- TBI, CNRS, INRA, INSAT, Université de Toulouse, Toulouse, France
Transport is a crucial step in the metabolism of glycosides by bacteria, which is itself key for microbiota function and equilibrium. However, most transport proteins are function-unknown or only predicted, limiting our understanding of how bacteria utilize glycosides. Here, we present an activity-based screening method to identify functional glycoside transporters from microbiomes. The method is based on the co-expression in Escherichia coli of genes encoding transporters and carbohydrate-active enzymes (CAZymes) from metagenomic polysaccharide utilization loci (PULs) cloned in fosmids. To establish the proof of concept of the methodology, we used two different metagenomic libraries derived from human gut microbiota to select 18 E. coli clones whose metagenomic sequence contained at least one putative glycoside transporter and one functional CAZyme, identified by screening for various glycoside-hydrolase activities. Growth tests were performed on plant-derived glycosides, which are the target substrates of the CAZymes identified in each PUL. This led to the identification of 10 clones that are able to utilize oligosaccharides as sole carbon sources, thanks to the production of transporters from the PTS, ABC, MFS, and SusCD families. Six of the 10 hit clones contain only one transporter, providing direct experimental evidence that these transporters are functional. In the six cases where two transporters are present in the sequence of a clone, the transporters’ function can be predicted from the flanking CAZymes or from similarity with transporters characterized previously, which facilitates further functional characterization. The results expand the understanding of how glycosides are selectively metabolized by bacteria and offers a new approach to screening for glycoside-transporter specificity toward oligosaccharides with defined structures.
Introduction
Bacteria have evolved diverse metabolic lifestyles and strategies to harvest energy from glycosides (Ndeh and Gilbert, 2018). According to the current paradigm, utilization of glycosides in the Bacteroidetes, a dominant phylum of the mammalian gut microbiota, is usually orchestrated by polysaccharide utilization loci (PULs), which encode carbohydrate-active enzymes (CAZymes) associated with SusCD-like transport systems, and sometimes also transporters of the ATP-binding cassette (ABC) or Major Facilitator Superfamily (MFS) families (Terrapon et al., 2018). In another dominant phylum of the mammalian gut microbiota, the Firmicutes, glycosides are metabolized by systems called gpPULs (Gram-positive PULs), which are analogous to canonical PUL systems. Rather than encoding TonB-dependent receptors, though, they code for a range of other transporters, including ABC, MFS, and phosphotransferase systems (PTS) (Sheridan et al., 2016). Depending on their degree of polymerization (DP), polysaccharides are partially degraded by cell surface-associated CAZymes to oligomers, which are then imported into the cell periplasm (for Gram-negative bacteria) or cell cytoplasm (Gram-positive) through transporters. In the cell periplasm or the cytoplasm, oligosaccharides are processed by intracellular CAZymes to monosaccharides that are ultimately assimilated into the central metabolism of the bacteria. Glycoside transporters are therefore critical for glycoside utilization by bacteria, and thus linked to their ability to settle in nutritional niches (Grondin et al., 2017).
Understanding how bacteria recognize and transport glycosides is key to understanding gut microbiota function and thus to improving health and preventing disease (Marques et al., 2018). For instance, ABC transporters are indeed likely to provide Bifidobacteria with a significant advantage in capturing oligosaccharides via their extracellular lipid-anchored solute-binding proteins (SBPs) in the competitive human gut niche (Theilmann et al., 2019). Furthermore, the presence of glycoside-specific PTS or MFS transporters in pathogenic bacteria, including Escherichia coli BEN2908 (Schouler et al., 2009), was found to be correlated with the colonization and virulence of pathogens in the gut. In addition, for biotechnological applications, better knowledge of glycoside uptake will enable the development of microbial cell factories and microbial consortia with the ability to efficiently convert non-refined biomass to high-value products (Hara et al., 2017).
Despite the importance of bacterial glycoside transporters for health and biotechnology, the rate of functional characterization of transporters is much slower than for CAZymes (Lombard et al., 2014). One of the major challenges lies in finding the correct substrates among the extremely wide diversity of oligosaccharides (estimated at as many as a few thousand Lapébie et al., 2019) for each transporter. CAZymes are classified into families based on sequence and mechanistic similarities in the CAZy database, and their function can be predicted based on homology with the numerous biochemically characterized members of the same family (Lombard et al., 2014). For transporters, the classification of membrane transport proteins based on the Transporter Classification (TC) system, which is accessible in the Transporter Classification Database (TCDB), is helpful too, since it incorporates both functional and phylogenetic information (Saier et al., 2016). However, predicting transporter substrate specificity is extremely difficult due to their low sequence similarity and to the fact that the vast majority of annotated glycoside-specific transporters lack experimental validation (Genee et al., 2016; Elbourne et al., 2017). Indeed, there are several challenges associated with the experimental investigation of glycoside transporter function. The usual method of identifying transporter specificity is based on monitoring the accumulation of radiolabeled compounds in whole cells, membrane vesicles or proteoliposomes (Blanvillain et al., 2007; Cao et al., 2011; McCoy et al., 2016). However, these assays can easily fail when the heterologous expressed transmembrane transport proteins are inactive due to targeting, insertion or folding issues, or when the transporters are insoluble or unstable in purification or refolding procedures (Majd et al., 2018). In addition, some substrates are not available in radioactive form or are prohibitively expensive, thus precluding large-scale identification trials. Transporter specificity can also be validated in native strains by transcriptional analysis after cultivating the target strains on glycosides (Martens et al., 2011), via complementation studies (Joglekar et al., 2018) or by analysis of the phenotype of knock-out mutants (Linke et al., 2013). Nevertheless, the success of these approaches is still limited because of (i) the cost of omics studies, which is prohibitive if one wants to screen the metabolization potential of a large panel of oligosaccharides with defined structures, (ii) the difficulty of genetic manipulation with some species, (iii) the fact that functional redundancy often occurs in bacteria (Jensen et al., 2002; Maharjan et al., 2007), (iv) the fact that most bacteria in natural ecosystems are uncultured (Steen et al., 2019). There is thus a need to develop new methods for rapid identification and validation of glycoside-transporter functions.
Here, we present a functional metagenomic approach for rapid identification of glycoside-transporters from uncultured human gut bacteria, which do not require radiolabeled substrates. The specificity of the transport systems was screened via growth assays in micro-plates of fosmid E. coli clones containing metagenomic PULs. These growth assays enabled us to rapidly identify several types of glycoside transporters that are functional in E. coli, revealing novel functions of uncultured bacteria of various taxonomical origins.
Results
Prediction of Potential Glycoside Transporters From Metagenomic Clones
Forty-three metagenomic clones isolated during previous functional screening campaigns of the human gut microbiome for their ability to hydrolyze plant polysaccharides and oligosaccharides (Tasse et al., 2010; Cecchini et al., 2013), and subsequently sequenced, were selected to investigate their ability to transport oligosaccharides. Each of these metagenomic clones comprises within its 30–40 kb DNA insert at least one gene encoding a CAZyme responsible for the glycoside-hydrolase activity being screened for. Of the 43 clones, 26 produced at least one endo-acting glycoside-hydrolase activity. They derived from a 156,000-clone metagenomic library constructed from the feces of a healthy adult with a fiber-rich diet (F library), screened for the degradation of plant polysaccharides via chromogenic assays (Tasse et al., 2010). Seventeen other clones produced at least one glycosidase activity. These were identified from 20,000 clones chosen at random from the F library, and from another 20,000-clone library constructed from the ileum mucosal microbiota (I library), screened for the ability to break down prebiotic oligosaccharides and polysaccharides (Cecchini et al., 2013).
