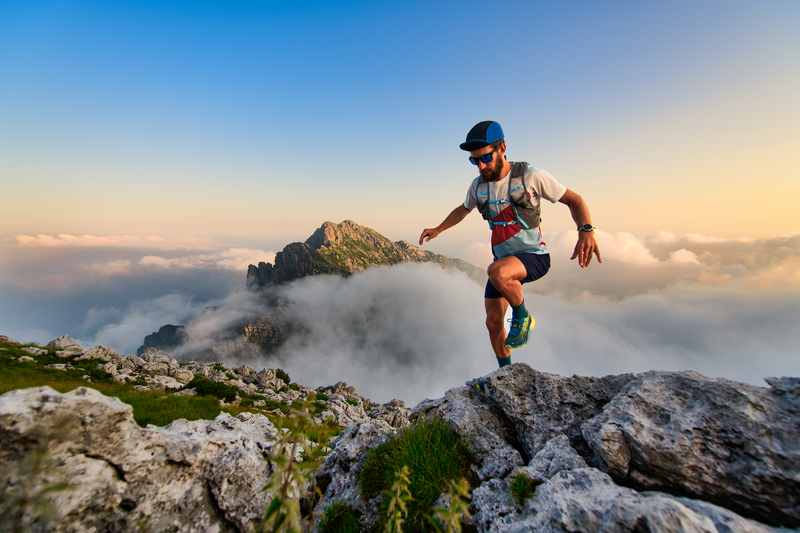
95% of researchers rate our articles as excellent or good
Learn more about the work of our research integrity team to safeguard the quality of each article we publish.
Find out more
REVIEW article
Front. Microbiol. , 24 March 2022
Sec. Antimicrobials, Resistance and Chemotherapy
Volume 13 - 2022 | https://doi.org/10.3389/fmicb.2022.807955
Beta-lactam antibiotics remain one of the most commonly prescribed drug classes, but they are limited by their propensity to cause hypersensitivity reactions (e.g., from allergy to anaphylaxis) as well as by the emergence of bacteria with a myriad of resistance mechanisms such as β-lactamases. While development efforts continue to focus on overcoming resistance, there are ongoing concerns regarding cross-contamination of β-lactams during manufacturing and compounding of these drugs. Additionally, there is a need to reduce levels of drugs such as β-lactam antibiotics in waste-water to mitigate the risk of environmental exposure. To help address future development of effective remediation chemistries and processes, it is desired to better understand the structural relationship among the most common β-lactams. This study includes the creation of a class-wide structural ordering of the entire β-lactam series, including both United States Food and Drug Association (US-FDA)-approved drugs and experimental therapies. The result is a structural relational map: the “Lactamome,” which positions each substance according to architecture and chemical end-group. We utilized a novel method to compare the structural relationships of β-lactam antibiotics among the radial cladogram and describe the positioning with respect to efficacy, resistance to hydrolysis, reported hypersensitivity, and Woodward height. The resulting classification scheme may help with the development of broad-spectrum treatments that reduce the risk of occupational exposure and negative environmental impacts, assist practitioners with avoiding adverse patient reactions, and help direct future drug research.
Since the first clinical uses of penicillin G in the 1930s and 1940s, β-lactam antimicrobials have enjoyed marked success in the treatment of bacterial infections. By 1944 penicillin was being manufactured at the rate of over a billion doses a year (Aldridge et al., 1999). Shortly after the introduction of the penam penicillin, the first chemical compounds of the cephem group were isolated from the fungus Cephalosporium acremonium by Giuseppe Brotzu in 1948, when crude filtrates of a culture were found to inhibit the growth of Staphylococcus aureus (Singh and Arrieta, 1999; Foye et al., 2008). The first cephem, cephalothin, became available for patients in the United States as a parenteral drug in 1964 (Greenwood, 2008). Interest in β-lactam antibiotics spurred scientists to explore a breadth of structural derivatives and platforms well beyond the above-mentioned natural substances in efforts to expand the spectrum. Those synthetic efforts have resulted in four major β-lactam classes: monobactam, penams, penems, and cephems (Figure 1).
Figure 1. The chemical graphs and scaffold of primary examples of major β-lactam antibiotic classes. Common atom numbering scheme of the chemotype is depicted and oriented above the example β-lactam ring. The X may be a carbon, an oxygen atom, or a sulfur atom. For example, penem structures with X=C are “carbapenems”; penem structures with X=O are “oxapenems”; and penem structures with X=S are “thiapenems,” or more commonly, simply “penems.”
The historical development process as well as structural and functional (e.g., spectra of activity) differences among the various classes of β-lactams are described in detail in several excellent reviews (Bush and Bradford, 2016; Ochoa-Aguilar et al., 2016; Lima et al., 2020). Aside from the impacts resulting from electron withdrawing substituents by different R groups, the key structural features of all β-lactam antibiotics include (1) the 2-azetidinone, a 4-membered cyclic amide required for their characteristic target acylation, (2) the level of cyclic amide ring strain imposed by bicyclic structural designs, and (3) an acidic moiety critical to their recognition by the transpeptidase active site (Figure 2).
Figure 2. Structural basis of lactam activity. (A) The D-Ala-D-Ala amino acid is depicted in red. (B) The 1st generation penam ampicillin is depicted in black. (C) D-Ala-D-Ala dipeptide overlay with the β-lactam ampicillin antibiotic.
While many previous studies have investigated the intricacies of drug binding and the impacts on efficacy and resistance, the relationship of molecular structure on the ability of chemical compounds and processes to decontaminate β-lactam residues from manufacturing and compounding facilities as well as terrestrial and aquatic environments has received less attention. Because of their perceived or actual potential to cause allergic responses in a substantial portion of the host population, governmental guidelines and regulations involve special precautions to reduce the risk of cross-contamination from β-lactams (Food and Drug Administration [FDA], 2013). The chemicals that might be candidates for decontamination of surfaces in facilities or for waste-water treatment should be effective against a broad range of drugs at levels that are already employed for disinfection and be readily biodegradable themselves.
As such, it was desired to assess the structural relationships among β-lactams to understand if a subset of drugs can serve as a proxy for others during development and testing. As a first step toward this attempt of a “read-across,” the most widely used β-lactam antibiotics have been reviewed, molecularly modeled, and structurally organized into relational maps termed a “Lactamome.”
The monocyclic β-lactams (or monobactam) class represents the simplest structural platform among these antibiotics. The monocyclic platform of this b-lactam was first isolated from Chromobacterium violaceum (Asai et al., 1981; Imada et al., 1981; Sykes et al., 1981; Decuyper et al., 2018; Goldberg et al., 2021). There are three major monocyclic β-lactams: aztreonam, tigemonam, nocardicin A, and two minor monocyclic β-lactams: carumonam (only approved in Japan) and tabtoxin. Tabtoxin, the only member retaining a nitrogen-hydrogen bond, is uniquely a glutamine synthetase inhibitor with lower activity against penicillin-binding proteins (PBPs). The others possess an N-sulfonic acid moiety or N-acetic acid moiety. Aztreonam (Figure 1), currently the only monocyclic β-lactams clinically used in the United States, exhibits potent inhibition of Gram-negative PBPs (Georgopapadakou et al., 1982; Sykes et al., 1982; Rittenbury, 1990; Cunha, 1993) but finds limited clinical utility owing to its lack of activity against Gram-positive or anaerobic bacteria. Because of its unique monocyclic β-lactam structure, aztreonam is thought to have no cross-sensitivity to patients allergic to penicillin (Gaeta et al., 2015). Currently there is pre-clinical research in aztreonam combination with other β-lactams and diazabicyclooctane monocyclic β-lactams against MDR/XDR Gram-negative bacteria (Bassetti et al., 2021; Bhatnagar et al., 2021). A second generation of monocyclic β-lactams is also under late stage clinical development (Osborn et al., 2019).
Penams are a class of β-lactams possessing an azabicyclo[3.2.0]heptane ring system, a carboxylic acid moiety at C3, and a sulfur atom at position one (Figure 1). The prototypic and most well-known penam is penicillin G (PNG or benzylpenicillin), isolated from Penicillium chrysogenum (Fleming, 2001). The other commonly used natural product penam is penicillin V, or phenoxymethylpenicillin. In the absence of β-lactamases, the natural penicillins show good Gram-positive activity but fail to inhibit the growth of many Gram-negative organisms (Libby and Holmberg, 1945; Eagle, 1946; Eagle and Technical Assistance of Arlyne D. Musselman, 1947; Nell and Hill, 1947; Thomsen and Larsen, 1962). A great deal of creative synthetic chemistry has been directed to the penam platform to modulate the spectrum of this class, predominantly via modifications at the C6 acylamino group (R1 side chain). Clinically useful penam derivatives have geminal dimethyl groups at C2, except tazobactam which is used in combination therapies as a β-lactamase inhibitor. The penam amoxicillin is one of the most often prescribed antibiotics in the United States, many other countries, and in veterinary medicine (Tao et al., 2019).
Penems are direct analogs of penams but with 2,3-unsaturation within the fused five-membered ring (Figure 1). The thiapenems, which contain a sulfur atom in the fused ring at position one, do not exist naturally but were synthesized in 1978 by chemists including R.B. Woodward (Ernest et al., 1978). The penem class is one of a few β-lactam antibiotics still being researched, and includes faropenem, ritipenem, and sulopenem (Batchelder et al., 2020; Butler et al., 2022; Sato et al., 2022). While no members of the thiapenem class are currently used in United States clinical settings, safety and efficacy data required for FDA approval are forthcoming. However, there are several FDA-approved members of a subclass of penems called carbapenems, which possess a carbon atom in place of the sulfur “X” atom. The structure of ertapenem is shown in Figure 1 as an example of this class. The C6 hydroxyethyl substituent possessed by members of the penem class represents a significant departure from penams and cephems, which generally exhibit large and variable aminoacyl substituents at C6. Differences in activity and spectrum among compounds of this class are influenced by cis alignment of the vicinal R1 hydroxyl and C6 hydrogen and dictated by the C2 substituent. Given their broad spectrum, as well as the β-lactamase stability afforded by their C6 R-hydroxyethyl side chain and trans C5-C6 hydrogen geometry (Basker et al., 1980), the carbapenems have found most of their clinical utility as antimicrobials for infections with multidrug-resistant organisms.
Cephems, also known as cephalosporins, differ from penems by having a dihydrothiazine ring fused to the β-lactam ring (Hughes, 2017a,b). First generation cephems generally possess an aromatic acylamino side chain at C7 and exhibit potent Gram-positive activity (Godzeski et al., 1963; Spencer et al., 1966a,b; Ryan et al., 1969; Hsieh and Ho, 1975).
As cephems entered a second generation, drug developers explored other substituents at C7, such as the replacement of the acetoxy group with various nucleophiles (i.e., carbamates, heterocyclic mercaptans, and pyridines). These cephems exhibit broader spectrum than their earlier counterparts, with some showing potent inhibition of Gram-negatives (Eykyn et al., 1973; Kosmidis et al., 1973; Hamilton-Miller et al., 1974; Neu, 1974a,b; Nomura et al., 1974; Wallick and Hendlin, 1974; Tsuchiya et al., 1978). The broad cephem class also contains the cephamycin (and cephalosporin) class which also has the cephem nucleus and includes the second generation cefoxitin and cefotetan (Kosmidis et al., 1973; Neu, 1974b; Wallick and Hendlin, 1974).
In third generation designs, the aryl group was modified to an aminothiazole, a change found to enhance Gram-negative activity. One notable outlier is cefoperazone, which borrows its C7 acylamino group from the ureidopenicillin piperacillin. This group exhibits not only further expanded spectrum compared to previous generations, but maintains stability to serine β-lactamases (Sosna et al., 1978; Kojo et al., 1979; Matsubara et al., 1979; O’Callaghan et al., 1980; Reiner et al., 1980; Shannon et al., 1980; Verbist and Verhaegen, 1980; Wise et al., 1980; Neu et al., 1981, 1984, 1989; Kamimura et al., 1984; Inamoto et al., 1988, 1990a,b; Yokoo et al., 1991).
With fourth generation cephems, quaternary nitrogen substituents were incorporated at C3 to enhance passive transport through the Gram-negative outer membrane, decrease affinity for β-lactamases, and promote departure of the leaving group (Machka and Braveny, 1983; Seibert et al., 1983; Khan et al., 1984; Kessler et al., 1985; Limbert et al., 1991; Chin et al., 1992; Hancock and Bellido, 1992).
The fifth generation of cephems was specifically engineered to combat various resistance problems while attempting to maintain a broad spectrum of activity (Entenza et al., 2002; Wootton et al., 2002; Zbinden et al., 2002; Ishikawa et al., 2003; Iizawa et al., 2004; Issa et al., 2004; Sader et al., 2005; Takeda et al., 2007a,b; Toda et al., 2008; Ito et al., 2016; Kohira et al., 2016; Dobias et al., 2017; Aoki et al., 2018). In general, these cephems maintain the alkoxyimino group, exchange the aminothiazole for an aminothiadiazole, and install conjugation or extended ring systems in their C3 substituents.
A unique and small modification to the cephem class is the oxacephem, which possesses an azabicyclo[4.2.0]oct-2-ene ring system, a carboxylic acid moiety at C4, and, as the name suggests, an oxygen at position one in place of sulfur. The major oxacephems are moxalactam (latamoxef) and flomoxef. These compounds were developed to reduce blood coagulation defects and, more specifically, target the bacterial genus Nocardia (Yazawa et al., 1989; Cazzola et al., 1993).
b-lactamase inhibitors include subclasses of penam β-lactams resistant to hydrolysis by β-lactamases. While the success of β-lactam antibiotics has been extraordinary, their long-term usage has collided with the evolution of resistant microbial strains. Myriad resistance determinants can contribute to such resistance, but β-lactamases are of particular concern due to their high catalytic efficiency and their potential for rapid dissemination by horizontal transfer on plasmids. Major β-lactam-based β-lactamase inhibitors include sulbactam, clavulanate, and tazobactam. These β-lactam molecules exhibit little direct antibacterial properties by themselves but serve a sacrificial role by tenaciously binding to β-lactamases to thereby enable the unimpeded performance of a partner β-lactam such as amoxicillin (Pechere and Kohler, 1999; Shapiro et al., 2021).
