- 1Shenzhen Stomatology Hospital (Pingshan), Southern Medical University, Shenzhen, China
- 2Department of Oral and Maxillofacial Surgery, The First Affiliated Hospital of Zhengzhou University, Zhengzhou, China
- 3Department of Stomatology, The First Affiliated Hospital of Xiamen University, Xiamen, China
- 4Microbiome Medicine Center, Department of Laboratory Medicine, Zhujiang Hospital, Southern Medical University, Guangzhou, China
- 5State Key Laboratory of Organ Failure Research, Southern Medical University, Guangzhou, China
Periodontitis is a chronic inflammatory oral disease that affects nearly 50% of all adults. Fusobacterium nucleatum (F. nucleatum) is known to be involved in the formation and development of periodontitis. Outer membrane vesicles (OMVs) harboring toxic bacterial components are continuously released during F. nucleatum growth and regulate the extent of the inflammatory response by controlling the functions of immune and non-immune cells in tissues. Macrophages are important immune cells in periodontal tissue that resist pathogen invasion and play an important role in the pathophysiological process of periodontitis. However, the role of the interaction between F. nucleatum OMVs and macrophages in the occurrence and development of periodontitis has not been studied. The purpose of this study was to clarify the effect of F. nucleatum OMVs on the polarization of macrophages and the roles of this specific polarization and F. nucleatum OMVs in the pathophysiology of periodontitis. The periodontitis model was established by inducing ligation in C57BL/6 mice as previously described. Micro-CT, RT-qPCR, hematoxylin-eosin (H&E) and tartrate acid phosphatase (TRAP) staining assays were performed to analyze the periodontal tissue, alveolar bone loss, number of osteoclasts and expression of inflammatory factors in gingival tissue. The changes in the state and cytokine secretion of bone marrow-derived macrophages (BMDMs) stimulated by F. nucleatum OMVs were observed in vivo by confocal microscopy, flow cytometry, Western blot and ELISA. Mouse gingival fibroblasts (MGFs) were isolated and then cocultured with macrophages. The effects of F. nucleatum OMVs on the proliferation and apoptosis of MGFs were analyzed by flow cytometry and lactate dehydrogenase (LDH) assays. The periodontitis symptoms of mice in the F. nucleatum OMVs + ligation group were more serious than those of mice in the simple ligation group, with more osteoclasts and more inflammatory factors (IL-1β, IL-6, and TNF-α) being observed in their gingival tissues. M0 macrophages transformed into M1 macrophages after the stimulation of BMDMs with F. nucleatum OMVs, and the M1 macrophages then released more inflammatory cytokines. Analysis of the coculture model showed that the MGF apoptosis and LDH release in the inflammatory environment were increased by F. nucleatum OMV treatment. In conclusion, F. nucleatum OMVs were shown to aggravate periodontitis, alveolar bone loss and the number of osteoclasts in an animal model of periodontitis. F. nucleatum OMVs promoted the polarization of macrophages toward the proinflammatory M1 phenotype, and the inflammatory environment further aggravated the toxicity of F. nucleatum OMVs on MGFs. These results suggest that M1 macrophages and F. nucleatum OMVs play roles in the occurrence and development of periodontitis.
Introduction
Periodontitis is a periodontal inflammatory disease caused by bacteria, and numerous pathogenic microorganisms destroy the immune defense system in periodontal tissue (Kaan et al., 2021; Szafranski et al., 2021; Wade, 2021). Proinflammatory cytokines produced by immune cells can amplify the inflammatory cascade in surrounding tissues, eventually leading to tissue destruction and tooth loss. At present, intervention measures for periodontitis treatment can only alleviate the intensity and progression of the disease and cannot completely cure it (Myneni et al., 2020; Sczepanik et al., 2020; Feres et al., 2021). Due to the complexity of the disease, the pathophysiological mechanism of periodontitis is not clear, and studying its pathogenesis is therefore necessary to elucidate new economic and effective treatments.
Fusobacterium nucleatum, a common opportunistic pathogen in oral microorganisms, is known to play an important role in the occurrence and development of periodontitis (Brennan and Garrett, 2019). This bacterium participates in plaque biofilm formation, bacterial colonization and mixed infections and can activate a variety of signaling pathways and regulate the biological functions of cells by binding to specific adhesins on corresponding cell ligands. F. nucleatum can also invade the oral cavity, colon, placental epithelial cells, immune cells, and keratinocytes, among other regions and tissue types. Internalized bacteria affect the synthesis and secretion of some cytokines, regulate biological behaviors such as cell proliferation and apoptosis, and lead to cell dysfunction.
Outer membrane vesicles (OMVs), which are considered to be ubiquitous across all areas of life, are produced by foaming of the bacterial membrane and can include all internal bacterial cell components (nucleic acids, proteins, lipids, and sugars) (Boopathi et al., 2021; Li C. et al., 2021). F. nucleatum OMVs are double-layered spherical membrane vesicles that are continuously secreted from the cell surface. Due to the protection provided by the vesicle membrane structure, pathogenic factors at high concentrations can prevent degradation, enter the host cytoplasm through endocytosis, transfer toxic components to host cells, and activate potential proinflammatory or necrotic pathways (Briaud and Carroll, 2020; Engevik, 2020). Therefore, F. nucleatum OMVs may be more toxic than the mother bacterium itself.
Macrophages play an important role in the immune response to periodontitis by controlling the pathogenicity of periodontal biofilms and activating the adaptive immune response (Almubarak et al., 2020; Galli and Saleh, 2020; Wang et al., 2021). As we all know, macrophages in a ground state of M0 can be driven to either M1 or M2 states of activation following exposure to external stimulation (Russell et al., 2019). M1 macrophages produce proinflammatory cytokines and osteoclasts, which aggravate the symptoms of periodontitis. M2 macrophages produce anti-inflammatory cytokines to combat periodontitis (Wu et al., 2020; Zhang B. et al., 2021). However, the effects of F. nucleatum extracellular vesicles on macrophage polarization in periodontal tissue and their roles in the development of periodontitis have not been reported.
Herein, we aimed to study the interaction between F. nucleatum OMVs and macrophages in subjects with periodontitis. In the ligation-induced periodontitis mouse model, the disease status, inflammation and alveolar bone loss caused by F. nucleatum OMVs were analyzed. The phenotypic changes and secreted cytokines of macrophages treated with F. nucleatum OMVs in vitro were analyzed. In the coculture model, the effects of F. nucleatum OMVs on the biological function of MGFs were observed.
Results
Characteristics of Fusobacterium nucleatum Outer Membrane Vesicles
Outer membrane vesicles were first isolated from F. nucleatum liquid medium by gradient centrifugation (Figure 1A), and their morphology was observed by transmission electron microscopy (TEM) (Figure 1B). Nanoparticle tracking analysis (NTA) showed that the OMVs ranged in size from 62–310 nm, with a peak at 131 ± 25 nm (Figure 1C). SDS-PAGE revealed that the F. nucleatum OMVs had a molecular weight of approximately 40 kD (Supplementary Figure 1A). Mass spectrometry identified the main component as a major outer membrane protein (OMP) that plays roles in (1) immune regulation and (2) adhesion and (3) serves as a channel protein (Supplementary Figure 1B). The OMVs of Escherichia coli (E. coli), Streptococcus salivarius (S. salivarius) K12 and Lactobacillus rhamnosus (L. rhamnosus) GG were also extracted and subsequently used as experimental controls (Figure 1D).
