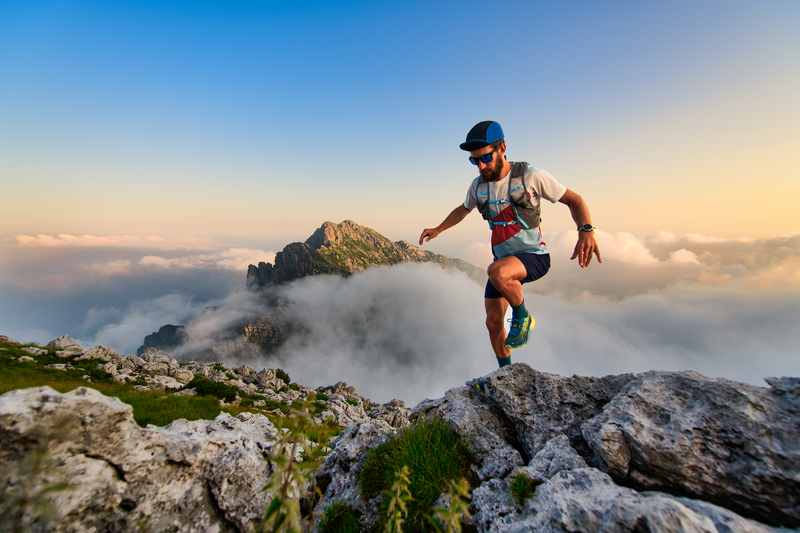
95% of researchers rate our articles as excellent or good
Learn more about the work of our research integrity team to safeguard the quality of each article we publish.
Find out more
ORIGINAL RESEARCH article
Front. Microbiol. , 06 January 2023
Sec. Aquatic Microbiology
Volume 13 - 2022 | https://doi.org/10.3389/fmicb.2022.1113388
This article is part of the Research Topic Insights in Aquatic Microbiology: 2022 View all 7 articles
The projected ocean acidification (OA) associated with increasing atmospheric CO2 alters seawater chemistry and hence the bio-toxicity of metal ions. However, it is still unclear how OA might affect the long-term resilience of globally important marine microalgae to anthropogenic metal stress. To explore the effect of increasing pCO2 on copper metabolism in the diatom Thalassiosira pseudonana (CCMP 1335), we employed an integrated eco-physiological, analytical chemistry, and transcriptomic approach to clarify the effect of increasing pCO2 on copper metabolism of Thalassiosira pseudonana across different temporal (short-term vs. long-term) and spatial (indoor laboratory experiments vs. outdoor mesocosms experiments) scales. We found that increasing pCO2 (1,000 and 2,000 μatm) promoted growth and photosynthesis, but decreased copper accumulation and alleviated its bio-toxicity to T. pseudonana. Transcriptomics results indicated that T. pseudonana altered the copper detoxification strategy under OA by decreasing copper uptake and enhancing copper-thiol complexation and copper efflux. Biochemical analysis further showed that the activities of the antioxidant enzymes glutathione peroxidase (GPX), catalase (CAT), and phytochelatin synthetase (PCS) were enhanced to mitigate oxidative damage of copper stress under elevated CO2. Our results provide a basis for a better understanding of the bioremediation capacity of marine primary producers, which may have profound effect on the security of seafood quality and marine ecosystem sustainability under further climate change.
Since the early 1900s, increasing atmospheric CO2 and associated ocean acidification (OA) have increased hydrogen ions (H+) concentration by 30% and dropped pH by 0.1 units in ocean surface waters (Vargas et al., 2017; Osborne et al., 2020). Unprecedented shifts in ocean chemistry are predicted to occur in the future, doubling the partial pressure of carbon dioxide and decreasing pH by 0.3 units by the end of the century (Shi et al., 2010; Gattuso et al., 2015). OA does not only alter the seawater pH but also changes the seawater carbonate system, including increased bicarbonate (HCO3−) and reduced carbonate (CO32−) concentrations (Feely et al., 2004; Waldbusser and Salisbury, 2014). The shifts in seawater chemistry may alter the chemical behavior of other elements such as metals, modifying their bioavailability and thus toxicity (Millero et al., 2009; Roberts et al., 2013; Campbell et al., 2014; Bautista-Chamizo et al., 2016; Stockdale et al., 2016). Bautista-Chamizo et al. (2016) demonstrated that lower pH increased toxicity of zinc by altering the bioavailability to the marine microalgae Pleurochrysis roscoffensis. de Orte et al. (2014) also reported that OA enhanced the release of metals such as aluminum, iron, zinc, cobalt, lead and copper from sediments to the water column and thus increased their toxicity to Phaeodactylum tricornutum. In contrast, Shi et al. (2010) found that OA reduced the bioavailability of dissolved Fe and decreased the Fe uptake rate of diatoms and coccolithophores. Furthermore, the interactions between elevated CO2 and metals are species-specific, and dependent on species developmental stage, metal biochemistry and the degree of acidification (Ivanina and Sokolova, 2015). Previous studies investigating the effects of OA on phytoplankton have been predominantly based on short-term studies, and long-term investigations into the responses of marine phytoplankton to OA are limited. However, marine microbes have enormous potential for rapid adaptation to environmental changes, due to their large population sizes and short generation times (Thoms et al., 2012; Reusch and Boyd, 2013; Schlüter et al., 2014). Therefore, it is important to investigate responses of globally important microbes under evolutionary relevant timescales to obtain more accurate estimates of their resilience to environmental changes.
Copper (Cu) is an essential micronutrient for the metabolism of plants and algae, that is required for the functioning of proteins involved in photosynthesis (plastocyanin) and respiration (cytochrome c) and acts as a redox cofactor in many enzymes such as cytochrome c oxidase and copper/zinc superoxide dismutase (Andresen et al., 2018; Scheiber et al., 2019). However, the redox properties that make Cu an essential element also contribute to its inherent toxicity in excess concentrations and can induce oxidative stress and damage to macromolecules (Navarrete et al., 2019). To detoxify copper, algae and plants have evolved specific homeostatic mechanisms. One such detoxifying mechanism is the synthesis of metal-binding ligands, such as phytochelatins and metallothioneins and the subsequent distribution and compartmentalization of Cu within different cellular compartments. The acquisition of Cu from the environment depends on membrane transporter proteins such as the CTR-like Cu transporter, ZRT/IRT-like protein (ZIP), and cation diffusion facilitator (CDF). However, Cu needs to be reduced from Cu2+ to Cu+ by a plasma membrane ferric reductase (FRE) before import into the cell, which is expected to be the rate limiting process for Cu2+ transport in Thalassiosira pseudonana. Intracellular Cu+ may also be removed from the cell via Cu-transporting P1B-type ATPases (CTP) to reduce its toxicity (Guo et al., 2015; Huang et al., 2016; Liu et al., 2019; Zúñiga et al., 2020).
