- 1State Key Laboratory for Conservation and Utilization of Subtropical Agro-bioresources, Guangdong Provincial Key Laboratory of Agricultural and Rural Pollution Abatement and Environmental Safety, Integrative Microbiology Research Centre, South China Agricultural University, Guangzhou, China
- 2Guangdong Laboratory for Lingnan Modern Agriculture, Guangzhou, China
- 3Environmental Technologies Division, CSIR-National Botanical Research Institute, Rana Pratap Marg, Lucknow, India
As members of the organochlorine group of insecticides, aldrin and dieldrin are effective at protecting agriculture from insect pests. However, because of excessive use and a long half-life, they have contributed to the major pollution of the water/soil environments. Aldrin and dieldrin have been reported to be highly toxic to humans and other non-target organisms, and so their use has gradually been banned worldwide. Various methods have been tried to remove them from the environment, including xenon lamps, combustion, ion conversion, and microbial degradation. Microbial degradation is considered the most promising treatment method because of its advantages of economy, environmental protection, and convenience. To date, a few aldrin/dieldrin-degrading microorganisms have been isolated and identified, including Pseudomonas fluorescens, Trichoderma viride, Pleurotus ostreatus, Mucor racemosus, Burkholderia sp., Cupriavidus sp., Pseudonocardia sp., and a community of anaerobic microorganisms. Many aldrin/dieldrin resistance genes have been identified from insects and microorganisms, such as Rdl, bph, HCo-LGC-38, S2-RDLA302S, CSRDL1A, CSRDL2S, HaRdl-1, and HaRdl-2. Aldrin degradation includes three pathways: the oxidation pathway, the reduction pathway, and the hydroxylation pathway, with dieldrin as a major metabolite. Degradation of dieldrin includes four pathways: oxidation, reduction, hydroxylation, and hydrolysis, with 9-hydroxydieldrin and dihydroxydieldrin as major products. Many studies have investigated the toxicity and degradation of aldrin/dieldrin. However, few reviews have focused on the microbial degradation and biochemical mechanisms of aldrin/dieldrin. In this review paper, the microbial degradation and degradation mechanisms of aldrin/dieldrin are summarized in order to provide a theoretical and practical basis for the bioremediation of aldrin/dieldrin-polluted environment.
Introduction
Due to the largescale exploitation of natural resources and the destruction of the natural environment by human beings, the pressure on the biosphere is increasing (Akhtar and Mannan, 2020). The main causes of ecological pollution are excessive mining activities, industry, waste disposal, and agrochemicals (Gupta et al., 2020). Persistent organic pollutants (POPs) are toxic synthetic chemicals impacting on the environment, and have become a hot topic. POPs can accumulate in organisms and act as endocrine disruptors or carcinogens. Although the government has banned the use of POPs, the harm caused by these pollutants to the environment has continued because of people’s misuse of them and the long half-life of POPs (Adithya et al., 2021). It is, therefore, urgent to find a safe and effective method to avoid long-term damage to the environment.
Aldrin (CAS 309-00-2) and dieldrin (CAS 60-57-1) are synthetic organochlorine cyclodiene pesticides used to control subterranean insect pests such as nargles root maggots, mole cricket grubs and weevils, in agriculture. Aldrin’s (1,2,3,4,10,10-Hexachloro-1,4,4α,5,8,8α-hexahydro-1,4-endo, exo-5,8-dimethanonaphthalene) molecular formula is C12H8Cl6 and its half-life in soil is estimated to be 1.5–5.2 years (Blaylock, 2005a,b). Dieldrin’s (1,2,3,4,10,10-Hexachloro-6,7-epoxy-1,4,4α,5,6,7,8,8α-octahydro-1,4-endo, exo-5,8-dimethanonaphthalene) molecular formula is C12H8Cl6O and its half-life in soil is approximately 5 years (Blaylock, 2005b). In the natural environment, aldrin is usually converted to dieldrin by biotic or abiotic mechanisms, and the half-life of dieldrin is significantly longer. This review discusses dieldrin and its degradation products aldrin together because of their similar structure and properties (Blaylock, 2005a,b). They were widely used in the late 1940s; however, due to their toxicity and accumulation in the food chain, they were gradually banned in developed and developing countries in the late 1970s and 1980s (Luzardo et al., 2006). Organochlorine pesticides (OCPs) are major POPs, which have been banned or restricted worldwide, including aldrin and dieldrin (Adeleye et al., 2019).
Although reports of resistance to the cyclodiene pesticides aldrin and dieldrin account for more than 60% of reported cases of resistance, the overall incidence of related cases of resistance are declining (Ffrench-Constant et al., 2000). Therefore, various countries have launched the monitoring of organochlorine pesticides (Tham et al., 2020). Developed countries banned them early on, so most of the reported toxicity incidents involving aldrin and dieldrin now come from developing countries. Pesticide contamination is more severe in poorer countries, and its negative effects on ecology and life are therefore more profound (Høyer et al., 2000). The degradation of POPs includes physical and chemical degradation and biodegradation. Physical, chemical, and biological factors in the natural environment promote the degradation of POPs, of which biological factors play the major role (Chen et al., 2013; Lin et al., 2020; Pang et al., 2020b). Microorganisms are among the most important biological factors, including bacteria, fungi, and algae (Boudh et al., 2019; Lin et al., 2020). Physical and chemical methods, such as electro-oxidation, incineration, microwave induction, and chemical oxidation, not only require large and expensive infrastructure, but also produce byproducts that cannot be completely degraded and sometimes are even more toxic than the parent compounds (Pang et al., 2020a; Zhang et al., 2020). Microbial degradation, as one of the traditional treatment methods, is more economical and environmentally friendly because it degrades more completely (Zhang et al., 2021). As a result of the extensive use of aldrin and dieldrin, many non-microbial resistance genes have been detected in many places. The researchers isolated many strains that were able to effectively degrade aldrin and dieldrin (Abbas et al., 2020). The carbon component is an important driver of microbial community and function. A large number of genomic and complex metabolic studies have confirmed that dieldrin degradation evolved in a low-carbon environment, which provides a good direction to further isolate degrading microorganisms (Krohn et al., 2021).
Aldrin and dieldrin have been used for more than 80 years, and a lot of research has been conducted to investigate their toxicity and degradation (Matsumoto et al., 2008; Birolli et al., 2015; Wang et al., 2020). However, few reviews have focused on the microbial degradation and degradation mechanisms of aldrin/dieldrin. In this review, the residues of aldrin/dieldrin and their toxic effects on non-target organisms are discussed. Moreover, the microbial strains and their degradation enzymes/genes for aldrin/dieldrin that are responsible for biodegradation are summarized. This review updates the knowledge on degradation strategies with a focus on the metabolic pathways and molecular mechanisms involved in the biodegradation of aldrin/dieldrin.
Toxicity of Aldrin and Dieldrin
Aldrin and dieldrin belong to the same group of pesticides, most of which have the same toxicology (Hidayati et al., 2021). They have been in use for more than 80 years and have had a profound impact on the world (Hua et al., 2021). Although these two pesticides are banned around the world, concentrations can still be detected in some places. The remaining aldrin and dieldrin in the environment have a persistent toxic effect on local ecosystems and non-target organisms. The toxicity and environmental fate of aldrin and dieldrin is shown in Figure 1.
Environmental Accumulation of Aldrin and Dieldrin
During the use of pesticides, only 10% of their volume is utilized, while the other 90% will remain in the ecosystem (Lin et al., 2022). The pesticide residues migrate to other places via volatilization, washing, dust, etc., which in turn causes wider pollution (Bhatt et al., 2021; Mishra et al., 2022). Here is a systematic summary of aldrin and dieldrin contamination reported in different places in recent years. A combination of gas chromatography and electron capture detection (GC-ECD) was used to determine organochlorine pesticides in two common leafy vegetables from southwest Nigeria. Risk assessments indicated that aldrin and dieldrin may pose a carcinogenic health risk to adults (60 kg) and children (16.7 kg) (Adeleye et al., 2019). The concentrations of dieldrin detected in surface and ground water consumed in the cacao crop-dominated agricultural catchment of Ancobra Basin, Ghana, may lead to cancer in local children (Affum et al., 2018). Surface water from the Thamirabarani River water system in southern India was detected at aldrin levels above the World Health Organization’s maximum residue limit for surface water (0.03 g⋅L–1) (Arisekar et al., 2019). A health risk assessment of fish in Lake Saint Lucia indicated that residual concentrations of aldrin and dieldrin in fish muscle tissue could lead to potential dietary risks in humans (Buah-Kwofie and Humphries, 2021). The estimated daily intake of aldrin or dieldrin was found to exceed the allowable daily intake in breastmilk from urban and semi-urban areas and was greater in urban than in semi-urban areas (Anand et al., 2021). An investigation of dieldrin exposure in 8-year-old Latinx boys and girls from rural, farm-worker families and urban, non-farm-worker families in North Carolina found that dieldrin was pervasive in the living environments of children in vulnerable immigrant communities (Arcury et al., 2021). Estimated daily intake values (EDI) of aldrin and dieldrin by cattle slaughtered in Benin, southern Nigeria exceeded the threshold, leading to a non-cancer health risk for children consuming contaminated cattle parts (Tongo and Ezemonye, 2015).
