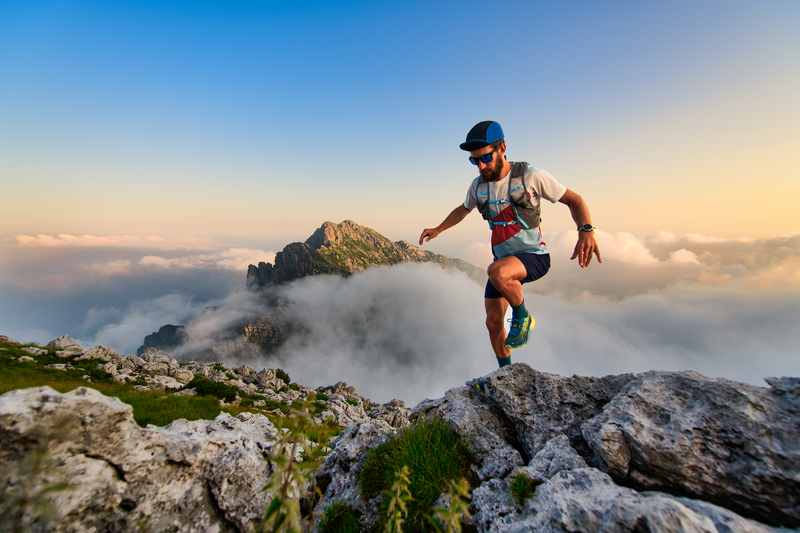
94% of researchers rate our articles as excellent or good
Learn more about the work of our research integrity team to safeguard the quality of each article we publish.
Find out more
ORIGINAL RESEARCH article
Front. Microbiol. , 28 February 2022
Sec. Antimicrobials, Resistance and Chemotherapy
Volume 13 - 2022 | https://doi.org/10.3389/fmicb.2022.793738
While Extended-Spectrum β-Lactamases (ESBL) and AmpC β-lactamases barely degrade carbapenem antibiotics, they are able to bind carbapenems and prevent them from interacting with penicillin-binding proteins, thereby inhibiting their activity. Further, it has been shown that Enterobacterales can become resistant to carbapenems when high concentrations of ESBL and AmpC β-lactamases are present in the bacterial cell in combination with a decreased influx of antibiotics (due to a decrease in porins and outer-membrane permeability). In this study, a targeted liquid chromatography-tandem mass spectrometry (LC-MS/MS) assay was developed for the detection of the Escherichia coli porins OmpC and OmpF, its chromosomal AmpC β-lactamase, and the plasmid-mediated CMY-2 β-lactamase. BlaCMY–2–like positive E. coli isolates were cultured in the presence of increasing concentrations of meropenem, and resistant mutants were analyzed using the developed LC-MS/MS assay, Western blotting, and whole genome sequencing. In five strains that became meropenem resistant, a decrease in OmpC and/or OmpF (caused by premature stop codons or gene interruptions) was the first event toward meropenem resistance. In four of these strains, an additional increase in MICs was caused by an increase in CMY-2 production, and in one strain this was most likely caused by an increase in CTX-M-15 production. The LC-MS/MS assay developed proved to be suitable for the (semi-)quantitative analysis of CMY-2-like β-lactamases and porins within 4 h. Targeted LC-MS/MS could have additional clinical value in the early detection of non-carbapenemase-producing carbapenem-resistant E. coli.
There are several antibiotic classes known, of which β-lactams are the most diverse and most frequently prescribed (Pandey and Cascella, 2020). β-lactam antibiotics can be categorized into several sub-classes, including the broad-spectrum carbapenem class. Carbapenems can withstand hydrolysis by most bacterial β-lactamases (including Extended-Spectrum β-Lactamases—ESBL) and are therefore considered one of the last resort antibiotics (Hawkey and Livermore, 2012). Unfortunately however, carbapenem-resistance rates are increasing worldwide (Miller and Humphries, 2016; Iovleva and Doi, 2017), with the increasing prevalence of carbapenem-resistant Enterobacterales (CRE) being especially worrying. Most CRE produce an acquired carbapenemase (Nordmann et al., 2011; Brolund et al., 2019). However, a second group of non-carbapenemase-producing CRE (non-CP-CRE) become resistant via a decrease in outer-membrane permeability combined with an increase in the production of other chromosomal or plasmid-encoded β-lactamases (Oteo et al., 2008; Adler et al., 2013; Goessens et al., 2013; Tangden et al., 2013; Miller and Humphries, 2016; Nordmann and Poirel, 2019). Some studies have even shown that up to 60% of carbapenem-resistant Escherichia coli in European hospitals comprised non-CP-CRE isolates (Grundmann et al., 2017; Nordmann and Poirel, 2019).
In E. coli, OmpC and OmpF are the two major porins responsible for the influx of β-lactams across the outer membrane (Jaffe et al., 1982; Choi and Lee, 2019). Alterations in the constriction regions of these porins can reduce permeation efficiency of β-lactams (Delcour, 2009; Vergalli et al., 2020). Further, a decrease in or complete loss of porin expression can also contribute to β-lactam resistance (Delcour, 2009; Vergalli et al., 2020). This decrease or absence of porins reduces the concentration of β-lactam molecules in the periplasm which can thereafter be bound and “trapped” by, for example, CMY-2-like β-lactamases (Goessens et al., 2013). Other β-lactamases that have been linked to carbapenem resistance in non-CP-CR E. coli include the class A β-lactamases TEM-1 and CTX-M, the class D β-lactamase OXA-1, as well as multiple class C β-lactamases including E. coli chromosomal AmpC (cAmpC) (Mammeri et al., 2008, 2010; Adler et al., 2013).
Unfortunately, no reliable phenotypic assays are currently available to specifically detect the absence of OmpC and OmpF. This information is important, as isolates which lack one or both of these main porins can rapidly become resistant to multiple antibiotics (including carbapenems) after antibiotic exposure (Pages et al., 2008; Goessens et al., 2013; van Boxtel et al., 2017; Vergalli et al., 2020). Additionally, the development of such an assay could aid in understanding the nature of carbapenem resistance in isolates that do not express carbapenemases. While real-time PCR assays are frequently used to confirm or screen for antimicrobial resistance mechanisms, they cannot detect a lack of porins, nor can they detect the actual production of β-lactamases. Although the relative abundance of proteins can be predicted based on mRNA using RT-qPCRs, porins are also regulated by RNA anti-sense regulators and by post-translational regulatory mechanisms which hamper quantitative prediction accuracy when using techniques based solely on detection of mRNA (Pages et al., 2008; Charretier et al., 2015b). In addition, frameshifts and premature stop codons are easily missed by RT-qPCR.
For the accurate detection and quantification of resistance mechanisms, and to predict their combined effect, a specific protein detection method would be more suitable. Before the molecular era, Western blotting was used to determine the presence of specific proteins, but nowadays MS-techniques are increasingly used for the detection of proteins. The use of matrix-assisted laser desorption/ionization time-of-flight (MALDI-TOF) mass spectrometry is widely implemented for bacterial identification in diagnostic medical microbiology laboratories, and its use has also previously been reported in research settings for the detection of AmpC-type β-lactamases (Hart et al., 2015; Espinosa et al., 2018), OmpC and OmpF porins (Hu et al., 2015), and several other resistance mechanisms (Oviano and Bou, 2019). However, the MALDI-TOF analyzer is primarily designed to generate a spectral fingerprint of the most abundant proteins and is less suited for the accurate identification, let alone quantification of less abundant protein markers (Intelicato-Young and Fox, 2013). In contrast, targeted liquid chromatography-tandem mass spectrometry (LS-MS/MS) offers increased sensitivity and specificity by separating and filtering peptides prior to detection. Therefore, targeted LC-MS/MS using a triple quadrupole or an Orbitrap mass spectrometer can be used for the accurate detection and quantification of many antimicrobial resistance mechanisms (Charretier et al., 2015a,b; Charretier and Schrenzel, 2016; Hassing et al., 2016; Wang et al., 2017, 2019; Cecchini et al., 2018; Foudraine et al., 2019, 2021a).