In line with OA, metal pollution is increasing in coastal environments due to increasing anthropogenic activities (for example, industrial, agricultural and domestic pollution) and this may affect the growth and species composition at the base of aquatic food webs (Davis et al., 2006; Miazek et al., 2015; Leung et al., 2017; Yung et al., 2017). Metals that form strong complexes with chloride (Cu+, Cd2+, and Hg2+) are mainly found in their free form and are not strongly influenced by changes in pH, while metals that form strong complexes with hydroxide (Al3+, Ga3+, In3+, and Be2+) or carbonate (Cu2+) will undergo significant changes in speciation as the pH of seawater decreases. It is expected that from 2,000 (pH 8.1) to 2,250 (pH 7.4) the fraction of Cu in the forms of CuCO3 and CuOH+ will decrease by 9 and 1%, respectively, while the toxic free ion concentration of copper (Cu2+) will increase by 24%, potentially increasing its bio-toxicity to marine biota (Millero et al., 2009).
Diatoms, as single-celled eukaryotes capable of photosynthesis, are distributed in marine and freshwater systems around the world (Armbrust et al., 2004), and are the most diverse algal group in the world, with at least 100,000 species (Falciatore et al., 2020). Diatoms contribute up to approximately 40% of oceanic primary productivity and are a critical component of coastal food webs, functionally sequestering carbon and nutrients and thereby playing a significant role in earth’s carbon cycle and the global biogeochemical cycles of nitrogen, phosphorus, and silicon (Armbrust, 2009; Wu et al., 2014; Benoiston et al., 2017). Early studies have indicated that diatoms respond differently to different global ocean changes. For example, it has been found that elevated CO2 enhances the growth rates of larger diatoms (Wu et al., 2014), and copper stress impacts diatoms at multiple cellular levels, including the morphological, behavioral, and physiological levels (Park et al., 2020). By conserving and utilizing energy in the cellular processes, diatoms have adopted unique adaptive strategies to respond to ocean warming and acidification (O'Donnell et al., 2018; Thangaraj and Sun, 2020; Zhong et al., 2021). Recent research has shown that OA reduced the toxicity of cadmium in Phaeodactylum tricornutum (Zhang et al., 2020). However, the metabolic pathways of copper in diatoms under future OA is unknown.
Here, we elucidated the effect of increasing CO2 on copper metabolism in the model diatom of T. pseudonana. Firstly, we characterized the effect of increasing CO2 on copper toxicity and its accumulation in T. pseudonana during a long-term selection period of 720 days. Secondly, to determine the adaptive capacity of T. pseudonana to increasing CO2, the long-term selected lines were grown under ambient or high CO2 with or without copper stress. Finally, we conducted the transcriptional and chemical analysis of long-term selected T. pseudonana under ambient and elevated pCO2 levels with or without copper exposure.
The diatom T. pseudonana (CCMP 1335) was obtained from the Yellow Sea Fisheries Research Institute Microalgae Culture Center of the National Marine Genetic Resource Center (Xu et al., 2022)1. In the laboratory, cells were grown in semi-continuous cultures in sterile seawater enriched with modified f/2 medium containing 100 μM N, 6 μM P and 100 μM Si and maintained at 20 ± 1°C under an irradiance of 120 μmol photons m−2 s−1 and a 12 h/12 h light–dark cycle (light on at 8:00 am and off at 8:00 pm).
The growth inhibition of T. pseudonana under different concentrations of copper (Cu), was quantified before the start of the experiment. T. pseudonana was grown in 400 ml cultures at cell densities of 8 × 104 cells ml−1 and supplemented with a series of copper (prepared with CuSO4·5H2O) ranging from 0 μmol L−1 (using the background concentration in natural seawater, < 0.03 μmol L−1) to 35 μmol L−1 for 96 h (during exponential phase). At the beginning and end of the experiment (96 h), 0.5 ml of T. pseudonana culture was collected and preserved in Lugol’s solution to estimate microalgal growth by directly counting cell numbers using a hemocytometer and optical microscope (Nikon, Tokyo, Japan). The growth rate (μ, day−1) was calculated using Equation (1):
where N1 and N0 represent cell concentrations at t1 and t0, respectively. The percentage of growth inhibition was calculated with reference to the control (no additional copper) using Equation (2):
where μ2 and μ1 represent the growth rate at a certain copper concentration and in the absence of copper, respectively. The concentrations of copper causing a 50% reduction in growth (IC50, 96 h, 20 μmol L−1, Supplementary Figure S1) was determined by linear interpolation. Although Cu was presented in the base medium, the group without extra copper addition was set as the control group. In the copper toxicity experiment, the compound EDTA (ethylenediaminetetraacetic acid, disodium salt, dehydrate; Na2EDTA·2H2O) and FeCl3·6H2O as well as other micronutrients in f/2 medium were not added into sterile seawater medium, due to the chelating properties of EDTA, which could decrease copper toxicity.
Four experiments were set up in this study (Supplementary Figure S2). Firstly, to assess the effect of elevated pCO2 on the physiological performance of T. pseudonana, a selection experiment was set up in laboratory, where algae were cultured under ambient and elevated pCO2 for 720 days. Secondly, to assess the evolutionary response of T. pseudonana to elevated pCO2, shift experiments were set up in the laboratory, where the selected lineages at ambient and elevated pCO2 were transferred into five different concentrations of pCO2. Thirdly, to compare the differences in response between the indoor and outdoor experiments, an outdoor culture system was set up, where T. pseudonana was cultured under ambient and elevated pCO2 using natural temperature and light. Fourthly, we conducted transcriptome sequencing of T. pseudonana using the long-term selected population (after 720 days’ selection in laboratory), to gain a mechanistic understanding of how elevated pCO2 influences copper metabolism of T. pseudonana. For all four experiments, algae were acclimated under ambient or elevated pCO2 for more than 30 generations, and then exposed to Cu without acclimation. In all experiments, the respective pCO2 levels of 420, 1,000, and 2,000 μatm were established by bubbling the liquid medium with air or air/CO2 premixed gas using a CO2 chamber (HP1000G-D, China, for the laboratory experiment) or CO2 Enricher (CE-100B; Wuhan Ruihua Instrument & 25 Equipment Ltd., for the outdoor culture system).
To study the effect of elevated CO2 on the physiological performance of T. pseudonana, three different pCO2 levels (420, 1,000, and 2,000 μatm; mimicking current and future pCO2 rises up to the year 2,300 under IPCC scenario RCP 8.5) were set up using nutrient-replete f/2 medium. T. pseudonana (acclimated at 20 ± 1°C under an irradiance of 120 μmol photons m−2 s−1) was transferred during the exponential phase of growth to an inoculum of the relevant CO2-modified f/2 medium at identical densities of 8 × 104 cells ml−1 and the cultures diluted every 4–6 days to maintain a stable carbonate system. In the selection experiment, algal cells were grown under semi-continuous culture conditions under the above pCO2 levels for 720 days. Three biological replicates were set up using 1,000 ml flasks with 800 ml medium for each pCO2 treatment. To study whether long-term selection under elevated pCO2 has an impact on how T. pseudonana deal with exposure to copper stress, cells from each selected sample were transferred at a density of 8 × 104 cells ml−1 into CO2-modified f/2 medium supplemented with or without copper for another 96 h at time intervals of 60 days (Supplementary Figure S2). Three concentrations of Cu exposure including a control (without additional Cu), low Cu (1 μmol L−1, as usually occurs in polluted coastal areas), and high Cu (20 μmol L−1, according to the IC50, 96 h) were set up in the copper exposure experiment.
