- 1College of Food Science, South China Agricultural University, Guangzhou, China
- 2Guangdong Provincial Key Laboratory of Microbial Safety and Health, State Key Laboratory of Applied Microbiology Southern China, Institute of Microbiology, Guangdong Academy of Sciences, Guangzhou, China
Mining novel specific molecular targets and establishing efficient identification methods are significant for detecting Pseudomonas aeruginosa, which can enable P. aeruginosa tracing in food and water. Pangenome analysis was used to analyze the whole genomic sequences of 2017 strains (including 1,000 P. aeruginosa strains and 1,017 other common foodborne pathogen strains) downloaded from gene databases to obtain novel species-specific genes, yielding a total of 11 such genes. Four novel target genes, UCBPP-PA14_00095, UCBPP-PA14_03237, UCBPP-PA14_04976, and UCBPP-PA14_03627, were selected for use, which had 100% coverage in the target strain and were not present in nontarget bacteria. PCR primers (PA1, PA2, PA3, and PA4) and qPCR primers (PA12, PA13, PA14, and PA15) were designed based on these target genes to establish detection methods. For the PCR primer set, the minimum detection limit for DNA was 65.4 fg/μl, which was observed for primer set PA2 of the UCBPP-PA14_03237 gene. The detection limit in pure culture without pre-enrichment was 105 colony-forming units (CFU)/ml for primer set PA1, 103 CFU/ml for primer set PA2, and 104 CFU/ml for primer set PA3 and primer set PA4. Then, qPCR standard curves were established based on the novel species-specific targets. The standard curves showed perfect linear correlations, with R2 values of 0.9901 for primer set PA12, 0.9915 for primer set PA13, 0.9924 for primer set PA14, and 0.9935 for primer set PA15. The minimum detection limit of the real-time PCR (qPCR) assay was 102 CFU/ml for pure cultures of P. aeruginosa. Compared with the endpoint PCR and traditional culture methods, the qPCR assay was more sensitive by one or two orders of magnitude. The feasibility of these methods was satisfactory in terms of sensitivity, specificity, and efficiency after evaluating 29 ready-to-eat vegetable samples and was almost consistent with that of the national standard detection method. The developed assays can be applied for rapid screening and detection of pathogenic P. aeruginosa, providing accurate results to inform effective monitoring measures in order to improve microbiological safety.
Introduction
Pseudomonas aeruginosa is a common cause of severe nosocomial infections. Patients with metabolic or hematological diseases or patients with malignant immunodeficiency or tumors are especially susceptible to P. aeruginosa infection, as are patients in intensive care units (Namaki et al., 2022). Pseudomonas aeruginosa is also the most common cause of ventilator-associated pneumonia and burn wound infections, both of which have a mortality rate of >30% (Kidd et al., 2015). Respiratory tract infection with P. aeruginosa is a major determinant of the severity of lung disease and is associated with significant incidence rate and mortality of cystic fibrosis (CF; Crull et al., 2018; Mesinele et al., 2022).
Pseudomonas aeruginosa is widely distributed in water, plants, soil, and humid natural environments, and easily contaminates different kinds of food (Oliver et al., 2015). In addition to being frequently found in bottled mineral water and tap water, P. aeruginosa has also been tested positive in ready-to-eat vegetables (Naze et al., 2010; Pelegrin et al., 2021; Ruiz-Roldán et al., 2021). Studies found the ready-to-eat vegetables that were a potential-although rare-vector for colistin- and carbapenem-resistant P. aeruginosa, the contamination rate of P. aeruginosa has reached 17.5% or 34% (Cai et al., 2015; Hölzel et al., 2018; Kapeleka et al., 2020; Junaid et al., 2021). That is to say, P. aeruginosa is a major contaminant of fresh vegetables, which might be a source of infection for susceptible persons within the community (Rahman et al., 2022). Transmission of P. aeruginosa along the food chain could cause gastrointestinal infections (Fakhkhari et al., 2022). More importantly, P. aeruginosa is the dominant spoilage bacteria and has the strongest spoilage potential in vegetable that are stored under aerobic conditions (Dharmarha et al., 2019; Jin et al., 2021). Additionally, the shelf life of ready-to-eat vegetables is seriously affected by P. aeruginosa, which will cause great economic losses (Godova et al., 2020). All told, the presence of P. aeruginosa in ready-to-eat vegetables causes food spoilage, reduced shelf life, and economic loss. Therefore, it is necessary to trace the occurrence of potential pollution of this pathogen, so as to provide a scientific basis for ensuring the safety of ready-to-eat vegetables.
Currently, the standard gold method for detecting P. aeruginosa in food is the conventional culture method, which is labor-intensive, expensive, and time-consuming (Zhou et al., 2020; Chon et al., 2021). Especially when the number of samples is large, it takes a long time to isolate and identify P. aeruginosa from ready-to-eat vegetables by traditional methods (Gharieb et al., 2022). In addition, the traditional culture method determines P. aeruginosa according to the green pigment produced by the strain. This method will lead to wrong judgment in actual inspection: one case is that some strains of P. aeruginosa do not produce this pigment, which leads to missed inspection. Another situation is that P. fluorescens produces the same pigment as P. aeruginosa, which makes it impossible to distinguish and cause false positive (Schroth et al., 2018; Junaid et al., 2021). For a long time, scientists have been committed to establishing a rapid and sensitive method for the detection of P. aeruginosa, but each method has its advantages and disadvantages (Tang et al., 2017). DNA fingerprinting and 16S DNA-based analyses were used to identify the harm of plant derived P. aeruginosa to humans and animals, which is complex and requires very professional inspectors (Ambreetha et al., 2021). Biosensor method and 16r RNA gene amplicon sequencing, which had high detection efficiency, were used to analyze P. aeruginosa of food microorganisms, but these methods need complex pretreatment (Zhong et al., 2020; Wind et al., 2021). Illumina whole gene sequencing has great advantages in accuracy, was used to analyze the distribution of P. aeruginosa after pasteurized milk, but it takes a lot of testing costs (Maske et al., 2021). Furthermore, 25 articles mentioned health risks from consuming fresh produce by antimicrobial-resistant bacteria, but none quantified the risk (Rahman et al., 2022). When the concentration of P. aeruginosa reaches a certain value, it may have the risk of colonization, so it is necessary to quantify its concentration (Kwok et al., 2021). Therefore, it is necessary to develop rapid, accurate, simple, and efficient diagnostic techniques or tools for the detection of P. aeruginosa in food, so as to monitor the pollution status and provide scientific basis for the prevention and control of foodborne P. aeruginosa.
PCR has been widely employed as a rapid and specific method for the detection of P. aeruginosa in a variety of foods and processing environments because of its high specificity, sensitivity, time savings, and easy operation. The target genes oprL and oprI have been used for the molecular detection of P. aeruginosa in burn patients. This approach is a valuable technique for the early and precise detection of P. aeruginosa (Jami Al-Ahmadi and Zahmatkesh Roodsari, 2016; Mapipa et al., 2021). A sensitive method has been developed to detect Pseudomonas pseudomallei from the soil with PCR by targeting specific flagellin genes (Tungpradabkul et al., 2005). However, most of the reported PCR-based methods for identifying and characterizing P. aeruginosa target bacterial virulence genes or 16S and 23S rRNA genes, which provide a limited number of targets (Wei et al., 2015; Wang et al., 2016). With the maturity of whole-genome sequencing technology and the increasing gene pool of new strains, some of the original targets cannot cover the detection of new themes. Therefore, it is vital to mine novel target genes with high species specificity for more accurate and efficient pathogen detection.
With the advancement of sequencing techniques, numerous genomes of P. aeruginosa and other Pseudomonas species have been described. Several novel specific target sequences, such as those of gyrB, ecfX, fliC, and algD, have been identified and applied to distinguish P. aeruginosa from other Pseudomonas spp. (Taee et al., 2014; Heidari et al., 2018; Wang et al., 2020; Khademi et al., 2021). The tremendous increase in the availability of bacterial genome sequences is allowing researchers to investigate and query pangenomes (Freschi et al., 2018).
Pangenome analysis has become a representative discipline for studying the entire repertoire of gene families in the genomes of pathogenic bacterial clades, which not only provides the whole set of genes shared by Pseudomonas species but also can also be applied in interspecies differentiation analysis to mine species-specific genes in order to use a wealth of genome data (Hilker et al., 2014).
In short, for the detection of P. aeruginosa, traditional methods are time-consuming and laborious, and the experimental conditions of immunological methods are limited, while the sensitivity and accuracy of the existing molecular methods need to be considered. There is an urgent need for novel specific molecular detection targets of P. aeruginosa in order to establish a rapid and efficient detection method. Exactly, the explosive development of whole gene sequencing technology has made mining targets become convenient. Therefore, we aimed at mining novel specific target gene sequences of P. aeruginosa based on the pangenome analysis and established high-specificity and high-sensitivity PCR and quantitative real-time PCR (qPCR) methods based on these targets. Furthermore, the established methods were applied to the detection of actual samples of ready-to-eat vegetables to master the pollution of P. aeruginosa in ready-to-eat vegetable industry, so as to provide a scientific basis for reducing pollution. The flowchart of the experimental method involved in this study is shown in Figure 1.
Materials and Methods
Screening Species-Specific Novel Target Genes for Pseudomonas aeruginosa
Genomic sequences of 1,000 P. aeruginosa strains and 1,017 other common foodborne pathogen strains were retrieved from the NCBI Genome Database (last accessed on November 30, 2019). The specific information for the sequences is provided in Supplementary Table S1. Pangenome analysis was used to identify P. aeruginosa species-specific genes. The research involved the evaluation of nucleotide sequence dissimilarity between P. aeruginosa and non-P. aeruginosa sequences (Pang et al., 2019). In brief, all nucleic acid sequences downloaded from the NCBI database were annotated using Prokka v1.11 (Seemann, 2014). Then, the output of Prokka was used to construct a pangenome by Roary v3.11.2 (Page et al., 2015), with a BLASTP identity cutoff of 85%. The absence/existence profile of all genes across strains was converted into a 0/1 matrix with a local script. The matrix was then used to identify P. aeruginosa species-specific genes, which were screened according to the following criteria: 100% presence in target species strains and 0% presence in all other bacterial species strains and non-P. aeruginosa strains. Then, these candidate targets were further screened against the nucleotide collection (nr/nt) databases using the online BLAST program1 and PCR verification to ensure specificity.
Specific Primer Design for PCR and Real-Time PCR
Primer Premier 6.0 software (PREMIER Biosoft International, Palo Alto, United States) was used to design primers targeting the screened conserved sequences of P. aeruginosa. Primers without hairpin structures or dimers and the highest rating score were selected. Their specificity was preliminarily verified by the NCBI Blast tool. Then, the primers listed in Table 1 were synthesized by Shanghai Sangon Company (Shanghai, China).
Bacterial Strains and Genomic DNA Extraction
This study used 134 bacterial strains (95 P. aeruginosa strains and 39 non-P. aeruginosa strains; Supplementary Table S2). They were purchased from the National Center for Medical Culture Collections (CMCC, Beijing, China), the American Type Culture Collection (ATCC, Manassas, VA, United States), and the China General Microbiological Culture Collection Center (CGMCC, Beijing, China). The other strains used in this study were part of our laboratory culture collection.
All strains were cultured in Luria-Bertani (LB) broth at 37°C. The bacterial cultures were then collected by centrifugation at 25°C and 12,000 × g for 5 min. Genomic DNA from these cells was extracted and purified using an EZNA Bacteria Genome Kit (Omega Bio-Tek Inc., Norcross, GA, United States) according to the manufacturer’s instructions. The concentration and purity of the DNA were estimated by agarose gel electrophoresis and by using a NanoDrop 2000c UV–Vis spectrophotometer (Thermo Fisher Scientific, Waltham, MA, United States). Extracted DNA was stored at −20°C until PCR and qPCR analysis.