In the current study, we developed a single targeted LC-MS/MS assay for the detection and (relative) quantification of the E. coli porins OmpC and OmpF, as well as CMY-2-like and cAmpC β-lactamases. Subsequently, we cultured several clinical E. coli isolates carrying blaCMY–2–like genes in the presence of meropenem to induce meropenem resistance in vitro. We then used the developed assay to analyze how the abundance of the porins and β-lactamases correlated to resistance, and in addition, we applied whole-genome sequencing (WGS) to place our findings within the context of the underlying genetic mechanisms.
Ethical approval was not required as only stored bacterial strains were used. A total of 15 clinical E. coli strains and two E. coli laboratory strains were used in this study. All strains were obtained from the Erasmus MC collection of bacterial isolates or had been used in the previously published study of van Boxtel et al. (2017). Twelve clinical strains were selected based on a phenotype which suggested the presence of plasmid-encoded AmpC-type β-lactamases with minimum inhibitory concentrations (MICs) of 2 mg/L or higher for third generation cephalosporins and MICs of 8 mg/L or higher for cefoxitin (Jacoby, 2009). The presence of CMY(-like) β-lactamase genes was confirmed by PCR using the primers described in the publication of Perez-Perez and Hanson (2002). In addition, three known cAmpC hyperproducers were included from a previous study (numbered 187, 188 and 190) (Foudraine et al., 2021b). Finally, the E. coli laboratory strain Top10F’ that expressed both OmpC and OmpF (Invitrogen, Thermo Fisher Scientific, Carlsbad, CA, United States) and the porin-deficient derivative CE1536 of strain BL21 (DE3) (Renault et al., 2012) were used as positive and negative control strains for the LC-MS/MS experiments. In addition, transformed derivatives of Top10F, i.e., Top10F’-p2761 and Top10F’-pmr42 which carried plasmids containing blaCMY–2 were used from a previous study as positive controls for Western blotting (van Boxtel et al., 2017).
All blaCMY–like-positive isolates were initially susceptible to meropenem with MICs of 0.25 mg/L or lower (VITEK 2, BioMérieux). To study the effect of prolonged meropenem exposure on these strains, each strain was exposed to increasing concentrations of meropenem (Sigma Aldrich, St. Louis, MO, United States) over a period of multiple weeks (Figure 1). First, isolates were grown in 5 ml brain hearth infusion broth (BHI). Next, 2.5 ml was divided over four vials containing 4.5 ml BHI broth with increasing meropenem concentrations and one growth control vial without meropenem, and 2.5 ml was stored. Subsequently, the vials were incubated overnight and the broth of the vial containing the highest meropenem concentration in which bacterial growth was observed was again divided over four new vials with increasing meropenem concentrations. This procedure was subsequently repeated for 15–25 cycles until a maximum meropenem concentration was attained. Meropenem concentrations in the vials ranged from half of the previous concentration at which growth still occurred, and increased in steps of twofold to four times the previous concentration at which growth occurred. After every two to four cycles, and preferably after growth in a vial with a higher concentration of meropenem, the broth with the highest meropenem concentration was also serially diluted and inoculated on Mueller Hinton II (MHII) agar plates containing half to four times the meropenem concentration of the vial. After overnight incubation, single mutant colonies on the agar plate with the highest meropenem concentration were isolated and stored at −80°C. These experiments of prolonged meropenem exposure were performed in triplicate by three different technicians for all twelve clinical isolates. Meropenem MICs of all stored mutants were determined by broth microdilution.
Reference sequences of blacAmpC (from E. coli K-12), blaCMY–2, ompC, and ompF were obtained from the NCBI nucleotide RefSeq database1. To select tryptic peptides from conserved protein regions, variant sequences were obtained from the NCBI nucleotide collection2 which was accessed multiple times in September 2019. For cAmpC, OmpC, and OmpF, the search was restricted to E. coli sequences (Taxid: 562). All variant sequences were aligned and translated and sequences with less than 80% query coverage were removed. Tryptic peptides from 6 to 20 amino acids without a cysteine and preferably without a methionine or a double lysine/arginine motif at the N- or C-terminus (ragged end) were selected as candidate peptides (Supplementary Table 1). In addition to porin and β-lactamase peptides, internal quality control peptides were included from a previous study for verification of adequate sample pre-treatment (Foudraine et al., 2021a). General peptide uniqueness of all candidate peptides was assessed by performing BLASTp searches in the NCBI non-redundant protein sequence database. The coverage and specificity of the CMY-2-like and cAmpC candidate peptides were also assessed in silico by comparing all class C β-lactamases provided by the Beta-Lactamase Database (Naas et al., 2017). Subsequently, a targeted LC-MS/MS experiment was performed to select the best performing peptide markers. For this purpose, nine previously characterized E. coli isolates (Foudraine et al., 2021b) including three cAmpC hyperproducers, two CMY-2-like positive isolates, and the laboratory strain Top10F were used. The mass error, peak height, number of peptide fragments and peak shape were evaluated for all candidate peptides. For CMY-2-like and OmpF, two peptides were selected, for cAmpC three peptides, and for OmpC four peptides (Table 1). Stable isotope-labeled (SIL) peptides were ordered for all selected peptides (Pepscan, Lelystad, Netherlands). Single and fixed concentrations of SIL peptides were determined in a separate experiment using the original/parental clinical isolates and the E. coli laboratory strain Top10F’ to obtain a ratio between endogenous and SIL peptides close to one. Detection of all selected peptides was combined into a single multiplex assay. Peptides were considered detected when the ratio dot products (rdotp) was above 0.95 and if the mass error was < 10 ppm (Foudraine et al., 2019). Endogenous peptide to SIL peptide ratios were calculated using Skyline software (MacCoss Lab Software, University of Washington, United States) to compare the relative quantities of porin and CMY peptides between parental and mutant isolates.
Table 1. In total, 14 peptides were selected and included in a multiplex LC-MS/MS assay for the detection of the β-lactamases cAmpC and CMY-2-like, the porins OmpC and OmpF, and three “housekeeping” proteins for quality control purpose.