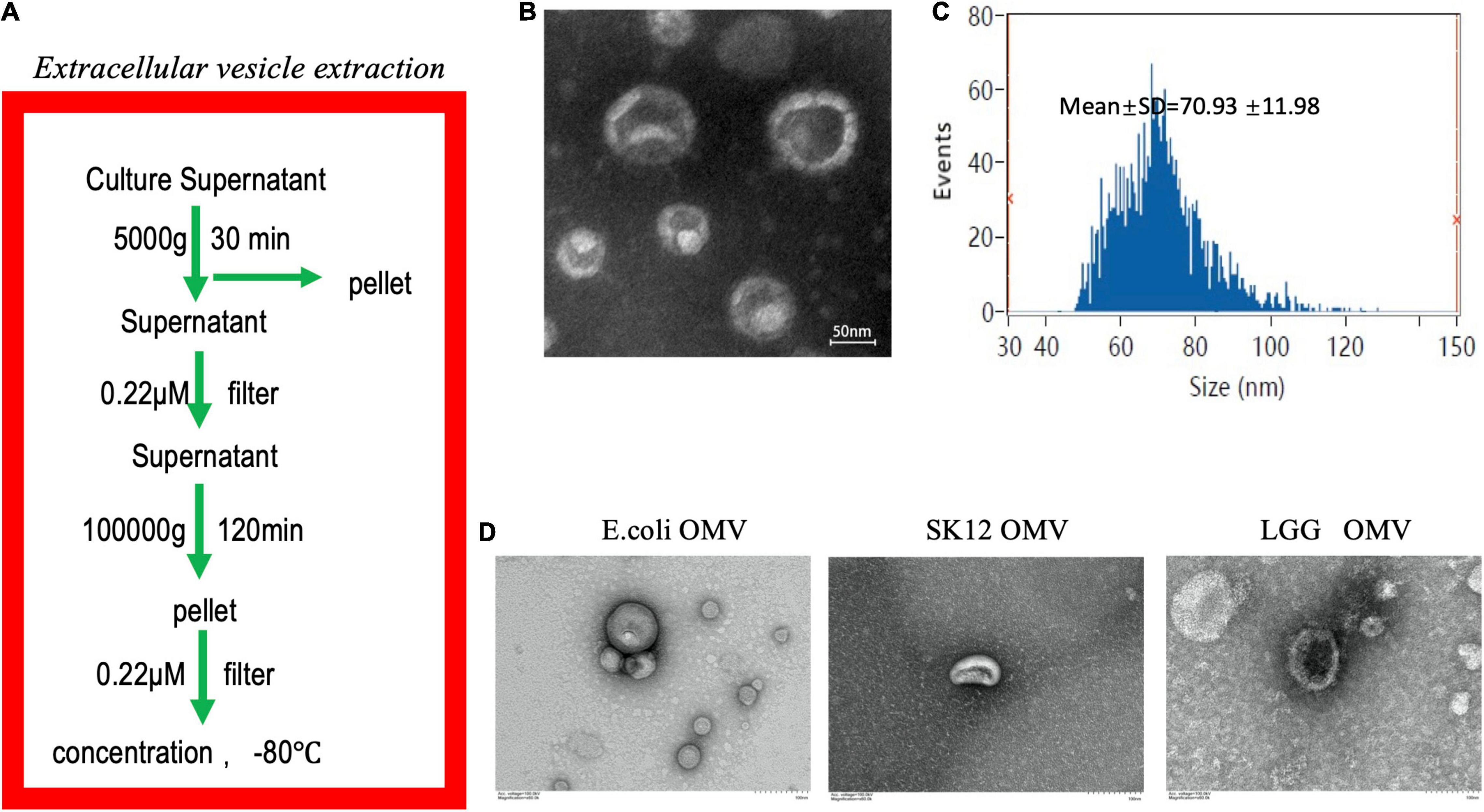
Figure 1. Extraction and identification of F. nucleatum OMVs. (A) Extraction of F. nucleatum OMVs by ultracentrifugation followed these steps. (B) F. nucleatum OMVs were observed by TEM and exhibited a similar tray structure. (C) NTA showed that the OMVs ranged in size from 62–310 nm, with a peak at 131 ± 25 nm. (D) The OMVs of E. coli, S. salivarius K12 and L. rhamnosus GG were extracted via a similar method and showed different morphologies.
Fusobacterium nucleatum Outer Membrane Vesicles Induce Significant Proinflammatory Profiles and Oxidative Stress in Macrophages
Macrophages play an important role in innate immunity. To study the role of F. nucleatum OMVs in inducing the production and secretion of cytokines in macrophages, bone marrow cells were isolated from healthy C57BL/6 mice and then induced to differentiate into BMDMs. The BMDMs were stimulated with F. nucleatum OMVs (1.0 μg/ml) for 24 h and were morphologically similar to M1 macrophages (Figure 2A). We next detected the cytokines TNF-α and inducible nitric oxide synthase (iNOS) as well as the phosphorylation of p65 in macrophages stimulated with F. nucleatum OMVs (Figure 2B). The mRNA level of iNOS and TNF-α was increased significantly after F. nucleatum OMVs or E. coli OMVs stimulation (Figure 2C). Arg-1 and CD163 are all M2 macrophages biomarkers. The expression of protein and mRNA of Arg-1 and CD163 did not change (Supplementary Figures 2A,B). However, the protein expression of CD86 increased significantly. Enzyme-linked immunosorbent assay (ELISA) analysis of M1 marker (TNF-α) and M2 marker (IL-10) in cultured supernatant of macrophages treated with OMVs for 24 h showed that TNF-α release increased significantly (Supplementary Figure 2C).
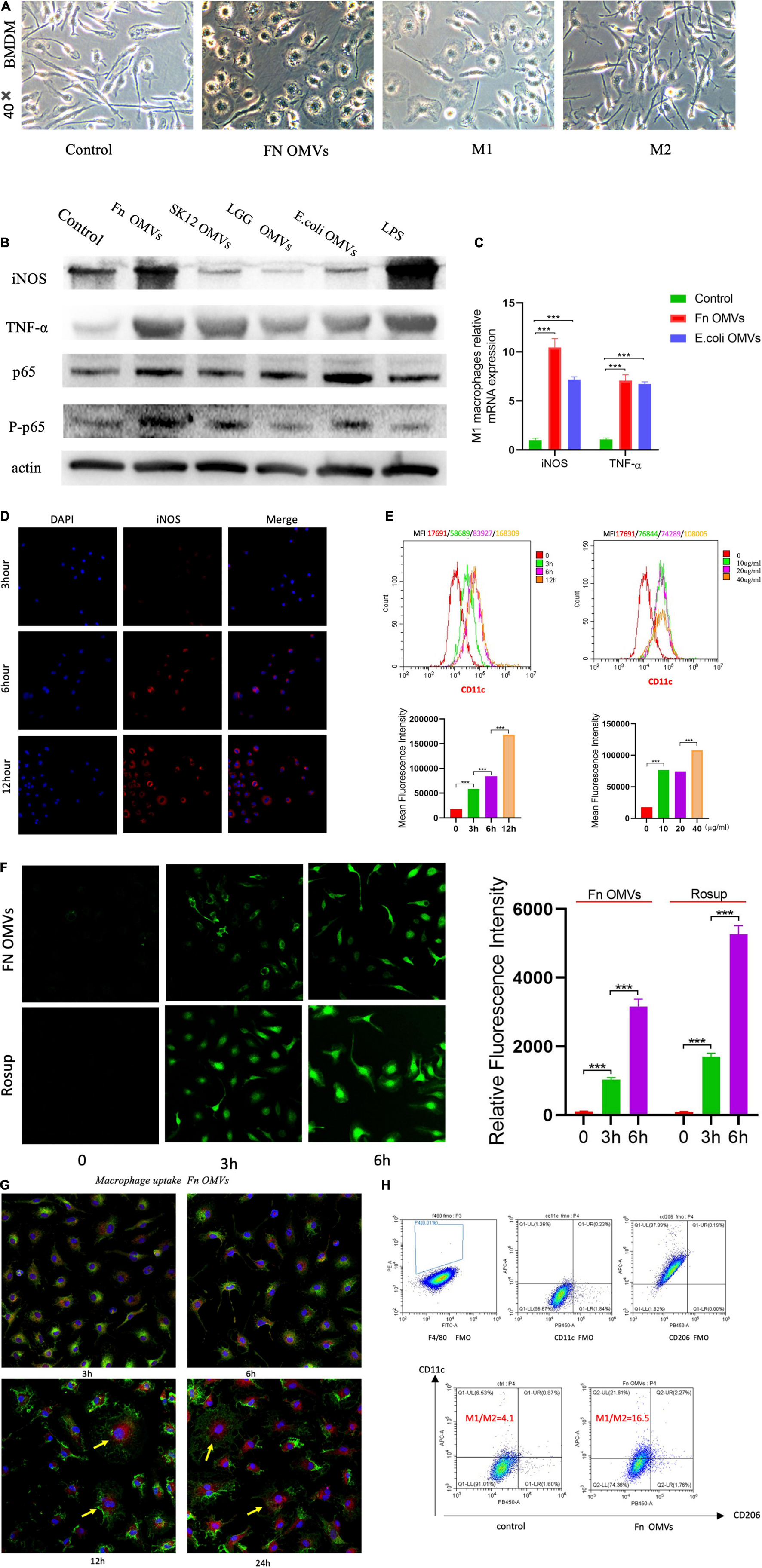
Figure 2. Fusobacterium nucleatum OMVs induce significant proinflammatory profiles and oxidative stress in macrophages. (A) BMDMs were stimulated separately with F. nucleatum OMVs (1.0 μg/ml), M1 macrophages (100 ng/ml) together with IFN-γ (50 ng/ml) or M2 macrophages (10 ng/ml) together with IL-13 (10 ng/ml) for 24 h. BMDMs were stimulated with F. nucleatum OMVs for 24 h and were morphologically similar to M1 macrophages. (B) F. nucleatum OMVs upregulated the expression of the cytokines TNF-α and iNOS, M1 macrophage markers. The expression of P-p65 was increased in macrophages, indicating that the OMVs activated the NF-κB pathway. (C) The mRNA level of TNF-α and iNOS increased significantly after OMVs stimulation for 24 h. (D) The fluorescence intensity of the M1 biomarkers iNOS were brightest in BMDMs stimulated with F. nucleatum OMVs (10 μg/ml) for 12 h. (E) Flow cytometry results showed that the expression of CD11c was most expression at F. nucleatum OMVs stimulation for 12 h. Furthermore, after more OMVs stimulated BMDM, CD11c expression increased. (F) The ROS levels were increased in macrophages stimulated with F. nucleatum OMVs for 3 h. ROS staining was brighter at 6 h than at 3 h. (G) Macrophages exhibited an morphology after being stimulated for 12 h. F. nucleatum OMVs were phagocytosed into cells and appears to surround cell nucleus. (yellow: cell nuclei; red: OMVs; green: membrane). (H) After the stimulation of RAW264.7 cells with F. nucleatum OMVs for 24 h, flow cytometry analysis revealed that the M1/M2 cell ratio was increased by fourfold compared with that in the control group. *P < 0.05, **P < 0.01, ***P < 0.001. FMO controls are as followed: F4/80 FMO control cells stained with FITC, BV 421, and APC conjugated antibodies. CD206 FMO control cells stained with FITC, PE, and APC conjugated antibodies. CD11c FMO control cells stained with FITC, PE, and BV 421 conjugated antibodies.
