- 1Research Group of Personalized Diet, Korea Food Research Institute, Iseo-myeon, South Korea
- 2Department of Rehabilitation Medicine of Korean Medicine, Dongguk University, Goyang-si, South Korea
- 3Department of Biomedical Sciences, Center for Global Future Biomedical Scientists at Chonnam National University, Gwangju, South Korea
Although drugs have been reported to modulate the gut microbiota, the effects of anti-obesity drugs on the gut microbiota remain unclear. Lorcaserin (LS) and phentermine (PT) are commonly used anti-obesity drugs. However, to our best knowledge, no studies have simultaneously assessed the effects of LS and PT on obesity and gut microbiota. This study aimed to explore the relationship between the anti-obesity effects of LS and PT and re-modulation of host gut microbiota. To test hypothesis, we fed C57BL/6J mice with a high-fat diet supplemented with LS and PT via oral gavage for 8 weeks. After sacrifice, body weight, fat accumulation, and serum biomarkers were measured, and the gut microbial composition was analyzed using 16 s rRNA amplicon sequencing. LS and PT were observed to modulate the gut microbial composition and restore gut microbial dysbiosis, as indicated by an increased Firmicutes/Bacteroidetes ratio. Significantly modulated genera by LS and PT treatment were strongly correlated with obesity-related markers. Additionally, LS and PT increased the mRNA level of G protein-coupled receptor 120 (GPR120) in the colon tissue. ASV3566, which corresponds to Eubacterium coprostanoligenes, was correlated with GPR120 and obesity-related markers such as glutamic pyruvic transaminase (GPT) and serum triglyceride (TG). In conclusion, LS and PT can modulate the gut microbiota dysbiosis and the gut microbiota plays a role in mediating the anti-obesity effect of drugs.
1. Introduction
Obesity has become a global public health problem induced by excessive body fat due to increased energy intake and reduced expenditure. Obesity increases the risk of chronic metabolic diseases, such as type 2 diabetes (T2D), cardiovascular disease, and chronic low-grade inflammation; therefore, effective management strategies are needed (Vijay-Kumar et al., 2010). Recent studies have shown that obesity and obesity-induced metabolic disorders are closely related to the gut microbiota (Zhao, 2013; Muscogiuri et al., 2019). The composition of the gut microbiota and obesity influence each other, and indicators of dysbiosis such as the Firmicutes/Bacteroidetes (F/B) ratio are also markers of obesity (Ley et al., 2006; Boulangé et al., 2016). Moreover, microbial metabolites such as short-chain fatty acids (SCFAs) also affect obesity and host metabolism, including glycemic control, fat metabolism, and regulation of inflammation and immunity (van der Hee and Wells, 2021).
Recently, the role of the gut microbiota in drug treatment for obesity has been considered since the gut microbiota is involved in drug response and metabolism (Wilson and Nicholson, 2009; Kang et al., 2013; Vila et al., 2020). Moreover, several studies have reported alterations in the gut microbiota following drug treatment in clinical trials (Pascale et al., 2019; Vila et al., 2020). Metformin, a first-line clinical medicine for T2DM, enhanced the relative abundance of Akkermansia, which has the potential for glucose metabolism and insulin modulation (Shin et al., 2014; Montandon and Jornayvaz, 2017). Nuciferine has the ability to reduce high-fat diet (HFD)-induced obesity by altering the relative abundance of the gut microbiota, which is related to SCFA production and metabolic parameters (Wang et al., 2020). Orlistat modified the gut microbial composition, and the increased bacterial species was correlated with several metabolic pathways (Ke et al., 2020). Lorcaserin (LS) and phentermine (PT) are commonly used anti-obesity drugs. LS is a serotonin agent that selectively stimulates serotonin 2C receptor agonist, and PT modulates catecholamines in the hypothalamus, reducing appetite (Kim et al., 2014). However, to our knowledge, no studies have been published on the effects of LS and PT on gut microbiota. In this study, we aimed to investigate the impact of LS and PT on the composition of the gut microbiota in a HFD model. This research may provide support for the application of LS and PT in the treatment of obesity.
2. Materials and methods
2.1. Animal studies
Six-week-old male C57BL/6 mice obtained from DBL Inc. (Eumseong-gun, South Korea) were acclimatized for 1 week under a controlled environment with a 12-h light/dark cycle at 25°C and 50–60% relative humidity with a chow diet (Purina Irradiated Laboratory Rodent Chow, Purina Korea, Seoul, South Korea). The animal study was approved by the Institutional Animal Care and Use Committee (IACUC-2019-06187) of Dongguk University Ilsan Hospital and performed according to the Guide for the Care and Use of Laboratory Animals (National Research Council, 2011).
To assess the efficacy of anti-obesity drug treatment in HFD-induced obese mice, mice were randomly divided into four groups: control group (NOR), HFD, HFD + LS, and HFD + PT; n = 7–8 per group. The mice in NOR were fed a 10% fat control diet (D12450B, Research Diets Inc., United States), and other mice were fed a 60% HFD (D12492, Research Diets Inc., New Brunswick, NJ, United States) for 4 weeks. Moreover, the mice in the HFD + LS and HFD + PT groups received LS (10 mg/kg/day) and PT (5 mg/kg/day), respectively, via oral gavage. The mice in the NOR and HFD groups, which were untreated, were orally administered with water. The treatments were administered five times a week for 8 weeks.
Body weight was measured once a week. Food intake was measured three times a week per cage, and was expressed as the average daily food intake per mouse. After the termination of the experimental period, all animals were subjected to overnight fasting. The animals were then sacrificed with a combination of Zoletil (tiletamine-zolazepam, Virbac, Carros, France) and Rompun (xylazine-hydrochloride, Bayer, Leverkusen, Germany; 1:1, v/v). Subsequently, blood samples were collected from the central aorta and rapidly transferred into a BD Vacutainer (BD, Franklin Lakes, NJ, United States). The blood samples were allowed to clot for 2 h at room temperature and then centrifuged at 3000 × g for 15 min. The sera were separated and stored at −80°C for further analyses. The visceral adipose tissue, perigonadal adipose tissues, and colon tissues were excised quickly, washed in ice-cold PBS (pH 7.4), dried, weighed, and stored at −80°C until use. The data for the NOR and HFD groups were retrieved from a simultaneous study by Song et al. (2022).
2.2. Serum biochemical analyses and oral glucose tolerance test (OGTT)
Serum triglyceride (TG), total cholesterol (TC), and glutamic pyruvic transaminase (GPT) levels were determined using commercial enzymatic assay kits (Asan Pharmaceutical Co., Seoul, South Korea). Serum insulin concentration was measured using an Ultra-Sensitive Mouse Insulin ELISA kit (MIOBS, Yokohama, Japan) according to the manufacturer’s instructions. For the OGTT, the animals were subjected to overnight (12 h) fasting and administered sterilized glucose solution (2 g/kg, Sigma, Louis, MO, United States) via oral gavage. The glucose levels in the blood samples collected from the tail vein were measured at five different time points (0, 30, 60, 90, and 120 min) using the ACCU-CHEK Active glucometer (ACCU-CHEK, Mannheim, Germany).
