- Biology and Biotechnology Lab, Department of Soil Science, University College of Agriculture and Natural Resources, University of Tehran, Karaj, Iran
This research aimed to optimize a lipopeptide biosurfactant produced from Bacillus sp. SHA302 due to its high efficiency of heavy metal release in soil. The results demonstrated that the metal release capacity of the lipopeptide biosurfactant alone increased with increasing the biosurfactant concentration. Among treatments with different biosurfactant concentrations plus acid, the highest metal release rates of 53.8% ± 1.4 and 39.3% ± 1.7 for Zn and Pb, respectively, were observed in the critical micelle concentration (CMC) + HCl treatment. The results of a factorial experiment designed for optimizing biosurfactant production showed that among five inexpensive carbon sources and six mineral nitrogen sources, sugar beet molasses (1%) and ammonium chloride (0.1%) were the most efficient sources in lowering the surface tension (ST) of the culture media to 32.2 ± 0.76 mN/m. The second step of the experiment was a Plackett–Burman design with 11 factors and showed that the four factors of pH, ammonium chloride, magnesium sulfate, and molasses significantly affected (P < 0.05) the changes in ST and biosurfactant production. The third step of the experiment was done using the response surface methodology (RSM) with a central composite design. The results showed that a pH of 7.3, 1.5 g/l of ammonium chloride, 0.3 g/l of magnesium sulfate, and 10% of sugar beet molasses yielded values of 29.2 ± 0.71 mN/m and 5.74 ± 0.52 g/l for the two variables of ST and biosurfactant production, respectively, which reached their most optimal levels.
Introduction
Heavy metals are considered permanent soil pollutants, especially in industrial countries (Santos et al., 2016). These pollutants can pose a hazard to human health and ecosystems through the food chain or entry into the soil and water resources. Heavy metal pollution is a concern globally (Chakraborty and Das, 2014; Mao et al., 2015). A promising solution to achieve low bioavailability of pollutants is the use of biosurfactants that play an essential role in the desorption of pollutants from the surface of soil particles. Biosurfactants increase the presence of pollutants in the solution phase, thereby increasing their availability to microorganisms that can break them down (Mao et al., 2015). Microorganisms produce surfactants to increase the solubility, biodegradation, and bioavailability of pollutants in their environments (Rane et al., 2017). Surfactants are amphiphilic molecules with various structural and functional groups and wide-ranging characteristics such as reducing surface and interfacial tension formation of micelle and microemulsion between two different phases. These characteristics increase the availability of the pollutants and their subsequent biodegradation (Pacwa-Płociniczak et al., 2011; Soberón-Chávez and Maier, 2011). Heavy metals are usually adsorbed as ions or charged ionic pairs to soil surfaces. Unlike organic pollutants, these compounds enter the soil solution either by forming non-ionic complexes or through an ionic exchange (Ochoa-Loza et al., 2001; Swarnkar et al., 2012).
Although biosurfactants have many benefits, their expensive production and low production quantity hinder their use in industry (Moussa et al., 2014). An effective method to reduce limitations in biosurfactant production is to determine optimal levels of nutrients and ideal conditions of microbial cultures (Kim and Kim, 2013). To optimize culture media for biosurfactant production, the culture conditions (temperature, shaking rate, pH, etc.) and media components (sources of nitrogen, carbon, micronutrients, etc.) should be identified and optimized among other constituents involved in the production (Singh et al., 2016). For industrial-scale biosurfactant production, the feedstock value can reach 50% of all production costs (Makkar et al., 2011). Therefore, the current research is focused on the development of production strategies such as culture media formulations using more economic substrates (de Oliveira et al., 2013). The usual optimization method includes changing one parameter at a time while other parameters are kept constant. Compared to the factorial design, this method usually does not show the interaction of parameters, a limitation that can be resolved using statistical methods such as response surface methodology (RSM) (Griffin et al., 1992; Malik and Kerkar, 2019). RSM is a vast statistical method in experimental design that predicts the best culture conditions through the evaluation of factor interactions with a minimal number of experiments (Myers et al., 2016). This statistical method has shown high efficiency in pollutant biodegradation processes and optimization of culture media components for the production of biological compounds, especially biosurfactants (Najafi et al., 2011; Huang et al., 2013; Salar et al., 2016).
Lipopeptide biosurfactants are produced from different Bacillus species with carbon sources and have a high potential for use in industry and bioremediation (Das and Mukherjee, 2007; Ghojavand et al., 2008). Therefore, the use of different bacterial species of this genus is highly advantageous for obtaining a valuable and effective bioremediation biosurfactant. Studies show that Bacillus spp. strains can use all recyclable sources, especially agricultural-industrial waste, as a carbon source, which further confirms the use of this genus and the related species for bioremediation (Das and Mukherjee, 2007).
This study aimed to evaluate a lipopeptide biosurfactant performance for eliminating heavy metal contamination from soil. In this study, the optimization of the production conditions of lipopeptide biosurfactant by Bacillus sp. SHA302 was examined for the first time. We introduced a modified culture medium that both significantly increased the production of lipopeptide biosurfactant and reduced the initial production costs. The biosurfactant production conditions using Bacillus sp. SHA302 were optimized with economic carbon sources of sugar beet molasses, potato peel, banana peel, orange peel, date extract, and other growth factors in three statistical analyses of a factorial experiment, Plackett–Burman design, and RSM.
Materials and Methods
Soil
The effect of the produced biosurfactant on heavy metal removal from the soil was examined using metal-contaminated soil (Hazrati et al., 2020), for which the physical and chemical characteristics were measured by standard methods (Supplementary Material 1).
Preparation of Inoculum
The Bacillus sp. SHA302 strain was obtained from the microbial collection of the University of Tehran, Iran, and prepared with the method of El-Sheshtawy and Doheim (2014). The strain was cultured at a 108 CFU/ml density in the Bushnell-Haas medium with N-hexane (1% V/V) as a carbon source. The microbial culture medium consisted of ammonium nitrate (1 g/l), potassium dihydrogen phosphate (1 g/l), potassium monohydrogen phosphate (1 g/l), magnesium sulfate (0.2 g/l), iron chloride (0.05 g/l), and calcium chloride (0.02 g/l). The carbon source of the medium was sterilized by a filter (0.22-μm pore size), and the pH of the medium was set to 7.0 using 1 N NaOH before sterilization. The biosurfactant screening tests were done in a prior study (unpublished data).
Extraction of Biosurfactant and Determination of Critical Micelle Concentration
The biosurfactant was extracted using the method of Sun et al. (2018). After 96 h of incubation, the bacterial suspension was centrifuged (8,000g) at 4°C for 20 min. Afterward, 6 N HCl was added to the supernatant to achieve a pH of 2, and the samples were stored at 4°C overnight. Afterward, samples were centrifuged at 8,000g for 20 min, and chloroform/methanol (2:1) was added to this solution and the resulting precipitate. Then, the entire process was repeated twice to enhance the extraction. In the end, the organic phase was separated, and the biosurfactant was weighed after evaporation of the solvent.
The critical micelle concentration (CMC) indicates the biosurfactant concentration at which surface tension (ST) reaches its lowest value and then remains constant. It was estimated by plotting the ST vs. biosurfactant concentration (Qiao and Shao, 2010). For this purpose, a stock solution of 1 g/l was prepared from the biosurfactant with sterile distilled water, and then different concentrations of biosurfactant were prepared from this stock solution. Afterward, ST in each concentration was measured in three replications.
Evaluation of the Biosurfactant Efficiency in the Heavy Metal Release From Soil
The biosurfactant efficiency for soil heavy metal release rate was evaluated with a slightly modified method of da Rocha Junior et al. (2019). Different concentrations of 2 × CMC (230 mg/l), CMC (115 mg/l), and 1.2 × CMC (57.5 mg/l) (25°C, solution volume 50 ml, shaking speed 50 rpm) were made from the biosurfactant, and the pH values of biosurfactant solutions were adjusted to 5.5 ± 0.1. The heavy metal release rate in the treatments was assessed in 1 day. Distilled water was used as a control. To measure the release rate, 5 g of dry soil was added to the soil with 50 ml of the biosurfactant solution (1:10) at different concentrations, and the samples were centrifuged at 5,000g for 10 min. The supernatant was discarded, and the soil was dried at room temperature. The heavy metal release rate from the dried soil was measured with an atomic absorption device (Shimadzu, Tokyo, Japan) according to the method of Mulligan et al. (1999). The biosurfactant adsorption rate to the soil was measured according to Chu and Chan (2003).
The Effects of Salinity, Temperature, and Different pHs on Bacterial Growth
In this study, a range of three salinity factors with different percentages of NaCl (0, 1, 2, 3, 4, and 5%; 30°C; and pH = 7), different temperatures (20, 25, 30, 35, 40, 45, 50, and 55°C and optimum NaCl concentration and pH), and pH values (5.5, 6, 6.5, 7, 7.5, 8, and 8.5 at 30°C) were applied in a nutrient broth medium. The bacterium was inoculated at a concentration of 4% (V/V) to the medium and incubated in a shaker incubator at 120 rpm for 24 h. Subsequently, optical densities (ODs) were read at 620 nm by a spectrophotometer (JENWAY 6705 UV/Vis), and the growth rate was measured in different conditions mentioned above.
The First Step of Optimization
Selection of Carbon and Nitrogen Sources With a Factorial Experimental Design
The carbon sources of the culture media were orange peel, potato peel, banana peel, date extract, and sugar beet molasses; the nitrogen sources were ammonium ferric citrate, ammonium sulfate, ammonium chloride, potassium nitrate, calcium nitrate, and sodium nitrate. The fruit peels were dried in an oven at 50°C for 4 days and then ground in a mortar to prepare the carbon sources. To prepare stock solutions of carbon, 10% (W/V) of each carbon source was added to distilled water and autoclaved, and the resulting extract was filtered afterward (Kulkarni et al., 2015). The SHA302 strain was cultured in 100 ml of Bushnell-Haas culture medium in 250-ml flasks with n-hexane along with each of these waste-based materials, including orange peel, potato peel, banana peel, date extract, and sugar beet molasses as carbon sources (1% V/V and 1% V/V, respectively) and mineral nitrogen sources (0.1% W/V) in a pH of 7.0 at 30°C by aeration at 150 rpm. The factorial experiment was carried out with 30 tests in three replicates to determine the best carbon and nitrogen sources in biosurfactant production. Surface tension as a response variable was evaluated in all treatments according to the method of Munguia and Smith (2001). All surface tension values were diluted 10-fold in all three statistical designs, including factorial experiment design, Plackett–Burman, and central composite design (CCD). Afterward, efficient carbon and nitrogen sources as well as other growth factors were designed for bacterial growth using the Plackett–Burman experiment to optimize the biosurfactant production.
The Second Step of Optimization
Design of Nutritional Factors and Culture Conditions With the Plackett–Burman Experiment
After choosing the best carbon and nitrogen sources for biosurfactant production from the first step, the next step of the experimental design was done with the Plackett–Burman method. Ten culture factors of carbon source; nitrogen source; inoculum percentage; salinity percentage; concentrations of iron chloride, magnesium sulfate, potassium monohydrogen phosphate, and potassium dihydrogen phosphate; temperature; and acidity were assessed for biosurfactant production using the Plackett–Burman method. This experiment was designed at two levels to examine n - 1 variables with n experiments. In this experiment, the lack of factor interactions was assumed as the null hypothesis (Kammoun et al., 2008). In this evaluation, 17 experiments were examined, consisting of 10 main factors, one dummy factor, and five central points; the central points were considered for better experimental error estimation (to make the lack of fit non-significant, five central points were considered), which are interpretable using linear equations demonstrated below.