Based on the functional annotation of the metagenomic DNA insert, we found that 18 of the 43 clones described above contained one or two predicted glycoside transport systems (Table 1) from the SusCD, MFS, ABC, and/or PTS families. The substrates chosen to test the transport ability of these 18 clones correspond to the oligosaccharides used to screen for glycosidase activity via positive selection on agar plates (Cecchini et al., 2013) and to the commercially available oligosaccharides which are components of the polysaccharides used to screen for endo-acting glycoside-hydrolase activities on agar plates (Tasse et al., 2010). However, the enzymatic activity of the fosmid clones might be due to CAZyme-encoding genes which are not part of the same operons (while being present in the same large metagenomic fragment) as the transporter-encoding genes, since PUL boundaries cannot be clearly defined without experimental validation (Moreno-Hagelsieb, 2015). We therefore used the list of known activities for each CAZy family, retrieved from the CAZy database (Lombard et al., 2014; Supplementary Table 1), to enlarge the panel of substrates tested for growth ability, based on all the putative activities of the glycoside hydrolase enzymes (GHs) present in the metagenomic sequences (clones marked with an asterisk in Table 1).
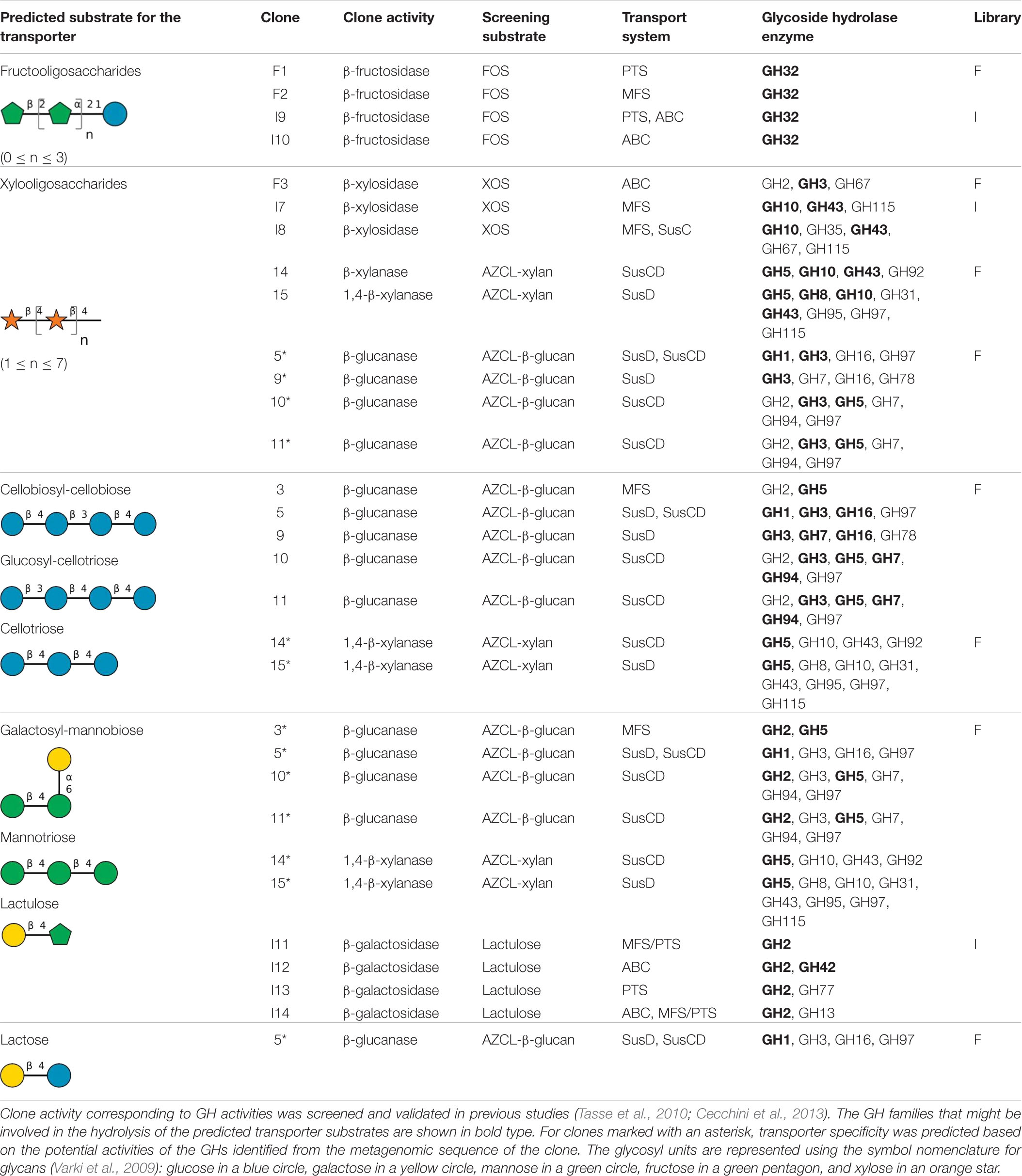
Table 1. Predicted substrate specificity of glycoside transporters of metagenomic clones, and corresponding transporters and CAZyme families identified in the clone sequences.
Screening for Glycoside Metabolizing Pathways
Utilization of glycosides requires the cooperation of transporters and CAZymes. Previously, we proved that metagenomic gene expression in fosmids is not controlled by promoters flanking the cloning site, but rather, by sequences randomly scattered across the metagenomic DNA insert, which are recognized as promoters by E. coli (Tauzin et al., 2016). Therefore, to assess the efficiency of cloning PULs in fosmids to (randomly) co-express at least two genes (encoding a transporter and a glycosidase), and to validate the specificity predictions for the transporters listed in Table 1, growth screening of the 18 metagenomic clones selected for the predicted oligosaccharides was performed in micro-plates. Their growth was characterized by monitoring the optical density value at 600 nm (OD600 nm) in M9 medium supplemented with selected oligosaccharides as sole carbon source within 48 h (Figure 1). The E. coli host transformed with an empty fosmid (named EPI) was used as a negative control. The increasing value of OD600 nm over time compared with that of EPI provides direct evidence of glycoside utilization by the clone tested.