With emphasis on the ecological environment, countries around the world have reported on their own environmental pollution. Rwanda’s first assessment of contaminants in its soil found levels of aldrin in the range of 0.38–0.59 μg⋅kg–1⋅dw (Umulisa et al., 2020). A decline in dieldrin concentrations in the Niagara River was detected using a Hilbert–Huang transform analysis (Tsai and Treadwell, 2019). Dieldrin has been found in water, fish, sediments, and large parasites in Okobaba, Lagos Lagoon, Nigeria (Bamidele et al., 2019). High concentrations of polyaromatic hydrocarbons (PAHs) and polychlorinated biphenyl (PCBs) were found in samples collected from coastal areas in the refinery area of the Kingdom of Bahrain (Bersuder et al., 2020). An organochlorine pesticide analysis of shrimp from all continents found that Asia was the continent with the highest reported values, with aldrin and dieldrin concentrations of 0.003 μg⋅L–1 (Maia et al., 2020). The concentrations of dieldrin in the water, soil, and sediment of the Ruffiji River Delta in Tanzania exceeded safety guidelines, with dire potential consequences if they are not managed and emergency interventions taken (Mwevura et al., 2021). The highest concentrations of dieldrin (0.99 ± 0.33 μg⋅kg–1) were found in the sediments of southern and southern river ecosystems in Nigeria (Ogbeide et al., 2019). Surveillance of pesticides in rivers and lakes in northern Greece found average annual concentrations of dieldrin (0.02 μg⋅L–1) exceeding the EU environmental quality standards (Papadakis et al., 2015). Concentrations of organochlorine pesticides detected in Serbian river and artificial lake sediments ranged from below the detection limit to 113 μg⋅kg–1 (Sakan et al., 2017). Aldrin (75.31 ng⋅L–1) and dieldrin (71.19 ng⋅L–1) were found in stream water in Mumbai, India, exceeding their respective standard levels but below the range set by the America Environmental Protection Agency (EPA) at 0.13 65.1 ng⋅L–1 (Singare, 2016).
Toxicity of Aldrin and Dieldrin in Living Systems
Aldrin and dieldrin pose a risk to human health throughout the world because they accumulate in the food chain and the human body cannot metabolize them (Maia et al., 2020). Dieldrin is immunogenic to humans, leading to dopaminergic neurodegeneration, which causes chemically immunohemolytic anemia or gives rise to Parkinson’s disease (Hamilton et al., 1978; Kanthasamy et al., 2005, 2020). Contact with dieldrin can induce the production of reactive oxygen species or cause proinflammatory reaction and DNA damage in human ovary surface epithelial cells (Shah et al., 2020). Aldrin acts as a ligand for androgens and alters epigenetic inheritance, which may contribute to prostate cancer development and growth. Exposure to dieldrin causes an aggravation of the behavioral and biochemical deficits induced by male-specific synuclein disease (Gezer et al., 2020). Dieldrin can induce estrogen action and inhibit androgen signaling pathways, which can lead to breast cancer (Aubé et al., 2011).
In mammals, as in humans, aldrin and dieldrin accumulate toxicity through the food chain. Dieldrin inhibited the activity of Mg2+-ATPase in rats and stimulated the activity of 5′-nucleotide enzyme and NADH-dehydrogenase in liver cell membranes, and L-ascorbic acid was needed to protect the Mg2+-ATPase (Bandyopadhyay et al., 1982). When rats were exposed to aldrin over a period of time, the relative liver weight increased significantly, and the survival rate of female rats decreased (Adam et al., 2015). In Punjab, India, bioaccumulation of 14 pesticides, including dieldrin and aldrin, has been found in the tissue stroma of dogs with malignant canine breast tumors (Gautam et al., 2020).
Toxicity of Aldrin and Dieldrin in Aquatic Organisms
The biodegradable potential of pesticides can have a significant impact on the population dynamics of aquatic species. In ecological model assessments, the population of aquatic species changes when pesticides remain in the water for long periods of time (Achema et al., 2021). Among non-target aquatic organisms, pesticides have the greatest negative impact on fish, followed by water fleas and algae (Affum et al., 2018). Endocrine reactions in fish are related to the pollutant load in sediments. In Lagos Lagoon, Nigeria, contaminated with dieldrin, male fish gradually feminized, while the reproductive health of other fish also changed accordingly (Adeogun et al., 2019). Dieldrin inhibited the liver cytochrome P-450 expression of female Micropterus salmoides during the breeding period (Barber et al., 2007). Aldrin/dieldrin is the contaminant most associated with oxidative damage in the livers of two wild fish species at the Furnas Hydroelectric Power Station (HPS) reservoir (Minas Gerais, Brazil) (Sakuragui et al., 2013). After 24 h exposure, dieldrin significantly affected Danio rerio and Daphnia pulex’s swimming behavioral response and sensitivity (Alla et al., 2021). As a teratogen, dieldrin can alter the expression of dopamine receptor 2A and dopamine transporter in zebrafish (Danio rerio) embryos (Sarty et al., 2017).
Degradation of Aldrin and Dieldrin
In the natural environment, aldrin and dieldrin remain in the air, soil, and water. The physical and chemical conditions directly affect the behavior and distribution of aldrin and dieldrin residues. During their interaction with organic or mineral components in the soil, natural degradation occurs. Due to the indiscriminate use of organic pollutants, which hardly degrade under natural conditions, many methods have been developed to facilitate their degradation, including physicochemical methods, the use of microorganisms, and gene therapy (Feng et al., 2020).
Degradation of Aldrin and Dieldrin by Physicochemical Methods
Under the irradiation of a xenon lamp below 290 nm, aldrin reacts with α-diketones such as glyoxal, phenylglyoxal, methyl glyoxal, diacetyl, 1-phenyl-1, 2-malondione, and phenyl to form the corresponding epoxide compound, dieldrin (Nojima and Isogami, 1993). After gas phase thermal decomposition and toxic combustion, the pyrolysis of dieldrin yields chlorinated benzene and chlorinated phenols, known as precursors of polychlorinated dibenzo-p-dioxins and dibenzo-furans (Dharmarathne et al., 2019). The non-pathogenic bacterium Shevanella oneidensis does not directly degrade dieldrin, but can efficiently reduce about 80% of dissolved ferric iron (Fe3+) to ferrous iron (Fe2+) within 72 h. The effective removal time of many organochlorine pesticides (OCPs) is greatly reduced by the catalysis of ferrous iron (Fe2+). When the iron dosage was increased from 2.5 × 103 mg⋅L–1 to 1.5 × 104 mg⋅L–1, the removal rate of dieldrin was increased by 23.3% (Abbas et al., 2020). Physicochemical reactions are rarely used in real life because they cannot completely degrade compounds and are limited to large instruments (Lin et al., 2020).