β-lactam antibiotics act through structural mimicry of the D-alanine-D-alanine motif in peptidoglycans of the bacterial cell wall (Figure 2). They inhibit bacterial transpeptidases which catalyze the cross-linkage of peptidoglycan through the formation of isopeptide bonds (Goffin and Ghuysen, 1998; Macheboeuf et al., 2006; Sauvage et al., 2008). Peptidoglycan is an essential component of the bacterial cell wall and plays major roles in protecting bacteria from environmental stress, ensuring osmotic stability. Cell wall degradation and resynthesis are critical to bacterial cell growth and division. The major target of β-lactams are PBPs, which are essential peptide cross-linking enzymes and are essential for peptidoglycan synthesis. In the presence of a β-lactam, the serine nucleophile attacks the lactam carbonyl, resulting in a stable acyl-enzyme complex (Yocum et al., 1979; Waxman et al., 1980). This action interrupts the integrity of the bacterial cell wall, diminishing the capacity for growth and division and removing protection from osmotic or tensile stress. A second major mechanism of action, specific to carbapenem β-lactams are the L,D-transpeptidases. For example, faropenem efficacy against mycobacteria is due to L,D transpeptidase covalent adduct formation (Lohans et al., 2019; Lu et al., 2020).
While target affinity and site accumulation are important factors, the efficacy of β-lactams has canonically been dominated by their propensity for chemical acylation, and therefore, a consequence of their ease of entry into the PBP site (i.e., a diffusion-limited kinetic process). This chemical reactivity is strongly related to the geometry at the β-lactam nitrogen and the degree of ring strain. In monocyclic structures, such as monobactams, the amide bond is stabilized by conjugation between the nitrogen lone pair and the carbonyl group. In bicyclic structures, the amide stabilization is attenuated by a third bonding partner, which creates a trigonal pyramidal (sp3) nitrogen geometry. The reduced amide resonance conferred by these bicyclic systems affords increased carbonyl electrophilicity and higher intrinsic reactivity to nucleophiles and nucleophile-containing enzymes such as transpeptidases, β-lactamases, and metallo-β-lactamases (Green et al., 1965; Morin et al., 1969; Sweet and Dahl, 1970; Glover and Rosser, 2012; Szostak et al., 2015; Ismalaj, 2022).
Considering the trigonal pyramid, defined by the nitrogen (i.e., the apex) and its three bond-pair substituents (i.e., the base) (Figure 3), the distortion from a planar (sp2) amide can be defined by a parameter known as the Woodward height (h-Woodward) (Ernest et al., 1978; Lang et al., 1978; Woodward, 1980; Meng et al., 2021; Ismalaj, 2022). Monocyclic monobactams, having an essentially planar geometry at nitrogen, tend to be the least reactive of the β-lactams with negligible h-Woodward values (Diaz et al., 2002).
Figure 3. Structural flexibility of β-lactams and the h-Woodward coefficient. (A) General bicyclic β-lactam structure rendered to show pyramidal nitrogen. (B) Depiction of h-Woodward, defined by height of nitrogen to trigonal pyramid ligand base. (C) Faropenem depicted in stick form. (D) Faropenem energy minimized (using MOE with the Amber10:ETH forcefield) and angle of the bent nitrogen bonds showing the h-Woodward of 0.5 Å.
Cephems are sterically predisposed toward Woodward heights of about 0.2–0.3 Å, due to conformational flexibility afforded by their six-membered ring fusion. However, cephalosporins have unique features that contribute to their chemical reactivities beyond h-Woodward. A competitive enamine resonance resulting from the delocalization of the nitrogen’s lone electron pair into the π electron system of the dihydrothiazine ring contributes to attenuated amide resonance (Morin et al., 1969; Sweet and Dahl, 1970). In the setting of this resonance, heteroatomic moieties at the C3 position can act as leaving groups upon ring opening. Whether or not it is a leaving group, the C3 substituent can participate in long range inductive effects with the β-lactam amide moiety–strongly electron-withdrawing groups are postulated to enhance the electrophilicity of the carbonyl (Morin et al., 1969; Boyd et al., 1975; Boyd, 1984).
Penams exhibit still larger h-Woodward (∼0.4 Å) values than cephams due to the additional strain imposed by five-membered ring fusion, and as such exhibit higher intrinsic reactivity to nucleophiles. Interestingly, molecules of the penam class can exist in two distinct conformational states, one in which the C3 carboxylate is in an equatorial position in relation to the ring system and one in which it is axial (Abrahamsson et al., 1963; James et al., 1968; Dexter and van der Veen, 1978).
Structural analysis reveals carbapenems and thiapenems as having h-Woodward values of 0.50–0.60 Å; these drugs are recognized as among the most reactive β-lactams. Such higher values may be attributed to the additional geometric constraints introduced by the unsaturation between C2 and C3 of the pyrrolidine ring, as well as replacement of the position one sulfur atom with a carbon atom in carbapenems. Their rapid acylation of PBPs have been ascribed to this highly activated β-lactam ring system. Such ring opening propensity has been shown by examining the kinetics of base hydrolysis (Woodward, 1980). Results indicate the penems are the most readily hydrolyzed of known β-lactams (Knox, 1961; Frere et al., 1975; Graves-Woodward and Pratt, 1998; Hujer et al., 2005; Silvaggi et al., 2005; Jeong et al., 2018).
Considering the evolution and history of β-lactams, this study utilized a novel method to examine the chemical similarity and interrelatedness of β-lactam antibiotics. To perform this analysis, the complete pairwise chemical similarity was calculated among β-lactam drugs approved by the FDA or compounds in late-stage clinical trials, to create a radial cladogram analogous to the common method of how genes, strains or species are compared in a phylogeny. The analysis was performed using the industry standard Tanimoto coefficient similarity score calculated from keyed organic chemical groups using ChemMine and visualized using Dendroscope (Huson et al., 2007; Backman et al., 2011; Peterson et al., 2013). The resulting “Lactamome” (Figure 4) was depicted with benzylpenicillin (penicillin G) as the top-most molecule, owing to its place as a foundational member. The resulting circular chemogram (dendrogram of chemicals) was color-coded according to known β-lactam chemical classes. The utility of chemical clustering is that relationships are unbiased and based solely on organic chemistry. Broadly speaking, the Lactamome shows the major penam and cephem clusters in relation to the minor monobactam, β-lactam-based β-lactamase inhibitor, oxacephem, carbapenem and penem classes. The positioning reflects the similarity of the atomic arrangements in fused or unfused ring systems in relation to the β-lactam foundation, coupled with the electronic effects of substituent end groups. Beginning with benzylpenicillin, penems cluster in four distinct subclasses. This is followed by two major branches and five subgroups of the cephem class.
Figure 4. The Lactamome: Radial chemogram of major β-lactams by chemical similarity. Clustering used hierarchical complete linkage MAACS-keyed Tanimoto coefficient. Clustering was performed using ChemMine and visualized using Dendroscope (Huson et al., 2007; Backman et al., 2011). Colors indicate major chemotypes. Note that the oxacephems: moxalactam and flomoxef, are colored purple. Bolded lactams marked with an asterisk indicate drugs with high allergy potential (>10%) relating to the R1 benzyl groups.
Monobactams fit in their own small cluster that branches from the cephem class. However, nocardicin has substituents with structural similarity to moxalactam and was positioned apart from other monobactams despite its single ring platform. β-lactamase inhibitors based on the core β-lactam structure (sulbactam, clavulanate, and tazobactam) make up their own cluster. Interestingly along with the penam mecillinam, members of this branch are highly related via their core chemistry (3,3-dimethyl-7-oxo-4-thia-1-azabicyclo[3.2.0]heptane-2-carboxylic acid, Figure 1 penam). Oxacephems (moxalactam and flomoxef) are uniquely located as branching off the cephem class.
While generational labeling is a common method for describing β-lactams, particularly cephems (cephalosporins), this convention is not always consistent among researchers or pharmaceutical companies. Using consensus information from multiple literature sources, the relevant drugs in the Lactamome were assigned colors according to a generational scheme (Figure 5B). However, the generational Lactamome did not reveal any apparent trends or relationships that signaled generation. In most cases, terminal clusters are the same generation or differ by only one generation. In the case of cephems, later generations exhibit a broader spectrum of activity and less cross-reactivity with penicillin (Trubiano et al., 2017). The carbapenem class, while important for multidrug resistant infections, does not readily conform to chemical generation labeling. Similarly, β-lactamase inhibitors, mecillinam, and monobactams do not conform to multigenerational binning.
Figure 5. Lactamomes depicting (A) chemotype, (B) compound generation, and (C) h-Woodward values. (A) The chemotype is a reproduction of Figure 4 to aid comparisons to the other figures. (B) The generational lactamome assigns generation levels (1st–5th) to individual drugs based on how they most currently referenced in literature. Individual drugs that do not conform to generational naming are colored in black. (C) h-Woodward lactamome is colored using a heatmap with higher h-Woodward values in green and lower h-Woodward values in red. Bolded lactams marked with an asterisk indicate drugs with high allergy (>10%) relating to the R1 benzyl groups.
While factors like affinity, site accumulation, and pharmacokinetics affect in vivo efficacy, the minimum inhibitory concentrations (MICs) of antibiotics correlate with those having higher h-Woodward values. Correspondingly, higher h-Woodward value drugs are more prone to hydrolysis, exhibiting shorter aqueous and biological half-lives. For each compound examined, a self-consistent field calculation with geometry optimization was carried out using the modified neglect of diatomic overlap (MNDO) algorithm. The height of the trigonal pyramid with the lactam nitrogen at its apex and the nitrogen’s three bonded substituents at its base (i.e., h-Woodward) was measured for the optimized structure. However, the trend is not perfect, for example, clavulanate, while among the most reactive of the β–lactams, exhibits poor antibacterial activity against many organisms.
When the Lactamome was colored according to h-Woodward, an interesting pattern emerged (Figure 5C). Monobactams, except for tigemonam, exhibited the lowest h values, most of the cephems exhibited relatively low h values, and the first-through-third generation penams occupied the middle range of Woodward height. Carbapenems and the oxapenem, clavulanate, with its additional ring strain from the unsaturation at C2, demonstrated the highest h-Woodward values of all of the β-lactams analyzed herein. Using the Woodward parameter as a measure of reactivity may therefore serve as a guide to the relative ease of hydrolytic decomposition of β-lactams, with monobactams representing the least susceptible.
Because the β-lactams are minimally metabolized in vivo, their serum half-lives are largely determined through primary renal clearance. As exceptions, the ureido class (e.g., cefoperazone, piperacillin) undergo significant biliary clearance. Lactams that are more susceptible to hydrolysis tend to have greater activity, but also exhibit shorter biological half-lives. For example, carbapenems and penams tend toward lower plasma stability than cephems. Hydrolytic instability is one of the main reasons that many β-lactam antimicrobials need to be administered parenterally, reconstituted at the bedside or refrigerated, and dosed on a strict and frequent schedule. Compounds possessing unique pharmacokinetic properties not representative of their class may have markedly divergent half-lives. For example, ceftriaxone, ertapenem, and temocillin exhibit extensive protein binding (t1/2 > 6 h), and both cephalothin and cefotaxime are rapidly converted to their desacetyl metabolites (t1/2 < 1 h). An interesting recent development involving the fermentation isolation of clavulanate focused heavily on the minimization of its hydrolysis to improve its yield during manufacturing (Veeraraghavan et al., 2021).
While short serum half-lives may present challenges during administration of β-lactams, the increased rate of degradation in vivo may reduce the risk of occupational and environmental exposure after excretion. Such susceptibility to hydrolysis ex vivo can be exploited by chemical processes to decontaminate residues during manufacturing, compounding, and administration, as well as by waste-water treatment facilities to further reduce environmental hazards.
While the optimal antibiotic strategy for patient care requires consideration of many factors, a primary criterion is the susceptibility of the infectious bacterial strain. Using The Sanford Guide to Antimicrobial Therapy (Gilbert et al., 2020), a “heat map” of susceptibility trends for β-lactam coverage by organism ultrastructure and metabolism was generated (Figure 6). When looking at the fractional activity against general classes of pathogenic bacteria, several specific patterns emerge. None of the β-lactams are particularly effective against cell wall-deficient microbes, which mostly include intracellular pathogens like mycoplasma and Chlamydia trachomatis. However, for infections typically caused by Gram-negative and Gram-positive bacteria, β-lactams present a myriad of options for good empiric or specific coverage. When comparing spectrum of activity versus h-Woodward values, a weak but correlative relationship is apparent. Beta-lactams with higher values generally exhibit broader spectrum of activity within and across groups of microbes than β-lactams with lower h-Woodward values. Notably, the monobactam aztreonam has the lowest h-Woodward value and a very narrow spectrum of activity. However, there are several exceptions, notably the relatively narrow spectrum of activity with nafcillin and oxacillin. As mentioned previously, this is due to the many factors affecting microbial killing, including site accumulation (particularly relevant in Gram-negative pathogens) and target affinity.
Figure 6. Antimicrobial spectra of β-lactams. Clinical activity data were taken from The Stanford Guide to Antimicrobial Therapy. A fractional coverage index was calculated using a weighted average of categorical coverage values reported. Heatmap color range is from green indicating high coverage and red indicating low coverage. Beta-lactams are ordered from highest h-Woodward value (ertapenem) to lowest (aztreonam).
Good antimicrobial stewardship requires the use of an antimicrobial with the narrowest possible spectrum, but which still provides a high likelihood of infection clearance. For this reason, clinicians need to think about the most likely pathogens involved in specific infectious processes to guide an appropriately targeted empiric therapy. Targeted therapy also highlights the sustained need for rapid, cost-effective, and accurate diagnostics. As an example, absent other risk factors, cellulitis is generally caused by S. pyogenes or methicillin-susceptible S. aureus (MSSA). This condition can be treated effectively with nafcillin, any of the oxacillin subclass, or a first-generation cephalosporin like cefazolin. Cefazolin is also a safe alternative for patients with nafcillin hypersensitivity (Gandhi et al., 2021). Coverage should be expanded only with good reason, including colonization with methicillin-resistant S. aureus (MRSA), open wounds, or specific exposures (e.g., Vibrio vulnificus with saltwater exposure or Aeromonas hydrophila with freshwater exposure). One of the key deficiencies of the β-lactam class is its minimal activity against atypical and intracellular pathogens, as they are more resistant to alterations in peptidoglycan synthases. Non-lactam drugs–tetracyclines, macrolides, and fluoroquinolones–are likely more appropriate treatments for these microorganisms due to the variety of anti-infective mechanisms.