PCR and Real-Time PCR Conditions for Pseudomonas aeruginosa Detection
The DNA extracted from bacterial strains was used for PCR and qPCR amplification. The PCR mixture consisted of 12.5 μl of 2 × Taq Master Mix (Vazyme, China), 1 μl of each primer (10 μM), 50 ng of DNA template, and sterile distilled H2O up to a final volume of 25 μl. PCR amplification was performed in a PTC-100 programmable thermal controller (MJ Research, Inc.), with an initial denaturation step of 98°C for 3 min, followed by 35 cycles at 95°C for 30 s, 58.0°C for 30 s, and 72°C for 30 s and a final extension step at 72°C for 10 min. The PCR products were separated by 2% agarose gel electrophoresis and visualized by ethidium bromide staining. All PCR assays in this study were conducted in triplicate.
For qPCR amplification, the total reaction volume was 20 μl, including 10 μl of TB Green™ Premix Ex Taq™ II (TaKaRa, Biotech, Dalian, China), 1 μl each of the forward and reverse primers (10 μM), 7 μl of sterile water, and 50 ng of the purified bacterial genomic DNA as a template. A LightCycler® 96 System (Roche, Switzerland) was used for thermal cycling, as follows: initial denaturation of DNA at 95°C for 30 s, followed by 40 cycles of denaturation at 95°C for 5 s and annealing at 55°C for 60 s. The qPCR assay was performed in triplicate with parallel analysis in 96-well plates. Sterile water was used in place of the DNA template as a negative control to ensure the absence of contaminants.
Specificity Evaluation of the Primers for PCR and qPCR Assays
All strains used for the verification of primer specificity in the PCR and qPCR assays were from our laboratory collection and are listed in Supplementary Table S2. Genomic DNA was extracted from 95 P. aeruginosa strains and 39 non-P. aeruginosa strains and used as a template to validate the specificity of the designed primers. One tube of PCR mixture was added to 2 μl of sterile distilled water instead of DNA template as a blank control. The PCR primer sets that could amplify a single target band with the expected length for the corresponding strains of P. aeruginosa that showed negative results for non-P. aeruginosa strains were considered species-specific primers and used for further evaluation. The reported toxA target gene, a major virulence factor in P. aeruginosa, was used in a comparative experiment (SN/T2206.12, 2016; Taee et al., 2014). The same experimental environment and strain sets and test set were maintained during the comparative experiment, only hanging the target to the toxA gene (Supplementary Table S4; Supplementary Figure S2).
Genomic DNAs from 63 P. aeruginosa strains and 32 other bacterial strains were used as a template for the qPCR amplification to evaluate the specificity of the qPCR assay. The qPCR assay was performed in triplicate with parallel analysis in 96-well plates (Supplementary Table S3).
Sensitivity and Interference Evaluation of Specific Primers Using Genomic DNA
Purified DNA of a known concentration extracted from P. aeruginosa ATCC 15442 was serially diluted 10-fold. Two microliters of diluted extracted DNA was used as a template in a 25 μl PCR. One tube of PCR mixture was added to 2 μl of sterile distilled water instead of DNA template as a blank control. The PCR results were analyzed, and the detection limit of the PCR was determined. Then, 2 μl of each dilution was used as the template for qPCR amplification. A Light Cycler® 96 qPCR system (Roche, Basel, Switzerland) was used for thermal cycling as follows: denaturation at 95°C for 60 s, followed by 40 cycles of denaturation at 95°C for 10 s and annealing at 60°C for 30 s. The data were analyzed using built-in software. All P. aeruginosa DNA was extracted for qPCR analysis in triplicate. The target gene with the best detection limit was selected for further study.
Pseudomonas aeruginosa ATCC 15442 and a common pathogen (Escherichia coli ATCC 25922) were used to validate the PCR assay’s accuracy and scope for interference. The strains were cultured in LB broth at 37°C for 18 h and then serially diluted (10-fold) with 8.5% sodium chloride solution. The density of P. aeruginosa cells was adjusted to 104 CFU/ml. Pseudomonas aeruginosa cultures were individually mixed with the interference testing strain at ratios of 1:103, 1:102, 1:10, 1:1, 10:1, and 102:1, and 103:1. Genomic DNA was extracted from the mixtures and used as a template for qPCR. Meanwhile, genomic DNA from P. aeruginosa cultures without the interference strain was used as the positive control template. The ability of the PCR assay to overcome interference was evaluated by 2.0% agarose gel electrophoresis.
Artificial Contamination Experiments
Pseudomonas aeruginosa ATCC 15442 was cultured in LB broth at 37°C for 18 h, and the cell concentration was estimated by plate counting. Tomato samples (10 g) sterilized with ultraviolet light were mixed with 89 ml of LB medium, and then, the mixtures were incubated at 37°C for 18 h. Next, 1 ml P. aeruginosa mixtures were added at final inoculum concentrations ranging from 100 to 108 CFU/g. Genomic DNA was extracted at the indicated time points from 1 ml samples and then analyzed by PCR and qPCR. The amplification system and procedure were performed as described in “PCR and Real-Time PCR Conditions for Pseudomonas aeruginosa Detection” section.
Detection of Pathogenic Pseudomonas aeruginosa in Samples of Ready-to-Eat Vegetables
A total of 29 ready-to-eat vegetable samples were collected from local markets in Guangdong Province, China, to validate the detection ability of PCR and qPCR. The ready-to-eat vegetables were sampled at random sites, and the samples were transported on ice to the laboratory for immediate analysis. The conventional culture method was used for testing based on the standard reference to detect P. aeruginosa in food for import and export (SN/T 2099-2008). Briefly, 25 g of each sample was randomly weighed, added to 225 ml of P. aeruginosa enrichment broth (SCDLP medium, Guangdong Huankai Co., Ltd., Guangzhou, China), and incubated at 37°C for 18 h. A loopful (approximately 10 μl) of the SCDLP enrichment culture was streaked into P. aeruginosa-selective agar plates (CN agar plates; Guangdong Huankai Co., Ltd., Guangzhou, China) and incubated at 37°C for 24 h. According to the manufacturer’s instructions, at least three presumptive colonies were selected to identify P. aeruginosa using the Bruker MALDI Biotyper identification system (MALDI, Bruker, Germany). Meanwhile, 1 ml of SCDLP broth enrichment culture was collected from each sample at 12 h. Genomic DNA was extracted from SCDLP broth enrichment cultures for PCR and qPCR.
Results
Identification of Specific Target Genes for Pseudomonas aeruginosa
Pangenome analysis was used to mine novel molecular targets for detecting P. aeruginosa in this study. A total of four genes (Table 1) were identified as specific to P. aeruginosa according to nucleotide sequence similarity. These gene sequences were present in 100% of the target P. aeruginosa, which did not exist in non-P. aeruginosa sequences available in the NCBI bacterial database according to BLASTN online.
After filtering using PCR analysis, four novel P. aeruginosa-specific targets, including group_98983 (1,000/1,000), phzA2 (1,000/1,000), group_75393 (1,000/1,000), and group_88276 (1,000/1,000), specific for the P. aeruginosa genes were uniquely present in all target strains but not in nontarget strains (Table 2; Supplementary Figure S1). The particular target gene phzA2 encodes a phenazine biosynthesis protein, and the specific target genes group_98983, group_75393, and group_88276 encode hypothetical proteins without assigned functions.
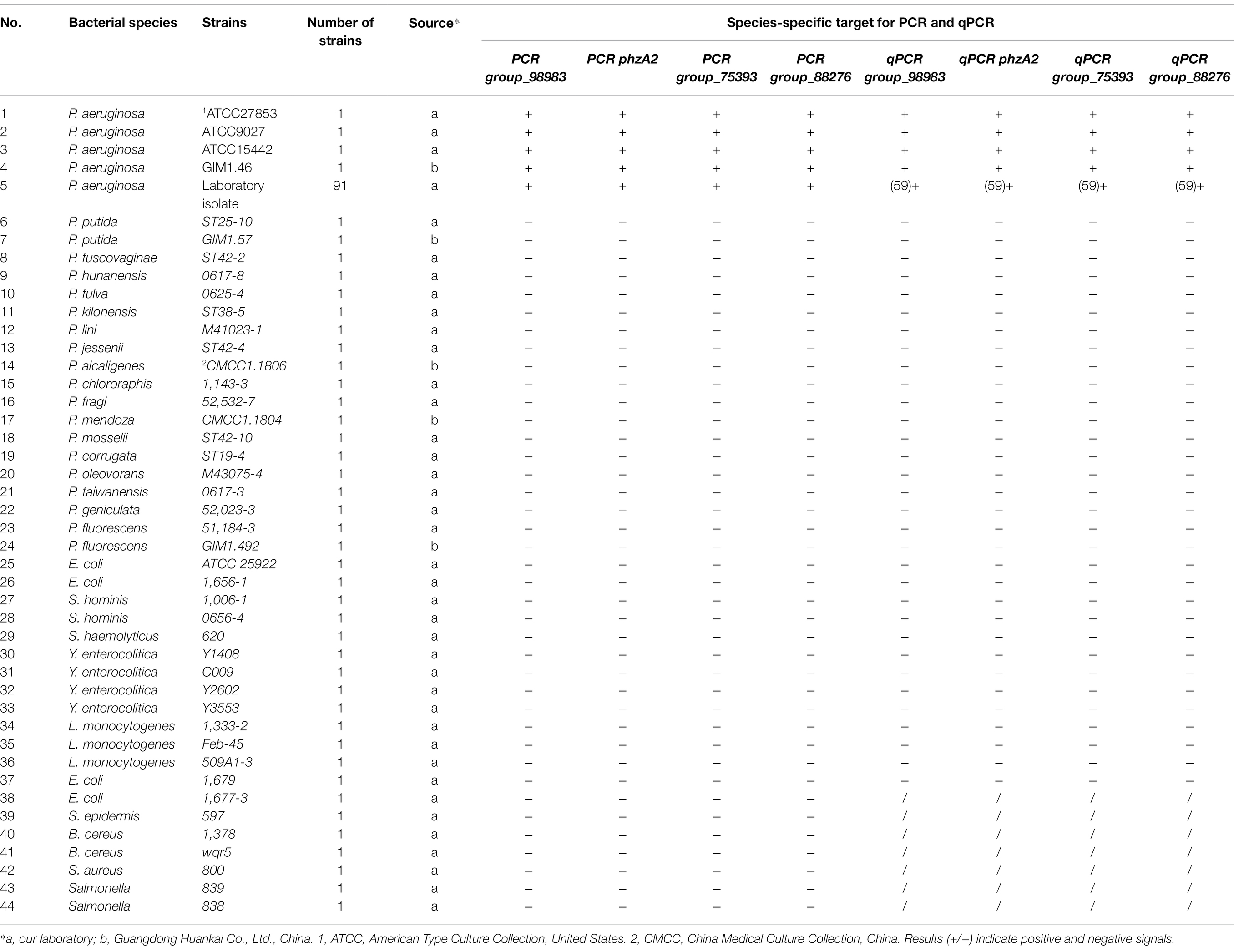
Table 2. Specificity results for PCR primers using P. aeruginosa and other foodborne pathogenic strains.
Diagnostic Specificity of the Novel Specific Primers
The results of specificity tests for the four PCR primer sets are shown in Table 2. These primers were prescreened with 95 P. aeruginosa strains and 39 non-P. aeruginosa strains. The four PCR primer sets showed perfect specificity for P. aeruginosa, and the bands of the four species-specific targets group_98983, phzA2, group_75393, and group_88276 exhibited separate fragments of 169, 325, 263, and 132 bp, respectively, which were obtained only with P. aeruginosa as the template. All the non-P. aeruginosa strains displayed negative results. The above four novel genes had a coverage rate of 100% among existing genes in the strains, while the detection rate of toxA genes was only 82.1% (78/95; Supplementary Figure S2).
The sensitivity of the genes specific to P. aeruginosa DNA was further evaluated. We used qPCR for further analysis based on the specific primers screened by the PCR method. As shown in Table 2, we selected the PA12, PA13, PA14, and PA15 primer sets for use. For accurate qPCR analysis, four primer sets were designed (Table 1). A total of 63 P. aeruginosa strains and 32 non-P. aeruginosa strains were used to verify the specificity of the qPCR primers, and the results are shown in Table 2. According to the Ct values and dissolution curves, all non-P. aeruginosa strains showed no amplification, while amplification was obtained for the target P. aeruginosa strains, indicating a high specificity of the primers with qPCRs.