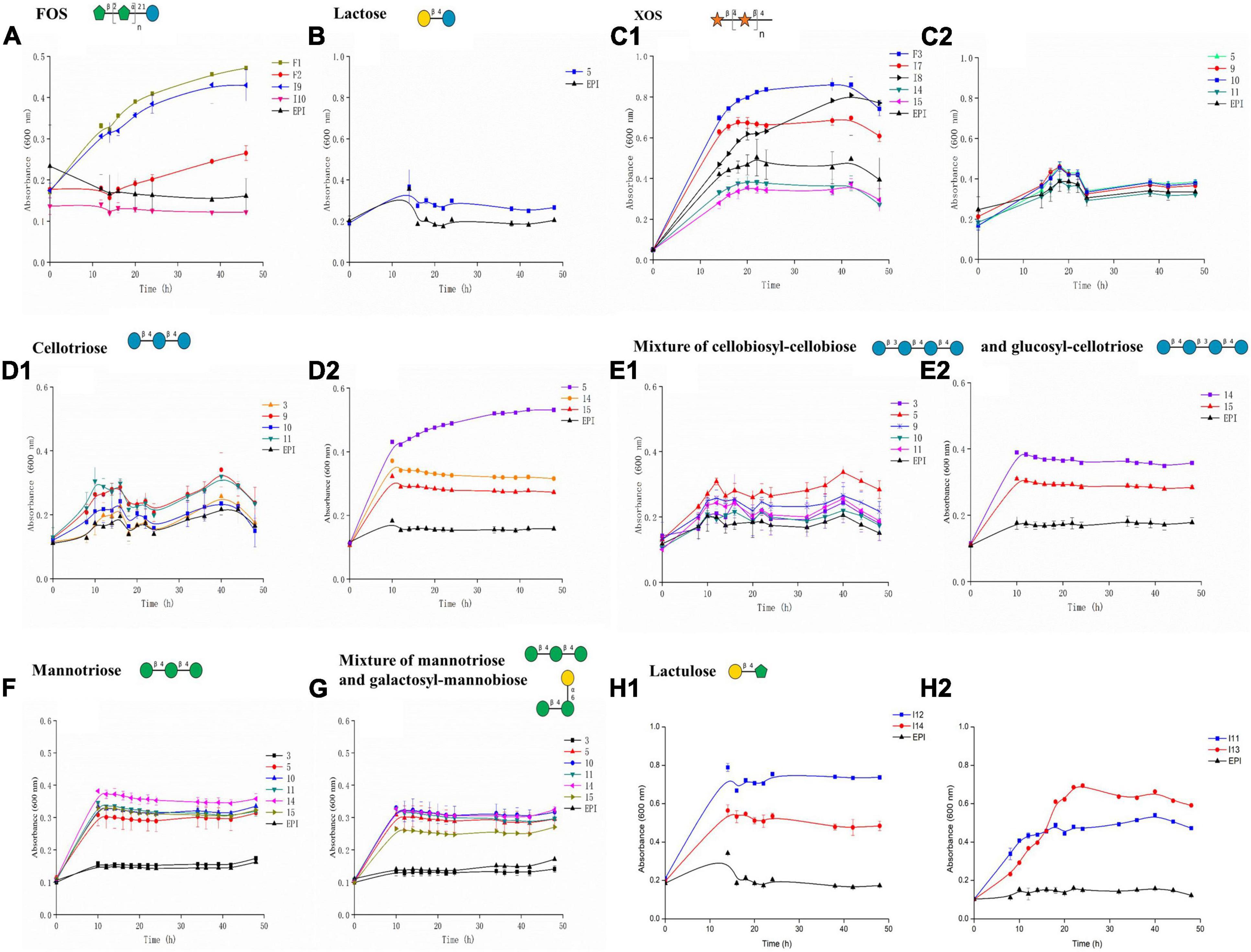
Figure 1. Growth of metagenomic clones on various oligosaccharides used as sole carbon sources: (A) FOS, (B) lactulose, (C) XOS, (D) cellotriose, (E) mixture of cellobiosyl-cellobiose and glucosyl-cellotriose, (F) mannotriose, (G) mixture of galactosyl-mannobiose and mannotriose, and (H) lactulose. The data represent the averages from biological triplicates, and the scale bars represent the standard deviation (SD).
A total of 44 growth assays were carried out for the 18 selected metagenomic clones, resulting in the identification of 10 hit clones with an OD600 nm value exceeding the value of the negative control EPI by 0.2. Such an increase is significant, since the OD600 nm value of the EPI strain increases by 0.3 after 48 h growth on 0.5% (m/v) glucose. The mean total hit yield was 77.1% when the primary screening for GH activity was based on positive selection on oligosaccharides as sole carbon sources, and 14.3% when it was based on chromogenic assays for screening on polysaccharides (Table 2). Based on sequence analyses, the size of the contigs for each of the 10 hit clones exhibits a wide range, from 13,000 to 43,000 bp, and clone I9 contains two contigs for one single metagenomic DNA insert (Table 3). The clone sequences are mainly assigned to Firmicutes and Bacteroidetes, the two dominant phyla in the human gut microbiota. The hit clones possess one or two transport systems including ABC, MFS, PTS, and/or SusCD, and from one to six glycoside hydrolases, variously exhibiting the ability to metabolize FOS, XOS, lactulose, or cellotriose. Clone 5, harboring a GH1, was tested on lactose, but was unable to grow on this substrate. Also, 7 clones were tested on mannooligosaccharides or mixed-linked glucooligosaccharides, since their sequences contain a GH that might be involved in the breakdown of these substrates. However, their growth ability was under the threshold fixed in this study to identify the most efficient transporters expressed in E. coli. Finally, all the 10 hit clones were able to grow on the same oligosaccharides that had been experimentally tested during the initial positive selection on solid medium (clones F1, F3, I7, I8, I9, I11, I12, I13, and I14) or on oligosaccharides derived from the polysaccharide used for chromogenic assays (clone 5).
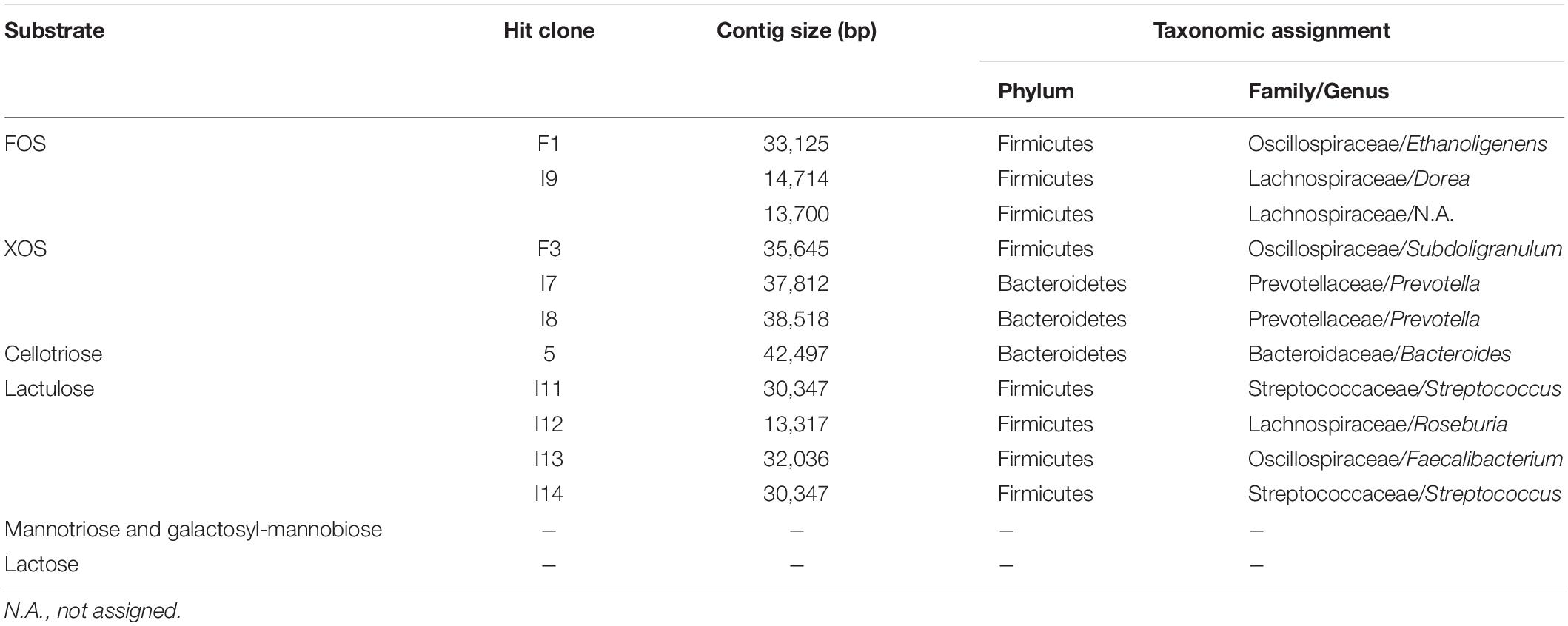
Table 3. Summary of hit clones with the substrate used for growth screening, their contig size and taxonomic assignment at phylum, family or genus.