Degradation of Aldrin and Dieldrin by Microorganisms
Bioremediation of aldrin and dieldrin is possible with the application of indigenous microbial strains (Arora, 2020; Bhatt et al., 2022). This requires effective microbial food and nutrients, known as the bioremediation triangle (Boudh et al., 2019). Microorganisms take pollutants as their main carbon and nitrogen sources or auxiliary nutrients to grow and consume pollutants so that they achieve the purpose of removing pollutants (Bourtzis et al., 2016; Cycoń et al., 2017; Mishra et al., 2021). In the development of bioremediation methods, it is very important to screen microorganisms that degrade organic pollutants and identify their metabolites. Bacteria, fungi and algae are used in biodegradation experiments (Zhao et al., 2017, 2020; Bhatt et al., 2020; Huang et al., 2020). Yeast is a common eukaryotic recipient cell for gene cloning experiments (Chen et al., 2012; Ali et al., 2020). Actinomycetes are particularly suitable for the colonization of terrestrial ecosystems because of their mycelial growth pattern and ability to produce large amounts of extracellular enzymes (Chen et al., 2011; Magan et al., 2022). However, there are no available reports on the ability of yeasts and actinomycetes to degrade aldrin/dieldrin. Till date, there are several bacteria and fungi that have been clearly reported to effectively degrade aldrin/dieldrin (Sakakibara et al., 2011; Xiao et al., 2011a; Purnomo et al., 2017). In addition, the degradation of a microbial consortium has been studied (Watanabe and Yoshikawa, 2008). Aldrin and dieldrin are degraded in the soil because a few microorganisms can efficiently metabolize them, as shown in Table 1.
Bacterial Degradation of Aldrin/Dieldrin
Pseudomonas is effective at degrading a variety of pesticides in water, such as dieldrin, aldrin, and heptachloride (Bandala et al., 2006; Birolli et al., 2019). However, due to the low bioavailability of dieldrin, it is difficult to isolate more degrading microorganisms. Matsumoto et al. (2008) obtained Burkholderia sp. Med-7 and Cupriavidus sp. Med-5 degrading bacteria with dieldrin as the only carbon source by screening bacteria with 1,2-epoxycyclohexane (ECH), which can degrade 49 and 38% of dieldrin, respectively. Enterobacter sp. LY402 is able to degrade 40.4% of dieldrin (5.0 mg⋅L–1) in 168 h (Gao et al., 2008). In addition, the effective strain Pseudonocardia sp. KSF27 was successfully isolated by using a soil–charcoal perfusion method with aldrin transdiol as an analog substrate (Sakakibara et al., 2011). The bioremediation potential of this strain is very high. It not only degrades 85% of 14.06 μM of dieldrin, but also degrades a variety of refractory pollutants, such as endosulfan, endosulfan sulfate, and heptachloride.
The above test results also prove that aldrin transdiol and ECH are effective structural analogs that can be used to isolate degrading microorganisms capable of degrading aldrin/dieldrin. This is also a good guideline that could be used to screen the degradation microorganisms of other hard-to-degrade compounds. The use of structural analogs is considered a useful method for isolating microorganisms with highly refractory compounds, and to be less time-consuming and labor-saving than conventional methods. In addition, co-metabolism plays an important role in the process of bioremediation and is an important direction for screening degrading bacteria (Matsumoto et al., 2008).
Fungal Degradation of Aldrin/Dieldrin
Fungi are generally more tolerant to high concentrations of contaminant chemicals than bacteria, and white rot fungi (WRF) have been especially well studied. In previous studies, WRF was able to oxidize a wider variety of refractory compounds, such as lindane, lignin, dichlorodiphenyltrichloroethane (DDT) (Xiao et al., 2011b; Hou et al., 2020; Xiao and Kondo, 2020). Therefore, WRF are potentially biodegradable fungi that are seen as a promising bioremediation agent, especially for compounds that are not easily degraded by bacteria.
To date, several genera of fungi have been investigated for their degradation potential of xenobiotics such as aldrin and dieldrin (Huang et al., 2021; Lin et al., 2021). Fragoeiro and Magan (2005) studied the WRF Phanerochaete chrysosporium and Trametes versicolor, which can completely degrade 5–30 mg⋅L–1 of dieldrin within 25 days. The wood-rotting fungal strain YK543, isolated from rotten wood, can degrade approximate 39.1% of dieldrin within 30 days (Fragoeiro and Magan, 2005; Kamei et al., 2010). Xiao also found three WRF, Phlebia acanthocystis, Phlebia brevispora, and Phlebia aurea. They all removed up to 50% of dieldrin and 90% of aldrin in a low-nitrogen medium within 42 days (Xiao et al., 2011a). However, 42 days is a long time, so it is important to isolate fungi that can rapidly degrade aldrin and dieldrin. For the first time, researchers isolated the marine fungus Penicillium miczynskii CBMAI 930, which can convert up to 90% (50 mg⋅L–1) of dieldrin within 14 days (Birolli et al., 2015). A more efficient WRF fungus, Pleurotus ostreatus, was isolated, which completely eliminated aldrin from potato dextrose broth (PDB) medium within 14 days (Purnomo et al., 2017). It also eliminated 18% of the dieldrin in a PDB medium within 14 days.
A strain of Mucor racemosus DDF isolated from endosulfan-contaminated soil was able to degrade dieldrin over a wide pH range (Kataoka et al., 2010). The DDF strain is able to grow rapidly in soil compared to white rot fungi, which is an advantage. In addition, M. racemosus DDF degrades not only dieldrin, but also many organochlorine pesticides like DDT and endosulfan. Therefore, M. racemosus DDF is a potential bioremediation microorganism. This study also showed that endosulfan-contaminated soil is a potential way to isolate dieldrin-degrading bacteria. In addition, dieldrin-degrading fungi such as Trichoderma viride, Mucor alternans, and Pleurotus ostreatus have been isolated by previous researchers, and these fungi can be used as potential microorganisms for bioremediation (Matsumura and Bous, 1967; Purnomo et al., 2017; Zhao et al., 2017). Fungi are more suitable candidates for the bioremediation of dieldrin than bacteria because fungi can degrade these chemicals in a short time with complete mineralization.
Degradation of Aldrin/Dieldrin by a Microbial Consortium
Compared with single-bacterium degradation of compounds, microbial consortium degradation has some advantages. Microbial consortium cultures can break down complex mixtures with different microorganisms, increasing the degradation capacity and efficiency (Meyer-Cifuentes et al., 2020; Gielnik et al., 2021; Miao et al., 2021). To date, several microbial consortia have been used to test the degradation of aldrin/dieldrin. The microbial consortium of Clostridium bifermentans, Clostridium glovolium, and other Clostridium sp. is the most efficient, with a degradation rate of 96% of 10 mg⋅L–1 dieldrin within 7 days (Maule et al., 1987). Another consortium, containing 11 morphologically identical anaerobic microorganisms, is also very efficient and degrades 75.6% of dieldrin (100 mg⋅L–1) and 65.4% of aldrin (100 mg⋅L–1) within 2 weeks (Watanabe and Yoshikawa, 2008). However, microbial consortium is not always efficient, probably because of the antagonism. A microbial consortium can transform 88% of dieldrin (9 mg⋅L–1) within 3 months, and 70% of aldrin (7 mg⋅L–1) within 160 days (Baczynski et al., 2004). A mixed indigenous microorganism containing Acidaminobacter, Clostridium, and an uncultured bacterial group can degrade low concentrations of dieldrin (from 0.5 to 10 μg⋅mL–1) (Chiu et al., 2005). According to these results, the use of a microbial consortium is an effective way to achieve aldrin/dieldrin degradation.
Degradation Pathways of Aldrin or Dieldrin
The degradation pathways of aldrin and dieldrin found in previous research are shown in Figure 2. Dieldrin is often found as a metabolite of aldrin, and aldrin-degrading microorganisms are usually able to degrade dieldrin. The degradation of aldrin can be divided into three types: the oxidation pathway, the reduction pathway, and the hydroxylation pathway (Bandala et al., 2006; Sakakibara et al., 2011; Xiao et al., 2011a). In the oxidation degradation pathway of aldrin, dieldrin is the main metabolite and has a stable structure. When the non-chlorinated double bond is epoxidized in the initial metabolism, aldrin can be converted to dieldrin. When the non-chlorinated double bond is oxidized, aldrin is metabolized by microorganisms to dihydrochlordenedicarboxylic acid and monohydroxy dihydrochlordenedicarboxylic acid. In the reductive degradation pathway, aldrin reacts to form monochlorodieldrin. For highly chlorinated organic compounds, dechlorination reactions are particularly interesting because they usually produce lower toxic metabolites or are readily degradable. When methylene is hydroxylated, aldrin forms 9-hydroxyaldrin.