Immunofluorescence analysis showed that the expression of iNOS increased in macrophages as the F. nucleatum OMVs stimulation time was extended (Figure 2D). The expression of CD11c was time-dependent and dose-dependent (Figure 2E), suggesting that the F. nucleatum OMVs induced the polarization of M0-like macrophages toward the proinflammatory M1-like phenotype. Considering the involvement of reactive oxygen species (ROS) in macrophage polarization, we estimated the ROS levels at different time points of macrophage activation, revealing that the level increased as the stimulation period extended (Figure 2F). At 12 h after the phagocytosis of F. nucleatum OMVs, the macrophage morphology was altered (Figure 2G), and the cells released more cytokines (Supplementary Figure 2D). Flow cytometry analysis of RAW264.7 cells stimulated with F. nucleatum OMVs for 24 h showed that the M1/M2 cell ratio was increased by fourfold (Figure 2H). Overall, these results suggest that F. nucleatum OMVs promote macrophage polarization toward a proinflammatory phenotype and increase cytokine release and oxidative damage.
Fusobacterium nucleatum Outer Membrane Vesicles and M1 Macrophages Induce Mouse Gingival Fibroblasts Death in the Proinflammatory Microenvironment
To simulate the effect of F. nucleatum OMVs on macrophages and GFs in periodontal tissue, MGFs were cultured (Figure 3A), and vimentin (Figure 3B) and FSP-1 (Supplementary Figure 3) was shown to be expressed at high levels in the cells. As revealed by confocal microscopy, macrophages engulfed numerous F. nucleatum OMVs within 12 h, while almost no F. nucleatum OMVs were detected in MGFs within 24 h, and the adhesion between F. nucleatum OMVs and the MGF cell membrane was not firm (Figure 3C). Therefore, we established a macrophage/MGF coculture model in a Transwell chamber to simulate the effects of inflammatory factors released by macrophages and F. nucleatum OMVs on MGFs (Figure 3D). After 24 h of treatment, flow cytometry showed that F. nucleatum OMVs alone were sufficient to affect the apoptosis of MGF cells. F. nucleatum OMVs and M1 macrophage treatment induced more severe apoptosis than that observed in the control group (Figure 3E). The level of LDH in culture medium is an indirect indicator of cell damage, and F. nucleatum OMVs were shown to stimulate the secretion of LDH from mouse MGFs. The combined use of F. nucleatum OMVs and M1 macrophages further exacerbated the release of LDH from MGFs (Figure 3F), indicating that the inflammatory environment further aggravates the toxicity of F. nucleatum OMVs on MGFs.
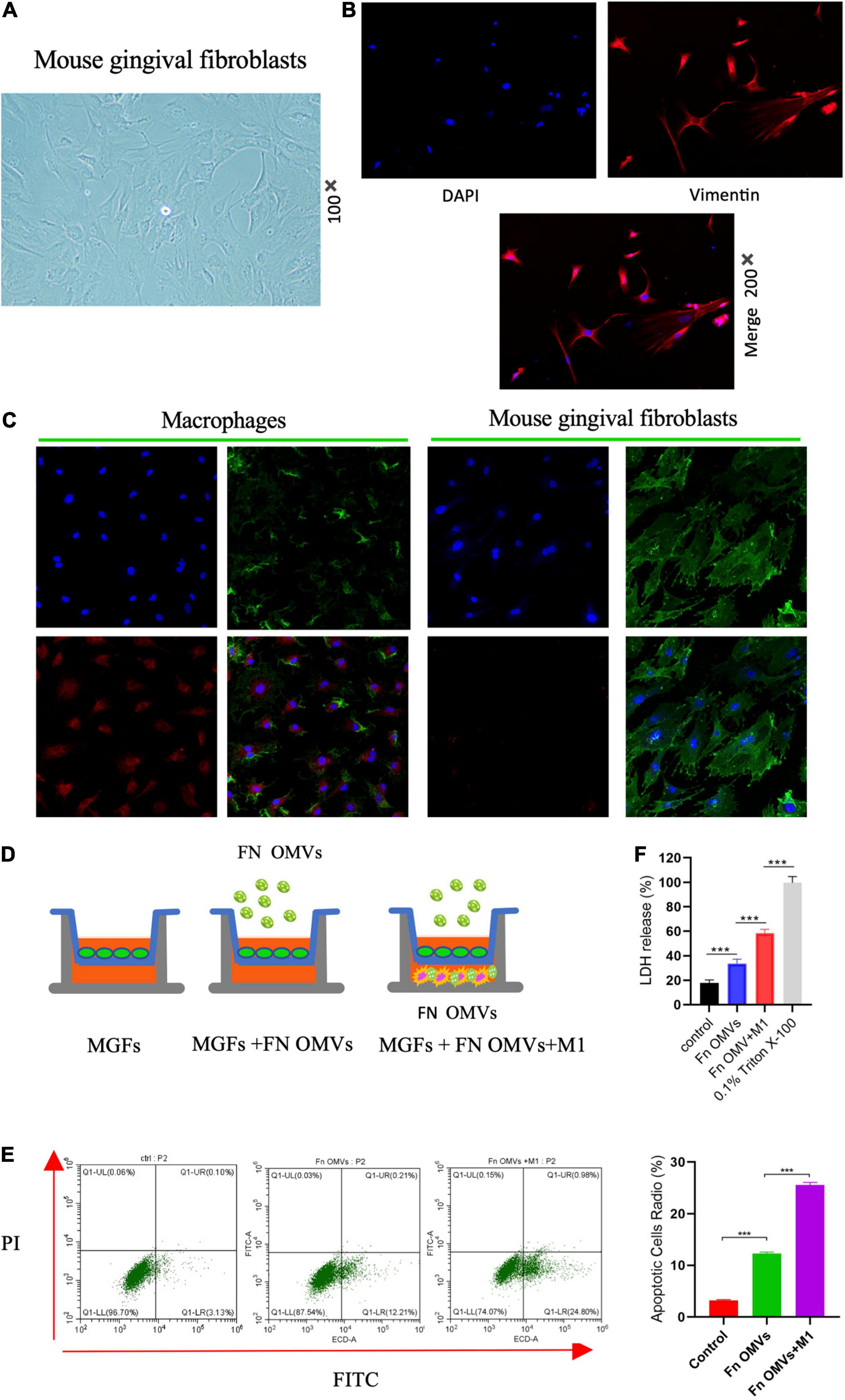
Figure 3. Fusobacterium nucleatum OMVs and M1 macrophages induce MGF death in the proinflammatory microenvironment. (A) MGFs were cultured, and they exhibited a slender, fibrous morphology (100×). (B) Vimentin was expressed at high levels in the cytoplasm. (C) Confocal microscopy showed that macrophages engulfed numerous F. nucleatum OMVs within 12 h, while almost no F. nucleatum OMVs were observed in MGFs within 24 h.(Blue: cell nucleus; Green: cell membrane; Red, Fn OMVs). (D) The macrophage/MGF coculture model simulated the interaction between macrophages and F. nucleatum OMVs in vivo. (E) Flow cytometry showed that F. nucleatum OMVs alone increased the apoptosis of MGFs after 24 h of treatment. F. nucleatum OMVs and M1 macrophage treatment induced more severe apoptosis than that observed in the control group. (F) F. nucleatum OMVs stimulated the secretion of LDH by MGFs. F. nucleatum OMVs and M1 macrophages in combination further exacerbated the release of LDH in MGFs. 0.1% Triton X-100 was used as positive control. *P < 0.05, **P < 0.01, ***P < 0.001.