At the end of each batch-culture experiment, a 10 ml aliquot from each replicate was collected and centrifuged at 10,000 g for 10 min at room temperature. The algal precipitate was rinsed twice with Milli-Q water and an ice-cold phosphate buffer to remove extracellular copper, and then harvested and stored at −20°C to estimate intracellular copper content (Angel et al., 2017).
During the selection experiment the pH in each culture medium was measured before and after each dilution with a pH meter (Orion ROSS, Fisher Scientific Instruments), which was calibrated before use (NBS, National Bureau of Standards; variation range ± 0.05). Temperature, salinity, and total alkalinity (TA) were also measured periodically during the selection experiment at time intervals of 60 days. The average value was used to calculate carbonate system parameters using the CO2SYS Package (Pierrot et al., 2006; Supplementary Table S1).
Following 720-day selection in the respective CO2 levels (corresponding to 833 generations, 920 generations and 887 generations in ambient pCO2 of 420 μatm and high pCO2 of 1,000 and 2,000 μatm, respectively), cells were transferred to another five increasing pCO2 concentrations, ranging from 420 to 2,000 μatm and maintained in semi-continuous batch culture and transferred to fresh media every 4–6 days (Supplementary Figure S2). After acclimation for 30 days, each lineage under the respective pCO2 was cultured with or without added exposure to Cu for another 96 h. The pH, temperature, salinity, and total alkalinity (TA) were measured before and after each dilution and the average was used to calculate the parameters of the carbonate system (Supplementary Table S2). Cells without Cu exposure were set as the control. In order to test acute Cu toxicity, high Cu (20 μmol L−1, according to the IC50, 96 h) was used in the Cu exposure experiment. Cell growth and intracellular copper accumulation were determined after 96 h, using the methods described above. The direct response to the selection (S) was measured by comparing the growth rate (or intracellular copper concentration) of elevated pCO2 (1,000, 2,000 μatm) selected cells to that of cells from the ambient pCO2 culture using Equation (3) (Brennan et al., 2017)
where E is the growth rate (or intracellular copper concentration) of elevated pCO2 selected cells, and A is the growth rate (or intracellular copper concentration) of ambient pCO2 selected cells.
To investigate the effect of increasing pCO2 on copper metabolism of T. pseudonana under more natural environmental conditions, cells selected at pCO2 values of 420, 1,000, and 2,000 μatm for 720 days in laboratory were transferred outdoors and cultured at the respective pCO2 on the sea (37°06 ‘N, 122°33 ‘E), using 10 L tanks with 8 L cultures, which were set as long-term selection treatments and deployed on the sea surface (LT; Supplementary Figure S3). Additionally, stock cells without any selection treatment in the laboratory were also transferred to outdoors and cultured under the same series of pCO2 of 420, 1,000, and 2,000 μatm, and set as short-term acute treatments and cultures also deployed on the sea surface (ST). After acclimation in filtered natural seawater under the respective pCO2 for 30 days, each lineage was cultured with or without additional Cu exposure for another 96 h. Cell growth and intracellular copper accumulation were determined after 96 h, using the methods described above. The variations of pH, temperature, salinity, and total alkalinity (TA) were measured every day, and were used to calculate the parameters of the carbonate system (Supplementary Figure S4).
To investigate the molecular responses of T. pseudonana to increasing pCO2 and copper exposure, cultures evolved under 420 and 1,000 μatm pCO2 for 720 days were transferred in triplicate to fresh media under the same CO2 conditions, with or without copper exposure (20 μmol L−1) for a further 24 h. At the end of the experiment, 250 ml of culture from each replicate were harvested, centrifuged at 4°C and frozen in liquid nitrogen and stored at −80°C for RNA extraction, transcriptional sequencing, and RT-qPCR. The primers designed for reference and target genes were designed and are listed in Supplementary Table S3. The raw sequence data reported in this paper have been deposited in the Genome Sequence Archive in BIG Data Center, Beijing Institute of Genomics, Chinese Academy of Sciences, under accession numbers CRA001653, that are publicly accessible at http://bigd.big.ac.cn/gsa. A further 100 ml of culture from each replicate was harvested, centrifuged at 4°C and frozen in liquid nitrogen and stored at −80°C for enzyme activity determination. The antioxidant enzyme activities of glutathione peroxidase (GPX), catalase (CAT) and ascorbate peroxidase (APX) were determined using commercial reagent kits (Comin Biotechnology Co., Ltd., Suzhou, China) following the manufacturer’s instructions.
The effects of elevated CO2 and Cu exposure on algal growth rate or intracellular copper concentration ([Cu]intra) over the selection period of 720 days were analyzed with a mixed effects model in R,2 using the packages lme4 and lmerTest. Algal growth rate or intracellular copper concentration ([Cu]intra) were used as the experimental variable to be explained. Replicates were used as random effects. To test the individual and combined effects of increasing pCO2 and Cu exposure on algal growth rate and intracellular copper concentration [Cu]intra across different culture duration in selection experiment, different pCO2 levels or different Cu exposure scenario or culture duration was set as one fixed effect. Different pCO2 levels, different Cu exposure scenario, and culture duration were then tested separately and each in interaction with pCO2, and the most parsimonious model was chosen for reporting based on the smallest AICc score (Akaike Information Criterion for small sample sizes); To test the algal adaptive response to increasing pCO2 in shift experiment, the assay pCO2 was set as a fixed factor and the different selection pCO2 was used as random effects; To test algal physiological responses to increasing pCO2 in outdoor culture experiment, different pCO2 levels were used as fixed factors. The different Cu exposure scenarios and selection length (short-term, STS and long-term, LTS) were used as random effects.
Elevated pCO2 significantly increased growth rates of T. pseudonana in all Cu exposure scenarios (Figure 1A; F4,88 = 4.00, p < 0.05). Under elevated pCO2 conditions without Cu, maximal growth rates were found at 1000 μatm pCO2 until month 14 (Figure 1A; F2,92 = 159.71, p < 0.001). Trajectories of algal growth rate over the course of the selection experiment differed substantially between the ambient and elevated pCO2. After acclimation for 24 months (approximately 1,000 generations), populations selected under 2,000 μatm pCO2 showed higher growth rates than populations selected at 1,000 and 420 μatm pCO2. In addition, the variation in growth rates between the ambient and elevated pCO2 reduced as the experiment proceeded (F2,100 = 56.03, p < 0.001).