Special consideration should be given to infections with highly resistant organisms that have been verified with culture-based methods or nucleic acid amplification testing (NAAT). Conditions in which Pseudomonas aeruginosa is commonly implicated include burn wounds, healthcare-associated pneumonia, diabetic ulcers, and cystic fibrosis. For patients with these conditions, only a handful of β-lactam options exist, including ceftolozane-tazobactam, piperacillin-tazobactam, ceftazidime, cefepime, and carbapenems (except for ertapenem). Until the approval of ceftaroline, β-lactams were largely ineffective against MRSA; and were less commonly used than glycopeptide macrocyclic agents like vancomycin and daptomycin. Guidelines stipulate ceftaroline as a last-resort option for treatment of microbiologically proven cases of vancomycin-intermediate S. aureus (VISA, generally defined as vancomycin MIC ≥ 2 μg/mL) for which daptomycin cannot be used (e.g., lung infections). Ceftobiprole, though not yet approved in the United States, will likely occupy a similar niche.
Extended-spectrum β-lactamase (ESBL) producing strains of E. coli and Klebsiella spp. have become increasingly common in intra-abdominal infections as well as urinary tract infections. Carbapenems retain activity against these strains due to their remarkable β-lactamase stability, and newer agents employing diazabicyclooctane (DBO) β-lactamase inhibitors (e.g., ceftazidime-avibactam, imipenem-relebactam, and aztreonam-avibactam, which is still in development) also are effective. Carbapenemase-producing Gram-negative enterics also have emerged, for which combinations of carbapenems with DBOs and boronic acids (e.g., imipenem-relebactam and meropenem-vaborbactam) remain clinically efficacious. As with all infectious treatments the spectrum, dose, route of administration, and drug allergy should be carefully considered.
Streptococcus pyogenes (also known as or Group A Streptococcus or GAS) is the causative pathogen in several human diseases, including pharyngitis, scarlet fever, and necrotizing fasciitis. The susceptibility of this organism to β-lactams has remained remarkably consistent over many decades although there are recent indications that mutations in the gene encoding a PBP are widespread and can result in reduced susceptibility (Macris et al., 1998; Musser et al., 2020). However, given the historically consistent susceptibility, it was selected as a model organism to examine further the potential relationship between h-Woodward values and susceptibility to β-lactam antibiotics. The data were extracted from 95 different studies involving 10 strains of S. pyogenes (Wallmark, 1962; Adams, 1963; Sidell et al., 1963; Gravenkemper et al., 1965; Thornhill et al., 1969; Bergeron et al., 1973; Eykyn et al., 1973, 1976; Neu, 1974b,1976; Shibata et al., 1975; Ernst et al., 1976; Goto, 1977; Stewart and Bodey, 1977; Bodey and Le Blanc, 1978; Chabbert and Lutz, 1978; Verbist, 1978; Wise et al., 1978, 1980, 1988, 1991; Fass, 1979, 1990; Kamimura et al., 1979, 1984; Neu et al., 1979, 1981, 1984, 1989; Watanakunakorn and Glotzbecker, 1979; Weaver et al., 1979; Angehrn et al., 1980; Fu and Neu, 1980; Greenwood et al., 1980; Hinkle et al., 1980; Baker et al., 1981; Bodey et al., 1981, 1985; Cherubin et al., 1981; Eickhoff and Ehret, 1981; Istre et al., 1981; Jones et al., 1981, 1984; Stamm et al., 1981; Tsuchiya et al., 1981; Ahonkhai et al., 1982; Fainstein et al., 1982; Scully et al., 1983; Kessler et al., 1985; Toda et al., 1985; Une et al., 1985; Vuye and Pijck, 1985; Neu and Chin, 1986; Okamoto et al., 1987; Mine et al., 1988; Shelton and Nelson, 1988; Kayser et al., 1989; Yu and Neu, 1989; Arisawa et al., 1991; Chin et al., 1991, 1992; Emberton and Finlay, 1991; Limbert et al., 1991; Poch et al., 1992; Yan et al., 1993; Coonan and Kaplan, 1994; Tsuji et al., 1995, 1998; Suzuki et al., 1996; Cocuzza et al., 1997; Woodcock et al., 1997; Fontana et al., 1998; Kriebernegg et al., 1998; Sakagawa et al., 1998; Yamaguchi et al., 1998; Fuchs et al., 2001; Livermore et al., 2001; Pendland et al., 2002; Iizawa et al., 2004; Marchese et al., 2004; Lev et al., 2005; Stefani et al., 2008; Tempera et al., 2010; Camara et al., 2013; Fujimoto et al., 2013; Sakata, 2013; Yang et al., 2015; Yanik et al., 2015; Cotroneo et al., 2020; Jean et al., 2020; Musser et al., 2020; Ubukata et al., 2020; Yanagihara et al., 2020).
As seen in the upper portion of Figure 7, β-lactams with higher h-Woodward values are the most potent options against S. pyogenes. Drugs with the lowest minimum inhibitory concentration values against 50% of the population (MIC50) also exhibit the lowest variation among different strains. Median susceptibility decreases (increased MIC50) at intermediate and higher h-Woodward values, although the overall trend suggests a parabolic relationship where drugs with intermediate h-Woodward values exhibit the lowest potency. Taken by class, penems are generally more biologically active than penams (0.007 vs. 0.024 μg/mL), and both exhibit greater efficacy than cephems (0.074 μg/ml). Aztreonam, the lone monobactam in use, was found to exhibit the lowest efficacy against S. pyogenes with a mean MIC50 of 16.5 μg/ml. The results of this analysis align with the previous observations related to the heat map, previously presented as Figure 6, that h-Woodward values impact antibacterial activity both within strains of S. pyogenes and across different groups of bacteria.
Figure 7. In vitro antimicrobial activity of lactams against S. pyogenes. A review of the literature on Gram-positive β-lactam activity was conducted in which susceptibility data were gleaned from 95 studies reporting data for at least 10 strains. MIC50 (presented as log values) were amassed, and geometric mean and geometric standard deviation were calculated. Geometric means for entire classes (e.g., penems) are shown in gray. Beta-lactams are ordered from highest h-Woodward value (ertapenem) to lowest (aztreonam).
Hypersensitivity to β-lactams is among the most commonly reported drug allergies, representing 42.6% of all drug-induced anaphylaxis (Zagursky and Pichichero, 2018). However, prevalence is likely over-reported due to misinterpretation of mild drug reactions (e.g., diarrhea, mild drug rash, attribution of illness symptoms to drug). In fact, many individuals with reported β-lactam allergy can tolerate β-lactams due to this misattribution, and many of those with remote history of true allergic reaction are sometimes able to tolerate the drugs years later due to waning sensitivity (Romano et al., 2014). Even so, β-lactam allergy remains a clinically relevant problem, with skin testing generally yielding confirmed rates around 8% (3–10%) for penicillins and 1% (1–2%) for cephalosporins. Hypersensitivity to carbapenems and monobactams is rarer.
Allergy is a result of immune activation in response to specific β-lactam substituents (Figure 1 and Supplementary Spreadsheet). The 2-azetidinone ring acts as a hapten, covalently modifying nucleophilic residues on host proteins to elicit an IgE response in individuals with specific human leukocyte antigen allotypes (Figure 8). While this covalently bound β-lactamoyl protein adduct is the major determinant of hypersensitivity, hydrolyzed small molecule products also can elicit some response.
Beta-lactam allergy is an immunologic type I hypersensitivity reaction, meaning that it occurs through mast cell degranulation in response to preformed IgE binding antigen recognition receptors (FcRε) on the mast cell surface. Subsequent release of histamine results in symptoms related to vasodilation (warmth and erythema), increased capillary permeability (edema), bronchoconstriction (dyspnea), and nociceptive stimulation (pain and pruritus). These symptoms are potentiated by the release of inflammatory eicosanoid derivatives, including prostaglandin D2, leukotriene B4, and leukotriene C4. In mild cases, this manifests as hives or wheezing, but in patients with high IgE titers, the reaction may proceed to anaphylaxis or anaphylactic shock. Because of the potential severity of the reaction, in addition to the poor accuracy of self-reporting mentioned above, identification of patients with true allergy through skin testing or measuring IgE against β-lactam products is critical to avoiding significant morbidity and mortality.
While not part of the group of IgE-mediated allergic syndrome, other significant immune reactions to the β-lactam class exist as well that are thought to occur through parallel mechanisms. Drug-induced immune hemolytic anemia (DIIHA) is a type II hypersensitivity reaction mediated by direct binding of IgG to erythrocytes, seen with relatively high frequency in β-lactam-treated subjects. One longitudinal study, documenting causes of DIIHA in Southern California over 30 years estimates that over 80% of cases are due to β-lactams (Garratty, 2009). Eosinophilic reactions are common as well, including drug-induced hypersensitivity syndrome (DiHS) with multiorgan involvement (previously known as drug reaction with eosinophilia and systemic symptoms or DRESS) caused by covalent modification of host proteins. Mucocutaneous eruption syndromes with epidermal detachment are also possible, arising from the activation of cytotoxic T-cells against keratinocytes.
There is a growing body of evidence suggesting the substituent adjacent to the lactam carbonyl (Figure 1), referred to as R1, is the primary driver of immunogenicity. Indeed, immunologic cross-reactivity is most often seen against structurally similar R1 groups of penams and cephems (i.e., ampicillin and cephalexin). One of the most prominent β-lactams associated with allergy is cefamandole, whose R1 is a hydroxybenzyl with a terminal aromatic. This structural relationship is not only common to such compounds as cephalexin (possessing an aminobenzyl moiety) but also to cephalothin, whose R1 aromatic is a thiophene, and cefuroxime whose R1 aromatic is a furan. These compounds, therefore, possess similar electronic and steric properties, or bioisosteric profiles. Such end group topology and electronic properties may play determinative roles in allergen character, possibly due to three-dimensional shape (“lock and key”) factors.
Compounds with 10% allergy response and containing similar aromatic R1 end groups have been bolded and tagged with asterisks in Figure 4. One careful study recommends skin reactivity testing for both penicillin and cephalosporin as 11% displayed positive skin test responses to the cephalosporin class, and 64% of cross-reactive patients responded negatively to cefamandole (Romano et al., 2004). In another study of 252 penicillin-allergic patients, ∼39% of patients treated with the cephalosporins cefaclor, cephalexin, cefadroxil, or cefamandole had an allergic response (Romano et al., 2018). Both studies show low cross-reactivity between penicillins and later-generation cephalosporins, such as ceftriaxone (∼3%). R1 groups containing phenyloxazole and piperazine also account for ∼3% of allergies. In cases where a patient may react in tests to dissimilar β-lactams, it is likely not due to true cross-reactivity, but rather to independent sensitivity to each drug. The low rate of cross-reactivity seen between penicillin and carbapenems (<1%), which possess a unique hydroxyethyl side chain, further supports the hypothesis that not only does the R1 bioisosterism play a dominant role, but aromatic end group chemistry appears causative (Romano et al., 2006, 2007; Atanaskovic-Markovic et al., 2008; Gaeta et al., 2015; Buonomo et al., 2016). Although not utilized on their own as an antibacterial therapy, β-lactam based β-lactam inhibitors also have been implicated in allergic reactions (Stover et al., 2019). The authors concluded that cross-allergenicity with β-lactams is likely with sulbactam and tazobactam. Cross-allergenicity is less likely with clavulanate, but still possible.
The involvement of the R2 side group in allergic response is the subject of greater uncertainty. While structural similarity of R2 has been considered as a possible factor, this group is frequently lost during ring opening, and any role it plays is generally quite small in comparison to the R1 side group. In the context of a β-lactam allergy, clinicians often look to different antimicrobial classes as alternatives. However, if the agent to which the patient has had an immune reaction is known, they may be able to use β-lactams of similar spectrum but differing chemical structures, both with regard to class and R1. For example, if a patient has had a severe reaction to amoxicillin in the past, cefpodoxime proxetil (a prodrug formulation) may be a suitable oral alternative due to its differently structured R1.
Similarly, clinicians often cite an allergy to penicillin G or amoxicillin as reason to avoid other penams, occasionally utilizing drugs of last defense when they might not be needed. As a clinical example, piperacillin-tazobactam is frequently avoided in the setting of a penicillin G allergy in favor of cefepime for similar Gram-negative enteric and pseudomonads coverage. Many clinicians, wary of cross-reactivity, will even avoid the cephalosporin class and prescribe a carbapenem. However, penicillin allergy may not preclude the use of piperacillin-tazobactam, as the benzyl functionality of penicillin G, to which the reaction likely occurred, is structurally very different from the R1 groups of cefepime or nitrogen-substituted R1 of piperacillin. For this reason, it is critical to keep accurate record of the β-lactam-based drugs to which the patient has reacted, as well as the type and severity of said reaction, so as to determine which other agents they are not likely to tolerate.
Although β-lactam antibiotics do not meet the criteria for hazardous drugs established by the National Institute of Occupational Safety and Health (NIOSH), manufacturing and compounding of these drugs requires attention to reduce the risk of cross-contamination or occupational exposure to sensitive individuals. Current good manufacturing practice (cGMP) regulations require the use of methods to address cross-contamination [e.g., Title 21, Sections 211.42(d), 211.46(d), and 211.176 of the Code of Federal Regulations]. To further assist manufacturers, the FDA issued a draft guideline in 2013 entitled “Non-Penicillin Beta-Lactam Drugs: A cGMP Framework for Preventing Cross-Contamination” (Food and Drug Administration [FDA], 2013). Although most compounding pharmacies are 503a-type facilities and not required to operate under cGMP, the FDA often applies the described guidelines to pharmacy compounders. The guidelines are quite restrictive, including requiring segregation of “facilities” for “operations relating to the manufacture, processing, and packing” of β-lactam antibiotics. Additionally, an updated guidance document regarding insanitary conditions at compounding facilities was issued in November 2020 (Food and Drug Administration [FDA], 2020). It lists several examples of insanitary conditions applicable to the production of sterile and non-sterile drug, including: “Processing of β-lactams without complete and comprehensive separation from non-β-lactam products.” While segregation may not be absolutely required (or feasible) for all compounding activities, it is important to reduce the risk of cross-contamination through effective decontamination of surfaces and equipment after manipulations involving β-lactam antibiotics.