2.3. Quantitative real-time PCR analysis
Total RNA was extracted from colonic tissue using TRIzol (Invitrogen Life Technologies, Carlsbad, CA, United States) according to the manufacturer’s instructions. The quality and quantity of crude RNA were examined using a NanoPhotometer (P330-IMPLEN, Munich, Germany). RNA was stored at −80°C for further analysis using RT-qPCR. An equal quantity of each RNA sample was reverse transcribed using an oligo deoxythymine 18-mer primer (Thermo Scientific, Waltham, MA, United States), and cDNA was synthesized using a cDNA RT PreMix kit (Bioneer, Daejeon, South Korea). Quantitative analysis of the mRNA expression of GPR41, GPR43, GPR120, IL-10, IL-13, TNF-α, IL-1β, IL-6, MCP-1 and glyceraldehyde-3-phosphatase dehydrogenase (GAPDH) was performed using RT-qPCR with a Light Cycler 480™ platform (Roche, Roche Applied Science, Mannheim, Germany) using a SYBR Green Real-time PCR Master Mix (Toyobo, Osaka, Japan). Data processing and analysis were performed using a dedicated Light Cycler software (Roche Applied Science, version 1.2). The Ct values were normalized using GAPDH, and the relative gene expression was quantified using the standard 2−△△Ct method. Primer sequences are listed in Supplementary Table 1.
2.4. Sequence-based gut microbiota analysis
Fresh fecal samples were collected 1 week before sacrifice and stored at −80°C until use. Genomic DNA was isolated from the samples using the QIAamp stool DNA mini kit (QIAGEN, Hilden, Germany) according to the manufacturer’s instructions. PCR amplification of the V1–V2 region of the 16S rRNA gene was performed using a thermal cycler system (Bio-Rad, Hercules, CA, United States). Then, sequencing reactions were performed using the Ion Torrent Personal Genome Machine (Ion PGM, Thermo Scientific, Wilmington, DE, United States) according to the manufacturer’s instructions. Raw sequence reads were quality-filtered, and quality-controlled reads were processed for diversity analysis and taxonomy assignment using the Quantitative Insights into the Microbial Ecology 2 (QIIME2) software package (Caporaso et al., 2010). All raw sequencing data described in this study are available at the DNA Data Bank of Japan (accession number DRA013284).
2.5. Statistical analysis
Significance was evaluated using one-way analysis of variance (ANOVA) followed by Dunnett’s multiple comparisons or Kruskal–Wallis test followed by Dunn’s test. Significance was set at p ≤ 0.05. To identify taxa with differing relative abundances among groups, the linear discriminant analysis effect size (LEfSe) method was conducted using a web-based program (Segata et al., 2011).1 The strength of the relationships between parameters was assessed using Spearman’s correlation test.
3. Results
3.1. Effects of anti-obesity drugs on HFD-induced obesity
To investigate the effects of anti-obesity drugs, we established an obese mouse model by feeding them HFD for 4 weeks, followed by treatment with LS or PT for 8 weeks. HFD led to significant increases in food intake, body weight, and fat accumulation compared to the NOR group (Figures 1A–F).
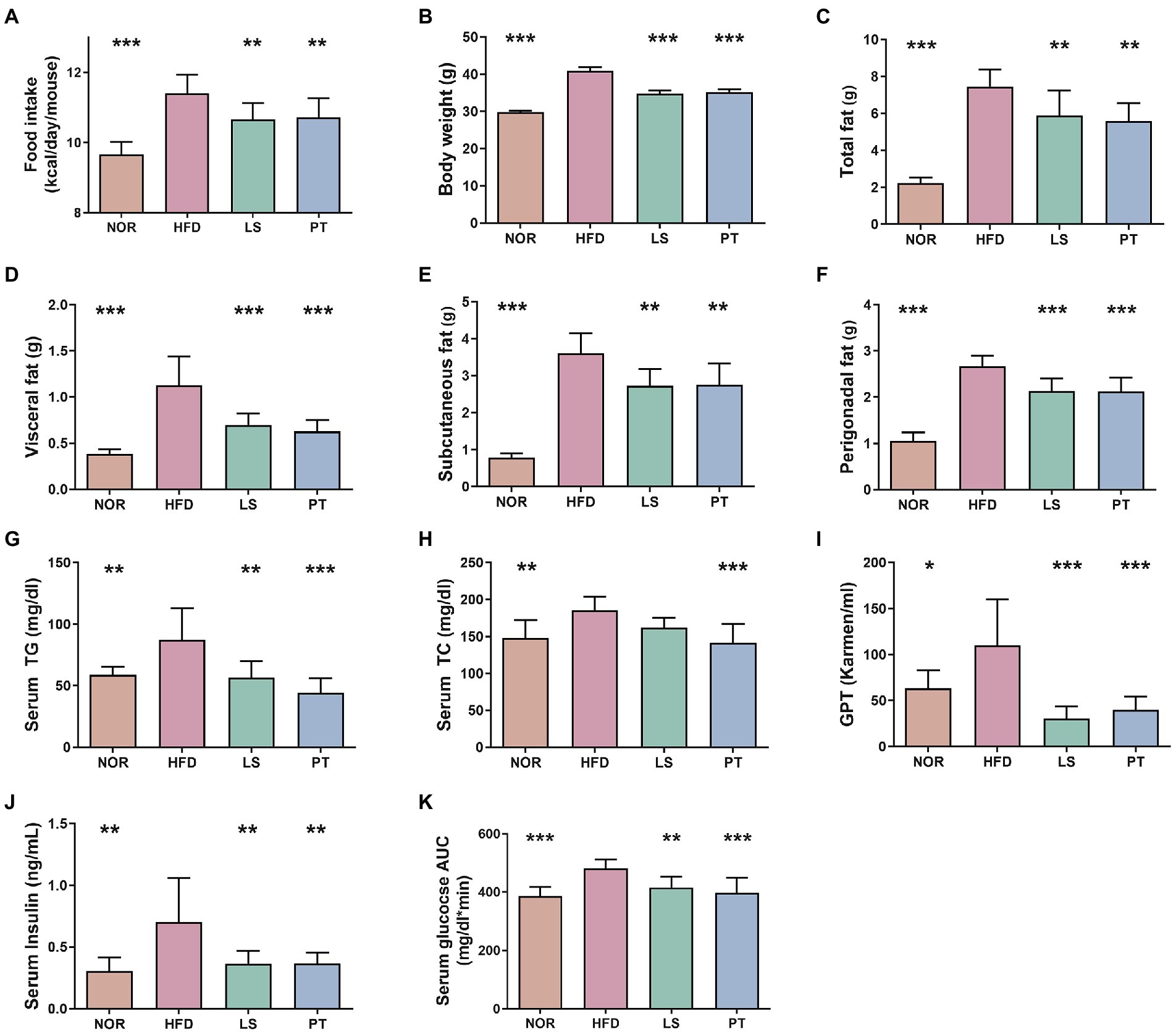
Figure 1. Effect of anti-obesity drugs on weight, serum biomarker, and glucose tolerance in a high-fat diet (HFD)-induced obese model after treatment for 8 weeks. (A) Food intake (B) Body weight. (C) Total fat. (D) Visceral fat. (E) Subcutaneous fat. (F) Perigonadal fat. (G) Serum triglyceride (TG). (H) Serum total cholesterol (TC). (I) Serum glutamic pyruvic transaminase (GPT). (J) Serum insulin. (K) Oral glucose tolerance test (OGTT) AUC. Data are expressed as the mean ± SEM. Statistical significance was assessed using one-way ANOVA. *p < 0.05, **p < 0.01, ***p < 0.001, vs. HFD. NOR, control group; LS, lorcaserin; PT, phentermine.