Sensitivity Evaluation and Interference Evaluation of the Novel Specific Primers
The results regarding the specificity of the PCR assay with novel specific primers are shown in Supplementary Table S2. No product bands were obtained with the 39 non-P. aeruginosa strains tested, and no cross-reactivity was observed. To determine the detection limit of the novel assay, the initial concentration of DNA from P. aeruginosa ATCC 15442 was 65.4 ng/μl. The detection limits using the genomic DNA of P. aeruginosa with the PA1, PA2, PA3, and PA4 primer sets were 65.4 pg/μl, 65.4 fg/μl, 654 fg/μl, and 6.54 pg/μl, respectively (Figure 2).
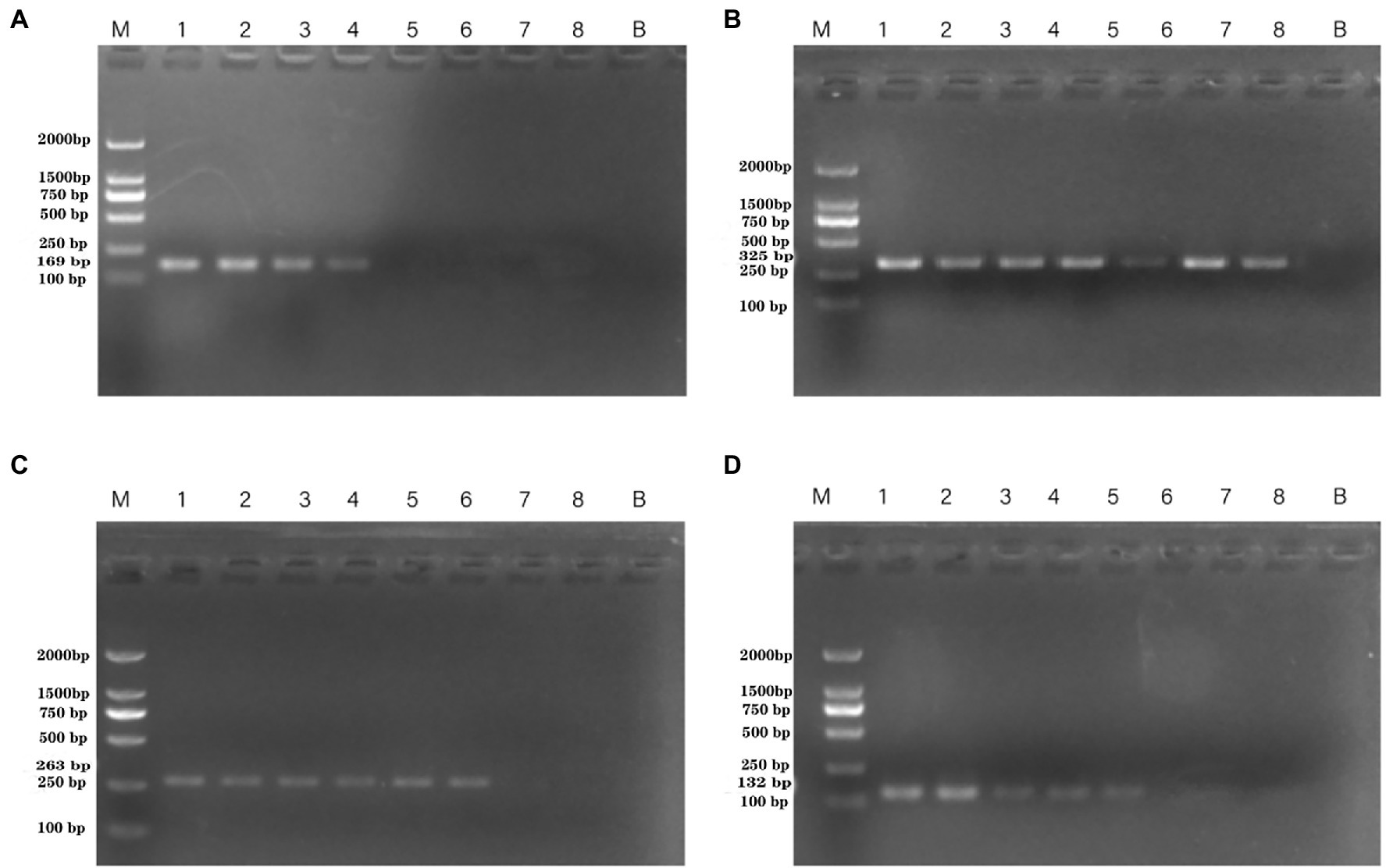
Figure 2. PCR detection sensitivity using dilutions of genomic DNA from Pseudomonas aeruginosa ATCC 15442. Lane M = DSTM 2000 marker (Dongsheng Biotechnology, Guangdong, China); lane N = negative control (double-distilled H2O); lanes 1–8 = 65.4 ng/μl, 6.54 ng/μl, 654 pg/μl, 65.4 pg/μl, 6.54 pg/μl, 654 fg/μl, and 65.4 fg/μl, 6.54 fg/μl, respectively. (A) Primer set PA1 (169 bp); (B) primer set PA2 (325 bp); (C) primer set PA3 (263 bp); and (D) primer set PA4 (132 bp).
DNA was then extracted from different dilutions of P. aeruginosa cultures and used as the template. Following PCR detection, cell concentrations ranging from 100 to 108 CFU/ml were used. The detection limits observed whole cells of P. aeruginosa with the PA1, PA2, PA3, and PA4 primer sets were 4.15 × 105 CFU/ml, 9.7 × 103 CFU/ml, 4.3 × 104 CFU/ml, and 4.3 × 104 CFU/ml, respectively (Figure 3).
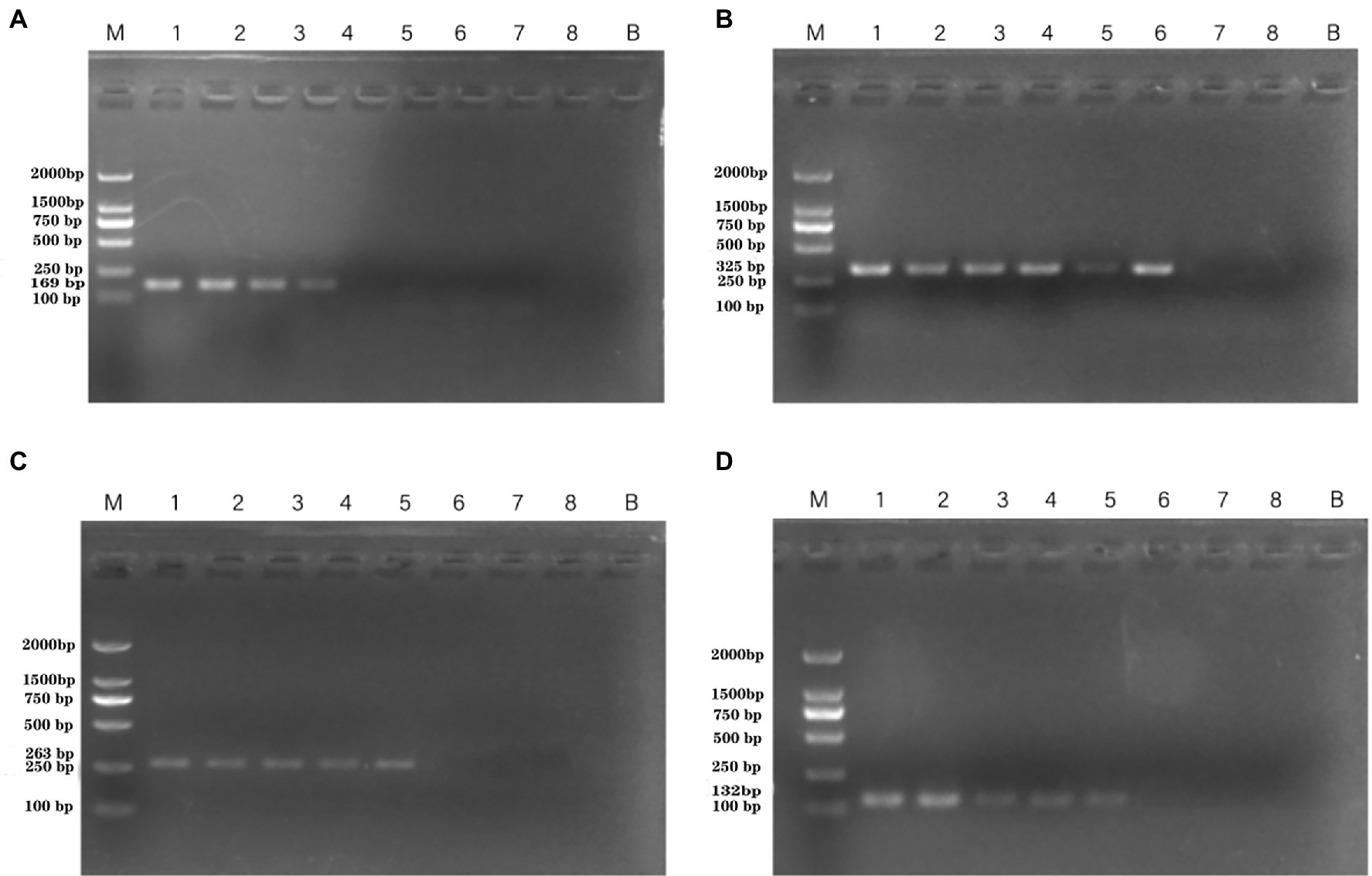
Figure 3. PCR detection sensitivity using dilutions of a pure culture of P. aeruginosa ATCC 15442. Lane M = DSTM 2000 marker (Dongsheng Biotechnology, Guangdong, China); lane N = negative control (double-distilled H2O); and lanes 1–8 = 2.07 × 108 CFU/ml, 2.07 × 107 CFU/ml, 1.85 × 106 CFU/ml, 4.15 × 105 CFU/ml, 4.3 × 104 CFU/ml, 9.7 × 103 CFU/ml, 1.4 × 102 CFU/ml, and 2 × 101 CFU/ml, respectively. (A) Primer set PA1 (169 bp); (B) primer set PA2 (325 bp); (C) primer set PA3 (263 bp); and (D) primer set PA4 (132 bp).
Standard curves were established based on the novel species-specific targets to quantify P. aeruginosa. As illustrated in Figures 4A–D, the four standard curves showed ideal linear correlations, with R2 values of 0.9901 for primer set PA12, 0.9915 for primer set PA13, 0.9924 for primer set PA14, and 0.9935 for primer set PA15. The detection limits were 103 CFU/ml for primer sets PA12 and PA15 and 102 CFU/ml for primer sets PA13 and PA14.
