- 1Shandong Research Center for Forestry Harmful Biological Control Engineering and Technology, College of Plant Protection, Shandong Agricultural University, Taian, China
- 2Research Institute of Forest Ecology, Environment and Protection, Chinese Academy of Forestry, Beijing, China
- 3Institute of Forestry New Technology, Chinese Academy of Forestry, Beijing, China
Botryosphaeriaceae, as a major family of the largest class of kingdom fungi Dothideomycetes, encompasses phytopathogens, saprobes, and endophytes. Many members of this family are opportunistic phytopathogens with a wide host range and worldwide geographical distribution, and can infect many economically important plants, including food crops and raw material plants for biofuel production. To date, however, little is known about the family evolutionary characterization, mating strategies, and pathogenicity-related genes variation from a comparative genome perspective. Here, we conducted a large-scale whole-genome comparison of 271 Dothideomycetes, including 19 species in Botryosphaeriaceae. The comparative genome analysis provided a clear classification of Botryosphaeriaceae in Dothideomycetes and indicated that the evolution of lifestyle within Dothideomycetes underwent four major transitions from non-phytopathogenic to phytopathogenic. Mating strategies analysis demonstrated that at least 3 transitions were found within Botryosphaeriaceae from heterothallism to homothallism. Additionally, pathogenicity-related genes contents in different genera varied greatly, indicative of genus-lineage expansion within Botryosphaeriaceae. These findings shed new light on evolutionary traits, mating strategies and pathogenicity-related genes variation of Botryosphaeriaceae.
Introduction
Dothideomycetes represents the largest and most important class of ascomycete fungi, including 23 orders, 110 families, 1,261 genera, and 19,000 species (Wijayawardene et al., 2017). The members of Dothideomycetes comprise both phytopathogenic (Ohm et al., 2012) and non-phytopathogenic fungi with diverse lifestyles (Ruibal et al., 2009) as well as many mycorrhizal fungi (Peter et al., 2016). Among these 110 families, Botryosphaeriaceae is an important and distinctive family. This is because it includes saprobes, endophytes, and phytopathogens, and it is one of the most widely geographically distributed groups of opportunistic plant pathogens. The host range of this family is very wide, and many economically important plants worldwide can be infected by them (Slippers and Wingfield, 2007). These pathogenic fungi can infect plants through wounds or natural openings, such as lenticels and stomata. Once they enter host tissues, they may survive as endophytes to stay at a biotrophic stage for a long time and turn into the destructive necrotrophic stage when the host is stressed (Yan et al., 2013; Morales-Cruz et al., 2015). The members of Botryosphaeriaceae can infect many woody plants and cause serious disease symptoms, such as dieback, branch canker, leaf spots, and fruit and seed rot (Marsberg et al., 2017). But the interaction of some of Botryosphaeriaceae fungi like Botryosphaeria dothidea (B. dothidea) with host plants includes a latent or endophytic phase, which makes the fungal infection easily be neglected (Bostock et al., 2014; Marsberg et al., 2017; Wang et al., 2018). Therefore, it is of substantial biological significance to explore the evolutionary characteristics of Botryosphaeriaceae at the level of Dothideomycetes.
The exceptional feature of Botryosphaeriaceae fungi is that it is difficult to observe their sexual structure under both natural and experimental conditions, but this does not mean that sexual reproduction does not occur for these fungi (Phillips et al., 2013). With more in-depth research being conducted, the mating strategies (homothallism or heterothallism) of increasing members of the Botryosphaeriaceae family have been reported (Bihon et al., 2012a,b, 2014; Billiard et al., 2012; Lopes et al., 2017, 2018), revealing some unresolved questions regarding their sexual reproduction. For example, how conservative are the nucleic acid and protein sequences of the mating type determination genes; how conservative are the genes and their arrangement at the mating type determination loci; how has the mating type evolved, and what is the origin type. In addition, the host range of different Botryosphaeriaceae fungi varies greatly. B. dothidea is a common pathogen with a wide range of hosts. Generally, the infection becomes symptomatic when the host is subjected to drought, physical damage, waterlogging, or freezing stress (Bostock et al., 2014; Marsberg et al., 2017; Wang et al., 2018). The symptoms primarily include canker on young seedlings, branches, and stems; the necrosis of branches; and fruit decay, which may lead to the death of the host in extreme cases (Kim et al., 2005; Tang et al., 2012). Botryosphaeria kuwatsukai (B. kuwatsukai) can also cause symptoms, such as fruit softening and decay, and severe canker of branches and stems (Wang et al., 2021). However, B. kuwatsukai has a relatively narrow host range and primarily infects apple and pear trees (Xu et al., 2015). Therefore, a systematic study of the mating strategies and differences of pathogenicity-related genes in Botryosphaeriaceae fungi will lead to a better understanding of their molecular evolutionary history and pathogenic characteristics.
Currently, genomics technology has been widely used to study many pathogenic fungi of plants, and greatly promoted the understanding of their evolution and pathogenic mechanisms (Islam et al., 2012; Blanco-Ulate et al., 2013; van der Nest et al., 2014; Nagel et al., 2018; Félix et al., 2019; Landi et al., 2020; Meile et al., 2020). For example, gene family expansion associated with virulence factors in wood-colonizing pathogenic fungi in the Botryosphaeriaceae was revealed via phylogenomic comparisons (Garcia et al., 2021); the comparative genome analyses of latent plant pathogens in the Botryosphaeriaceae were conducted to define their genomes (Nagel et al., 2021). In addition, a large-scale comparative genomic analysis can better reveal the physiological characteristics and evolutionary history of fungi (Guttman et al., 2014; Haridas et al., 2020; Miyauchi et al., 2020). Therefore, in this study, 167 Dothideomycetes fungi, including 19 Botryosphaeriaceae species, were fully sequenced, and a comparative genomics approach was used to comprehensively analyze the molecular evolution characteristics, mating strategies and pathogenicity within Botryosphaeriaceae fungi. The sequence differences of related genes benefit our understanding of the evolutionary history of Botryosphaeriaceae fungi and provide useful information for the prevention and control of the diseases caused by these fungi.
Materials and Methods
Fungal Strains
A total of 167 Dothideomycetes fungal strains, including 160 Botryosphaeriaceae fungi (19 species) (Supplementary Table 1), from the Fungal Strain Library of Shandong Agricultural University, Tai’an, China, were sequenced in this study. The sequencing data are found at NCBI (PRJNA777748).
Sequencing and Genome Assembly
The CTAB (hexadecyltrimethylammonium bromide) method (Murray and Thompson, 1980) was used to extract high-quality DNA. A 500 bp DNA fragment library was constructed, and PE150 sequencing was performed using Illumina HiSeq 4,000 (San Diego, CA, United States). The raw data obtained from sequencing were inputted into Trimmomatic v0.39 (Bolger et al., 2014) for quality control, and reads with an average quality of less than 30 were filtered. Jellyfish v2.3.0 (Marçais and Kingsford, 2011) was used to calculate k-mer distribution and GenomeScope v2.0 (Ranallo-Benavidez et al., 2020) was then used to assess genome size of each fungus. The assembly was carried out using SPAdes v3.13.1 (Bankevich et al., 2012).
Gene Prediction and Genome Annotation
RepeatModeler v2.0.11 was used to construct custome repeat libraries for each assembly, and RepeatMasker v4.1.12 was used to determine repeat contents with the custom repeat libraries. For ab initio gene prediction, GeneMark-ES v4.48_3.60 (Lomsadze et al., 2014) and AUGUSTUS v3.2.1 (Stanke and Morgenstern, 2005) were used with default parameters. First, GeneMark-ES was used to predict gene models, then the models were used to train AUGUSTUS. Exonerate v2.2.0 (Slater and Birney, 2005) was used for homology comparison prediction. Finally, MAKER v3.01.03 (Campbell et al., 2014) was used to predict protein-coding genes by combining the gene models from GeneMark-ES, AUGUSTUS, and Exonerate. BUSCO v4.1.4 was used to evaluate the completeness of genomes and genome annotations based on pezizomycotina_odb9 (3156 core ortholog genes) (Waterhouse et al., 2018). Functional annotations on the putative genes were performed using the following softwares: BLAST v2.8.1 + (Camacho et al., 2009) for the NCBI non-redundant protein (NR, 2020-06), SwissProt and FunSecKB2 databases (E-value threshold of 1E-05); HMMER v3.2.1 (Eddy, 2008) for the Pfam 32.0 and TransportDB 2.0 databases; KofamKOALA v1.2.0 (Aramaki et al., 2020) for KEGG annotation; and dbCAN2 (Zhang et al., 2018) for annotating carbohydrate active enzymes (CAZy).
Evolutionary Analysis of Botryosphaeriaceae Fungi
To construct an accurate evolutionary tree, eight outgroups (Supplementary Table 1) were selected and OrthoFinder v2.3.11 (Emms and Kelly, 2015) was used for gene family clustering. According to the clustering results, single copy orthogroups were extracted and aligned using MAFFT v7.427 (Katoh et al., 2002). These processed single copy orthogroups were then concatenated using a self-written Perl script and filtered using Gblock v0.91b (Castresana, 2000). PartitiosnFinder v2.1.1 (Lanfear et al., 2017) and RAxML v.8.2.2 (Stamatakis, 2014) were used to determine the optimal amino acid substitution model and build the Maximum Likelihood (ML) tree with 1,000 bootstrap replicates, respectively.
The known ecologies states (multistate and binary) and FUNGuild were used to determine the ecologies of each species (Nguyen et al., 2016). Mesquite v3.61 software was then used to infer ancestral ecological character states (Haridas et al., 2020).
Functional Enrichment Analysis of Botryosphaeriaceae
To find the differences in gene function annotation of Botryosphaeriaceae fungi with different lifestyles, scipy.stats package in Python was used to perform the two-tailed Fisher exact test for functional annotation with p-value threshold of 0.01. To reduce false positives, functional annotations with the number of genes less than 100 were filtered.
Mating Strategies of Botryosphaeriaceae
Mating Type Genes and Surrounding Genes
To determine the presence of mating type genes in the Botryosphaeriaceae fungal genome, the mating type genes of Diplodia sapinea (D. sapinea) and some neighboring genes (KF551229 and KF551228) (Bihon et al., 2012a) were used as templates to search homologous genes from the putative Botryosphaeriaceae fungal genes that has been generated in this study, using the partial alignment mode in BLASTx (Camacho et al., 2009). The mating type genes obtained were then inputted into the NCBI’s conserved domain database (Marchler-Bauer et al., 2015) to determine their functional domains.
Comparison of the Arrangement of Mating Type Loci
To compare the arrangement of mating type genes and their surrounding genes in the Botryosphaeriaceae fungal genome, a BLASTn alignment was conducted and then EasyFig version 2.2.2 (Sullivan et al., 2011) was used for collinearity analysis, with the E value threshold set to 1e–4.
Phylogenetic Comparison and Ancestral State Reconstruction
To study the mating strategies of Botryosphaeriaceae fungi, ML evolutionary trees of 24 Botryosphaeriaceae fungi were constructed with single-copy genes (using the same method described in section: Comparison of the Arrangement of Mating Type Loci). Briefly, OrthoFinder v2.3.11 (Emms and Kelly, 2015) was used to cluster gene families and extract single copy orthogroups. Each orthogroup was aligned and concatenated with MAFFT v7.427 (Katoh et al., 2002). Gblock v0.91b (Castresana, 2000) was used for filtering, and finally, RAxML v.8.2.2 (Stamatakis, 2014) was used to build an ML tree with the LG + I + G + F model. Mesquite v3.613 and the Mk1 likelihood model were used to reconstruct the evolutionary process of homothallism (hom) and heterothallism (het).
Changes in Genes Related to the Pathogenicity of Botryosphaeriaceae Fungi
The production of phytotoxic compounds in pathogenic fungi, such as secondary metabolite, secreted proteins, and carbohydrate-active enzymes, is one of the important infective weapons (Amselem et al., 2011). The genomes of Botryosphaeriaceae were searched for genes encoding the phytotoxic compounds and then the number difference of these genes was statistically analyzed as previously described (Wang et al., 2018).