Figure 1. Growth rates and intracellular copper concentration of T. pseudonana in the selection experiment under ambient (420 μatm, grey) and elevated pCO2 (1,000 μatm, blue; and 2,000 μatm, red) with three gradients copper exposure. (A) Changes in growth rate (day−1); (B) Shift in intracellular copper concentration ([Cu]intra). The symbols represent the means and error bars represent standard deviations of triplicate cultures.
In contrast to the variation in growth rate, elevated pCO2 significantly reduced copper accumulation within algal cells in all Cu exposure scenarios (Figure 1B; F = 79.632, p < 0.001). Populations selected under 1,000 and 2,000 μatm pCO2 showed opposite trends in copper accumulation across the selection periods. Over 24 months of selection, copper accumulation gradually increased in populations selected under 1,000 μatm pCO2, whereas copper accumulation gradually decreased in populations selected under 2,000 μatm pCO2, in each of the control, the low Cu or high Cu treatments.
We found evidence of adaptation to elevated CO2 conditions by measuring the direct response to selection (growth rate of evolved populations relative to that of control assayed in the same selection environment). Populations evolved under 1,000 μatm and 2,000 μatm pCO2 showed a positive response to selection in their respective selection environments, indicating that evolution under elevated pCO2 conditions increases growth rates beyond the plastic response (of the evolved control populations). While populations evolved under elevated pCO2 conditions converge on a similar evolved growth rates (Figure 1), populations evolved under the highest pCO2 conditions (2,000 μatm) evolved more than populations evolved under pCO2 1,000 μatm to arrive at the same endpoint. However, the strength of selection in each environment is similar (indicated by the initial population growth rate of evolved control populations in the same environments; Figure 2). Population growth rates generally increased with increasing pCO2 across all selected lines, including treatments without (Figure 2A; F4,38 = 6.441, p < 0.001) or with Cu exposure (Figure 2B; F4,38 = 2.924, p < 0.05). However, growth rates in elevated pCO2-selected samples decreased and showed lower levels than the ambient-selected ones, when they were shifted back to ambient pCO2 at 420 μatm or a slightly higher pCO2 at 700 μatm.
Figure 2. Growth rates and intracellular copper concentration of T. pseudonana in the shift experiment under elevated pCO2 with or without additive copper exposure. Box plots show the response to selection measured as the difference in growth rate (day−1) without (A) or with (B) copper exposure and intracellular copper concentration ([Cu]intro) without (C) or with (D) copper exposure, between populations evolved to elevated CO2 (1,000 and 2,000 μatm) and the evolved control populations (420 μatm), under the same environmental conditions. The direct response to selection is highlighted in yellow and compares the population growth rate of a population evolved in the selection environment with the plastic response of a control population to that same environment. The dashed line shows the average growth rate of the evolved control populations in each environment (indicated on x axis and panel labels). Distance from the dashed line shows the difference in responses between the evolved populations and the evolved control in the same environment.
When copper is added to the environments, simulating environmental pollution of the aquatic environment, the benefits of evolution under elevated pCO2 are reversed in all but the highest pCO2 environments (Figures 2B,D; copper accumulation is greater in selected lines than the plastic response indicated by the dashed line). Overall, copper accumulation decreased significantly with increasing pCO2 in the shift experiments under treatments both without (Figure 2C; F4,40 = 11.23, p < 0.001) and with Cu exposure (Figure 2D; F4,40 = 5.12, p < 0.001), indicating that copper accumulation may be reduced in photosynthetic algae under future pCO2 conditions.
In outdoor culture experiments, population growth rates increased under elevated pCO2 (1,000 and 2,000 μatm), in both the short-term (ST) or long-term (LT) acclimation experiments and in all copper treatments (Figures 3A,B; F1,69 = 28.40, p < 0.001). By comparison between ST and LT samples, the former showed the highest growth at 1,000 μatm pCO2, whereas the latter showed a maximum at 2,000 μatm pCO2.
Figure 3. Growth rates and intracellular copper concentration of T. pseudonana in outdoor culture experiments after short-term (ST) and long-term (LT) acclimation under different pCO2 with or without added copper. Changes in growth rate to increasing pCO2 without (A) or with (B) copper exposure; Shift in intracellular copper concentration ([Cu]intra) to increasing pCO2 without (C) or with (D) copper exposure. The values represent the means and error bars represent standard deviations of triplicate cultures.
Elevated pCO2 (1,000 and 2,000 μatm) also reduced copper accumulation across all experimental treatments, even using the natural seawater where the background Cu concentration was less than 0.03 μmol L−1 (Figures 3C,D; F1,68 = 15.18, p < 0.001). Copper accumulation in the short-and long-term mirrored the pattern found for growth rates. Under the control treatment without Cu exposure, intracellular copper concentration ([Cu]intra) in ST samples was reduced by 38.0 and 30.5% under 1,000 and 2,000 μatm pCO2 respectively, and that in LT samples was reduced by 31.7 and 46.3% under 1,000 and 2,000 μatm pCO2, respectively. Under Cu exposure, [Cu]intra in ST samples was reduced by 33.4 and 29.5% under 1,000 and 2,000 μatm, respectively, and that in LT samples was reduced by 37.0 and 43.7% under 1,000 and 2,000 μatm pCO2, respectively.
Transcriptome analysis as well as real-time quantitative polymerase chain reaction (RT-qPCR; Figure 4A; Supplementary Figure S5) revealed 32 differentially expressed genes (Supplementary Dataset 1) associated with copper metabolism among the different treatments. Although the Cu+ transporter CTR was up-regulated under elevated pCO2, the reduction reaction from Cu2+ to Cu+ by FRE was significantly down-regulated. As a result, Cu2+ uptake rate was reduced (Supplementary Figure S6). Within the cell, glutathione synthetase (GS), glutathione reductase (GR), and phytochelatin synthetase (PCS) were up-regulated to synthesize phytochelatins (PCn), which can be used to chelate with Cu+ and sequester it in the vacuole. Elevated pCO2 also resulted in down-regulation of the gene expression of Cu chaperones (COX17 and SCO1) to decrease Cu toxicity to mitochondrion. Cu-transporting P1B-type ATPases (CTP) were up-regulated under elevated pCO2 to enhance the efflux of intracellular Cu+ from the cell, this was also verified by a measured increase in Cu efflux rate under elevated pCO2 (Supplementary Figure S7). Additionally, to eliminate reactive oxygen species (ROS) induced by Cu stress under elevated pCO2, glutathione peroxidase (GPX) and catalase (CAT) were up-regulated both in gene expression and enzyme activity (Figure 4C). However, ascorbate peroxidase (APX) showed decreased activity under higher pCO2 (1,000 and 2,000 μatm), compared with ambient pCO2 (420 μatm), when exposed to copper.