Identification of Functional Glycoside Transporters
In the metagenomic clones, it is likely that the CAZymes could not be secreted or attached at the cell surface of the recombinant host (Tasse et al., 2010) because of the very low secretion potential of E. coli (Kleiner-Grote et al., 2018). The growth of hit clones thus requires functional transporters to import oligosaccharides into cells for further degradation by CAZymes. Therefore, the strategy of using growth assays for screening clones encoding both transporters and CAZymes provides direct experimental evidence of glycoside transporter functionality.
The sequences of the 10 hit clones shown in Figure 2 include 14 transport systems belonging to the ABC, PTS, MFS, and/or SusCD families. For six clones (F1, F3, I7, I11, I12, and I13), there is only one putative glycoside transporter in each clone (Figure 2), leading to straightforward identification of the likely specificity of the transporter (given that some genes of unknown function are present in the contigs). Among these six clones, we identified a PTS transporter for FOS (clone F1), and an ABC transport system and an MFS transporter for XOS (clones F3 and I7, respectively). For clones able to grow on lactulose, an ABC transport system and a PTS transporter were identified in clones I12 and I13, respectively, while clone I11 harbors a putative protein named “lactose transporter.” This putative protein includes both a PTS_IIA domain and an MFS domain in the polypeptide chain. For the other four clones (I8, I9, 5, and I14), two transport systems were annotated within the same metagenomic insert (Figure 2). These two transport systems might be working in synergy, completely independently or share redundant function. It is difficult to determine which of the two transporters detected was responsible for the substrate internalization in the growth assays based only on the organization of transporter- and CAZyme-encoding genes. It is indeed impossible to define PUL boundaries in the absence of experimental evidence of operon presence in the native strain from which the metagenomic DNA fragments derive, and because PULs could have been truncated during the construction of the metagenomic library. In particular, clone 5 harbors two SusCD transport systems which could belong to two different PULs based on the distance between them and their genomic environment. One of the PULs lacks the SusC protein, likely due to truncation when constructing the metagenomic library. Thus, the functional transporter in clone 5 can only be the entire SusCD system. In the native strain, it is also possible that the two PULs work in synergy, as demonstrated for several PULs such as the pectin, xylan and N-glycan PULs (Cuskin et al., 2015; Rogowski et al., 2015; Luis et al., 2018). In clone I9, it is impossible at this stage to know whether it is the PTS or the ABC transport system that is responsible for the transport of FOS. Clone I7 is partially redundant with clone I8, sharing 99% nucleotide identity from GH115 to GH43, which covers only one transport system. Clone I8 actually has two transport systems, MFS and SusC, while clone I7 harbors only the MFS transporter. The absence of SusD from clone I8, which could be due to truncation of the PUL when constructing the metagenomic library, suggests that the SusC transport system cannot import any glycosides (Koropatkin et al., 2008; Tauzin et al., 2016; Tamura et al., 2019). Although further investigation is required to confirm this hypothesis, this suggests that the MFS transporter is more likely responsible for the uptake of XOS than the incomplete SusCD transport system in clone I8. Two transport systems are present in clone I14, one annotated as a “lactose transporter,” and an ABC transporter. The “lactose transporter”-encoding gene was clustered with the GH2-encoding gene. The DNA segment including both the GH2 and the lactose transporter showed complete synteny with the corresponding part of sequence I11. This sequence contains just one transport system, shown here to be responsible for the use of lactulose. This implies that the “lactose transporter” might be responsible for the import of lactulose by clone I14 too. Nevertheless, even though the I14 ABC transport system is remote from the “lactose transporter” and the GH2, at this stage we cannot exclude that the ABC might be involved in lactulose utilization too.
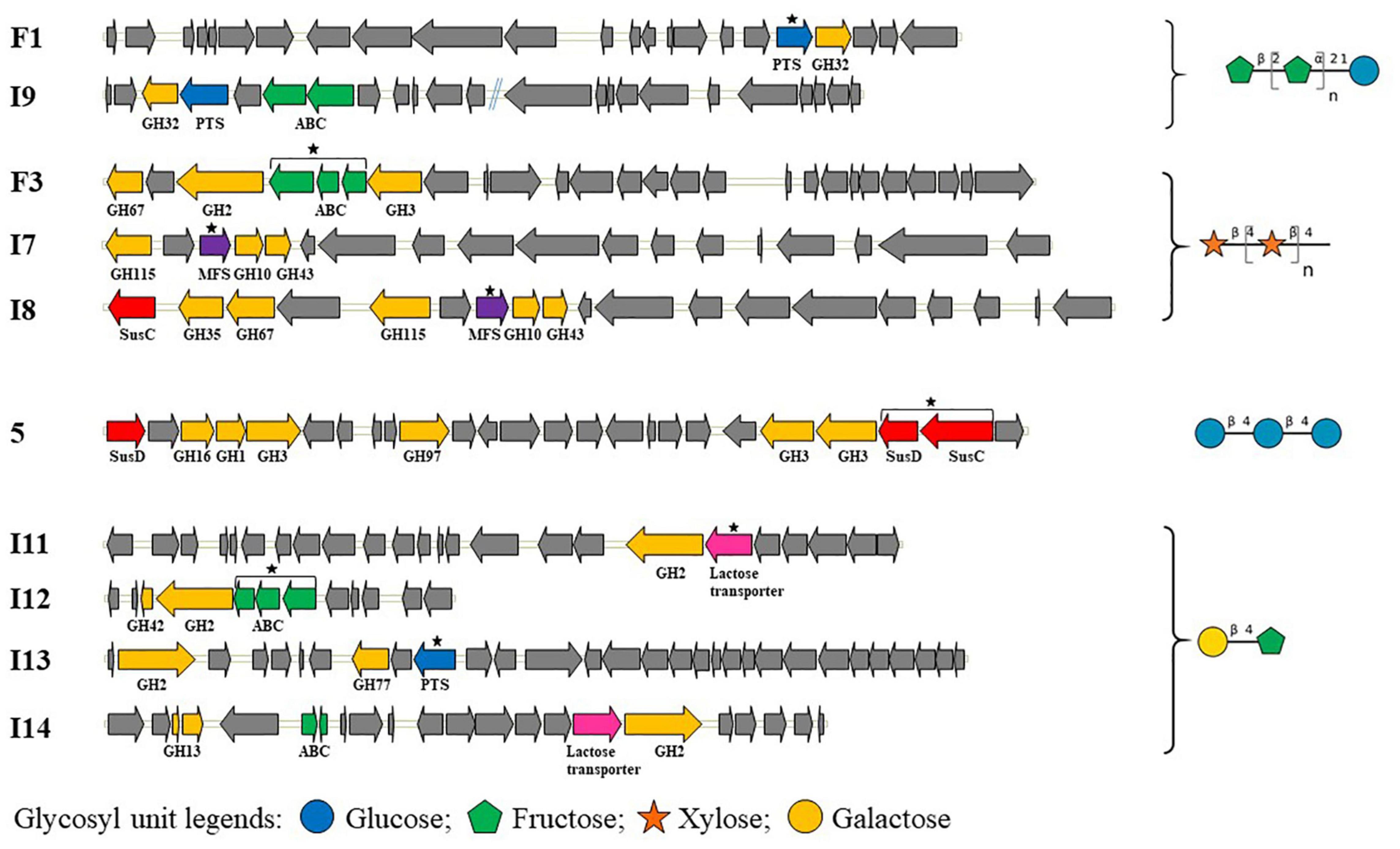
Figure 2. Gene organization of hit clones able to grow on different glycosides. Black stars denote the transporters predicted to be responsible for the clone phenotypes, because they are the only glycoside transport systems detected in the metagenomic PULs or because the SusD component of the SusCD system is missing. The two contigs of clone I9 are separated by blue double lines. Genes are represented by arrows and colored based on their function: genes encoding GHs are shown in yellow, glycoside transporters in blue for PTS, green for ABC, red for SusCD, purple for MFS and pink for “lactose transporter,” and other functions in gray.