In this equation, Y is the response variable (surface tension and quantity of biosurfactant produced), b0 is the y-intercept, and bi is the effect of the estimated variable. The effect of each variable is calculated using the difference between the average values calculated at the highest (+1) and lowest (−1) levels (Kammoun et al., 2008; Hippolyte et al., 2018). For each factor, a p-value of 0.05 was determined as the significant effect of the factor in biosurfactant production. The experiment was designed with the Minitab 16 software.
The Third Step of Optimization
Experimental Design With Response Surface Methodology
Effective factor levels and the interaction of different culture medium components affecting substantial biosurfactant production were analyzed and evaluated with the RSM and the CCD. In this study, the experimental design was composed of 30 experiments with independent variables each at five levels (α+, 1+, 0, 1−, and α−) with six chosen central points for the experiments. Alpha was calculated using the formula α = 2k/4. The number of all experimental components was estimated using the formula n = 2k - 1 + 2k + x0, where n is the number of all experiments, k is the number of factors being assessed, and x0 is the number of central points (Dong and Sartaj, 2016; Khoobbakht et al., 2016). Experiments were done in three replicates to find optimal biosurfactant production efficiency, and the obtained data averages were incorporated into a polynomial quadratic regression model.
In this equation, Y shows the estimated response variable (reduction of surface tension and quantity of produced biosurfactant); A0 shows the amount of response in the central point; and Ai, Aii, and Aij show the linear regression, second-order, and interaction terms, respectively. Xi and Xj show orthogonal-coded variables, n is the number of dependent variables, and ε shows random error (Montgomery, 2017; Hippolyte et al., 2018). Experiments were designed with the Design Expert Version 12 software.
Results and Discussion
The Effect of Lipopeptide Biosurfactant on the Release of Heavy Metals From the Soil
It is difficult to predict the efficiency of removing heavy metals from the soil by surfactants, and this is due to the complex and different conditions of the soil system. These factors include soil type, soil contamination intensity, age of contaminants, soil structure, soil solution pH, cation exchange capacity and soil pore size, and surfactant characteristics and concentration, and all are involved in the removal efficiency of surfactant from the soil (Singh and Cameotra, 2004; Xue et al., 2018; Pourfadakari et al., 2019). A critical capability of surfactants is their ability to form micelles (Silva et al., 2014). An increase in biosurfactant concentration lowers ST in the solution, which continues to decrease until the biosurfactant reaches a concentration termed CMC in which micelles are formed (Marchant and Banat, 2012). The results of this study show that from a biosurfactant concentration of 115 mg/l, the ST value was stabilized (36.5 ± 0.6 mN/m) and continued until the end (Figure 1). Many researchers have suggested different CMC values for lipopeptide biosurfactants; for instance, the surface tension of 34 mN/m, CMC of 650 mg/l, and strain Agrobacterium fabrum SLAJ731 (Sharma et al., 2019); surface tension of 28–30 mN/m, CMC of about 100 mg/l, and strain B. megaterium pL 6 (Pueyo et al., 2009); and surface tension of 32 mN/m, CMC of 350 mg/l, and strain Bacillus subtilis ZNI5 (Mnif et al., 2022). The biosurfactant concentration effect on pollutant removal showed that zinc and lead release percentages from soil increased by increasing the biosurfactant concentration. The highest lead and zinc release rates (53.8% ± 1.4 and 39.3% ± 1.7, respectively) were measured in the CMC and acid treatment (Figure 2). With an increase in the biosurfactant concentration, heavy metals in adsorption sites form complexes with the biosurfactant and are further released into the soil solution. Anionic biosurfactants form non-ionic complexes with metallic cations in the soil with higher stability than metallic complexes with soil particles (Franzetti et al., 2014). da Rocha Junior et al. (2019) reported an increase in the removal rate of three metals (lead, zinc, and copper) with increasing the surfactant concentration. The results of Elouzi et al. (2012) in assessing the efficiency of rhamnolipid biosurfactant for the removal of lead and zinc showed an increase in metal removal with increasing the rhamnolipid concentration.
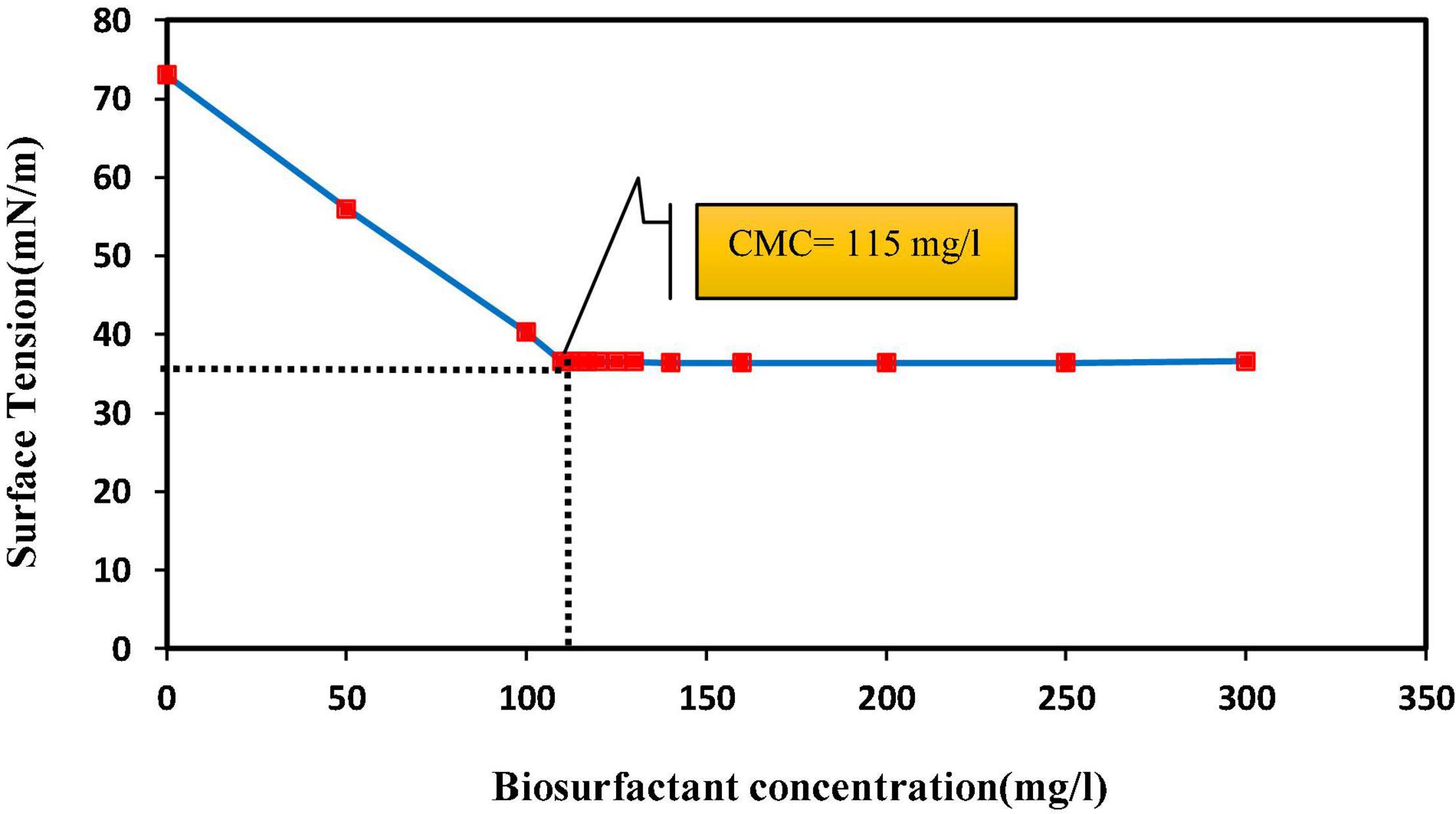
Figure 1. Critical micelle concentration (CMC) of biosurfactant produced by Bacillus sp. SHA302 (in Bushnell-Haas culture medium with carbon sources of glucose and n-hexane for 96 h).
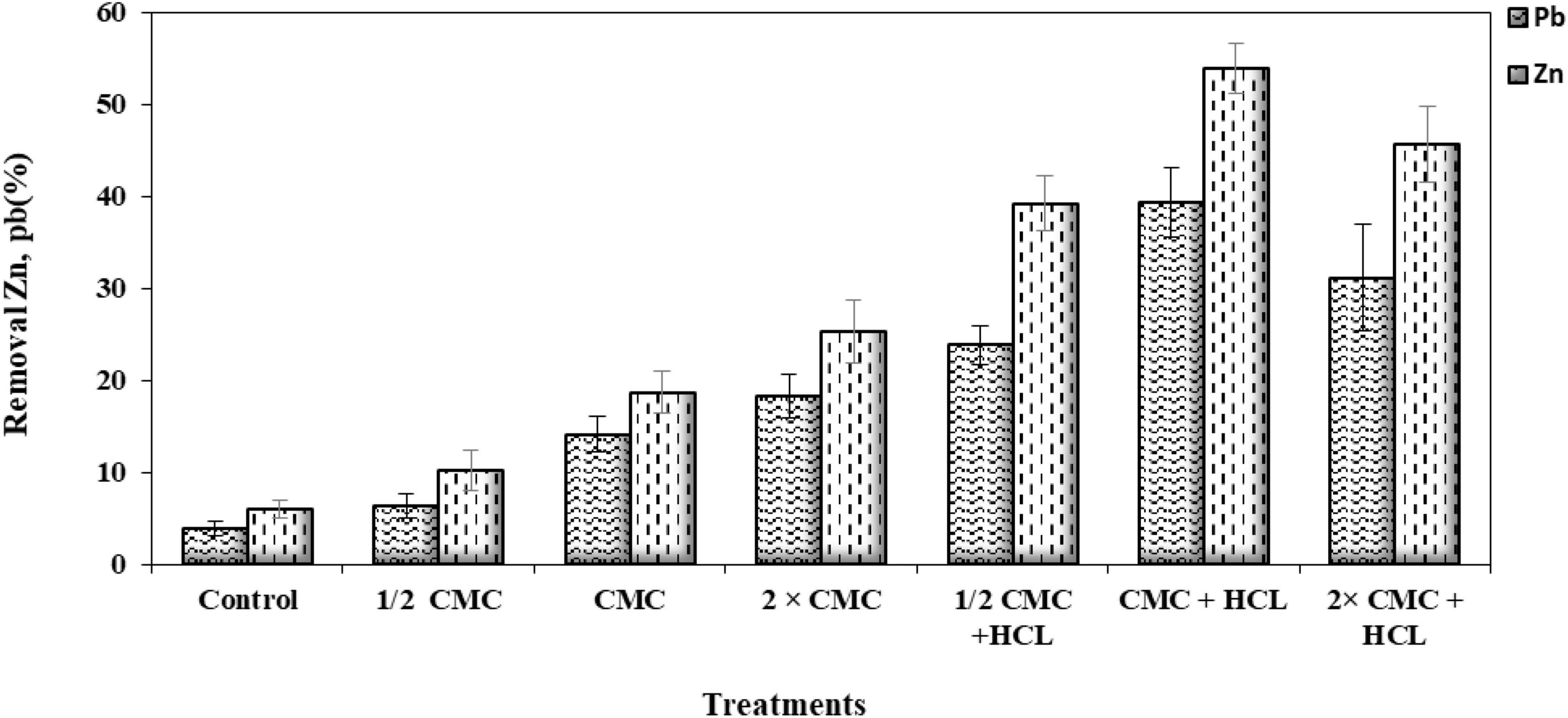
Figure 2. Release of lead (Pb) and zinc (Zn) from contaminated soil at different biosurfactant concentrations in 24 h (HCl, hydrochloric acid; CMC, critical micelle concentration).