Specifically, 23 Botryosphaeriaceae fungi (7 genera) and 10 other representative fungi were included to analyze changes in genes related to the pathogenicity in Botryosphaeriaceae. The 10 representative fungi contained 1 biotrophic fungus (Puccinia graminis), 2 necrotrophic fungi (Valsa mali and Pyrenophora triticirepentis), 2 saprophytic fungi (Neurospora crassa and Rhizopus oryzae), 3 hemibiotrophic fungi (Pyricularia oryzae, Colletotrichum higginsianum, and Zymoseptoria tritici), 1 symbiotic fungus (Laccaria bicolor), and 1 endophytic fungus (Peltaster fructicola).
Results
Genome Sequencing, Assemby, and Annotation
In this study, 167 genomes of Dothideomycetes, including 160 Botryosphaeriaceae (19 species), were sequenced, assembled, and annotated (Supplementary Table 1). All genomes were sequenced at average coverage 166 ± 48 x. The average assembled genome lengths of 167 Dothideomycetes ranged between 28.85 and 61.78 Mb, which were consistent with the sizes estimated by k-mer counting approach. The contig N50 values of assembled genomes varied from 41.81 to 779.02 kb with a mean of 241.77 kb. The repeat contents of assembled genomes varied from 0.95 to 10.98% with a mean of 5.18%. All assembled genomes have a high completeness with an average of 94.8 ± 4.3%, and the similar result was also found in genome annotations (average of 98.0 ± 2.9%).
Classification Based on Whole-Genome Data
To better understand the evolutionary characteristics of Botryosphaeriaceae, we used 167 newly sequenced in this study and 112 genomes available in public database to construct a whole-genome phylogenetic tree using 480 single-copy gene families. The phylogenetic tree was clearly divided into 2 clades, corresponding to 2 subclasses, namely Pleosporomycetidae and Dothideomycetidae (Supplementary Figure 1). A comprehensive phylogenetic analysis revealed that the members of Botryosphaeriaceae that belonged to Botryosphaeriales were added to Pleosporomycetidae (Figure 1 and Supplementary Table 2).
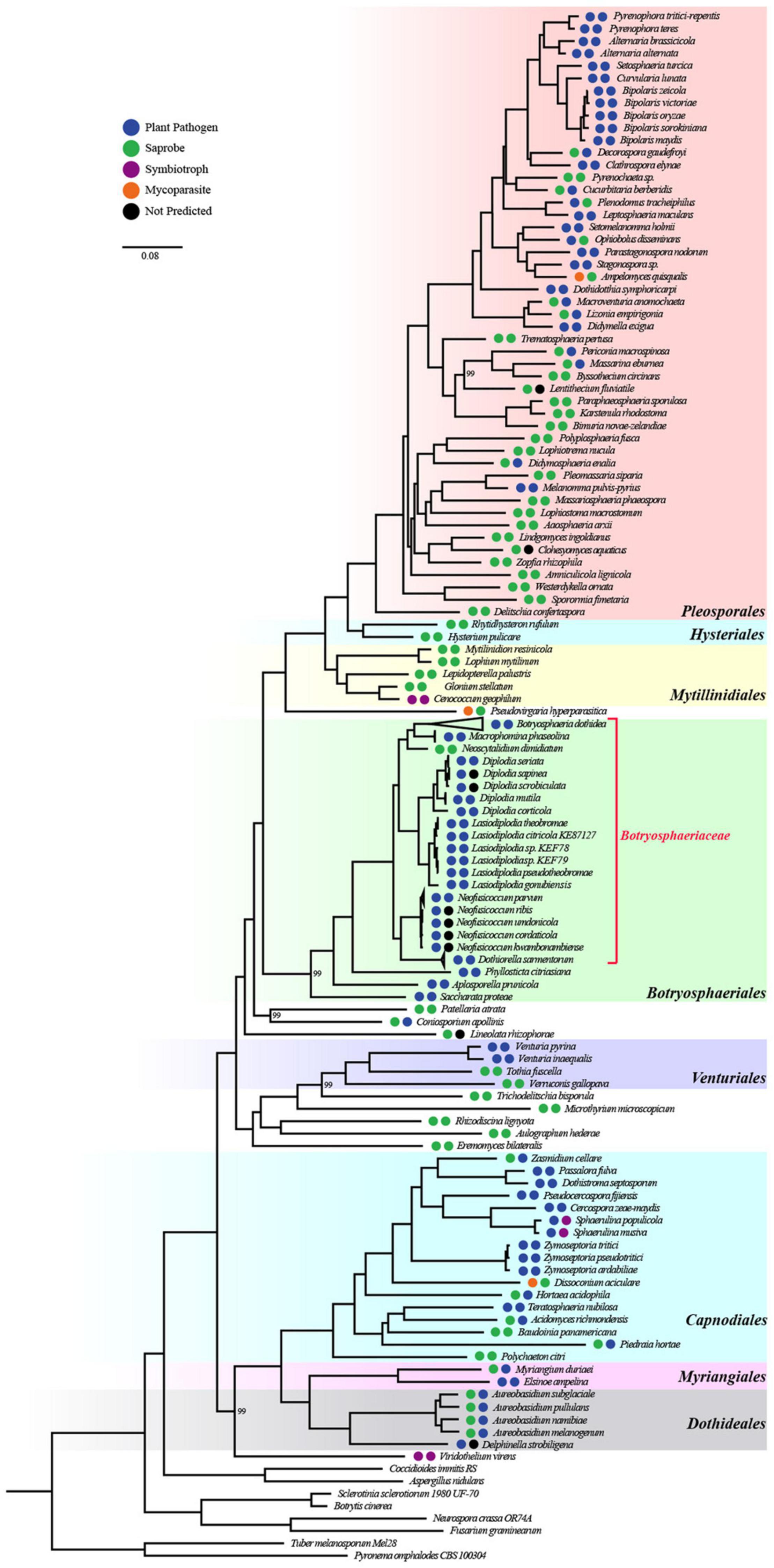
Figure 1. Whole-genome-based phylogenetic tree of 271 species from Dothideomycetes and 8 outgroups. All bootstrap values are 100% except for those shown. The orders of Dothideomycetes were well classified and were displayed by different colors. The two circles left of species names standed for lifestyle classification according to organism data and FunGuild, respectively.
Saprophytic Fungi Have a Larger Genome Size Than Phytopathogenic Fungi
The genome sizes of Dothideomycetes ranged from 17 (Piedraia hortae) to 177 Mbp (Cenococcum geophilum), and 7,896–34,881 protein-coding genes were detected (Supplementary Figures 2, 3 and Supplementary Table 1). Compared with non-pathogenic fungi, pathogenic fungi usually have a smaller genome (Supplementary Figure 2). The genome size of Botryosphaeriaceae is in the range of 28.85 (Aureobasidium pullulans) −61.78 Mbp (Dothiorella sarmentorum) (47.19 Mbp on average), and 11,505–16,851 proteion-coding genes were predicted (13,765 genes in average).
Saprophytic Fungi Are the Possible Evolutionary Ancestors of Botryosphaeriaceae Fungi
To infer the ecological characteristics of ancestors of Botryosphaeriaceae fungi, we analyzed their lifestyle evolution process at the class level. The results showed that the ancestral lifestyle was likely to be the saprophytic type (Figure 2), which is also supported by the maximum likelihood analysis (Supplementary Figure 4 and Supplementary Table 3). During the evolution of Dothideomycetes fungi, at least 6 transitions from non-phytopathogenic (NPP) to phytopathogenic (PP) were detected, including 4 major transitions, which are presented at the MRCA node of Mycosphaerellaceae (Dothideomycetidae) (Node 225: NPP = 0.1868, PP = 0.8132), Venturia (Pleosporomycetidae) (Node 189: NPP = 0.0039, PP = 0.9961) and Botryosphaeriales (Node 20: NPP = 0.0984, PP = 0.9016), along with the branching point of Setomelanomma-Bipolaris (Node 98: NPP = 0.0074, PP = 0.9926). In addition, the Botryosphaeriaceae fungi have undergone at least 3 transitions from saprophytic to pathogenic fungi, including Node 176 (NPP = 0.9859, PP = 0.0141), Node 180 (NPP = 0.9992, PP = 0.0008) and Node 246 (NPP = 0.9101, PP = 0.0899) (Supplementary Table 3).
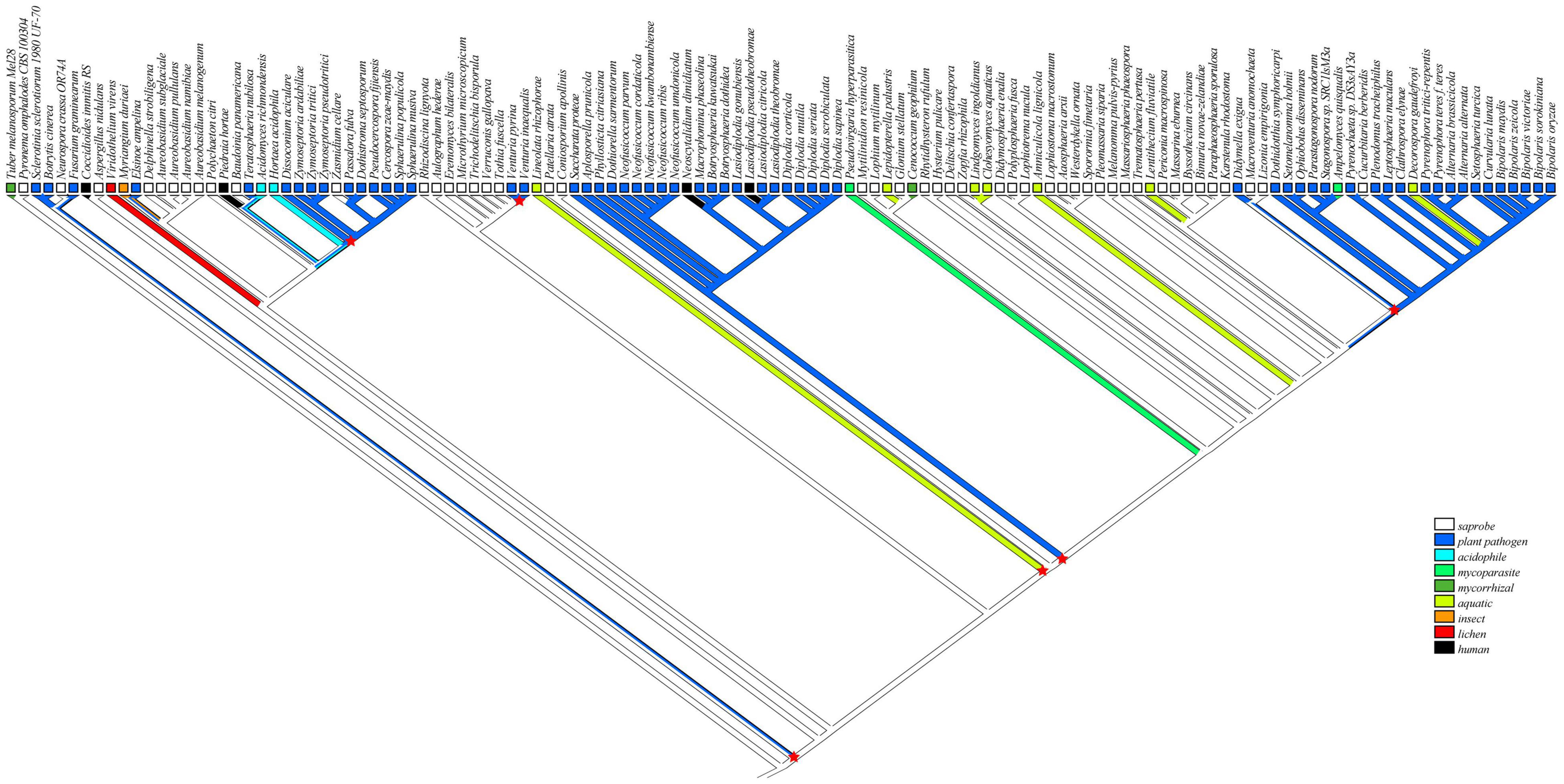
Figure 2. Reconstruction of ancestral lifestyle character state of Dothideomycetes using Mesquite based on parsimony model as saprobe. Major lifestyle shifts were marked by six red star symbols.