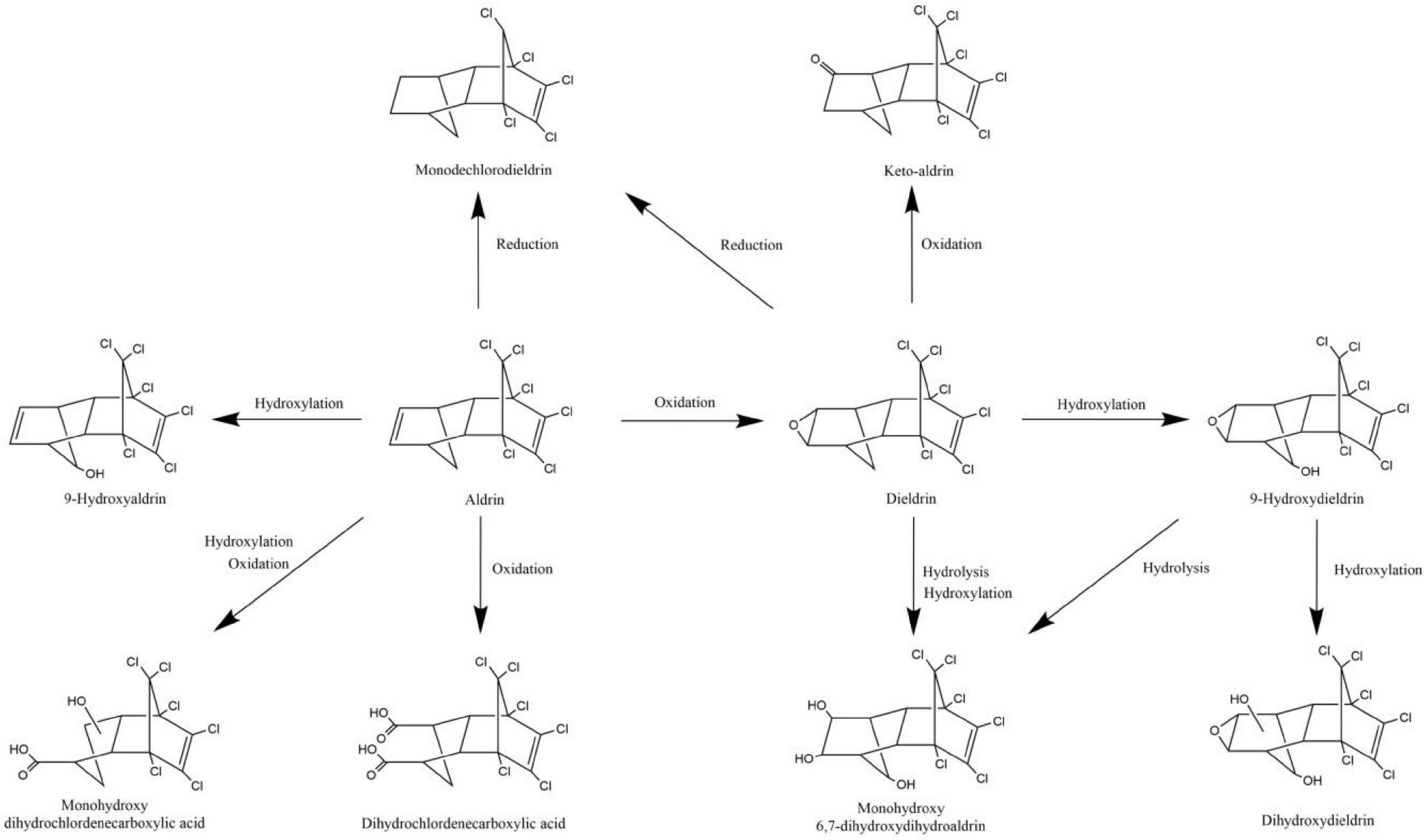
Figure 2. Biodegradation pathways of aldrin and dieldrin (Bandala et al., 2006; Sakakibara et al., 2011; Xiao et al., 2011a).
Degradation of dieldrin includes four pathways: oxidation, reduction, hydroxylation, and hydrolysis (Bandala et al., 2006; Sakakibara et al., 2011; Xiao et al., 2011a). In the presence of microorganisms, dieldrin is oxidized to keto-aldrin or reduced to monochlorodieldrin, which is the same as the reduction product of aldrin. Dieldrin is hydrolyzed to monohydroxy 6, 7-dihydroxydihydroaldrin or 9-hydroxydieldrin. 9-Hydroxydifferent can be further hydrolyzed to 6, 7-dihydroxydihydroaldrin or dihydroxydieldrin, both of which are highly water-soluble (Bandala et al., 2006; Sakakibara et al., 2011).
Based on this degradation pathway, it is inferred that oxidative degradation and reductive dechlorination are important degradation mechanisms of aldrin and dieldrin (Sakakibara et al., 2011). However, neither dieldrin nor aldrin has been documented for the eventual mineralization. In order to further promote the development of biodegradation of aldrin and dieldrin, it is necessary to better understand the degradation mechanism.
Gene(s) Involved in the Degradation of Aldrin and Dieldrin
The gene identified as having a high level of resistance to dieldrin (Rdl) was first identified in mutant Drosophila, where the single amino acid Ala at position 302 was replaced by Ser/Gly (Ffrench-Constant et al., 1993). The SCD strain is resistant to chemical insecticide and the Bacillus thuringiensis toxin. When researchers knocked out the HaRdl-1 gene in the SCD strain, they found that it was more resistant to dieldrin, but when they knocked out the HaRdl-2 gene in the SCD strain, it was more sensitive to dieldrin. Therefore, it can be speculated that SCD strains develop target-based resistance to dieldrin through a loss of function mutation of HaRdl-1 or enhancement of expression of HaRdl-2 (Wang et al., 2020). The Rdl gene is present in most types of organisms that feed on crops, while those that do not feed on crops apparently have no resistance. Mutations in Rdl occur once or in large numbers, depending on the population biology of the insects. The Rdl sites are large (over 50 kb in size) and the alternative splicing is complex.
When the gene at the Rdl locus is deleted, mutated insects cannot survive (Ffrench-Constant et al., 2000). In general, most insect species contain only one Rdl homologous sequence, such as the Red flour beetle, whiteflies, the coffee berry borer, and Drosophila species (Ffrench-Constant et al., 2000). However, pea aphids have two copies of Rdl (Rdl1 and Rdl2), which are likely derived from recent gene duplicates (Del Villar and Jones, 2018). Chilo suppressalis contains two subtypes of cDNA of the RDL subunit (CSRDL1A and CSRDL2S). Although CSRDL2S expression is twice as high as CSRDL1A at all growth stages, they have similar expression patterns (Sheng et al., 2018). G-aminobutyric acid (GABA), a gated chloride ion channel, is an important inhibitory neurotransmitter in the animal nervous system. As a model insect of Lepidoptera, the silkworm (Bombyx mori) has the largest known insect GABA gene family in the world, including three Rdls (Rdl1, Rdl2, and Rdl3). The three Rdls subunits may be generated by two repeated events, among which Rdl1 gene has an RNA editing site, and Rdl1 and Rdl3 can perform alternative splicing (Yu et al., 2010).
In nematode worms, scientists identified a new cyst-ring GABA receptor subcluster (HCo-LGC-38) that is moderately sensitive to fipronil and dieldrin (Siddiqui et al., 2012). S2-RDLA302S of the Drosophila melanogaster cell line increased its resistance to dieldrin (Buckingham et al., 1996). The expression of the heterologous bph gene in F113pcb can enable transgenic microorganisms in the rhizosphere to utilize biphenyl as the sole carbon source (Brazil et al., 1995).
Worryingly, Rdl sites have also been shown to be resistant to new insecticides such as fipronil; this cross-resistance must be overcome or avoided (Ffrench-Constant et al., 2000). Lindane and cyclodiene pesticides are the first generation of non-competitive antagonists (NCAs) that enable insects to produce Rdl GABA receptors. Fipronil is a second-generation NCA, and extra attention needs to be paid to fipronil-resistant insects to prevent further agricultural losses (Nakao, 2017). Alanine 302 of exon 7 of the Rdl was replaced by a serine in the cat flea (Ctenocephalides felis) population, thus developing resistance to fipronil (Daborn et al., 2004). Alanine 302 of exon 7 of Rdl was replaced by a serine in the cat flea population, leading to resistance to fipronil (Daborn et al., 2004). However, the A302S mutation of Rdl has limited cross-resistance to fipronil and is not detected in field populations of the American housefly (Gao et al., 2007). These are grouped in Table 2 for easy reference.