Additionally, the results of this study agree with those of Mulligan et al. (1999) in zinc and copper removal from sediments using a lipopeptide biosurfactant. Doong et al. (1998) presented evidence of an increase in metal removal by increasing the surfactant concentration up to CMC, beyond which a constant trend was seen in the removal of metals. The effective surfactant concentration for the removal of heavy metal pollutants depends on surfactant type and soil conditions (da Rocha Junior et al., 2019). The results of this study on metal removal efficiency by the addition of acid showed an increase in the release rates of both metals up to the CMC concentration and a lowered release rate at the 2 × CMC concentration. This shows that the added acid causes the H+ cation to attract to exchange sites and substitute the surface-adsorbed metals, followed by the entry of metals from the adsorption site into the soil solution and their placement within the biosurfactant micelle. At the 2 × CMC concentration, the increased biosurfactant concentrations lead to the competition of the biosurfactant molecules with soil particles for the adsorption of metals and H+ cations. Since the hydrated shell of metals is larger than H+, they are attached to the exchange sites with less energy than H+.
On the other hand, the biosurfactant tends to form a more stable complex, and an increase in its concentration in the soil solution forms a stable surfactant–H complex (McBride, 1994; Bohn et al., 2001). Heavy metals are prone to precipitation at high pH values, which results in lowered pollutant removal and increases metal solubility at a low pH (Yong and Phadungchewit, 1993; Sun et al., 2021). Wu et al. (2015) found that an increase in pH (increased pH-dependent negative charges) reduced the adsorption of a rhamnolipid biosurfactant to the soil, which causes more reactions of the biosurfactant with metals and makes them effective. The lowered metal release rate in the 2 × CMC concentration with acid treatment can be attributed to increased pH-dependent charges with reduced pH and the biosurfactant adsorption to soil with an increase in its concentration, thereby reducing its efficiency. Sarubbo et al. (2018) showed that the biosurfactant produced from Candida guilliermondii at a concentration of 0.42% could remove more than 98% of the initial amount of lead and zinc ions.
Grzywaczyk et al. (2021) displayed that soil washing with saponin solution removes 86% zinc and 70% copper. Their results in three different soil types displayed more removal of zinc against copper, and this biosurfactant was considered an effective and environmentally friendly remediation agent. In an investigation on lipopeptide biosurfactant, CMC plus acid treatment had the highest removal of lead (98.7%) and 2 × CMC plus acid treatment had the highest removal of zinc (59.1%) from sandy soil with artificial contamination (Jimoh and Lin, 2020). An important issue concerning surfactant efficiency is their adsorption to the soil. The results of this study show a 23.3% ± 1.2 adsorption of this anionic biosurfactant to the soil (Figure 3). Biosurfactant adsorption to the soil reduces its efficiency in eliminating organic and mineral pollutants (Lee et al., 2000). Biosurfactant adsorption to soil also depends on surfactant characteristics, ionic charge, and soil properties (Calvo et al., 2009).
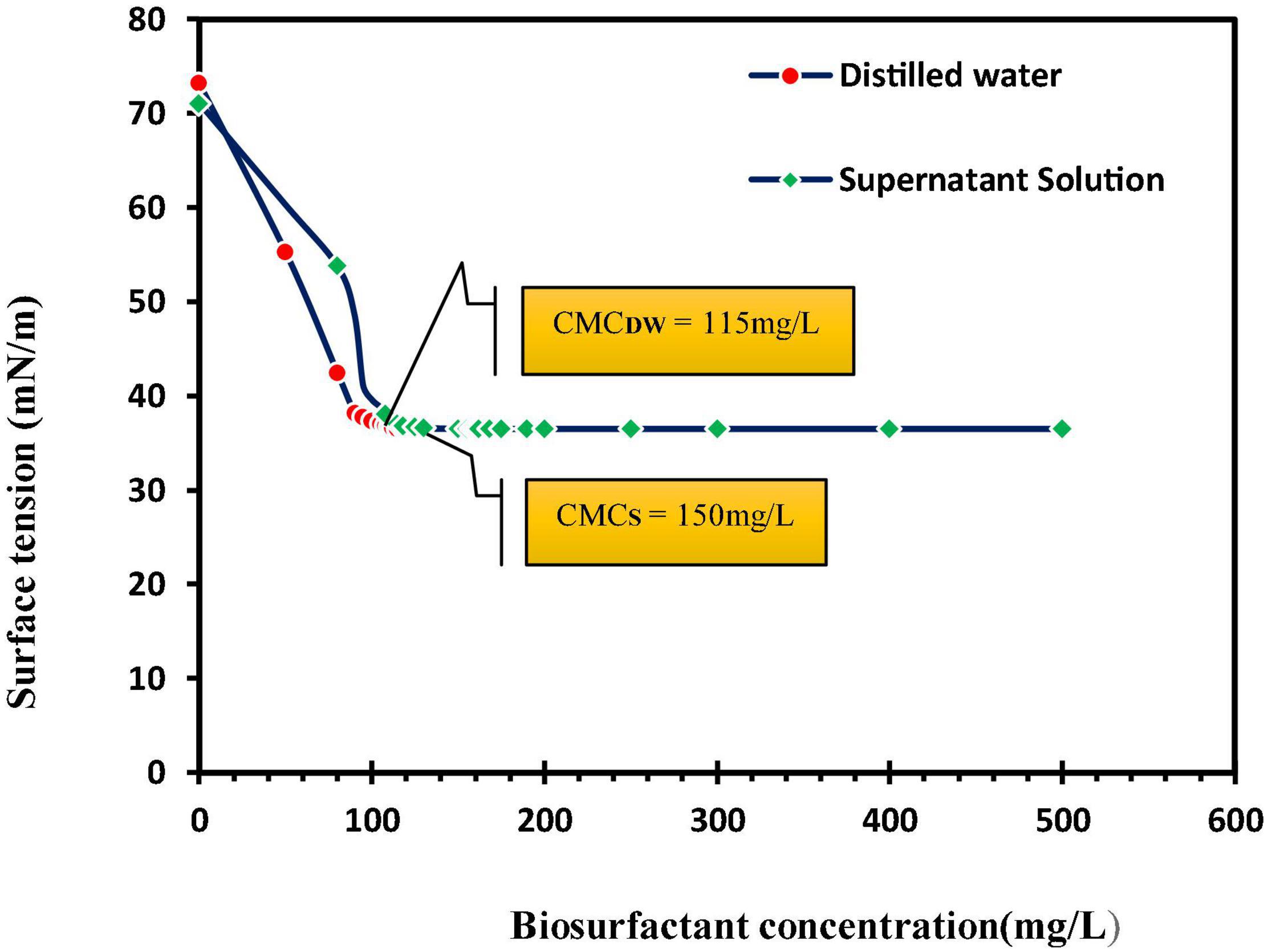
Figure 3. Adsorption of biosurfactant to contaminated soil at different biosurfactant concentrations, CMCDW, critical micelle concentration measured in distilled water; CMCS, the measured critical micelle concentration of supernatant solutions [supernatant solutions obtained by centrifugation of a mixture of biosurfactants (at different concentrations) and soil].
Choosing Carbon and Nitrogen Sources
The carbon and mineral nitrogen treatments were assessed at a pH of 7.0, 30°C, and an aeration speed of 150 rpm. The results demonstrated that the highest reduction in surface tension (32.2 ± 0.76 mN/m) was obtained in the presence of sugar beet molasses as a carbon source and ammonium chloride as a nitrogen source, which were significantly different from the other nitrogen and carbon sources. The smallest reduction (52.4 ± 0.7 mN/m) in ST was seen with orange peel as a carbon source and the ammonium ferric citrate salt. The results showed that ammonium chloride as a nitrogen source significantly lowered ST alongside three carbon sources of molasses, potato peel, and date syrup (Figure 4). The results of the previous study (data not shown) displayed that the lipopeptide biosurfactant produced in the Bushnell-Haas culture medium with n-hexane and glucose carbon sources had ST and produced biosurfactant values of 36.5 ± 0.23 mN/m and 0.92 ± 0.05 g/l, respectively. Presently, sugar beet molasses is used as a carbon source for biosurfactant production due to its low cost, mineral content, organic compounds, vitamins, and high total sugar content compared to other commonplace carbon sources, such as sucrose and glucose, and is very important for fermentation. Molasses has been reported as an economical carbon source in surfactant production by Bacillus spp. (Saimmai et al., 2011). Abdel-Mawgoud et al. (2008) reported a low-cost surfactin production by B. subtilis BS5 using an optimized culture medium containing 16% molasses and 5 g/l of NaNO3, with a significant increase of 1.12 g/l in surfactin yield. Rane et al. (2017) assessed B. subtilis ANR-mediated surfactant production utilizing different carbon sources of glucose, molasses, orange peel, potato peel, banana peel, and bagasse extract. They concluded that 4% molasses carbon source led to the highest biosurfactant production of 0.19 g/l. Joshi et al. (2008) assessed Bacillus licheniformis K51, B. subtilis 20B, B. subtilis R1, and Bacillus sp. HS3 for surfactant production. They observed that sugar beet molasses as a carbon source could result in surface tensions of 29.67, 29.33, 30.33, and 30.67 mN/m, respectively. In another study on optimizing the production of lipopeptide biosurfactants produced by B. subtilis strain Al-Dhabi-130 with inexpensive sources, molasses was identified as the best source for the production of this biosurfactant (Al-Dhabi et al., 2020).