Lorcaserin and PT supplementation significantly decreased food intake compared with HFD (Figure 1A). Notably, LS and PT supplementation affected body weight and fat accumulation upon HFD feeding. LS and PT supplementation significantly decreased body weight compared with that in the HFD group (Figure 1B). In addition, LS and PT supplementation significantly decreased weights of total fat, visceral fat, subcutaneous fat, and perigonadal fat (Figures 1C–F).
The anti-obesity drugs also influenced serum biochemical parameters, such as TG, TC, GPT, insulin, OGTT levels, and fasting glucose (Figures 1G–K). HFD led to significant increases in all serum biochemical parameters compared to the NOR group. Compared to the HFD group, the LS group had significantly reduced serum biochemical parameters, except for TC. Furthermore, the PT group had significantly reduced all serum biochemical parameters compared to the HFD group.
3.2. Anti-obesity drugs altered the composition of the gut microbiota
To assess the effect of anti-obesity drugs on the gut microbiota, 16S rRNA amplicon sequencing was performed using fecal samples. A total of 6,370,426 reads were generated, and a total of 3,417,057 high-quality reads were obtained through quality control, such as denoising and chimera removal. For subsequent analysis, 25,795 reads per sample were used through even subsampling.
Beta diversity analysis was performed to evaluate the overall structural changes in the gut microbiota. The resulting of PCoA plot showed separation among groups, and significance was determined using the PC1 value on the unweighted UniFrac distance matrix (Figures 2A, B). LS and PT treatment resulted in a significant structural shift along with the PC1 values from the HFD group to the direction of the NOR group. The resulting of PLS-DA plot at phylum level also showed separation among groups, and the taxa with greater absolute values on component 1 was visualized using a bar plot (Supplementary Figure 1). The most relevant original variables were Firmicutes and Bacteroides. LS and PT treatments significantly restored the F/B ratio (Figure 2C).
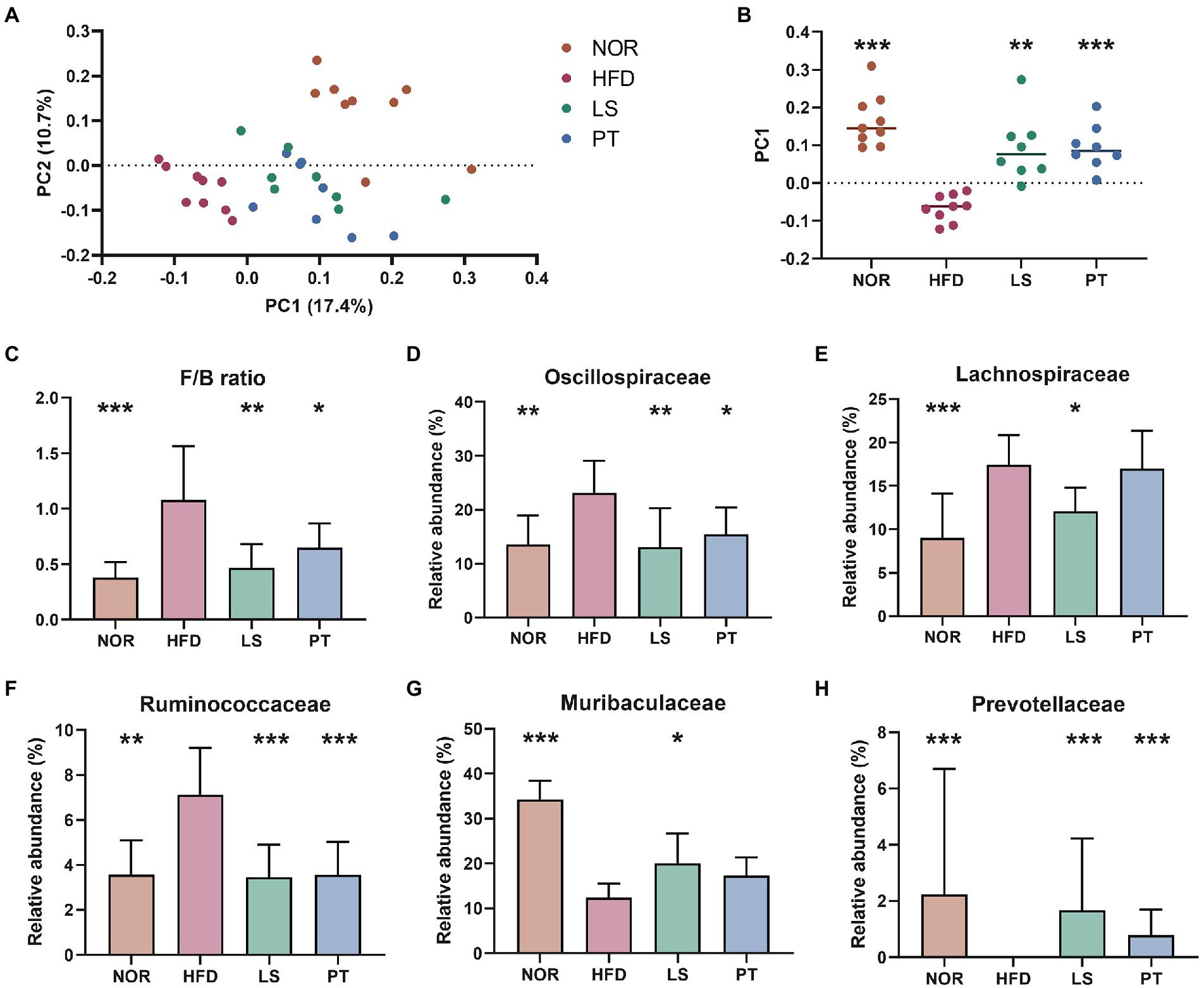
Figure 2. Effect of anti-obesity drugs on the structure of the gut microbiota in a high-fat diet (HFD)-induced obese model after treatment for 8 weeks. (A) Unweighted UniFrac PCoA plot based on the ASV abundance of each mouse. (B) Box plot of PC1 values in each group. (C) The F/B ratio was calculated as a biomarker of gut dysbiosis. (D–H) The relative abundance at the family level significantly changes with HFD. Values are presented as the mean ± SEM. Statistical significance was assessed using the Kruskal–Wallis test. *p < 0.05, **p < 0.01, ***p < 0.001, vs. HFD. NOR, control group; LS, lorcaserin; PT, phentermine.
At family level, the resulting of PLS-DA plot showed clear separation among groups (Supplementary Figures 2A–D). Of note, PT treatment showed a return to NOR group. The taxa with greater absolute values on component 1 was visualized using a bar plot (Supplementary Figures 2E–G). The most relevant original variables were Lachnospiraceae, Muribaculaceae, Oscillospiraceae, Prevotellaceae, Ruminococcaceae, and Streptococcaceae. Statistical test revealed a significant increase in Oscillospiraceae, Lachnospiraceae, and Ruminococcaceae, and a decrease in the abundance of Muribaculaceae and Prevotellaceae in the HFD group compared to those in the NOR group (Figures 2D–H). LS treatment significantly restored these taxa to levels similar to those in the NOR group. PT treatment significantly restored the levels of Oscillospiraceae, Ruminococcaceae, and Prevotellaceae.