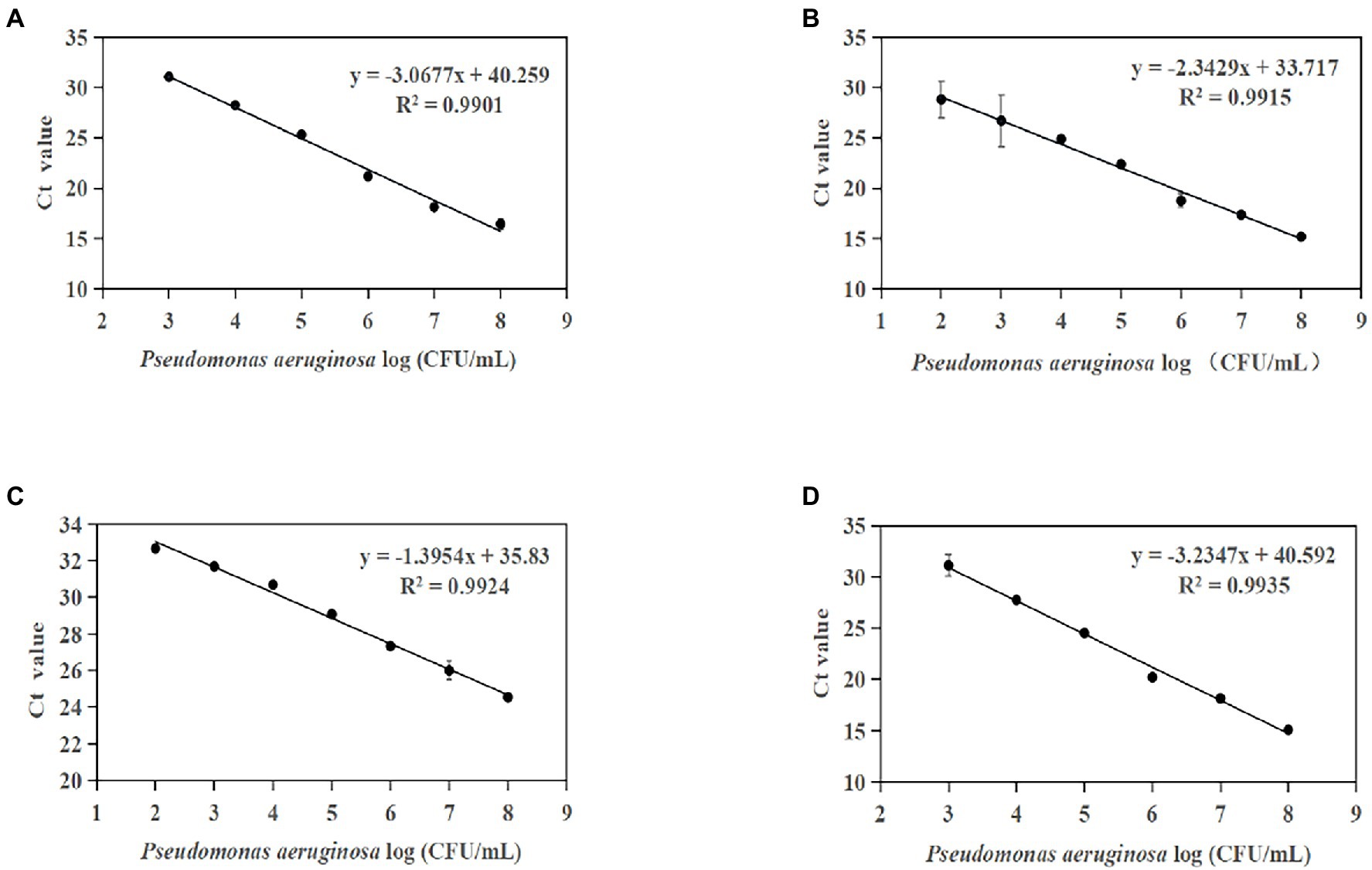
Figure 4. Standard curves established by plotting cycle threshold (Ct) values against the log numbers of P. aeruginosa in pure culture. (A) Primer set PA12 in a range of 103–108 CFU/ml; (B) primer set PA13 in a range of 102–108 CFU/ml; (C) primer set PA14 in a range of 102–108 CFU/ml; and (D) primer set PA15 in a range of 103–108 CFU/ml.
Artificially contaminated tomato was used to evaluate the sensitivity, specificity, and reliability of the primer sets PA1, PA2, PA3, and PA4. The cell concentrations of P. aeruginosa added to tomato were 101–108 CFU/ml. Following PCR detection, cell concentrations of 104–106 CFU/ml were used (Figure 5). The detection limits of the PA1, PA2, PA3, and PA4 primer sets were 1.33 × 106 CFU/ml, 1.33 × 104 CFU/ml, 1.33 × 105 CFU/ml, and 1.33 × 105 CFU/ml, respectively.
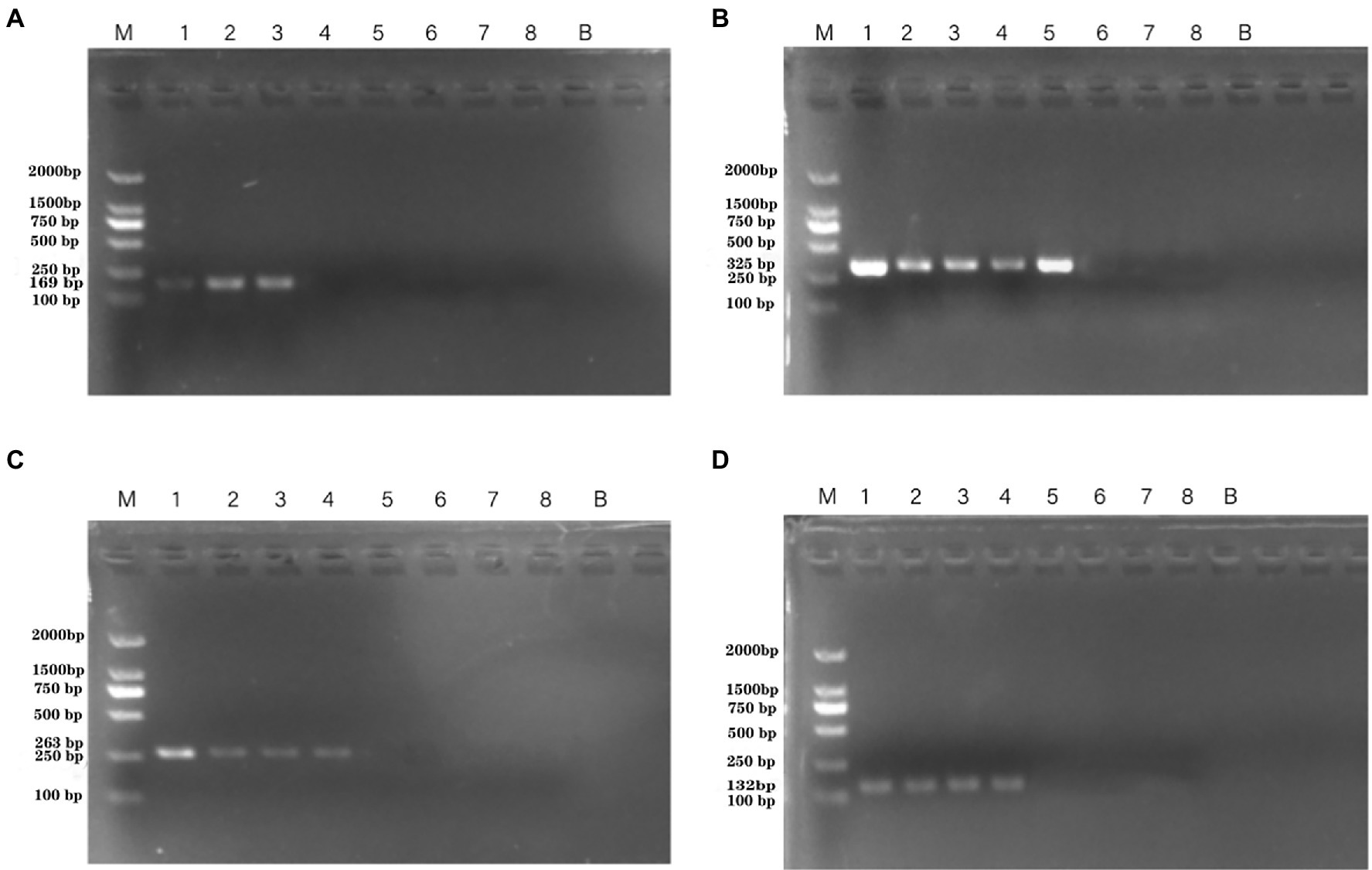
Figure 5. PCR detection sensitivity using dilutions of a pure culture of P. aeruginosa ATCC 15442 in spiked tomato lane M = DSTM 2000 marker (Dongsheng Biotechnology, Guangdong, China); lane N = negative control (double-distilled H2O); and lanes 1–8 = 1.33 × 108 CFU/ml, 1.33 × 107 CFU/ml, 1.33 × 106 CFU/ml, 1.33 × 105 CFU/ml, 1.33 × 104 CFU/ml, 1.33 × 103 CFU/ml, 1.33 × 102 CFU/ml, and 2 × 101 CFU/ml, respectively. (A) Primer set PA1 (169 bp); (B) primer set PA2 (325 bp); (C) primer set PA3 (263 bp); and (D) primer set PA4 (132 bp).
Furthermore, the optimized conditions for the qPCR assay were used to establish a standard curve for P. aeruginosa detection in artificially contaminated samples. The linear detection range of these methods was 1.33 × 102 CFU/g to 1.33 × 108 CFU/g (Figures 6A–D). The four standard curves showed ideal linear correlations, with R2 values of 0.9944 for primer set PA12, 0.9851 for primer set PA13, 0.9814 for primer set PA14, and 0.9853 for primer set PA13. The LOD values of the four novel species-specific targets were 1.33 × 104 CFU/g for primer sets PA12 and PA15, 1.33 × 103 CFU/g for primer sets PA13 and PA14. Compared with the endpoint PCR method, the qPCR method was more sensitive by an order of magnitude.
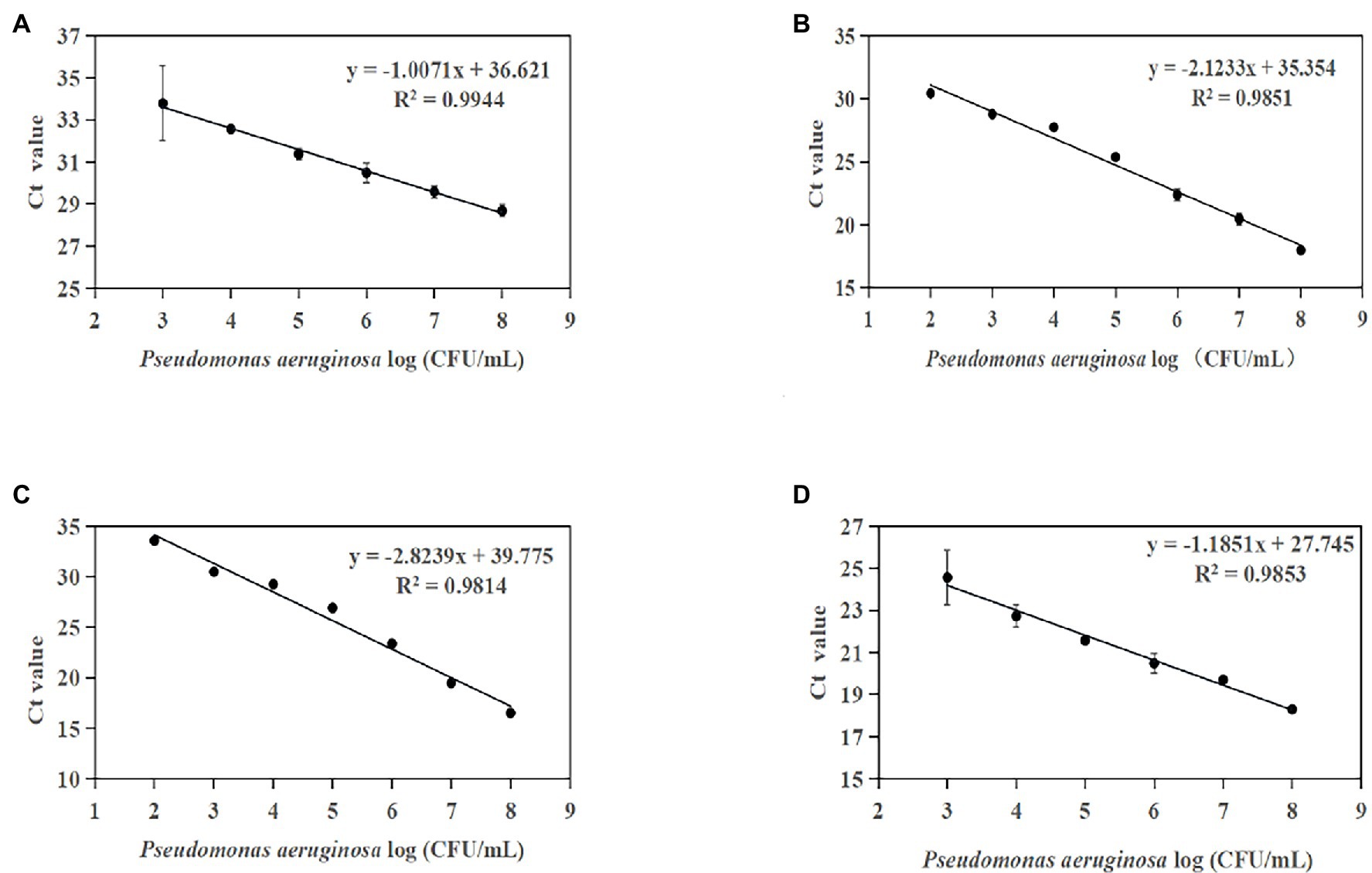
Figure 6. Standard curves established by plotting cycle threshold (Ct) values against the log numbers of P. aeruginosa in pure culture from artificially contaminated tomatoes. (A) Primer set PA12 in a range of 104–108 CFU/ml; (B) primer set PA13 in a range of 103–108 CFU/ml; (C) primer set PA14 in a range of 103–108 CFU/ml; and (D) primer set PA15 in a range of 104–108 CFU/ml.