Discussion
Development of a Screening Method to Identify Glycoside Transporters From Uncultured Bacteria
Glycoside metabolism in bacteria is orchestrated by specific operons involving glycoside-degrading enzymes and transporters, for which the generic PUL appellation can be used (Ndeh and Gilbert, 2018). Here, basing our study on the PUL paradigm, we screened the specificity of glycoside transporters from uncultured bacteria, the sequences of which were previously identified by activity-based functional metagenomics targeting GH activities. We identified 10 metagenomic PULs (three being partially or fully redundant) with glycoside transporter components that are functional in E. coli.
In total, 14 transporters of four types (PTS, ABC, MFS, and SusCD) were highlighted from only 44 growth assays, demonstrating the efficiency of this strategy for identifying glycoside transporters with various specificities, structures and transport mechanisms. The present method is highly reproducible, quantitative, and can be performed at medium throughput, screening around 50 clones in 2 days by automatically monitoring growth with a thermostatic microplate reader. This assay requires less than 10 mg of oligosaccharides for each clone, tested in triplicate. It does not require the use of chromogenic, fluorogenic, or radiolabeled substrates. It thus enables easy screening of transporter specificity toward the natural oligosaccharides that bacteria need to take up in nature, preventing positive clones from being missed and avoiding false positives with chemically modified substrates, which have a different structure from natural compounds. Screening for oligosaccharides also provides direct access to transporter function, in contrast to transcriptomic studies which are based on the use of the polymeric substrate before any enzymatic degradation to trigger PUL expression. It also requires 10 times less substrate than the growth assays required for transcriptomic analyses (Martens et al., 2011), which require sufficient cells to be retrieved for RNA extraction and sequencing. And of course, our approach allows for screening for the specificity of transporters from uncultured bacteria.
Nevertheless, there are some limitations in screening for transporter specificity in recombinant E. coli cells transformed with PUL-containing fosmids. First, the yield of hit clones producing both a functional glycosidase and a functional transporter is low. As shown in this study, depending on the approach taken in the primary screen targeting GH activity, the hit yield varies between 14 and 77%. Of course, the highest hit yield was obtained when the primary screen was a solid-plate assay based on positive selection on oligosaccharides used as sole carbon sources. Even if the screening principles appear identical in solid medium and in liquid medium, they are in fact slightly different. In solid plate screening campaigns, automates grid several cells of the same clone at a single location on the agar plate. In the GH producing hits, when one of these cells breaks down, it triggers nearby hydrolytic activity. Monosaccharides are then produced in the immediate environment of the other cells in the clonal population, allowing them to grow in turn, and thereby making it easy to visualize cell growth. This principle explains why the hit yield is not 100% after the secondary screening for the transport functions in liquid medium. Screening in liquid medium could be carried out directly in a single stage, without primary screening for GH function. This would require 10 times more substrate for each clone (without replicates), though, and the maximum hit yield would drop to 0.7‰. This value corresponds to the maximum mean probability (4.7‰) of isolating a clone producing an active GH from a library of fosmid clones [8‰ for endo-acting GHs (Tasse et al., 2010) and 1.35‰ for glycosidases (Cecchini et al., 2013) multiplied by that of isolating a functional transporter from GHs producing E. coli fosmid clones (14%, as shown in the present study)]. In addition, the probability of producing both a functional glycosidase and a functional transporter from a (meta)genomic PUL cloned into a fosmid is lower than 4.8%, since no hit was obtained from the 21 assays performed based on sequence analysis. This low hit yield is due to several reasons. Firstly, in fosmids, gene expression is spurious as a result of the random presence of sigma factor sequences along the fosmid DNA insert, which are recognized by E. coli to trigger gene expression (Tauzin et al., 2016). Secondly, for some multi-domains transporters, several genes of the metagenomic locus have to be concomitantly expressed at similar levels. Finally, to be functional, the recombinant transporters must be properly addressed and folded into the cell membranes of the host strain, and, for some of them, functionally complemented by E. coli components, such as TonB for SusCD systems or the phosphoenolpyruvate dependent glycoside phosphorylation machinery for PTSs (Wang et al., 2020).
Screening the transporter function by expressing the genes of PULs in E. coli fosmid clones is thus a strategy which requires automated screening facilities. It is also a costly approach, but one for which the cost could be reduced by 106 by using droplet milli/microfluidics, as we recently showed (Dagkesamanskaya et al., 2018). Lastly, by using this technology, the transporter function can be proven only after truncation of the PUL in cases where there are several identified transporter sequences in the cloned DNA fragment (Tauzin et al., 2016), and also in order to exclude a potential role of unknown proteins whose function cannot be predicted in the phenotype detected.
Major Facilitator Superfamily, ATP-Binding Cassette, Phosphotransferase Systems, and SusCD Transporters From Various Gram-Positive and Gram-Negative Uncultured Bacteria Are Functional in the E. coli Membrane
All the metagenomic DNA sequences of the hit clones were assigned to the two main phyla in mammalian gut microbiomes, Bacteroidetes and Firmicutes. Sequences from bacteria of five different families and eight different genera were identified. However, in any case, it was not possible to annotate the hit sequences to species level, highlighting the functional diversity that remains to be discovered from gut microbiomes by using metagenomics.
Bacteroidetes transporters from both the MFS and SusCD families were highlighted in the present study. We previously demonstrated that these two types of membrane proteins can be functional when produced in recombinant E. coli cells, whose membrane structure is similar to that of Bacteroidetes, both being Gram-negative bacteria (Tauzin et al., 2016). No PTS or ABC systems were identified in these Bacteroidetes sequences. This is logical for PTS, which, to our knowledge, have never been reported in Bacteroidetes, in contrast to MFS and ABC (Tauzin et al., 2016; Zafar and Saier, 2018) which are described in this phylum, although no Bacteroidetes ABC transporter has previously been shown to be involved in carbohydrate transport.
We identified a greater diversity of transporter types from Firmicutes, since three PTS, four ABC, and two lactose transporters including one PTS_IIA domain and one MFS domain were highlighted in the Firmicutes’ PULs. MFS, ABC, and PTS systems are all used by native E. coli strains for nutrient uptake (Saier, 2000). In addition, several PTS involved in oligosaccharide utilization by Gram-positive bacteria were previously successfully expressed in E. coli (Lai and Ingram, 1993; Lai et al., 1997; Wang et al., 2020), despite the difference in cell membrane organization. The localization in E. coli of the different components of the transporters identified here from Gram-positive bacteria will need to be explored.