Fusobacterium nucleatum Outer Membrane Vesicles Aggravate Periodontitis in a Mouse Model
To observe the effects of F. nucleatum OMVs in vivo, a mouse model of periodontitis was constructed, and the mice were divided into four experimental groups: a non-ligation group, ligation + PBS group, ligation + F. nucleatum OMV group and ligation + F. nucleatum group (Figure 4A). Compared with those of mice in the simple ligation group, the maxilla of F. nucleatum OMV-treated mice showed more extensive alveolar bone loss and a twofold reduction in alveolar bone density as determined by micro-CT analysis (Figure 4B). At week 9, the cement-enamel junction-alveolar bone crest (CEJ-ABC) distance in the F. nucleatum OMV treatment group was greater than that in the simple ligation group. The CEJ-ABC distance in mice treated with F. nucleatum OMVs was 1.3-fold higher than that in mice of the simple ligation group (Figure 4C). H&E staining showed that the degree of alveolar bone loss in the periodontal tissues of mice treated with F. nucleatum OMVs was more extensive than that in the tissues of mice from the simple ligation group. The periodontal tissue and alveolar bone were intact in the non-ligation group (Figure 4D). The results of the histological analyses of periodontal tissue destruction and alveolar bone loss support the results of the micro-CT analysis. Alveolar bone loss is induced by the osteoclast activity being increased in subjects with periodontitis, and the TRAP staining of tissue sections revealed a significant increase in the number of osteoclasts in the F. nucleatum OMV treatment group (Figure 4E). Quantitative analysis of osteoclasts in the tissue sections revealed that the number of osteoclasts in F. nucleatum OMV-treated mice was more than twofold higher than that in control mice (Figure 4F). The expression of inflammatory factors (IL-1β, IL-6, and TNF-α) in the corresponding gingival tissues differed between the F. nucleatum OMV and PBS treatment groups (Figure 4G). In conclusion, the in vivo experiments revealed that the periodontitis in F. nucleatum OMVs + ligation group mice was more severe than that of mice in the PBS + ligation group and that F. nucleatum OMVs promoted the occurrence and development of periodontitis.
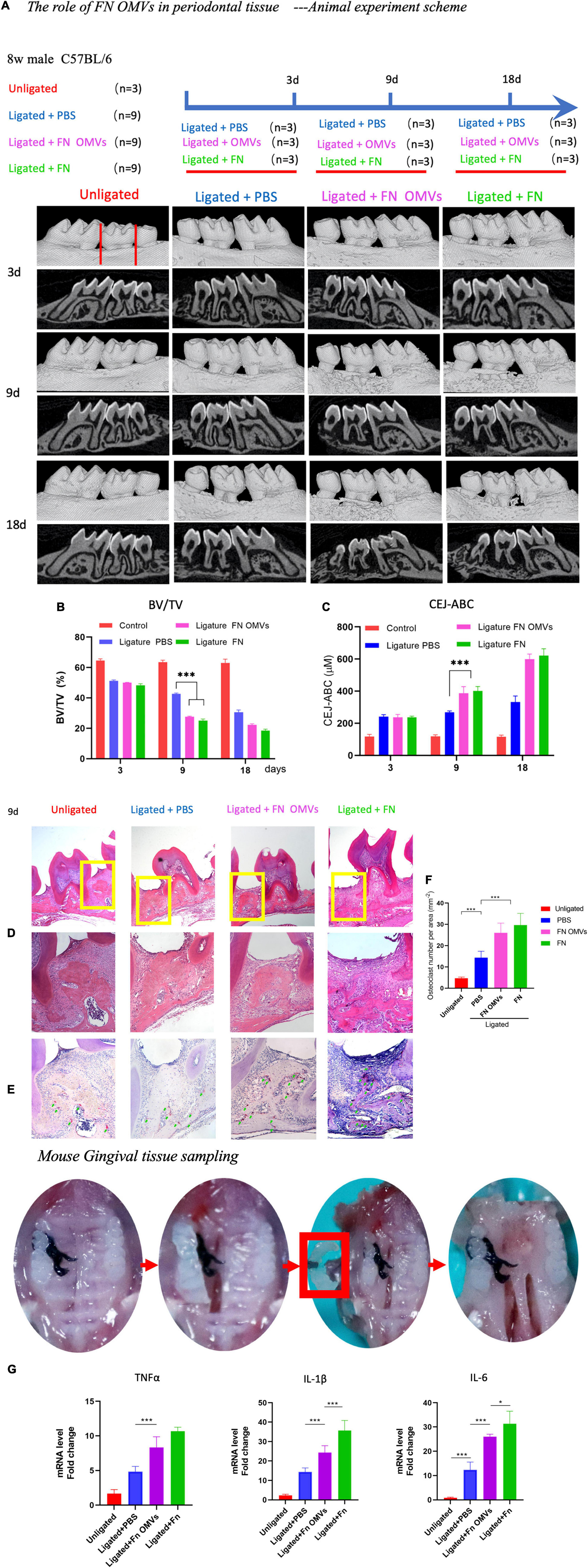
Figure 4. Fusobacterium nucleatum OMVs aggravate periodontitis and alveolar bone loss in a mouse model. (A) The periodontitis mouse model was constructed, and the mice were divided into four experimental groups: a non-ligation group, ligation + PBS group, ligation + F. nucleatum OMV group and ligation + F. nucleatum group. The detailed scheme is shown in the section “Materials and Methods”. (B,C) Micro-CT images of the mouse maxillas showed more extensive alveolar bone loss and reductions in alveolar bone density. Quantitative analysis of the BV/TV (B) and CEJ-ABC distances (C) as determined by micro-CT. (D–F) Histological images of H&E and TRAP staining. The extent of alveolar bone loss in periodontal tissue (D) and quantitative analysis of osteoclasts (E,F) are shown. (G) Expression of inflammatory factors (IL-1β, IL-6, and TNF-α) in corresponding mouse gingival tissues. The data are presented as the mean ± SD. Significant differences between the groups, *P < 0.05, **P < 0.01, ***P < 0.001. CEJ, cement-enamel junction; ABC, alveolar bone crest; BV/TV, bone volume/total volume.
Discussion
As a periodontal pathogen, F. nucleatum plays an indispensable role in the formation and growth of oral biofilms, affects the occurrence and development of periodontitis and is the main microorganism of biofilms in periodontal pockets (Thurnheer et al., 2019; Curtis et al., 2020). Advances in research have revealed an increasing number of virulence factors (Ellis and Kuehn, 2010; Zanzoni et al., 2017), and known virulence factors, new functions and mechanisms are continuously being reported (Li D. H. et al., 2021; Zhang Z. et al., 2021). In addition to its significant “bridging” characteristics, the adhesin of F. nucleatum can bind to non-immune and immune cells, leading to gingival inflammation that subsequently develops into an irreversible periodontal disease. FadA adhesin binds to E-cadherin expressed by epithelial and endothelial cells, and β-catenin then enters the nucleus, activates Wnt signaling pathways and promotes cell proliferation (Rubinstein et al., 2013, 2019). The binding of Fap2 to the gal/galNAc polysaccharide on the cell surface is conducive to the bacterial internalization of non-phagocytic cells (Parhi et al., 2020). In addition to Fap2, the non-specific porin FomA is one of the most highly expressed OMPs, accounting for approximately 30% of such proteins (Nakagaki et al., 2010). In addition, metabolites produced by F. nucleatum, such as fatty acids, hydrogen sulfide and endotoxins, enhance the immune response of the host (Tateishi et al., 2012; Kezuka et al., 2018; Dahlstrand Rudin et al., 2021). F. nucleatum can also invade and reside in epithelial cells of the human gastrointestinal tract and promote intestinal inflammation (Richardson et al., 2020). In general, the molecular mechanisms by which F. nucleatum interacts with host cells and regulates innate immunity are not well understood.
During the process of infection, the OMVs released by bacteria can bypass the contact between cells so that key substances, such as active compounds and proteins, can pass through and play an important role in intercellular communication. Recent studies have linked OMVs with immune and disease processes. Studies have shown that almost all gram-negative bacteria can activate target cells by secreting OMVs and transferring toxic components (Deo et al., 2020). Depending on the type of target cells, bacterial species and number of OMVs, the bacterial-host interactions mediated by OMVs can cause non-immunogenic, inflammatory and/or cytotoxic reactions. OMVs can function independently of bacteria, mediate intestinal epithelial reactions and inflammation, and play a role in the pathogenesis of inflammatory bowel disease (IBD) (Durant et al., 2020; Tulkens et al., 2020). F. nucleatum secretes OVMs carrying a variety of harmful molecules, which can alter the interactions between microorganisms and the host (Liu J. et al., 2019). F. nucleatum OMV stimulation was previously shown to effectively recruit intestinal macrophages, which were differentiated into a proinflammatory phenotype, significantly increase the apoptosis of epithelial cells and cause intestinal barrier dysfunction (Liu et al., 2021). However, whether F. nucleatum OMVs also have this function in periodontal tissue has not been reported.