Differences in the Gene Families of Plant Pathogenic and Saprophytic Fungi
To investigate the differences between plant pathogenic and saprophytic fungi from the perspective of gene family contractions and expansions, we used Fisher’s exact test to perform these analyses on gene families. Differences in 78 Pfam and 58 GO annotations were found between saprophytic and plant pathogenic fungi. In the saprophytic fungi, 42 Pfam and 35 GO terms showed more than 20% of expansion, and 31 Pfam and 14 GO terms displayed more than 20% of contraction. In plant pathogenic fungi, 32 Pfam and 17 GO terms showed greater than 20% of expansion, and 35 Pfam and 21 terms displayed more than 20% of contraction. Compared with saprophytic fungi, the plant pathogenic fungi contained more gene families that showed contraction. Compared with saprophytic fungi, 94 Pfam and 67 GO terms of Botryosphaeriaceae fungi showed greater than 20% of expansion, while 52 Pfam and 44 GO terms displayed contractions (> 20%) (Supplementary Table 4).
Mating Strategies of Botryosphaeriaceae Fungi
Mating Type Genes
To determine the mating type genes, MAT1-1 and MAT1-2 of 24 fungi (7 genera and 19 species) were analyzed in the Botryosphaeriaceae family. The results showed that the Botryosphaeria, Neofusicoccum, and Dothiorella genomes harbored both MAT1-1 and MAT1-2, indicating that they were homothallic. Diplodia, Macrophomina, and Neoscytalidium genomes harbored either MAT1-1 or MAT1-2, indicating that they were heterothallic fungi. Lasiodiplodia fungi had various mating strategies, including homothallism (L. gonubiensis) and heterothallism (Lasiodiplodia citricola, L. pseudotheobromae, and L. theobromae) (Supplementary Table 5). In addition, the protein domains of MAT1-1-1 and MAT1-2-1 were highly conservative. All the MAT1-1-1 proteins contain MATalpha domains, and all the MAT1-2-1 proteins contain MAT_HMG-box domains (Supplementary Table 6). Compared with the neighboring genes, the nucleic acid sequences of the mating type genes were poorly conserved (Supplementary Table 7), while the length of the coding sequence and the position and size of the intron were largely conservative.
Arrangement of Mating Loci
Arrangement analysis of mating loci showed that there were three types of arrangements at the mating type determining loci of Botryosphaeriaceae (Figure 3). In most Botryosphaeriaceae genomes (e.g., Diplodia sapinea), the MAT1 gene was primarily located between a collinear region that contains 4 protein-coding genes and 1 putative integral membrane (PIM) protein (Figure 3). The PIM contains pleckstrin homology domains and DUF2404. The four genes in the collinear region encode, in order, a DNA lyase (APN2), cytochrome c oxidase subunit VIa (CoxVIa), anaphase-promoting complex subunit 5 (APC5), and complex I intermediate-associated protein 30 (CIA30). According to the positional relation between the MAT1 and APN2 genes (the MAT1 gene was located at the proximal end of APN2), the second type of arrangement was observed in Botryosphaeria, L. gonubiensis (Lasiodiplodia), M. phaseolina (Macrophomina), and N. dimidiatum (Neoscytalidium). For example, the MAT1 gene of M. phaseolina and B. dothidea was located upstream of the APN2 gene, while in L. gonubiensis and N. dimidiatum, the MAT1 gene was located downstream of the APN2 gene, and the four genes in the collinear region were arranged in reverse order. However, the opposite arrangement was observed in the two strains of B. kuwatsukai-the MAT1 gene of the PG2 strain was located downstream of the APN2 gene, while LW030101 was located upstream. A similar type of reversed arrangement as the one found for B. kuwatsukai was also identified in two B. dothidea strains. The third type of arrangement was primarily observed for Neofusicoccum species in which the two subtypes of MAT1, MAT1-1, and MAT1-2, were not located in conjunction; they were either located both distantly from the collinear region or at different chromosomes (or scaffolds) (Figure 3).
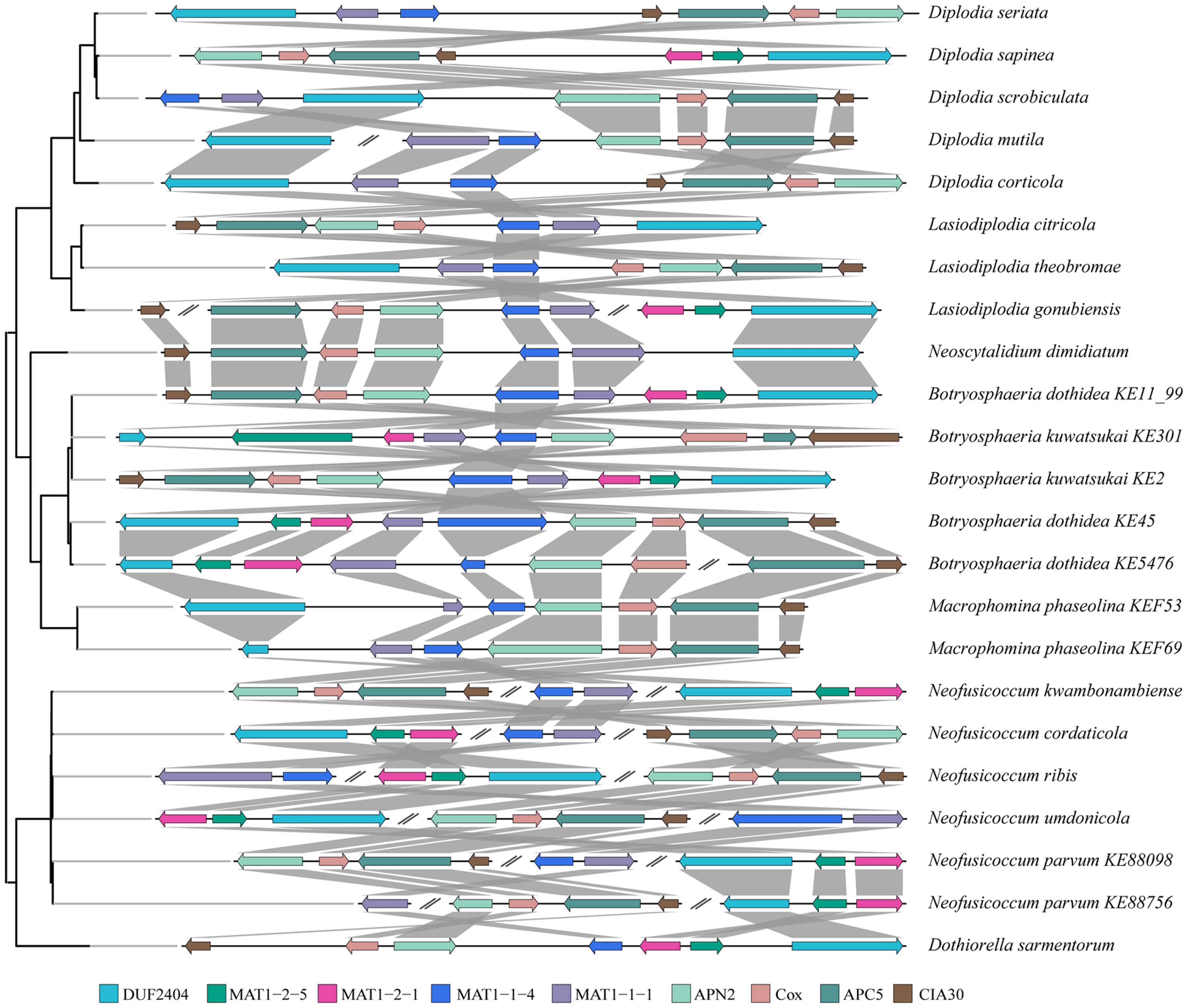
Figure 3. Pairwise mating type and surrounding genes comparison between species of Botryosphaeriaceae. Genes (color coded arrows) were on genomic sequences (horizontal lines). Organization of genes were indicated by gray box. Abbreviations of genes: putative integral membrane protein containing DUF2404 domain (DUF2404), DNA lyase (APN2), cytochrome C oxidase subunit Via (Cox), anaphase-promoting complex subunit 5 (APC5) and Complex I intermediate-associated protein 30 (CIA30).
Reconstruction of the Ancestral State of the Mating Strategy
To understand the evolutionary characteristics of the mating type of Botryosphaeriaceae, we selected 24 representative Botryosphaeriaceae strains to reconstruct the evolutionary process of homothallism and heterothallism mating strategies in Botryosphaeriaceae (Supplementary Figure 5). Our analysis showed that het mating was likely to be the ancestral type (Node 2, het = 0.6198, hom = 0.3802) (Supplementary Figure 5 and Supplementary Table 8). During the course of evolution, Botryosphaeriaceae fungi have undergone at least 2 major transitions to their hom strategy. The first was located at the branching point of Lasiodiplodia theobromae and L. citricola (Node 14: het = 0.8291, hom = 0.1709), and the other transition was primarily observed at the branching point of Neofusicoccum and Dothiorella (Node 26: het = 0.4344, hom = 0.5656) (Supplementary Figure 5 and Supplementary Table 8).
Changes in Genes Related to the Pathogenicity of Botryosphaeriaceae
Secondary Metabolism
To find the key enzymes involved in the synthesis of secondary metabolites in Botryosphaeriaceae fungi, we used Pfam annotations to find 3 types of genes that encode these key enzymes, such as polyketide synthase (PKS), non-ribosomal peptide synthetase (NRPS), and dimethylallyl tryptophane synthase (DMATS). The PKS genes of Botryosphaeriaceae fungi differed significantly from each other (p = 2.0 × 10–16) (Figure 4A). Among all the species, the Macrophomina fungi contained the largest number of PKS genes (31 on average), while the Dothiorella fungi contained the least number (8 on average) (Supplementary Table 9). The genes related to secondary metabolites in fungi also included those that encode cytochrome P450 enzymes, regulatory factors, and transporters. Cytochromes P450 can catalyze the transformation of hydrophobic intermediates in the primary and secondary metabolic pathways and plays an important role in fungi. Compared with other fungi, Botryosphaeriaceae fungi contained the largest number of genes that encode cytochromes P450 (133-267). Among all the species, Neofusicoccum fungi contained the highest number of cytochromes P450 (267), followed by Botryosphaeria (n = 251) (Figure 4B). The ATP-binding cassette (ABC) or major facilitator superfamily (MFS) gene families played important roles in the transport of secondary metabolites. The numbers of ABC or MFS in Botryosphaeriaceae fungi were higher than those of other species (ABC: 268-353; MFS: 16-62). Among all the species, Botryosphaeria fungi contained the largest number of MFS (n = 62), while Neoscytalidium dimidiatum fungi contain the least amount (n = 16) (Figure 4B).