Figure 4. The effect of elevated pCO2 on genes expression and enzyme activity involved in the copper metabolic pathway of T. pseudonana. (A) Heat map of representative gene expression in copper metabolic pathway of T. pseudonana under elevated pCO2 with or without copper exposure. (B) Schematic of the altered copper metabolic pathway in T. pseudonana under elevated pCO2. Arrows in red or blue represented the up-or down-regulated genes under elevated pCO2. Black arrows indicated that there were not consistent variation trends for the functionally similar genes with different ID number. (C) Activities of three antioxidant enzymes (units/mg protein), including, glutathione peroxidase (GPX), ascorbate peroxidase (APX), and catalase (CAT) under elevated pCO2 with or without copper exposure.
In this study, we provided both a quantitative and a mechanistic understanding of how diatoms might respond to heavy metal stress under future ocean acidification using both a 720-day laboratory selection experiment, outdoor culture experiments, and transcriptomic sequencing. Long-term selection experiments showed that elevated pCO2 promoted algal growth rate and reduced copper accumulation within algal cells, and thus alleviated copper stress to T. pseudonana. Shift experiments using the long-term selected cells indicated adaptive evolution potential of T. pseudonana under elevated pCO2. Biochemical and transcriptomic analysis further showed that T. pseudonana employed a specific copper detoxification strategy under elevated pCO2.
Many studies have found different responses between short-term acclimation and long-term adaptation of phytoplankton to elevated pCO2 (Schlüter et al., 2016; Li et al., 2017; Tong et al., 2018). Our findings are in line with previous studies indicating selection under elevated pCO2 promoted algal growth rates in diatom species (Wu et al., 2014; Zhu et al., 2017; Qu et al., 2018). The positive effect of elevated pCO2 may result from the reduced energy requirement of carbon concentration mechanisms (CCMs) with the saved energy being used to support carbon fixation and growth (Raven et al., 2011). Hopkinson et al. (2011) reported that doubling of ambient pCO2 reduced CCM-related energy expenditure by ~20% and decreased the total energy demand on carbon fixation by up to 6%. As the selection experiment continued, the response differences between ambient and elevated pCO2 decreased among different treatments after 24 months selection, which may be due to an effect induced by adaptation to the laboratory setting (Collins, 2016). Shift experiments further confirmed the evolutionary responses of T. pseudonana to high pCO2, in that the long-term selected samples under elevated pCO2 showed a lower growth rate than ambient selected ones when they were transplanted back into ambient pCO2 conditions. Collins and Bell (2004) found that the long-term selected cells under high pCO2 showed less efficient carbon concentration mechanisms, or a higher per-cell requirement for inorganic carbon, which could result in decreased growth rate when they were assayed under ambient pCO2.
When the algae were exposed to copper stress, elevated pCO2 significantly reduced intracellular copper accumulation and alleviated the negative effect of copper stress (Supplementary Figure S8). Changes to the seawater carbonate chemistry under elevated pCO2 did not change external copper concentration in the culture medium (Supplementary Figure S9), suggesting that reduced copper accumulation under elevated pCO2 is biologically mediated. This is supported by the study of de los Santos et al. (2019) who also found that projected OA would ameliorate copper toxicity on photosynthetic performance of Zostera noltei when pH decreased from 8.36 to 8.03. Our findings add to the growing body of evidence that adaptive evolution of the marine diatom community under projected OA would increase their resilience to harsh environments (Domingues et al., 2014; Valenzuela et al., 2018; Dong et al., 2020; Xu et al., 2022).
Previous studies have documented that elevated pCO2 does not only directly affect primary producers but also changes the distribution, speciation and bioavailability of organic and inorganic trace metals and will therefore modify their interaction with organisms (Millero et al., 2009; Campbell et al., 2014; Stockdale et al., 2016). Decreasing pH could increase the concentration of Cu2+ by reducing complex formation with CO32-and OH−, thereby altering the bioavailability and increasing copper toxicity to marine organisms (Millero et al., 2009; Roberts et al., 2013). On the other hand, there is competition between H+ and Cu to prevent Cu2+ from binding at the cell surface (Gao et al., 2017). The decreased inhibitory effect of Cu on Ulva prolifera under elevated pCO2 (1,000 μatm) suggests that the competition between H+ and Cu outcompetes the increased availability of Cu2+ in the medium (Gao et al., 2017). Here, although the effect of increasing pCO2 on Cu speciation in the medium were not determined, the free Cu2+ concentration should increase in background seawater medium as Millero et al. (2009) has previously indicated. However, results consistently found reduced Cu bioaccumulation in T. pseudonana under elevated pCO2, both with and without additional Cu exposure (Figures 1–3). Therefore, it suggested that the alleviation of toxicity could be due to the elevated CO2 per se but not to the reduced pH.
Transcriptome analysis indicated that algal selected under elevated pCO2 performed a specific copper detoxification strategy, that includes down-regulation of the reduction reaction from Cu2+ to Cu+ at the cell membrane to decrease copper uptake, up-regulation of biosynthesis of phytochelatins to transform free toxic Cu+ to less toxic organic forms, and up-regulation of a Cu+ transporter to enhance Cu+ efflux from the cell to decrease the concentration of free ions of Cu in the algal cells. All these processes could mitigate the oxidative stress in cells and enhance its tolerance to Cu exposure (Figure 4). Recent studies have demonstrated that Cu2+ is reduced to Cu+ extracellularly, which is an obligatory first step in Cu uptake in an oceanic diatom and is mediated by biological processes (Kong and Price, 2020).
Our results indicated that 1 and 20 μΜ Cu exposure inhibited growth and induced significant oxidative stress and damage in T. pseudonana (Figures 1, 4). Although Cu is one of the redox trace elements in biological systems and a basic cofactor of many enzymes (Wang and Ki, 2019), excess copper induces oxidative stress in the diatom and increases accumulation of reactive oxygen species (ROS), which would destroy macromolecules such as proteins, nucleic acids and lipids (Anu et al., 2016). In response to the oxidative stress resulting from copper exposure, Chlamydomonas reinhardtii was found to increase activities of the antioxidant enzymes glutathione S-transferase (GST), glutathione peroxidase (GPX), superoxide dismutase (SOD) and peroxidase (POD) to eliminate ROS (Zheng et al., 2011; Jiang et al., 2016). Bielmyer-Frasera et al. (2018) studied the physiological responses of two coral species, Acropora cervicornis and Pocillopora damicornis to OA and copper exposure. They found that copper exposure increased activities of the antioxidant enzymes CAT, GPX, and GR of these two species. By comparison with copper exposure alone, copper exposure under high pCO2 (1,000 μatm) further increased activities of CAT, GPX, GR of Acropora cervicornis. This is supported by our results in that the high pCO2 selected T. pseudonana showed higher activities of GPX and CAT when exposed to external stress than ambient-grown cells did.