At genus level, the resulting of PLS-DA plot at family level showed clear separation among groups (Supplementary Figures 3A–D). Of note, PT treatment showed a return to NOR group. The taxa with greater absolute values on component 1 was visualized using a bar plot (Supplementary Figures 3E–G). LEfSe was performed to identify significantly different taxa between the HFD groups and NOR or drug treatment groups (Figure 3). A total of 39 genera were significantly different between the NOR and the HFD groups. Compared to the HFD group, 13 and 12 genera in the LS and PT groups, respectively, matched those in the NOR group. Notably, LS and PT treatment restored the abundance of Prevotellaceae_UC001, Prevotellaceae_NK3B31_group, and Alloprevotella and increased the level of Colidextribacter and Mucispirillum due to HFD compared to the NOR group (Figures 3A–C).
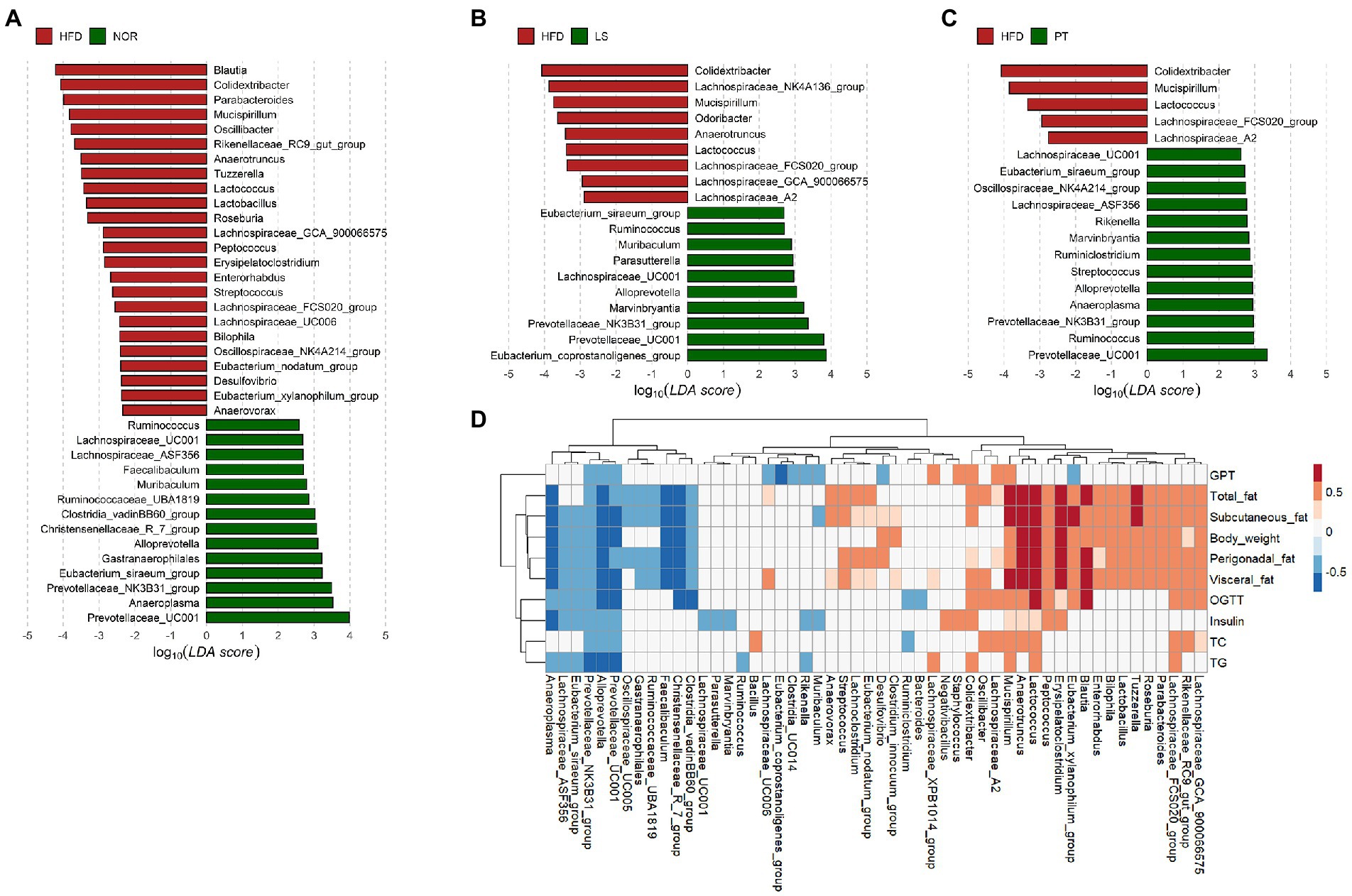
Figure 3. Association of microbiota with anti-obesity drugs and obesity-related parameters. (A–C) LEfSe analysis identifies the significant differently abundant taxa with a cutoff value of log10(LDA score) above 2.0. (A) control group (NOR)-, (B) lorcaserin (LS)-, and (C) phentermine (PT)-enriched taxa are indicated with a positive LDA score (green), while taxa enriched in high-fat diet (HFD) are characterized by a negative score (red). (D) A heatmap generated using Spearman correlation shows the association of significantly differential taxa among groups from the LEfSe results with obesity-related markers. Only correlations with a significance p < 0.05 are represented. Color scale indicates the value of the correlation coefficient.
Spearman’s rank correlations were used to identify the genera that were strongly correlated with obesity-related markers. Most of the significantly different taxa from the LEfSe results showed a significant correlation with obesity-related markers (Figure 3D). Notably, Prevotellaceae_UC001, Prevotellaceae_NK3B31_group, and Alloprevotella were significantly negatively correlated with all obesity-related markers. In addition, Mucispirillum exhibited a significant positive correlation with all obesity-related markers. Colidextribacter showed a significant positive correlation with fat accumulation, insulin, TG, and GPT.
3.3. Effects of anti-obesity drugs on GPRs and its association with the gut microbiota
G protein-coupled receptors (GPRs) are mediators of host-microbial interactions (Cohen et al., 2017; Melhem et al., 2019). To determine whether GPRs are involved in the effects of anti-obesity drugs and the effect of gut microbiota, we investigated the effect of anti-obesity drugs on the gene expressions of GPRs in colonic tissues (Figure 4). Anti-obesity drugs did not affect the gene expression of GPR41 and GPR43 (Figures 4A, B). Although no significant difference was observed between the NOR group and the HFD group, GPR120 was significantly increased after treatment with LS and PT, as compared with the HFD group (Figure 4C).