Conclusion and Future Prospects
Aldrin and dieldrin are widely used for their insecticidal activity, but are restricted because of the toxic effect on the environment. Both insecticides have been used for a long time, and their toxic effects on non-target organisms cannot be ignored as aldrin and dieldrin accumulate in the environment and in food chains. Residual aldrin and dieldrin are toxic to a variety of animals, including humans, dogs, fish, shrimp, and other non-target organisms. Therefore, it is necessary to develop efficient, economical, and environmentally friendly technologies for the degradation of aldrin and dieldrin. Microorganisms have the potential to detoxify exogenous compounds easily through several metabolic pathways, and detoxification by indigenous microorganisms is recognized as the most promising remediation approach. A microbial consortium has reported as having superior performance over a pure culture. The reason for the improved performance is the reduction of the metabolic burden. However, the published literature on the microbial remediation of aldrin/dieldrin-contaminated sites remains inadequate. In addition, the development of biodegradable genes/enzymes and degradation microorganisms must be an ongoing project in the future. In recent years, the biodegradation of pesticides based on omics technology has provided a new direction for the bioremediation of environmental pollution by pesticides. Omics-based evaluations could explore in more depth the mechanisms involved in the bioremediation of these xenobiotics.
Author Contributions
SC conceived of the presented idea. SP and ZL contributed to the writing and prepared the figures and tables. JL, YZ, SM, PB, and SC participated in revising the manuscript. All authors approved it for publication.
Funding
This study was supported by grants from the Key-Area Research and Development Program of Guangdong Province, China (2020B0202090001), the Natural Science Foundation of Guangdong Province, China (2021A1515010889 and 2022A1515010422), and the Science and Technology Planning Project of Guangdong Province, China (2021B1212040008 and 20210205).
Conflict of Interest
The authors declare that the research was conducted in the absence of any commercial or financial relationships that could be construed as a potential conflict of interest.
Publisher’s Note
All claims expressed in this article are solely those of the authors and do not necessarily represent those of their affiliated organizations, or those of the publisher, the editors and the reviewers. Any product that may be evaluated in this article, or claim that may be made by its manufacturer, is not guaranteed or endorsed by the publisher.
References
Abbas, T., Wadhawan, T., Khan, A., McEvoy, J., and Khan, E. (2020). Virgin (Fe0) and microbially regenerated (Fe2+) iron turning waste for treating chlorinated pesticides in water. J. Hazard. Mater. 398:122980. doi: 10.1016/j.jhazmat.2020.122980
Achema, K., Okuonghae, D., and Tongo, I. (2021). Dual-level toxicity assessment of biodegradable pesticides to aquatic species. Ecol. Complex. 45: 100911.
Adam, T., Emily, N., and McCain, W. (2015). “Wildlife toxicity assessment for aldrin and dieldrin,” in Wildlife Toxicity Assessments for Chemicals of Military Concern, eds M. A. Williams, G. Reddy, M. J. Quinn, and M. S. Johnson (Amsterdam: Elsevier), 367–384. doi: 10.1016/b978-0-12-800020-5.00021-1
Adeleye, A., Sosan, M., and Oyekunle, J. (2019). Dietary exposure assessment of organochlorine pesticides in two commonly grown leafy vegetables in South-western Nigeria. Heliyon 5:e01895. doi: 10.1016/j.heliyon.2019.e01895
Adeogun, A., Ibor, O., Chukwuka, A., Regoli, F., and Arukwe, A. (2019). The intersex phenomenon in Sarotherodon melanotheron from Lagos lagoon (Nigeria): occurrence and severity in relation to contaminants burden in sediment. Environ. Pollut. 244, 747–756. doi: 10.1016/j.envpol.2018.10.091
Adithya, S., Jayaraman, R., Krishnan, A., Malolan, R., Gopinath, K., Arun, J., et al. (2021). A critical review on the formation, fate and degradation of the persistent organic pollutant hexachlorocyclohexane in water systems and waste streams. Chemosphere 271:129866. doi: 10.1016/j.chemosphere.2021.129866
Affum, A., Acquaah, S., Osae, S., and Kwaansa-Ansah, E. (2018). Distribution and risk assessment of banned and other current-use pesticides in surface and groundwaters consumed in an agricultural catchment dominated by cocoa crops in the Ankobra Basin, Ghana. Sci. Total Environ. 633, 630–640. doi: 10.1016/j.scitotenv.2018.03.129
Akhtar, N., and Mannan, M. (2020). Mycoremediation: expunging environmental pollutants. Biotechnol. Rep. 26:e00452.
Ali, S. S., Al-Tohamy, R., Xie, R., El-Sheekh, M. M., and Sun, J. (2020). Construction of a new lipase- and xylanase-producing oleaginous yeast consortium capable of reactive azo dye degradation and detoxification. Bioresesour. Techchnol. 313:123631. doi: 10.1016/j.biortech.2020.123631
Alla, L., Monshi, M., Siddiqua, Z., Shields, J., Alame, K., Wahls, A., et al. (2021). Detection of endocrine disrupting chemicals in Danio rerio and Daphnia pulex: step-one, behavioral screen. Chemosphere 271:129442. doi: 10.1016/j.chemosphere.2020.129442
Anand, N., Chakraborty, P., and Ray, S. (2021). Human exposure to organochlorine, pyrethroid and neonicotinoid pesticides: comparison between urban and semi-urban regions of India. Environ. Pollut. 270:116156. doi: 10.1016/j.envpol.2020.116156
Arcury, T., Chen, H., Quandt, S., Talton, J., Anderson, K., Scott, R., et al. (2021). Pesticide exposure among Latinx children: comparison of children in rural, farmworker and urban, non-farmworker communities. Sci. Total Environ. 763:144233. doi: 10.1016/j.scitotenv.2020.144233
Arisekar, U., Shakila, R., Jeyasekaran, G., Shalini, R., Kumar, P., Malani, A., et al. (2019). Accumulation of organochlorine and pyrethroid pesticide residues in fish, water, and sediments in the Thamirabarani river system of southern peninsular India. Environ. Nanotechnol. Monit. Manag. 11:100194. doi: 10.1016/j.enmm.2018.11.003
Arora, P. K. (2020). Bacilli-mediated degradation of xenobiotic compounds and heavy metals. Front. Bioeng. Biotechnol. 8:570307. doi: 10.3389/fbioe.2020.570307
Aubé, M., Larochelle, C., and Ayotte, P. (2011). Differential effects of a complex organochlorine mixture on the proliferation of breast cancer cell lines. Environ. Res. 111, 337–347. doi: 10.1016/j.envres.2011.01.010
Baczynski, T., Grotenhuis, T., and Knipscheer, P. (2004). The dechlorination of cyclodiene pesticides by methanogenic granular sludge. Chemosphere 55, 653–659. doi: 10.1016/j.chemosphere.2003.11.029
Bamidele, A., Abayomi, A., Iyabo, A., and Giwa, M. (2019). Parasitic fauna, histopathological alterations, and organochlorine pesticides contamination in Chrysichthys nigrodigitatus (Lacepede, 1803) (Bagridae) from Lagos. Lagoon, Nigeria. Sci. Afr. 5:e00130. doi: 10.1016/j.sciaf.2019.e00130
Bandala, E., Andres-Octaviano, J., Pastrana, P., and Torres, L. (2006). Removal of aldrin, dieldrin, heptachlor, and heptachlor epoxide using activated carbon and/or Pseudomonas fluorescens free cell cultures. J. Environ. Sci. Health B 41, 553–569.
Bandyopadhyay, S., Tiwari, R., Bhattacharyya, A., and Chatterjee, G. (1982). Effect of dieldrin on rat liver plasma membrane enzymes. Toxicol. Lett. 11, 131–134.
Barber, D., McNally, A., Garcia-Reyero, N., and Denslow, N. (2007). Exposure to p,p’-DDE or dieldrin during the reproductive season alters hepatic CYP expression in largemouth bass (Micropterus salmoides). Aquat. Toxicol. 81, 27–35.