Another concern involves the environmental persistence of antibiotics, including β-lactams, in the environment. Antibiotics used to treat humans and livestock can escape into the environment by several routes, including improper disposal and excretion through urine and feces (Kivits et al., 2018; Szekeres et al., 2018). Conventional waste-water treatment processes are not effective at removing or destroying these drugs (Song et al., 2019). Persistence of these drugs in the environment can therefore result in selective pressures on environmental bacteria, leading to development of resistance genes which then may be transferred to human or animal pathogens (Bengtsson-Palme et al., 2018). β-lactams with intermediate or high h-Woodward values are more susceptible to decontamination via alkaline or acid hydrolysis at the 2-azetidinone ring (Lang et al., 1978). The kinetics of inactivation by such processes are related to the extent of ring activation. Thus, cephems tend to be more resistant to degradation, followed by penams, while carbapenems are the most susceptible.
While further studies are needed, there is evidence that oxidizing agents may decontaminate β-lactam antibiotics (Lorcheim, 2011; He et al., 2014; Zhang et al., 2017). These chemicals commonly are used for disinfection of surfaces contaminated with β-lactam antibiotics as well as in waste-water treatment systems. Additional research should establish how oxidizers such as peracetic acid, hydrogen peroxide, and hypochlorous acid would perform as decontamination strategies within the four major classes of β-lactam antibiotics, and whether such oxidants would differ in efficacy by pH and h-Woodward.
β-lactams remain an important and highly utilized class of antibiotics. In the outpatient setting, amoxicillin and cephalexin are consistently among the most prescribed drugs in the United States, and ceftriaxone and piperacillin-tazobactam are staples of empiric inpatient treatment. Over their decades of prominence, however, concerns have arisen among clinicians and laboratories regarding their efficacy and environmental persistence. Among these concerns are serious allergic responses and acquisition of drug resistance by environmental bacteria.
While non-β-lactam antibiotics have gained increased attention, the utility of β-lactam antibiotics remains important, not only in the human clinical situation but also in a wide array of veterinary uses. The current 5th generation β-lactam are undergoing clinical trials and new β-lactams are expected to see less development, as non-β-lactam antibiotics, and combinations of β-lactams with lactamase inhibitors take center stage (Figure 5B). The current work structurally classified the β-lactam class of antimicrobials to aid clinical decision making, as well as inform patient, worker, and environmental safety. We have organized our structural analysis into an easily visualized tool, the Lactamome (Figure 4), which can be colorized according to several chemical properties, including overall molecular shape (Figures 3, 5A) and propensity for reaction with nucleophiles, i.e., h-Woodward (Figure 3). It should also be noted that when colored by allergic potential, one would obtain a chemogram strongly resembling Figure 5C, depicting h-Woodward.
The h-Woodward colored Lactamome (Figure 5C) indicates that higher values for penems and penams (Figures 2, 5) would be the most susceptible to chemically catalyzed degradation. This greater propensity for hydrolysis is important in reducing occupational exposures, especially given that the highest rate of reported β-lactam hypersensitivity is to the penam class. Logically, more stringent cleaning is needed if a more allergy-prone lactam is used, but fortunately, the penams’ high h-Woodward values indicate decontamination protocols involving hydrolysis should be efficient. In the case of cephems, while their overall rate of allergenicity is somewhat lower than that of penams, many first-generation agents from this subclass have high structural similarity to the first-generation penams (e.g., penicillin and cephalexin) and are, therefore, more likely to be allergens (Figure 5B). Therefore, care should be taken in the decontamination of surfaces or areas exposed to those cephems with a higher possibility for cross-reactivity.
Antibacterial efficacy also appears to trend with h-Woodward values. Drugs with higher h-Woodward values tend to have lower MICs against S. pyogenes and activity against a broader spectrum of bacteria (Figure 7). However, given the multitude of mechanisms involved with susceptibility across different species and types of bacteria, it is not clear if h-Woodward values would be a reliable criterion for matching β-lactams with particular pathogens (Figure 6). It is apparent that the “generational” nomenclature is a function of product development over time and generally is not well correlated with molecular structure or activity.
Unlike the correlation of h-Woodward values with both high intrinsic potency and high hydrolysis potential, allergenicity is impacted by additional factors, including the position of the R1 group (Figure 8). In terms of selecting alternate β-lactams due to allergy, the Lactamome, when used in combination with the spectrum data (Figure 6) provides information for treatment options. These analyses also may guide future studies of efficacy to broaden recommended uses of known β-lactams. For example, if a patient with spontaneous bacterial peritonitis has a documented anaphylactic response to empiric cefotaxime, they are likely to have an adverse reaction to structurally similar ceftriaxone as well. In these situations, guidelines suggest a fluoroquinolone as the next suitable option. Using the structure and spectrum data presented, however, one might consider ampicillin-sulbactam, a decision for which positive data exists but a full-scale prospective non-inferiority trial has not been conducted (Guo et al., 2019).
The structural characterization presented in this study may provide a helpful guide to selecting antibiotic agents, especially for persons having certain allergic response histories, as well as to inform proper decontamination methods. The Lactamome may assist clinicians in assessing structural similarity and therefore help recognize, relatively speaking, which drug would be most effective, but exhibit lower risks of clinical cross-sensitivity. By first grouping the β-lactam antibiotics and then using coloring schemes, it is possible to quickly probe the entire genus. The Lactamome should also be useful in alerting practitioners to potential workplace hazards and assist in the development of decontamination strategies to reduce the risk of cross-contamination, occupational exposure, and environmental persistence.
All authors conceived and wrote the manuscript together. JT generated the spectrum and activity graphs. YP and AM generated the lactamome chemograms.
We would like to thank Contec, Inc. and South Carolina Research Authority for financial support.
This study received funding from Contec, Inc. The funder Contec, Inc. had the following involvement with the study: LP and MW helped conceive of the analysis, aided in analysis, co-wrote the manuscript, helped with graphical design, and aided in revisions.
LP and MW were employed by Contec, Inc. MB was employed by Intramed Plus.
The remaining authors declare that the research was conducted in the absence of any commercial or financial relationships that could be construed as a potential conflict of interest.
All claims expressed in this article are solely those of the authors and do not necessarily represent those of their affiliated organizations, or those of the publisher, the editors and the reviewers. Any product that may be evaluated in this article, or claim that may be made by its manufacturer, is not guaranteed or endorsed by the publisher.
The Supplementary Material for this article can be found online at: https://www.frontiersin.org/articles/10.3389/fmicb.2022.807955/full#supplementary-material
Abrahamsson, S., Hodgkin, D. C., and Maslen, E. N. (1963). The crystal structure of phenoxymethylpenicillin. Biochem. J. 86, 514–535. doi: 10.1042/bj0860514
Adams, C. W. (1963). Multiple factors in the pathogenesis of atherosclerosis. Guys Hosp. Rep. 112, 222–253.
Ahonkhai, V. I., Cherubin, C. E., and Shulman, M. A. (1982). In vitro activity of cefodizime (HR-221). Antimicrob. Agents Chemother. 22, 715–718. doi: 10.1128/aac.22.4.715
Aldridge, S., Parascandola, J., Sturchio, J. L., and American Chemical Society, and Royal Society of Chemistry (1999). The Discovery and Development of Penicillin 1928-1945. Washington, DC: American Chemical Society.
Angehrn, P., Probst, P. J., Reiner, R., and Then, R. L. (1980). Ro 13-9904, a long-acting broad-spectrum cephalosporin: in vitro and in vivo studies. Antimicrob. Agents Chemother. 18, 913–921. doi: 10.1128/aac.18.6.913
Aoki, T., Yoshizawa, H., Yamawaki, K., Yokoo, K., Sato, J., Hisakawa, S., et al. (2018). Cefiderocol (S-649266), a new siderophore cephalosporin exhibiting potent activities against Pseudomonas aeruginosa and other gram-negative pathogens including multi-drug resistant bacteria: structure activity relationship. Eur. J. Med. Chem. 155, 847–868. doi: 10.1016/j.ejmech.2018.06.014
Arisawa, M., Sekine, Y., Shimizu, S., Takano, H., Angehrn, P., and Then, R. L. (1991). In vitro and in vivo evaluation of Ro 09-1428, a new parenteral cephalosporin with high antipseudomonal activity. Antimicrob. Agents Chemother. 35, 653–659. doi: 10.1128/aac.35.4.653
Asai, M., Haibara, K., Muroi, M., Kintaka, K., and Kishi, T. (1981). Sulfazecin, a novel beta-lactam antibiotic of bacterial origin. Isolation and chemical characterization. J. Antibiot. 34, 621–627. doi: 10.7164/antibiotics.34.621
Atanaskovic-Markovic, M., Gaeta, F., Medjo, B., Viola, M., Nestorovic, B., and Romano, A. (2008). Tolerability of meropenem in children with IgE-mediated hypersensitivity to penicillins. Allergy 63, 237–240. doi: 10.1111/j.1398-9995.2007.01532.x
Backman, T. W., Cao, Y., and Girke, T. (2011). ChemMine tools: an online service for analyzing and clustering small molecules. Nucleic Acids Res. 39, W486–W491. doi: 10.1093/nar/gkr320
Baker, C. N., Thornsberry, C., and Facklam, R. R. (1981). Synergism, killing kinetics, and antimicrobial susceptibility of group A and B streptococci. Antimicrob. Agents Chemother. 19, 716–725. doi: 10.1128/aac.19.5.716
Basker, M. J., Boon, R. J., and Hunter, P. A. (1980). Comparative antibacterial properties in vitro of seven olivanic acid derivatives: MM 4550, MM 13902, MM 17880, MM 22380, MM 22381, MM 22382 and MM 22383. J. Antibiot. 33, 878–884. doi: 10.7164/antibiotics.33.878
Bassetti, M., Giacobbe, D. R., Castaldo, N., Russo, A., and Vena, A. (2021). Role of new antibiotics in extended-spectrum beta- lactamase-, AmpC- infections. Curr. Opin. Infect. Dis. 34, 748–755. doi: 10.1097/QCO.0000000000000789
Batchelder, H. R., Story-Roller, E., Lloyd, E. P., Kaushik, A., Bigelow, K. M., Maggioncalda, E. C., et al. (2020). Development of a penem antibiotic against Mycobacteroides abscessus. Commun. Biol. 3:741. doi: 10.1038/s42003-020-01475-2
Bengtsson-Palme, J., Kristiansson, E., and Larsson, D. G. J. (2018). Environmental factors influencing the development and spread of antibiotic resistance. FEMS Microbiol. Rev. 42:fux053. doi: 10.1093/femsre/fux053
Bergeron, M. G., Brusch, J. L., Barza, M., and Weinstein, L. (1973). Bactericidal activity and pharmacology of cefazolin. Antimicrob. Agents Chemother. 4, 396–401. doi: 10.1128/aac.4.4.396
Bhatnagar, A., Ransom, E. M., Machado, M. J., Boyd, S., Reese, N., Anderson, K., et al. (2021). Assessing the in vitro impact of ceftazidime on aztreonam/avibactam susceptibility testing for highly resistant MBL-producing Enterobacterales. J. Antimicrob. Chemother. 76, 979–983. doi: 10.1093/jac/dkaa531
Bodey, G. P., Fainstein, V., and Hinkle, A. M. (1981). Comparative in vitro study in new cephalosporins. Antimicrob. Agents Chemother. 20, 226–230. doi: 10.1128/aac.20.2.226
Bodey, G. P., Ho, D. H., and LeBlanc, B. (1985). In vitro studies of BMY-28142, a new broad-spectrum cephalosporin. Antimicrob. Agents Chemother. 27, 265–269. doi: 10.1128/aac.27.2.265
Bodey, G. P., and Le Blanc, B. (1978). Piperacillin: in vitro evaluation. Antimicrob. Agents Chemother. 14, 78–87. doi: 10.1128/aac.14.1.78
Boyd, D. B. (1984). Electronic structures of cephalosporins and penicillins. 15. Inductive effect of the 3-position side chain in cephalosporins. J. Med. Chem. 27, 63–66. doi: 10.1021/jm00367a012
Boyd, D. B., Hermann, R. B., Presti, D. E., and Marsh, M. M. (1975). Electronic structures of cephalosporins and penicillins. 4. Modeling acylation by the beta-lactam ring. J. Med. Chem. 18, 408–417. doi: 10.1021/jm00238a018
Buonomo, A., Pascolini, L., Rizzi, A., Aruanno, A., Pecora, V., Ricci, A. G., et al. (2016). Cross-reactivity and tolerability of ertapenem in patients with IgE-mediated hypersensitivity to beta-lactams. J. Investig. Allergol. Clin. Immunol. 26, 100–105. doi: 10.18176/jiaci.0019
Bush, K., and Bradford, P. A. (2016). beta-lactams and beta-lactamase inhibitors: an overview. Cold Spring Harb. Perspect. Med. 6:a025247. doi: 10.1101/cshperspect.a025247
Butler, M. S., Gigante, V., Sati, H., Paulin, S., Al-Sulaiman, L., Rex, J. H., et al. (2022). Analysis of the clinical pipeline of treatments for drug resistant bacterial infections: despite progress, more action is needed. Antimicrob. Agents Chemother. doi: 10.1128/AAC.01991-21 [Online ahead of print].
Camara, M., Dieng, A., and Boye, C. S. (2013). Antibiotic susceptibility of Streptococcus pyogenes isolated from respiratory tract infections in dakar, senegal. Microbiol. Insights 6, 71–75. doi: 10.4137/MBI.S12996
Cazzola, M., Brancaccio, V., De Giglio, C., Paterno, E., Matera, M. G., and Rossi, F. (1993). Flomoxef, a new oxacephem antibiotic, does not cause hemostatic defects. Int. J. Clin. Pharmacol. Ther. Toxicol. 31, 148–152.