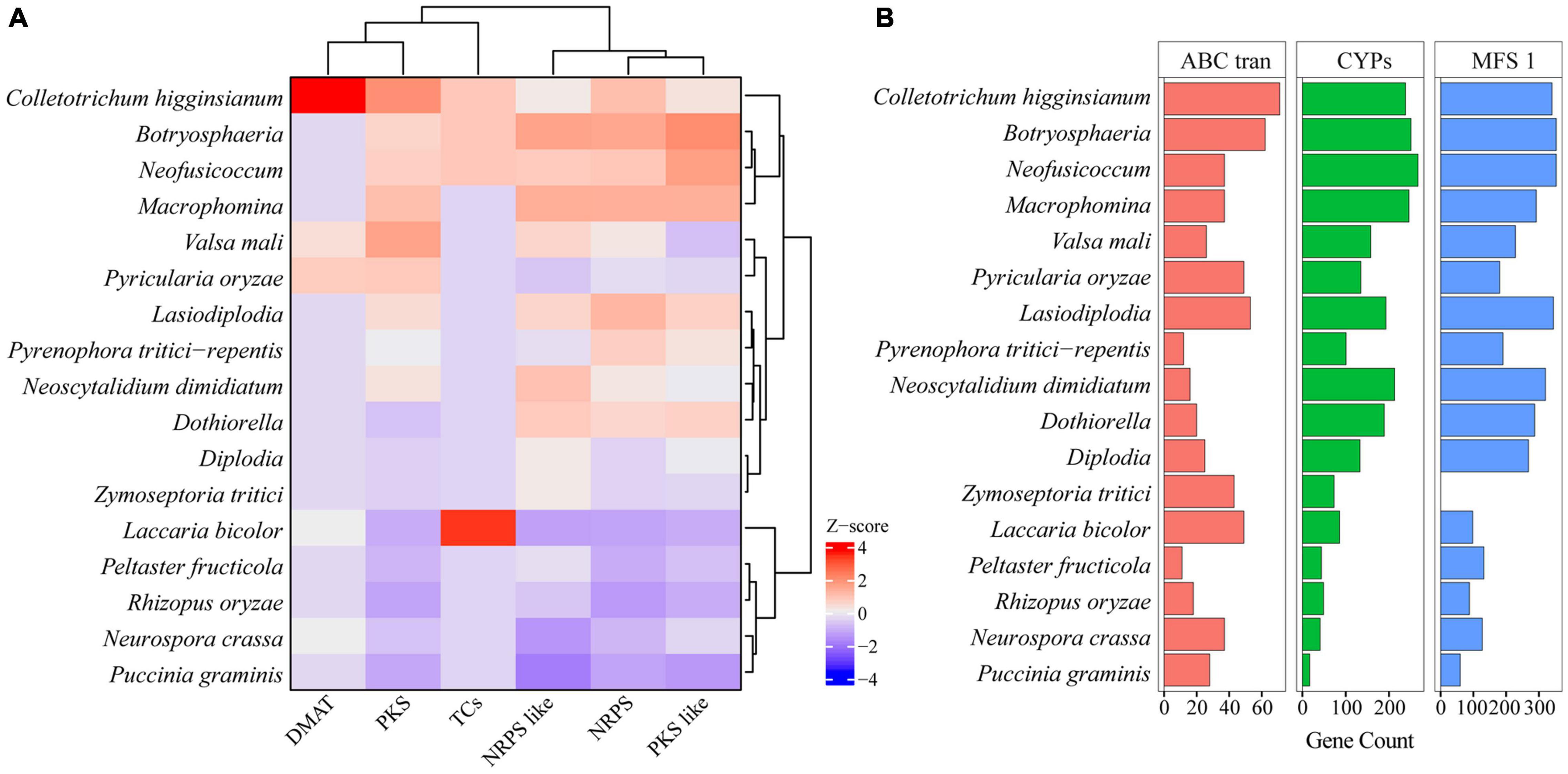
Figure 4. Comparing backbone and related genes of secondary metabolism among Botryosphaeriaceae fungi and other 10 fungal species. (A) Key gene family of secondary metabolism. In each column, Z-score was used to describe the trend of over-represented (+ 4–0) and down-represented (0 to −4) gene family. (B) Gene family number comparison of MFS_1, ABC_tran and CYPs among Botryosphaeriaceae fungi and other 10 fungal species.
Secreted Proteins
Pathogens can secrete a battery of proteins, which are deployed to the host-pathogen interface during infection, and these secreted proteins played important roles in fungal pathogenicity (O’Connell et al., 2012; Fernandes et al., 2014; Félix et al., 2016). In this study, we predicted the secreted proteins of Botryosphaeriaceae (Figure 5). The number of secreted proteins in Botryosphaeria (the B. dothidea fungi contain 1,034, and the B. kuwatsukai fungi contain 939) was not significantly different from Macrophomina (n = 1,118), and the B. kuwatsukai fungi seemed to secrete less proteins. The number of proteins secreted by the Neofusicoccum fungi varied greatly (922–1,028). Among all the species, the N. parvum fungi contained the largest number (n = 1,028), and N. cordaticola fungi contained the least (n = 922). Compared with other fungal genera, the Diplodia genus contained fewer secreted proteins (671–866) (Figure 5 and Supplementary Table 10).
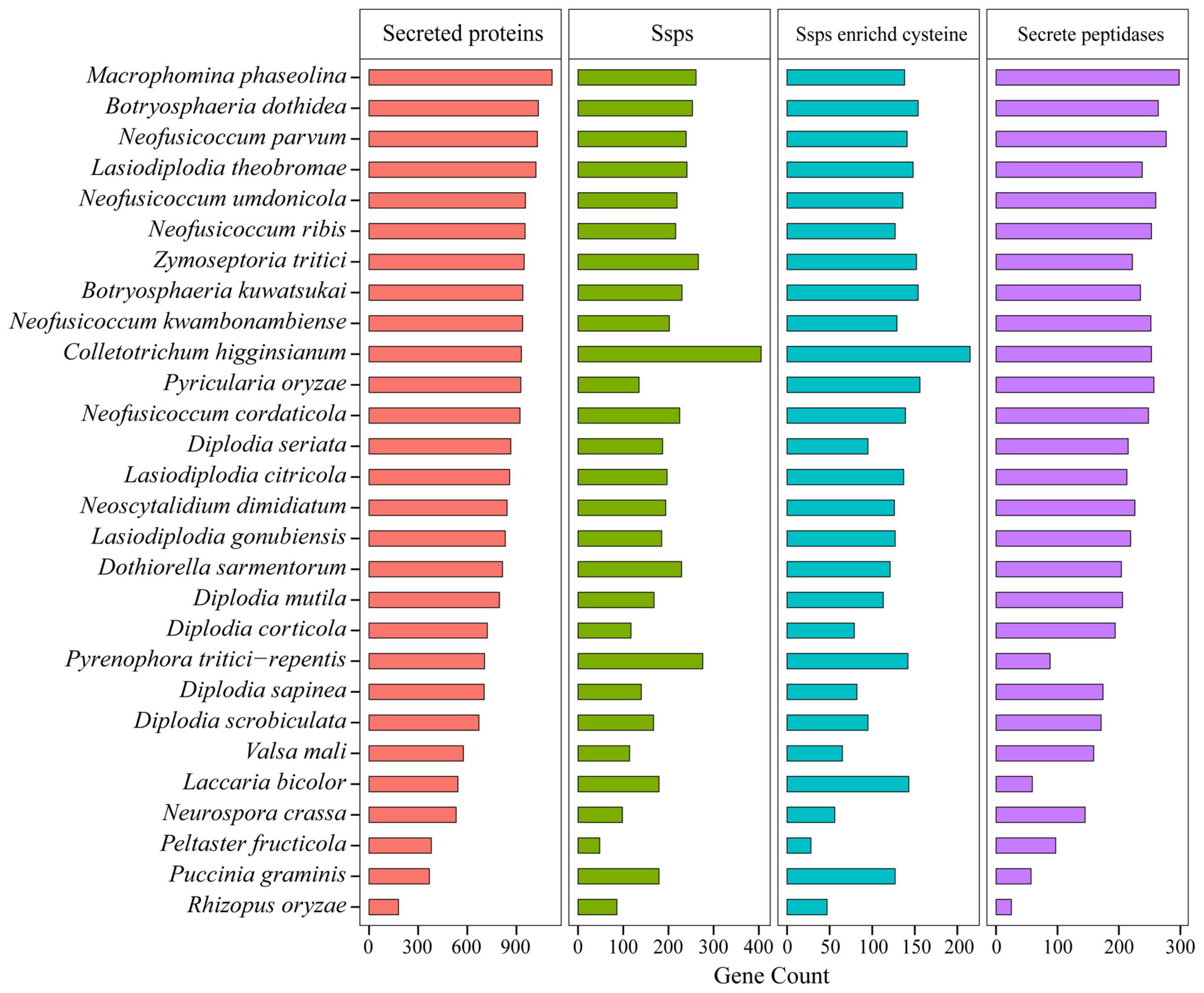
Figure 5. Comparison of secreted proteins among Botryosphaeriaceae fungi and other 10 fungal species.
The transfer of secreted effector proteins to host plant cells was the key to pathogenesis of many plant pathogenic microorganisms. Secreted proteins less than 200 amino acids in length and rich in cysteine were considered as candidate secreted effectors (Wang et al., 2018). The Botryosphaeriaceae family contained two genera Macrophomina and Botryosphaeria that may release a large number of secreted proteins, and the number of small, secreted proteins of these two genera was also higher than that of other genera. For example, M. phaseolina (261), B. dothidea (253), and B. kuwatsukai (230) displayed significant expansions of gene families. Small secretory proteins rich in cysteine were the most abundant in Botryosphaeria fungi and the least in Diplodia fungi (79-113) (Figure 5 and Supplementary Table 10). In Botryosphaeria, both B. dothidea and B. kuwatsukai contained 154 small secretory proteins rich in cysteine, and the Pfam annotations of them included 19 and 21 known functional domains, respectively (Supplementary Table 11). By comparing the Pfam annotations of B. dothidea and B. kuwatsukai secretory proteins, we found that ribonuclease, the cerato-platanin family, and cysteine-rich secretory protein (CAP) family were only present in B. kuwatsukai, while the cell wall integrity and stress response component (WSC) domain, a putative carbohydrate binding domain was only present in B. dothidea (Supplementary Table 11).
Pathogenic and saprophytic fungi can secrete peptidases into their surroundings to degrade a variety of host-related proteases (Wang et al., 2018). This degradation mechanism has potential benefits in eliminating the activity of antifungal host proteins and providing nutrients. Compared with other fungi, Botryosphaeriaceae fungi contained a higher number of secretory peptidases. Among all the species, the M. phaseolina (Macrophomina) fungi contained the largest number of secretory peptidases (n = 298), followed by B. dothidea (n = 264) and B. kuwatsukai (n = 235), but both were similar to the semi-biotrophic phytopathogenic pathogens C. higginsianum (n = 233) and P. oryzae (n = 240). Diplodia fungi contain the lowest number of secreted proteins (n = 192; p = 0.8 × 10–3) (Figure 5 and Supplementary Table 10).
Carbohydrate Active Enzymes
The ability to degrade complex carbohydrates in plants is an important aspect of the lifestyle of phytopathogenic fungi (Wang et al., 2018). Compared with other species, the fungi in Botryosphaeriaceae family contained a higher number of carbohydrate active enzyme-related gene families, such as genes that encode glycoside hydrolases (GHs), polysaccharide lyases (PLs), carbohydrate esterases (CEs), auxiliary activities (AAs), and carbohydrate binding modules (CBMs). Some representative species include N. parvum (753), B. dothidea (750), N. kwambonambiense (724), L. theobromae (719), N. ribis (716), N. umdonicola (716), and N. cordaticola (709). Within the Botryosphaeriaceae family, N. parvum has the largest number of carbohydrate active enzyme-related gene families (753), followed by B. dothidea (750), and B. kuwatsukai (675). Most Diplodia fungi contain fewer carbohydrate activity enzyme-related gene families, such as D. scrobiculata (522), D. corticola (548), D. mutila (577), and D. sapinea (556). However, for some species, such as D. seriata (662), there was a small increase in the number (Figure 6A and Supplementary Table 12).