Original E. coli isolates and mutants stored at −80°C were thawed and subcultured on Trypticase™ Soy Agar II plates supplemented with 5% sheep blood (Becton Dickinson, New Jersey, United States) and used to inoculate 4 ml of MH broth. After culturing for at least 5 h at 37°C and 150 rpm, suspensions were diluted using MH broth to an absorbance of 0.98–1.02 at 600 nm wavelength (OD600 DiluPhotometer™, München, Germany). Thereafter, 1 ml was transferred and centrifuged for 5 min at 21,000 g. Pellets were washed once with 500 μl of phosphate-buffered saline solution (pH 7.3 ± 0.2, NaCl 8.0 g/L, KCl 0.2 g/L, Na2HPO3 1.15 g/L, KH2PO4 0.2 g/L) and stored at −20°C. After thawing, bacterial pellets were suspended in 100 μl of water with 5% sodium deoxycholate and 7.5 mM dithiothreitol and SIL peptides were added (final concentrations in sample digest of 5 fmol/μl for CMY-2-like, OmpF and OmpC peptides, and 2.5 fmol/μl for cAmpC peptides). Samples were then sonicated for 5 min (Branson 2510 Ultrasonic Cleaner, Branson Ultrasonics, Danbury, United States) and incubated for 10 min at 80°C, 450 rpm. Next, 900 μl of water, 50 μl of Tris–HCl buffer (50 mM, pH 8) and 10 μl of trypsin (1 μg/μl) (Worthington, NJ, United States) were added, and samples were digested for 2 h at 37°C and 450 rpm. Subsequently, 30 μl of 5% trifluoroacetic acid was added, and samples were incubated for 5 min at 37°C and 450 rpm. Finally, samples were centrifuged for 10 min at 21,000 g and 4°C, and 500 μl of the supernatant was stored at −20°C.
Stored digests were thawed and loaded onto Evotips (Evosep, Odense, Denmark) according to the manufacturer’s instructions and as previously described (Bache et al., 2018; Foudraine et al., 2019). LC-MS/MS was performed using the Evosep One (Evosep, Odense, Denmark) coupled to an Orbitrap mass spectrometer (Q Exactive HF Hybrid Quadrupole-Orbitrap, Thermo Fisher Scientific, Bremen, Germany). LC was performed using the manufacturer’s separation method of 11.5 min (100 samples/day) (Bache et al., 2018). The Q Exactive HF system was operated in parallel reaction monitoring (PRM) mode. The following settings were used: a quadrupole isolation window of 0.6 m/z units, an automatic gain control target value of 1 × 106 ions, a maximum fill time of 150 ms and a resolving power of 30,000 at 400 m/z. A normalized collision energy of 27% was used for all peptides. Measurements were unscheduled in the peptide selection experiments. Measurements were scheduled with retention windows of 2 min for each peptide in the subsequent experiments. LC-MS/MS data was analyzed in Skyline daily 19.1 or later (MacCoss Lab Software, University of Washington, United States). The mass spectrometry proteomics data have been deposited to the n ProteomeXchange Consortium via the PRIDE (Perez-Riverol et al., 2019) partner repository with the dataset identifier PXD025363.
For the preparation of whole cell lysates, bacteria were cultured at 37°C in lysogeny broth. For the detection of porins, cells were collected by centrifugation from overnight grown cultures, resuspended in 50 mM Tris–HCl, 5 mM EDTA, pH 8.5, and disrupted by ultrasonication (Branson B-12, Emerson, St. Louis, MO, United States). For the detection of β-lactamases, cells from exponentially growing cultures were first converted to spheroplasts and then disrupted by sonication after a freeze-thaw cycle as described (Paltansing et al., 2015). Sodium dodecyl sulfate-polyacrylamide gel electrophoresis (SDS-PAGE) analysis of the lysates was performed according to Laemmli (1970). To improve the separation of the porins OmpC and OmpF, gels were used containing 5 M urea. For Western blot analysis of the porins OmpC and OmpF, a polyclonal antiserum raised against the E. coli porin PhoE was used that was previously shown to cross-react with OmpC and OmpF (Goessens et al., 2013). The E. coli cAmpC and CMY-2-like β-lactamases were detected using antisera previously described (Goessens et al., 2013; van Boxtel et al., 2017).
Genomes of the original isolates and selected mutants were sequenced by Novogene (Beijing, China) using an Illumina HiSeq X Ten system. The genomes were assembled using Unicycler v0.4 with default parameters (Wick et al., 2017). A cgMLST analysis was performed for E. coli strains based on SeqSphere+3 to assess isolate heterogeneity and rule out contamination. To screen for the presence of additional β-lactamase genes, all genome sequences were analyzed using ABRicate4 and the CARD database, version 3.0.9 (Alcock et al., 2020). The coverage of contigs containing blaCMY–2–like and blaCTX–M–15 genes were compared to the coverage of chromosomal contigs in each isolate using the coverage depths calculated by the Unicycler and CLC (Qiagen, Hilden, Germany) assemblers.
Coding sequences for the investigated genes were extracted from the genomic assemblies using the sequence extraction tool in BioNumerics v7.6 software (Applied Maths, St.-Martens-Latem, Belgium). Reference sequences for the extraction were taken from the genome of E. coli MG1655 (NC_000913). Extracted sequences were translated and the protein sequences were compared to that of the parent strain to identify amino-acid changes. If no full-length coding sequence could be extracted, the structural integrity of the gene was verified by read-mapping using the CLC Genomics Workbench (Qiagen, Hilden, Germany). Mapped reads were visually inspected for issues (Supplementary Figure 1).
Peptides were selected for the detection of CMY-2-like, cAmpC, OmpC and OmpF using LC-MS/MS (Table 1). To assess if the peptides were specific, in silico specificity analysis was performed for all candidate peptides, and the results are described here for the selected peptides. The peptide SSSDLLR (cAmpC) was also present in a mechanosensitive ion channel of Burkholderia oklahomensis. The peptide NYPNPAR (cAmpC) was also present in the class C β-lactamase EDC-1 of Edwarsiella tarda (Naas et al., 2017). The peptide TEQQIADIVNR (CMY-2-like) was also present in the CMY-2-like similar class C β-lactamase EC-68 of an uncultured bacterium (Naas et al., 2017). The selected OmpC and OmpF peptides were not present in non-porin proteins in Enterobacterales. However, there is significant homology between porin sequences from different Enterobacterales spp., and the selected OmpC and OmpF peptides were also present in OmpC and OmpF orthologs of other bacterial species.
Of the 12 strains cultured under increasing meropenem concentrations, four strains showed an approximate 100-fold increase in meropenem MICs resulting in MICs of 4 mg/L or higher. This trend was found in all three replicate experiments. Three strains showed a similar increase, but in only one out of the three replicate experiments, and no increase or a moderate 5 to 10-fold increase in the two other replicate experiments. Experiments for the remaining strains showed a moderate increase in MICs or no increase at all. For the subsequent analysis, the strains that became resistant to meropenem (MICs ≥ 8 mg/L) were selected in order to analyze the underlying mechanisms. Therefore, one replicate series was selected for each of the four strains that showed significant MIC increases in all replicate experiments (R1281, B2591, R2723, R2761), one replicate series of strain B2582 was selected that showed a significant increase in MICs while the other replicate series did not, and one replicate series was selected which only showed a modest increase in MICs (R1810). Key characteristics of the selected strains and their mutants are shown in Table 2. None of the strain carried carbapenemase genes as determined by WGS.
Table 2. Characteristics of all mutants and identified causes for the loss of OmpC and/or OmpF, and the increase in CMY-2-like β-lactamases at the DNA level.
A significant decrease of OmpC was observed in all five selected series that developed meropenem resistance (Figures 2A–E), but not in the control series (Figure 2F). The first mutants of series R2723 and R2761 already showed a decrease in OmpC, observed by both LC-MS/MS and Western blot analysis (Figures 2A,B). The other three series, i.e., B2591, R1281 and B2582 showed a delayed decrease of OmpC (Figures 2C,D,E). In all mutant isolates in which OmpC was detected on Western blot, all four selected peptides were detected by LC-MS/MS as well. In addition, in the mutant isolates in which OmpC was not detected on Western blot, one to three peptides of OmpC were still detected at low ratios and only when mutations resulting in premature stop codons occurred after the corresponding peptide coding regions (Tables 1, 2). The OmpC peptide VAFAGLK was even detected in all mutant isolates. OmpC remained clearly detectable in the control series R1810 by both Western blot analysis and LC-MS/MS analysis. Remarkably, OmpC abundance even increased in mutants 2–5 of this series suggesting an increase in expression (Figure 2F).