Chabbert, Y. A., and Lutz, A. J. (1978). HR 756, the syn isomer of a new methoxyimino cephalosporin with unusual antibacterial activity. Antimicrob. Agents Chemother. 14, 749–754. doi: 10.1128/aac.14.5.749
Cherubin, C. E., Corrado, M. L., Sierra, M. F., Gombert, M. E., and Shulman, M. (1981). Susceptibility of gram-positive cocci to various antibiotics, including cefotaxime, moxalactam, and N-formimidoyl thienamycin. Antimicrob. Agents Chemother. 20, 553–555. doi: 10.1128/aac.20.4.553
Chin, N. X., Gu, J. W., Fang, W., and Neu, H. C. (1991). In vitro activity and beta-lactamase stability of GR69153, a new long-acting cephalosporin. Antimicrob. Agents Chemother. 35, 259–266. doi: 10.1128/aac.35.2.259
Chin, N. X., Gu, J. W., Fang, W., and Neu, H. C. (1992). In vitro activity of cefquinome, a new cephalosporin, compared with other cephalosporin antibiotics. Diagn. Microbiol. Infect. Dis. 15, 331–337. doi: 10.1016/0732-8893(92)90019-p
Cocuzza, C., Blandino, G., Mattina, R., Nicoletti, F., and Nicoletti, G. (1997). Antibiotic susceptibility of group a streptococci in 2 Italian cities: Milano and Catania. Microb. Drug Resist. 3, 379–384. doi: 10.1089/mdr.1997.3.379
Coonan, K. M., and Kaplan, E. L. (1994). In vitro susceptibility of recent North American group a streptococcal isolates to eleven oral antibiotics. Pediatr. Infect. Dis. J. 13, 630–635. doi: 10.1097/00006454-199407000-00009
Cotroneo, N., Rubio, A., Critchley, I. A., Pillar, C., and Pucci, M. J. (2020). In Vitro and In Vivo characterization of tebipenem, an oral carbapenem. Antimicrob. Agents Chemother. 64:e02240-19. doi: 10.1128/AAC.02240-19
Decuyper, L., Jukic, M., Sosic, I., Zula, A., D’Hooghe, M., and Gobec, S. (2018). Antibacterial and beta-lactamase inhibitory activity of monocyclic beta-lactams. Med. Res. Rev. 38, 426–503. doi: 10.1002/med.21443
Dexter, D. D., and van der Veen, J. M. (1978). Conformations of penicillin G: crystal structure of procaine penicillin G monohydrate and a refinement of the structure of potassium penicillin G. J. Chem. Soc. Perkin 1, 185–190. doi: 10.1039/p19780000185
Diaz, N., Suarez, D., Sordo, T. L., Tunon, I., and Silla, E. (2002). Water-assisted alkaline hydrolysis of monobactams: a theoretical study. Chemistry 8, 859–867.
Dobias, J., Denervaud-Tendon, V., Poirel, L., and Nordmann, P. (2017). Activity of the novel siderophore cephalosporin cefiderocol against multidrug-resistant Gram-negative pathogens. Eur. J. Clin. Microbiol. Infect. Dis. 36, 2319–2327. doi: 10.1007/s10096-017-3063-z
Eagle, H. (1946). The relative activity of penicillins F, G, K, and X against spirochetes and streptococci in vitro. J. Bacteriol. 52, 81–88. doi: 10.1128/JB.52.1.81-88.1946
Eagle, H., and Technical Assistance of Arlyne D. Musselman (1947). The therapeutic activity of penicillins F, G, K, and X in experimental infections with pneumococcus type I and Streptococcus pyogenes. J. Exp. Med. 85, 175–186. doi: 10.1084/jem.85.2.175
Eickhoff, T. C., and Ehret, J. (1981). Comparative in vitro studies of Ro 13-9904, a new cephalosporin derivative. Antimicrob. Agents Chemother. 19, 435–442. doi: 10.1128/aac.19.3.435
Emberton, P., and Finlay, D. B. (1991). Normal roentgen variant simulating disease: coronal suture overlying maxilla simulating fracture. Clin. Radiol. 43:217. doi: 10.1016/s0009-9260(05)80485-4
Entenza, J. M., Hohl, P., Heinze-Krauss, I., Glauser, M. P., and Moreillon, P. (2002). BAL9141, a novel extended-spectrum cephalosporin active against methicillin-resistant Staphylococcus aureus in treatment of experimental endocarditis. Antimicrob. Agents Chemother. 46, 171–177. doi: 10.1128/aac.46.1.171-177.2002
Ernest, I., Gosteli, J., Greengrass, C. W., Holick, W., and Woodward, R. B. (1978). The penems, a new class of beta-lactam antibiotics: 6-acylaminopenem-3-carboxylic acids. J. Am. Chem. Soc. 100, 8214–8222. doi: 10.1021/ja00494a032
Ernst, E. C., Berger, S., Barza, M., Jacobus, N. V., and Tally, F. P. (1976). Activity of cefamandole and other cephalosporins against aerobic and anaerobic bacteria. Antimicrob. Agents Chemother. 9, 852–855. doi: 10.1128/aac.9.5.852
Eykyn, S., Jenkins, C., King, A., and Phillips, I. (1973). Antibacterial activity of cefamandole, a new cephalosporin antibiotic, compared with that of cephaloridine, cephalothin, and cephalexin. Antimicrob. Agents Chemother. 3, 657–661. doi: 10.1128/aac.3.6.657
Eykyn, S., Jenkins, C., King, A., and Phillips, I. (1976). Antibacterial activity of cefuroxime, a new cephalosporin antibiotic, compared with that of cephaloridine, cephalothin, and cefamandole. Antimicrob. Agents Chemother. 9, 690–695. doi: 10.1128/aac.9.4.690
Fainstein, V., Weaver, S., and Bodey, G. P. (1982). Comparative in vitro study of SQ26, 776. Antimicrob. Agents Chemother. 21, 294–298. doi: 10.1128/aac.21.2.294
Fass, R. J. (1979). In vitro activity of LY127935. Antimicrob. Agents Chemother. 16, 503–509. doi: 10.1128/aac.16.4.503
Fass, R. J. (1990). In vitro activity of BAY v 3522, a new oral cephalosporin. Antimicrob. Agents Chemother. 34, 1855–1857. doi: 10.1128/aac.34.9.1855
Fleming, A. (2001). On the antibacterial action of cultures of a penicillium, with special reference to their use in the isolation of B. influenzae. 1929. Bull. World Health Organ. 79, 780–790.
Fontana, R., Altamura, M., Arcamone, F., Mazzariol, A., Morandotti, G., Sperning, R., et al. (1998). MEN 10700, a new penem antibiotic: in-vitro activity and its correlation with beta-lactamase stability, PBP affinity and diffusion through the bacterial cell wall. J. Antimicrob. Chemother. 41, 513–525. doi: 10.1093/jac/41.5.513
Food and Drug Administration [FDA] (2013). Non-Penicillin Beta-Lactam Drugs: A CGMP Framework for Preventing Cross-Contamination, ed. CDER (Silver Spring, MD: Food and Drug Administration).
Food and Drug Administration [FDA] (2020). Insanitary Conditions at Compounding Facilities - Guidance for Industry, ed. CDER (Silver Spring, MD: Food Drug Administration).
Foye, W. O., Lemke, T. L., and Williams, D. A. (2008). Foye’s Principles of Medicinal Chemistry. Philadelphia, PA: Lippincott Williams & Wilkins.
Frere, J. M., Ghuysen, J. M., and Iwatsubo, M. (1975). Kinetics of interaction between the exocellular DD-carboxypeptidase-transpeptidase from Streptomyces R61 and beta-lactam antibiotics. A choice of models. Eur. J. Biochem. 57, 343–351. doi: 10.1111/j.1432-1033.1975.tb02307.x
Fu, K. P., and Neu, H. C. (1980). Antibacterial activity of ceftizoxime, a beta-lactamase-stable cephalosporin. Antimicrob. Agents Chemother. 17, 583–590. doi: 10.1128/aac.17.4.583
Fuchs, P. C., Barry, A. L., and Brown, S. D. (2001). In vitro activities of ertapenem (MK-0826) against clinical bacterial isolates from 11 North American medical centers. Antimicrob. Agents Chemother. 45, 1915–1918. doi: 10.1128/AAC.45.6.1915-1918.2001
Fujimoto, K., Takemoto, K., Hatano, K., Nakai, T., Terashita, S., Matsumoto, M., et al. (2013). Novel carbapenem antibiotics for parenteral and oral applications: in vitro and in vivo activities of 2-aryl carbapenems and their pharmacokinetics in laboratory animals. Antimicrob. Agents Chemother. 57, 697–707. doi: 10.1128/AAC.01051-12
Gaeta, F., Valluzzi, R. L., Alonzi, C., Maggioletti, M., Caruso, C., and Romano, A. (2015). Tolerability of aztreonam and carbapenems in patients with IgE-mediated hypersensitivity to penicillins. J. Allergy Clin. Immunol. 135, 972–976. doi: 10.1016/j.jaci.2014.10.011
Gandhi, A. M., Shah, M. D., Donohue, L. E., Cox, H. L., and Eby, J. C. (2021). Tolerability of cefazolin in nafcillin-intolerant patients for the treatment of methicillin-susceptible Staphylococcus aureus infections. Clin. Infect. Dis. 73, 1650–1655. doi: 10.1093/cid/ciab368
Garratty, G. (2009). Drug-induced immune hemolytic anemia. Hematology Am. Soc. Hematol. Educ. Program 2009, 73–79. doi: 10.1182/asheducation-2009.1.73
Georgopapadakou, N. H., Smith, S. A., and Sykes, R. B. (1982). Mode of action of azthreonam. Antimicrob. Agents Chemother. 21, 950–956. doi: 10.1128/aac.21.6.950
Gilbert, D. N., Chambers, H. F., Saag, M. S., Pavia, A. T., Black, D., Boucher, H. W., et al. (2020). The Sanford Guide to Antimicrobial Therapy 2020. Sperryville, VA: Antimicrobial Therapy, Inc.
Glover, S. A., and Rosser, A. A. (2012). Reliable determination of amidicity in acyclic amides and lactams. J. Org. Chem. 77, 5492–5502. doi: 10.1021/jo300347k
Godzeski, C. W., Brier, G., and Pavey, D. E. (1963). Cephalothin, a new cephalosporin with a broad antibacterial spectrum. I. In vitro studies employing the gradient plate technique. Appl. Microbiol. 11, 122–127.
Goffin, C., and Ghuysen, J. M. (1998). Multimodular penicillin-binding proteins: an enigmatic family of orthologs and paralogs. Microbiol. Mol. Biol. Rev. 62, 1079–1093.
Goldberg, J. A., Kumar, V., Spencer, E. J., Hoyer, D., Marshall, S. H., Hujer, A. M., et al. (2021). A gamma-lactam siderophore antibiotic effective against multidrug-resistant Pseudomonas aeruginosa, Klebsiella pneumoniae, and Acinetobacter spp. Eur. J. Med. Chem. 220:113436. doi: 10.1016/j.ejmech.2021.113436
Goto, S. (1977). The in vitro and in vivo antibacterial activity of cefuroxime. Proc. R. Soc. Med. 70, (Suppl. 9), 56–62.
Gravenkemper, C. F., Bennett, J. V., Brodie, J. L., and Kirby, W. M. (1965). Dicloxacillin. In Vitro and pharmacologic comparisons with Oxacillin and Cloxacillin. Arch. Intern. Med. 116, 340–345. doi: 10.1001/archinte.1965.03870030020005
Graves-Woodward, K., and Pratt, R. F. (1998). Reaction of soluble penicillin-binding protein 2a of methicillin-resistant Staphylococcus aureus with beta-lactams and acyclic substrates: kinetics in homogeneous solution. Biochem. J. 332(Pt 3), 755–761. doi: 10.1042/bj3320755
Green, G. F., Page, J. E., and Staniforth, S. E. (1965). Cephalosporanic acids. I. Infrared absorption and proton magnetic resonance spectra of cephalosporin and penicillin analogues. J. Chem. Soc. 65, 1595–1605. doi: 10.1039/jr9650001595
Greenwood, D. (2008). Antimicrobial Drugs: Chronicle of a Twentieth Century Medical Triumph. Oxford: Oxford University Press.
Greenwood, D., Pearson, N., Eley, A., and O’Grady, F. (1980). Comparative in vitro activities of cefotaxime and ceftizoxime (FK749): new cephalosporins with exceptional potency. Antimicrob. Agents Chemother. 17, 397–401. doi: 10.1128/aac.17.3.397
Guo, J., Shi, J., Wang, H., Chen, H., Liu, S., Li, J., et al. (2019). Emerging Gram-positive bacteria and drug resistance in cirrhosis patients with spontaneous bacterial peritonitis: a retrospective study. Exp. Ther. Med. 17, 4568–4576. doi: 10.3892/etm.2019.7502
Hamilton-Miller, J. M., Kerry, D. W., and Brumfitt, W. (1974). An in vivo comparison of cefoxitin, a semi-synthetic cephamycin, with cephalothin. J. Antibiot. 27, 42–48. doi: 10.7164/antibiotics.27.42
Hancock, R. E., and Bellido, F. (1992). Factors involved in the enhanced efficacy against gram-negative bacteria of fourth generation cephalosporins. J. Antimicrob. Chemother. 29, (Suppl. A), 1–6. doi: 10.1093/jac/29.suppl_a.1
He, X., Mezyk, S. P., Michael, I., Fatta-Kassinos, D., and Dionysiou, D. D. (2014). Degradation kinetics and mechanism of beta-lactam antibiotics by the activation of H2O2 and Na2S2O8 under UV-254nm irradiation. J. Hazard. Mater. 279, 375–383. doi: 10.1016/j.jhazmat.2014.07.008
Hinkle, A. M., LeBlanc, B. M., and Bodey, G. P. (1980). In vitro evaluation of cefoperazone. Antimicrob. Agents Chemother. 17, 423–427. doi: 10.1128/aac.17.3.423
Hsieh, W. C., and Ho, S. W. (1975). Evaluation of antibacterial activities of cephalosporin antibiotics: cefazolin, cephaloridine, cephalothin, and cephalexin. Zhonghua Min. Guo Wei Sheng Wu Xue Za Zhi 8, 1–11.