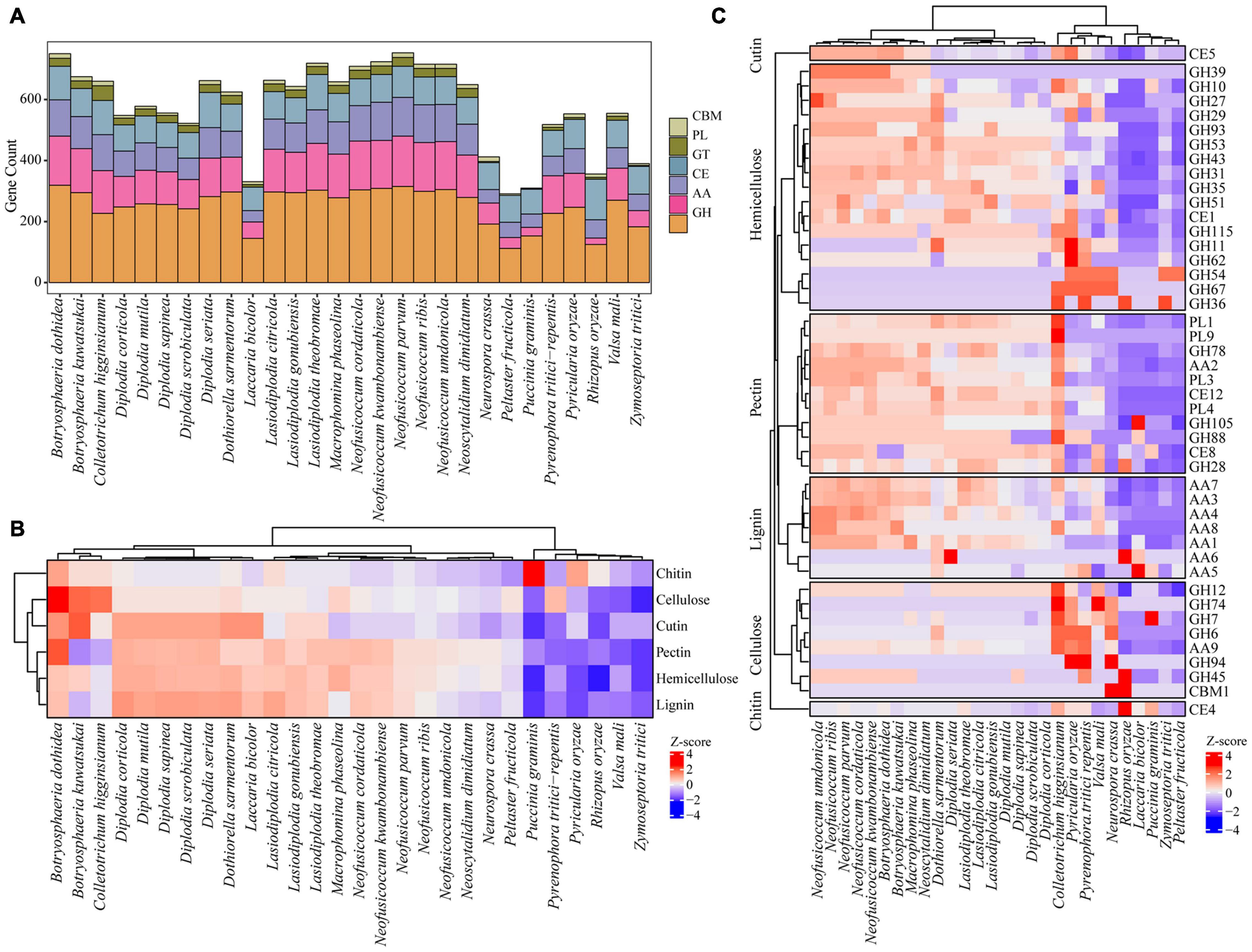
Figure 6. Comparing CAZy among Botryosphaeriaceae fungi and other 10 fungal species. (A) Six CAZy classes: CBMs, Carbohydrate-Binding Modules; PLs, Polysaccharide Lyases; GTs, Glycosyl Transferases; CEs, Carbohydrate Esterases; AAs, Auxiliary Activities; GHs, Glycoside Hydrolases. (B) Distribution of CAZy related to cellulose, hemicellulose and lignin degradation. (C) Comparison of selected enzymes involved in PCW (plant cell wall) degradation among Botryosphaeriaceae fungi and other 10 fungal species. In heatmaps, Z-score were used to describe over-represented (+ 4–0) and down-represented (0 to −4) gene family.
The plant cell wall is a complex network structure composed of different polysaccharides, including cellulose, hemicellulose, pectin, and lignin (Terrett and Dupree, 2019). Proteins encoded by fungal carbohydrate active genes and related auxiliary genes can degrade plant cell walls into simple monomers that are absorbed as the carbon source to provide energy for fungi (Islam et al., 2012; Wang et al., 2018). Compared with other fungi, those of Botryosphaeriaceae contain expanded gene families involved in the degradation of lignin, cellulose, hemicellulose, and pectin. For example, the gene family involved in the degradation of lignin (107 genes in average) was significantly larger than that in other species (54 genes in average; p = 2.0 × 10–4, t-test). The number of gene families involved in the degradation of hemicellulose and pectin also expanded significantly, with 67 vs. 34 (p = 2.0 × 10–3) and 70 vs. 32 (p = 2.0 × 10–3), respectively. Among the Botryosphaeriaceae fungi, compared with other genera, it was found that Neofusicoccum fungi contain the largest number of gene families involved in the degradation of plant cell walls (287–329), followed by Botryosphaeria (219–314) and Macrophomina (291–306), while Diplodia fungi contain the least number of gene families (215–259) (Figures 6B,C and Supplementary Table 13).
Discussion
In this study, we conducted a large-scale whole-genome sequencing of 167 Dothideomycetes fungi, including 19 species, and comprehensively analyzed evolutionary traits, mating strategies and changes in pathogenic genes in Dothideomycetes fungi. Our results can advance our understanding of the evolutionary history of Botryosphaeriaceae fungi.
Our results also confirmed that the 167 Dothideomycetes fungi can be divided into two subclasses, Pleosporomycetidae and Dothideomycetidae, and Botryosphaeriaceae belongs to Pleosporomycetidae, indicating that it is accurate to classify fungi using phylogenies based on phylogenomics (Haridas et al., 2020). Our results inferred the ancestral lifestyle of Botryosphaeriaceae fungus as saprobe, and these fungi have undergone at least three transitions from saprophytic to phytopathogenic states. This result is consistent with Schoch et al. (2009). They inferred that the Dothideomycetes fungi have experienced multiple transitions from saprophytic pathogens to lichens to phytopathogens, along with multiple transitions from terrestrial to aquatic lifestyles (Schoch et al., 2009). In addition, a larger genome size and a higher number of protein-coding genes are usually associated with saprophytic fungi compared with phytopathogenic fungi. This result is consistent with the findings of Schuelke et al. (2017). They found that in the Geosmithia genus, compared with non-pathogenic fungi, the pathogenic fungi in this family have smaller genomes (Schuelke et al., 2017).
Previous studies have shown that phytopathogenic fungi have a smaller genome and contain fewer protein-coding genes compared with saprophytic fungi, which is related to the expansion and contraction of gene families (Ohm et al., 2012; Haridas et al., 2018). In this study, many gene families of phytopathogenic fungi showed contractions, primarily including genes which contain the Pfam domain tetratricopeptide repeat (TPR). TPR can interact with a variety of proteins, such as the anaphase-promoting complex, NADPH oxidases, and HSP90-binding proteins (Das et al., 1998; Kaneko et al., 2006; Kondo et al., 2010). The TPR protein is also part of the plant hormone signaling pathways (Schapire et al., 2006). The family enrichment results found that multiple members of the TRP family proteins (TPR 1, 3, 8, 10, 11, 12, 16, and 19) contracted in phytopathogenic fungi. This contraction may be due to a reduction of signal related TPR proteins in pathogenic fungi. This is because during the process of pathogenic fungal infection, fungal signal related TPR proteins will be affected by plant hormone signals (Haridas et al., 2020). Some other Pfam domains also contracted significantly in phytopathogenic fungi, including PF17111 (the fungal N-terminal domain of STAND proteins), PF05729 (the NACHT domain), and PF14479 (involved in prion inhibition and propagation). These domains are in heterokaryons and play an important role in incompatibility (Paoletti and Saupe, 2009; Greenwald et al., 2010; Daskalov et al., 2012). The contraction of Pfam domains associated with incompatibility in the heterokaryons suggests that other strategies to reduce the level of incompatibility in heterokaryons also exist to improve the adaptability of pathogenic fungi (Ishikawa et al., 2012). Simultaneously, compared with saprophytic fungi, many GO terms are contracted in phytopathogenic fungi, including protein kinases (GO: 0004672), transcription factors (GO: 0003700), zinc ion binding (GO: 0008270), and regulation of nitrogen utilization (GO: 0006808). Given the complexity of amino acid biosynthetic pathways and energy requirements, fungi rely on the absorption of plant amino acids to conserve their own energy. This could be one of the reasons for the contraction of GO terms in plant pathogens (Staats et al., 2013; Mur et al., 2017).
Botryosphaeriaceae fungi display both types of mating strategies, homothallism and heterothallism, which are determined by the single locus MAT1 (Mur et al., 2017). In this study, the mating type genes of 24 Botryosphaeriaceae fungal isolates (19 species) were analyzed, and we found that these genes were not highly conserved in terms of their nucleotide and amino acid sequences. However, for two subtype genes, two domains, MATalpha_HMGbox (MAT1-1-1) and MATA_HMG-box (MAT1-2-1), are more conservative. This is consistent with the fact that the mating type genes of fungi have almost no differences within a species, but they are highly divergent between species (Turgeon, 1998). In many ascomycete fungi, it is a conservative trend that the MATalpha and MATA_HMG-box regions contain introns (Arie et al., 2000; Paoletti et al., 2005; Duong et al., 2013; de miccolis et al., 2016). In D. sapinea and D. seriata, the introns in the MATA_HMG-box region of the MAT1-2-1 gene were lost, but the amino acid sequences that flank the lost intron site remain intact (Nagel et al., 2018). This phenomenon is consistent with the intron loss model derived from Poly-A primed mRNA (Sharpton et al., 2008). In this study, with the exception of Neofusicoccum, the arrangement of MAT1-1 and MAT1-2 genes is highly conserved in six other genera. They all are located between a collinear region that contains four protein-coding genes and one PIM protein, which is the same arrangement as found in most Phyllostictaceae fungi (Bihon et al., 2014; Wang et al., 2016). The arrangement of mating type genes of Neofusicoccum fungi is exceptional. The locations of the two subtypes of mating type genes are not in conjunction; they are either both located distantly from the collinear region or at different chromosomes (or scaffolds) (Lopes et al., 2017). Similarly, this discontinuous arrangement of mating type genes also exists in Aspergillus nidulans (Galagan et al., 2005), Curvularia cymbopogonis (Galagan et al., 2005), and Neosartorya fischeri (Rydholm et al., 2007).
The mating type gene MAT1 has two subtypes—MAT1-1 and MAT1-2. When two subtypes are both harbored in the genome, the mating strategy is hom, and if only one is present, it is het (Idnurm, 2011). The repetitive sequence-mediated deletion of one or more mating type genes can cause non-directional changes in mating types, such as the transition from self-fertility to self-sterility (Wilken et al., 2014; Xu et al., 2016; Yun et al., 2017). Such repetitive sequences were not observed in this study. Therefore, Botryosphaeriaceae fungi are unlikely to undergo a non-directional change regarding mating types (Nagel et al., 2018). In addition, to further understand the evolutionary characteristics of the fungal mating type in Botryosphaeriaceae, we reconstructed the ancestral state of the Botryosphaeriaceae fungal mating type and found that the het mating strategy is the ancestral type. Moreover, the fungi in this family experienced a number of transitions to the homothallism strategy, a shift that is common in ascomycete fungi (Inderbitzin et al., 2005; Yokoyama et al., 2006; Nygren et al., 2011; Gioti et al., 2012).
In this study, many gene families of the Botryosphaeriaceae fungi have shown significant expansions and contractions, and this change is conducive to the adaptation of fungi to the living environment (Alkan et al., 2013). These contracted gene families include genes that encode secondary metabolite synthases, secreted proteins, and carbohydrate active enzymes. In the family of genes that encode secondary metabolite synthases, NPRS and PKS gene clusters are responsible for the synthesis of toxic peptides and the production of naphthalenone pentaketides, respectively, in Botryosphaeriaceae fungi (D. seriata, L. theobromae and N. parvum) and other pathogenic fungi (A. fumigatus, Diaporthe ampelina, Phaeomoniella chlamydospora, and Togninia minima) (Andolfi et al., 2011; Paolinelli-Alfonso et al., 2016). Although the gene clusters of secondary metabolites are not regulated during infection, a large number of products of these gene clusters, which are significantly expanded in the genome, may be involved in the induction of disease symptoms and host adaptation (Yan et al., 2018). Cytochrome P450 is a superfamily of monooxygenases. In addition to participating in the post-synthesis modification of a variety of metabolites, it can also promote the adaptation of fungi to specific ecological niches by altering potentially harmful chemicals in the environment (Siewers et al., 2005). Here, we found the expansion of this gene family, which can explain the wide host range of Botryosphaeriaceae fungi. This is because these genes are also associated with some physiological characters; thus, their expansions are likely to promote pathogenic evolution (Nierman et al., 2005). In this study, expansions are obvious for the genes that encode ABC transporters in Botryosphaeriaceae fungi, indicating that these fungi have evolved stronger virulence and capacity against plant defense compounds (Han et al., 2001; Luini et al., 2010). Sugar synergistic transporters belong to the MFS family, and they play important roles in fungal spore formation, intercellular communication, and pathogenicity (Gaur et al., 2008). In the Botryosphaeriaceae fungi, we found that the sugar synergistic transporter gene family showed different degrees of expansion, which probably makes it more adaptive to different host environments (e.g., different pH values), and more resistant when interacting with various plant pathogens (Zhang et al., 2012; Yin et al., 2015).