In this study, we first obtained F. nucleatum OMVs by ultrafiltration and then determined that they contained pathogenic factors such as F. nucleatum autotransporter 2 (Fap2), adhesin a (FadA), and F. nucleatum OMP A (FomA) by mass spectrometry analysis (Supplementary Excel file). These pathogenic factors can destroy the biofilm structure and the acid-resistant environment around the teeth, resulting in the occurrence and development of periodontitis (Meng et al., 2021).
Macrophages are key effector cells that protect against heterologous microorganism invasion, mainly killing bacteria through phagocytosis (Locati et al., 2020). Effector cells have phagocytes containing enzymes that can produce ROS and reactive nitrogen species (RNS), and bacteria are phagocytized by macrophages to form phagosomes. They contract in phagosomes and are killed by ROS. Macrophages produce chemokines and inflammatory factors that recruit and activate other immune cells to the site of infection. At the same time, blood monocytes move to the infection site and differentiate into inflammatory macrophages during the anti-infection process (Russell et al., 2019), thus playing a role in defense against bacterial infection.
The effect of F. nucleatum OMVs on macrophage polarization is controversial at present. Some researchers believe that they promote macrophage polarization toward the M1 phenotype, while others report that F. nucleatum OMVs promote M2 type polarization (Chen et al., 2018; Liu L. et al., 2019; Martin-Gallausiaux et al., 2020; Hu et al., 2021; Liu et al., 2021). We extracted mouse bone marrow mesenchymal cells and induced them to differentiate into macrophages. The cell morphology was altered and the expression of M1 macrophage markers was increased after stimulation by F. nucleatum OMVs. Flow cytometry revealed that the expression of CD11c on the macrophage surface was time- and dose-dependent, and ELISA showed that the expression levels of the inflammatory factors IL-1β, IL-6, and TNF-α were increased. Recent studies reported that F. nucleatum is a facultative intracellular bacterium that can survive and limit proliferation in macrophages. Live F. nucleatum infection can inhibit macrophage apoptosis by activating the PI3K and ERK pathways (Xue et al., 2018; Guo et al., 2020). In conclusion, these data suggest that F. nucleatum OMVs promote the transformation of macrophages toward the proinflammatory phenotype and increase the release of corresponding cytokines.
Gingival fibroblasts, the main cell type of periodontal connective tissue, have no tolerance for bacterial stimulation and can continuously respond to exogenous stimulation. The pathogenic effect of F. nucleatum on GFs and the possible mechanism have not been fully clarified (Ebersole et al., 2020). F. nucleatum can inhibit the proliferation of GFs by regulating the Akt/MAPK pathway. F. nucleatum also activates the NF-κB signaling pathway, promotes the production of ROS and the secretion of inflammatory cytokines, and then promotes the apoptosis of GFs (Kang et al., 2019b). Of course, these studies assessed the direct interaction between F. nucleatum OMVs and GFs. When F. nucleatum OMVs enter periodontal tissue in vivo, immune cells first play an immune defense role; for example, macrophages phagocytize OMVs to resist GF damage. After the treatment of MGFs with F. nucleatum OMVs for 24 h, no adhesion or invasion was observed between the OMVs and MGFs. After 12 h, numerous OMVs were phagocytosed by the macrophages, which exhibited an altered cellular morphology. Therefore, we established a macrophage/GF coculture model and found that F. nucleatum OMVs increased the GF necrosis in the inflammatory microenvironment. F. nucleatum OMVs are thought to promote the M1 polarization of macrophages and the release of cytokines to induce the formation of an inflammatory microenvironment in the surrounding tissues, which increases the extent of MGF damage induced by F. nucleatum OMVs.
Limitations of This Study and Follow-Up Research Directions
1. In this study, the effect of F. nucleatum OMVs on mouse periodontal tissue was observed and compared with those of PBS and F. nucleatum. Previous animal experiments suggested that F. nucleatum and P. gingivalis in combination promote periodontitis. However, our in vivo experimental results show that only F. nucleatum OMVs or F. nucleatum cause periodontitis, and Meng et al. (2021) reported similar results. They found that F. nucleatum produced amyloid-like FadA as a scaffold for biofilm formation, conferred cells with acid tolerance, and mediated its binding to host cells. Amyloid-like FadA was shown to induce periodontal bone loss and promote colorectal cancer (CRC) progression in mice (Meng et al., 2021). Other research teams also found that F. nucleatum could inhibit cell proliferation, promote cell apoptosis, and elevate pro-inflammatory cytokine production of osteoblasts or Gingiva-Derived Mesenchymal Stem Cells (Kang et al., 2019a; Gao et al., 2020). More experiments are needed to support this conclusion in the future.
2. Further verification in vivo is needed. For example, by knocking out the expression of FadA in F. nucleatum, scientists might determine whether F. nucleatum or F. nucleatum OMVs can still promote the development of periodontitis. The pathogenic factors of F. nucleatum must be identified before clinical transformation can occur.
Materials and Methods
Bacteria and Cell Culture
Fusobacterium nucleatum ATCC 25586 was grown anaerobically (10% CO2, 10% H2, and 80% N2) on brain heart infusion (BHI) agar plates supplemented with 5% defibrinated sheep blood, 5 μg/mL hemin, 1 μg/mL vitamin K1, and 0.5% yeast extract. The bacteria on the agar plate were scraped into BHI liquid medium and then grown to the logarithmic growth phase prior to being used in experiments. L. rhamnosus and S. salivarius were purchased from BeNa Culture Collection (Beijing, China), and E. coli was provided by the Microbiome Medicine Center of Zhejiang Hospital.
Mouse gingival fibroblasts were purchased from Procell Life Science&Technology Co., Ltd. (Wu Han, China) and cultured in α-MEM (Thermo Fisher Scientific, Waltham, MA, United States) supplemented with 10% fetal bovine serum (Thermo Fisher Scientific, Waltham, MA, United States), 100 U/ml penicillin and 100 μg/ml streptomycin in an incubator with 5% CO2 at 37°C. When the cells reached 90% confluence, they were digested with 0.25% trypsin and subcultured. Early MGFs were used before the fifth generation, and MGFs were extracted as described previously (Mizraji et al., 2013).
The mouse macrophage cell line RAW264.7 (ATCC TIB-71) was cultured in Dulbecco’s modified Eagle’s medium (DMEM, Thermo Fisher Scientific, Waltham, MA, United States) supplemented with 10% fetal bovine serum (Thermo Fisher Scientific, Waltham, MA, United States), 1% penicillin and streptomycin (Life Technologies, Waltham, MA, United States), and 1% L-glutamine.
Preparation of Outer Membrane Vesicles From Fusobacterium nucleatum, Lactobacillus rhamnosus, Streptococcus salivarius, and Escherichia coli
Briefly, after pelleting the bacterial cultures (5,000 g for 30 min), the obtained supernatants were filtered (0.22 μm) to remove parental bacterial debris and other contaminants. The supernatant was ultracentrifuged at 4°C for 120 min at 100,000 g and washed with PBS twice to obtain the crude OMVs. The purified OMVs were refiltered (0.22 μm) and then subjected to sucrose density gradient ultracentrifugation in a 45 Ti rotor at 100,000 g for 2 h at 4°C. The final pellets were resuspended in PBS and stored at -80°C, and the concentration was determined by a BCA protein analysis kit (KeyGEN BioTECH Co., Nanjing, China).
Identification and Observation of Outer Membrane Vesicles
The extracted OMVs were collected in tubes, and their diameters and particle numbers were measured by NTA (Particle Metrix Co., Germany). In addition, the OMVs were fixed in 2.5% glutaraldehyde, dehydrated in a series of acetone and embedded. The samples were sliced into thin sections and morphological observed on a transmission electron microscope (HT7700, Hitachi, Japan).
Isolation of Bone Marrow-Derived Macrophages
Bone marrow cells were collected from the femurs and tibias of C57BL/6 wild-type mice (6 weeks old) and cultured as previously described (Ying et al., 2013). Briefly, the cells were differentiated into BMDMs in DMEM (Thermo Fisher Scientific, Waltham, MA, United States) supplemented with 10% fetal bovine serum (Thermo Fisher Scientific, Waltham, MA, United States) and antibiotics in the presence of 25 μg/ml macrophage colony-stimulating factor (M-CSF; R&D Systems, Minneapolis, MN, United States) for 7 days in a 37°C incubator.
Flow Cytometry
The expression of CD11b, CD206, CD11c, and F4/80 in BMSC-derived macrophages were analyzed using the FACS Calibur (Becton Dickinson, United States) and the CellQuest software (Becton Dickinson). The adherent cells were washed with PBS and collected using Accutase (Nacalai Tesque, Kyoto, Japan) and resuspended in 50 μL staining buffer (BD Pharmingen, Franklin Lakes, NJ, United States). The harvested cells were blocked with 2 μL Human Trustain FcX (Fc receptor Blocking Solution; BioLegend) for 10 min at room temperature, and stained with 2 μL antibodies in the dark for 30 min at 4°C. FITC anti- mouse CD11b, FITC anti-human CD11b, Alexa Fluor®488 anti-human CD11b, BV421 anti-mouse CD206, PE anti-mouse F4/80 and APC anti-mouse CD11c (BioLegend).