Hughes, D. L. (2017a). Patent review of manufacturing routes to fifth-generation cephalosporin drugs. Part 1, ceftolozane. Org. Process Res. Dev. 21, 430–443. doi: 10.1021/acs.oprd.7b00033
Hughes, D. L. (2017b). Patent review of manufacturing routes to fifth-generation cephalosporin drugs. Part 2, ceftaroline fosamil and ceftobiprole medocaril. Org. Process Res. Dev. 21, 800–815. doi: 10.1021/acs.oprd.7b00143
Hujer, A. M., Kania, M., Gerken, T., Anderson, V. E., Buynak, J. D., Ge, X., et al. (2005). Structure-activity relationships of different beta-lactam antibiotics against a soluble form of Enterococcus faecium PBP5, a type II bacterial transpeptidase. Antimicrob. Agents Chemother. 49, 612–618. doi: 10.1128/AAC.49.2.612-618.2005
Huson, D. H., Richter, D. C., Rausch, C., Dezulian, T., Franz, M., and Rupp, R. (2007). Dendroscope: an interactive viewer for large phylogenetic trees. BMC Bioinformatics 8:460. doi: 10.1186/1471-2105-8-460
Iizawa, Y., Nagai, J., Ishikawa, T., Hashiguchi, S., Nakao, M., Miyake, A., et al. (2004). In vitro antimicrobial activity of T-91825, a novel anti-MRSA cephalosporin, and in vivo anti-MRSA activity of its prodrug, TAK-599. J. Infect. Chemother. 10, 146–156. doi: 10.1007/s10156-004-0309-3
Imada, A., Kitano, K., Kintaka, K., Muroi, M., and Asai, M. (1981). Sulfazecin and isosulfazecin, novel beta-lactam antibiotics of bacterial origin. Nature 289, 590–591. doi: 10.1038/289590a0
Inamoto, Y., Chiba, T., Kamimura, T., and Takaya, T. (1988). FK 482, a new orally active cephalosporin synthesis and biological properties. J. Antibiot. 41, 828–830. doi: 10.7164/antibiotics.41.828
Inamoto, Y., Chiba, T., Sakane, K., Kamimura, T., and Takaya, T. (1990a). [Studies on FK482. II. Synthesis and structure-activity relationships of 7 beta-[(Z)-2-(2-aminothiazol-4-yl)-2-substituted acetamido]-3-vinyl-3-cephem-4-carboxylic acid derivatives]. Yakugaku Zasshi 110, 246–257. doi: 10.1248/yakushi1947.110.4_246
Inamoto, Y., Sakane, K., Kamimura, T., and Takaya, T. (1990b). [Studies on FK482 (Cefdinir). III. Synthesis and structure-activity relationships of 7 beta-[(Z)-2-aryl-2-hydroxyiminoacetamido]-3-vinyl-3- cephem-4-carboxylic acid derivatives]. Yakugaku Zasshi 110, 658–664. doi: 10.1248/yakushi1947.110.9_658
Ishikawa, T., Matsunaga, N., Tawada, H., Kuroda, N., Nakayama, Y., Ishibashi, Y., et al. (2003). TAK-599, a novel N-phosphono type prodrug of anti-MRSA cephalosporin T-91825: synthesis, physicochemical and pharmacological properties. Bioorg. Med. Chem. 11, 2427–2437. doi: 10.1016/s0968-0896(03)00126-3
Issa, N. C., Rouse, M. S., Piper, K. E., Wilson, W. R., Steckelberg, J. M., and Patel, R. (2004). In vitro activity of BAL9141 against clinical isolates of gram-negative bacteria. Diagn. Microbiol. Infect. Dis. 48, 73–75. doi: 10.1016/j.diagmicrobio.2003.09.006
Istre, G. R., Welch, D. F., Marks, M. I., and Moyer, N. (1981). Susceptibility of group A beta-hemolytic Streptococcus isolates to penicillin and erythromycin. Antimicrob. Agents Chemother. 20, 244–246. doi: 10.1128/aac.20.2.244
Ito, A., Nishikawa, T., Matsumoto, S., Yoshizawa, H., Sato, T., Nakamura, R., et al. (2016). Siderophore cephalosporin cefiderocol utilizes ferric iron transporter systems for antibacterial activity against Pseudomonas aeruginosa. Antimicrob. Agents Chemother. 60, 7396–7401. doi: 10.1128/AAC.01405-16
James, M. N., Hall, D., and Hodgkin, D. C. (1968). Crystalline modifications of ampicillin I: the trihydrate. Nature 220, 168–170. doi: 10.1038/220168a0
Jean, S. S., Lee, W. S., Ko, W. C., and Hsueh, P. R. (2020). In vitro susceptibility of ceftaroline against clinically important Gram-positive cocci, Haemophilus species and Klebsiella pneumoniae in Taiwan: results from the antimicrobial testing leadership and surveillance (ATLAS) in 2012-2018. J. Microbiol. Immunol. Infect. 54, 627–631. doi: 10.1016/j.jmii.2020.04.017
Jeong, J. H., Cha, H. J., and Kim, Y. G. (2018). Crystal structures of penicillin-binding protein D2 from Listeria monocytogenes and structural basis for antibiotic specificity. Antimicrob. Agents Chemother. 62:e00796-18. doi: 10.1128/AAC.00796-18
Jones, R. N., Barry, A. L., Thornsberry, C., and Wilson, H. W. (1981). In vitro antimicrobial activity evaluation of cefodizime (HR221), a new semisynthetic cephalosporin. Antimicrob. Agents Chemother. 20, 760–768. doi: 10.1128/aac.20.6.760
Jones, R. N., Thornsberry, C., and Barry, A. L. (1984). In vitro evaluation of HR810, a new wide-spectrum aminothiazolyl alpha-methoxyimino cephalosporin. Antimicrob. Agents Chemother. 25, 710–718. doi: 10.1128/aac.25.6.710
Kamimura, T., Kojo, H., Matsumoto, Y., Mine, Y., Goto, S., and Kuwahara, S. (1984). In vitro and in vivo antibacterial properties of FK 027, a new orally active cephem antibiotic. Antimicrob. Agents Chemother. 25, 98–104. doi: 10.1128/aac.25.1.98
Kamimura, T., Matsumoto, Y., Okada, N., Mine, Y., Nishida, M., Goto, S., et al. (1979). Ceftizoxime (FK 749), a new parenteral cephalosporin: in vitro and in vivo antibacterial activities. Antimicrob. Agents Chemother. 16, 540–548. doi: 10.1128/aac.16.5.540
Kayser, F. H., Morenzoni, G., Strassle, A., and Hadorn, K. (1989). Activity of meropenem, against gram-positive bacteria. J. Antimicrob. Chemother. 24, (Suppl. A), 101–112. doi: 10.1093/jac/24.suppl_a.101
Kessler, R. E., Bies, M., Buck, R. E., Chisholm, D. R., Pursiano, T. A., Tsai, Y. H., et al. (1985). Comparison of a new cephalosporin, BMY 28142, with other broad-spectrum beta-lactam antibiotics. Antimicrob. Agents Chemother. 27, 207–216. doi: 10.1128/aac.27.2.207
Khan, N. J., Bihl, J. A., Schell, R. F., LeFrock, J. L., and Weber, S. J. (1984). Antimicrobial activities of BMY-28142, cefbuperazone, and cefpiramide compared with those of other cephalosporins. Antimicrob. Agents Chemother. 26, 585–590. doi: 10.1128/aac.26.4.585
Kivits, T., Broers, H. P., Beeltje, H., van Vliet, M., and Griffioen, J. (2018). Presence and fate of veterinary antibiotics in age-dated groundwater in areas with intensive livestock farming. Environ. Pollut. 241, 988–998. doi: 10.1016/j.envpol.2018.05.085
Kohira, N., West, J., Ito, A., Ito-Horiyama, T., Nakamura, R., Sato, T., et al. (2016). In vitro antimicrobial activity of a siderophore cephalosporin, S-649266, against Enterobacteriaceae clinical isolates, including carbapenem-resistant strains. Antimicrob. Agents Chemother. 60, 729–734. doi: 10.1128/AAC.01695-15
Kojo, H., Nishida, M., Goto, S., and Kuwahara, S. (1979). Antibacterial activity of ceftizoxime (FK 749), a new cephalosporin, against cephalosporin-resistant bacteria, and its stability to beta-lactamase. Antimicrob. Agents Chemother. 16, 549–553. doi: 10.1128/aac.16.5.549
Kosmidis, J., Hamilton-Miller, J. M., Gilchrist, J. N., Kerry, D. W., and Brumfitt, W. (1973). Cefoxitin, a new semi-synthetic cephamycin: an in-vitro and in-vivo comparison with cephalothin. Br. Med. J. 4, 653–655. doi: 10.1136/bmj.4.5893.653
Kriebernegg, I., Feierl, G., Grisold, A., and Marth, E. (1998). In-vitro susceptibility of group A beta-haemolytic streptococci (GABHS) to penicillin, erythromycin, clarithromycin and azithromycin in Styria, Austria. Zentralbl. Bakteriol. 287, 33–39. doi: 10.1016/s0934-8840(98)80139-4
Lang, M., Prasad, K., Holick, W., Gosteli, J., Ernest, I., and Woodward, R. B. (1978). The penems, a new class of beta-lactam antibiotics. 2. Total synthesis of racemic 6-unsubstituted representatives. J. Am. Chem. Soc. 101, 6296–6301.
Lev, E. I., Kleiman, N. S., Birnbaum, Y., Harris, D., Korbling, M., and Estrov, Z. (2005). Circulating endothelial progenitor cells and coronary collaterals in patients with non-ST segment elevation myocardial infarction. J. Vasc. Res. 42, 408–414. doi: 10.1159/000087370
Libby, R. L., and Holmberg, N. L. (1945). The activity of penicillins G and X in vitro. Science 102, 303–304. doi: 10.1126/science.102.2647.303
Lima, L. M., Silva, B., Barbosa, G., and Barreiro, E. J. (2020). beta-lactam antibiotics: an overview from a medicinal chemistry perspective. Eur. J. Med. Chem. 208:112829. doi: 10.1016/j.ejmech.2020.112829
Limbert, M., Isert, D., Klesel, N., Markus, A., Seeger, K., Seibert, G., et al. (1991). Antibacterial activities in vitro and in vivo and pharmacokinetics of cefquinome (HR 111V), a new broad-spectrum cephalosporin. Antimicrob. Agents Chemother. 35, 14–19. doi: 10.1128/aac.35.1.14
Livermore, D. M., Carter, M. W., Bagel, S., Wiedemann, B., Baquero, F., Loza, E., et al. (2001). In vitro activities of ertapenem (MK-0826) against recent clinical bacteria collected in Europe and Australia. Antimicrob. Agents Chemother. 45, 1860–1867. doi: 10.1128/AAC.45.6.1860-1867.2001
Lohans, C. T., Chan, H. T. H., Malla, T. R., Kumar, K., Kamps, J., McArdle, D. J. B., et al. (2019). Non-hydrolytic beta-lactam antibiotic fragmentation by l,d-transpeptidases and serine beta-lactamase cysteine variants. Angew. Chem. Int. Ed Engl. 58, 1990–1994. doi: 10.1002/anie.201809424
Lu, Z., Wang, H., Zhang, A., Liu, X., Zhou, W., Yang, C., et al. (2020). Structures of Mycobacterium tuberculosis penicillin-binding protein 3 in complex with five beta-lactam antibiotics reveal mechanism of inactivation. Mol. Pharmacol. 97, 287–294. doi: 10.1124/mol.119.118042
Macheboeuf, P., Contreras-Martel, C., Job, V., Dideberg, O., and Dessen, A. (2006). Penicillin binding proteins: key players in bacterial cell cycle and drug resistance processes. FEMS Microbiol. Rev. 30, 673–691. doi: 10.1111/j.1574-6976.2006.00024.x
Machka, K., and Braveny, I. (1983). In vitro activity of HR 810, a new broad-spectrum cephalosporin. Eur. J. Clin. Microbiol. 2, 345–349. doi: 10.1007/BF02019465
Macris, M. H., Hartman, N., Murray, B., Klein, R. F., Roberts, R. B., Kaplan, E. L., et al. (1998). Studies of the continuing susceptibility of group A streptococcal strains to penicillin during eight decades. Pediatr. Infect. Dis. J. 17, 377–381. doi: 10.1097/00006454-199805000-00006
Marchese, A., Gualco, L., Schito, A. M., Debbia, E. A., and Schito, G. C. (2004). In vitro activity of ertapenem against selected respiratory pathogens. J. Antimicrob. Chemother. 54, 944–951. doi: 10.1093/jac/dkh445
Matsubara, N., Minami, S., Muraoka, T., Saikawa, I., and Mitsuhashi, S. (1979). In vitro antibacterial activity of cefoperazone (T-1551), a new semisynthetic cephalosporin. Antimicrob. Agents Chemother. 16, 731–735. doi: 10.1128/aac.16.6.731
Meng, G., Zhang, J., and Szostak, M. (2021). Acyclic twisted amides. Chem. Rev. 121, 12746–12783. doi: 10.1021/acs.chemrev.1c00225
Mine, Y., Kamimura, T., Watanabe, Y., Tawara, S., Matsumoto, Y., Shibayama, F., et al. (1988). In vitro antibacterial activity of FK482, a new orally active cephalosporin. J. Antibiot. 41, 1873–1887. doi: 10.7164/antibiotics.41.1873
Morin, R. B., Jackson, B. G., Mueller, R. A., Lavagnino, E. R., Scanlon, W. B., and Andrews, S. L. (1969). Chemistry of cephalosporin antibiotics. XV. Transformations of penicillin sulfoxide. A synthesis of cephalosporin compounds. J. Am. Chem. Soc. 91, 1401–1407. doi: 10.1021/ja01034a023
Musser, J. M., Beres, S. B., Zhu, L., Olsen, R. J., Vuopio, J., Hyyrylainen, H. L., et al. (2020). Reduced in vitro susceptibility of Streptococcus pyogenes to beta-lactam antibiotics associated with mutations in the pbp2x gene is geographically widespread. J. Clin. Microbiol. 58:e01993-19. doi: 10.1128/JCM.01993-19
Nell, E. E., and Hill, J. H. (1947). Comparison of the in vitro antigonococcal actions of penicillins G, F, K, and X. Am. J. Syph. Gonorrhea Vener. Dis. 31, 14–19.