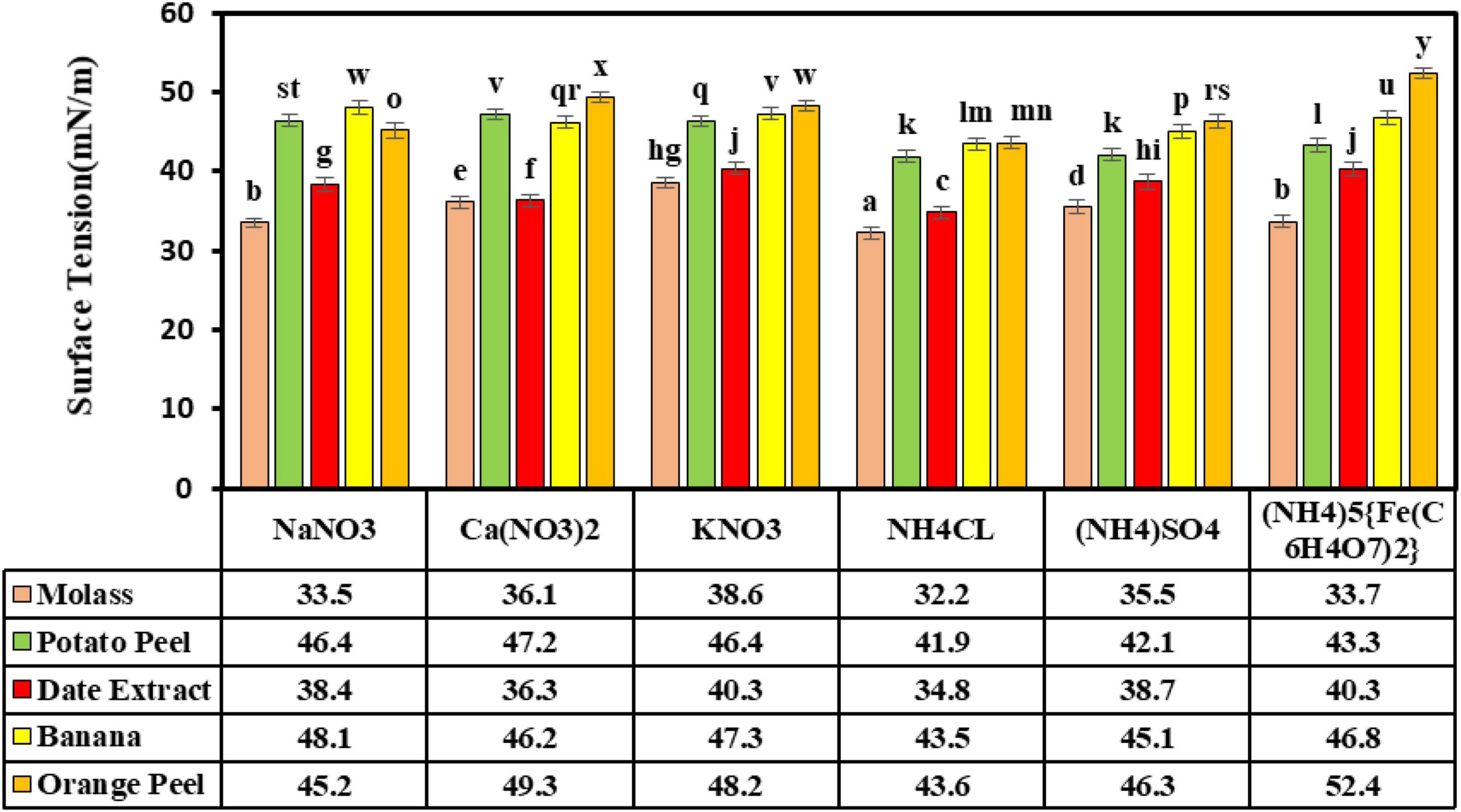
Figure 4. The effects of carbon and nitrogen sources in the production of biosurfactant. Means with the same letters are not significantly different at P ≤ 0.05. Error bars indicate the standard deviation (n = 3). Duncan test was used for comparisons of means.
Designing Nutritional Factors and Culture Conditions With the Plackett–Burman Design
The screened factors were respectively molasses percentage (X1), ammonium chloride percentage (X2), inoculum percentage (X3), salinity percentage (X4), iron chloride concentration (X5), magnesium sulfate (X6), potassium monohydrogen phosphate concentration (X7), potassium dihydrogen phosphate concentration (X8), temperature (X9), and pH (X10), which are illustrated along with the three repetition average of ST and the produced biosurfactant in Table 1. Salinity range, temperature, and pH in the Plackett–Burman experiment design were determined using average bacterial growth in different ranges of salinity, temperature, and pH (Supplementary Material 2). The results showed that only four out of the 11 factors, i.e., ammonium chloride concentration, molasses percentage, pH, and magnesium sulfate concentrations, were chosen for further evaluation based on their significance levels (P-value < 5%) (Table 2). The significance of these factors means that changes in their values led to the highest effect on changes in ST and biosurfactant production. According to Table 2, the statistical analyses of the models show the significance of the two evaluated statistical models with the regression coefficient percentage (R2) and adjusted regression coefficient (R2adj) > 80%. Rane et al. (2017) and Hippolyte et al. (2018) considered a regression coefficient of >80% as a threshold for assessing the statistical sufficiency of models. The model coefficients can be calculated using the multivariate regression analysis of responses, ST (y1), and the extracted biosurfactant (y2). Negative coefficients in the y1 model show the positive effect of these factors in biosurfactant production and better efficiency in lowering ST. Additionally, the effect coefficients will be positive if changes in factor levels cause an increase in ST. The results of Eq. 2 demonstrated that factors with high effect coefficients showed bigger effects on ST changes in the culture medium, with the highest effects of ST changes caused by pH (P > 0.000, F = 646.84), ammonium chloride (P > 0.000, F = 139.28), magnesium sulfate (P > 0.001, F = 48.34), and molasses percentage (P > 0.008, F = 18.49) (except for pH, the other factors had negative effect coefficients). In Eq. 3, the effect coefficients for factors were similar to the y1 model (except for pH, the other factors had positive effect coefficients). In both the estimated response variables, pH had the greatest effect coefficient. The four factors of pH, ammonium chloride, magnesium sulfate, and molasses percentage were chosen for the RSM to better evaluate and analyze their individual levels. Rane et al. (2017) studied optimum biosurfactant production by B. subtilis strain ANR with a Plackett–Burman experimental design. They showed that temperature and molasses were the most significant parameters among factors affecting the production. On the other hand, Almeida et al. (2017) reported that the inoculum percentage and molasses as a carbon source were the most effective factors in surfactant production. In another study on the optimization of lipopeptide biosurfactant produced by Paenibacillus D9 (optimal temperature of 30°C, 1.5 ml inoculation, 3% diesel fuel, 4 mM magnesium sulfate, 1% ammonium sulfate, and pH = 7.00), production was reported at 4.11 g/l with a surface tension of 32.2 mN/m (Jimoh and Lin, 2020).
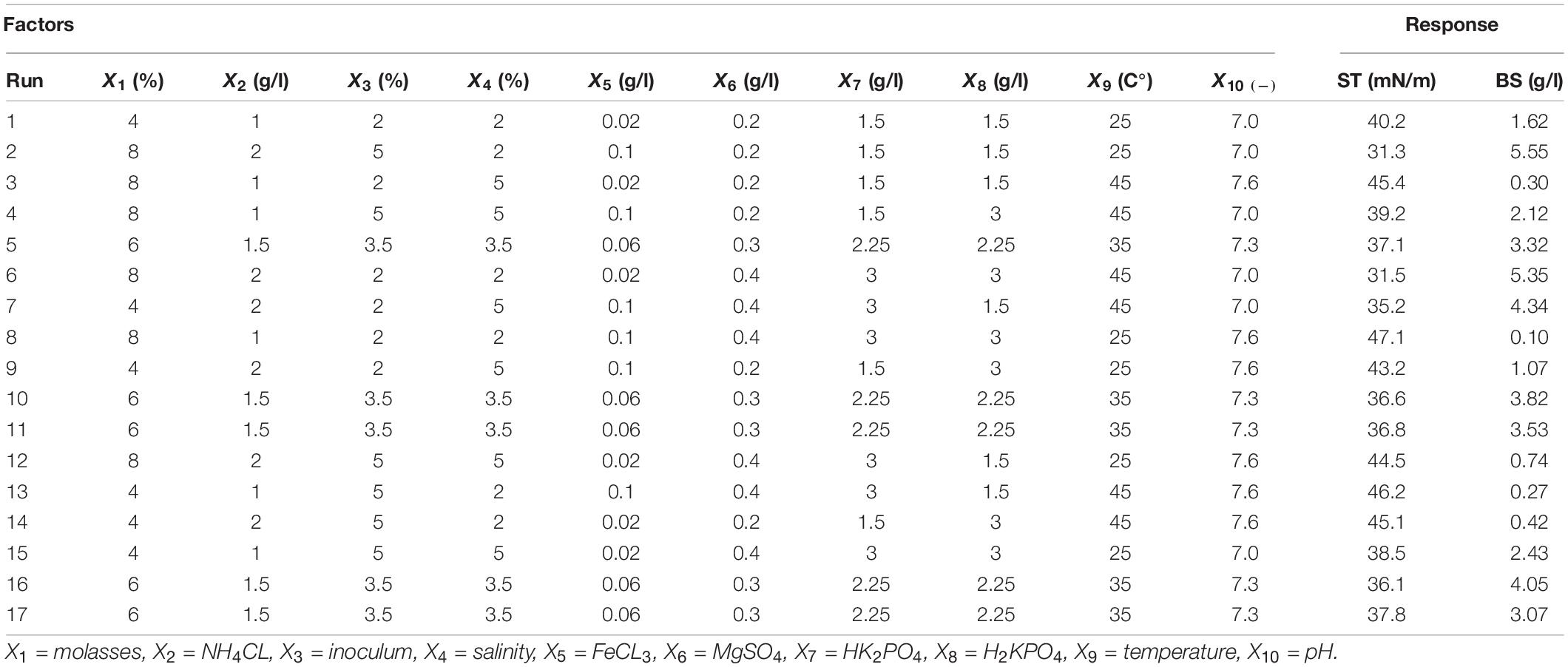
Table 1. The Plackett–Burman experimental design matrix along with results from surface tension (ST) and produced biosurfactant (BS) as the response variables.
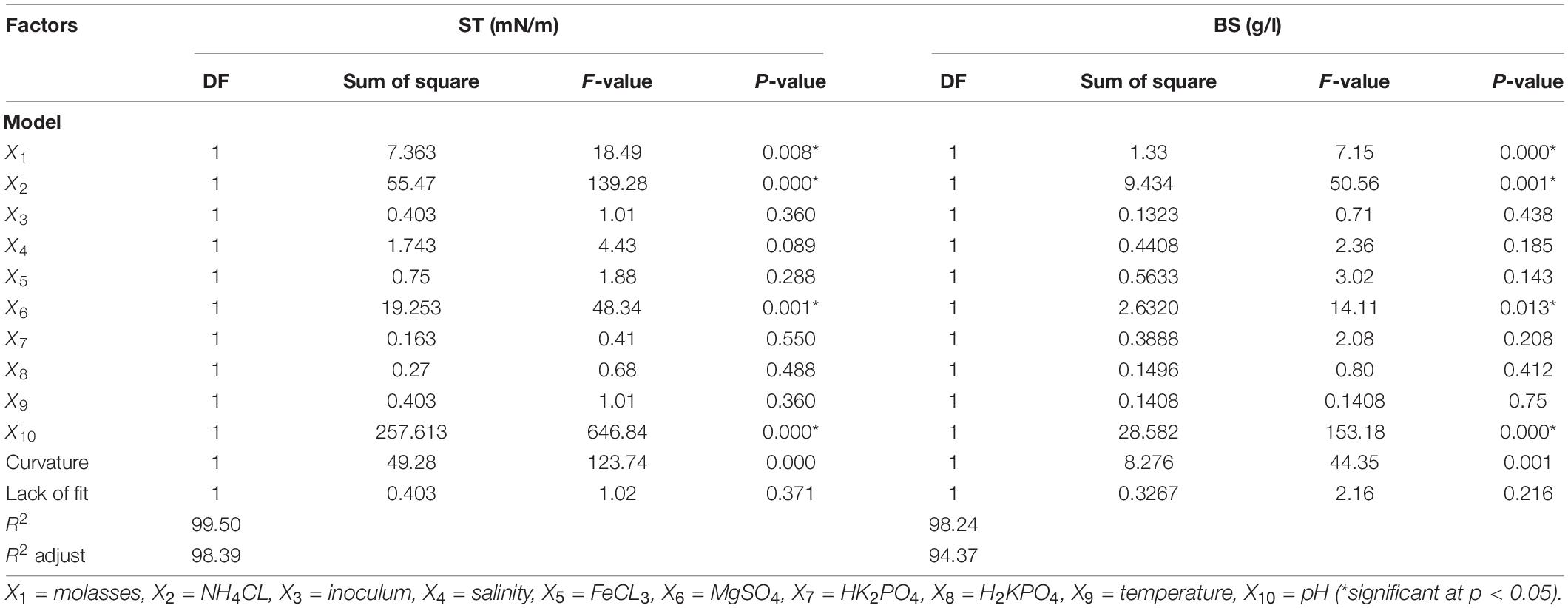
Table 2. Analysis of variance (ANOVA) for surface tension (ST) and produced biosurfactant (BS) as the response variables based on Plackett–Burman experiment.