Our results indicate that elevated pCO2 promotes growth and decreases Cu accumulation in a diatom, and the response to OA depends on the pCO2 level and the timescale of OA, which sheds new light on how carbon enrichment might counteract the negative effects of copper toxicity. However, whether the influence of elevated pCO2 on Cu bio-toxicity to T. pseudonana is consistent with other microorganisms and with other trace metals or other environmental pressures remains to be further investigated. Furthermore, transcriptomic analysis demonstrated that the long-term selected diatoms under elevated pCO2 employed a specific copper detoxification strategy under further OA scenarios, indicating phenotypic trait responses to OA resulting from genetic influences. This highlights the importance of long-term selection on the potential of algae to adapt to elevated pCO2 and thus change their biotic response to abiotic environmental changes. This detoxification effect could be transmitted from a primary producer through trophic transfer in the food chain, and may help us to understand the resilience potential of marine primary producers and maintain fisheries and ecosystem security under global climate change. Altogether, our study provides novel insights on the biogeochemical cycle of copper regulated by marine primary producers under global climate change.
The datasets presented in this study can be found in online repositories. The names of the repository/repositories and accession number(s) can be found in the article/Supplementary material.
NY designed the study. DX and SH provided the environmental and ecological context. DX, SH, XF, XZ, WW, JB, GB, and NY analyzed the data and interpreted the results. DX, SH, JB, GB, and NY wrote the manuscript. All authors contributed substantially to manuscript revisions.
This work was supported by the National Natural Science Foundation of China (41976110 and 31772075); the Young Taishan Scholars Program to DX, Taishan Scholars Funding and Talent Projects of Distinguished Scientific Scholars in Agriculture; Marine S&T Fund of Shandong Province for Pilot National Laboratory for Marine Science and Technology (Qingdao) (Nos. 2021QNLM050103-1); Central Public-interest Scientific Institution Basal Research Fund, CAFS (NO. 2020TD27); and China Agriculture Research System (CARS-50).
The authors declare that the research was conducted in the absence of any commercial or financial relationships that could be construed as a potential conflict of interest.
All claims expressed in this article are solely those of the authors and do not necessarily represent those of their affiliated organizations, or those of the publisher, the editors and the reviewers. Any product that may be evaluated in this article, or claim that may be made by its manufacturer, is not guaranteed or endorsed by the publisher.
The Supplementary material for this article can be found online at: https://www.frontiersin.org/articles/10.3389/fmicb.2022.1113388/full#supplementary-material
Andresen, E., Peiter, E., and Küpper, H. (2018). Trace metal metabolism in plants. J. Exp. Bot. 69, 909–954. doi: 10.1093/jxb/erx465
Angel, B. M., Simpson, S. L., Granger, E., Goodwyn, K., and Jolley, D. F. (2017). Time-averaged concentrations are effective for predicting chronic toxicity of varying copper pulse exposures for two freshwater green algae species. Environ. Pollut. 230, 787–797. doi: 10.1016/j.envpol.2017.07.013
Anu, P. R., Bijoy Nandan, S., Jayachandran, P. R., and Don Xavier, N. D. (2016). Toxicity effects of copper on the marine diatom, Chaetoceros calcitrans. Reg. Stud. Mar. Sci. 8, 498–504. doi: 10.1016/j.rsma.2016.07.001
Armbrust, E. V. (2009). The life of diatoms in the world's oceans. Nature 459, 185–192. doi: 10.1038/nature08057
Armbrust, E. V., Berges, J. A., Bowler, C., Green, B. R. M. D., Putnam, N. H., Zhou, S., et al. (2004). The genome of the diatom Thalassiosira pseudonana: ecology, evolution, and metabolism. Science 306, 79–86. doi: 10.1126/science.1101156
Bautista-Chamizo, E., De Orte, M. R., DelValls, T. Á., and Riba, I. (2016). Simulating CO2 leakages from CCS to determine Zn toxicity using the marine microalgae Pleurochrysis roscoffensis. Chemosphere 144, 955–965. doi: 10.1016/j.chemosphere.2015.09.041
Benoiston, A., Ibarbalz, F. M., Bittner, L., Guidi, L., Jahn, O., Dutkiewicz, S., et al. (2017). The evolution of diatoms and their biogeochemical functions. Philos. Trans. R. Soc. Lond. Ser. B Biol. Sci. 372:20160397. doi: 10.1098/rstb.2016.0397
Bielmyer-Frasera, G. K., Patel, P., Capo, T., and Grosell, M. (2018). Physiological responses of corals to ocean acidification and copper exposure. Mar. Pollut. Bull. 133, 781–790. doi: 10.1016/j.marpolbul.2018.06.048
Brennan, G. L., Colegrave, N., and Collins, S. (2017). Evolutionary consequences of multidriver environmental change in an aquatic primary producer. Proc. Natl. Acad. Sci. U. S. A. 114, 9930–9935. doi: 10.1073/pnas.1703375114
Campbell, A. L., Mangan, S., Ellis, R. P., and Lewis, C. (2014). Ocean acidification increases copper toxicity to the early life history stages of the polychaete Arenicola marina in artificial seawater. Environ. Sci. Technol. 48, 9745–9753. doi: 10.1021/es502739m
Collins, S. (2016). Growth rate evolution in improved environments under prodigal son dynamics. Evol. Appl. 9, 1179–1188. doi: 10.1111/eva.12403
Collins, S., and Bell, G. (2004). Phenotypic consequences of 1,000 generations of selection at elevated CO2 in a green alga. Nature 431, 566–569. doi: 10.1038/nature02945
Davis, A. K., Hildebrand, M., and Palenik, B. (2006). Gene expression induced by copper stress in the diatom Thalassiosira pseudonana. Eukaryot. Cell 5, 1157–1168. doi: 10.1128/EC.00042-06