The susceptibility of the PCR and qPCR assay to interference by nontarget DNA was determined by mixing P. aeruginosa and non-P. aeruginosa strains (E. coli ATCC 25922) at different ratios. Only one clear band was generated for mixtures of all strains tested for the PCR assay. The brightness of the band was comparable to that obtained by analyzing a pure P. aeruginosa culture (Figure 7). All amplifications had similar cycle threshold (Ct) values (Figure 8), regardless of the target-to-interfering strain ratio, suggesting that the presence of non-P. aeruginosa strains (E. coli ATCC 25922) did not interfere with L. monocytogenes serotype 4c detection. This result indicated that even a high abundance of E. coli ATCC 25922 did not interfere with the detection of P. aeruginosa.
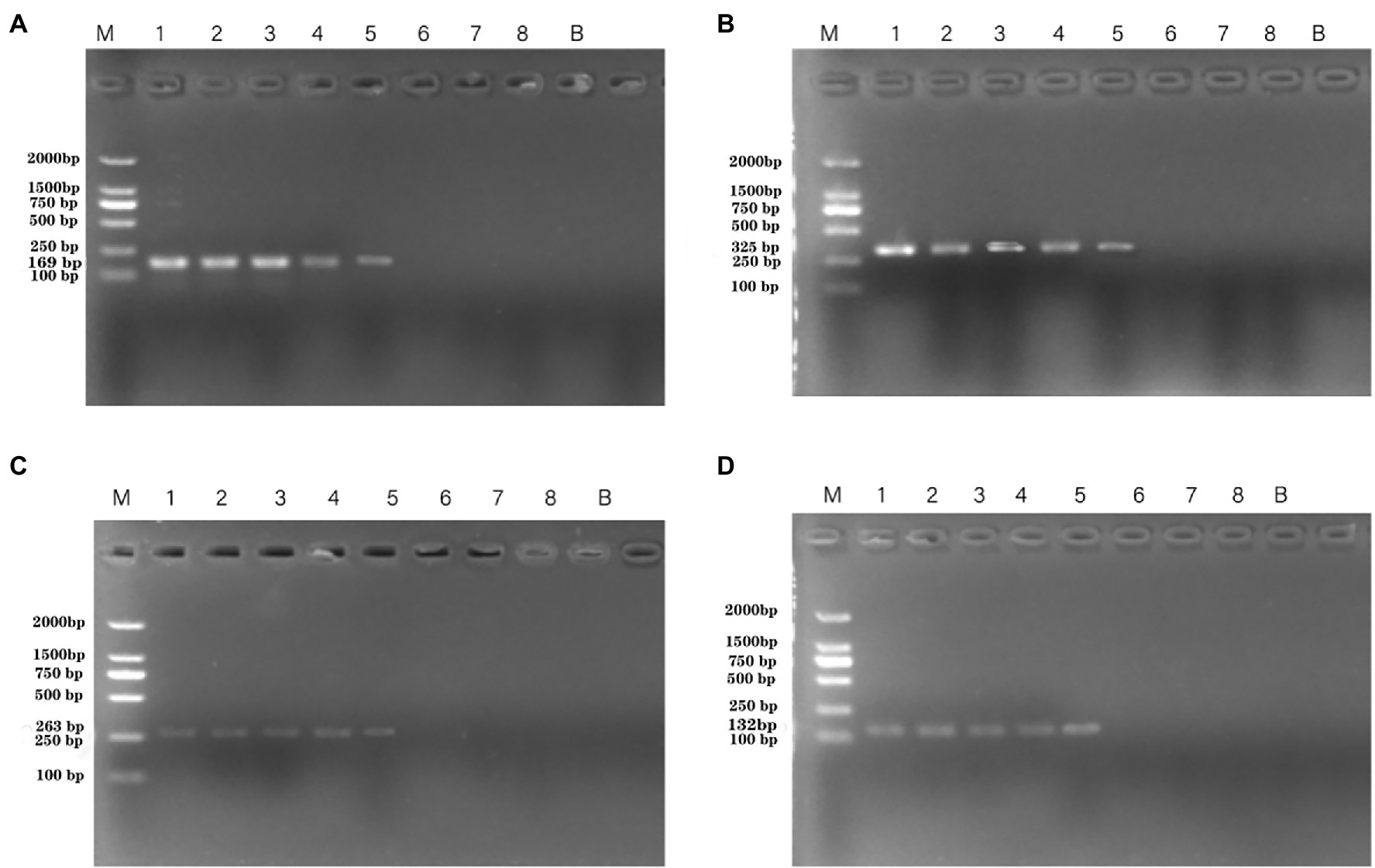
Figure 7. PCR assays using primers targeting P. aeruginosa ATCC 15442 (4.6 × 104 CFU/ml) testing for interference with different concentrations of E. coli ATCC 25922. Lane M = DSTM 2000 marker (Dongsheng Biotechnology, Guangdong, China); lanes 1–5 = mixtures of Escherichia coli ATCC 25922 concentration mixed with 1.462 × 108 CFU/ml, 1.462 × 106 CFU/ml, 1.462 × 104 CFU/ml, 1.9 × 102 CFU/ml, 0 CFU/ml, respectively. (A) Primer set PA1 (169 bp) mixed with E. coli ATCC 25922; (B) primer set PA2 (325 bp) mixed with E. coli ATCC 25922; (C) primer set PA3(263 bp) mixed with E. coli ATCC 25922; and (D) primer set PA4 (132 bp) mixed with E. coli ATCC 25922.
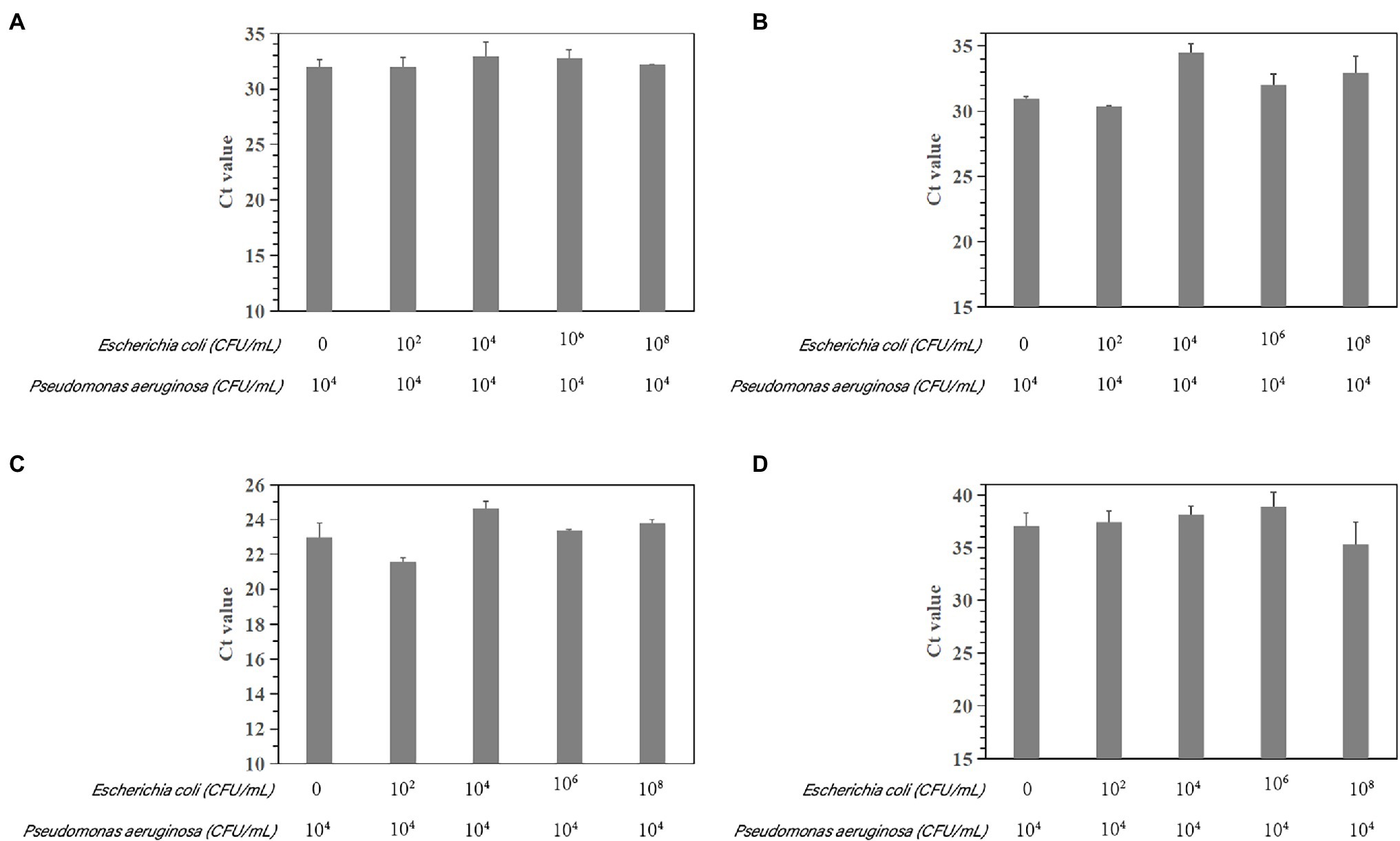
Figure 8. Assessment of interference with the real-time PCR-based detection of P. aeruginosa by coinfection with E. coli ATCC 25922. Detection of P. aeruginosa ATCC 15442 (4.6 × 104 CFU/ml) in the presence of E. coli ATCC 25922 (0, 1.9 × 102 CFU/ml, 1.462 × 104 CFU/ml, 1.462 × 106 CFU/ml, and 1.462 × 108 CFU/ml). (A) Primer set PA12 mixed with E. coli ATCC 25922; (B) primer set PA13 mixed with E. coli ATCC 25922; (C) primer set PA14 mixed with E. coli ATCC 25922; and (D) primer set PA15 mixed with E. coli ATCC 25922.
Application of the PCR Assay for the Analysis of Ready-to-Eat Vegetables
To verify the practicality and effectiveness of the developed PCR and qPCR methods, we next used these assays to detect P. aeruginosa in 29 unspiked ready-to-eat vegetable samples (Table 3). Among the 29 strains identified by the traditional MALDI (BRUKER, Germany) method, 14 ready-to-eat vegetable samples were positive. For species-specific targeting of group_98983 and group_88276 by the PCR and qPCR methods, the overall positive detection rate was 14/29, the same as that obtained with the traditional MALDI method. However, the PCR and qPCR methods with the species-specific target phzA2 and group_75393 were positive for 15 samples, consistent with the rate obtained by qPCRs. These results indicated that the four PCR primers and four qPCR primers designed by the novel species-specific target could be used to achieve the same positive detection results as the traditional MALDI method with the same initial inoculum. The established methods are accurate and reliable for the evaluation of actual samples of ready-to-eat vegetables.

Table 3. Test results for the detection of P. aeruginosa in ready-to-eat vegetable samples obtained using different methods.
Discussion
The identification of P. aeruginosa has traditionally relied on phenotypic and biochemical methods, which take a long time to perform and require extensive hands-on work by the technologist, both for setup and for ongoing evaluation. Genotype-based identification methods circumvent the problem of variable phenotypes to enable more accurate species identification. Recently, molecular techniques have been developed for detecting P. aeruginosa based on its virulence genes, such as toxA, ecfX, fliC, and oprL (Taee et al., 2014; Wang et al., 2019, 2020).