Figure 2. Analysis of the presence of CMY-2-like, cAmpC, and the porins OmpC and OmpF in mutant isolates of different E. coli strains selected under increasing meropenem concentrations using LC-MS/MS and Western blotting. On the Y-axis the meropenem minimum inhibitory concentration and mass spectrometry signal intensity of each endogenous peptide divided by signal intensity of each stable isotope labeled (SIL) peptide. When a peptide was not detected in a mutant isolate, the corresponding symbol was left out. All detected peptides were shown even when ratios to SIL approached zero. Molecular weight standard proteins are indicated (in kDa) at the left of the blots. Strains Top10F’-p2761 and Top10F’-pmr42 were used in the Western blot analysis as positive controls for CMY-2 and strain 187 as a positive control for cAmpC. (A–F) Represent the different clinical strains referred to throughout the “Results” section.
OmpF was only detected by LC-MS/MS in the first three mutants of series R2723 and B2591 but not on Western blot. In strain R2761, only one peptide of OmpF was detected in mutants 1 and 2. Interestingly, OmpF expression increased in mutant 4 of series R1281 (Figure 2D) as observed by both LC-MS/MS and Western blotting, while both porins were less abundant or absent in subsequent mutants. In series B2582, OmpF was detected in all mutants using LC-MS/MS with low endogenous to SIL peptide ratios of around 0.05, whilst it was abundantly detected by Western blot analysis, except in mutant six (Figure 2E). In the control series, OmpF was detected in all mutants by both LC-MS/MS and Western blot analysis.
After a decrease in OmpC and OmpF, CMY-2 β-lactamase abundance increased with a concomitant increase in meropenem MICs in the series R2723, B2591, R1281 and B2582. A similar increase in meropenem MICs was observed in series R2761 while CMY-2 abundance did not increase (Figure 2B). In the control series R1810, neither the CMY-2 abundance nor the meropenem MICs increased. Interestingly, in contrast to CMY-2-like, cAmpC was not detected by LC-MS/MS in any of the original isolates nor in the mutants derived from them, but it was detected in the cAmpC positive control strain (data not shown). This indicated that the increase in meropenem MICs of the mutants was not caused by an increase in cAmpC production.
To analyze the underlying genetic alterations possibly responsible for the decrease in porins and increase in CMY-2 β-lactamases, WGS analysis was performed on the parental and mutant isolates (Table 2). Mutations in ompC genes resulting in premature stop codons or ompC gene interruptions coincided with modest increases in meropenem MICs in all strains, except in the control strain R1810 in which no mutations in ompC occurred. In strain R2723, different mutations were responsible for the loss of OmpC in different mutants. A mutation resulting in a stop codon (Q267X) was observed in the first mutant, a gene interruption was observed in the second mutant, and a mutation resulting in Q376X which only resulted in the loss of the last three OmpC amino acids was observed in the third mutant. A different gene interruption was observed in mutants four to seven. In strains R2761, B2591, and R1281, the mutations resulting in Y152X, Y344X, and Q104X were responsible for the loss of OmpC, respectively. Finally, in strain B2582, a mutation resulting in Q171X was responsible for the loss of OmpC, but remarkably this mutation was not observed in the last mutant. In addition, no other mutations that could explain the loss of OmpC were found in the ompC gene of this mutant.
Of the three strains that lost OmpF, gene interruptions were responsible for its loss in strain R2723 and B2591. In strain B2591, one gene interruption occurred in mutant four, while a different interruption was observed in mutants five to seven. In strain R1281, no mutations were detected in the ompF gene that accounted for the sudden increase and subsequent loss of OmpF.
In addition to porin genes, blaCMY–2–like genes were analyzed in the original isolates and in their mutants to reveal the mechanisms responsible for the observed increases in CMY-2-like β-lactamases (Table 2). All blaCMY–2–like genes appeared to be located on plasmids or were flanked by an insertion sequence element and BLAST analysis of the corresponding contigs showed near complete similarity to known plasmid sequences. No mutations were demonstrated in the promoter regions of the blaCMY–2–like genes indicating that an increase in gene copy numbers, e.g., by loss of plasmid copy-number control (van Boxtel et al., 2017), was most likely responsible for the increase in CMY-2 β-lactamases. This was confirmed by comparing the blaCMY–2–like gene coverage in each mutant with the coverage in the original isolate. All final mutants that showed an in increase in CMY-2 abundance also showed an increase in gene coverage by at least sevenfold. In strain R2761, no increase in coverage of blaCMY–2–like occurred; however, a 4.5-fold increase in coverage of blaCTX–M–15 was observed in the final mutant of strain R2761 compared to the coverage in the original isolate.
The increasing prevalence of CRE is threatening the success of β-lactam antibiotics (Brolund et al., 2019). While carbapenemases are the usual cause of carbapenem resistance, a decrease in membrane permeability and a concomitant increase of certain non-carbapenemase β-lactamases can also cause carbapenem resistance in Enterobacterales (Oteo et al., 2008; Adler et al., 2013; Goessens et al., 2013; Tangden et al., 2013; Miller and Humphries, 2016; Nordmann and Poirel, 2019). Several authors have previously described carbapenem resistance in E. coli with ESBL and/or AmpC-type β-lactamase genes that lack porins OmpC and/or OmpF (Oteo et al., 2008; Yang et al., 2010; Wozniak et al., 2012; Tangden et al., 2013; Ho et al., 2016; Nuramrum et al., 2017; Chang et al., 2019; Tian et al., 2020). Others have further characterized isolates in which this occurred and performed additional genetic experiments (Mammeri et al., 2008; Girlich et al., 2009; Adler et al., 2013; Goessens et al., 2013; van Boxtel et al., 2017). However, non-CP-CRE have never been studied using an advanced protein detection method such as LC-MS/MS. Furthermore, while several techniques are available for both the detection of carbapenemase activity (Tamma and Simner, 2018) as well as for the identification of specific carbapenemases (Boutal et al., 2018; Traczewski et al., 2018), no accepted assay is currently available to detect (a lack of) porins in routine diagnostic medical microbiology laboratories. This is unfortunate, as a lack of porins together with the presence of non carbapenemase β-lactamases is responsible for carbapenem resistance in a significant number of E. coli and other Enterobacterales (Nordmann and Poirel, 2019). Rapid detection of this combination could aid in earlier selection of optimal antibiotic therapy that is not affected by a lack of porins and/or the presence of AmpC-type β-lactamases.
In the current study, several clinical E. coli isolates were cultured under increasing meropenem concentrations, and the underlying mechanisms of the resulting resistant mutants were studied using a newly developed LC-MS/MS assay, Western blotting, and WGS. First, we used a genoproteomic approach to select tryptic peptides for the detection of the E. coli porins OmpC and OmpF, and for the β-lactamases CMY-2-like and cAmpC. Since genes are prone to mutations, conserved protein regions were selected in which these mutations do not frequently occur. Further, specificity was assessed for all porin peptides to assure correct identification of the porins of interest. For CMY-2-like, two peptides were selected that together covered all CMY-2-like β-lactamases. Both peptides were previously used for the detection of CMY-2-like as well, albeit using MALDI-TOF mass spectrometry (Hart et al., 2015). For cAmpC, the three selected peptides together covered 373 of the 395 annotated AmpC variants in the Beta-Lactamase Database (Naas et al., 2017). Detection of all of the selected peptides and additional SIL peptides was combined into a single multiplex assay with a time-to-result of less than 4 h.