Non-canonical ATP-Binding Cassette Transporters Are Involved in Oligosaccharide Utilization by Bacteria
Among the ABC transport systems identified, there are two that lack an SBP in clones I9 and I14. Further characterization of this SBP-deficient ABC transporter should shed new light on the mechanism of ABC transporters involved in carbohydrate utilization. With regard to the PTS transport systems, the F1 system lacks the PTS_EIIA domain [the inner carbohydrate-specific domain through which the phosphate group transits to the PTS_EIIB domain and, ultimately, to the oligosaccharide internalized through the transmembrane PTS_EIIC component (Saier, 2015)]. Moreover, the “lactose transporter” identified in clones I11 and I14 for lactulose uptake are original proteins, as the “lactose transporter” contains two domains (a PTS_EIIA and an MFS) belonging to two different types of transporters. The DNA sequences encoding the “lactose transporter” and GH2 of clones I11 and I14 are found near-identical in the genomes of numerous Streptococcus thermophilus strains. This demonstrates that the chimeric organization of the transporters identified is not an artifact of read assembly in our metagenomic sequences. The characterization of the role of each component of this I11/I14 original transporter will need to be determined by protein engineering.
Conclusion
The main purpose of this study was to identify functional transporters from uncultured bacteria. The approach we described in this paper allowed us to identify functional transporters from various families and of different taxonomical origins. We demonstrated the generic application of this strategy, which is compatible with all kinds of known oligosaccharide transporter families described to date, regardless of the Gram status of the bacteria from which they originate. It is now possible to prove transporter translocation function at medium throughput, while other recombinant approaches only target binding ability. We highlighted several gene clusters involved in dietary fiber utilization by gut bacteria, including FOS and lactulose, which are widely used as prebiotics (Bindels et al., 2015). The detailed mechanism and specificity of each of these transporters, especially those with the most novel structure or whose genes are the most abundant in gut microbiomes, will need to be deciphered in future studies.
Materials and Methods
Metagenomic Clones
The metagenomic clones used for the present screening were obtained from the F or I metagenomic libraries constructed as previously described (Tasse et al., 2010; Cecchini et al., 2013). Briefly, the F library was derived from a fecal sample collected from a healthy 30-year-old male who followed a pescatarian diet. The individual did not eat any functional food such as prebiotics or probiotics, nor did he receive any antibiotics or other drugs in the 6 months prior to sampling. The I library was derived from a distal ileum sample obtained from a 51-year-old male patient undergoing colonoscopy and surgery for suspected lower colon cancer, after undergoing preparatory cleansing. The metagenomic DNA fragments were cloned into pCC1FOS fosmids and transformed into EPI100 E. coli cells (Epicenter Technologies).
Carbohydrate Substrates
The following commercial prebiotic oligosaccharides and polysaccharides were used for screening: fructooligosaccharides (Actilight-FOS, Beghin Meiji, France), xylooligosaccharides (Iro Taihe International, China), lactulose (TEVA, France), lactose (Sigma), cellobiosyl-cellobiose (Megazyme), glucosyl-cellotriose (Megazyme), cellotriose (Megazyme), galactosyl-mannobiose (Megazyme), and mannotriose (Megazyme). All of them were prepared at 10 mg/mL in sterilized MilliQH2O and then filtered using a 0.20 mm Minisart RC4 syringe filter.
Growth Assays
All the metagenomic clones tested in our study were stored in glycerol stocks stored at −80°C. They were recovered on Luria-Bertani (LB) agar plates supplemented with 12.5 mg/l chloramphenicol. Single colony inoculates were then grown overnight at 37°C in tubes containing 2 mL LB broth supplemented with 12.5 mg/L chloramphenicol, with orbital shaking at 200 rpm. Cultures in 48-well microplates of 500 μL of M9 medium supplemented with 12.5 mg/L chloramphenicol and 0.5% (w/v) oligosaccharides were then started at an initial OD600 nm of 0.05 by inoculating the wells from the overnight precultures. The M9 medium contained 17.4 g/L Na2HPO4⋅12H2O, 3.03 g/L KH2PO4, 0.51 g/L NaCl, 2.04 g/L NH4Cl, 0.49 g/L MgSO4, 4.38 mg/L CaCl2, 15 mg/L Na2EDTA⋅2H2O, 4.5 mg/L ZnSO4⋅7H2O, 0.3 mg/L CoCl2⋅6H2O, 1 mg/L MnCl2⋅4H2O, 1 mg/L H3BO3, 0.4 mg/L Na2MoO4⋅2H2O, 3 mg/L FeSO4⋅7H2O, 0.3 mg/L CuSO4⋅5H2O, 0.1 g/L thiamine, and 0.02 g/L leucine. Cell growth was monitored at 37°C, with orbital shaking at 600 rpm. The optical density of the cultures was measured at 600 nm over 48 h, using a plate reader (Infinite M200pro, 593 TECAN).
Bioinformatic Analysis
The functional annotation of the metagenomic sequences was retrieved from previous studies (Tasse et al., 2010; Cecchini et al., 2013). Functional annotation of the transporter-encoding genes was confirmed by analyzing the conserved domains of the putative proteins against the NCBI Conserved Domains Database using BLAST (the NCBI Basic Local Alignment Search Tool), with an E-value threshold of 0.01 (Marchler-Bauer et al., 2017). When an incomplete transport system was detected (missing the neighboring SusD gene, or one of the components of canonical PTS and ABC transporters), we checked for the presence of the complementary component by analyzing the conserved domains of the two proteins encoded by the genes before or after the main transporter-encoding gene. Sequence identity between metagenomic DNA inserts was analyzed using BLAST (Boratyn et al., 2013). Taxonomic assignment of the contig sequences was performed using PhyloPythiaS, model Generic 2013–800 Genera1 (Patil et al., 2012).
Data Availability Statement
The datasets presented in this study can be found in online repositories. The names of the repository/repositories and accession number(s) can be found below: https://www.ncbi.nlm.nih.gov/genbank/, GU942948.1; www.ncbi.nlm.nih.gov/genbank/, HE663537; www.ncbi.nlm.nih.gov/genbank/, HE717013; www.ncbi.nlm.nih.gov/genbank/, HE717008; www.ncbi.nlm.nih.gov/genbank/, HE717009; www.ncbi.nlm.nih. gov/genbank/, HE717006; www.ncbi.nlm.nih.gov/genbank/, HE717007; https://www.ncbi.nlm.nih.gov/genbank/, HE717018; www.ncbi.nlm.nih.gov/genbank/, HE717019; www.ncbi.nlm.nih. gov/genbank/, HE717014; and www.ncbi.nlm.nih.gov/genbank/, HE717020.
Author Contributions
GP-V: conceptualization, writing—review and editing, and resources. AT, ZW, and EL: methodology and investigation. ZW and AT: writing—original draft. GP-V, AT, and ZW: funding acquisition. GP-V and AT: supervision. All authors contributed to the article and approved the submitted version.
Funding
This study was carried out using the equipment at the PICT-ICEO facility, which was supported by grants from the Région Midi-Pyrénées, the European Regional Development Fund and the Institut National de la Recherche Agronomique et de l’Environnement (INRAE). This research was funded by the European Union’s Horizon 2020 Framework Programme (MSCA-IF-2015_707457, CaTSYS, and LEIT-BIO-2015-685474, Metafluidics). ZW was supported by the INSA Toulouse and the China Scholarship Council under the “CSC—UT/INSA Program.”