Experiment Design With the Response Surface Methodology
At this stage, a CCD with six replications in the central point was used to determine the effect of each factor and their interactions on biosurfactant production. The ammonium chloride (X1), magnesium sulfate (X2), molasses (X3), and pH (X4) factors were assessed as orthogonal variables along with two response variables of ST (y1) and the produced biosurfactant (y2). Each parameter in this design was assessed at five levels (−α, +1, 0, −1, and +α), and zero was considered as the central coded value. Optimum amounts in CCD were acquired by solving regression equations and the analysis of results from two- and three-dimensional contour plots. The CCD experimental design matrix is shown in Table 3, and the regression equation analysis of each response variable, along with model coefficients and relevant equations, is demonstrated below.
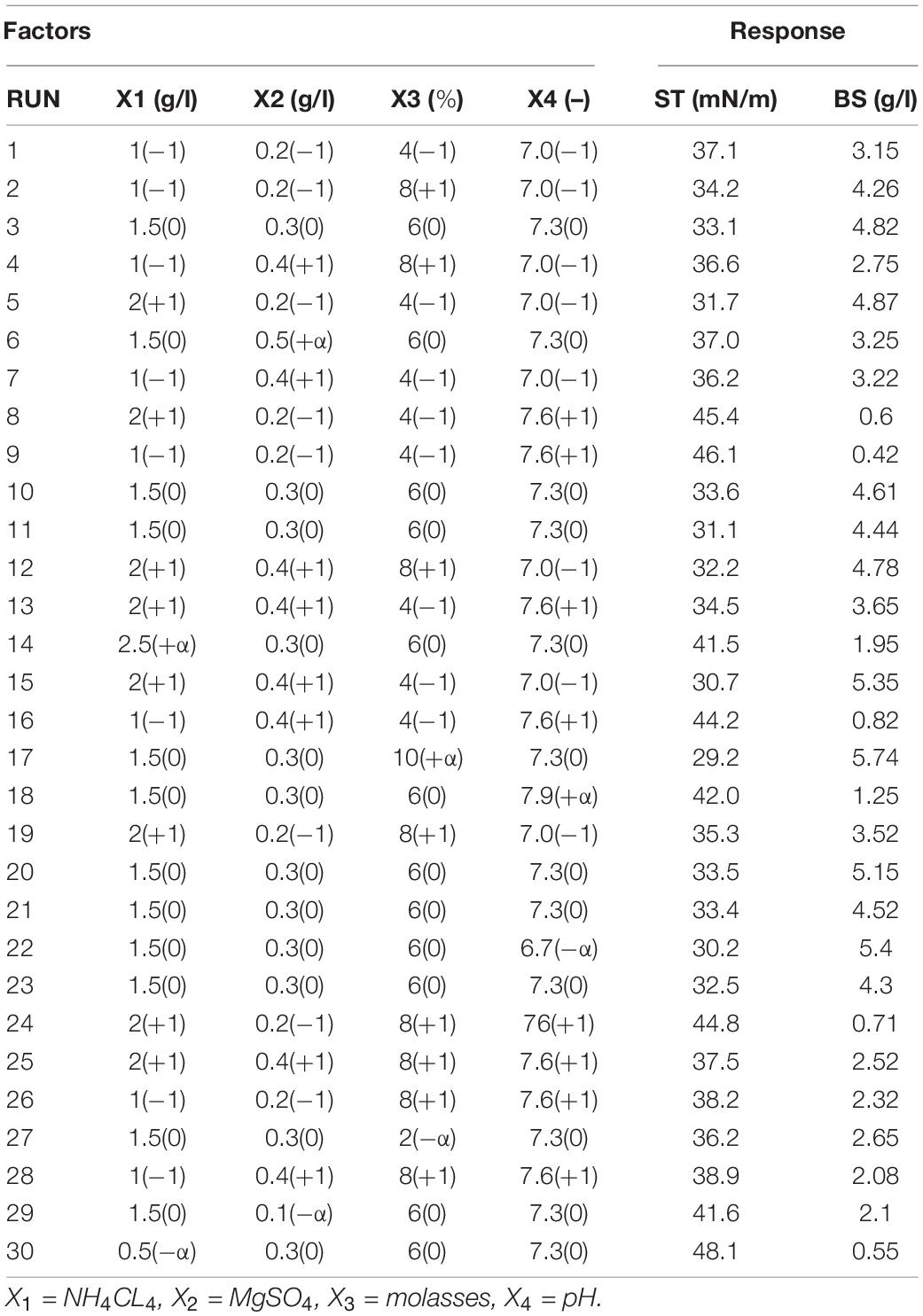
Table 3. Central composite design (CCD) experiment matrix for two response variables of surface tension (ST) and produced biosurfactant (BS).
The results of statistical competence evaluation of models Y1 and Y2 showed R2 and R2-adj values >90%, suggesting that about 90% of independent variable responses can be explained by these models (Table 4). These results corroborate those of Sayyad et al. (2007), who reported that an R2 of a model closer to 100% better explains the variation between predicted amounts by the model and empirical amounts. According to Table 3, the highest biosurfactant production and the lowest ST are seen in 10% molasses, 1.5 g/l of nitrogen, 0.3 g/l of magnesium sulfate, and a pH of 7.3. In both y1 and y2 models, the pH with the highest effect coefficient was most effective in both models (Eqs 4, 5). Additionally, Supplementary Material 3 shows the effect of predicted values vs. real model values. In this context, a proximal distribution of observed values to the line is indicative of the proximity of actual values to predicted ones so that R2 values of 95.08% and 94.26% were calculated for Y1 and Y2 models, respectively.
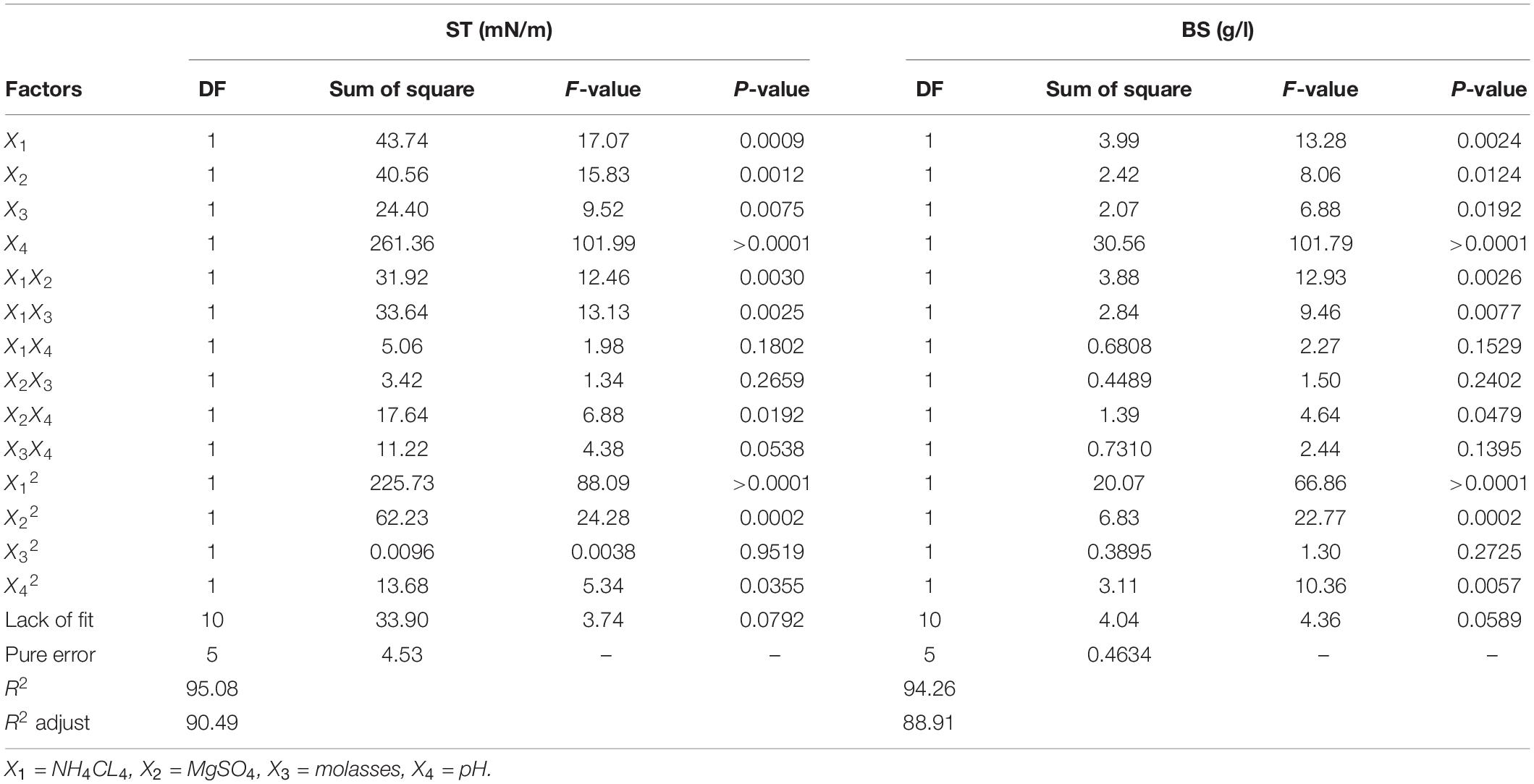
Table 4. Analysis of variance (ANOVA) for the two response variables of surface tension (ST) and produced biosurfactant (BS) based on the central composite design.
Assessment of Variable Interactions in the Response Surface Methodology
The results clearly demonstrated that magnesium sulfate and ammonium chloride concentrations, molasses percentage, and pH significantly affected the reductions of ST and the produced level of the biosurfactant. The interactions of these variables with response values were assessed using three-dimensional plots against both response variables. The results showed that ST initially decreased and then increased at a low molasses concentration (2%) with an increase in ammonium chloride level. The same trend was also seen at higher molasses concentrations, but the intensity of ST changes was higher at low quantities of molasses than at higher levels (Figure 5A). The lowest ST amount (1.5 g/l) was seen in 10% molasses and the central point of ammonium chloride, and ST increased by increasing the distance from the central point of ammonium chloride. According to Figure 5B, an increase in molasses percentage increased the produced biosurfactant level, and ammonium chloride concentration in the central point had the highest effect on increasing biosurfactant production. Therefore, the medial quantity of ammonium chloride (1.5 g/l) and the highest molasses percentage (10%) had the most significant effect on biosurfactant production.