Bersuder, P., Smith, A., Hynes, C., Warford, L., Barber, J., Losada, S., et al. (2020). Baseline survey of marine sediments collected from the Kingdom of Bahrain: PAHs, PCBs, organochlorine pesticides, perfluoroalkyl substances, dioxins, brominated flame retardants and metal contamination. Mar. Pollut. Bull. 161:111734. doi: 10.1016/j.marpolbul.2020.111734
Bhatt, P., Bhandari, G., Bhatt, K., Maithani, D., Mishra, S., Gangola, S., et al. (2021). Plasmid-mediated catabolism for the removal of xenobiotics from the environment. J. Hazard. Mater. 420:126618. doi: 10.1016/j.jhazmat.2021.126618
Bhatt, P., Pandey, S. C., Joshi, S., Chaudhary, P., Pathak, V. M., Huang, Y., et al. (2022). Nanobioremediation: a sustainable approach for the removal of toxic pollutants from the environment. J. Hazard. Mater. 427:128033. doi: 10.1016/j.jhazmat.2021.128033
Bhatt, P., Rene, E. R., Kumar, A. J., Zhang, W., and Chen, S. (2020). Binding interaction of allethrin with esterase: bioremediation potential and mechanism. Bioresour. Technol. 315:123845. doi: 10.1016/j.biortech.2020.123845
Birolli, W., Yamamoto, K., de Oliveira, J., Nitschke, M., Seleghim, M., and Porto, A. L. M. (2015). Biotransformation of dieldrin by the marine fungus Penicillium miczynskii CBMAI 930. Biocatal. Agric. Biotechnol. 4, 39–43. doi: 10.1016/j.bcab.2014.06.002
Birolli, W. G., Lima, R. N., and Porto, A. L. M. (2019). Applications of marine-derived microorganisms and their enzymes in biocatalysis and biotransformation, the underexplored potentials. Front. Microbiol. 10:1453. doi: 10.3389/fmicb.2019.01453
Blaylock, B. (2005a). “Aldrin,” in Encyclopedia of Toxicology (Second Edition), ed. P. Wexler (New York: Elsevier), 66–68.
Blaylock, B. (2005b). “Dieldrin,” in Encyclopedia of Toxicology (Second Edition), ed. P. Wexler (New York: Elsevier), 15–17.
Boudh, S., Singh, J., and Chaturvedi, P. (2019). “Microbial resources mediated bioremediation of persistent organic pollutants,” in new and future developments in microbial biotechnology and bioengineering, Chap. 19, ed. J. S. Singh (Amsterdam: Elsevier), 283–294.
Bourtzis, K., Lees, R., Hendrichs, J., and Vreysen, M. (2016). More than one rabbit out of the hat: radiation, transgenic and symbiont-based approaches for sustainable management of mosquito and tsetse fly populations. Acta Trop. 157, 115–130. doi: 10.1016/j.actatropica.2016.01.009
Brazil, G., Kenefick, L., Callanan, M., Haro, A., De lorenzo, V., Dowling, D., et al. (1995). Construction of a rhizosphere Pseudomonad with potential to degrade polychlorinated biphenyls and detection of bph gene expression in the rhizosphere. Appl. Environ. Microbiol. 61, 1946–1952. doi: 10.1128/aem.61.5.1946-1952.1995
Buah-Kwofie, A., and Humphries, M. (2021). Organochlorine pesticide accumulation in fish and catchment sediments of Lake St Lucia: risks for Africa’s largest estuary. Chemosphere 274:129712. doi: 10.1016/j.chemosphere.2021.129712
Buckingham, S., Matsuda, K., Hosie, A., Baylis, H., Squire, M., Lansdell, S., et al. (1996). Wild-type and insecticide-resistant homo-oligomeric GABA Receptors of Drosophila melanogaster stably expressed in a Drosophila cell line. Neuropharmacology 35, 1393–1401. doi: 10.1016/s0028-3908(96)00087-1
Chen, S., Dong, Y., Chang, C., Deng, Y., Zhang, X., Zhong, G., et al. (2013). Characterization of a novel cyfluthrin-degrading bacterial strain Brevibacterium aureum and its biochemical degradation pathway. Bioresour. Technol. 132, 16–23. doi: 10.1016/j.biortech.2013.01.002
Chen, S., Lai, K., Li, Y., Hu, M., Zhang, Y., and Zeng, Y. (2011). Biodegradation of deltamethrin and its hydrolysis product 3-phenoxybenzaldehyde by a newly isolated Streptomyces aureus strain HP-S-01. Appl. Microbiol. Biotechnol. 90, 1471–1483. doi: 10.1007/s00253-011-3136-3
Chen, S., Luo, J., Hu, M., Geng, P., and Zhang, Y. (2012). Microbial detoxification of bifenthrin by a novel yeast and its potential for contaminated soils treatment. PLoS One 7:e30862. doi: 10.1371/journal.pone.0030862
Chiu, T., Yen, J., Hsieh, Y., and Wang, Y. (2005). Reductive transformation of dieldrin under anaerobic sediment culture. Chemosphere 60, 1182–1189. doi: 10.1016/j.chemosphere.2005.02.018
Cycoń, M., Mrozik, A., and Piotrowska-Seget, Z. (2017). Bioaugmentation as a strategy for the remediation of pesticide-polluted soil: a review. Chemosphere 172, 52–71. doi: 10.1016/j.chemosphere.2016.12.129
Daborn, P., McCart, C., Woods, D., and Ffrench-Constant, R. (2004). Detection of insecticide resistance-associated mutations in cat flea Rdl by TaqMan-allele specific amplification. Pestic. Biochem. Phys. 79, 25–30.
Del Villar, S., and Jones, A. (2018). Cloning and functional characterisation of the duplicated Rdl Subunits from the Pea Aphid, Acyrthosiphon pisum. Int. J. Mol. Sci. 19:2235. doi: 10.3390/ijms19082235
Dharmarathne, N., Mackie, J., Kennedy, E., and Stockenhuber, M. (2019). Thermal oxidation of dieldrin and concomitant formation of toxic products including polychlorinated dibenzo-p-dioxin and dibenzofuran (PCDD/F). Chemosphere 225, 209–216. doi: 10.1016/j.chemosphere.2019.03.010
Feng, Y., Huang, Y., Zhan, H., Bhatt, P., and Chen, S. (2020). An overview of strobilurin fungicide degradation:current status and future perspective. Front. Microbiol. 11:389. doi: 10.3389/fmicb.2020.00389
Ffrench-Constant, R., Anthony, N., Aronstein, K., Rocheleau, T., and Stilwell, G. (2000). Cyclodiene insecticide resistance: from molecular to population genetics. Annu. Rev. Entomol. 45, 449–466.
Ffrench-Constant, R., Rochelean, T., Steichen, J., and Chalmers, A. (1993). A point mutation in a Drosophila GABA receptor confers insecticide resistance. Nature 363, 449–451. doi: 10.1038/363449a0
Fragoeiro, S., and Magan, N. (2005). Enzymatic activity, osmotic stress and degradation of pesticide mixtures in soil extract liquid broth inoculated with Phanerochaete chrysosporium and Trametes versicolor. Environ. Microbiol. 7, 348–355. doi: 10.1111/j.1462-2920.2005.00699.x
Gao, B., Jia, L., Xu, L., and Xie, J. (2008). Aerobic degradation of dieldrin by Enterobacter sp. LY402. J. Biotechnol. 136:698.
Gao, J., Kozaki, T., Leichter, C., Rinkevich, F., Shono, T., and Scott, J. (2007). The A302S mutation in Rdl that confers resistance to cyclodienes and limited cross-resistance to fipronil is undetectable in field populations of house flies from the USA. Pestic. Biochem. Phys. 88, 66–70.
Gautam, S., Sood, N., Gupta, K., Joshi, C., Gill, K., Kaur, R., et al. (2020). Bioaccumulation of pesticide contaminants in tissue matrices of dogs suffering from malignant canine mammary tumors in Punjab, India. Heliyon 6:e05274. doi: 10.1016/j.heliyon.2020.e05274
Gezer, A., Kochmanski, J., VanOeveren, S., Cole-Strauss, A., Kemp, C., Patterson, J., et al. (2020). Developmental exposure to the organochlorine pesticide dieldrin causes male-specific exacerbation of α-synuclein-preformed fibril-induced toxicity and motor deficits. Neurobiol. Dis. 141:104947. doi: 10.1016/j.nbd.2020.104947
Gielnik, A., Pechaud, Y., Huguenot, D., Cebron, A., Esposito, G., and van Hullebusch, E. (2021). Functional potential of sewage sludge digestate microbes to degrade aliphatic hydrocarbons during bioremediation of a petroleum hydrocarbons contaminated soil. J. Environ. Manag. 280:111648. doi: 10.1016/j.jenvman.2020.111648
Gupta, A., Patel, A., Gupta, D., Singh, G., and Mishra, V. (2020). “Rhizospheric remediation of organic pollutants from the soil; a green and sustainable technology for soil clean up,” in Abatement of Environmental Pollutants, Chap. 13, eds P. Singh, A. Kumar, and A. Borthakur (Amsterdam: Elsevier), 263–286.