However, deficiency and mutation of some virulence factors in P. aeruginosa strains can result in false results because of existing pathogenic factors, which may cause a potential threat of food poisoning (Baloyi et al., 2021). Since numerous microbial genome sequences have been completed and published with the development of sequencing technology and bioinformatics, many researchers have focused on exploring and screening novel specific target markers that could replace some target genes with poor specificity.
In this study, we developed PCR and qPCR methods to detect P. aeruginosa in food. The methods aimed at new species-specific gene targets were particular and sensitive. Vegetables from retail markets and supermarkets were widely contaminated by P. aeruginosa and have resistant or reduced susceptibilities antibiotic (Rahman et al., 2022). Pseudomonas aeruginosa as spoilage organisms in the ready-to-eat vegetables was distinguished by its capability to persist in highly antibiotic-resistant biofilm accumulation, which seriously affects shelf life (Allydice-Francis and Brown, 2012). While P. aeruginosa is considered an opportunistic pathogen, several reports have indicated that the organism can also cause infections in healthy hosts (Mateu-Borras et al., 2022). In addition, there was evidence that environmental isolates were as virulent as clinical strains (Li et al., 2018; D’Souza et al., 2020). Previous studies have found that P. aeruginosa can highly contaminate vegetables, revealing the potential hazard of salad vegetables and the possibility of food-related outbreaks of disease (Abrahale et al., 2019; Perez-Diaz et al., 2019; Villagran-de La Mora et al., 2020). Therefore, rapid detection of pathogenic P. aeruginosa is crucial in the vegetable supply chain. The consumption of ready-to-eat vegetables contaminated by P. aeruginosa may seriously impact human health.
However, traditional detection methods for P. aeruginosa may cause false positives or missed positives and are considerably time-consuming. Automated systems such as VITEK 2, which walkway system that works on the principle of photometry, promise shorter turnaround times to detect P. aeruginosa, but these systems have a low rate of accuracy in the identification (Torrecillas et al., 2020; Bhalla et al., 2021; Miranda-Ulloa et al., 2021; Pintado-Berninches et al., 2021; Viedma et al., 2021). Immunological approaches use the highly specific binding between antigens and antibodies and facilitate qualitative or quantitative detection that is based on specific reactions resulted from antigen antibody binding (Rainbow et al., 2020). High-sensitivity detection has been reached by modern immunoassay approaches, but their relatively tedious procedures have limited further development (Bhalla et al., 2021; Miranda-Ulloa et al., 2021; Viedma et al., 2021). In addition, greatest drawback of immunofluorescence methods is a low signal-to-noise ratio, which may lower its detection specificity (Pintado-Berninches et al., 2021). Electrochemical analysis can use the electrochemical characteristics of materials for qualitative and quantitative detection, which is fast and sensitive, but it needs compact experimental equipment to complete the experiment (Sabat et al., 2021; Zuccarello et al., 2021). Matrix-assisted laser desorption/ionization time of flight mass spectrometry (MALDI-TOF-MS) states an advanced technology and owns a very good application prospects in identifying P. aeruginosa (Wilhelm et al., 2021). MALDI-TOF-MS was used to accurately and rapidly identify the five high-risk clones of P. aeruginosa sequence ST111, ST175, ST235, ST253, and ST395, also was applied in P. aeruginosa drug resistance analysis such as carbapenemase (Mulet et al., 2021). MALDI-TOF-MS exhibits limited resolving power, and therefore does not supply sequence-based ID necessarily; microbial ID using MALDI-TOF-MS is based on spectral fingerprint patterns rather than the identity of each spectral peak (Ayhan et al., 2021).
The development of PCR-based detection methods for species-specific classification would provide an independent means for confirming species identity (Jami Al-Ahmadi and Zahmatkesh Roodsari, 2016). The current PCR detection methods for P. aeruginosa species target the virulence genes toxA or the 16S rRNA and 23S rRNA genes (Taee et al., 2014; Wang et al., 2016). With the development of sequencing technology and bioinformatics, many microbial genome sequences have been collected. Many researchers have sought to find new novel specific gene targets to replace the current target genes with poor specificity (Taee et al., 2014). Previously, specific target genes for P. aeruginosa was identified from sigma 70-factor sequences available from GenBank2 and then aligned using CLUSTALW software (Chowdhury and Garai, 2017; Wang et al., 2020). Neighbor-joining trees have been computed through the PHYLO_WIN graphical tool (Sánchez-Herrera et al., 2017). Specificity is the key to the success of conventional PCR, but it is also the most important reason for the failure of PCR detection. With the rapid development of whole-genome sequencing and bioinformatics, it has become more economical, convenient, and effective to identify specific targets by pangenome analysis than by using other molecular target screening methods. In this study, we used a large number of genome sequences (n = 2017) for pangenome analysis to identify specific gene targets of P. aeruginosa. According to the pangenome and PCR analyses, four novel P. aeruginosa-specific targets were 100% specific to the targeted P. aeruginosa genomes and did not detect nontarget P. aeruginosa genomes. However, the P. aeruginosa-specific targets reported in the previous studies, including ecfX, 16S rDNA, fliC, exotoxin A, oprI, algD, and oprL, were present in 99.7%, 96.8%, 96.7%, 95%, 99.5%, 89.4%, and 96.9% of the target strains, respectively (Table 4). Except for the fliC gene, which showed low specificity, all of the genes had very high specificity, especially the ecfX and gyrB genes, whose detection was not associated with false positive or false negative results (Tang et al., 2017). In addition, the detection limits of primer pairs (103–104 CFU/ml) corresponding to these new target genes are similar to those of existing molecular detection targets (Tang et al., 2017). Consequently, the specific target of P. aeruginosa obtained by this method has good specificity. Its sensitivity can meet the needs of existing molecular detection methods. Moreover, it can represent the unique detection target of pathogenic P. aeruginosa in ready-to-eat vegetables and their downstream products.
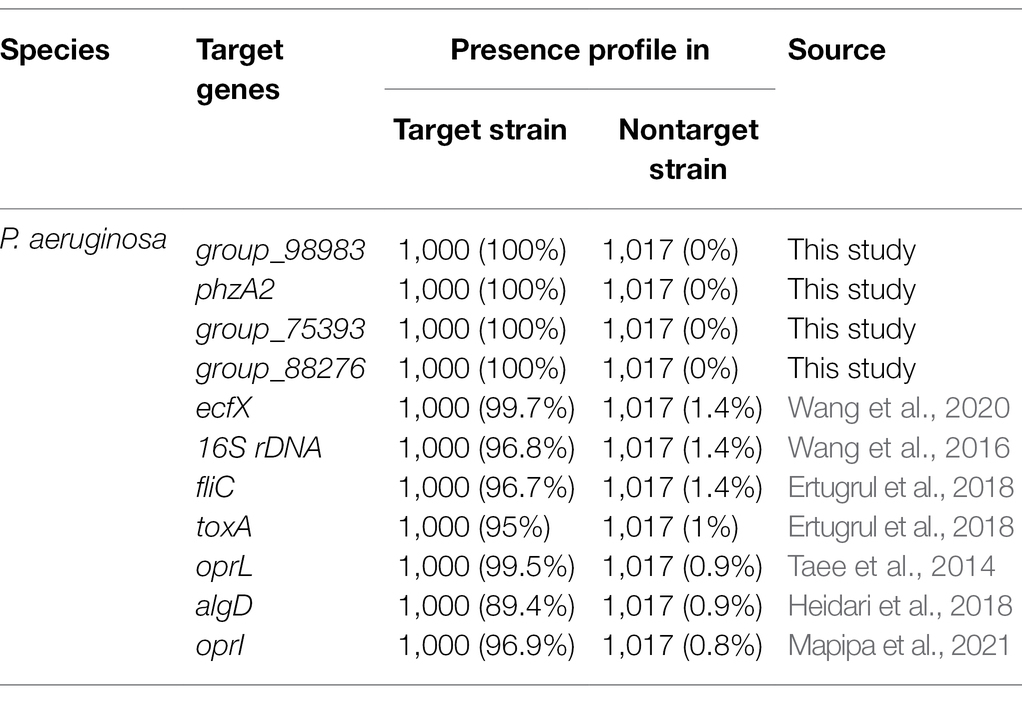
Table 4. Presence profile of novel and reported P. aeruginosa species-specific gene targets for target and nontarget strains.
The PCR assay developed in the current study combines four specific primer sets (PA1, PA2, PA3, and PA4) based on novel molecular markers (UCBPP-PA14_00095, UCBPP-PA14_03237, UCBPP-PA14_04976, and UCBPP-PA14_03627, respectively) and allows simultaneous identification of pathogenic P. aeruginosa. The minimum detection limits of the assays were 103–104 CFU/ml for P. aeruginosa when pure enriched cultures were analyzed, which are comparable to those for PCRs reported in previous studies (Tang et al., 2017). These observations indicated that the new PCR assay could be used to detect P. aeruginosa in samples more rapidly (the overall assay time, including 4–12 h of pre-enrichment, DNA extraction, and the PCR assay, was only 5–17 h) than by using the standard culture method (4–7 days).
We designed the primers PA1, PA2, PA3, and PA4 according to the targets UCBPP-PA14_00095, UCBPP-PA14_03237, UCBPP-PA14_04976, and UCBPP-PA14_03627, respectively. Real-time PCR methods were established on the basis of the above findings. The minimum detection limit of the qPCR assay for P. aeruginosa was 102 CFU/ml. The equations of the qPCR method showed good linearity. These values, comparable to those of most qPCR methods used for foods, were obtained without prior enrichment. Sarabaegi and Roushani (2019) reported a qPCR assay that detected a level of 2.7 × 102 CFU/ml for P. aeruginosa in water.
Similarly, Fortunato et al. (2021) used a qPCR method to detect P. aeruginosa in soil and manure with a detection limit of 104 CFU/g. Notably, the entire assay, including DNA extraction and qPCR, can be completed within 2 h. Compared with other assays, such as traditional culture and conventional PCR methods, the qPCR assay is more sensitive, more specific, time-efficient, and labor-saving.
We applied these methods to detect P. aeruginosa in actual samples of ready-to-eat vegetables, the results of which were consistent with the results of traditional culture methods. The positivity rate of P. aeruginosa was approximately 50% (n = 29), which was equivalent to that for fresh-cut fruits and vegetables (Savic et al., 2021). The positivity rate showed that the contamination of ready-to-eat vegetables by P. aeruginosa was significantly higher than that of other types of food, such as cooked meat products, cold ready-to-eat foods, and drinking water which was 6.25%, 17.65%, and 1.19%, respectively (Cai et al., 2015). This favorable rate of P. aeruginosa was due to dominant flora of vegetable plant saprophytic bacteria (Jin et al., 2021). Pseudomonas aeruginosa carried by water sources and raw materials may also cause contamination at all points in the sequence of vegetable irrigation, circulation, and clean vegetable processing in an open environment and eventually contaminate ready-to-eat vegetable products. It was found that P. aeruginosa strains from vegetables were genetically and functionally similar to clinical isolates in genetics and function (Ambreetha et al., 2021). Therefore, the development of rapid and sensitive detection methods for P. aeruginosa in ready-to-eat vegetables is of great significance to epidemiological research.
In this research, the methods of basic new specific molecular targets face two major limitations in practical application. On the one hand, with the increase of the number of test samples, false positive results may occur. On the other hand, this method belongs to the variable temperature nucleic acid amplification method, which needs to use a specific variable temperature amplification instrument to successfully complete the experiment. Although the method based on new specific targets can grasp the pollution level of P. aeruginosa in ready-to-eat vegetables, there are many uncertainties in the transmission mechanism of P. aeruginosa in the food chain. In subsequent experiments, we plan to analyze the serotype and evolutionary relationship of P. aeruginosa strains isolated from food samples in order to trace the way of P. aeruginosa contamination.