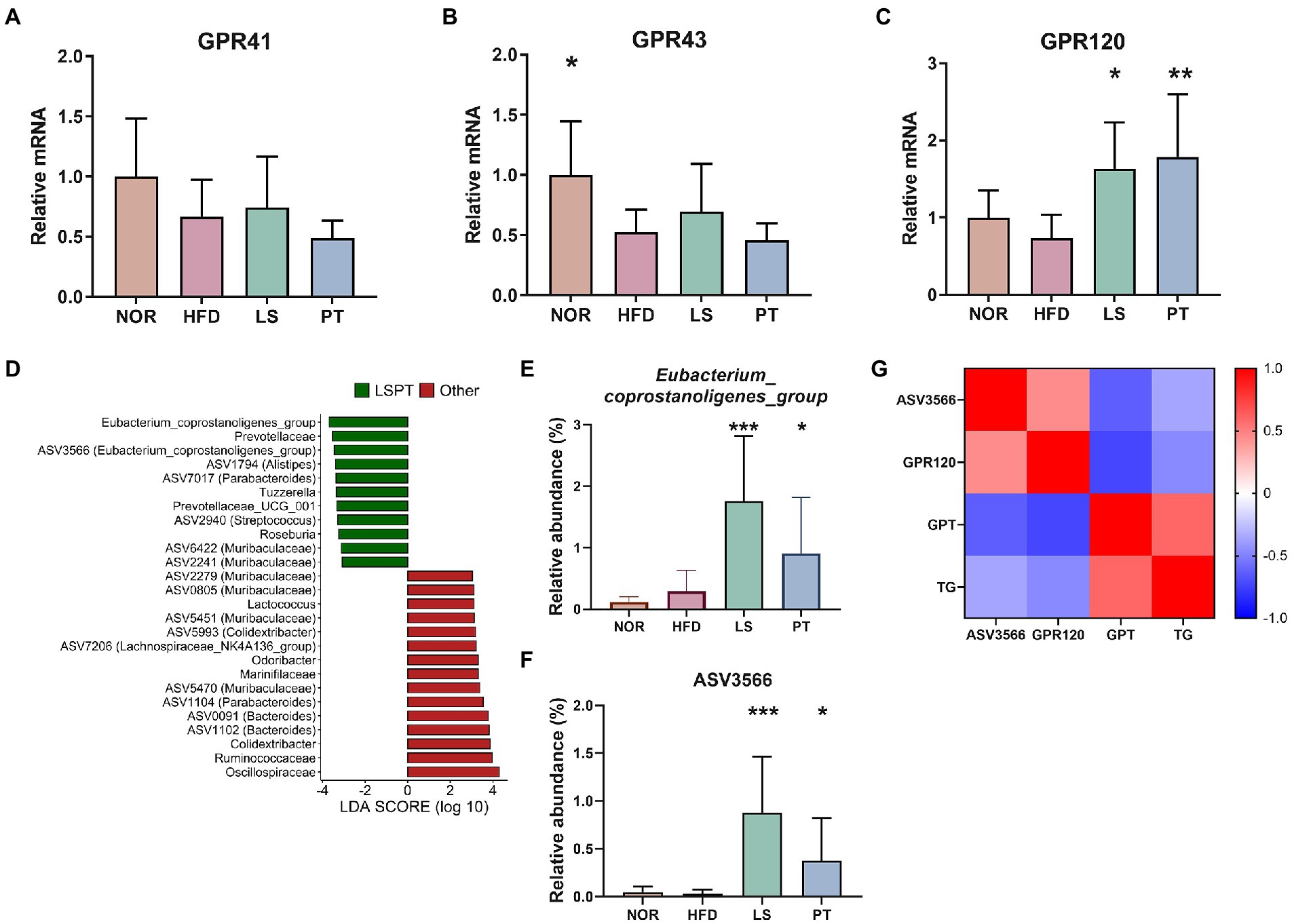
Figure 4. Effect of anti-obesity drugs on GPRs in colonic tissue and the association of the gut microbiota and GPRs. (A–C) The mRNA gene expression of GPRs in high-fat diet (HFD)-induced obese model. (D) LEfSe plot of gut microbiota at the genus and ASV levels in the comparison between LSPT (LS + PT) and others (NOR + HFD). The relative abundance of (E) Eubacterium_coprostanoligenes_group and (F) ASV3566 in each group. (G) A heatmap generated using Spearman correlation shows associations of ASV3566 with GPR120, GPT, and TG. The color scale indicates the value of the correlation coefficient. Data are expressed as the mean ± SEM. Statistical significance was assessed using one-way ANOVA. *p < 0.05, **p < 0.01, ***p < 0.001, vs. HFD. HFD, high-fat diet; NOR, control group; LS, lorcaserin; PT, phentermine.
Furthermore, we identified the microorganisms correlated with GPR120, which was a result of the LS and PT treatment. First, we performed the LEfSe analysis to detect microorganisms increased by LS and PT. Results comparing LS and PT groups with other groups showed that Eubacterium_coprostanoligenes_group had the highest LDA score (Figure 4D). The relative abundance of Eubacterium_coprostanoligenes_group was significantly higher in the LS and PT groups than in the NOR and HFD groups (Figure 4E). ASV3566, which corresponds to Eubacterium coprostanoligenes, was rare in other groups but was significantly abundant in LS and PT groups (Figure 4F). Next, using Spearman’s correlation analysis, we found that ASV3566 had a significant positive correlation with GPR120 and a significant negative correlation with GPT and TG (Figure 4G). Moreover, GPR120 had a significant negative correlation with GPR and TG.
GPR120 is known to regulate adipogenesis, inflammation, glucose uptake, and insulin resistance (Song et al., 2017). Therefore, we next investigated the regulation of adipose inflammation by LS and PT (Supplementary Figure 4). IL-10, which is anti-inflammatory cytokine, was not significantly increased by LS and PT treatment in the HFD model (Supplementary Figure 4A). However, IL-13, which is anti-inflammatory cytokine, was significantly increased by LS and PT treatment in the HFD model (Supplementary Figure 4B). Moreover, the pro-inflammatory cytokines TNF-a and IL-1b were significantly decreased by LS treatment in the HFD model (Supplementary Figures 4C, D). Notably, the pro-inflammatory cytokines IL-6 and MCP-1 were significantly decreased by LS and PT treatment in the HFD model (Supplementary Figures 4E, F).
4. Discussion
In this study, we hypothesized that anti-obesity drugs such as LS and PT induce changes in the gut microbiota. Our study revealed that drug supplementation can prevent HFD-induced obesity and restore gut microbial dysbiosis. Modulated members of the gut microbiota correlated with obesity-related markers. Furthermore, drug supplementation elevated the mRNA level of GPR120 and relieved adipose inflammation. Notably, we found the association of Eubacterium coprostanoligenes ASV3566 with GPR120 and TG. These results indicate that LS and PT can modulate the gut microbiota dysbiosis and the gut microbiota plays a role in mediating the anti-obesity effect of drugs.