When peptides for other resistance mechanisms, such as ESBLs, carbapenemases (Foudraine et al., 2019), fluoroquinolone resistance mechanisms (Hassing et al., 2016), and aminoglycoside resistance mechanisms (Foudraine et al., 2021a) would be added to the current panel of CMY, E. coli cAmpC, OmpC and OmpF, a single multiplex LC-MS/MS assay would be able to detect several prevalent mechanisms that confer resistance to beta-lactams, fluoroquinolones and aminoglycosides in for instance E. coli. While such an assay could be used to confirm or rule out the presence of specific resistance mechanisms after routine susceptibility testing, it may be more beneficial to use it as a rapid screening test for important liquid cultures such as blood cultures. If the currently used protocol would be automated, such an assay could compete in turnaround time with other assays used for blood cultures including rapid phenotypic and genotypic methods (Idelevich and Becker, 2019; Banerjee and Humphries, 2021). In addition, it would offer the ability to detect proteins semi-quantitatively which offers unique information compared to other techniques (Charretier and Schrenzel, 2016; Wan Nur Ismah et al., 2018; Foudraine et al., 2021b).
To study the sequential development of carbapenem resistance in E. coli carrying blaCMY–2–like genes and to evaluate the ability of the developed LC-MS/MS assay to simultaneously detect CMY-2 and porins, we exposed twelve blaCMY–2–like positive strains to increasing meropenem concentrations and selected six strains for further analysis. A comparable selection procedure has previously been demonstrated to result in mutations similar to those found in clinical non-carbapenemase producing meropenem-resistant E. coli (van Boxtel et al., 2017). In all of our E. coli strains that developed meropenem resistance, a decrease in porin abundance was observed by LC-MS/MS which coincided with interruptions or premature stop codons in the corresponding genes. The timing of this occurrence differed as for instance strains R2723 and R2761 lost their porin OmpC significantly earlier during the selection procedure than strains B2582 and B2591. These differences might be explained by the random timing of mutation events. Alternatively, it could also be hypothesized that bacteria need a certain genomic background in order to overcome a drastic event, such as the sudden loss of porins, which is expected to lead to nutritional deficiency (Ferenci, 2005). If bacteria are not prepared for such an event through mutational adaptation, they will experience a severe growth defect, and they will quickly be outcompeted by fitter strains (Adler et al., 2013; Knopp and Andersson, 2015). Another explanation may be the number of porins initially present. Strains with a single porin are more likely to lose this porin when this is advantageous than strains with two or more porins as the effect of losing one of two porins will not offer much benefit in inhibiting β-lactam uptake. Furthermore, the loss of two or more porins caused by mutational events at the same time is not that likely.
Gene interruptions in the mutants studied were most likely caused by insertion sequence elements in the coding regions of ompC and/or ompF (Fernandez and Hancock, 2012; van Boxtel et al., 2017). Unfortunately, this could not be confirmed in the current analysis due to the use of short-read sequencing.
In four of the five strains that became meropenem resistant, the increase in CMY-2 β-lactamase coincided with an increase in gene copy numbers as demonstrated by an increase in gene-coverage. Interestingly, the decrease in OmpC preceded the increase of CMY-2 in all four strains. The necessity of a decrease in OmpC prior to an increase in CMY-2-like was shown by the control series R1810. In this series, no decrease in OmpC was observed and it could be hypothesized that some tolerability to meropenem is required before additional mutations can occur. In mutant series R2761, a decrease in OmpC preceded an increase in meropenem MICs but an increase in CMY-2-like abundance was not observed by LC-MS/MS. This was in contrast to the results of a previous study, which did show an increase in CMY-2 abundance due to the loss of plasmid copy-number control when meropenem resistance developed in strain R2761 (van Boxtel et al., 2017). Differences between the previous and the current study could be explained by the different mutant selection procedures used. In the current study, single colonies were used that derived from an ongoing liquid culture which resulted in different subpopulations with distinct mutations. Furthermore, the stored colonies were thawed and sampled multiple times to obtain fresh cultures for LC-MS/MS, WGS and Western blot analysis which could have resulted in additional discrepancies. In contrast to the other isolates, strain R2761 also carried a blaOXA–1 and a blaCTX–M gene, which together with the decrease in porins could have played a role in development of meropenem resistance. An increase in CTX-M and a decrease in porins was also previously demonstrated to result in meropenem resistance in E. coli (Adler et al., 2013). Expression of chromosomal ampC was neither observed by LC-MS/MS nor by Western blotting in any of the mutants. This could be due to the fact that these strains already expressed CMY-2-like β-lactamases, and there are several DNA alterations required before regular E. coli turn into cAmpC hyperproducers (Livermore, 1995; Paltansing et al., 2015), and perhaps exposure to meropenem does not select for chromosomal AmpC mutants.
Overall, most LC-MS/MS observations were in agreement with the results of the Western blot analysis, however, some observed differences are worth mentioning. The peptide VAFAGLK (OmpC) was detected in all isolates, even when the porin was no longer detected on Western blot. This indicated that the part of ompC before a premature stopcodon or gene interruption was still transcribed and translated, and that some peptides from this part could still be detected using the highly sensitive LC-MS/MS assay. Only when all four OmpC peptides were detected, the porin was also detected by Western blotting. In general, OmpF abundance was significantly lower than OmpC abundance, and in some of the mutants, OmpF was only detected by LC-MS/MS. In strain B2582, Western blot analysis most likely detected another porin instead of OmpF (e.g., NmpC, OmpN, PhoE or a porin encoded by a prophage) (Nikaido, 2003) as it was no longer detected in mutant six (as opposed to LC-MS/MS results), and LC-MS/MS ratios for OmpF peptides were only around 0.05 while Western blot analysis showed clear bands. Apparently, this porin was important for the uptake of meropenem, as meropenem MICs increased substantially after its loss.
The current study is in line with previous studies which demonstrated that a decrease in porins and an increase in AmpC-type β-lactamases results in carbapenem resistance in E. coli (Mammeri et al., 2008, 2010; Oteo et al., 2008; Goessens et al., 2013; van Boxtel et al., 2017). In addition, this study shows that LC-MS/MS can be applied for the rapid semi-quantitative analysis of CMY-2-like β-lactamases and porins, which could be useful in the routine diagnostic medical microbiology laboratory for the early detection of non-CP-CRE and the differentiation from CP-CRE.
The datasets presented in this study can be found in online repositories. The names of the repository/repositories and accession number(s) can be found below: https://www.ebi.ac.uk/ena, PRJEB44241, ERP128274; http://www.proteomexchange.org/, PXD025363.
WG, JT, DF, AW, CK, and TL designed the study. CA, DF, AW, RB, MK, and LD performed and/or supervised the laboratory work. CA, DF, AW, RB, CK, NS, LD, JT, and WG analyzed the data. NS and LD curated the data in online repositories. WG, JT, TL, CK, JH, and AV supervised the study. WG and TL obtained project funding. WG performed the project administration. CA, AW, RB, CK, and DF visualized the data. DF and CA wrote the first version of the manuscript. All authors edited and approved the manuscript.