Conflict of Interest
The authors declare that the research was conducted in the absence of any commercial or financial relationships that could be construed as a potential conflict of interest.
Publisher’s Note
All claims expressed in this article are solely those of the authors and do not necessarily represent those of their affiliated organizations, or those of the publisher, the editors and the reviewers. Any product that may be evaluated in this article, or claim that may be made by its manufacturer, is not guaranteed or endorsed by the publisher.
Supplementary Material
The Supplementary Material for this article can be found online at: https://www.frontiersin.org/articles/10.3389/fmicb.2022.816462/full#supplementary-material
Supplementary Table 1 | Known enzymatic activity of glycoside hydrolase (GH) families in the selected clones based on the CAZy database and the substrates selected (based on activities in bold) for growth tests.
Footnotes
References
Bindels, L. B., Delzenne, N. M., Cani, P. D., and Walter, J. (2015). Towards a more comprehensive concept for prebiotics. Nat. Rev. Gastroenterol. Hepatol. 12, 303–310. doi: 10.1038/nrgastro.2015.47
Blanvillain, S., Meyer, D., Boulanger, A., Lautier, M., Guynet, C., Denancé, N., et al. (2007). Plant carbohydrate scavenging through TonB-dependent receptors: a feature shared by phytopathogenic and aquatic bacteria. PLoS One 2:e224. doi: 10.1371/journal.pone.0000224
Boratyn, G. M., Camacho, C., Cooper, P. S., Coulouris, G., Fong, A., Ma, N., et al. (2013). BLAST: a more efficient report with usability improvements. Nucleic Acids Res. 41, 29–33. doi: 10.1093/nar/gkt282
Cao, Y., Jin, X., Levin, E. J., Huang, H., Zong, Y., Quick, M., et al. (2011). Crystal structure of a phosphorylation-coupled saccharide transporter. Nature 473, 50–54. doi: 10.1038/nature09939
Cecchini, D. A., Laville, E., Laguerre, S., Robe, P., Leclerc, M., Doré, J., et al. (2013). Functional Metagenomics reveals novel pathways of prebiotic breakdown by human gut bacteria. PLoS One 8:e72766. doi: 10.1371/journal.pone.0072766
Cuskin, F., Lowe, E. C., Temple, M. J., Zhu, Y., Cameron, E. A., Pudlo, N. A., et al. (2015). Human gut Bacteroidetes can utilize yeast Mannan through a selfish mechanism. Nature 517, 165–169. doi: 10.1038/nature13995
Dagkesamanskaya, A., Langer, K., Tauzin, A. S., Rouzeau, C., Lestrade, D., Potocki-Veronese, G., et al. (2018). Use of photoswitchable fluorescent proteins for droplet-based microfluidic screening. J. Microbiol. Methods 147, 59–65. doi: 10.1016/j.mimet.2018.03.001
Elbourne, L. D. H., Tetu, S. G., Hassan, K. A., and Paulsen, I. T. (2017). TransportDB 2.0: a database for exploring membrane transporters in sequenced genomes from all domains of life. Nucleic Acids Res. 45, D320–D324. doi: 10.1093/nar/gkw1068
Genee, H. J., Bali, A. P., Petersen, S. D., Siedler, S., Bonde, M. T., Gronenberg, L. S., et al. (2016). Functional mining of transporters using synthetic selections. Nat. Chem. Biol. 12, 1015–1022. doi: 10.1038/nchembio.2189
Grondin, J. M., Tamura, K., Déjean, G., Abbott, D. W., and Brumer, H. (2017). Polysaccharide Utilization Loci: fuelling microbial communities. J. Bacteriol. 199:e00860-16. doi: 10.1128/JB.00860-16
Hara, K. Y., Kobayashi, J., Yamada, R., Sasaki, D., Kuriya, Y., Hirono-Hara, Y., et al. (2017). Transporter engineering in biomass utilization by yeast. FEMS Yeast Res. 17, 1–14. doi: 10.1093/femsyr/fox061
Jensen, J. B., Peters, N. K., and Bhuvaneswari, T. V. (2002). Redundancy in periplasmic binding protein-dependent transport systems for trehalose, sucrose, and maltose in Sinorhizobium meliloti. J. Bacteriol. 184, 2978–2986. doi: 10.1128/JB.184.11.2978-2986.2002
Joglekar, P., Sonnenburg, E. D., Higginbottom, S. K., Earle, K. A., Morland, C., Shapiro-Ward, S., et al. (2018). Genetic variation of the SusC/SusD homologs from a polysaccharide utilization locus underlies divergent fructan specificities and functional adaptation in Bacteroides thetaiotaomicron strains. mSphere 3:e00185-18. doi: 10.1128/mSphereDirect.00185-18
Kleiner-Grote, G. R. M., Risse, J. M., and Friehs, K. (2018). Secretion of recombinant proteins from E. coli. Eng. Life Sci. 18, 532–550. doi: 10.1002/elsc.201700200
Koropatkin, N. M., Martens, E. C., Gordon, J. I., and Smith, T. J. (2008). Starch catabolism by a prominent human gut symbiont is directed by the recognition of amylose helices. Structure 16, 1105–1115. doi: 10.1016/j.str.2008.03.017
Lai, X., and Ingram, L. O. (1993). Cloning and sequencing of a Cellobiose phosphotransferase system operon from Bacillus stearothermophilus XL-65-6 and functional expression in Escherichia coli. J. Bacteriol. 175, 6441–6450. doi: 10.1128/jb.175.20.6441-6450.1993
Lai, X., Davis, F. V., Hespell, R. B., and Ingram, L. O. (1997). Cloning of cellobiose phosphoenolpyruvate-dependent phosphotransferase genes: functional expression in recombinant. Appl. Environ. Microbiol. 63, 355–363. doi: 10.1128/aem.63.2.355-363.1997
Lapébie, P., Lombard, V., Drula, E., Terrapon, N., and Henrissat, B. (2019). Bacteroidetes use thousands of enzyme combinations to break down glycans. Nat. Commun. 10:2043. doi: 10.1038/s41467-019-10068-5
Linke, C. M., Woodiga, S. A., Meyers, D. J., Buckwalter, C. M., Salhi, H. E., and King, S. J. (2013). The ABC transporter encoded at the pneumococcal fructooligosaccharide utilization locus determines the ability to utilize long- and short-chain fructooligosaccharides. J. Bacteriol. 195, 1031–1041. doi: 10.1128/JB.01560-12
Lombard, V., Golaconda Ramulu, H., Drula, E., Coutinho, P. M., and Henrissat, B. (2014). The carbohydrate-active enzymes database (CAZy) in 2013. Nucleic Acids Res. 42, 490–495. doi: 10.1093/nar/gkt1178
Luis, A. S., Briggs, J., Zhang, X., Farnell, B., Ndeh, D., Labourel, A., et al. (2018). Dietary pectic glycans are degraded by coordinated enzyme pathways in human colonic Bacteroides. Nat. Microbiol. 3, 210–219. doi: 10.1038/s41564-017-0079-1
Maharjan, R. P., Seeto, S., and Ferenci, T. (2007). Divergence and redundancy of transport and metabolic rate-yield strategies in a single Escherichia coli population. J. Bacteriol. 189, 2350–2358. doi: 10.1128/JB.01414-06