Neu, H. C. (1974b). Cefoxitin, a semisynthetic cephamycin antibiotic: antibacterial spectrum and resistance to hydrolysis by gram-negative beta-lactamases. Antimicrob. Agents Chemother. 6, 170–176. doi: 10.1128/aac.6.2.170
Neu, H. C. (1974a). Cefamandole, a cephalosporin antibiotic with an unusually wide spectrum of activity. Antimicrob. Agents Chemother. 6, 177–182. doi: 10.1128/aac.6.2.177
Neu, H. C. (1976). Mecillinam, a novel penicillanic acid derivative with unusual activity against gram-negative bacteria. Antimicrob. Agents Chemother. 9, 793–799. doi: 10.1128/aac.9.5.793
Neu, H. C., and Chin, N. X. (1986). In vitro activity and beta-lactamase stability of a new difluoro oxacephem, 6315-S. Antimicrob. Agents Chemother. 30, 638–644. doi: 10.1128/aac.30.5.638
Neu, H. C., Chin, N. X., and Labthavikul, P. (1984). Comparative in vitro activity and beta-lactamase stability of FR 17027, a new orally active cephalosporin. Antimicrob. Agents Chemother. 26, 174–180. doi: 10.1128/aac.26.2.174
Neu, H. C., Fu, K. P., Aswapokee, N., Aswapokee, P., and Kung, K. (1979). Comparative activity and beta-lactamase stability of cefoperazone, a piperazine cephalosporin. Antimicrob. Agents Chemother. 16, 150–157. doi: 10.1128/aac.16.2.150
Neu, H. C., Meropol, N. J., and Fu, K. P. (1981). Antibacterial activity of ceftriaxone (Ro 13-9904), a beta-lactamase-stable cephalosporin. Antimicrob. Agents Chemother. 19, 414–423. doi: 10.1128/aac.19.3.414
Neu, H. C., Saha, G., and Chin, N. X. (1989). Comparative in vitro activity and beta-lactamase stability of FK482, a new oral cephalosporin. Antimicrob. Agents Chemother. 33, 1795–1800. doi: 10.1128/aac.33.10.1795
Nomura, H., Fugono, T., Hitaka, T., Minami, I., Azuma, T., Morimoto, S., et al. (1974). Semisynthetic beta-lactam antibiotics. 6. 1 Sulfocephalosporins and their antipseudomonal activities. J. Med. Chem. 17, 1312–1315. doi: 10.1021/jm00258a017
O’Callaghan, C. H., Acred, P., Harper, P. B., Ryan, D. M., Kirby, S. M., and Harding, S. M. (1980). GR 20263, a new broad-spectrum cephalosporin with anti-pseudomonal activity. Antimicrob. Agents Chemother. 17, 876–883. doi: 10.1128/aac.17.5.876
Ochoa-Aguilar, A., Ventura-Martinez, R., Sotomayor-Sobrino, M. A., Gomez, C., and Morales-Espinoza, M. R. (2016). Review of antibiotic and non-antibiotic properties of beta-lactam molecules. Antiinflamm. Antiallergy Agents Med. Chem. 15, 3–14. doi: 10.2174/1871523015666160517114027
Okamoto, S., Hamana, Y., Inoue, M., and Mitsuhashi, S. (1987). In vitro and in vivo antibacterial activities of T-2588, a new oral cephalosporin, compared with those of other oral beta-lactam antibiotics. Antimicrob. Agents Chemother. 31, 1111–1116. doi: 10.1128/aac.31.7.1111
Osborn, M., Stachulski, N., Sun, H., Blais, J., Venishetty, V., Raccuglia, M., et al. (2019). A first-in-human study to assess the safety and pharmacokinetics of LYS228, a novel intravenous monobactam antibiotic in healthy volunteers. Antimicrob. Agents Chemother. 63:e02592-18. doi: 10.1128/AAC.02592-18
Pechere, J. C., and Kohler, T. (1999). Patterns and modes of beta-lactam resistance in Pseudomonas aeruginosa. Clin. Microbiol. Infect. 5, (Suppl. 1), S15–S18. doi: 10.1111/j.1469-0691.1999.tb00719.x
Pendland, S. L., Neuhauser, M. M., and Prause, J. L. (2002). In vitro bactericidal activity of ABT-773 and amoxicillin against erythromycin-susceptible and -resistant strains of Streptococcus pyogenes. J. Antimicrob. Chemother. 49, 671–674. doi: 10.1093/jac/49.4.671
Peterson, Y. K., Cameron, R. B., Wills, L. P., Trager, R. E., Lindsey, C. C., Beeson, C. C., et al. (2013). beta-adrenoceptor agonists in the regulation of mitochondrial biogenesis. Bioorg. Med. Chem. Lett. 23, 5376–5381. doi: 10.1016/j.bmcl.2013.07.052
Poch, G., Brunner, F., and Kuhberger, E. (1992). Construction of antagonist dose-response curves for estimation of pA2-values by schild-plot analysis and detection of allosteric interactions. Br. J. Pharmacol. 106, 710–716. doi: 10.1111/j.1476-5381.1992.tb14399.x
Reiner, R., Weiss, U., Brombacher, U., Lanz, P., Montavon, M., Furlenmeier, A., et al. (1980). Ro 13-9904/001, a novel potent and long-acting parenteral cephalosporin. J. Antibiot. 33, 783–786. doi: 10.7164/antibiotics.33.783
Romano, A., Gaeta, F., Valluzzi, R. L., Zaffiro, A., Caruso, C., and Quaratino, D. (2014). Natural evolution of skin-test sensitivity in patients with IgE-mediated hypersensitivity to cephalosporins. Allergy 69, 806–809. doi: 10.1111/all.12390
Romano, A., Gueant-Rodriguez, R. M., Viola, M., Pettinato, R., and Gueant, J. L. (2004). Cross-reactivity and tolerability of cephalosporins in patients with immediate hypersensitivity to penicillins. Ann. Intern. Med. 141, 16–22. doi: 10.7326/0003-4819-141-1-200407060-00010
Romano, A., Valluzzi, R. L., Caruso, C., Maggioletti, M., Quaratino, D., and Gaeta, F. (2018). Cross-reactivity and tolerability of cephalosporins in patients with IgE-mediated hypersensitivity to penicillins. J. Allergy Clin. Immunol. Pract. 6, 1662–1672. doi: 10.1016/j.jaip.2018.01.020
Romano, A., Viola, M., Gueant-Rodriguez, R. M., Gaeta, F., Pettinato, R., and Gueant, J. L. (2006). Imipenem in patients with immediate hypersensitivity to penicillins. N. Engl. J. Med. 354, 2835–2837. doi: 10.1056/NEJMc053529
Romano, A., Viola, M., Gueant-Rodriguez, R. M., Gaeta, F., Valluzzi, R., and Gueant, J. L. (2007). Brief communication: tolerability of meropenem in patients with IgE-mediated hypersensitivity to penicillins. Ann. Intern. Med. 146, 266–269. doi: 10.7326/0003-4819-146-4-200702200-00005
Ryan, C. W., Simon, R. L., and Van Heyningen, E. M. (1969). Chemistry of cephalosporin antibiotics. 13. Desacetoxycephalosporins. The synthesis of cephalexin and some analogs. J. Med. Chem. 12, 310–313. doi: 10.1021/jm00302a026
Sader, H. S., Fritsche, T. R., Kaniga, K., Ge, Y., and Jones, R. N. (2005). Antimicrobial activity and spectrum of PPI-0903M (T-91825), a novel cephalosporin, tested against a worldwide collection of clinical strains. Antimicrob. Agents Chemother. 49, 3501–3512. doi: 10.1128/AAC.49.8.3501-3512.2005
Sakagawa, E., Otsuki, M., Oh, T., and Nishino, T. (1998). In-vitro and in-vivo antibacterial activities of CS-834, a new oral carbapenem. J. Antimicrob. Chemother. 42, 427–437. doi: 10.1093/jac/42.4.427
Sakata, H. (2013). Susceptibility and emm type of Streptococcus pyogenes isolated from children with severe infection. J. Infect. Chemother. 19, 1042–1046. doi: 10.1007/s10156-013-0617-6
Sato, J., Kusano, H., Aoki, T., Shibuya, S., Yokoo, K., Komano, K., et al. (2022). Discovery of a tricyclic beta-lactam as a potent antimicrobial agent against carbapenem-resistant Enterobacterales, including strains with reduced membrane permeability and four-amino acid insertion into penicillin-binding protein 3: structure-activity-relationships and in vitro and in vivo activities. ACS Infect. Dis. doi: 10.1021/acsinfecdis.1c00549
[Online ahead of print].Sauvage, E., Kerff, F., Terrak, M., Ayala, J. A., and Charlier, P. (2008). The penicillin-binding proteins: structure and role in peptidoglycan biosynthesis. FEMS Microbiol. Rev. 32, 234–258. doi: 10.1111/j.1574-6976.2008.00105.x
Scully, B. E., Jules, K., and Neu, H. C. (1983). In vitro activity and beta-lactamase stability of cefodizime, an aminothiazolyl iminomethoxy cephalosporin. Antimicrob. Agents Chemother. 23, 907–913. doi: 10.1128/aac.23.6.907
Seibert, G., Klesel, N., Limbert, M., Schrinner, E., Seeger, K., Winkler, I., et al. (1983). HR 810, a new parenteral cephalosporin with a broad antibacterial spectrum. Arzneimittelforschung 33, 1084–1086. doi: 10.1002/chin.198350201
Shannon, K., King, A., Warren, C., and Phillips, I. (1980). In vitro antibacterial activity and susceptibility of the cephalosporin Ro 13-9904 to beta-lactamases. Antimicrob. Agents Chemother. 18, 292–298. doi: 10.1128/aac.18.2.292
Shapiro, A. B., Moussa, S. H., McLeod, S. M., Durand-Reville, T., and Miller, A. A. (2021). Durlobactam, a new diazabicyclooctane beta-lactamase inhibitor for the treatment of acinetobacter infections in combination with sulbactam. Front. Microbiol. 12:709974. doi: 10.3389/fmicb.2021.709974
Shelton, S., and Nelson, J. D. (1988). In vitro susceptibilities of common pediatric pathogens to LY163892. Antimicrob. Agents Chemother. 32, 268–270. doi: 10.1128/aac.32.2.268
Shibata, M., Kashiwagi, Y., Tokue, S., Tominaga, Y., and Okubo, M. (1975). [Drug susceptibility of hemolytic streptococci isolated from patients with scarlet fever during 1973]. Kansenshogaku Zasshi 49, 94–97. doi: 10.11150/kansenshogakuzasshi1970.49.94
Sidell, S., Burdick, R. E., Brodie, J., Bulger, R. J., and Kirby, W. M. (1963). New antistaphylococcal antibiotics. Comparative in vitro and in vivo activity of cephalothin, nafcillin, cloxacillin, oxacillin, and methicillin. Arch. Intern. Med. 112, 21–28. doi: 10.1001/archinte.1963.03860010067005
Silvaggi, N. R., Josephine, H. R., Kuzin, A. P., Nagarajan, R., Pratt, R. F., and Kelly, J. A. (2005). Crystal structures of complexes between the R61 DD-peptidase and peptidoglycan-mimetic beta-lactams: a non-covalent complex with a “perfect penicillin”. J. Mol. Biol. 345, 521–533. doi: 10.1016/j.jmb.2004.10.076
Song, Z., Zhang, X., Ngo, H. H., Guo, W., Wen, H., and Li, C. (2019). Occurrence, fate and health risk assessment of 10 common antibiotics in two drinking water plants with different treatment processes. Sci. Total Environ. 674, 316–326. doi: 10.1016/j.scitotenv.2019.04.093
Sosna, J. P., Murray, P. R., and Medoff, G. (1978). Comparison of the in vitro activities of HR756 with cephalothin, cefoxitin, and cefamandole. Antimicrob. Agents Chemother. 14, 876–879. doi: 10.1128/aac.14.6.876
Spencer, J. L., Flynn, E. H., Roeske, R. W., Siu, F. Y., and Chauvette, R. R. (1966a). Chemistry of cephalosporin antibiotics. VII. Synthesis of cephaloglycin and some homologs. J. Med. Chem. 9, 746–750. doi: 10.1021/jm00323a024
Spencer, J. L., Siu, F. Y., Flynn, E. H., Jackson, B. G., Sigal, M. V., Higgins, H. M., et al. (1966b). Chemistry of cephalosporin antibiotics. 8. Synthesis and structure-activity relationships of cephaloridine analogues. Antimicrob. Agents Chemother. 6, 573–580.
Stamm, J. M., Girolami, R. L., Shipkowitz, N. L., and Bower, R. R. (1981). Antimicrobial activity of cefmenoxime (SCE-1365). Antimicrob. Agents Chemother. 19, 454–460. doi: 10.1128/aac.19.3.454
Stefani, S., Mezzatesta, M. L., Fadda, G., Mattina, R., Palu, G., Rossano, F., et al. (2008). Antibacterial activity of cefditoren against major community-acquired respiratory pathogens recently isolated in Italy. J. Chemother. 20, 561–569. doi: 10.1179/joc.2008.20.5.561
Stewart, D., and Bodey, G. P. (1977). Azlocillin: in vitro studies of a new semisynthetic penicillin. Antimicrob. Agents Chemother. 11, 865–870. doi: 10.1128/aac.11.5.865
Stover, K. R., Barber, K. E., and Wagner, J. L. (2019). Allergic reactions and cross-reactivity potential with beta-lactamase inhibitors. Pharmacy 7:77. doi: 10.3390/pharmacy7030077
Suzuki, Y., Ishihara, R., Ishii, Y., Nakazawa, A., Deguchi, K., Matsumoto, Y., et al. (1996). [Antimicrobial activity of cefodizime against clinical isolates]. Jpn. J. Antibiot. 49, 947–965.