A previous study reported that two types of gut microbes, Firmicutes and Bacteroidetes, were predominant in the human gut, and a high population of Bacteroidetes was observed in obese people (Ley et al., 2006). Since then, the F/B ratio has been used as a marker of gut microbial dysbiosis and as an indicator in obesity research (Indiani et al., 2018; Stojanov et al., 2020; Palmas et al., 2021). In our study, LS and PT significantly reduced the increased F/B ratio due to HFD feeding. Other studies have shown the results of drug-induced anti-obesity effects and reduction in the F/B ratio. Wang et al. (2020) showed that nuciferine supplementation significantly lowered the F/B ratio increased by HFD in an obese rat model. Ke et al. (2020) showed that orlistat treatment decreased the F/B ratio in an obese mouse model. Furthermore, An et al. (2018) showed that treatment with cordycepin, a major bioactive component from Cordyceps militaris, prevented body weight gain and decreased the F/B ratio in HFD-induced obese rats. These findings were consistent with our results. The increase in Firmicutes is linked to an increase in the breakdown of indigestible polysaccharides and the production of SCFAs. SCFAs are the primary end-products of bacterial fermentation of non-digestible carbohydrates in the gut that have an impact on host metabolism, such as gut integrity, glucose homeostasis, lipid metabolism, appetite regulation, and immune function (Morrison and Preston, 2016). However, SCFAs act as an energy source, and an excessive supply of SCFAs can lead to an increase in energy harvest (Murphy et al., 2010). Several studies have shown an increase in energy harvesting along with an increase in fecal SCFAs in obese individuals (Turnbaugh et al., 2006; Schwiertz et al., 2010; Fernandes et al., 2014; Rahat-Rozenbloom et al., 2014; Goffredo et al., 2016).
Furthermore, we found that the relative abundance of Oscillospiraceae, Lachnospiraceae, and Ruminococcaceae in the Firmicutes phylum was significantly increased by HFD. An increased Oscillospiraceae abundance is not commonly observed after an HFD or in obese individuals. However, some reports have shown that obesity increases Lachnospiraceae and Ruminococcaceae. Zeng et al. (2016) demonstrated that an increase in the abundance of Lachnospiraceae was accompanied by inflammation in HFD-fed mice. Colonization of Lachnospiraceae in germ-free ob/ob mice induced significant increases in mesenteric adipose tissue weight (Kameyama and Itoh, 2014). A study on Mexican children suggested that the abundance of Lachnospiraceae was significantly enhanced in overweight and obese individuals (Murugesan et al., 2015). Another study on Mexican women observed a significant increase in the abundance of Lachnospiraceae and Ruminococcaceae in individuals with obesity alone and obesity with metabolic syndrome (Chávez-Carbajal et al., 2019). An Italian cohort study showed that Lachnospiraceae and Ruminococcus gnavus (belonging to the Ruminococcaceae family) were significantly increased in overweight and obese patients and were positively correlated with body fat (Palmas et al., 2021). These results are consistent with our results. Moreover, LS and PT supplementation significantly restored the HFD-induced increase in Lachnospiraceae and Ruminococcaceae. We also observed that the relative abundance of Prevotellaceae and its genera, such as Prevotellaceae_UCG_001, Prevotellaceae_NK3B31_group, and Alloprevotella, was significantly decreased by HFD. Moreover, we observed that the altered levels of these taxa were recovered by LS and PT supplementation. Prevotellaceae_UCG_001 was significantly decreased in ob/ob mice and enriched by prebiotic inulin treatment. In addition, Prevotellaceae_UCG_001 had a significant negative correlation with liver indices and TC/HFDL-C and a significant positive correlation with the AMPK signaling pathways, which have downstream metabolic functions that promote fatty acid metabolism and glycolysis and inhibit hepatic fatty acid synthesis, cholesterol synthesis, and gluconeogenesis (Song et al., 2019). Prevotellaceae_NK3B31_group and Alloprevotella were notably decreased in the HFD group, and these changes were reversed by polyphenolic-enriched peach peel extract in the HFD mouse model (Kan et al., 2020). Concurringly, LS and PT supplementation significantly restored the decreased levels of Prevotellaceae and Alloprevotella by HFD. Moreover, correlation analysis showed that Prevotellaceae_UC001, Prevotellaceae_NK3B31_group, and Alloprevotella were significantly negatively correlated with obesity-related markers, including body weight, fat accumulation, and metabolic parameters. These results suggest that the recovery of HFD-induced microbial dysbiosis is linked to the prevention of obesity by drugs. Therefore, we postulate that changes in the gut microbiota caused by LS and PT supplementation may have a positive anti-obesity effect.
Additionally, LS and PT recovered the HFD-induced increase in the level of Colidextribacter. Colidextribacter was positively correlated with fat accumulation, insulin, TG, and GPT. Colidextribacter was identified in 2017, and Colidextribacter massiliensis is its only known species (Ricaboni et al., 2016). Wang et al. (2021) reported that the administration of olive fruit extracts improved antioxidant activity, which was associated with an increase in Alloprevotella sp. and a decrease in Colidextribacter. Colidextribacter was positively correlated with products of lipid peroxidation, such as malondialdehyde (MDA), and negatively correlated with antioxidant enzyme activities, such as total antioxidant activity (T-AOC), superoxide dismutase (SOD), and glutathione peroxidase (GSH-Px). Oxidative stress is a state of excessive reactive oxygen species, and an imbalance in antioxidant defense has been reported to be associated with obesity (Fernández-Sánchez et al., 2011). Decreased antioxidant defense markers, such as SOD and GSH-Px, and oxidative stress markers, such as MDA, have been observed in obese and diabetic individuals (Lee et al., 2015; Gunawardena et al., 2019). Yesilbursa et al. (2005) demonstrated that obesity is associated with enhanced lipid peroxidation and Orlistat, which is a drug designed to treat obesity by promoting weight reduction and lipid peroxidation. These observations suggest that one of the anti-obesity mechanisms of the gut microbiota may be mediated by antioxidant defense.
It is widely agreed that obesity and immunity are closely related. Specifically, enlargement of adipose tissue is associated with prominent inflammatory responses, such as infiltrating immune cells and increases in pro-inflammatory cytokines and adipokines (Sell et al., 2012). Oh et al. (2010) found that GPR120 functions as an omega-3 fatty acid receptor and mediates anti-inflammatory effects by repressing macrophage-induced tissue inflammation. Eicosapentaenoic acids, which are polyunsaturated free fatty acids such as omega-3, also inhibit high-fat/high-sucrose-induced inflammation in adipose tissue via GPR120 (Yamada et al., 2017). Indeed, GPR120 dysfunction leads to obesity in mice, and the genetic mutation of GPR120, which inhibits GPR120 signaling activity, has been observed in obese children and adults (Ichimura et al., 2012). Tanagho and Shohdy (2016) evaluated GPR120 as a potential target receptor for the treatment of obesity. In the present study, we found that LS and PT increased the level of GPR120 compared to that in the HFD group. Moreover, improvements in adipose tissue inflammation were observed following administration of LS and PT in the HFD model. These results suggest that the effects of LS and PT administration on GPR120 and adipose tissue inflammation may be a possible mechanism of anti-obesity effect of LS and PT. We measured GPR120 in colon tissue; however, it is necessary to conduct further studies to confirm the expression level of GPR120 in adipose tissue, and to clarify the association between GPR120 in adipose tissue and drugs mediated by the gut microbiota. In addition, further studies on morphological changes of mice colon or cecum will also help to understand the relationship between anti-obesity drugs and gut microbiota.
In this study, LS and PT administration did not affect GPR41 and GPR43. We only investigate the mRNA levels of GPR41 and GPR43 using RT-qPCR, but the Western blot is also widely used and may help to better understand the effect of LS and PT on GPR41 and GPR43. Further studies using various approaches including the Western blot are needed.