Previous studies have showed that secreted proteins play important roles in the infection process of pathogenic fungi (Cobos et al., 2010; O’Connell et al., 2012; Fernandes et al., 2014; Félix et al., 2016, 2019). In this study, the secretory protein gene families of Botryosphaeriaceae fungi have expanded to different degrees, but there are large differences between different genera, which may be related to the different infection ranges of Botryosphaeriaceae fungi (Wang et al., 2018). In addition, the gene families of carbohydrate active enzymes in Botryosphaeriaceae fungi also showed different degrees of expansion. Among these families, the glycoside hydrolase family GH33 is composed of sialidase, which can hydrolyze the glycosidic bonds of terminal sialic acid residues in oligosaccharides. Sialidase can function as a pathogenic factor, facilitating the adaption to the host by evading host recognition or inhibiting host defense responses (Alviano et al., 2004). The cell wall of most dicotyledonous plants is composed of approximately 35% pectin. Pectin-degrading enzymes contribute to the degradation of the cell wall. This local degradation of the cell wall is necessary for fungi to enter the plant cytoplasm and replicate in the host tissue (ten Have et al., 1998). Similar to the highly pathogenic Colletotrichum higginsianum, Neofusicoccum, and Botryosphaeria both possess a larger number of genes encoding pectin-degrading enzymes, which may explain the difference in pathogenicity between Botryosphaeriaceae fungi (Wang et al., 2018). Many genes encoding cellulase (AA9 and GH12) and hemicellulase (GH31 and GH43) have been significantly expanded in Neofusicoccum and Botryosphaeria fungi. These expansions may explain the rapid infection and colonization of Botryosphaeriaceae fungi in woody plants (Yan et al., 2018).
Conclusion
In conclusion, we constructed a phylogenetic tree using whole-genome data and clarified the taxonomic position of Botryosphaeriaceae in Dothideomycetes. Heterothallism is the ancestral mating type of Botryosphaeriaceae fungi, and these fungi have undergone at least 3 transitions from heterothallism to homothallism. The host range of Botryosphaeriaceae infection is closely related to the changes in the number of pathogenic genes. Our results provide important insights into the evolutionary history, mating strategies and pathogenicity-related genes variation in Botryosphaeriaceae.
Data Availability Statement
The datasets presented in this study can be found in online repositories. The names of the repository/repositories and accession number(s) can be found below: https://zenodo.org/record/5184447#.YRUFTci0yAc; the sequencing data are found at NCBI (PRJNA777748).
Author Contributions
CY and HL developed the concept of this study and were main contributors to writing the manuscript. CY, YD, QL, and JZ performed all experiments, data analysis, and prepared figures. CY, HL, SC, XX, AL, and XZ contributed to the manuscript edit and review. All authors read and approved the final manuscript.
Funding
This research was supported by the National Key R&D Program of China (2017YFD0600102-7) and Agricultural Science and Technology Fund of Shandong Province of China (Innovation Project of Forestry Science and Technology) Project (2019L Y003-4).
Conflict of Interest
The authors declare that the research was conducted in the absence of any commercial or financial relationships that could be construed as a potential conflict of interest.
Publisher’s Note
All claims expressed in this article are solely those of the authors and do not necessarily represent those of their affiliated organizations, or those of the publisher, the editors and the reviewers. Any product that may be evaluated in this article, or claim that may be made by its manufacturer, is not guaranteed or endorsed by the publisher.
Acknowledgments
We are grateful to Xiangwen Hou and Jian Ning Liu (KeGene Science & Technology Co., Ltd.) for help in analysis of data and improving the manuscript.
Supplementary Material
The Supplementary Material for this article can be found online at: https://www.frontiersin.org/articles/10.3389/fmicb.2022.800981/full#supplementary-material
Supplementary Figure 1 | Whole-genome-based phylogenetic tree of 271 species from Dothideomycetes and 8 outgroups. The two subclass Pleosporomycetidae and Dothideomycetidae were well resolved.
Supplementary Figure 2 | Comparison of genome size between pathogen and saprobe fungi.
Supplementary Figure 3 | Comparison of gene count between pathogen and saprobe fungi.
Supplementary Figure 4 | Reconstruction of ancestral binary character (non-plant pathogen and plant pathogen) of Dothideomycetes using Mesquite based on Mk1 model.
Supplementary Figure 5 | Reconstruction of ancestral mating type (heterothallism and homothallism) of Dothideomycetes using Mesquite based on Mk1 model as heterothallism.
Supplementary Table 1 | List of 271 Dothideomycetes fungi and 8 outgroups.
Supplementary Table 2 | Classification of 19 Botryosphaeriaceae fungi.
Supplementary Table 3 | Reconstruction of ancestral binary character (non-plant pathogen and plant pathogen) of Dothideomycetes using Mesquite based on Mk1 model.
Supplementary Table 4 | Pfam and GO enrichment of Dothideomycetes fungi.
Supplementary Table 5 | Mating type of Botryosphaeriaceae fungi.
Supplementary Table 6 | Conserved protein domains of Botryosphaeriaceae fungi mating type genes.
Supplementary Table 7 | Conservation of mating type genes of Botryosphaeriaceae fungi.
Supplementary Table 8 | Reconstruction of ancestral mating type of Botryosphaeriaceae fungi.
Supplementary Table 9 | Gene number of backbone and related genes of secondary metabolism of Botryosphaeriaceae fungi and 8 outgroups.
Supplementary Table 10 | Gene number of secreted proteins of Botryosphaeriaceae fungi and 8 outgroups.
Supplementary Table 11 | Pfam annotations of secreted proteins of B. dothidea and B. kuwatsukai.
Supplementary Table 12 | Gene number of CAZy of Botryosphaeriaceae fungi and 8 outgroups.
Supplementary Table 13 | Genes number of CZAy related to cellulose, hemicellulose and lignin degradation of Botryosphaeriaceae fungi and 8 outgroups.
Footnotes
- ^ http://www.repeatmasker.org/RepeatModeler/
- ^ http://www.repeatmasker.org/
- ^ http://www.mesquiteproject.org/
References
Alkan, N., Espeso, E. A., and Prusky, D. (2013). Virulence regulation of phytopathogenic fungi by pH. Antioxid. Redox. Signal. 19, 1012–1025. doi: 10.1089/ars.2012.5062
Alviano, D. S., Rodrigues, M. L., Almeida, C. A., Santos, A. L., Couceiro, J. N., Soares, R. M., et al. (2004). Differential expression of sialylglycoconjugates and sialidase activity in distinct morphological stages of Fonsecaea pedrosoi. Arch. Microbiol. 181, 278–286. doi: 10.1007/s00203-004-0653-9
Amselem, J., Cuomo, C. A., van Kan, J. A., Viaud, M., Benito, E. P., Couloux, A., et al. (2011). Genomic analysis of the necrotrophic fungal pathogens Sclerotinia sclerotiorum and Botrytis cinerea. PLoS Genetics 7:e1002230. doi: 10.1371/journal.pgen.1002230
Andolfi, A., Mugnai, L., Luque, J., Surico, G., Cimmino, A., and Evidente, A. (2011). Phytotoxins produced by fungi associated with grapevine trunk diseases. Toxins (Basel) 3, 1569–1605. doi: 10.3390/toxins3121569
Aramaki, T., Blanc-Mathieu, R., Endo, H., Ohkubo, K., Kanehisa, M., Goto, S., et al. (2020). KofamKOALA: KEGG Ortholog assignment based on profile HMM and adaptive score threshold. Bioinformatics 36, 2251–2252. doi: 10.1093/bioinformatics/btz859
Arie, T., Kaneko, I., Yoshida, T., Noguchi, M., Nomura, Y., and Yamaguchi, I. (2000). Mating-type genes from asexual phytopathogenic ascomycetes Fusarium oxysporum and Alternaria alternata. Mol. Plant Microbe Interact. 13, 1330–1339. doi: 10.1094/MPMI.2000.13.12.1330
Bankevich, A., Nurk, S., Antipov, D., Gurevich, A. A., Dvorkin, M., Kulikov, A. S., et al. (2012). SPAdes: a new genome assembly algorithm and its applications to single-cell sequencing. J. Comput. Biol. 19, 455–477. doi: 10.1089/cmb.2012.0021
Bihon, W., Burgess, T., Slippers, B., Wingfield, M. J., and Wingfield, B. D. (2012a). High levels of genetic diversity and cryptic recombination is widespread in introduced Diplodia pinea populations. Australas Plant Pathol. 41, 41–46. doi: 10.1007/s13313-011-0086-2
Bihon, W., Slippers, B., Burgess, T., Wingfield, M. J., and Wingfield, B. D. (2012b). Diverse sources of infection and cryptic recombination revealed in South African Diplodia pinea populations. Fungal Biol. 116, 112–120. doi: 10.1016/j.funbio.2011.10.006
Bihon, W., Wingfield, M. J., Slippers, B., Duong, T. A., and Wingfield, B. D. (2014). MAT gene idiomorphs suggest a heterothallic sexual cycle in a predominantly asexual and important pine pathogen. Fungal Genet. Biol. 62, 55–61. doi: 10.1016/j.fgb.2013.10.013
Billiard, S., López-Villavicencio, M., Hood, M. E., and Giraud, T. (2012). Sex, outcrossing and mating types: unsolved questions in fungi and beyond. J. Evol. Biol. 25, 1020–1038. doi: 10.1111/j.1420-9101.2012.02495.x
Blanco-Ulate, B., Rolshausen, P., and Cantu, D. (2013). Draft genome sequence of Neofusicoccum parvum isolate UCR-NP2, a fungal vascular pathogen associated with grapevine cankers. Genome Announc. 1:e00339-13. doi: 10.1128/genomeA.00339-13
Bolger, A. M., Lohse, M., and Usadel, B. (2014). Trimmomatic: a flexible trimmer for Illumina sequence data. Bioinformatics 30, 2114–2120. doi: 10.1093/bioinformatics/btu170
Bostock, R. M., Pye, M. F., and Roubtsova, T. V. (2014). Predisposition in plant disease: exploiting the nexus in abiotic and biotic stress perception and response. Annu. Rev. Phytopathol. 52, 517–549. doi: 10.1146/annurev-phyto-081211-172902
Camacho, C., Coulouris, G., Avagyan, V., Ma, N., Papadopoulos, J., Bealer, K., et al. (2009). BLAST+: architecture and applications. BMC Bioinformatics 10:421. doi: 10.1186/1471-2105-10-421
Campbell, M. S., Holt, C., Moore, B., and Yandell, M. (2014). Genome annotation and curation using MAKER and MAKER-P. Curr. Protoc. Bioinform. 48:4.11.1-39. doi: 10.1002/0471250953.bi0411s48
Castresana, J. (2000). Selection of conserved blocks from multiple alignments for their use in phylogenetic analysis. Mol. Biol. Evol. 17, 540–552. doi: 10.1093/oxfordjournals.molbev.a026334
Cobos, R., Barreiro, C., Mateos, R. M., and Coque, J. J. (2010). Cytoplasmic- and extracellular-proteome analysis of Diplodia seriata: a phytopathogenic fungus involved in grapevine decline. Proteome Sci. 8:46. doi: 10.1186/1477-5956-8-46
Das, A. K., Cohen, P. W., and Barford, D. (1998). The structure of the tetratricopeptide repeats of protein phosphatase 5: implications for TPR-mediated protein-protein interactions. EMBO J. 17, 1192–1199. doi: 10.1093/emboj/17.5.1192
Daskalov, A., Paoletti, M., Ness, F., and Saupe, S. J. (2012). Genomic clustering and homology between HET-S and the NWD2 STAND protein in various fungal genomes. PLoS One 7:e34854. doi: 10.1371/journal.pone.0034854
de miccolis, M., Rotolo, C., Pollastro, S., and Faretra, F. (2016). Molecular analysis of the mating type (MAT1) locus in strains of the heterothallic ascomycete Botrytis cinerea. Plant Pathol. 65, 1321–1332.