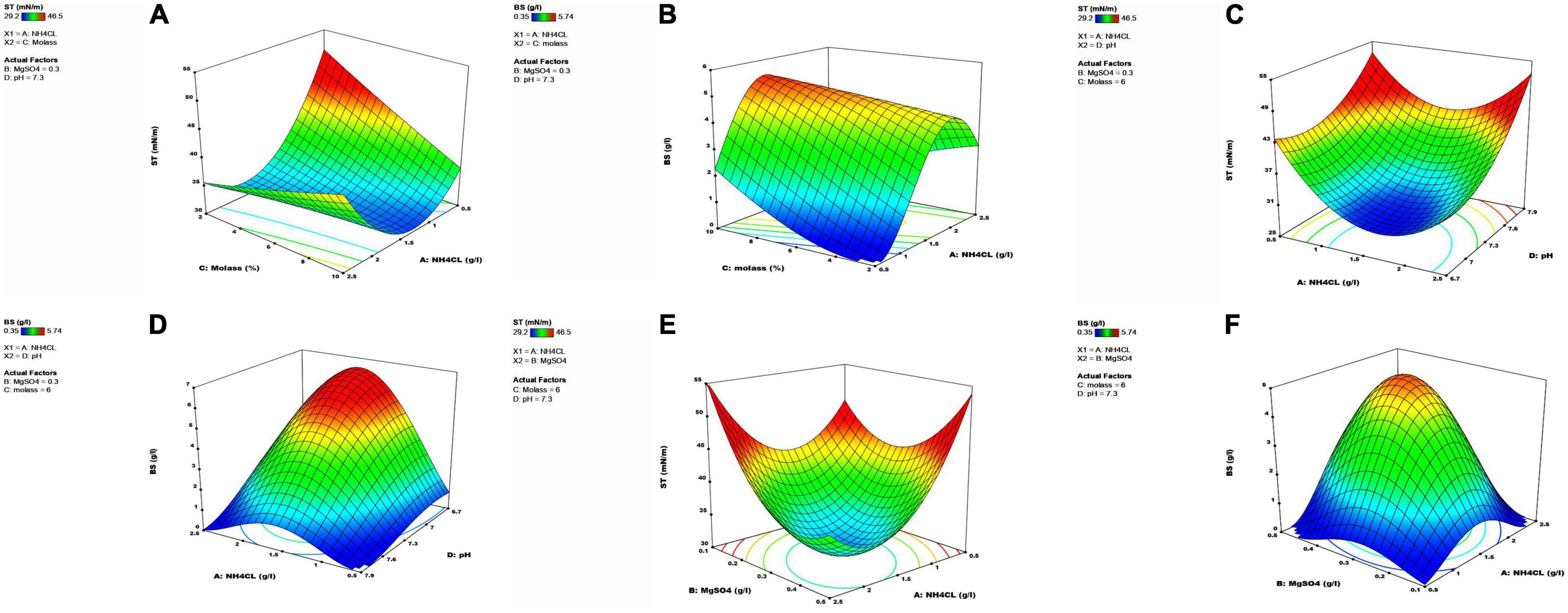
Figure 5. Three-dimensional graphs showing the interactive effects of two independent factors on response variables, while in each graph, the third and fourth variables are set at its center point. (A) ST as a function of NH4CL and molasses, (B) BS as a function of NH4CL and molasses, (C) ST as a function of pH and NH4CL, (D) BS as a function of pH and NH4CL, (E) ST as a function of MgSO4 and NH4CL, (F) BS as a function of MgSO4 and NH4CL.
A nitrogen source is one of the critical factors in biosurfactant production (Burkovski, 2003). Nitrogen is a nutrient that plays an essential role in cellular metabolism. A decrease in nitrogen content in the culture medium interrupts protein production and shifts cellular metabolism to carbohydrate production, thereby increasing biosurfactant production. The presence of high amounts of nitrogen sources in the culture medium lowers biosurfactant production and directs metabolism toward cellular growth (Abalos et al., 2002). This study observed a reduction in biosurfactant production by increasing ammonium chloride concentrations above the central point concentration. High concentrations of salt and minerals in molasses lower biosurfactant production and activity due to an increase in osmotic pressure; therefore, it reduces cell viability and ceases biosurfactant production (Doelle and Doelle, 1990). Wei et al. (2005) showed that biosurfactant production increased initially with an increase in the molasses concentration until it reached a maximum, and the production became stable or decreased afterward. During the optimization of the Candida tropicalis strain, an increase in molasses percentage resulted in a significantly reduced surface tension (Almeida et al., 2017). Similarly, our results showed that an increase in molasses percentage increased the biosurfactant production level, which can be due to the halophilic property of the bacterium, for which salinity may not be a growth-limiting factor at a high molasses concentration (refer to Supplementary Material 2a).
At low ammonium chloride quantities, ST rose with increasing pH, and this increase was more pronounced at lower ammonium chloride concentrations (Figure 5C). In low pH values, an increase in ammonium chloride first led to an increase in biosurfactant production, but it decreased at concentrations higher than the central point of ammonium chloride. The highest biosurfactant production occurred at the central point concentration of ammonium chloride, and changing intensity was higher at low than at high pHs (Figure 5D). One of the important characteristics of most organisms is their strong dependency on pH conditions for growth and secondary metabolite production (Najafi et al., 2011). The SHA302 strain could grow in a pH range of 5.0–9.0, with the highest performance for biosurfactant production in pH 7.3. Since microorganisms grow well at a pH of 7, biosurfactant production is less affected at lower pHs than at higher pHs, which significantly reduces the production. The results of this study showed that the SHA302 strain had the highest growth at a pH of 7.5 (refer to Supplementary Material 2c), and biosurfactant production decreased with an increase in pH. As demonstrated in Figure 5E, ST initially decreased at low ammonium chloride quantities and then increased by increasing magnesium sulfate levels. ST showed a decrease at high ammonium chloride concentrations. As shown in Figure 5F, different ammonium chloride quantities and an increase in magnesium sulfate resulted in an initial increase in the biosurfactant production and then began to decrease with the highest production at the central point concentration (NHCl = 1.5 g/l, MgSO4 = 0.3 g/l) for both factors.
Finding Optimum Conditions for Biosurfactant Production
The optimum conditions for both models (surface tension/biosurfactant production) are shown in Figure 6. Independent input variables can be adjusted to their maximum, minimum, or any target point, while the response variable is usually set on minimum or maximum values. The software adjusted these conditions by placing molasses at the highest concentration (10%) and the three factors of ammonium chloride, pH, and magnesium sulfate on predetermined levels of 1–2 g/l, 7–7.6, and 0.2–0.4 g/l, respectively. The results showed that by placing molasses at a maximum and the other factors in the determined range (Figure 3), the surface tension and the amount of extracted biosurfactant were 30.08 mN/m and 5.89 g/l, respectively. To compare the accuracy of the estimated response values by the software with the actual values, ST and the produced biosurfactant (BS) were measured at the amounts suggested by the software. The obtained amounts were 30.48 mN/m and 5.76 g/l, respectively, indicating the high precision of the production optimization model with the values obtained from the experiment.
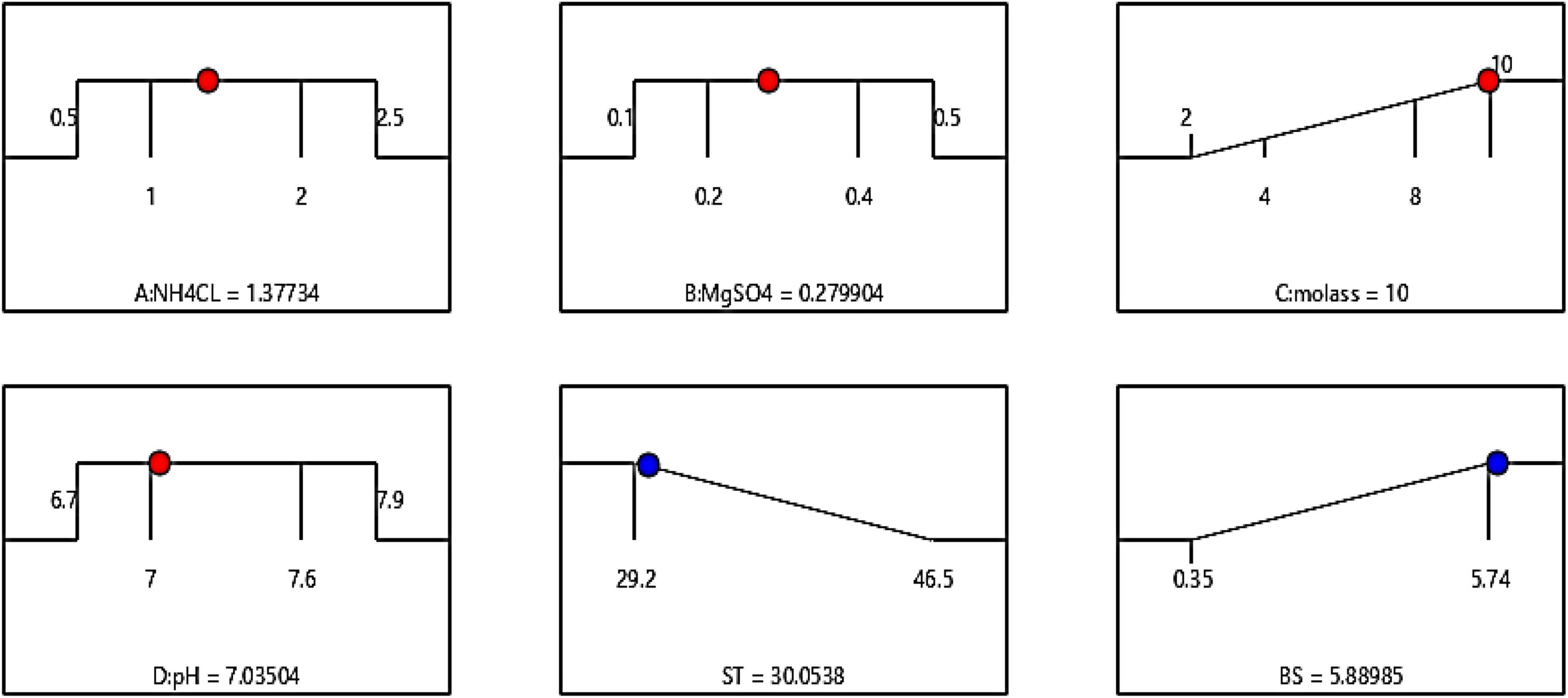
Figure 6. Optimal conditions predicted by the software, by adjusting the molasses factor levels to the maximum value (10%).
Economic Challenges and New Production Approaches
The global market for biosurfactants is growing by 5.6% from 2017 to 2022 and may reach 5.52 billion dollars (Gaur and Manickam, 2021). Considering the increasing interest in the use of biosurfactants in recent decades, the market for the production of these compounds is fundamentally missing because of the lack of access to cheap raw materials and economic issues related to production (Helmy et al., 2011). The cost of producing biosurfactants commonly relies upon the volume of the bioreactor, the cost of the raw materials, and the cost of separating them. In the production process of biosurfactants, the rate of biosurfactant foaming, the volume of the bioreactor, and the biosurfactant’s viscosity can have harmful effects on its production (Dolman et al., 2019). Promising strategies for increasing the production of biosurfactants in the last decade have been used to overcome the economic challenges of its production, some of which are as follows: the use of growth stimulants (Roelants et al., 2016), application of nanoparticles (Kiran et al., 2014), production of biosurfactants simultaneously with other biological compounds (Kavuthodi et al., 2015), the use of immobilized microorganisms (Heyd et al., 2011) and, so on. These strategies can be cost-effective in reducing the cost of producing biosurfactants. Therefore, taking these strategies more seriously is an essential step towards increasing productivity, making the production process more economical, as well as resolving concerns about solid waste disposals by converting them efficiently into low-cost industrial solids. Such solutions make agricultural waste valuable and profitable products (Singh et al., 2019).