Conclusion
In conclusion, we successfully mined four novel specific target gene sequences of P. aeruginosa with high specificity and sensitivity used pangenome analysis. Based on these new targets, high-specificity and high-sensitivity PCR and qPCR assays were established for rapid detection of P. aeruginosa. Furthermore, the established PCR and qPCR methods were applied to the whole cell detection in practical samples of ready-to-eat vegetables. Comparing the positive results of P. aeruginosa in ready-to-eat vegetables, the detection method based on the new target is consistent with the detection method of standard culture and is not disturbed by nontarget bacteria in the detection environment. Hence, the developed assays based on the novel specific target can be applied for rapid screening and detecting P. aeruginosa in ready-to-eat vegetables, providing a scientific basis for the monitoring of foodborne P. aeruginosa.
Data Availability Statement
The original contributions presented in the study are included in the article/Supplementary Material; further inquiries can be directed to the corresponding authors.
Author Contributions
CW: investigation, methodology, data curation, and writing original draft. QY: project administration and data curation. AJ and JZ: supervision and resources. YS, FL, BZ, XX, QG, RP, and YD: data curation. SW and MC: validation. QW and JW: supervision and writing review and editing. All authors contributed to the article and approved the submitted version.
Funding
This study was supported by National Key Research and Development Program of China (2017YFC1600403), Guangdong Provincial Key Laboratory (2020B121201009) and GDAS’ Special Project of Science and Technology Development (2020GDASYL-20200401002).
Conflict of Interest
The authors declare that the research was conducted in the absence of any commercial or financial relationships that could be construed as a potential conflict of interest.
Publisher’s Note
All claims expressed in this article are solely those of the authors and do not necessarily represent those of their affiliated organizations, or those of the publisher, the editors and the reviewers. Any product that may be evaluated in this article, or claim that may be made by its manufacturer, is not guaranteed or endorsed by the publisher.
Supplementary Material
The Supplementary Material for this article can be found online at: https://www.frontiersin.org/articles/10.3389/fmicb.2022.820431/full#supplementary-material
Footnotes
References
Abrahale, K., Sousa, S., Albuquerque, G., Padrao, P., and Lunet, N. (2019). Street food research worldwide: a scoping review. J. Hum. Nutr. Diet. 32, 152–174. doi: 10.1111/jhn.12604
Allydice-Francis, K., and Brown, P. D. (2012). Diversity of antimicrobial resistance and virulence determinants in Pseudomonas aeruginosa associated with fresh vegetables. Int. J. Microbiol. 2012:426241. doi: 10.1155/2012/426241
Ambreetha, S., Marimuthu, P., Mathee, K., and Balachandar, D. (2021). Rhizospheric and endophytic Pseudomonas aeruginosa in edible vegetable plants share molecular and metabolic traits with clinical isolates. J. Appl. Microbiol. doi: 10.1111/jam.15317
Ayhan, K., Cosansu, S., Orhan-Yanikan, E., and Gulseren, G. (2021). Advance methods for the qualitative and quantitative determination of microorganisms. Microchem. J. 166:106188, 106188. doi: 10.1016/j.microc.2021.106188
Baloyi, I. T., Adeosun, I. J., Yusuf, A. A., and Cosa, S. (2021). In silico and in vitro screening of antipathogenic properties of Melianthus comosus (Vahl) against Pseudomonas aeruginosa. Antibiotics 10, 1–23. doi: 10.3390/antibiotics10060679
Bhalla, M., Heinzinger, L. R., Morenikeji, O. B., Marzullo, B., Thomas, B. N., and Ghanem, E. N. B. (2021). Transcriptome profiling reveals CD73 and age-driven changes in neutrophil responses against Streptococcus pneumoniae. Infect. Immun. 89:e0025821. doi: 10.1128/IAI.00258-21
Cai, S. F., Zhang, Q., and Huang, Y. X. (2015). Monitoring and analysis of Pseudomonas aeruginosa in food. Chin. J. Health Lab. Technol. 26, 875–905.
Chon, J., Jung, J. Y., Ahn, Y., Bae, D., Khan, S., Seo, K., et al. (2021). Detection of campylobacter jejuni from fresh produce: comparison of culture- and PCR-based techniques, and metagenomic approach for analyses of the microbiome before and after enrichment. J. Food Prot. 84, 1704–1712. doi: 10.4315/JFP-20-408
Chowdhury, B., and Garai, G. (2017). A review on multiple sequence alignment from the perspective of genetic algorithm. Genomics 109, 419–431. doi: 10.1016/j.ygeno.2017.06.007
Crull, M. R., Somayaji, R., Ramos, K. J., Caldwell, E., Mayer-Hamblett, N., Aitken, M. L., et al. (2018). Changing rates of chronic Pseudomonas aeruginosa infections in cystic fibrosis: a population-based cohort study. Clin. Infect. Dis. 67, 1089–1095. doi: 10.1093/cid/ciy215
Dharmarha, V., Guron, G., Boyer, R. R., Niemira, B. A., Pruden, A., Strawn, L. K., et al. (2019). Gamma irradiation influences the survival and regrowth of antibiotic-resistant bacteria and antibiotic-resistance genes on romaine lettuce. Front. Microbiol. 10:710. doi: 10.3389/fmicb.2019.00710
D'Souza, C., Prithvisagar, K. S., Deekshit, V. K., Karunasagar, I., and Kumar, B. K. (2020). Exploring the pathogenic potential of Vibrio vulnificus isolated from seafood harvested along the Mangaluru coast, India. Microorganisms 8:999. doi: 10.3390/microorganisms8070999
Ertugrul, B. M., Oryasin, E., Lipsky, B. A., Willke, A., and Bozdogan, B. (2018). Virulence genes fliC, toxA and phzS are common among Pseudomonas aeruginosa isolates from diabetic foot infections. Infect. Dis. (Lond). 50, 273–279. doi: 10.1080/23744235.2017.1393839
Fakhkhari, P., Tajeddin, E., Azimirad, M., Salmanzadeh-Ahrabi, S., Abdi-Ali, A., Nikmanesh, B., et al. (2022). Involvement of Pseudomonas aeruginosa in the occurrence of community and hospital acquired diarrhea, and its virulence diversity among the stool and the environmental samples. Int. J. Environ. Health Res. 32, 61–71. doi: 10.1080/09603123.2020.1726300
Fortunato, G., Vaz-Moreira, I., Nunes, O. C., and Manaia, C. M. (2021). Effect of copper and zinc as sulfate or nitrate salts on soil microbiome dynamics and blaVIM-positive Pseudomonas aeruginosa survival. J. Hazard. Mater. 415:125631. doi: 10.1016/j.jhazmat.2021.125631
Freschi, L., Vincent, A. T., Jeukens, J., Emond-Rheault, J. G., Kukavica-Ibrulj, I., Dupont, M., et al. (2018). The Pseudomonas aeruginosa pan-genome provides new insights on its population structure, horizontal gene transfer, and pathogenicity. Genome Biol. Evol. 11, 109–120. doi: 10.1093/gbe/evy259
Gharieb, R., Saad, M., Khedr, M., El Gohary, A., and Ibrahim, H. (2022). Occurrence, virulence, carbapenem resistance, susceptibility to disinfectants and public health hazard of Pseudomonas aeruginosa isolated from animals, humans and environment in intensive farms. J. Appl. Microbiol. 132, 256–267. doi: 10.1111/jam.15191
Godova, G. V., Ovod, A. A., Astakhova, N. V., and Kalashnikova, Y. A. (2020). Histological study of lettuce and basil by infection with Ps. aeruginosa and Ps. fluorescens in vitro. Izv. Timi. Agri. Acad. 56–69. doi: 10.26897/0021-342X-2020-3-56-69
Heidari, H., Hadadi, M., Sedigh Ebrahim-Saraie, H., Mirzaei, A., Taji, A., Hosseini, S. R., et al. (2018). Characterization of virulence factors, antimicrobial resistance patterns and biofilm formation of Pseudomonas aeruginosa and Staphylococcus spp. strains isolated from corneal infection. J. Fr. Ophtalmol. 41, 823–829. doi: 10.1016/j.jfo.2018.01.012
Hilker, R., Munder, A., Klockgether, J., Losada, P. M., and Tümmler, B. (2014). Interclonal gradient of virulence in the Pseudomonas aeruginosa pangenome from disease and environment. Environ. Microbiol. 17, 29–46. doi: 10.1111/1462-2920.12606
Hölzel, C. S., Tetens, J. L., and Schwaiger, K. (2018). Unraveling the role of vegetables in spreading antimicrobial-resistant bacteria: a need for quantitative risk assessment. Foodborne Pathog. Dis. 15, 671–688. doi: 10.1089/fpd.2018.2501
Jami Al-Ahmadi, G., and Zahmatkesh Roodsari, R. (2016). Fast and specific detection of Pseudomonas aeruginosa from other pseudomonas species by PCR. Ann. Burn. Fire Disasters 29, 264–267.