This work was supported by both Netherlands Enterprise Agency and the European Union Through Horizon 2020 by means of a Eurostars grant (project number 18128). In addition, this project was in part supported by a research grant from the Dutch ZonMw organization (project number 205200006).
The authors declare that the research was conducted in the absence of any commercial or financial relationships that could be construed as a potential conflict of interest.
All claims expressed in this article are solely those of the authors and do not necessarily represent those of their affiliated organizations, or those of the publisher, the editors and the reviewers. Any product that may be evaluated in this article, or claim that may be made by its manufacturer, is not guaranteed or endorsed by the publisher.
We would like to thank Evosep Biosystems for allowing us to work with the Evosep One. We are grateful to Vernon Brown, Sander ten Have, Inge van der Heijden, and Heleen van der Spek for their substantial effort in culturing the strains. We are grateful to Merel Raaphorst and Willemien Zandijk for their respective aid in LC-MS/MS and WGS sample pre-treatment. We would also like to thank Eva Nijsten for her work in characterizing one of the strains.
The Supplementary Material for this article can be found online at: https://www.frontiersin.org/articles/10.3389/fmicb.2022.793738/full#supplementary-material
Supplementary Figure 1 | Application of read-mapping to check the structural integrity of genes. The example shows the read-mapping results of the B2591 parent strain and its mutants for the ompF gene using the gene from E. coli MG1655 plus 100 nucleotide flanking sequences as a reference. Colors in the cumulative coverage graph and from individual reads are as follows. Dark-blue: forward paired read; light blue: reverse paired read; green: forward unpaired read; red: reverse unpaired read. The vertical colored lines indicate sequence variations relative to the reference sequence. Below the cumulative coverage graph, a fraction of the individual reads are shown. The red oval indicates regions where the structural integrity of the gene was affected. In mutant 4, the gene was affected at a different position than in mutants 5–7.
Adler, M., Anjum, M., Andersson, D. I., and Sandegren, L. (2013). Influence of acquired beta-lactamases on the evolution of spontaneous carbapenem resistance in Escherichia coli. J. Antimicrob. Chemother. 68, 51–59. doi: 10.1093/jac/dks368
Alcock, B. P., Raphenya, A. R., Lau, T. T. Y., Tsang, K. K., Bouchard, M., Edalatmand, A., et al. (2020). CARD 2020: antibiotic resistome surveillance with the comprehensive antibiotic resistance database. Nucleic Acids Res. 48, D517–D525. doi: 10.1093/nar/gkz935
Bache, N., Geyer, P. E., Bekker-Jensen, D. B., Hoerning, O., Falkenby, L., Treit, P. V., et al. (2018). A Novel LC system embeds analytes in pre-formed gradients for rapid, ultra-robust proteomics. Mol. Cell Proteomics 17, 2284–2296. doi: 10.1074/mcp.TIR118.000853
Banerjee, R., and Humphries, R. (2021). Rapid antimicrobial susceptibility testing methods for blood cultures and their clinical impact. Front. Med. (Lausanne) 8:635831. doi: 10.3389/fmed.2021.635831
Boutal, H., Vogel, A., Bernabeu, S., Devilliers, K., Creton, E., Cotellon, G., et al. (2018). A multiplex lateral flow immunoassay for the rapid identification of NDM-, KPC-, IMP- and VIM-type and OXA-48-like carbapenemase-producing Enterobacteriaceae. J. Antimicrob. Chemother. 73, 909–915. doi: 10.1093/jac/dkx521
Brolund, A., Lagerqvist, N., Byfors, S., Struelens, M. J., Monnet, D. L., Albiger, B., et al. (2019). Worsening epidemiological situation of carbapenemase-producing Enterobacteriaceae in Europe, assessment by national experts from 37 countries, July 2018. Euro Surveill. 24:1900123. doi: 10.2807/1560-7917.ES.2019.24.9.1900123
Cecchini, T., Yoon, E. J., Charretier, Y., Bardet, C., Beaulieu, C., Lacoux, X., et al. (2018). Deciphering multifactorial resistance phenotypes in Acinetobacter baumannii by genomics and targeted label-free proteomics. Mol. Cell Proteomics 17, 442–456. doi: 10.1074/mcp.RA117.000107
Chang, Y. T., Siu, L. K., Wang, J. T., Wu, T. L., Chen, Y. H., Chuang, Y. C., et al. (2019). Resistance mechanisms and molecular epidemiology of carbapenem-nonsusceptible Escherichia coli in Taiwan, 2012-2015. Infect. Drug Resist. 12, 2113–2123.
Charretier, Y., and Schrenzel, J. (2016). Mass spectrometry methods for predicting antibiotic resistance. Proteomics Clin. Appl. 10, 964–981. doi: 10.1002/prca.201600041
Charretier, Y., Kohler, T., Cecchini, T., Bardet, C., Cherkaoui, A., Llanes, C., et al. (2015b). Label-free SRM-based relative quantification of antibiotic resistance mechanisms in Pseudomonas aeruginosa clinical isolates. Front. Microbiol. 6:81. doi: 10.3389/fmicb.2015.00081
Charretier, Y., Dauwalder, O., Franceschi, C., Degout-Charmette, E., Zambardi, G., Cecchini, T., et al. (2015a). Rapid bacterial identification, resistance, virulence and type profiling using selected reaction monitoring mass spectrometry. Sci. Rep. 5:13944. doi: 10.1038/srep13944
Choi, U., and Lee, C. R. (2019). Distinct roles of outer membrane porins in antibiotic resistance and membrane integrity in Escherichia coli. Front. Microbiol. 10:953. doi: 10.3389/fmicb.2019.00953
Delcour, A. H. (2009). Outer membrane permeability and antibiotic resistance. Biochim. Biophys. Acta 1794, 808–816.
Espinosa, R. F., Rumi, V., Marchisio, M., Cejas, D., Radice, M., Vay, C., et al. (2018). Fast and easy detection of CMY-2 in Escherichia coli by direct MALDI-TOF mass spectrometry. J. Microbiol. Methods 148, 22–28. doi: 10.1016/j.mimet.2018.04.001
Ferenci, T. (2005). Maintaining a healthy SPANC balance through regulatory and mutational adaptation. Mol. Microbiol. 57, 1–8. doi: 10.1111/j.1365-2958.2005.04649.x
Fernandez, L., and Hancock, R. E. (2012). Adaptive and mutational resistance: role of porins and efflux pumps in drug resistance. Clin. Microbiol. Rev. 25, 661–681. doi: 10.1128/CMR.00043-12
Foudraine, D. E., Dekker, L. J. M., Strepis, N., Bexkens, M. L., Klaassen, C. H. W., Luider, T. M., et al. (2019). Accurate detection of the four most prevalent carbapenemases in E. coli and K. pneumoniae by high-resolution mass spectrometry. Front. Microbiol. 10:2760. doi: 10.3389/fmicb.2019.02760
Foudraine, D. E., Strepis, N., Klaassen, C. H. W., Raaphorst, M. N., Verbon, A., Luider, T. M., et al. (2021a). Rapid and accurate detection of aminoglycoside modifying enzymes and 16S ribosomal RNA methyltransferases by targeted LC-MS/MS. J. Clin. Microbiol. 59:e0046421. doi: 10.1128/JCM.00464-21
Foudraine, D. E., Strepis, N., Stingl, C., Ten Kate, M. T., Verbon, A., Klaassen, C. H. W., et al. (2021b). Exploring antimicrobial resistance to beta-lactams, aminoglycosides and fluoroquinolones in E. coli and K. pneumoniae using proteogenomics. Sci. Rep. 11:12472. doi: 10.1038/s41598-021-91905-w
Girlich, D., Poirel, L., and Nordmann, P. (2009). CTX-M expression and selection of ertapenem resistance in Klebsiella pneumoniae and Escherichia coli. Antimicrob. Agents Chemother. 53, 832–834. doi: 10.1128/AAC.01007-08
Goessens, W. H., van der Bij, A. K., van Boxtel, R., Pitout, J. D., van Ulsen, P., Melles, D. C., et al. (2013). Antibiotic trapping by plasmid-encoded CMY-2 beta-lactamase combined with reduced outer membrane permeability as a mechanism of carbapenem resistance in Escherichia coli. Antimicrob. Agents Chemother. 57, 3941–3949. doi: 10.1128/AAC.02459-12
Grundmann, H., Glasner, C., Albiger, B., Aanensen, D. M., Tomlinson, C. T., Andrasevic, A. T., et al. (2017). Occurrence of carbapenemase-producing Klebsiella pneumoniae and Escherichia coli in the European survey of carbapenemase-producing Enterobacteriaceae (EuSCAPE): a prospective, multinational study. Lancet Infect. Dis. 17, 153–163. doi: 10.1016/S1473-3099(16)30257-2
Hart, P. J., Wey, E., McHugh, T. D., Balakrishnan, I., and Belgacem, O. (2015). A method for the detection of antibiotic resistance markers in clinical strains of Escherichia coli using MALDI mass spectrometry. J. Microbiol. Methods 111, 1–8.