Conclusion
This research aimed at using biosurfactants in the release of heavy metals and finding optimum conditions for biosurfactant production with the Bacillus sp. SHA302 strain using low-cost feedstock. The Bacillus sp. strain SHA302, with 93.98% phylogenetic similarity to registered strains in GenBank, is the first report in biosurfactant production, and a strain with this low percentage of similarity is most likely novel. In this study, a new culture medium was formulated to produce lipopeptide biosurfactant, resulting in increased productivity, reduced costs, and commercialization in agricultural industries. The cost reduction results displayed that to produce 1 L of formulated medium and 1 g of low-purity biosurfactant, 0.13-dollar and 2.1-dollar costs are required, which are pretty economical quantities to produce. Fortunately, with a meager purification rate, this biosurfactant showed outstanding efficiency in agriculture, and this issue played an essential role in reducing production costs. Our previous study reported the high efficiency of this biosurfactant in releasing oil pollutants from soil, and this study demonstrated its ability in heavy metal removal from the soil. Therefore, the optimization of this biosurfactant can be an effective solution for treating saline-sodic soils with long-term contamination (both petroleum hydrocarbons and heavy metals).
Data Availability Statement
The original contributions presented in the study are included in the article/Supplementary Material, further inquiries can be directed to the corresponding author.
Author Contributions
SK: conception and design of the study, analysis and/or interpretation of data, writing and drafting the manuscript, and revising the manuscript critically for the final version. HG: writing and drafting the manuscript and revising the manuscript critically for the final version. AP: conception and design of the study, analysis and/or interpretation of data, and revising the manuscript critically for the final version. HA: interpretation of soil analysis. All authors contributed to the article and approved the submitted version.
Conflict of Interest
The authors declare that the research was conducted in the absence of any commercial or financial relationships that could be construed as a potential conflict of interest.
Publisher’s Note
All claims expressed in this article are solely those of the authors and do not necessarily represent those of their affiliated organizations, or those of the publisher, the editors and the reviewers. Any product that may be evaluated in this article, or claim that may be made by its manufacturer, is not guaranteed or endorsed by the publisher.
Acknowledgments
We would like to thank the University College of Agriculture and Natural Resources, University of Tehran, for its financial support to conduct this research.
Supplementary Material
The Supplementary Material for this article can be found online at: https://www.frontiersin.org/articles/10.3389/fmicb.2022.785985/full#supplementary-material
References
Abalos, A., Maximo, F., Manresa, M. A., and Bastida, J. (2002). Utilization of response surface methodology to optimize the culture media for the production of rhamnolipids by Pseudomonas aeruginosa AT10. J. Chem. Technol. Biotechnol. Int. Res. Process Environ. Clean Technol. 77, 777–784.
Abdel-Mawgoud, A. M., Aboulwafa, M. M., and Hassouna, N. A.-H. (2008). Characterization of surfactin produced by Bacillus subtilis isolate BS5. Appl. Biochem. Biotechnol. 150, 289–303. doi: 10.1007/s12010-008-8153-z
Al-Dhabi, N. A., Esmail, G. A., and Valan Arasu, M. (2020). Enhanced Production of biosurfactant from Bacillus subtilis strain Al-Dhabi-130 under solid-state fermentation using date molasses from Saudi Arabia for bioremediation of crude-oil-contaminated soils. Int. J. Environ. Rese. Public Health 17:8446. doi: 10.3390/ijerph17228446
Almeida, D. G., Soares Da Silva, R. D. C. F., Luna, J. M., Rufino, R. D., Santos, V. A., and Sarubbo, L. A. (2017). Response surface methodology for optimizing the production of biosurfactant by Candida tropicalis on industrial waste substrates. Front. Microbiol. 8:157. doi: 10.3389/fmicb.2017.00157
Bohn, H., Mcneal, B., and O’Connor, G. (2001). Soil Chemistry. New York, NY: John Willey & Sons. Inc, 155–171.
Burkovski, A. (2003). Ammonium assimilation and nitrogen control in Corynebacterium glutamicum and its relatives: an example for new regulatory mechanisms in actinomycetes. FEMS Microbiol. Rev. 27, 617–628. doi: 10.1016/S0168-6445(03)00067-6
Calvo, C., Manzanera, M., Silva-Castro, G., Uad, I., and González-López, J. (2009). Application of bioemulsifiers in soil oil bioremediation processes. Future prospects. Sci. Total Environ. 407, 3634–3640. doi: 10.1016/j.scitotenv.2008.07.008
Chakraborty, J., and Das, S. (2014). “Biosurfactant-based bioremediation of toxic metals,” in Microbial Biodegradation and Bioremediation, ed. S. Das (Amsterdam: Elsevier). doi: 10.1016/j.jhazmat.2021.126253
Chu, W., and Chan, K. (2003). The mechanism of the surfactant-aided soil washing system for hydrophobic and partial hydrophobic organics. Sci. Total Environ. 307, 83–92. doi: 10.1016/S0048-9697(02)00461-8
da Rocha Junior, R. B., Meira, H. M., Almeida, D. G., Rufino, R. D., Luna, J. M., Santos, V. A., et al. (2019). Application of a low-cost biosurfactant in heavy metal remediation processes. Biodegradation 30, 215–233. doi: 10.1007/s10532-018-9833-1
Das, K., and Mukherjee, A. K. (2007). Crude petroleum-oil biodegradation efficiency of Bacillus subtilis and Pseudomonas aeruginosa strains isolated from a petroleum-oil contaminated soil from North-East India. Bioresour. Technol. 98, 1339–1345. doi: 10.1016/j.biortech.2006.05.032
de Oliveira, D. W., França, I. W., Félix, A. K., Martins, J. J., Giro, M. E., Melo, V. M., et al. (2013). Kinetic study of biosurfactant production by Bacillus subtilis LAMI005 grown in clarified cashew apple juice. Coll. Surf. B Biointerfaces 101, 34–43. doi: 10.1016/j.colsurfb.2012.06.011
Doelle, M. B., and Doelle, H. W. (1990). Sugar-cane molasses fermentation by Zymomonas mobilis. Appl. Microbiol. Biotechnol. 33, 31–35.
Dolman, B. M., Wang, F., and Winterburn, J. B. (2019). Integrated production and separation of biosurfactants. Process Biochem. 83, 1–8.
Dong, S., and Sartaj, M. (2016). Statistical analysis and optimization of ammonia removal from landfill leachate by sequential microwave/aeration process using factorial design and response surface methodology. J. Environ. Chem. Eng. 4, 100–108.
Doong, R.-A., Wu, Y.-W., and Lei, W.-G. (1998). Surfactant enhanced remediation of cadmium contaminated soils. Water Sci. Technol. 37, 65–71.
Elouzi, A. A., Akasha, A. A., Elgerbi, A. M., El-Baseir, M., and El Gammudi, B. A. (2012). Removal of heavy metals contamination by bio-surfactants (Rhamnolipids). J. Chem. Pharm. Res. 4, 4337–4341.
El-Sheshtawy, H., and Doheim, M. (2014). Selection of Pseudomonas aeruginosa for biosurfactant production and studies of its antimicrobial activity. Egypt. J. Pet. 23, 1–6.
Franzetti, A., Gandolfi, I., Fracchia, L., Van Hamme, J., Gkorezis, P., Marchant, R., et al. (2014). Biosurfactant use in heavy metal removal from industrial effluents and contaminated sites. Biosurfactants Prod. Util. Process. Technol. Econ. 159, 361–370. doi: 10.1201/b17599-20
Gaur, V. K., and Manickam, N. (2021). “Microbial biosurfactants: production and applications in circular bioeconomy,” in Biomass, Biofuels, Biochemicals, eds A. Pandey, R. D. Tyagi, and S. Varjani (Amsterdam: Elsevier).
Ghojavand, H., Vahabzadeh, F., Roayaei, E., and Shahraki, A. K. (2008). Production and properties of a biosurfactant obtained from a member of the Bacillus subtilis group (PTCC 1696). J. Colloid Interface Sci. 324, 172–176. doi: 10.1016/j.jcis.2008.05.001
Griffin, H., Greene, R., and Cotta, M. (1992). Isolation and characterization of an alkaline protease from the marine shipworm bacterium. Curr. Microbiol. 24, 111–117.
Grzywaczyk, A., Smułek, W., Smułek, G., Ślachciński, M., and Kaczorek, E. (2021). Application of natural surfactants for improving the leaching of zinc and copper from different soils. Environ. Technol. Innov. 24:101926.
Hazrati, S., Farahbakhsh, M., Heydarpoor, G., and Besalatpour, A. A. (2020). Mitigation in availability and toxicity of multi-metal contaminated soil by combining soil washing and organic amendments stabilization. Ecotoxicol. Environ. Saf. 201:110807. doi: 10.1016/j.ecoenv.2020.110807
Helmy, Q., Kardena, E., Funamizu, N., and Wisjnuprapto (2011). Strategies toward commercial scale of biosurfactant production as potential substitute for it’s chemically counterparts. Int. J. Biotechnol. 12, 66–86. doi: 10.1504/ijbt.2011.042682
Heyd, M., Franzreb, M., and Berensmeier, S. (2011). Continuous Rhamnolipid production with integrated product removal by foam fractionation and magnetic separation of immobilized Pseudomonas aeruginosa. Biotechnol. Prog. 27, 706–716. doi: 10.1002/btpr.607
Hippolyte, M. T., Augustin, M., Hervé, T. M., Robert, N., and Devappa, S. (2018). Application of response surface methodology to improve the production of antimicrobial biosurfactants by Lactobacillus paracasei subsp. tolerans N2 using sugar cane molasses as substrate. Bioresour. Bioprocess. 5:48.
Huang, X., Peng, K., Feng, Y., Liu, J., and Lu, L. (2013). Separation and characterization of effective demulsifying substances from surface of Alcaligenes sp. S-XJ-1 and its application in water-in-kerosene emulsion. Bioresour. Technol. 139, 257–264. doi: 10.1016/j.biortech.2013.04.043
Jimoh, A. A., and Lin, J. (2020). Biotechnological applications of Paenibacillus sp. D9 lipopeptide biosurfactant produced in low-cost substrates. Appl. Biochem. Biotechnol. 191, 921–941. doi: 10.1007/s12010-020-03246-5
Joshi, S., Bharucha, C., Jha, S., Yadav, S., Nerurkar, A., and Desai, A. J. (2008). Biosurfactant production using molasses and whey under thermophilic conditions. Bioresour. Technol. 99, 195–199. doi: 10.1016/j.biortech.2006.12.010
Kammoun, R., Naili, B., and Bejar, S. (2008). Application of a statistical design to the optimization of parameters and culture medium for α-amylase production by Aspergillus oryzae CBS 819.72 grown on gruel (wheat grinding by-product). Bioresour. Technol. 99, 5602–5609. doi: 10.1016/j.biortech.2007.10.045
Kavuthodi, B., Thomas, S. K., and Sebastian, D. (2015). Co-production of pectinase and biosurfactant by the newly isolated strain Bacillus subtilis BKDS1. Br. Microbiol. Res. J. 10, 1–12. doi: 10.9734/bmrj/2015/19627
Khoobbakht, G., Najafi, G., Karimi, M., and Akram, A. (2016). Optimization of operating factors and blended levels of diesel, biodiesel and ethanol fuels to minimize exhaust emissions of diesel engine using response surface methodology. Appl. Therm. Eng. 99, 1006–1017.
Kim, B., and Kim, J. (2013). Optimization of culture conditions for the production of biosurfactant by Bacillus subtilis JK-1 using response surface methodology. J. Korean Soc. Appl. Biol. Chem. 56, 279–287.