Duong, T. A., de, Beer, Z. W., Wingfield, B. D., and Wingfield, M. J. (2013). Characterization of the mating-type genes in Leptographium procerum and Leptographium profanum. Fungal Biol. 117, 411–421. doi: 10.1016/j.funbio.2013.04.005
Eddy, S. R. (2008). A probabilistic model of local sequence alignment that simplifies statistical significance estimation. PLoS Comput. Biol. 4:e1000069. doi: 10.1371/journal.pcbi.1000069
Emms, D. M., and Kelly, S. (2015). OrthoFinder: solving fundamental biases in whole genome comparisons dramatically improves orthogroup inference accuracy. Genome Biol. 16:157. doi: 10.1186/s13059-015-0721-2
Félix, C., Duarte, A. S., Vitorino, R., Guerreiro, A. C., Domingues, P., Correia, A. C., et al. (2016). Temperature modulates the secretome of the phytopathogenic fungus Lasiodiplodia theobromae. Front. Plant Sci. 7:1096. doi: 10.3389/fpls.2016.01096
Félix, C., Meneses, R., Gonçalves, M., Tilleman, L., Duarte, A. S., Jorrín-Novo, J. V., et al. (2019). A multi-omics analysis of the grapevine pathogen Lasiodiplodia theobromae reveals that temperature affects the expression of virulence- and pathogenicity-related genes. Sci. Rep. 9:13144. doi: 10.1038/s41598-019-49551-w
Fernandes, I., Alves, A., Correia, A., Devreese, B., and Esteves, A. C. (2014). Secretome analysis identifies potential virulence factors of Diplodia corticola, a fungal pathogen involved in cork oak (Quercus suber) decline. Fungal Biol. 118, 516–523. doi: 10.1016/j.funbio.2014.04.006
Galagan, J. E., Calvo, S. E., Cuomo, C., Ma, L. J., Wortman, J. R., Batzoglou, S., et al. (2005). Sequencing of Aspergillus nidulans and comparative analysis with A. fumigatus and A. oryzae. Nature 438, 1105–1115.
Garcia, J. F., Lawrence, D. P., Morales-Cruz, A., Travadon, R., Minio, A., Hernandez-Martinez, R., et al. (2021). Phylogenomics of plant-associated Botryosphaeriaceae species. Front. Microbiol. 12:652802. doi: 10.3389/fmicb.2021.652802
Gaur, M., Puri, N., Manoharlal, R., Rai, V., Mukhopadhayay, G., Choudhury, D., et al. (2008). MFS transportome of the human pathogenic yeast Candida albicans. BMC Genomics 9:579. doi: 10.1186/1471-2164-9-579
Gioti, A., Mushegian, A. A., Strandberg, R., Stajich, J. E., and Johannesson, H. (2012). Unidirectional evolutionary transitions in fungal mating systems and the role of transposable elements. Mol. Biol. Evol. 29, 3215–3226. doi: 10.1093/molbev/mss132
Greenwald, C. J., Kasuga, T., Glass, N. L., Shaw, B. D., Ebbole, D. J., and Wilkinson, H. H. (2010). Temporal and spatial regulation of gene expression during asexual development of Neurospora crassa. Genetics 186, 1217–1230. doi: 10.1534/genetics.110.121780
Guttman, D. S., McHardy, A. C., and Schulze-Lefert, P. (2014). Microbial genome-enabled insights into plant-microorganism interactions. Nat. Rev. Genet. 15, 797–813. doi: 10.1038/nrg3748
Han, Y., Liu, X., Benny, U., Kistler, H. C., and VanEtten, H. D. (2001). Genes determining pathogenicity to pea are clustered on a supernumerary chromosome in the fungal plant pathogen Nectria haematococca. Plant J. 25, 305–314. doi: 10.1046/j.1365-313x.2001.00969.x
Haridas, S., Albert, R., Binder, M., Bloem, J., LaButti, K., Salamov, A., et al. (2020). 101 Dothideomycetes genomes: a test case for predicting lifestyles and emergence of pathogens. Stud. Mycol. 96, 141–153. doi: 10.1016/j.simyco.2020.01.003
Haridas, S., Salamov, A., and Grigoriev, I. V. (2018). Fungal genome annotation. Methods Mol. Biol. 1775, 171–184. doi: 10.1007/978-1-4939-7804-5_15
Idnurm, A. (2011). Sex and speciation: the paradox that non-recombining DNA promotes recombination. Fungal Biol. Rev. 25, 121–127. doi: 10.1016/j.fbr.2011.07.003
Inderbitzin, P., Harkness, J., Turgeon, B. G., and Berbee, M. L. (2005). Lateral transfer of mating system in Stemphylium. Proc. Natl. Acad. Sci. U S A. 102, 11390–11395. doi: 10.1073/pnas.0501918102
Ishikawa, F. H., Souza, E. A., Shoji, J. Y., Connolly, L., Freitag, M., Read, N. D., et al. (2012). Heterokaryon incompatibility is suppressed following conidial anastomosis tube fusion in a fungal plant pathogen. PLoS One 7:e31175. doi: 10.1371/journal.pone.0031175
Islam, M. S., Haque, M. S., Islam, M. M., Emdad, E. M., Halim, A., Hossen, Q. M., et al. (2012). Tools to kill: genome of one of the most destructive plant pathogenic fungi Macrophomina phaseolina. BMC Genomics 13:493. doi: 10.1186/1471-2164-13-493
Kaneko, A., Umeyama, T., Utena-Abe, Y., Yamagoe, S., Niimi, M., and Uehara, Y. (2006). Tcc1p, a novel protein containing the tetratricopeptide repeat motif, interacts with Tup1p to regulate morphological transition and virulence in Candida albicans. Eukaryot. Cell 5, 1894–1905. doi: 10.1128/EC.00151-06
Katoh, K., Misawa, K., Kuma, K., and Miyata, T. (2002). MAFFT: a novel method for rapid multiple sequence alignment based on fast Fourier transform. Nucleic Acids Res. 30, 3059–3066. doi: 10.1093/nar/gkf436
Kim, K., Kim, K. R., and Park, E. (2005). An infection model of apple white rot based on conidial germination and appressorium formation of Botryosphaeria dothidea. Plant Pathol. J. 21, 322–327. doi: 10.5423/ppj.2005.21.4.322
Kondo, Y., Ohara, N., Sato, K., Yoshimura, M., Yukitake, H., Naito, M., et al. (2010). Tetratricopeptide repeat protein-associated proteins contribute to the virulence of Porphyromonas gingivalis. Infect. Immun. 78, 2846–2856. doi: 10.1128/IAI.01448-09
Landi, L., Pollastro, S., Rotolo, C., Romanazzi, G., Faretra, F., and De Miccolis Angelini, R. M. (2020). Draft genomic resources for the brown rot fungal pathogen Monilinia laxa. Mol. Plant Microbe Interact. 33, 145–148. doi: 10.1094/MPMI-08-19-0225-A
Lanfear, R., Frandsen, P. B., Wright, A. M., Senfeld, T., and Calcott, B. (2017). PartitionFinder 2: new methods for selecting partitioned models of evolution for molecular and morphological phylogenetic analyses. Mol. Biol. Evol. 34, 772–773. doi: 10.1093/molbev/msw260
Lomsadze, A., Burns, P. D., and Borodovsky, M. (2014). Integration of mapped RNA-Seq reads into automatic training of eukaryotic gene finding algorithm. Nucleic Acids Res. 42:e119. doi: 10.1093/nar/gku557
Lopes, A., Linaldeddu, B. T., Phillips, A. J. L., and Alves, A. (2018). Mating type gene analyses in the genus diplodia: from cryptic sex to cryptic species. Fungal Biol. 122, 629–638. doi: 10.1016/j.funbio.2018.03.012
Lopes, A., Phillips, A. J., and Alves, A. (2017). Mating type genes in the genus Neofusicoccum: mating strategies and usefulness in species delimitation. Fungal Biol. 121, 394–404. doi: 10.1016/j.funbio.2016.08.011
Luini, E., Fleurat-Lessard, P., Rousseau, L., Roblin, G., and Berjeaud, J.-M. (2010). Inhibitory effects of polypeptides secreted by the grapevine pathogens Phaeomoniella chlamydospora and Phaeoacremonium aleophilum on plant cell activities. Physiol. Mol. Plant Pathol. 74, 403–411.
Marçais, G., and Kingsford, C. (2011). A fast, lock-free approach for efficient parallel counting of occurrences of k-mers. Bioinformatics 27, 764–770. doi: 10.1093/bioinformatics/btr011
Marchler-Bauer, A., Derbyshire, M. K., Gonzales, N. R., Lu, S., Chitsaz, F., Geer, L. Y., et al. (2015). CDD: NCBI’s conserved domain database. Nucleic Acids Res. 43, D222–D226. doi: 10.1093/nar/gku1221
Marsberg, A., Kemler, M., Jami, F., Nagel, J. H., Postma-Smidt, A., Naidoo, S., et al. (2017). Botryosphaeria dothidea: a latent pathogen of global importance to woody plant health. Mol. Plant Pathol. 18, 477–488. doi: 10.1111/mpp.12495
Meile, L., Peter, J., Puccetti, G., Alassimone, J., McDonald, B. A., and Sánchez-Vallet, A. (2020). Chromatin dynamics contribute to the spatiotemporal expression pattern of virulence genes in a fungal plant pathogen. mBio 11:e02343-20. doi: 10.1128/mBio.02343-20
Miyauchi, S., Kiss, E., Kuo, A., Drula, E., Kohler, A., Sánchez-García, M., et al. (2020). Large-scale genome sequencing of mycorrhizal fungi provides insights into the early evolution of symbiotic traits. Nat. Commun. 11:5125. doi: 10.1038/s41467-020-18795-w
Morales-Cruz, A., Amrine, K. C., Blanco-Ulate, B., Lawrence, D. P., Travadon, R., Rolshausen, P. E., et al. (2015). Distinctive expansion of gene families associated with plant cell wall degradation, secondary metabolism, and nutrient uptake in the genomes of grapevine trunk pathogens. BMC Genomics 16:469. doi: 10.1186/s12864-015-1624-z
Mur, L. A. J., Simpson, C., Kumari, A., Gupta, A. K., and Gupta, K. J. (2017). Moving nitrogen to the centre of plant defence against pathogens. Ann. Bot. 119, 703–709. doi: 10.1093/aob/mcw179
Murray, M. G., and Thompson, W. F. (1980). Rapid isolation of high molecular weight plant DNA. Nucleic Acids Res. 8, 4321–4325. doi: 10.1093/nar/8.19.4321
Nagel, J. H., Wingfield, M. J., and Slippers, B. (2018). Evolution of the mating types and mating strategies in prominent genera in the Botryosphaeriaceae. Fungal Genet. Biol. 114, 24–33. doi: 10.1016/j.fgb.2018.03.003
Nagel, J. H., Wingfield, M. J., and Slippers, B. (2021). Increased abundance of secreted hydrolytic enzymes and secondary metabolite gene clusters define the genomes of latent plant pathogens in the Botryosphaeriaceae. BMC Genomics 22:589. doi: 10.1186/s12864-021-07902-w
Nguyen, N. H., Song, Z., Bates, S. T., Branco, S., Tedersoo, L., Menke, J., et al. (2016). FUNGuild: an open annotation tool for parsing fungal community datasets by ecological guild. Fungal Ecol. 20, 241–248. doi: 10.1016/j.funeco.2015.06.006
Nierman, W. C., Pain, A., Anderson, M. J., Wortman, J. R., Kim, H. S., Arroyo, J., et al. (2005). Genomic sequence of the pathogenic and allergenic filamentous fungus Aspergillus fumigatus. Nature 438, 1151–1156. doi: 10.1038/nature04332
Nygren, K., Strandberg, R., Wallberg, A., Nabholz, B., Gustafsson, T., García, D., et al. (2011). A comprehensive phylogeny of Neurospora reveals a link between reproductive mode and molecular evolution in fungi. Mol. Phylogenet. Evol. 59, 649–663. doi: 10.1016/j.ympev.2011.03.023
O’Connell, R. J., Thon, M. R., Hacquard, S., Amyotte, S. G., Kleemann, J., Torres, M. F., et al. (2012). Lifestyle transitions in plant pathogenic Colletotrichum fungi deciphered by genome and transcriptome analyses. Nat. Genet. 44, 1060–1065. doi: 10.1038/ng.2372
Ohm, R. A., Feau, N., Henrissat, B., Schoch, C. L., Horwitz, B. A., Barry, K. W., et al. (2012). Diverse lifestyles and strategies of plant pathogenesis encoded in the genomes of eighteen Dothideomycetes fungi. PLoS Pathog 8:e1003037. doi: 10.1371/journal.ppat.1003037
Paoletti, M., and Saupe, S. J. (2009). Fungal incompatibility: evolutionary origin in pathogen defense? Bioessays 31, 1201–1210. doi: 10.1002/bies.200900085
Paoletti, M., Rydholm, C., Schwier, E. U., Anderson, M. J., Szakacs, G., Lutzoni, F., et al. (2005). Evidence for sexuality in the opportunistic fungal pathogen Aspergillus fumigatus. Curr. Biol. 15, 1242–1248. doi: 10.1016/j.cub.2005.05.045
Paolinelli-Alfonso, M., Villalobos-Escobedo, J. M., Rolshausen, P., Herrera-Estrella, A., Galindo-Sánchez, C., López-Hernández, J. F., et al. (2016). Global transcriptional analysis suggests Lasiodiplodia theobromae pathogenicity factors involved in modulation of grapevine defensive response. BMC Genomics 17:615. doi: 10.1186/s12864-016-2952-3
Peter, M., Kohler, A., Ohm, R. A., Kuo, A., Krützmann, J., Morin, E., et al. (2016). Ectomycorrhizal ecology is imprinted in the genome of the dominant symbiotic fungus Cenococcum geophilum. Nat. Commun. 7:12662. doi: 10.1038/ncomms12662
Phillips, A. J., Alves, A., Abdollahzadeh, J., Slippers, B., Wingfield, M. J., Groenewald, J. Z., et al. (2013). The Botryosphaeriaceae: genera and species known from culture. Stud. Mycol. 76, 51–167. doi: 10.3114/sim0021
Ranallo-Benavidez, T. R., Jaron, K. S., and Schatz, M. C. (2020). GenomeScope 2.0 and Smudgeplot for reference-free profiling of polyploid genomes. Nat. Commun. 11:1432. doi: 10.1038/s41467-020-14998-3
Ruibal, C., Gueidan, C., Selbmann, L., Gorbushina, A. A., Crous, P. W., Groenewald, J. Z., et al. (2009). Phylogeny of rock-inhabiting fungi related to Dothideomycetes. Stud. Mycol. 64, 123s–133s. doi: 10.3114/sim.2009.64.06
Rydholm, C., Dyer, P. S., and Lutzoni, F. (2007). DNA sequence characterization and molecular evolution of MAT1 and MAT2 mating-type loci of the self-compatible ascomycete mold Neosartorya fischeri. Eukaryot. Cell 6, 868–874. doi: 10.1128/EC.00319-06
Schapire, A. L., Valpuesta, V., and Botella, M. A. (2006). TPR proteins in plant hormone signaling. Plant Signal. Behav. 1, 229–230. doi: 10.4161/psb.1.5.3491
Schoch, C. L., Crous, P. W., Groenewald, J. Z., Boehm, E. W., Burgess, T. I., de Gruyter, J., et al. (2009). A class-wide phylogenetic assessment of Dothideomycetes. Stud. Mycol. 64, 1s–15s. doi: 10.3114/sim.2009.64.01
Schuelke, T. A., Wu, G., Westbrook, A., Woeste, K., Plachetzki, D. C., Broders, K., et al. (2017). Comparative genomics of pathogenic and nonpathogenic beetle-vectored fungi in the Genus Geosmithia. Genome Biol. Evol. 9, 3312–3327. doi: 10.1093/gbe/evx242
Sharpton, T. J., Neafsey, D. E., Galagan, J. E., and Taylor, J. W. (2008). Mechanisms of intron gain and loss in Cryptococcus. Genome Biol. 9:R24.