Jin, S., Ding, Z., and Xie, J. (2021). Study of postharvest quality and antioxidant capacity of freshly cut amaranth after blue LED light treatment. Plants 10:1614. doi: 10.3390/plants10081614
Junaid, K., Ejaz, H., Asim, I., Younas, S., Yasmeen, H., Abdalla, A. E., et al. (2021). Heavy metal tolerance trend in extended-spectrum beta-lactamase encoding strains recovered from food samples. Int. J. Environ. Res. Public Health 18:4718. doi: 10.3390/ijerph18094718
Kapeleka, J. A., Sauli, E., Sadik, O., and Ndakidemi, P. A. (2020). Co-exposure risks of pesticides residues and bacterial contamination in fresh fruits and vegetables under smallholder horticultural production systems in Tanzania. PLoS One 15:e0235345. doi: 10.1371/journal.pone.0235345
Khademi, F., Maarofi, K., Arzanlou, M., Dogaheh, H. P., and Sahebkar, A. (2021). Which missense mutations associated with DNA gyrase and topoisomerase IV are involved in Pseudomonas aeruginosa clinical isolates resistance to ciprofloxacin in Ardabil? Gene Rep. 24:101211. doi: 10.1016/j.genrep.2021.101211
Kidd, T. J., Magalhaes, R. J. S., Paynter, S., and Bell, S. C. (2015). The social network of cystic fibrosis centre care and shared Pseudomonas aeruginosa strain infection: a cross-sectional analysis. Lancet Respir. Med. 3, 640–650. doi: 10.1016/S2213-2600(15)00228-3
Kwok, W. C., Ho, J. C. M., Tam, T. C. C., Ip, M. S. M., and Lam, D. C. L. (2021). Risk factors for Pseudomonas aeruginosa colonization in non-cystic fibrosis bronchiectasis and clinical implications. Respir. Res. 22:132. doi: 10.1186/s12931-021-01729-5
Li, Y., Liu, X., Tang, K., Wang, P., Zeng, Z., Guo, Y., et al. (2018). Excisionase in Pf filamentous prophage controls lysis-lysogeny decision-making in Pseudomonas aeruginosa. Mol. Microbiol. 111, 495–513. doi: 10.1111/mmi.14170
Mapipa, Q., Digban, T. O., Nnolim, N. E., and Nwodo, U. U. (2021). Antibiogram profile and virulence signatures of Pseudomonas aeruginosa isolates recovered from selected agrestic hospital effluents. Sci. Rep. 11:11800. doi: 10.1038/s41598-021-91280-6
Maske, B. L., Pereira, G. V. D. M., Neto, D. P. D. C., Lindner, J. D. D., Letti, L. A. J., Pagnoncelli, M. G., et al. (2021). Presence and persistence of Pseudomonas sp. during Caspian Sea-style spontaneous milk fermentation highlights the importance of safety and regulatory concerns for traditional and ethnic foods. Food Sci. Technol. 41, 273–283. doi: 10.1590/fst.15620
Mateu-Borras, M., Zamorano, L., Gonzalez-Alsina, A., Sanchez-Diener, I., Domenech-Sanchez, A., Oliver, A., et al. (2022). Molecular analysis of the contribution of alkaline protease A and elastase B to the virulence of Pseudomonas aeruginosa bloodstream infections. Front. Cell. Infect. Microbiol. 11:816356. doi: 10.3389/fcimb.2021.816356
Mesinele, J., Ruffin, M., Kemgang, A., Guillot, L., Boelle, P., and Corvol, H. (2022). Risk factors for Pseudomonas aeruginosa airway infection and lung function decline in children with cystic fibrosis. J. Cyst. Fibros. 21, 45–51. doi: 10.1016/j.jcf.2021.09.017
Miranda-Ulloa, E., Romero-Ruiz, S., Amorín-Uscata, B., Serrano-Segura, K., Briceño-Espinoza, R., and Cárdenas-Bustamante, F. (2021). Estandarización y validación de un Western Blot para el diagnóstico del virus de inmunodeficiencia humana. Rev. Fac. Med. Hum. 21, 674–681. doi: 10.25176/rfmh.v21i4.4023
Mulet, X., Fernandez-Esgueva, M., Norte, C., Zamorano, L., Del Barrio-Tofino, E., and Oliver, A. (2021). Validation of MALDI-TOF for the early detection of the ST175 high-risk clone of Pseudomonas aeruginosa in clinical isolates belonging to a Spanish nationwide multicenter study. Enferm. Infecc. Microbiol. Clin. 39, 279–282. doi: 10.1016/j.eimc.2020.05.022
Namaki, M., Habibzadeh, S., Vaez, H., Arzanlou, M., Safarirad, S., Bazghandi, S. A., et al. (2022). Prevalence of resistance genes to biocides in antibiotic-resistant Pseudomonas aeruginosa clinical isolates. Mol. Biol. Rep. 49, 2149–2155. doi: 10.1007/s11033-021-07032-2
Naze, F., Jouen, E., Randriamahazo, R. T., Simac, C., Laurent, P., Bleriot, A., et al. (2010). Pseudomonas aeruginosa outbreak linked to mineral water bottles in a neonatal intensive care unit: fast typing by use of high-resolution melting analysis of a variable-number tandem-repeat locus. J. Clin. Microbiol. 48, 3146–3152. doi: 10.1128/JCM.00402-10
Oliver, A., Mulet, X., Lopez-Causape, C., and Juan, C. (2015). The increasing threat of Pseudomonas aeruginosa high-risk clones. Drug Resistance Updates 21–22, 41–59. doi: 10.1016/j.drup.2015.08.002
Page, A. J., Cummins, C. A., Martin, H., Wong, V. K., Sandra, R., Holden, M., et al. (2015). Roary: rapid large-scale prokaryote pan genome analysis. Bioinformatics 31, 3691–3693. doi: 10.1093/bioinformatics/btv421
Pang, R., Xie, T., Wu, Q., Li, Y., Lei, T., Zhang, J., et al. (2019). Comparative genomic analysis reveals the potential risk of vibrio parahaemolyticus isolated from ready-to-eat foods in China. Front. Microbiol. 10:186. doi: 10.3389/fmicb.2019.00186
Pelegrin, A. C., Palmieri, M., Mirande, C., Oliver, A., Moons, P., Goossens, H., et al. (2021). Pseudomonas aeruginosa: a clinical and genomics update. FEMS Microbiol. Rev. 45:fuab026. doi: 10.1093/femsre/fuab026
Perez-Diaz, I. M., Hayes, J. S., Medina, E., Webber, A. M., Butz, N., Dickey, A. N., et al. (2019). Assessment of the non-lactic acid bacteria microbiota in fresh cucumbers and commercially fermented cucumber pickles brined with 6% NaCl. Food Microbiol. 77, 10–20. doi: 10.1016/j.fm.2018.08.003
Pintado-Berninches, L., Montes-Worboys, A., Manguan-Garcia, C., Arias-Salgado, E. G., Serrano, A., Fernandez-Varas, B., et al. (2021). GSE4-loaded nanoparticles a potential therapy for lung fibrosis that enhances pneumocyte growth, reduces apoptosis and DNA damage. FASEB J. 35:e21422. doi: 10.1096/fj.202001160RR
Rahman, M., Alam, M., Luies, S. K., Kamal, A., Ferdous, S., Lin, A., et al. (2022). Contamination of fresh produce with antibiotic-resistant bacteria and associated risks to human health: a scoping review. Int. J. Environ. Res. Public Health 19:360. doi: 10.3390/ijerph19010360
Rainbow, J., Sedlackova, E., Jiang, S., Maxted, G., Moschou, D., Richtera, L., et al. (2020). Integrated electrochemical biosensors for detection of waterborne pathogens in low-resource settings. Biosensors 10:36. doi: 10.3390/bios10040036
Ruiz-Roldán, L., Rojo-Bezares, B., Lozano, C., López, M., Chichón, G., Torres, C., et al. (2021). Occurrence of Pseudomonas spp. in raw vegetables: molecular and phenotypical analysis of their antimicrobial resistance and virulence-related traits. Int. J. Mol. Sci. 22:12626. doi: 10.3390/ijms222312626
Sabat, A. J., Pantano, D., Akkerboom, V., Bathoorn, E., and Friedrich, A. W. (2021). Pseudomonas aeruginosa and Staphylococcus aureus virulence factors as biomarkers of infection. Biol. Chem. 402, 1565–1573. doi: 10.1515/hsz-2021-0243
Sánchez-Herrera, K., Sandoval, H., Mouniee, D., Ramírez-Durán, N., Bergeron, E., Boiron, P., et al. (2017). Molecular identification of Nocardia species using the sodA gene: identificación molecular de especies de Nocardia utilizando el gen sodA. New Microbes New Infect. 19, 96–116. doi: 10.1016/j.nmni.2017.03.008
Sarabaegi, M., and Roushani, M. (2019). A nano-sized chitosan particle based electrochemical aptasensor for sensitive detection of P. aeruginosa. Anal. Methods 11, 5591–5597. doi: 10.1039/c9ay01509d
Savic, A., Topalic-Trivunovic, L., Velemir, A., Papuga, S., and Kalaba, V. (2021). Attachment and survival of bacteria on apples with the creation of a kinetic mathematical model. Braz. J. Microbiol. 52, 837–846. doi: 10.1007/s42770-021-00425-2
Schroth, M. N., Cho, J. J., Green, S. K., and Kominos, S. D. (2018). Epidemiology of Pseudomonas aeruginosa in agricultural areas. J. Med. Microbiol. 67, 1191–1201. doi: 10.1099/jmm.0.000758
Seemann, T. (2014). Prokka: rapid prokaryotic genome annotation. Bioinformatics 30, 2068–2069. doi: 10.1093/bioinformatics/btu153
Taee, S. R., Khansarinejad, B., Abtahi, H., Najafimosleh, M., and Ghaznavi-Rad, E. (2014). Detection of algD, oprL and exoA genes by new specific primers as an efficient, rapid and accurate procedure for direct diagnosis of Pseudomonas aeruginosa strains in clinical samples. Jundishapur J. Microbiol. 7:e13583. doi: 10.5812/jjm.13583
Tang, Y., Ali, Z., Zou, J., Jin, G., Zhu, J., Yang, J., et al. (2017). Detection methods for Pseudomonas aeruginosa: history and future perspective. RSC Adv. 7, 51789–51800. doi: 10.1039/C7RA09064A
Torrecillas, M., Fuster, B., Belda, M., Del Remedio Guna, M., Tormo, N., and Gimeno, C. (2020). Evaluation of a mass spectrometry and Vitek 2 combined protocol for rapid identification and susceptibility testing of enterobacterales directly from positive blood cultures. Enferm. Infecc. Microbiol. Clin. 38, 375–378. doi: 10.1016/j.eimc.2019.12.012
Tungpradabkul, S., Senapin, S., and Panyim, S. (2005). PCR-based method for isolation of the flagellin genes from Pseudomonas species. J. Gen. Appl. Microbiol. 44, 231–234. doi: 10.1016/S0277-5387(97)00461-0
Viedma, M. D. P. M., Panossian, S., Gifford, K., Garcia, K., Figueroa, I., Parham, L., et al. (2021). Evaluation of ELISA-based multiplex peptides for the detection of human serum antibodies induced by Zika virus infection across various countries. Viruses 13:1319. doi: 10.3390/v13071319
Villagran-de La Mora, Z., Esther Macias-Rodriguez, M., Arratia-Quijada, J., Sughey Gonzalez-Torres, Y., Nuno, K., and Villarruel-Lopez, A. (2020). Clostridium perfringens as foodborne pathogen in broiler production: pathophysiology and potential strategies for controlling necrotic enteritis. Animals 10:1718. doi: 10.3390/ani10091718
Wang, Y., Geng, Y., and Hao, B. (2016). Study on the detection method of Rhodopseudomonas palustris with 16S rDNA PCR. Sichuan Animal & Veterinary Sciences. 5, 20–25.
Wang, J., Xiang, J., Sun, X., Li, R., Jiang, Y., Chen, Z., et al. (2020). Development and evaluation of a real-time recombinase-aid amplification assay for rapid detection of Pseudomonas aeruginosa. Chin. J. Food Hygiene 32, 524–529. doi: 10.13590/j.cjfh.2020.05.010
Wang, Z., Zuo, J., Gong, J., Hu, J., and Han, X. (2019). Development of a multiplex PCR assay for the simultaneous and rapid detection of six pathogenic bacteria in poultry. AMB Express 9:185. doi: 10.1186/s13568-019-0908-0
Wei, L., Qing-Ping, W. U., Zhang, J. M., Ke-Gang, W. U., Guo, W. P., and Que, S. H. (2015). The pollution survey of Pseudomonas aeruginosa in mineral water and spring water and the analyses of virulence genes and antibiotic resistance of the isolates. Microbiol. China 42, 125–132. doi: 10.13344/j.microbiol.china.140331
Wilhelm, C. M., Forni, G. D. R., Carneiro, M. D. S., and Barth, A. L. (2021). Y establishing a quantitative index of meropenem hydrolysis for the detection of KPC- and NDM-producing bacteria by MALDI-TOF MS. J. Microbiol. Methods 187:106268. doi: 10.1016/j.mimet.2021.106268
Wind, L., Keenum, I., Gupta, S., Ray, P., Knowlton, K., Ponder, M., et al. (2021). Integrated metagenomic assessment of multiple pre-harvest control points on lettuce resistomes at field-scale. Front. Microbiol. 12:683410. doi: 10.3389/fmicb.2021.683410
Zhong, Z., Gao, R., Chen, Q., and Jia, L. (2020). Dual-aptamers labeled polydopamine-polyethyleneimine copolymer dots assisted engineering a fluorescence biosensor for sensitive detection of Pseudomonas aeruginosa in food samples. Spectrochim. Acta A 224:117417. doi: 10.1016/j.saa.2019.117417
Zhou, Y., Wan, Q., Cai, Z., Lu, M., Qu, X., Wu, Q., et al. (2020). Development and evaluation of loop-mediated isothermal amplification-based kit for rapid detection of Pseudomonas aeruginosa in packaged drinking water. Microbiology China 47, 1982–1992. doi: 10.13344/j.microbiol.china.190710
Keywords: novel target gene, Pseudomonas aeruginosa, pangenome analysis, PCR, ready-to-eat vegetables
Citation: Wang C, Ye Q, Jiang A, Zhang J, Shang Y, Li F, Zhou B, Xiang X, Gu Q, Pang R, Ding Y, Wu S, Chen M, Wu Q and Wang J (2022) Pseudomonas aeruginosa Detection Using Conventional PCR and Quantitative Real-Time PCR Based on Species-Specific Novel Gene Targets Identified by Pangenome Analysis. Front. Microbiol. 13:820431. doi: 10.3389/fmicb.2022.820431
Edited by:
Mostafa Ghanem, University of Maryland, College Park, United StatesReviewed by:
Sinosh Skariyachan, St. Pius X College, Rajapuram, IndiaQiao Lin, University of Pittsburgh, United States
Copyright © 2022 Wang, Ye, Jiang, Zhang, Shang, Li, Zhou, Xiang, Gu, Pang, Ding, Wu, Chen, Wu and Wang. This is an open-access article distributed under the terms of the Creative Commons Attribution License (CC BY). The use, distribution or reproduction in other forums is permitted, provided the original author(s) and the copyright owner(s) are credited and that the original publication in this journal is cited, in accordance with accepted academic practice. No use, distribution or reproduction is permitted which does not comply with these terms.
*Correspondence: Qingping Wu, wuqp203@163.com; Juan Wang, wangjuan@scau.edu.cn
†These authors have contributed equally to this work