Kiran, G. S., Nishanth, L. A., Priyadharshini, S., Anitha, K., and Selvin, J. (2014). Effect of Fe nanoparticle on growth and glycolipid biosurfactant production under solid state culture by marine Nocardiopsissp. MSA13A. BMC Biotechnol. 14:48. doi: 10.1186/1472-6750-14-48
Kulkarni, S., Kanekar, P., Jog, J., Sarnaik, S., and Nilegaonkar, S. (2015). Production of copolymer, poly (hydroxybutyrate-co-hydroxyvalerate) by Halomonas campisalis MCM B-1027 using agro-wastes. Int. J. Biol. Macromol. 72, 784–789. doi: 10.1016/j.ijbiomac.2014.09.028
Lee, J.-F., Liao, P.-M., Kuo, C.-C., Yang, H.-T., and Chiou, C. T. (2000). Influence of a nonionic surfactant (Triton X-100) on contaminant distribution between water and several soil solids. J. Colloid Interface Sci. 229, 445–452. doi: 10.1006/jcis.2000.7039
Makkar, R. S., Cameotra, S. S., and Banat, I. M. (2011). Advances in utilization of renewable substrates for biosurfactant production. AMB Express 1:5. doi: 10.1186/2191-0855-1-5
Malik, R., and Kerkar, S. (2019). Enhancing biosurfactant production by hypersaline Bacillus amyloliquefaciens SK27 using response surface methodology and genetic algorithm. Curr. Sci. 117, 847–852.
Mao, X., Jiang, R., Xiao, W., and Yu, J. (2015). Use of surfactants for the remediation of contaminated soils: a review. J. Hazard. Mater. 285, 419–435. doi: 10.1016/j.jhazmat.2014.12.009
Marchant, R., and Banat, I. M. (2012). Microbial biosurfactants: challenges and opportunities for future exploitation. Trends Biotechnol. 30, 558–565. doi: 10.1016/j.tibtech.2012.07.003
McBride, M. B. (1994). Environnaental Chemistry of Soils. New York, NY: Oxford University Press, Inc.
Mnif, I., Rajhi, H., Bouallegue, A., Trabelsi, N., and Ghribi, D. (2022). Characterization of lipopeptides biosurfactants produced by a newly isolated strain Bacillus subtilis ZNI5: potential environmental application. J. Polym. Environ. 30, 1–14. doi: 10.1007/s10924-021-02361-6
Moussa, T., Mohamed, M., and Samak, N. (2014). Production and characterization of di-rhamnolipid produced by Pseudomonas aeruginosa TMN. Braz. J. Chem. Eng. 31, 867–880. doi: 10.1590/0104-6632.20140314s00002473
Mulligan, C. N., Yong, R. N., and Gibbs, B. F. (1999). Removal of heavy metals from contaminated soil and sediments using the biosurfactant surfactin. J. Soil Contam. 8, 231–254. doi: 10.1016/s0304-3894(01)00224-2
Munguia, T., and Smith, C. A. (2001). Surface tension determination through capillary rise and laser diffraction patterns. J. Chem. Educ. 78:343. doi: 10.1021/ed078p343
Myers, R., Montgomery, D., and Anderson-Cook, C. (2016). Response Surface Methodology, Product and Process Optimization Using Experimental Design. New York, NY: Wiley.
Najafi, A., Rahimpour, M., Jahanmiri, A., Roostaazad, R., Arabian, D., Soleimani, M., et al. (2011). Interactive optimization of biosurfactant production by Paenibacillus alvei ARN63 isolated from an Iranian oil well. Colloids Surf. B Biointerfaces 82, 33–39. doi: 10.1016/j.colsurfb.2010.08.010
Ochoa-Loza, F. J., Artiola, J. F., and Maier, R. M. (2001). Stability constants for the complexation of various metals with a rhamnolipid biosurfactant. J. Environ. Qual. 30, 479–485. doi: 10.2134/jeq2001.302479x
Pacwa-Płociniczak, M., Płaza, G. A., Piotrowska-Seget, Z., and Cameotra, S. S. (2011). Environmental applications of biosurfactants: recent advances. Int. J. Mol. Sci. 12, 633–654. doi: 10.3390/ijms12010633
Pourfadakari, S., Ahmadi, M., Jaafarzadeh, N., Takdastan, A., Ghafari, S., and Jorfi, S. (2019). Remediation of PAHs contaminated soil using a sequence of soil washing with biosurfactant produced by Pseudomonas aeruginosa strain PF2 and electrokinetic oxidation of desorbed solution, effect of electrode modification with Fe3O4 nanoparticles. J. Hazard. Mater. 379:120839. doi: 10.1016/j.jhazmat.2019.120839
Pueyo, M. T., Bloch, C., Carmona-Ribeiro, A. M., and Di Mascio, P. (2009). Lipopeptides produced by a soil Bacillus megaterium strain. Microb. Ecol. 57, 367–378. doi: 10.1007/s00248-008-9464-x
Qiao, N., and Shao, Z. (2010). Isolation and characterization of a novel biosurfactant produced by hydrocarbon-degrading bacterium Alcanivorax dieselolei B-5. J. Appl. Microbiol. 108, 1207–1216. doi: 10.1111/j.1365-2672.2009.04513.x
Rane, A. N., Baikar, V. V., Ravi Kumar, V., and Deopurkar, R. L. (2017). Agro-industrial wastes for production of biosurfactant by Bacillus subtilis ANR 88 and its application in synthesis of silver and gold nanoparticles. Front. Microbiol. 8:492. doi: 10.3389/fmicb.2017.00492
Roelants, S. L., Ciesielska, K., De Maeseneire, S. L., Moens, H., Everaert, B., Verweire, S., et al. (2016). Towards the industrialization of new biosurfactants: biotechnological opportunities for the lactone esterase gene from Starmerella bombicola. Biotechnol. Bioeng. 113, 550–559. doi: 10.1002/bit.25815
Saimmai, A., Sobhon, V., and Maneerat, S. (2011). Molasses as a whole medium for biosurfactants production by Bacillus strains and their application. Appl. Biochem. Biotechnol. 165, 315–335. doi: 10.1007/s12010-011-9253-8
Salar, R. K., Purewal, S. S., and Bhatti, M. S. (2016). Optimization of extraction conditions and enhancement of phenolic content and antioxidant activity of pearl millet fermented with Aspergillus awamori MTCC-548. Resour. Efficient Technol. 2, 148–157.
Santos, D. K. F., Rufino, R. D., Luna, J. M., Santos, V. A., and Sarubbo, L. A. (2016). Biosurfactants: multifunctional biomolecules of the 21st century. Int. J. Mol. Sci. 17:401. doi: 10.3390/ijms17030401
Sarubbo, L., Brasileiro, P., Silveira, G., Luna, J., and Rufino, R. (2018). Application of a low cost biosurfactant in the removal of heavy metals in soil. Chem. Eng. Trans. 64, 433–438.
Sayyad, S. A., Panda, B. P., Javed, S., and Ali, M. (2007). Optimization of nutrient parameters for lovastatin production by Monascus purpureus MTCC 369 under submerged fermentation using response surface methodology. Appl. Microbiol. Biotechnol. 73, 1054–1058. doi: 10.1007/s00253-006-0577-1
Sharma, S., Verma, R., and Pandey, L. M. (2019). Crude oil degradation and biosurfactant production abilities of isolated Agrobacterium fabrum SLAJ731. Biocatal. Agric. Biotechnol. 21:101322. doi: 10.1016/j.bcab.2019.101322
Silva, R. D. C. F., Almeida, D. G., Rufino, R. D., Luna, J. M., Santos, V. A., and Sarubbo, L. A. (2014). Applications of biosurfactants in the petroleum industry and the remediation of oil spills. Int. J. Mol. Sci. 15, 12523–12542. doi: 10.3390/ijms150712523
Singh, J. S., Koushal, S., Kumar, A., Vimal, S. R., and Gupta, V. K. (2016). Book review: microbial inoculants in sustainable agricultural productivity-Vol. II: functional application. Front. Microbiol. 7:2105. doi: 10.3389/fmicb.2016.02105
Singh, P., and Cameotra, S. S. (2004). Potential applications of microbial surfactants in biomedical sciences. Trends Biotechnol. 22, 142–146. doi: 10.1016/j.tibtech.2004.01.010
Singh, P., Patil, Y., and Rale, V. (2019). Biosurfactant production: emerging trends and promising strategies. J. Appl. Microbiol. 126, 2–13. doi: 10.1111/jam.14057
Soberón-Chávez, G., and Maier, R. M. (2011). “Biosurfactants: a general overview,” in Biosurfactants, ed. G. Soberón-Chávez (Berlin: Springer-Verlag), 1–11. doi: 10.1007/978-3-642-14490-5_1
Sun, W., Cao, W., Jiang, M., Saren, G., Liu, J., Cao, J., et al. (2018). Isolation and characterization of biosurfactant-producing and diesel oil degrading Pseudomonas sp. CQ2 from Changqing oil field, China. RSC Adv. 8, 39710–39720.
Sun, W., Zhu, B., Yang, F., Dai, M., Sehar, S., Peng, C., et al. (2021). Optimization of biosurfactant production from Pseudomonas sp. CQ2 and its application for remediation of heavy metal contaminated soil. Chemosphere 265:129090. doi: 10.1016/j.chemosphere.2020.129090
Swarnkar, V., Agrawal, N., and Tomar, R. (2012). Sorption of chromate and arsenate by surfactant modified erionite (E-SMZ). J. Dispers. Sci. Technol. 33, 919–927. doi: 10.1080/01932691.2011.580183
Wei, Y.-H., Chou, C.-L., and Chang, J.-S. (2005). Rhamnolipid production by indigenous Pseudomonas aeruginosa J4 originating from petrochemical wastewater. Biochem. Eng. J. 27, 146–154. doi: 10.1016/j.bej.2005.08.028
Wu, W., Hu, Y., Guo, Q., Yan, J., Chen, Y., and Cheng, J. (2015). Sorption/desorption behavior of triclosan in sediment–water–rhamnolipid systems: effects of pH, ionic strength, and DOM. J. Hazard. Mater. 297, 59–65. doi: 10.1016/j.jhazmat.2015.04.078
Xue, W., Huang, D., Zeng, G., Wan, J., Zhang, C., Xu, R., et al. (2018). Nanoscale zero-valent iron coated with rhamnolipid as an effective stabilizer for immobilization of Cd and Pb in river sediments. J. Hazard. Mater. 341, 381–389. doi: 10.1016/j.jhazmat.2017.06.028
Keywords: optimization, response surface methodology (RSM), soil bioremediation, lipopeptide biosurfactant, heavy metals
Citation: Kalvandi S, Garousin H, Pourbabaee AA and Alikhani HA (2022) Formulation of a Culture Medium to Optimize the Production of Lipopeptide Biosurfactant by a New Isolate of Bacillus sp.: A Soil Heavy Metal Mitigation Approach. Front. Microbiol. 13:785985. doi: 10.3389/fmicb.2022.785985
Received: 29 September 2021; Accepted: 18 January 2022;
Published: 08 March 2022.
Edited by:
Parthipan Punniyakotti, Pondicherry University, IndiaReviewed by:
Vivek Rangarajan, Birla Institute of Technology and Science, IndiaSabarinathan Devaraj, Athenese, United States
Copyright © 2022 Kalvandi, Garousin, Pourbabaee and Alikhani. This is an open-access article distributed under the terms of the Creative Commons Attribution License (CC BY). The use, distribution or reproduction in other forums is permitted, provided the original author(s) and the copyright owner(s) are credited and that the original publication in this journal is cited, in accordance with accepted academic practice. No use, distribution or reproduction is permitted which does not comply with these terms.
*Correspondence: Ahmad Ali Pourbabaee, cG91cmJhYmFlaUB1dC5hYy5pcg==