Hassing, R. J., Goessens, W. H., Zeneyedpour, L., Sultan, S., van Kampen, J. J., Verbon, A., et al. (2016). Detection of amino acid substitutions in the GyrA protein of fluoroquinolone-resistant typhoidal Salmonella isolates using high-resolution mass spectrometry. Int. J. Antimicrob. Agents 47, 351–356. doi: 10.1016/j.ijantimicag.2016.01.018
Hawkey, P. M., and Livermore, D. M. (2012). Carbapenem antibiotics for serious infections. BMJ 344:e3236. doi: 10.1136/bmj.e3236
Ho, P. L., Cheung, Y. Y., Wang, Y., Lo, W. U., Lai, E. L., Chow, K. H., et al. (2016). Characterization of carbapenem-resistant Escherichia coli and Klebsiella pneumoniae from a healthcare region in Hong Kong. Eur. J. Clin. Microbiol. Infect. Dis. 35, 379–385.
Hu, Y. Y., Cai, J. C., Zhou, H. W., Zhang, R., and Chen, G. X. (2015). Rapid detection of porins by matrix-assisted laser desorption/ionization-time of flight mass spectrometry. Front. Microbiol. 6:784. doi: 10.3389/fmicb.2015.00784
Idelevich, E. A., and Becker, K. (2019). How to accelerate antimicrobial susceptibility testing. Clin. Microbiol. Infect. 25, 1347–1355. doi: 10.1016/j.cmi.2019.04.025
Intelicato-Young, J., and Fox, A. (2013). Mass spectrometry and tandem mass spectrometry characterization of protein patterns, protein markers and whole proteomes for pathogenic bacteria. J. Microbiol. Methods 92, 381–386. doi: 10.1016/j.mimet.2013.01.004
Iovleva, A., and Doi, Y. (2017). Carbapenem-resistant Enterobacteriaceae. Clin. Lab. Med. 37, 303–315.
Jaffe, A., Chabbert, Y. A., and Semonin, O. (1982). Role of porin proteins OmpF and OmpC in the permeation of beta-lactams. Antimicrob. Agents Chemother. 22, 942–948. doi: 10.1128/AAC.22.6.942
Knopp, M., and Andersson, D. I. (2015). Amelioration of the fitness costs of antibiotic resistance due to reduced outer membrane permeability by upregulation of alternative Porins. Mol. Biol. Evol. 32, 3252–3263. doi: 10.1093/molbev/msv195
Laemmli, U. K. (1970). Cleavage of structural proteins during the assembly of the head of bacteriophage T4. Nature 227, 680–685. doi: 10.1038/227680a0
Livermore, D. M. (1995). beta-Lactamases in laboratory and clinical resistance. Clin. Microbiol. Rev. 8, 557–584. doi: 10.1128/CMR.8.4.557
Mammeri, H., Guillon, H., Eb, F., and Nordmann, P. (2010). Phenotypic and biochemical comparison of the carbapenem-hydrolyzing activities of five plasmid-borne AmpC beta-lactamases. Antimicrob. Agents Chemother. 54, 4556–4560. doi: 10.1128/AAC.01762-09
Mammeri, H., Nordmann, P., Berkani, A., and Eb, F. (2008). Contribution of extended-spectrum AmpC (ESAC) beta-lactamases to carbapenem resistance in Escherichia coli. FEMS Microbiol. Lett. 282, 238–240. doi: 10.1111/j.1574-6968.2008.01126.x
Miller, S., and Humphries, R. M. (2016). Clinical laboratory detection of carbapenem-resistant and carbapenemase-producing Enterobacteriaceae. Expert Rev. Anti Infect. Ther. 14, 705–717. doi: 10.1080/14787210.2016.1206815
Naas, T., Oueslati, S., Bonnin, R. A., Dabos, M. L., Zavala, A., Dortet, L., et al. (2017). Beta-lactamase database (BLDB) – structure and function. J. Enzyme Inhib. Med. Chem. 32, 917–919. doi: 10.1080/14756366.2017.1344235
Nikaido, H. (2003). Molecular basis of bacterial outer membrane permeability revisited. Microbiol. Mol. Biol. Rev. 67, 593–656. doi: 10.1128/MMBR.67.4.593-656.2003
Nordmann, P., and Poirel, L. (2019). Epidemiology and diagnostics of carbapenem resistance in gram-negative bacteria. Clin. Infect. Dis. 69, S521–S528. doi: 10.1093/cid/ciz824
Nordmann, P., Naas, T., and Poirel, L. (2011). Global spread of carbapenemase-producing Enterobacteriaceae. Emerg. Infect. Dis. 17, 1791–1798.
Nuramrum, S., Chanawong, A., Lunha, K., Lulitanond, A., Sangka, A., Wilailuckana, C., et al. (2017). Molecular characterization of carbapenemase-nonproducing clinical isolates of Escherichia coli (from a Thai University Hospital) with reduced carbapenem susceptibility. Jpn. J. Infect. Dis. 70, 628–634. doi: 10.7883/yoken.JJID.2017.156
Oteo, J., Delgado-Iribarren, A., Vega, D., Bautista, V., Rodriguez, M. C., Velasco, M., et al. (2008). Emergence of imipenem resistance in clinical Escherichia coli during therapy. Int. J. Antimicrob. Agents 32, 534–537. doi: 10.1016/j.ijantimicag.2008.06.012
Oviano, M., and Bou, G. (2019). Matrix-assisted laser desorption ionization-time of flight mass spectrometry for the rapid detection of antimicrobial resistance mechanisms and beyond. Clin. Microbiol. Rev. 32:e00037–18. doi: 10.1128/CMR.00037-18
Pages, J. M., James, C. E., and Winterhalter, M. (2008). The porin and the permeating antibiotic: a selective diffusion barrier in Gram-negative bacteria. Nat. Rev. Microbiol. 6, 893–903. doi: 10.1038/nrmicro1994
Paltansing, S., Kraakman, M., van Boxtel, R., Kors, I., Wessels, E., Goessens, W., et al. (2015). Increased expression levels of chromosomal AmpC beta-lactamase in clinical Escherichia coli isolates and their effect on susceptibility to extended-spectrum cephalosporins. Microb. Drug Resist. 21, 7–16. doi: 10.1089/mdr.2014.0108
Pandey, N., and Cascella, M. (2020). Beta Lactam Antibiotics. Book Accesion: NBK545311. Available online at: https://www.ncbi.nlm.nih.gov/pubmed/31424895 (accessed April 12, 2021).