Sweet, R. M., and Dahl, L. F. (1970). Molecular architecture of the cephalosporins. Insights into biological activity based on structural investigations. J. Am. Chem. Soc. 92, 5489–5507. doi: 10.1021/ja00721a032
Sykes, R. B., Bonner, D. P., Bush, K., and Georgopapadakou, N. H. (1982). Azthreonam (SQ 26,776), a synthetic monobactam specifically active against aerobic gram-negative bacteria. Antimicrob. Agents Chemother. 21, 85–92. doi: 10.1128/aac.21.1.85
Sykes, R. B., Cimarusti, C. M., Bonner, D. P., Bush, K., Floyd, D. M., Georgopapadakou, N. H., et al. (1981). Monocyclic beta-lactam antibiotics produced by bacteria. Nature 291, 489–491. doi: 10.1038/291489a0
Szekeres, E., Chiriac, C. M., Baricz, A., Szoke-Nagy, T., Lung, I., Soran, M. L., et al. (2018). Investigating antibiotics, antibiotic resistance genes, and microbial contaminants in groundwater in relation to the proximity of urban areas. Environ. Pollut. 236, 734–744. doi: 10.1016/j.envpol.2018.01.107
Szostak, R., Aube, J., and Szostak, M. (2015). Determination of structures and energetics of small- and medium-sized one-carbon-bridged twisted amides using AB initio molecular orbital methods: implications for amidic resonance along the C-N rotational pathway. J. Org. Chem. 80, 7905–7927. doi: 10.1021/acs.joc.5b00881
Takeda, S., Ishii, Y., Hatano, K., Tateda, K., and Yamaguchi, K. (2007a). Stability of FR264205 against AmpC beta-lactamase of Pseudomonas aeruginosa. Int. J. Antimicrob. Agents 30, 443–445. doi: 10.1016/j.ijantimicag.2007.05.019
Takeda, S., Nakai, T., Wakai, Y., Ikeda, F., and Hatano, K. (2007b). In vitro and in vivo activities of a new cephalosporin, FR264205, against Pseudomonas aeruginosa. Antimicrob. Agents Chemother. 51, 826–830. doi: 10.1128/AAC.00860-06
Tao, W., Ivanovska, V., Schweickert, B., and Muller, A. (2019). Proxy indicators for antibiotic consumption; surveillance needed to control antimicrobial resistance. Bull. World Health Organ. 97, 3–3A. doi: 10.2471/BLT.18.227348
Tempera, G., Furneri, P. M., Carlone, N. A., Cocuzza, C., Rigoli, R., Musumeci, R., et al. (2010). Antibiotic susceptibility of respiratory pathogens recently isolated in Italy: focus on cefditoren. J. Chemother. 22, 153–159. doi: 10.1179/joc.2010.22.3.153
Thomsen, V. F., and Larsen, S. O. (1962). [In vitro activity of penicillin. Comparative studies on benzylpenicillin, phenoxymethylpenicillin, phenoxyethylpenicillin and dimethoxyphenylpenicillin]. Nord. Med. 67, 111–119.
Thornhill, T. S., Levison, M. E., Johnson, W. D., and Kaye, D. (1969). In vitro antimicrobial activity and human pharmacology of cephalexin, a new orally absorbed cephalosporin C antibiotic. Appl. Microbiol. 17, 457–461.
Toda, A., Ohki, H., Yamanaka, T., Murano, K., Okuda, S., Kawabata, K., et al. (2008). Synthesis and SAR of novel parenteral anti-pseudomonal cephalosporins: discovery of FR264205. Bioorg. Med. Chem. Lett. 18, 4849–4852. doi: 10.1016/j.bmcl.2008.07.085
Toda, M., Arao, N., Nohara, C., Susaki, K., and Tachibana, A. (1985). In vitro studies on the antibacterial activities of YM-13115, a new broad-spectrum cephalosporin. Antimicrob. Agents Chemother. 27, 565–569. doi: 10.1128/aac.27.4.565
Trubiano, J. A., Stone, C. A., Grayson, M. L., Urbancic, K., Slavin, M. A., Thursky, K. A., et al. (2017). The 3 Cs of antibiotic allergy-classification, cross-reactivity, and collaboration. J. Allergy Clin. Immunol. Pract. 5, 1532–1542. doi: 10.1016/j.jaip.2017.06.017
Tsuchiya, K., Kondo, M., Kida, M., Nakao, M., Iwahi, T., Nishi, T., et al. (1981). Cefmenoxime (SCE-1365), a novel broad-spectrum cephalosporin: in vitro and in vivo antibacterial activities. Antimicrob. Agents Chemother. 19, 56–65. doi: 10.1128/aac.19.1.56
Tsuchiya, K., Kondo, M., and Nagatomo, H. (1978). SCE-129, antipseudomonal cephalosporin: in vitro and in vivo antibacterial activities. Antimicrob. Agents Chemother. 13, 137–145. doi: 10.1128/aac.13.2.137
Tsuji, M., Ishii, Y., Ohno, A., Miyazaki, S., and Yamaguchi, K. (1995). In vitro and in vivo antibacterial activities of S-1090, a new oral cephalosporin. Antimicrob. Agents Chemother. 39, 2544–2551. doi: 10.1128/aac.39.11.2544
Tsuji, M., Ishii, Y., Ohno, A., Miyazaki, S., and Yamaguchi, K. (1998). In vitro and in vivo antibacterial activities of S-4661, a new carbapenem. Antimicrob. Agents Chemother. 42, 94–99. doi: 10.1128/AAC.42.1.94
Ubukata, K., Wajima, T., Morozumi, M., Sakuma, M., Tajima, T., Matsubara, K., et al. (2020). Changes in epidemiologic characteristics and antimicrobial resistance of Streptococcus pyogenes isolated over 10 years from Japanese children with pharyngotonsillitis. J. Med. Microbiol. 69, 443–450. doi: 10.1099/jmm.0.001158
Une, T., Otani, T., Sato, M., Ikeuchi, T., Osada, Y., Ogawa, H., et al. (1985). In vitro and in vivo activities of DN-9550, a new broad-spectrum cephalosporin. Antimicrob. Agents Chemother. 27, 473–478. doi: 10.1128/aac.27.4.473
Veeraraghavan, B., Bakthavatchalam, Y. D., and Sahni, R. D. (2021). Orally administered amoxicillin/clavulanate: current role in outpatient therapy. Infect. Dis. Ther. 10, 15–25. doi: 10.1007/s40121-020-00374-7
Verbist, L. (1978). In vitro activity of piperacillin, a new semisynthetic penicillin with an unusually broad spectrum of activity. Antimicrob. Agents Chemother. 13, 349–357. doi: 10.1128/aac.13.3.349
Verbist, L., and Verhaegen, J. (1980). GR-20263: a new aminothiazolyl cephalosporin with high activity against Pseudomonas and Enterobacteriaceae. Antimicrob. Agents Chemother. 17, 807–812. doi: 10.1128/aac.17.5.807
Vuye, A., and Pijck, J. (1985). In vitro antibacterial activity of BMY-28142, a new extended-spectrum cephalosporin. Antimicrob. Agents Chemother. 27, 574–577. doi: 10.1128/aac.27.4.574
Wallick, H., and Hendlin, D. (1974). Cefoxitin, a semisynthetic cephamycin antibiotic: susceptibility studies. Antimicrob. Agents Chemother. 5, 25–32. doi: 10.1128/aac.5.1.25
Wallmark, G. (1962). Comparison in vitro of new penicillins. Special reference to methicillin-resistant staphylococci. Arch. Intern. Med. 110, 787–800. doi: 10.1001/archinte.1962.03620230233032
Watanakunakorn, C., and Glotzbecker, C. (1979). Comparative in vitro activity of LY 127935 (6059-S), seven cephalosporins, three aminoglycosides, carbenicillin, and ticarcillin. J. Antibiot. 32, 1019–1024. doi: 10.7164/antibiotics.32.1019
Waxman, D. J., Yocum, R. R., and Strominger, J. L. (1980). Penicillins and cephalosporins are active site-directed acylating agents: evidence in support of the substrate analogue hypothesis. Philos. Trans. R. Soc. Lond. B Biol. Sci. 289, 257–271. doi: 10.1098/rstb.1980.0044
Weaver, S. S., Bodey, G. P., and LeBlanc, B. M. (1979). Thienamycin: new beta-lactam antibiotic with potent broad-spectrum activity. Antimicrob. Agents Chemother. 15, 518–521. doi: 10.1128/aac.15.4.518
Wise, R., Andrews, J. M., Ashby, J. P., and Matthews, R. S. (1988). In vitro activity of lomefloxacin, a new quinolone antimicrobial agent, in comparison with those of other agents. Antimicrob. Agents Chemother. 32, 617–622. doi: 10.1128/aac.32.5.617
Wise, R., Andrews, J. M., Ashby, J. P., and Thornber, D. (1991). Ceftibuten: a new orally absorbed cephalosporin. In vitro activity against strains from the United Kingdom. Diagn. Microbiol. Infect. Dis. 14, 45–52. doi: 10.1016/0732-8893(91)90089-x
Wise, R., Andrews, J. M., and Bedford, K. A. (1980). Comparison of in vitro activity of GR 20263, a novel cephalosporin derivative, with activities of other beta-lactam compounds. Antimicrob. Agents Chemother. 17, 884–889. doi: 10.1128/aac.17.5.884
Wise, R., Rollason, T., Logan, M., Andrews, J. M., and Bedford, K. A. (1978). HR 756, a highly active cephalosporin: comparison with cefazolin and carbenicillin. Antimicrob. Agents Chemother. 14, 807–811. doi: 10.1128/aac.14.6.807
Woodcock, J. M., Andrews, J. M., Brenwald, N. P., Ashby, J. P., and Wise, R. (1997). The in-vitro activity of faropenem, a novel oral penem. J. Antimicrob. Chemother. 39, 35–43. doi: 10.1093/jac/39.1.35
Woodward, R. B. (1980). Penems and related substances. Philos. Trans. R. Soc. Lond. B Biol. Sci. 289, 239–250. doi: 10.1098/rstb.1980.0042
Wootton, M., Bowker, K. E., Holt, H. A., and MacGowan, A. P. (2002). BAL 9141, a new broad-spectrum pyrrolidinone cephalosporin: activity against clinically significant anaerobes in comparison with 10 other antimicrobials. J. Antimicrob. Chemother. 49, 535–539. doi: 10.1093/jac/49.3.535
Yamaguchi, K., Domon, H., Miyazaki, S., Tateda, K., Ohno, A., Ishii, K., et al. (1998). In vitro and in vivo antibacterial activities of CS-834, a new oral carbapenem. Antimicrob. Agents Chemother. 42, 555–563. doi: 10.1128/AAC.42.3.555
Yan, S., Mendelman, P. M., and Stevens, D. L. (1993). The in vitro antibacterial activity of ceftriaxone against Streptococcus pyogenes is unrelated to penicillin-binding protein 4. FEMS Microbiol. Lett. 110, 313–317. doi: 10.1111/j.1574-6968.1993.tb06341.x
Yanagihara, K., Matsumoto, T., Tokimatsu, I., Tsukada, H., Fujikura, Y., Miki, M., et al. (2020). Nationwide surveillance of bacterial respiratory pathogens conducted by the surveillance committee of Japanese society of chemotherapy, the Japanese association for infectious diseases, and the Japanese society for clinical microbiology in 2016: general view of the pathogens’ antibacterial susceptibility. J. Infect. Chemother. 26, 873–881. doi: 10.1016/j.jiac.2020.05.006
Yang, Q., Zhang, H., Cheng, J., Xu, Z., Hou, X., and Xu, Y. (2015). Flomoxef showed excellent in vitro activity against clinically important gram-positive and gram-negative pathogens causing community- and hospital-associated infections. Diagn. Microbiol. Infect. Dis. 81, 269–274. doi: 10.1016/j.diagmicrobio.2015.01.001
Yanik, K., Guluzade, E., Bilgin, K., Karadag, A., Eroglu, C., Birinci, A., et al. (2015). Ceftaroline activity on certain respiratory tract and wound infection agents at the minimum inhibitory concentration level. J. Infect. Dev. Ctries. 9, 1086–1090. doi: 10.3855/jidc.6300
Yazawa, K., Mikami, Y., Uno, J., Otozai, K., and Arai, T. (1989). In-vitro activity of flomoxef, a new oxacephem group antibiotic, against Nocardia in comparison with other cephalosporins. J. Antimicrob. Chemother. 24, 921–925. doi: 10.1093/jac/24.6.921
Yocum, R. R., Waxman, D. J., Rasmussen, J. R., and Strominger, J. L. (1979). Mechanism of penicillin action: penicillin and substrate bind covalently to the same active site serine in two bacterial D-alanine carboxypeptidases. Proc. Natl. Acad. Sci. U.S.A. 76, 2730–2734. doi: 10.1073/pnas.76.6.2730
Yokoo, C., Goi, M., Onodera, A., Fukushima, H., and Nagate, T. (1991). Studies on cephalosporin antibiotics. IV. Synthesis, antibacterial activity and oral absorption of new 3-(2-substituted-vinylthio)-7 beta-[(Z)-2-(2-aminothiazol-4-yl)-2- (carboxymethoxyimino)acetamido]cephalosporins. J. Antibiot. 44, 1422–1431. doi: 10.7164/antibiotics.44.1422
Yu, K. W., and Neu, H. C. (1989). Antibacterial activity and beta-lactamase stability of MDL 19,592, an oral cephalosporin. Diagn. Microbiol. Infect. Dis. 12, 441–443. doi: 10.1016/0732-8893(89)90117-x
Zagursky, R. J., and Pichichero, M. E. (2018). Cross-reactivity in beta-lactam allergy. J. Allergy Clin. Immunol. Pract. 6, 72–81.e1. doi: 10.1016/j.jaip.2017.08.027
Zbinden, R., Punter, V., and von Graevenitz, A. (2002). In vitro activities of BAL9141, a novel broad-spectrum pyrrolidinone cephalosporin, against gram-negative nonfermenters. Antimicrob. Agents Chemother. 46, 871–874. doi: 10.1128/aac.46.3.872-875.2002
Keywords: hydrolysis, decomposition, chemical informatics, lactam antibiotics, antibiotic allergy (including penicillin and cephalosporin β-lactams), antimicrobial activity, deactivation
Citation: Turner J, Muraoka A, Bedenbaugh M, Childress B, Pernot L, Wiencek M and Peterson YK (2022) The Chemical Relationship Among Beta-Lactam Antibiotics and Potential Impacts on Reactivity and Decomposition. Front. Microbiol. 13:807955. doi: 10.3389/fmicb.2022.807955
Received: 02 November 2021; Accepted: 14 February 2022;
Published: 24 March 2022.
Edited by:
Rustam Aminov, University of Aberdeen, United KingdomReviewed by:
Krisztina M. Papp-Wallace, Louis Stokes Cleveland VA Medical Center, United StatesCopyright © 2022 Turner, Muraoka, Bedenbaugh, Childress, Pernot, Wiencek and Peterson. This is an open-access article distributed under the terms of the Creative Commons Attribution License (CC BY). The use, distribution or reproduction in other forums is permitted, provided the original author(s) and the copyright owner(s) are credited and that the original publication in this journal is cited, in accordance with accepted academic practice. No use, distribution or reproduction is permitted which does not comply with these terms.
*Correspondence: Yuri K. Peterson, cGV0ZXJzeUBtdXNjLmVkdQ==
Disclaimer: All claims expressed in this article are solely those of the authors and do not necessarily represent those of their affiliated organizations, or those of the publisher, the editors and the reviewers. Any product that may be evaluated in this article or claim that may be made by its manufacturer is not guaranteed or endorsed by the publisher.
Research integrity at Frontiers
Learn more about the work of our research integrity team to safeguard the quality of each article we publish.