de los Santos, C. B., Arenas, F., Neuparth, T., and Santos, M. M. (2019). Interaction of short-term copper pollution and ocean acidification in seagrass ecosystems: toxicity, bioconcentration and dietary transfer. Mar. Pollut. Bull. 142, 155–163. doi: 10.1016/j.marpolbul.2019.03.034
de Orte, M. R., Sarmiento, A. M., Basallote, M. D., Rodríguez-Romero, A., Riba, I., and delValls, A. (2014). Effects on the mobility of metals from acidification caused by possible CO2 leakage from sub-seabed geological formations. Sci. Total Environ. 470-471, 356–363. doi: 10.1016/j.scitotenv.2013.09.095
Domingues, R. B., Guerra, C. C., Barbosa, A. B., Brotas, V., and Galvão, H. M. (2014). Effects of ultraviolet radiation and CO2 increase on winter phytoplankton assemblages in a temperate coastal lagoon. J. Plankton Res. 36, 672–684. doi: 10.1093/plankt/fbt135
Dong, F., Wang, P., Qian, W., Tang, X., Zhu, X., Wang, Z., et al. (2020). Mitigation effects of CO2-driven ocean acidification on Cd toxicity to the marine diatom Skeletonema costatum. Environ. Pollut. 259:113850. doi: 10.1016/j.envpol.2019.113850
Falciatore, A., Jaubert, M., Bouly, J., Bailleul, B., and Mock, T. (2020). Diatom molecular research comes of age: model species for studying phytoplankton biology and diversity. Plant Cell 32, 547–572. doi: 10.1105/tpc.19.00158
Feely, R. A., Sabine, C. L., Lee, K., Berelson, W., Kleypas, J., Fabry, V. J., et al. (2004). Impact of anthropogenic CO2 on the CaCO3 system in the oceans. Science 305, 362–366. doi: 10.1126/science.1097329
Gao, G., Liu, Y., Li, X., Feng, Z., Xu, Z., Wu, H., et al. (2017). Expected CO2-induced ocean acidification modulates copper toxicity in the green tide alga Ulva prolifera. Environ. Exp. Bot. 135, 63–72. doi: 10.1016/j.envexpbot.2016.12.007
Gattuso, J., Magnan, A., Bille, R., Cheung, W. W. L., Howes, E. L., Joos, F., et al. (2015). Contrasting futures for ocean and society from different anthropogenic CO2 emissions scenarios. Science 349:aac4722. doi: 10.1126/science.aac4722
Guo, J., Green, B. R., and Maldonado, M. T. (2015). Sequence analysis and gene expression of potential components of copper transport and homeostasis in Thalassiosira pseudonana. Protist 166, 58–77. doi: 10.1016/j.protis.2014.11.006
Hopkinson, B. M., Dupont, C. L., Allen, A. E., and Morel, F. M. M. (2011). Efficiency of the CO2-concentrating mechanism of diatoms. Proc. Natl. Acad. Sci. U. S. A. 108, 3830–3837. doi: 10.1073/pnas.1018062108
Huang, X., Deng, F., Yamaji, N., Pinson, S. R. M., Fujii-Kashino, M., Danku, J., et al. (2016). A heavy metal P-type ATPase OsHMA4 prevents copper accumulation in rice grain. Nat. Commun. 7, 1–13. doi: 10.1038/ncomms12138
Ivanina, A. V., and Sokolova, I. M. (2015). Interactive effects of metal pollution and ocean acidification on physiology of marine organisms. Curr. Zool. 61, 653–668. doi: 10.1093/czoolo/61.4.653
Jiang, Y., Zhu, Y., Hu, Z., Lei, A., and Wang, J. (2016). Towards elucidation of the toxic mechanism of copper on the model green alga Chlamydomonas reinhardtii. Ecotoxicology 25, 1417–1425. doi: 10.1007/s10646-016-1692-0
Kong, L. L., and Price, N. M. (2020). A reduction-dependent copper uptake pathway in an oceanic diatom. Limnol. Oceanogra. 65, 601–611. doi: 10.1002/lno.11329
Leung, P. T. Y., Yi, A. X., Ip, J. C. H., Mak, S. S. T., and Leung, K. M. Y. (2017). Photosynthetic and transcriptional responses of the marine diatom Thalassiosira pseudonana to the combined effect of temperature stress and copper exposure. Mar. Pollut. Bull. 124, 938–945. doi: 10.1016/j.marpolbul.2017.03.038
Li, F. T., Beardall, J., Collins, S., and Gao, K. S. (2017). Decreased photosynthesis and growth with reduced respiration in the model diatom Phaeodactylum tricornutum grown under elevated CO2 over 1800 generations. Glob. Change Biol. 23, 127–137. doi: 10.1111/gcb.13501
Liu, X. S., Feng, S. J., Zhang, B. Q., Wang, M. Q., Cao, H. W., Rono, J. K., et al. (2019). OsZIP1 functions as a metal efflux transporter limiting excess zinc, copper and cadmium accumulation in rice. BMC Plant Biol. 19:283. doi: 10.1186/s12870-019-1899-3
Miazek, K., Iwanek, W., Remacle, C., Richel, A., and Goffin, D. (2015). Effect of metals, metalloids and metallic nanoparticles on microalgae growth and industrial product biosynthesis: a review. Int. J. Mol. Sci. 16, 23929–23969. doi: 10.3390/ijms161023929
Millero, F. J., Woosley, R., Ditrolio, B., and Waters, J. (2009). Effect of ocean acidification on the speciation of metals in seawater. Oceanography 22, 72–85. doi: 10.5670/oceanog.2009.98
Navarrete, A., González, A., Gómez, M., Contreras, R. A., Díaz, P., Lobos, G., et al. (2019). Copper excess detoxification is mediated by a coordinated and complementary induction of glutathione, phytochelatins and metallothioneins in the green seaweed Ulva compressa. Plant Physiol. Biochem. 135, 423–431. doi: 10.1016/j.plaphy.2018.11.019
O'Donnell, D. R., Hamman, C. R., Johnson, E. C., Kremer, C. T., Klausmeier, C. A., and Litchman, E. (2018). Rapid thermal adaptation in a marine diatom reveals constraints and trade-offs. Glob. Chang. Biol. 24, 4554–4565. doi: 10.1111/gcb.14360
Osborne, E. B., Thunell, R. C., Gruber, N., Feely, R. A., and Benitez-Nelson, C. R. (2020). Decadal variability in twentieth-century ocean acidification in the California current ecosystem. Nat. Geosci. 13, 43–49. doi: 10.1038/s41561-019-0499-z
Park, J., Lee, H., Depuydt, S., Han, T., and Pandey, L. K. (2020). Assessment of five live-cell characteristics in periphytic diatoms as a measure of copper stress. J. Hazard. Mater. 400:123113. doi: 10.1016/j.jhazmat.2020.123113
Pierrot, D, Lewis, E, and Wallace, D. (2006). MS Excel program developed for CO2 system calculations. ORNL/CDIAC-105a. Carbon Dioxide Information Analysis Center, Oak Ridge National Laboratory, US Department of Energy, Oak Ridge, TN.