Enzyme-Linked Immunosorbent Assay
The ELISA was performed to measure the expression levels of proteins in periodontal tissue extracts and conditioned culture medium of mouse macrophages. The periodontal tissue cell lysate was prepared as described previously (Marchesan et al., 2018). The concentrations of the proinflammatory cytokines TNF-α, IL-6, and IL-1β in periodontal tissue extracts and cell culture supernatants were measured using a Mouse ELISA Standard Kit (Cusabio, Wuhan, China) according to the manufacturer’s protocol.
Measurement of Reactive Oxygen Species
Reactive oxygen species levels were detected by a DCFH-DA probe (Beyotime, Shanghai, China). After incubation with a ROS staining solution at 37°C, macrophages were washed three times with PBS, and H2O2 served as the reference. Fluorescence was detected by a fluorescence microplate reader (Olympus, Tokyo, Japan).
Lactate Dehydrogenase Release Assay
The LDH levels of MGFs were measured following 24 h of co-culture using LDH activity kits (cat. no. A020-2-2; Jiancheng, Nanjing, China) according to the manufacturer’s protocol. The absorbance of each well was measured at 450 nm to determine the LDH activity.
Development of the Ligation-Induced Periodontitis Mouse Model
A total of 30 wild-type male C57BL/6 mice (6 weeks old, male, 20 g body weight) were used in this study. Male C57BL/6 mice were obtained from Guangdong Medical Laboratory Animal Center and bred at the Experimental Animal Research Center of Southern Medical University. The Experimental Animal Ethics Committee of Southern Medical University approved all of the animal care and study protocols (LAEC-2020-224FS). Studies involving animals were performed in compliance with all relevant ethical regulations. For antibiotic treatment, the mice were given a combination of vancomycin (0.5 mg/ml), gentamicin (1 mg/ml), metronidazole (1 mg/ml), and ampicillin (1 mg/ml) via their drinking water for 1 week according to a previous study (Xu et al., 2021).
Ligation-induced periodontitis was induced as described previously (Abe and Hajishengallis, 2013; Pathak et al., 2021). Briefly, the mice were anesthetized by isoflurane and fixed on animal-specific fixed plates in the prone position. The oral cavity was disinfected with 75% ethanol, and a 5-0 silk thread was ligated around the left upper second molar and fixed with a surgical knot. All the knots were placed on the palatal side. After the ligation was completed, the mouse’s tongue was slightly pulled out of the mouth and placed in a warmer place until the mouse woke up. The mice were fed normal chow and had free access to drinking water.
In addition to assuring that the ligation silk line was in place every day, the mice in the PBS, F. nucleatum OMV and F. nucleatum groups were injected with PBS, F. nucleatum OMVs and F. nucleatum via the ligation silk line every day. Next, 1 × 109 colony-forming units (CFUs) of F. nucleatum or 1 μg/ml F. nucleatum OMVs in a 1 ml volume were administered to the maxillary molar teeth via the silk threads once every 3 days. If the silk thread was loose or the thread knot was incomplete, the mouse was excluded from the experiment. The day of ligation was considered as day 0. After 3, 9, and 18 days of ligation, the mice were sacrificed by cervical dislocation, and periodontal tissue samples were collected and analyzed to detect pathophysiological changes that were indicative of periodontitis.
Microcomputed Tomography
Mouse maxillary specimens fixed with paraformaldehyde were scanned by a high-resolution micro-CT (Bruker-Micro-CT, Kontich, Belgium). Data were acquired at 45 keV, with a 10 μm isotropic voxel size. Three-dimensional reconstruction was performed from a set of 400 slices, and the CEJ-ABC was measured from the cementoenamel junction to the ABC. SkyScan Data viewer software was used to measure the buccal and palatal bone resorption heights of the maxillary second molars.
Histopathological Staining
Paraffin-embedded periodontal tissue sections were sliced at a thickness of 5 μm and then stained with H&E to observe changes in alveolar bone, periodontal ligaments and gingival connective tissue. Images were obtained by microscopy (Olympus, Tokyo, Japan).
Tartrate Acid Phosphatase Staining
To visualize osteoclasts, tissue sections were stained using a TRAP kit (Servicebio, Wuhan, China). TRAP-stained histological tissue sections were examined under a microscope (Olympus, Tokyo, Japan), and images of predefined areas were captured. The TRAP-positive osteoclasts in the alveolar bone tissue section between the first and second molars were counted.
RNA Extraction and Quantitative Real-Time PCR Analysis
Total RNA was isolated from cells using TRIzol (Thermo Fisher Scientific, Waltham, MA, United States) according to the manufacturer’s protocol as we previously described (Chen et al., 2020). The total RNA was reverse transcribed into cDNA using Reverse Transcriptase Premix. Quantitative real-time PCR was performed on the ABI vii7 system using primers for the indicated genes and SYBR Green Master Mix according to the manufacturer’s instructions. The relative quantification values for each gene were calculated by the 2–ΔΔCt method, and GAPDH was used as the internal reference. The primers used (Sangon Biotech Co., Ltd., Shanghai, China) are shown in the Supplementary Table.
Immunoblot Analysis
Cells were lysed in RIPA buffer (150 mM sodium chloride, 1% Triton X-100, 0.1% SDS, 1% sodium deoxycholate, 50 mM Tris–HCl at pH 7.5, and 2 mM EDTA) containing a protease and phosphatase inhibitor cocktail (KeyGEN, Nanjing, China). The cell extracts were mixed with Protein 5× Sample Buffer (Beyotime, Shanghai, China) and boiled for 10 min. The protein extracts were subjected to SDS-PAGE and transferred onto PVDF membranes (Millipore, Burlington, MA, United States). The membranes were blocked with 5% skim milk in Tris-buffered saline supplemented with 0.1% Tween 20 for 1 h at RT and then incubated with the following primary antibodies at 4°C: β-actin (13E5), phospho-NF-κB p65 (Ser536) (93H1), NF-κB p65 (D14E12), and iNOS (D6B6S) (all purchased from Cell Signaling Technology, Danvers, MA, United States). After washing with TBST and incubating for 1 h with anti-mouse IgG (7076) or anti-rabbit IgG (7074) secondary antibodies (Cell Signaling Technologies) at RT, the protein signals were visualized using electrochemiluminescence detection kits (Merck Millipore, Billerica, MA, United States).
Statistical Analysis
Statistical analysis was performed using Prism (GraphPad Software, v8.4.0.671). The results were appropriately compared by the unpaired t-test or ANOVA and are described in each figure legend. All data are presented as the mean ± standard deviation (SD). *p < 0.05 and **p < 0.01 indicated statistical significance, and the statistical significance threshold was set to P < 0.05.
Data Availability Statement
The original contributions presented in the study are included in the article/Supplementary Material, further inquiries can be directed to the corresponding authors.
Ethics Statement
The animal study was reviewed and approved by the Experimental Animal Ethics Committee of Southern Medical University approved all of the animal care and study protocols (LAEC-2020-224FS).
Author Contributions
GC completed the purification, analysis, and identification of extracellular vesicles. QS participated in bacterial culture, cell culture, and some testing. QC participated in animal experimental modeling and early coculture of extracellular vesicles and gingival fibroblasts. HZ designed and directed the whole project. All authors reviewed and edited the manuscript.
Funding
This study was supported by the National Natural Science Foundation of China Youth Fund (81702979), National Natural Science Foundation of China, and Science Fund for Distinguished Young Scholars (81925026).
Conflict of Interest
The authors declare that the research was conducted in the absence of any commercial or financial relationships that could be construed as a potential conflict of interest.
Publisher’s Note
All claims expressed in this article are solely those of the authors and do not necessarily represent those of their affiliated organizations, or those of the publisher, the editors and the reviewers. Any product that may be evaluated in this article, or claim that may be made by its manufacturer, is not guaranteed or endorsed by the publisher.
Acknowledgments
We are grateful to Liping Wang and Haobo Sun (Guangzhou Medical University) for their excellent technical assistance with the mouse model of periodontitis. We thank Can Xiao, Yan He, Kun Wen, Muxuan Chen, Feng Zhang, and Jun Man for their in vitro tests and guidance. We thank the members of the Microbiome Medicine Center for their helpful discussions.
Supplementary Material
The Supplementary Material for this article can be found online at: https://www.frontiersin.org/articles/10.3389/fmicb.2022.815638/full#supplementary-material
Supplementary Figure 1 | (A) The molecular weight of F. nucleatum OMVs was approximately 40 kD as determined by SDS-PAGE. (B) Mass spectrometry revealed the main component to be a major outer membrane protein that plays roles in (1) immune regulation and (2) and adhesion as well as serves as a (3) channel protein.