Majd, H., King, M. S., Palmer, S. M., Smith, A. C., Elbourne, L. D. H., Paulsen, I. T., et al. (2018). Screening of candidate substrates and coupling ions of transporters by thermostability shift assays. Elife 7:e38821. doi: 10.7554/eLife.38821
Marchler-Bauer, A., Bo, Y., Han, L., He, J., Lanczycki, C. J., Lu, S., et al. (2017). CDD/SPARCLE: functional classification of proteins via subfamily domain architectures. Nucleic Acids Res. 45, D200–D203. doi: 10.1093/nar/gkw1129
Marques, F. Z., Mackay, C. R., and Kaye, D. M. (2018). Beyond gut feelings: how the gut microbiota regulates blood pressure. Nat. Rev. Cardiol. 15, 20–32. doi: 10.1038/nrcardio.2017.120
Martens, E. C., Lowe, E. C., Chiang, H., Pudlo, N. A., Wu, M., McNulty, N. P., et al. (2011). Recognition and degradation of plant cell wall polysaccharides by two human gut symbionts. PLoS Biol. 9:e1001221. doi: 10.1371/journal.pbio.1001221
McCoy, J. G., Ren, Z., Stanevich, V., Lee, J., Mitra, S., Levin, E. J., et al. (2016). The structure of a sugar transporter of the glucose EIIC superfamily provides insight into the elevator mechanism of membrane transport. Structure 24, 956–964. doi: 10.1016/j.str.2016.04.003
Moreno-Hagelsieb, G. (2015). The power of operon rearrangements for predicting functional associations. Comput. Struct. Biotechnol. J. 13, 402–406. doi: 10.1016/j.csbj.2015.06.002
Ndeh, D., and Gilbert, H. J. (2018). Biochemistry of complex glycan depolymerisation by the human gut microbiota. FEMS Microbiol. Rev. 42, 146–164. doi: 10.1093/femsre/fuy002
Patil, K. R., Roune, L., and McHardy, A. C. (2012). The PhyloPythiaS web server for taxonomic assignment of metagenome sequences. PLoS One 7:e38581. doi: 10.1371/journal.pone.0038581
Rogowski, A., Briggs, J. A., Mortimer, J. C., Tryfona, T., Terrapon, N., Lowe, E. C., et al. (2015). Glycan complexity dictates microbial resource allocation in the large intestine. Nat. Commun. 6:7481. doi: 10.1038/ncomms8481
Saier, M. H. (2000). Families of transmembrane sugar transport proteins. Mol. Microbiol. 35, 699–710. doi: 10.1046/j.1365-2958.2000.01759.x
Saier, M. H. (2015). The bacterial phosphotransferase system: new frontiers 50 years after its discovery. J. Mol. Microbiol. Biotechnol. 25, 73–78. doi: 10.1159/000381215
Saier, M. H., Reddy, V. S., Tsu, B. V., Ahmed, M. S., Li, C., and Moreno-Hagelsieb, G. (2016). The Transporter Classification Database (TCDB): recent advances. Nucleic Acids Res. 44, D372–D379. doi: 10.1093/nar/gkv1103
Schouler, C., Taki, A., Chouikha, I., Moulin-Schouleur, M., and Gilot, P. (2009). A genomic island of an extraintestinal pathogenic Escherichia coli strain enables the metabolism of fructooligosaccharides, which improves intestinal colonization. J. Bacteriol. 91, 388–393. doi: 10.1128/JB.01052-08
Sheridan, P. O., Martin, J. C., Lawley, T. D., Browne, H. P., Harris, H. M. B., Bernalier-Donadille, A., et al. (2016). Polysaccharide utilization loci and nutritional specialization in a dominant group of butyrate-producing human colonic Firmicutes. Microb. Genomics 2:e000043. doi: 10.1099/mgen.0.000043
Steen, A. D., Crits-Christoph, A., Carini, P., DeAngelis, K. M., Fierer, N., Lloyd, K. G., et al. (2019). High proportions of bacteria and archaea across most biomes remain uncultured. ISME J. 13, 3126–3130. doi: 10.1038/s41396-019-0484-y
Tamura, K., Foley, M. H., Gardill, B. R., Dejean, G., Schnizlein, M., Bahr, C. M. E., et al. (2019). Surface glycan-binding proteins are essential for cereal beta-glucan utilization by the human gut symbiont Bacteroides ovatus. Cell. Mol. Life Sci. 76, 4319–4340. doi: 10.1007/s00018-019-03115-3
Tasse, L., Bercovici, J., Pizzut-Serin, S., Robe, P., Tap, J., Klopp, C., et al. (2010). Functional metagenomics to mine the human gut microbiome for dietary fiber catabolic enzymes. Genome Res. 20, 1605–1612. doi: 10.1101/gr.108332.110
Tauzin, A. S., Laville, E., Xiao, Y., Nouaille, S., Le Bourgeois, P., Heux, S., et al. (2016). Functional characterization of a gene locus from an uncultured gut Bacteroides conferring xylo-oligosaccharides utilization to Escherichia coli. Mol. Microbiol. 102, 579–592. doi: 10.1111/mmi.13480
Terrapon, N., Lombard, V., Drula, É, Lapébie, P., Al-Masaudi, S., Gilbert, H. J., et al. (2018). PULDB: the expanded database of Polysaccharide Utilization Loci. Nucleic Acids Res. 46, D677–D683. doi: 10.1093/nar/gkx1022
Theilmann, M. C., Fredslund, F., Svensson, B., Lo Leggio, L., and Abou Hachem, M. (2019). Substrate preference of an ABC importer corresponds to selective growth on β-(1,6)-galactosides in Bifidobacterium animalis subsp. lactis. J. Biol. Chem. 294, 11701–11711. doi: 10.1074/jbc.ra119.008843
Varki, A., Cummings, R. D., Esko, J. D., Freeze, H. H., Stanley, P., et al. (2009). Symbol nomenclature for glycan representation. Proteomics 9, 5398–5399. doi: 10.1002/pmic.200900708
Wang, Z., Tauzin, A. S., Laville, E., Tedesco, P., Létisse, F., Terrapon, N., et al. (2020). Harvesting of prebiotic fructooligosaccharides by nonbeneficial human gut bacteria. mSphere 5:e00771-19. doi: 10.1128/mSphere.00771-19
Keywords: microbiome, oligosaccharides, CAZymes, functional metagenomics, transporters
Citation: Wang Z, Tauzin AS, Laville E and Potocki-Veronese G (2022) Identification of Glycoside Transporters From the Human Gut Microbiome. Front. Microbiol. 13:816462. doi: 10.3389/fmicb.2022.816462
Received: 16 November 2021; Accepted: 14 February 2022;
Published: 25 March 2022.
Edited by:
Rolf Daniel, University of Göttingen, GermanyReviewed by:
Changyi Zhang, University of Illinois at Urbana–Champaign, United StatesLixin Luo, South China University of Technology, China
Copyright © 2022 Wang, Tauzin, Laville and Potocki-Veronese. This is an open-access article distributed under the terms of the Creative Commons Attribution License (CC BY). The use, distribution or reproduction in other forums is permitted, provided the original author(s) and the copyright owner(s) are credited and that the original publication in this journal is cited, in accordance with accepted academic practice. No use, distribution or reproduction is permitted which does not comply with these terms.
*Correspondence: Gabrielle Potocki-Veronese, dmVyb25lc2VAaW5zYS10b3Vsb3VzZS5mcg==
†Present address: Zhi Wang, School of Chemistry Engineering, Zhengzhou University, Zhengzhou, China