Perez-Perez, F. J., and Hanson, N. D. (2002). Detection of plasmid-mediated AmpC beta-lactamase genes in clinical isolates by using multiplex PCR. J. Clin. Microbiol. 40, 2153–2162. doi: 10.1128/JCM.40.6.2153-2162.2002
Perez-Riverol, Y., Csordas, A., Bai, J., Bernal-Llinares, M., Hewapathirana, S., Kundu, D. J., et al. (2019). The PRIDE database and related tools and resources in 2019: improving support for quantification data. Nucleic Acids Res. 47, D442–D450. doi: 10.1093/nar/gky1106
Renault, M., Tommassen-van Boxtel, R., Bos, M. P., Post, J. A., Tommassen, J., and Baldus, M. (2012). Cellular solid-state nuclear magnetic resonance spectroscopy. Proc. Natl. Acad. Sci. U.S.A. 109, 4863–4868. doi: 10.1073/pnas.1116478109
Tamma, P. D., and Simner, P. J. (2018). Phenotypic detection of carbapenemase-producing organisms from clinical isolates. J. Clin. Microbiol. 56:e01140–18. doi: 10.1128/JCM.01140-18
Tangden, T., Adler, M., Cars, O., Sandegren, L., and Lowdin, E. (2013). Frequent emergence of porin-deficient subpopulations with reduced carbapenem susceptibility in ESBL-producing Escherichia coli during exposure to ertapenem in an in vitro pharmacokinetic model. J. Antimicrob. Chemother. 68, 1319–1326. doi: 10.1093/jac/dkt044
Tian, X., Zheng, X., Sun, Y., Fang, R., Zhang, S., Zhang, X., et al. (2020). Molecular mechanisms and epidemiology of carbapenem-resistant Escherichia coli isolated from chinese patients during 2002-2017. Infect. Drug Resist. 13, 501–512.
Traczewski, M. M., Carretto, E., Canton, R., Moore, N. M., and Carba, R. S. T. (2018). Multicenter evaluation of the xpert carba-r assay for detection of carbapenemase genes in gram-negative isolates. J. Clin. Microbiol. 56:e00272–18. doi: 10.1128/JCM.00272-18
van Boxtel, R., Wattel, A. A., Arenas, J., Goessens, W. H., and Tommassen, J. (2017). Acquisition of carbapenem resistance by plasmid-encoded-AmpC-expressing Escherichia coli. Antimicrob. Agents Chemother. 61, e1413–e1416. doi: 10.1128/AAC.01413-16
Vergalli, J., Bodrenko, V. I., Masi, M., Moynie, L., Acosta-Gutierrez, S., Naismith, J. H., et al. (2020). Porins and small-molecule translocation across the outer membrane of Gram-negative bacteria. Nat. Rev. Microbiol. 18, 164–176. doi: 10.1038/s41579-019-0294-2
Wan Nur Ismah, W. A. K., Takebayashi, Y., Findlay, J., Heesom, K. J., Jimenez-Castellanos, J. C., Zhang, J., et al. (2018). Prediction of fluoroquinolone susceptibility directly from whole-genome sequence data by using liquid chromatography-tandem mass spectrometry to identify mutant genotypes. Antimicrob. Agents Chemother. 62:e01814–17. doi: 10.1128/AAC.01814-17
Wang, H., Chen, Y., Strich, J. R., Drake, S. K., Youn, J. H., Rosenberg, A. Z., et al. (2019). Rapid detection of colistin resistance protein MCR-1 by LC-MS/MS. Clin. Proteomics 16:8. doi: 10.1186/s12014-019-9228-2
Wang, H., Drake, S. K., Youn, J. H., Rosenberg, A. Z., Chen, Y., Gucek, M., et al. (2017). Peptide markers for rapid detection of KPC carbapenemase by LC-MS/MS. Sci. Rep. 7:2531. doi: 10.1038/s41598-017-02749-2
Wick, R. R., Judd, L. M., Gorrie, C. L., and Holt, K. E. (2017). Unicycler: resolving bacterial genome assemblies from short and long sequencing reads. PLoS Comput. Biol. 13:e1005595. doi: 10.1371/journal.pcbi.1005595
Wozniak, A., Villagra, N. A., Undabarrena, A., Gallardo, N., Keller, N., Moraga, M., et al. (2012). Porin alterations present in non-carbapenemase-producing Enterobacteriaceae with high and intermediate levels of carbapenem resistance in Chile. J. Med. Microbiol. 61, 1270–1279. doi: 10.1099/jmm.0.045799-0
Yang, Q., Wang, H., Sun, H., Chen, H., Xu, Y., and Chen, M. (2010). Phenotypic and genotypic characterization of Enterobacteriaceae with decreased susceptibility to carbapenems: results from large hospital-based surveillance studies in China. Antimicrob. Agents Chemother. 54, 573–577. doi: 10.1128/AAC.01099-09
Keywords: meropenem, E. coli, OmpC, OmpF, CMY-2-like, liquid chromatography-mass spectrometry, parallel reaction monitoring, antimicrobial resistance
Citation: Foudraine DE, Aarents CNM, Wattel AA, van Boxtel R, Strepis N, ten Kate MT, Verbon A, Luider TM, Klaassen CHW, Hays J, Dekker LJM, Tommassen J and Goessens WHF (2022) Liquid Chromatography-Tandem Mass Spectrometry Analysis Demonstrates a Decrease in Porins and Increase in CMY-2 β-Lactamases in Escherichia coli Exposed to Increasing Concentrations of Meropenem. Front. Microbiol. 13:793738. doi: 10.3389/fmicb.2022.793738
Received: 12 October 2021; Accepted: 26 January 2022;
Published: 28 February 2022.
Edited by:
Benjamin Andrew Evans, University of East Anglia, United KingdomReviewed by:
Jean-philippe Charrier, BioMérieux, FranceCopyright © 2022 Foudraine, Aarents, Wattel, van Boxtel, Strepis, ten Kate, Verbon, Luider, Klaassen, Hays, Dekker, Tommassen and Goessens. This is an open-access article distributed under the terms of the Creative Commons Attribution License (CC BY). The use, distribution or reproduction in other forums is permitted, provided the original author(s) and the copyright owner(s) are credited and that the original publication in this journal is cited, in accordance with accepted academic practice. No use, distribution or reproduction is permitted which does not comply with these terms.
*Correspondence: Dimard E. Foudraine, ZC5mb3VkcmFpbmVAZXJhc211c21jLm5s
Disclaimer: All claims expressed in this article are solely those of the authors and do not necessarily represent those of their affiliated organizations, or those of the publisher, the editors and the reviewers. Any product that may be evaluated in this article or claim that may be made by its manufacturer is not guaranteed or endorsed by the publisher.
Research integrity at Frontiers
Learn more about the work of our research integrity team to safeguard the quality of each article we publish.