Next, we found that Eubacterium_coprostanoligenes_group was increased after LS and PT administration. Furthermore, ASV3566, corresponding to Eubacterium_coprostanoligenes_group, showed a significant correlation with GPR120, GPT, and TG. A recent study reported that Eubacterium_coprostanoligenes_group is one of the hub genera in the fecal micro-ecosystem of HFD-fed subjects and mediates the effect of HFD on dyslipidemia through sphingosine (Wei et al., 2021). The study also found an association of Eubacterium_coprostanoligenes_group with host lipid metabolism, such as GPT and TG. An HFD-fed mouse model showed a significant negative correlation of the Eubacterium coprostanoligenes group with changes in body weight, liver weight, and fasting glucose (Chen et al., 2021). Eubacterium coprostanoligenes was reported to reduce cholesterol to coprostanol (Freier et al., 1994). Oral administration of E. coprostanoligenes ATCC 51222 lowered the cholesterol concentration in germ-free mice and rabbits (Li et al., 1995, 1998). Overall, we presumed that enriching Eubacterium_coprostanoligenes_group and ASV3566 might contribute to the anti-obesity effect by participating in the regulation of GPR120 and metabolic disorders. Nevertheless, simple correlation result of this study may not be sufficient to establish the association between the anti-obesity effect of LS and PT on gut microbiota and GPR120. Further studies such as using Gpr120−/− mice will be needed to elucidate sufficient associations among drugs, gut microbiota, and GPR120.
Treatment with LS for 1 year led to significant reductions in waist circumference and inflammatory markers such as high-sensitivity C-reactive protein and fibrinogen compared to placebo treatment (Redman and Ravussin, 2010). Treatment with PT for 13 weeks also led to significant reductions in waist circumference compared to placebo treatment (Kim et al., 2006). However, PT had an inflammatory effect. Moreover, the effects of LS and PT on gut microbiota have not been studied in humans. Further human studies identifying the effect of LS and PT on gut microbiota and their association with changes in fat deposition and inflammation would provide a more in-depth understanding of drug metabolism and involvement of gut microbiota in human metabolism. In addition, energy expenditure and activity levels were not measured in this study. These need to be measured in future studies. Research confirming the efficacy of various drugs using a mouse model can provide an important basis for applying drugs for therapeutic use in humans, and evidence for understanding drug metabolism.
In summary, we investigated the relationship between anti-obesity drugs and the gut microbiota. Our results demonstrated that anti-obesity drugs LS and PT induced changes in the gut microbiota composition in the HFD-induced obese mouse model. LS and PT have anti-obesity effects independent of the gut microbiota; however, some indicators such as visceral fat and GPT are affected by gut microbiota. In addition, LS and PT increase the level of GPR120 and alleviate inflammation in adipose tissue, which is influenced by the gut microbiota. Thus, we propose that anti-obesity drugs can affect the gut microbiota and that the synergistic actions of the gut microbiota can be expected to have an anti-obesity effect.
Data availability statement
The datasets presented in this study can be found in online repositories. The names of the repository/repositories and accession number(s) can be found at: https://www.ddbj.nig.ac.jp/, DRA013284.
Ethics statement
The animal study was reviewed and approved by the Institutional Animal Care and Use Committee (IACUC-2019-06187) of Dongguk University Ilsan Hospital.
Author contributions
E-JS: formal analysis, visualization, and writing—original draft. NS: formal analysis and writing—original draft. SJ: resource and supervision. Y-DN and HK: conceptualization, supervision, and writing—review and editing. All authors contributed to the article and approved the submitted version.
Funding
This work was supported by the Main Research Program of the Korea of Food Research Institute (KFRI) funded by the Ministry of Science and ICT (grant number E0170600-06), by National Research Fund of Korean Government (grant number 2019R1A6A3A01096837), and by a grant of the Korea Health Technology R&D Project through the Korea Health Industry Development Institute (KHIDI), funded by the Ministry of Health and Welfare, Republic of Korea (grant number HF20C0020).
Conflict of interest
The authors declare that the research was conducted in the absence of any commercial or financial relationships that could be construed as a potential conflict of interest.
Publisher’s note
All claims expressed in this article are solely those of the authors and do not necessarily represent those of their affiliated organizations, or those of the publisher, the editors and the reviewers. Any product that may be evaluated in this article, or claim that may be made by its manufacturer, is not guaranteed or endorsed by the publisher.
Supplementary material
The Supplementary material for this article can be found online at: https://www.frontiersin.org/articles/10.3389/fmicb.2022.1109651/full#supplementary-material
Footnotes
References
An, Y., Li, Y., Wang, X., Chen, Z., Xu, H., Wu, L., et al. (2018). Cordycepin reduces weight through regulating gut microbiota in high-fat diet-induced obese rats. Lipids Health Dis. 17:276. doi: 10.1186/s12944-018-0910-6
Boulangé, C. L., Neves, A. L., Chilloux, J., Nicholson, J. K., and Dumas, M.-E. (2016). Impact of the gut microbiota on inflammation, obesity, and metabolic disease. Genome Med. 8:42. doi: 10.1186/s13073-016-0303-2
Caporaso, J. G., et al. (2010). QIIME allows analysis of high-throughput community sequencing data. Nat. Methods 7, 335–336. doi: 10.1038/nmeth.f.303
Chávez-Carbajal, A., Nirmalkar, K., Perez-Lizaur, A., Hernandez-Quiroz, F., Ramirez-Del-Alto, S., Garcia-Mena, J., et al. (2019). Gut microbiota and predicted metabolic pathways in a sample of Mexican women affected by obesity and obesity plus metabolic syndrome. Int. J. Mol. Sci. 20:438. doi: 10.3390/ijms20020438
Chen, Y.-T., Hsu, A.-H., Chiou, S.-Y., Lin, Y.-C., and Lin, J.-S. (2021). AB-kefir reduced body weight and ameliorated inflammation in adipose tissue of obese mice fed a high-fat diet, but not a high-sucrose diet. Nutrients 13:2182. doi: 10.3390/nu13072182
Cohen, L. J., Esterhazy, D., Kim, S. H., Lemetre, C., Aguilar, R. R., Gordon, E. A., et al. (2017). Commensal bacteria make GPCR ligands that mimic human signalling molecules. Nature 549, 48–53. doi: 10.1038/nature23874