Supplementary Figure 2 | (A) M1 macrophages marker (CD86) and M2 macrophages markers (Arg-1 and CD163) were detected by western blot after OMVs or IL-4 + IL-13 for 24 h. IL-4/IL-13 were used for positive control. OMVs could not change the protein expression of M2 macrophages markers. (B) The mRNA level of Arg-1 and CD163 was not altered significantly after OMVs or IL-4 + IL-13 stimulation for 24 h. (C) ELISA analysis of M1 marker (TNF-α) and M2 marker (IL-10) in cultured supernatant of macrophages treated with OMVs or IL-4 + IL-13 for 24 h. (D) Cytokines (IL-1β, TNF-α, and IL-6) secreted by macrophages gradually increased with time. After stimulation with Fn OMVs for 12 h, macrophages secreted the most inflammatory factors.
Supplementary Figure 3 | Immunofluorescence analysis showed the expression of fibroblasts markers FSP1 in MGFs.
References
Abe, T., and Hajishengallis, G. (2013). Optimization of the ligature-induced periodontitis model in mice. J. Immunol. Methods 394, 49–54. doi: 10.1016/j.jim.2013.05.002
Almubarak, A., Tanagala, K. K. K., Papapanou, P. N., Lalla, E., and Momen-Heravi, F. (2020). Disruption of monocyte and macrophage homeostasis in periodontitis. Front. Immunol. 11:330. doi: 10.3389/fimmu.2020.00330
Boopathi, S., Liu, D., and Jia, A. Q. (2021). Molecular trafficking between bacteria determines the shape of gut microbial community. Gut Microbes 13:1959841. doi: 10.1080/19490976.2021.1959841
Brennan, C. A., and Garrett, W. S. (2019). Fusobacterium nucleatum - symbiont, opportunist and oncobacterium. Nat. Rev. Microbiol. 17, 156–166. doi: 10.1038/s41579-018-0129-6
Briaud, P., and Carroll, R. K. (2020). Extracellular vesicle biogenesis and functions in gram-positive bacteria. Infect. Immun. 88:e433-420. doi: 10.1128/IAI.00433-20
Chen, G., Tu, Y., Aladelusi, T. O., Zhao, S., Chen, J., Jin, L., et al. (2020). Knocking down B7H3 expression enhances cell proliferation of SHEDs via the SHP1/AKT signal axis. Biochem. Biophys. Rese. Commun. 531, 282–289. doi: 10.1016/j.bbrc.2020.06.154
Chen, T., Li, Q., Wu, J., Peng, W., Li, H., Wang, J., et al. (2018). Fusobacterium nucleatum promotes M2 polarization of macrophages in the microenvironment of colorectal tumours via a TLR4-dependent mechanism. Cancer Immunol. Immunother. 67, 1635–1646. doi: 10.1007/s00262-018-2233-x
Curtis, M. A., Diaz, P. I., and Van Dyke, T. E. (2020). The role of the microbiota in periodontal disease. Periodontol. 2000 83, 14–25. doi: 10.1111/prd.12296
Dahlstrand Rudin, A., Khamzeh, A., Venkatakrishnan, V., Basic, A., Christenson, K., and Bylund, J. (2021). Short chain fatty acids released by Fusobacterium nucleatum are neutrophil chemoattractants acting via free fatty acid receptor 2 (FFAR2). Cell Microbiol. 23:e13348. doi: 10.1111/cmi.13348
Deo, P., Chow, S. H., Han, M. L., Speir, M., Huang, C., Schittenhelm, R. B., et al. (2020). Mitochondrial dysfunction caused by outer membrane vesicles from Gram-negative bacteria activates intrinsic apoptosis and inflammation. Nat. Microbiol. 5, 1418–1427. doi: 10.1038/s41564-020-0773-2
Durant, L., Stentz, R., Noble, A., Brooks, J., Gicheva, N., Reddi, D., et al. (2020). Bacteroides thetaiotaomicron-derived outer membrane vesicles promote regulatory dendritic cell responses in health but not in inflammatory bowel disease. Microbiome 8:88. doi: 10.1186/s40168-020-00868-z
Ebersole, J. L., Kirakodu, S. S., and Gonzalez, O. A. (2020). Oral microbiome interactions with gingival gene expression patterns for apoptosis, autophagy and hypoxia pathways in progressing periodontitis. Immunology 162, 405–417. doi: 10.1111/imm.13292
Ellis, T. N., and Kuehn, M. J. (2010). Virulence and immunomodulatory roles of bacterial outer membrane vesicles. Microbiol. Mol. Biol. Rev. 74, 81–94. doi: 10.1128/MMBR.00031-09
Engevik, M. A. (2020). Fusobacterium nucleatum secretes outer membrane vesicles and promotes intestinal inflammation. mbio 12:e2706-20. doi: 10.1128/mBio.02706-20
Feres, M., Retamal-Valdes, B., Goncalves, C., Cristina Figueiredo, L., and Teles, F. (2021). Did Omics change periodontal therapy? Periodontol. 2000 85, 182–209. doi: 10.1111/prd.12358
Galli, G., and Saleh, M. (2020). Immunometabolism of macrophages in bacterial infections. Front. Cell. Infect. Microbiol. 10:607650. doi: 10.3389/fcimb.2020.607650
Gao, H., Sun, T., Yang, F., Yuan, J., Yang, M., Kang, W., et al. (2020). The pathogenic effects of Fusobacterium nucleatum on the proliferation, osteogenic differentiation, and transcriptome of osteoblasts. Front. Cell Dev Biol. 8:807. doi: 10.3389/fcell.2020.00807
Guo, S., Chen, J., Chen, F., Zeng, Q., Liu, W. L., and Zhang, G. (2020). Exosomes derived from Fusobacterium nucleatum-infected colorectal cancer cells facilitate tumour metastasis by selectively carrying miR-1246/92b-3p/27a-3p and CXCL16. Gut Online ahead of print doi: 10.1136/gutjnl-2020-321187
Hu, L., Liu, Y., Kong, X., Wu, R., Peng, Q., Zhang, Y., et al. (2021). Fusobacterium nucleatum facilitates M2 macrophage polarization and colorectal carcinoma progression by activating TLR4/NF-kappaB/S100A9 cascade. Front. Immunol. 12:658681. doi: 10.3389/fimmu.2021.658681
Kaan, A. M. M., Kahharova, D., and Zaura, E. (2021). Acquisition and establishment of the oral microbiota. Periodontol. 2000 86, 123–141. doi: 10.1111/prd.12366
Kang, W., Jia, Z., Tang, D., Zhang, Z., Gao, H., He, K., et al. (2019b). Fusobacterium nucleatum facilitates apoptosis, ROS generation, and inflammatory cytokine production by activating AKT/MAPK and NF-kappaB signaling pathways in human gingival fibroblasts. Oxid. Med. Cell Longev. 2019:1681972. doi: 10.1155/2019/1681972
Kang, W., Ji, X., Zhang, X., Tang, D., and Feng, Q. (2019a). Persistent exposure to Fusobacterium nucleatum triggers chemokine/cytokine release and inhibits the proliferation and osteogenic differentiation capabilities of human gingiva-derived mesenchymal stem cells. Front. Cell. Infect. Microbiol. 9:429. doi: 10.3389/fcimb.2019.00429
Kezuka, Y., Ishida, T., Yoshida, Y., and Nonaka, T. (2018). Structural insights into the catalytic mechanism of cysteine (hydroxyl) lyase from the hydrogen sulfide-producing oral pathogen, Fusobacterium nucleatum. Biochem. J. 475, 733–748. doi: 10.1042/BCJ20170838
Li, C., Zhu, L., Wang, D., Wei, Z., Hao, X., Wang, Z., et al. (2021). T6SS secretes an LPS-binding effector to recruit OMVs for exploitative competition and horizontal gene transfer. ISME J. 16, 500–510. doi: 10.1038/s41396-021-01093-8
Li, D. H., Li, Z. P., Yan, Z., Zhou, G.-Z., Ren, R.-R., Zhao, H.-J., et al. (2021). Fecal Fusobacterium nucleatum harbored virulence gene fadA are associated with ulcerative colitis and clinical outcomes. Microb. Pathog. 157:104964. doi: 10.1016/j.micpath.2021.104964
Liu, J., Hsieh, C. L., Gelincik, O., Devolder, B., Sei, S., Zhang, S., et al. (2019). Proteomic characterization of outer membrane vesicles from gut mucosa-derived fusobacterium nucleatum. J. Proteomics 195, 125–137. doi: 10.1016/j.jprot.2018.12.029
Liu, L., Liang, L., Liang, H., Wang, M., Lu, B., Xue, M., et al. (2019). Fusobacterium nucleatum aggravates the progression of colitis by regulating M1 macrophage polarization via AKT2 pathway. Front. Immunol. 10:1324. doi: 10.3389/fimmu.2019.01324
