance in the yeast Saccharomyces cerevisiae is dependent on cellular oleic acid content. Appl. Environ. Microbiol. 69, 1499–1503. doi: 10.1128/AEM.69.3.1499
Yunoki, K., Yasui, Y., Hirose, S., and Ohnishi, M. (2005). Fatty acids in must prepared from eleven grapes grown in Japan: comparison with wine and effect on fatty acid ethyl ester formation. Lipids 40, 361–367. doi: 10.1007/s11745-006-1395-z
Keywords: fatty acids, ergosterol, wine, yeasts, acetic acid, metabolic flux, acetyl-CoA
Citation: Mbuyane LL, Bauer FF, Bloem A, Camarasa C, Ortiz-Julien A and Divol B (2022) Species-Dependent Metabolic Response to Lipid Mixtures in Wine Yeasts. Front. Microbiol. 13:823581. doi: 10.3389/fmicb.2022.823581
Received: 27 November 2021; Accepted: 14 April 2022;
Published: 23 May 2022.
Edited by:
Rosanna Tofalo, University of Teramo, ItalyCopyright © 2022 Mbuyane, Bauer, Bloem, Camarasa, Ortiz-Julien and Divol. This is an open-access article distributed under the terms of the Creative Commons Attribution License (CC BY). The use, distribution or reproduction in other forums is permitted, provided the original author(s) and the copyright owner(s) are credited and that the original publication in this journal is cited, in accordance with accepted academic practice. No use, distribution or reproduction is permitted which does not comply with these terms.
*Correspondence: Benoit Divol, ZGl2b2xAc3VuLmFjLnph
Disclaimer: All claims expressed in this article are solely those of the authors and do not necessarily represent those of their affiliated organizations, or those of the publisher, the editors and the reviewers. Any product that may be evaluated in this article or claim that may be made by its manufacturer is not guaranteed or endorsed by the publisher.
Research integrity at Frontiers
Learn more about the work of our research integrity team to safeguard the quality of each article we publish.