Hamilton, H., Morgan, D., and Simmons, A. (1978). A pesticide (dieldrin)-induced immunohemolytic anemia. Environ. Res. 17, 155–164. doi: 10.1016/0013-9351(78)90018-x
Hidayati, N., Asia, L., Khabouchi, I., Torre, F., Widowati, I., Sabdono, A., et al. (2021). Ecological risk assessment of persistent organic pollutants (POPs) in surface sediments from aquaculture system. Chemosphere 263:128372. doi: 10.1016/j.chemosphere.2020.128372
Hou, L., Ji, D., Dong, W., Yuan, L., Zhang, F., Li, Y., et al. (2020). The synergistic action of electro-fenton and white-rot fungi in the degradation of lignin. Front. Bioeng. Biotechnol. 8:99. doi: 10.3389/fbioe.2020.00099
Hua, Q., Adamovsky, O., Vespalcova, H., Boyda, J., Schmidt, J., Kozuch, M., et al. (2021). Microbiome analysis and predicted relative metabolomic turnover suggest bacterial heme and selenium metabolism are altered in the gastrointestinal system of zebrafish (Danio rerio) exposed to the organochlorine dieldrin. Environ. Pollut. 268:115715. doi: 10.1016/j.envpol.2020.115715
Huang, Y., Lin, Z., Zhang, W., Pang, S., Bhatt, P., Rene, E. R., et al. (2020). New insights into the microbial degradation of D-cyphenothrin in contaminated water/soil environments. Microorganisms 8:473. doi: 10.3390/microorganisms8040473
Huang, Y., Zhang, W., Pang, S., Chen, J., Bhatt, P., Mishra, S., et al. (2021). Insights into the microbial degradation and catalytic mechanisms of chlorpyrifos. Environ. Res. 194:110660. doi: 10.1016/j.envres.2020.110660
Kamei, I., Takagi, K., and Kondo, R. (2010). Bioconversion of dieldrin by wood-rotting fungi and metabolite detection. Pest Manag. Sci. 66, 888–891. doi: 10.1002/ps.1958
Kanthasamy, A., Kitazawa, M., Kanthasamy, A., and Anantharam, V. (2005). Dieldrin-induced neurotoxicity: relevance to parkinson’s disease pathogenesis. NeuroToxicology 26, 701–719. doi: 10.1016/j.neuro.2004.07.010
Kanthasamy, A., Luo, J., Rokad, D., and Charli, A. (2020). “Disruption of intracellular signaling,” in An Introduction to Interdisciplinary Toxicology, Chap. 7, eds C. N. Pope and J. Liu (Cambridge, Massachusetts: Academic Press), 81–96.
Kataoka, R., Takagi, K., Kamei, I., Kiyota, H., and Sato, Y. (2010). Biodegradation of dieldrin by a soil fungus isolated from a soil with annual endosulfan applications. Environ. Sci. Technol. 44, 6343–6349. doi: 10.1021/es1000227
Krohn, C., Jin, J., Wood, J., Hayden, H., Kitching, M., Ryan, J., et al. (2021). Highly decomposed organic carbon mediates the assembly of soil communities with traits for the biodegradation of chlorinated pollutants. J. Hazard. Mater. 404:124077. doi: 10.1016/j.jhazmat.2020.124077
Lin, Z., Pang, S., Zhang, W., Mishra, S., Bhatt, P., and Chen, S. (2020). Degradation of acephate and its intermediate methamidophos: mechanisms and biochemical pathways. Front. Microbiol. 11:2045. doi: 10.3389/fmicb.2020.02045
Lin, Z., Pang, S., Zhou, Z., Wu, X., Bhatt, P., and Chen, S. (2021). Current insights into the microbial degradation for butachlor: strains, metabolic pathways, and molecular mechanisms. Appl. Microbiol. Biotechnol. 105, 4369–4381. doi: 10.1007/s00253-021-11346-3
Lin, Z., Pang, S., Zhou, Z., Wu, X., Li, J., Huang, Y., et al. (2022). Novel pathway of acephate degradation by the microbial consortium ZQ01 and its potential for environmental bioremediation. J. Hazard. Mater. 426:127841. doi: 10.1016/j.jhazmat.2021.127841
Luzardo, O., Goethals, M., Zumbado, M., Álvarez-León, E., Cabrera, F., Serra-Majem, L., et al. (2006). Increasing serum levels of non-DDT-derivative organochlorine pesticides in the younger population of the Canary Islands (Spain). Sci. Total Environ. 367, 129–138. doi: 10.1016/j.scitotenv.2006.01.027
Magan, N., Gouma, S., Fragoeiro, S., Shuaib, M. E., and Bastos, A. C. (2022). “Bacterial and fungal bioremediation strategies,” in Microbial Biodegradation and Bioremediation (Second Edition), eds S. Das and H. R. Dash (Amsterdam: Elsevier), 193–212.
Maia, M., Sousa, S., Correia-Sa, M., Delerue-Matos, C., Calhau, C., and Domingues, V. (2020). Organochlorine pesticides, brominated flame retardants, synthetic musks and polycyclic aromatic hydrocarbons in shrimps. An overview of occurrence and its implication on human exposure. Heliyon 6:e04870. doi: 10.1016/j.heliyon.2020.e04870
Matsumoto, E., Kawanaka, Y., Yun, S., and Oyaizu, H. (2008). Isolation of dieldrin- and endrin-degrading bacteria using 1,2-epoxycyclohexane as a structural analog of both compounds. Appl. Microbiol. Biotechnol. 80, 1095–1103. doi: 10.1007/s00253-008-1670-4
Matsumura, F., and Bous, G. (1967). Dieldrin, degradation by soil microorganism. Science 156, 959–961. doi: 10.1126/science.156.3777.959
Maule, A., Plyte, S., and Quirk, A. (1987). Dehalogenation of organochlorine insecticides by mixed anaerobic microbial populations. Pestic. Biochem. Phys. 27, 229–236.
Meyer-Cifuentes, I., Werner, J., Jehmlich, N., Will, S., Neumann-Schaal, M., and Ozturk, B. (2020). Synergistic biodegradation of aromatic-aliphatic copolyester plastic by a marine microbial consortium. Nat. Commun. 11:5790. doi: 10.1038/s41467-020-19583-2
Miao, Y., Heintz, M., Bell, C., Johnson, N., Polasko, A., Favero, D., et al. (2021). Profiling microbial community structures and functions in bioremediation strategies for treating 1,4-dioxane-contaminated groundwater. J. Hazard. Mater. 408:124457. doi: 10.1016/j.jhazmat.2020.124457
Mishra, S., Huang, Y., Li, J., Wu, X., Zhou, Z., Lei, Q., et al. (2022). Biofilm-mediated bioremediation is a powerful tool for the removal of environmental pollutants. Chemosphere 294:133609. doi: 10.1016/j.chemosphere.2022.133609
Mishra, S., Pang, S., Zhang, W., Lin, Z., Bhatt, P., and Chen, S. (2021). Insights into the microbial degradation and biochemical mechanism of carbamates. Chemosphere 279:130500.
Mwevura, H., Kylin, H., Vogt, T., and Bouwman, H. (2021). Dynamics of organochlorine and organophosphate pesticide residues in soil, water, and sediment from the Rufiji River Delta, Tanzania. Reg. Stud. Mar. Sci. 41:101607.
Nakao, T. (2017). Mechanisms of resistance to insecticides targeting Rdl GABA receptors in planthoppers. Neurotoxicology 60, 293–298. doi: 10.1016/j.neuro.2016.03.009
Nojima, K., and Isogami, C. (1993). Studies on photochemical reactions of air pollutants. XI. Photochemical epoxidation of aldrin with various α-diketones in air. Chemosphere 26, 921–928.