Siewers, V., Viaud, M., Jimenez-Teja, D., Collado, I. G., Gronover, C. S., Pradier, J. M., et al. (2005). Functional analysis of the cytochrome P450 monooxygenase gene bcbot1 of Botrytis cinerea indicates that botrydial is a strain-specific virulence factor. Mol. Plant Microbe Interact. 18, 602–612. doi: 10.1094/MPMI-18-0602
Slater, G. S., and Birney, E. (2005). Automated generation of heuristics for biological sequence comparison. BMC Bioinformatics 6:31. doi: 10.1186/1471-2105-6-31
Slippers, B., and Wingfield, M. J. (2007). Botryosphaeriaceae as endophytes and latent pathogens of woody plants: diversity, ecology and impact. Fungal Biol. Rev. 21, 90–106.
Staats, C. C., Kmetzsch, L., Schrank, A., and Vainstein, M. H. (2013). Fungal zinc metabolism and its connections to virulence. Front. Cell Infect. Microbiol. 3:65. doi: 10.3389/fcimb.2013.00065
Stamatakis, A. (2014). RAxML version 8: a tool for phylogenetic analysis and post-analysis of large phylogenies. Bioinformatics 30, 1312–1313. doi: 10.1093/bioinformatics/btu033
Stanke, M., and Morgenstern, B. (2005). AUGUSTUS: a web server for gene prediction in eukaryotes that allows user-defined constraints. Nucleic Acids Res. 33, W465–W467. doi: 10.1093/nar/gki458
Sullivan, M. J., Petty, N. K., and Beatson, S. A. (2011). Easyfig: a genome comparison visualizer. Bioinformatics 27, 1009–1010. doi: 10.1093/bioinformatics/btr039
Tang, W., Ding, Z., Zhou, Z. Q., Wang, Y. Z., and Guo, L. Y. (2012). Phylogenetic and pathogenic analyses show that the causal agent of apple ring rot in China is Botryosphaeria dothidea. Plant Dis. 96, 486–496. doi: 10.1094/PDIS-08-11-0635
ten Have, A., Mulder, W., Visser, J., and van Kan, J. A. (1998). The endopolygalacturonase gene Bcpg1 is required for full virulence of Botrytis cinerea. Mol. Plant Microbe Interact. 11, 1009–1016. doi: 10.1094/MPMI.1998.11.10.1009
Terrett, O. M., and Dupree, P. (2019). Covalent interactions between lignin and hemicelluloses in plant secondary cell walls. Curr. Opin. Biotechnol. 56, 97–104. doi: 10.1016/j.copbio.2018.10.010
Turgeon, B. G. (1998). Application of mating type gene technology to problems in fungal biology. Annu. Rev. Phytopathol. 36, 115–137. doi: 10.1146/annurev.phyto.36.1.115
van der Nest, M. A., Bihon, W., De Vos, L., Naidoo, K., Roodt, D., Rubagotti, E., et al. (2014). Draft genome sequences of Diplodia sapinea, Ceratocystis manginecans, and Ceratocystis moniliformis. IMA Fungus 5, 135–140.
Wang, B., Liang, X., Gleason, M. L., Zhang, R., and Sun, G. (2018). Comparative genomics of Botryosphaeria dothidea and B. kuwatsukai, causal agents of apple ring rot, reveals both species expansion of pathogenicity-related genes and variations in virulence gene content during speciation. IMA Fungus 9, 243–257. doi: 10.5598/imafungus.2018.09.02.02
Wang, B., Liang, X., Hao, X., Dang, H., Hsiang, T., Gleason, M. L., et al. (2021). Comparison of mitochondrial genomes provides insights into intron dynamics and evolution in Botryosphaeria dothidea and B. kuwatsukai. Environ. Microbiol. 23, 5320–5333. doi: 10.1111/1462-2920.15608
Wang, N. Y., Zhang, K., Huguet-Tapia, J. C., Rollins, J. A., and Dewdney, M. M. (2016). Mating type and simple sequence repeat markers indicate a clonal population of Phyllosticta citricarpa in Florida. Phytopathology 106, 1300–1310. doi: 10.1094/PHYTO-12-15-0316-R
Waterhouse, R. M., Seppey, M., Simão, F. A., Manni, M., Ioannidis, P., Klioutchnikov, G., et al. (2018). BUSCO applications from quality assessments to gene prediction and phylogenomics. Mol. Biol. Evol. 35, 543–548. doi: 10.1093/molbev/msx319
Wijayawardene, N., Hyde, K., Rajeshkumar, K. C., Hawksworth, D., Madrid, H., Kirk, P., et al. (2017). Notes for genera: Ascomycota. Fungal Divers 86, 1–594.
Wilken, P. M., Steenkamp, E. T., Wingfield, M. J., de, Beer, Z. W., and Wingfield, B. D. (2014). DNA loss at the Ceratocystis fimbriata mating locus results in self-sterility. PLoS One 9:e92180. doi: 10.1371/journal.pone.0092180
Xu, C., Wang, C., Ju, L., Zhang, R., Biggs, A. R., Tanaka, E., et al. (2015). Multiple locus genealogies and phenotypic characters reappraise the causal agents of apple ring rot in China. Fungal Divers 71, 215–231.
Xu, L., Jardini, T. M., and Chen, W. (2016). Direct repeat-mediated DNA deletion of the mating type MAT1-2 genes results in unidirectional mating type switching in Sclerotinia trifoliorum. Sci. Rep. 6:27083. doi: 10.1038/srep27083
Yan, J. Y., Xie, Y., Zhang, W., Wang, Y., Liu, J. K., Hyde, K. D., et al. (2013). Species of Botryosphaeriaceae involved in grapevine dieback in China. Fungal Divers 61, 221–236. doi: 10.1007/s13225-013-0251-8
Yan, J. Y., Zhao, W. S., Chen, Z., Xing, Q. K., Zhang, W., Chethana, K. W. T., et al. (2018). Comparative genome and transcriptome analyses reveal adaptations to opportunistic infections in woody plant degrading pathogens of Botryosphaeriaceae. DNA Res. 25, 87–102. doi: 10.1093/dnares/dsx040
Yin, Z., Liu, H., Li, Z., Ke, X., Dou, D., Gao, X., et al. (2015). Genome sequence of Valsa canker pathogens uncovers a potential adaptation of colonization of woody bark. New Phytol. 208, 1202–1216. doi: 10.1111/nph.13544
Yokoyama, E., Arakawa, M., Yamagishi, K., and Hara, A. (2006). Phylogenetic and structural analyses of the mating-type loci in Clavicipitaceae. FEMS Microbiol. Lett. 264, 182–191. doi: 10.1111/j.1574-6968.2006.00447.x
Yun, S. H., Kim, H. K., Lee, T., and Turgeon, B. G. (2017). Self-fertility in Chromocrea spinulosa is a consequence of direct repeat-mediated loss of MAT1-2, subsequent imbalance of nuclei differing in mating type, and recognition between unlike nuclei in a common cytoplasm. PLoS Genet 13:e1006981. doi: 10.1371/journal.pgen.1006981
Zhang, H., Yohe, T., Huang, L., Entwistle, S., Wu, P., Yang, Z., et al. (2018). dbCAN2: a meta server for automated carbohydrate-active enzyme annotation. Nucleic Acids Res. 46, W95–W101. doi: 10.1093/nar/gky418
Keywords: Botryosphaeriaceae, phytopathogen, evolution traits, mating strategies, pathogenicity-related genes
Citation: Yu C, Diao Y, Lu Q, Zhao J, Cui S, Xiong X, Lu A, Zhang X and Liu H (2022) Comparative Genomics Reveals Evolutionary Traits, Mating Strategies, and Pathogenicity-Related Genes Variation of Botryosphaeriaceae. Front. Microbiol. 13:800981. doi: 10.3389/fmicb.2022.800981
Received: 24 October 2021; Accepted: 03 February 2022;
Published: 23 February 2022.
Edited by:
Baolei Jia, Chung-Ang University, South KoreaReviewed by:
Rufina Hernandez, Center for Scientific Research and Higher Education in Ensenada (CICESE), MexicoArtur Alves, University of Aveiro, Portugal
Copyright © 2022 Yu, Diao, Lu, Zhao, Cui, Xiong, Lu, Zhang and Liu. This is an open-access article distributed under the terms of the Creative Commons Attribution License (CC BY). The use, distribution or reproduction in other forums is permitted, provided the original author(s) and the copyright owner(s) are credited and that the original publication in this journal is cited, in accordance with accepted academic practice. No use, distribution or reproduction is permitted which does not comply with these terms.
*Correspondence: Huixiang Liu, aHhsaXU3MjJAMTI2LmNvbQ==