Liu, L., Liang, L., Yang, C., Zhou, Y., and Chen, Y. (2021). Extracellular vesicles of Fusobacterium nucleatum compromise intestinal barrier through targeting RIPK1-mediated cell death pathway. Gut Microbes 13, 1–20. doi: 10.1080/19490976.2021.1902718
Locati, M., Curtale, G., and Mantovani, A. (2020). Diversity, mechanisms, and significance of macrophage plasticity. Annu. Rev. Pathol. 15, 123–147. doi: 10.1146/annurev-pathmechdis-012418-012718
Marchesan, J., Girnary, M. S., Jing, L., Miao, M. Z., Zhang, S., Sun, L., et al. (2018). An experimental murine model to study periodontitis. Nat. Protoc. 13, 2247–2267. doi: 10.1038/s41596-018-0035-4
Martin-Gallausiaux, C., Malabirade, A., Habier, J., and Wilmes, P. (2020). Fusobacterium nucleatum extracellular vesicles modulate gut epithelial cell innate immunity via FomA and TLR2. Front. Immunol. 11:583644. doi: 10.3389/fimmu.2020.583644
Meng, Q., Gao, Q., Mehrazarin, S., Tangwanichgapong, K., Wang, Y., Huang, Y., et al. (2021). Fusobacterium nucleatum secretes amyloid-like FadA to enhance pathogenicity. EMBO Rep. 22:e52891. doi: 10.15252/embr.202152891
Mizraji, G., Segev, H., Wilensky, A., and Hovav, A. H. (2013). Isolation, processing and analysis of murine gingival cells. J. Vis. Exp. 77:e50388. doi: 10.3791/50388
Myneni, S. R., Brocavich, K., and H Wang, H. (2020). Biological strategies for the prevention of periodontal disease: probiotics and vaccines. Periodontol. 2000 84, 161–175. doi: 10.1111/prd.12343
Nakagaki, H., Sekine, S., Terao, Y., Toe, M., Tanaka, M., Ito, H. O., et al. (2010). Fusobacterium nucleatum envelope protein FomA is immunogenic and binds to the salivary statherin-derived peptide. Infect. Immun. 78, 1185–1192. doi: 10.1128/IAI.01224-09
Parhi, L., Alon-Maimon, T., Sol, A., Nejman, D., Shhadeh, A., Fainsod-Levi, T., et al. (2020). Breast cancer colonization by Fusobacterium nucleatum accelerates tumor growth and metastatic progression. Nat. Commun. 11:3259. doi: 10.1038/s41467-020-16967-2
Pathak, J. L., Fang, Y., Chen, Y., Ye, Z., Guo, X., Yan, Y., et al. (2021). Downregulation of macrophage-specific Act-1 intensifies periodontitis and alveolar bone loss possibly via TNF/NF-kappaB signaling. Front. Cell. Dev. Biol. 9:628139. doi: 10.3389/fcell.2021.628139
Richardson, M., Ren, J., Rubinstein, M. R., Taylor, J. A., Friedman, R. A., Shen, B., et al. (2020). Analysis of 16S rRNA genes reveals reduced Fusobacterial community diversity when translocating from saliva to GI sites. Gut Microbes. 12, 1–13. doi: 10.1080/19490976.2020.1814120
Rubinstein, M. R., Baik, J. E., Lagana, S. M., Han, R. P., Raab, W. J., Sahoo, D., et al. (2019). Fusobacterium nucleatum promotes colorectal cancer by inducing Wnt/beta-catenin modulator Annexin A1. EMBO Rep. 20:e47638. doi: 10.15252/embr.201847638
Rubinstein, M. R., Wang, X., Liu, W., Hao, Y., Cai, G., and Han, Y. W. (2013). Fusobacterium nucleatum promotes colorectal carcinogenesis by modulating E-cadherin/beta-catenin signaling via its FadA adhesin. Cell Host Microbe 14, 195–206. doi: 10.1016/j.chom.2013.07.012
Russell, D. G., Huang, L., and VanderVen, B. C. (2019). Immunometabolism at the interface between macrophages and pathogens. Nat. Rev. Immunol. 19, 291–304. doi: 10.1038/s41577-019-0124-9
Sczepanik, F. S. C., Grossi, M. L., Casati, M., Goldberg, M., Glogauer, M., Fine, N., et al. (2020). Periodontitis is an inflammatory disease of oxidative stress: we should treat it that way. Periodontol. 2000 84, 45–68. doi: 10.1111/prd.12342
Szafranski, S. P., Slots, J., and Stiesch, M. (2021). The human oral phageome. Periodontol. 2000 86, 79–96. doi: 10.1111/prd.12363
Tateishi, F., Hasegawa-Nakamura, K., Nakamura, T., Oogai, Y., Komatsuzawa, H., Kawamata, K., et al. (2012). Detection of Fusobacterium nucleatum in chorionic tissues of high-risk pregnant women. J. Clin. Periodontol. 39, 417–424. doi: 10.1111/j.1600-051X.2012.01855.x
Thurnheer, T., Karygianni, L., Flury, M., and Belibasakis, G. N. (2019). Fusobacterium species and subspecies differentially affect the composition and architecture of supra- and subgingival biofilms models. Front. Microbiol. 10:1716. doi: 10.3389/fmicb.2019.01716
Tulkens, J., Vergauwen, G., Van Deun, J., Geeurickx, E., Dhondt, B., Lippens, L., et al. (2020). Increased levels of systemic LPS-positive bacterial extracellular vesicles in patients with intestinal barrier dysfunction. Gut 69, 191–193. doi: 10.1136/gutjnl-2018-317726
Wade, W. G. (2021). Resilience of the oral microbiome. Periodontol. 2000 86, 113–122. doi: 10.1111/prd.12365
Wang, W., Zheng, C., Yang, J., and Li, B. (2021). Intersection between macrophages and periodontal pathogens in periodontitis. J. Leukoc. Biol. 110, 577–583. doi: 10.1002/JLB.4MR0421-756R
Wu, X., Chen, H., Wang, Y., and Gu, Y. (2020). Akt2 affects periodontal inflammation via altering the M1/M2 ratio. J. Dent. Res. 99, 577–587. doi: 10.1177/0022034520910127
Xu, K., Gao, X., Xia, G., Chen, M., Zeng, N., Wang, S., et al. (2021). Rapid gut dysbiosis induced by stroke exacerbates brain infarction in turn. Gut Online ahead of print doi: 10.1136/gutjnl-2020-323263
Xue, Y., Xiao, H., Guo, S., Xu, B., Liao, Y., Wu, Y., et al. (2018). Indoleamine 2,3-dioxygenase expression regulates the survival and proliferation of Fusobacterium nucleatum in THP-1-derived macrophages. Cell Death Dis. 9:355. doi: 10.1038/s41419-018-0389-0
Ying, W., Cheruku, P. S., Bazer, F. W., Safe, S. H., and Zhou, B. (2013). Investigation of macrophage polarization using bone marrow derived macrophages. J. Vis. Exp. 23:50323. doi: 10.3791/50323
Zanzoni, A., Spinelli, L., Braham, S., and Brun, C. (2017). Perturbed human sub-networks by Fusobacterium nucleatum candidate virulence proteins. Microbiome 5:89. doi: 10.1186/s40168-017-0307-1
Zhang, B., Yang, Y., Yi, J., Zhao, Z., and Ye, R. (2021). Hyperglycemia modulates M1/M2 macrophage polarization via reactive oxygen species overproduction in ligature-induced periodontitis. J. Periodontal. Res. 56, 991–1005. doi: 10.1111/jre.12912
Keywords: Fusobacterium nucleatum, outer membrane vesicles, periodontitis, macrophages, inflammation
Citation: Chen G, Sun Q, Cai Q and Zhou H (2022) Outer Membrane Vesicles From Fusobacterium nucleatum Switch M0-Like Macrophages Toward the M1 Phenotype to Destroy Periodontal Tissues in Mice. Front. Microbiol. 13:815638. doi: 10.3389/fmicb.2022.815638
Received: 15 November 2021; Accepted: 31 January 2022;
Published: 21 March 2022.
Edited by:
Marcelo J. Kuroda, University of California, Davis, United StatesCopyright © 2022 Chen, Sun, Cai and Zhou. This is an open-access article distributed under the terms of the Creative Commons Attribution License (CC BY). The use, distribution or reproduction in other forums is permitted, provided the original author(s) and the copyright owner(s) are credited and that the original publication in this journal is cited, in accordance with accepted academic practice. No use, distribution or reproduction is permitted which does not comply with these terms.
*Correspondence: QiaoLing Cai, Y2FpcWlhb2xpbmcxMjAwM0AxNjMuY29t; HongWei Zhou, YmlvZGVncmFkYXRpb25AZ21haWwuY29t