Ogbeide, O., Uhunamure, G., Okundaye, F., and Ejeomo, C. (2019). First report on probabilistic risk assessment of pesticide residues in a riverine ecosystem in South-South Nigeria. Chemosphere 231, 546–561. doi: 10.1016/j.chemosphere.2019.05.105
Pang, S., Lin, Z., Zhang, Y., Zhang, W., Alansary, N., Mishra, S., et al. (2020b). Insights into the toxicity and degradation mechanisms of imidacloprid via physicochemical and microbial approaches. Toxics 8:65. doi: 10.3390/toxics8030065
Pang, S., Lin, Z., Zhang, W., Mishra, S., Bhatt, P., and Chen, S. (2020a). Insights into the microbial degradation and biochemical mechanisms of neonicotinoids. Front. Microbiol. 11:868. doi: 10.3389/fmicb.2020.00868
Papadakis, E., Vryzas, Z., Kotopoulou, A., Kintzikoglou, K., Makris, K., and Papadopoulou-Mourkidou, E. (2015). A pesticide monitoring survey in rivers and lakes of northern Greece and its human and ecotoxicological risk assessment. Ecotoxicol. Environ. Saf. 116, 1–9. doi: 10.1016/j.ecoenv.2015.02.033
Purnomo, A., Nawfa, R., Martak, F., Shimizu, K., and Kamei, I. (2017). Biodegradation of aldrin and dieldrin by the white-rot fungus Pleurotus ostreatus. Curr. Microbiol. 74, 320–324.
Sakakibara, F., Takagi, K., Kataoka, R., Kiyota, H., Sato, Y., and Okada, S. (2011). Isolation and identification of dieldrin-degrading Pseudonocardia sp. strain KSF27 using a soil-charcoal perfusion method with aldrin trans-diol as a structural analog of dieldrin. Biochem. Biophys. Res. Commun. 411, 76–81. doi: 10.1016/j.bbrc.2011.06.096
Sakan, S., Ostojić, B., and Ðorđević, D. (2017). Persistent organic pollutants (POPs) in sediments from river and artificial lakes in Serbia. J. Geochem. Explor. 180, 91–100.
Sakuragui, M., Paulino, M., Pereira, C., Carvalho, C., Sadauskas-Henrique, H., and Fernandes, M. (2013). Integrated use of antioxidant enzymes and oxidative damage in two fish species to assess pollution in man-made hydroelectric reservoirs. Environ. Pollut. 178, 41–51. doi: 10.1016/j.envpol.2013.02.032
Sarty, K., Cowie, A., and Martyniuk, C. (2017). The legacy pesticide dieldrin acts as a teratogen and alters the expression of dopamine transporter and dopamine receptor 2a in zebrafish (Danio rerio) embryos. Comp. Biochem. Physiol. C Toxicol. Pharmacol. 194, 37–47. doi: 10.1016/j.cbpc.2017.01.010
Shah, H., Sharma, T., and Banerjee, B. (2020). Organochlorine pesticides induce inflammation, ROS production, and DNA damage in human epithelial ovary cells: an in vitro study. Chemosphere 246:125691. doi: 10.1016/j.chemosphere.2019.125691
Sheng, C., Jia, Z., Ozoe, Y., Huang, Q., Han, Z., and Zhao, C. (2018). Molecular cloning, spatiotemporal and functional expression of GABA receptor subunits Rdl1 and Rdl2 of the rice stem borer Chilo suppressalis. Insect Biochem. Mol. Biol. 94, 18–27. doi: 10.1016/j.ibmb.2018.01.003
Siddiqui, S., Brown, D., Accardi, M., and Forrester, S. (2012). Hco-LGC-38 is novel nematode cys-loop GABA receptor subunit. Mol. Biochem. Parasitol. 185, 137–144. doi: 10.1016/j.molbiopara.2012.08.004
Singare, P. (2016). Distribution and risk assessment of suspected endocrine-disrupting pesticides in creek water of Mumbai, India. Mar. Pollut. Bull. 102, 72–83. doi: 10.1016/j.marpolbul.2015.11.055
Tham, T., Anh, H., Phuong, B., Trinh, L., Thuy, N., Yen, N., et al. (2020). Contamination status and temporal trends of persistent toxic substances in sediment cores from coastal areas of central Vietnam. Mar. Pollut. Bull. 156:111222. doi: 10.1016/j.marpolbul.2020.111222
Tongo, I., and Ezemonye, L. (2015). Human health risks associated with residual pesticide levels in edible tissues of slaughtered cattle in Benin City, Southern Nigeria. Toxicol. Rep. 2, 1117–1135. doi: 10.1016/j.toxrep.2015.07.008
Tsai, C., and Treadwell, H. (2019). Analysis of trends and variability of toxic concentrations in the Niagara River using the Hilbert-Huang transform method. Ecol. Inform. 51, 129–150.
Umulisa, V., Kalisa, D., Skutlarek, D., and Reichert, B. (2020). First evaluation of DDT (dichlorodiphenyltrichloroethane) residues and other persistence organic pollutants in soils of Rwanda: nyabarongo urban versus rural wetlands. Ecotoxicol. Environ. Saf. 197:110574. doi: 10.1016/j.ecoenv.2020.110574
Wang, J., Zhao, X., Yan, R., Wu, S., Wu, Y., and Yang, Y. (2020). Reverse genetics reveals contrary effects of two Rdl-homologous GABA receptors of Helicoverpa armigera on the toxicity of cyclodiene insecticides. Pestic. Biochem. Phys. 170:104699. doi: 10.1016/j.pestbp.2020.104699
Watanabe, K., and Yoshikawa, H. (2008). Enrichment and isolation of anaerobic microorganisms concerned with reductive degradation of hexachlorobenzene from soils. J. Pestic. Sci. 33, 166–170.
Xiao, P., and Kondo, R. (2020). Potency of Phlebia species of white rot fungi for the aerobic degradation, transformation and mineralization of lindane. J. Microbiol. 58, 395–404. doi: 10.1007/s12275-020-9492-x
Xiao, P., Mori, T., Kamei, I., Kiyota, H., Takagi, K., and Kondo, R. (2011a). Novel metabolic pathways of organochlorine pesticides dieldrin and aldrin by the white rot fungi of the genus Phlebia. Chemosphere 85, 218–224. doi: 10.1016/j.chemosphere.2011.06.028
Xiao, P., Mori, T., Kamei, I., and Kondo, R. (2011b). A novel metabolic pathway for biodegradation of DDT by the white rot fungi, Phlebia lindtneri and Phlebia brevispora. Biodegradation 22, 859–867. doi: 10.1007/s10532-010-9443-z
Yu, L. L., Cui, Y. J., Lang, G. J., Zhang, M. Y., and Zhang, C. X. (2010). The ionotropic & gamma;-aminobutyric acid receptor gene family of the silkworm, Bombyx Mori. Genome 53, 688–697.
Zhang, W., Lin, Z., Pang, S., Bhatt, P., and Chen, S. (2020). Insights into the biodegradation of lindane (gamma-hexachlorocyclohexane) using a microbial system. Front. Microbiol. 11:522. doi: 10.3389/fmicb.2020.00522
Zhang, W., Pang, S., Lin, Z., Mishra, S., Bhatt, P., and Chen, S. (2021). Biotransformation of perfluoroalkyl acid precursors from various environmental systems: advances and perspectives. Environ. Pollut. 272:115908. doi: 10.1016/j.envpol.2020.115908
Zhao, F., Zhang, D., Xu, C., Liu, J., and Shen, C. (2020). The enhanced degradation and detoxification of chlortetracycline by Chlamydomonas reinhardtii. Ecotoxicol. Environ. Saf. 196:110552. doi: 10.1016/j.ecoenv.2020.110552
Keywords: aldrin, dieldrin, environmental fate, toxicity, microbial degradation, degradation mechanisms
Citation: Pang S, Lin Z, Li J, Zhang Y, Mishra S, Bhatt P and Chen S (2022) Microbial Degradation of Aldrin and Dieldrin: Mechanisms and Biochemical Pathways. Front. Microbiol. 13:713375. doi: 10.3389/fmicb.2022.713375
Received: 22 May 2021; Accepted: 24 February 2022;
Published: 29 March 2022.
Edited by:
Angela F. Jozala, University of Sorocaba, BrazilReviewed by:
Ajar Nath Yadav, Eternal University, IndiaMuhammad Rizwan Ul Haq, King Faisal University, Saudi Arabia
Copyright © 2022 Pang, Lin, Li, Zhang, Mishra, Bhatt and Chen. This is an open-access article distributed under the terms of the Creative Commons Attribution License (CC BY). The use, distribution or reproduction in other forums is permitted, provided the original author(s) and the copyright owner(s) are credited and that the original publication in this journal is cited, in accordance with accepted academic practice. No use, distribution or reproduction is permitted which does not comply with these terms.
*Correspondence: Shaohua Chen, c2hjaGVuQHNjYXUuZWR1LmNu