Qu, P., Fu, F. X., and Hutchins, D. A. (2018). Responses of the large centric diatom Coscinodiscus sp to interactions between warming, elevated CO2, and nitrate availability. Limnol. Oceanogr. 63, 1407–1424. doi: 10.1002/lno.10781
Raven, J. A., Giordano, M., Beardall, J., and Maberly, S. C. (2011). Algal and aquatic plant carbon concentrating mechanisms in relation to environmental change. Photosynth. Res. 109, 281–296. doi: 10.1007/s11120-011-9632-6
Reusch, T. B. H., and Boyd, P. W. (2013). Experimental evolution meets marine phytoplankton. Evolution 67, 1849–1859. doi: 10.1111/evo.12035
Roberts, D. A., Birchenough, S. N. R., Lewis, C., Sanders, M. B., Bolam, T., and Sheahan, D. (2013). Ocean acidification increases the toxicity of contaminated sediments. Glob. Chang. Biol. 19, 340–351. doi: 10.1111/gcb.12048
Scheiber, I. F., Pilátová, J., Malych, R., Kotabová, E., Krijt, M., Vyoral, D., et al. (2019). Copper and iron metabolism in Ostreococcus tauri - the role of phytotransferrin, plastocyanin and a chloroplast copper-transporting ATPase. Metallomics 11, 1657–1666. doi: 10.1039/c9mt00078j
Schlüter, L., Lohbeck, K. T., Gröger, J. P., Riebesell, U., and Reusch, T. B. H. (2016). Long-term dynamics of adaptive evolution in a globally important phytoplankton species to ocean acidification. Sci. Adv. 2:e1501660. doi: 10.1126/sciadv.1501660
Schlüter, L., Lohbeck, K. T., Gutowska, M. A., Gröger, J. P., Riebesell, U., and Reusch, T. B. H. (2014). Adaptation of a globally important coccolithophore to ocean warming and acidification. Nat. Clim. Chang. 4, 1024–1030. doi: 10.1038/nclimate2379
Shi, D., Xu, Y., Hopkinson, B. M., and Morel, F. M. M. (2010). Effect of ocean acidification on iron availability to marine phytoplankton. Science 327, 676–679. doi: 10.1126/science.1183517
Stockdale, A., Tipping, E., Lofts, S., and Mortimer, R. J. G. (2016). Effect of ocean acidification on organic and inorganic speciation of trace metals. Environ. Sci. Technol. 50, 1906–1913. doi: 10.1021/acs.est.5b05624
Thangaraj, S., and Sun, J. (2020). Transcriptomic reprogramming of the oceanic diatom Skeletonema dohrniiunder warming ocean and acidification. Environ. Microbiol. 23, 980–995. doi: 10.1111/1462-2920.15248
Thoms, M. K., Kremer, C. T., Klausmeier, C. A., and Litchman, E. (2012). A global pattern of thermal adaptation in marine phytoplankton. Science 338, 1085–1088. doi: 10.1126/science.1224836
Tong, S., Gao, K., and Hutchins, D. A. (2018). Adaptive evolution in the coccolithophore Gephyrocapsa oceanica following 1,000 generations of selection under elevated CO2. Glob. Chang. Biol. 24, 3055–3064. doi: 10.1111/gcb.14065
Valenzuela, J. J., Jacob, J., García, L., de Lomana, A., Lee, A., Armbrust, E. V., et al. (2018). Ocean acidification conditions increase resilience of marine diatoms. Nat. Commun. 9:2328. doi: 10.1038/s41467-018-04742-3
Vargas, C. A., Lagos, N. A., Lardies, M. A., Duarte, C., Manríquez, P. H., Aguilera, V. M., et al. (2017). Species-specific responses to ocean acidification should account for local adaptation and adaptive plasticity. Nat. Ecol. Evol. 1:84. doi: 10.1038/s41559-017-0084
Waldbusser, G. G., and Salisbury, J. E. (2014). Ocean acidification in the coastal zone from an organism's perspective: multiple system parameters, frequency domains, and habitats. Annu. Rev. Mar. Sci. 6, 221–247. doi: 10.1146/annurev-marine-121211-172238
Wang, H., and Ki, J. (2019). Molecular characterization and expression analysis of copper-zinc superoxide dismutases from the freshwater alga Closterium ehrenbergii under metal stress. Environ. Toxicol. 35, 5–14. doi: 10.1002/tox.22837
Wu, Y., Campbell, D. A., Irwin, A. J., Suggett, D. J., and Finkel, Z. V. (2014). Ocean acidification enhances the growth rate of larger diatoms. Limnol. Oceanogr. 59, 1027–1034. doi: 10.4319/lo.2014.59.3.1027
Xu, D., Schaum, C. E., Li, B., Chen, Y., Tong, S., Fu, F. X., et al. (2022). Acclimation and adaptation to elevated pCO2 increase arsenic resilience in marine diatoms. ISME J. 15, 1599–1613. doi: 10.1038/s41396-020-00873-y
Yung, M. M. N., Kwok, K. W. H., Djurišić, A. B., Giesy, J. P., and Leung, K. M. Y. (2017). Influences of temperature and salinity on physicochemical properties and toxicity of zinc oxide nanoparticles to the marine diatom Thalassiosira pseudonana. Sci. Rep. 7, 3662–3669. doi: 10.1038/s41598-017-03889-1
Zhang, X., Xu, D., Huang, S., Wang, S., Han, W., Liang, C., et al. (2020). The effect of elevated pCO2 on cadmium resistance of a globally important diatom. J. Hazard. Mater. 396:122749. doi: 10.1016/j.jhazmat.2020.122749
Zheng, Q., Meng, Q., Wei, Y. Y., and Yang, Z. M. (2011). Alleviation of copper-induced oxidative damage in Chlamydomonas reinhardtii by carbon monoxide. Arch. Environ. Contam. Toxicol. 61, 220–227. doi: 10.1007/s00244-010-9602-6
Zhong, J., Guo, Y., Liang, Z., Huang, Q., Lu, H., Pan, J., et al. (2021). Adaptation of a marine diatom to ocean acidification and warming reveals constraints and trade-offs. Sci. Total Environ. 771:145167. doi: 10.1016/j.scitotenv.2021.145167
Zhu, Z., Qu, P., Gale, J., Fu, F. X., and Hutchins, D. A. (2017). Individual and interactive effects of warming and CO2 on Pseudo-nitzschia subcurvata and Phaeocystis antarctica, two dominant phyto-plankton from the Ross Sea, Antarctica. Biogeosciences 14, 1–15. doi: 10.5194/bg-2017-18
Keywords: ocean acidification, copper accumulation, copper toxicity, adaptation, Thalassiosira pseudonana
Citation: Xu D, Huang S, Fan X, Zhang X, Wang Y, Wang W, Beardall J, Brennan G and Ye N (2023) Elevated CO2 reduces copper accumulation and toxicity in the diatom Thalassiosira pseudonana. Front. Microbiol. 13:1113388. doi: 10.3389/fmicb.2022.1113388
Received: 01 December 2022; Accepted: 16 December 2022;
Published: 06 January 2023.
Edited by:
Jin Zhou, Tsinghua University, ChinaReviewed by:
Guo Fu Chen, Harbin Institute of Technology, Weihai, ChinaCopyright © 2023 Xu, Huang, Fan, Zhang, Wang, Wang, Beardall, Brennan and Ye. This is an open-access article distributed under the terms of the Creative Commons Attribution License (CC BY). The use, distribution or reproduction in other forums is permitted, provided the original author(s) and the copyright owner(s) are credited and that the original publication in this journal is cited, in accordance with accepted academic practice. No use, distribution or reproduction is permitted which does not comply with these terms.
*Correspondence: Georgina Brennan, ✉ Zy5sLmIuZG9vbmFuQGdtYWlsLmNvbQ==; Naihao Ye, ✉ eWVuaEB5c2ZyaS5hYy5jbg==
†These authors have contributed equally to this work
Disclaimer: All claims expressed in this article are solely those of the authors and do not necessarily represent those of their affiliated organizations, or those of the publisher, the editors and the reviewers. Any product that may be evaluated in this article or claim that may be made by its manufacturer is not guaranteed or endorsed by the publisher.
Research integrity at Frontiers
Learn more about the work of our research integrity team to safeguard the quality of each article we publish.