Fernandes, J., Su, W., Rahat-Rozenbloom, S., Wolever, T. M. S., and Comelli, E. M. (2014). Adiposity, gut microbiota and faecal short chain fatty acids are linked in adult humans. Nutr. Diabetes 4:e121. doi: 10.1038/nutd.2014.23
Fernández-Sánchez, A., Madrigal-Santillán, E., Bautista, M., Esquivel-Soto, J., Morales-González, A., Esquivel-Chirino, C., et al. (2011). Inflammation, oxidative stress, and obesity. Int. J. Mol. Sci. 12, 3117–3132. doi: 10.3390/ijms12053117
Freier, T. A., Beitz, D. C., Li, L., Hartman, P. A., et al. (1994). Characterization of Eubacterium coprostanoligenes sp. nov., a cholesterol-reducing anaerobe. Int. J. Syst. Bacteriol. 44, 137–142. doi: 10.1099/00207713-44-1-137
Goffredo, M., Mass, K., Parks, E. J., Wagner, D. A., McClure, E. A., Graf, J., et al. (2016). Role of gut microbiota and short chain fatty acids in modulating energy harvest and fat partitioning in youth. J. Clin. Endocrinol. Metab. 101, 4367–4376. doi: 10.1210/jc.2016-1797
Gunawardena, H. P., Silva, K. D. R. R., Sivakanesan, R., and Katulanda, P. (2019). Increased lipid peroxidation and erythrocyte glutathione peroxidase activity of patients with type 2 diabetes mellitus: implications for obesity and central obesity. Obesity Med. 15:100118. doi: 10.1016/j.obmed.2019.100118
Ichimura, A., Hirasawa, A., Poulain-Godefroy, O., Bonnefond, A., Hara, T., Yengo, L., et al. (2012). Dysfunction of lipid sensor GPR120 leads to obesity in both mouse and human. Nature 483, 350–354. doi: 10.1038/nature10798
Indiani, C., Rizzardi, K. F., Castelo, P. M., Ferraz, L. F. C., Darrieux, M., and Parisotto, T. M. (2018). Childhood obesity and Firmicutes/Bacteroidetes ratio in the gut microbiota: a systematic review. Child. Obes. 14, 501–509. doi: 10.1089/chi.2018.0040
Kameyama, K., and Itoh, K. (2014). Intestinal colonization by a Lachnospiraceae bacterium contributes to the development of diabetes in obese mice. Microbes Environ. 29, 427–430. doi: 10.1264/jsme2.ME14054
Kan, J., Chen, C., Huo, T., Xie, W., Hui, Y., Liu, J., et al. (2020). Polyphenolic-enriched peach peels extract regulates lipid metabolism and improves the gut microbiota composition in high fat diet-fed mice. J. Funct. Foods 72:104082. doi: 10.1016/j.jff.2020.104082
Kang, M. J., Kim, H. G., Kim, J. S., Oh, D. G., Um, Y. J., Seo, C. S., et al. (2013). The effect of gut microbiota on drug metabolism. Expert Opin. Drug Metab. Toxicol. 9, 1295–1308. doi: 10.1517/17425255.2013.807798
Ke, J., An, Y., Cao, B., Lang, J., Wu, N., and Zhao, D. (2020). Orlistat-induced gut microbiota modification in obese mice. Evid. Based Complement. Alternat. Med. 2020:9818349. doi: 10.1155/2020/9818349
Kim, K. K., Cho, H. J., Kang, H. C., Youn, B. B., and Lee, K. R. (2006). Effects on weight reduction and safety of short-term phentermine administration in Korean obese people. Yonsei Med. J. 47, 614–625. doi: 10.3349/ymj.2006.47.5.614
Kim, G. W., Lin, J. E., Blomain, E. S., and Waldman, S. A. (2014). Antiobesity pharmacotherapy: new drugs and emerging targets. Clin. Pharmacol. Ther. 95, 53–66. doi: 10.1038/clpt.2013.204
Lee, S. M., Cho, Y. H., Lee, S. Y., Jeong, D. W., Cho, A. R., Jeon, J. S., et al. (2015). Urinary malondialdehyde is associated with visceral abdominal obesity in middle-aged men. Mediat. Inflamm. 2015:524291. doi: 10.1155/2015/524291
Ley, R. E., Turnbaugh, P. J., Klein, S., and Gordon, J. I. (2006). Human gut microbes associated with obesity. Nature 444, 1022–1023. doi: 10.1038/4441022a
Li, L., Buhman, K. K., Hartman, P. A., and Beitz, D. C. (1995). Hypocholesterolemic effect of Eubacterium coprostanoligenes ATCC 51222 in rabbits. Lett. Appl. Microbiol. 20, 137–140. doi: 10.1111/j.1472-765X.1995.tb00410.x
Li, L., Batt, S. M., Wannemuehler, M., Dispirito, A., and Beitz, D. C. (1998). Effect of feeding of a cholesterol-reducing bacterium, Eubacterium coprostanoligenes, to germ-free mice. Lab. Anim. Sci. 48, 253–255.
Melhem, H., Kaya, B., Ayata, C. K., Hruz, P., and Niess, J. H. (2019). Metabolite-sensing G protein-coupled receptors connect the diet-microbiota-metabolites Axis to inflammatory bowel disease. Cells 8:450. doi: 10.3390/cells8050450
Montandon, S. A., and Jornayvaz, F. R. (2017). Effects of antidiabetic drugs on gut microbiota composition. Genes 8:250. doi: 10.3390/genes8100250
Morrison, D. J., and Preston, T. (2016). Formation of short chain fatty acids by the gut microbiota and their impact on human metabolism. Gut Microbes. 7, 189–200. doi: 10.1080/19490976.2015.1134082
Murphy, E. F., Cotter, P. D., Healy, S., Marques, T. M., O'Sullivan, O., Fouhy, F., et al. (2010). Composition and energy harvesting capacity of the gut microbiota: relationship to diet, obesity and time in mouse models. Gut 59, 1635–1642. doi: 10.1136/gut.2010.215665
Murugesan, S., Ulloa-Martínez, M., Martínez-Rojano, H., Galván-Rodríguez, F. M., Miranda-Brito, C., Romano, M. C., et al. (2015). Study of the diversity and short-chain fatty acids production by the bacterial community in overweight and obese Mexican children. Eur. J. Clin. Microbiol. Infect. Dis. 34, 1337–1346. doi: 10.1007/s10096-015-2355-4
Muscogiuri, G., Cantone, E., Cassarano, S., Tuccinardi, D., Barrea, L., Savastano, S., et al. (2019). Gut microbiota: a new path to treat obesity. Int. J. Obes. Suppl. 9, 10–19. doi: 10.1038/s41367-019-0011-7
National Research Council (2011). Guide for the Care and Use of Laboratory Animals (8th) Edn. Washington, DC: The National Academies Press.
Oh, D. Y., Talukdar, S., Bae, E. J., Imamura, T., Morinaga, H., Fan, W. Q., et al. (2010). GPR120 is an omega-3 fatty acid receptor mediating potent anti-inflammatory and insulin-sensitizing effects. Cells 142, 687–698. doi: 10.1016/j.cell.2010.07.041
Palmas, V., Pisanu, S., Madau, V., Casula, E., Deledda, A., Cusano, R., et al. (2021). Gut microbiota markers associated with obesity and overweight in Italian adults. Sci. Rep. 11:5532. doi: 10.1038/s41598-021-84928-w
Pascale, A., Marchesi, N., Govoni, S., Coppola, A., and Gazzaruso, C. (2019). The role of gut microbiota in obesity, diabetes mellitus, and effect of metformin: new insights into old diseases. Curr. Opin. Pharmacol. 49, 1–5. doi: 10.1016/j.coph.2019.03.011
Rahat-Rozenbloom, S., Fernandes, J., Gloor, G. B., and Wolever, T. M. S. (2014). Evidence for greater production of colonic short-chain fatty acids in overweight than lean humans. Int. J. Obes. 38, 1525–1531. doi: 10.1038/ijo.2014.46
Redman, L. M., and Ravussin, E. (2010). Lorcaserin for the treatment of obesity. Drugs Today (Barcelona, Spain: 1998) 46, 901–910. doi: 10.1358/dot.2010.46.12.1556433
Ricaboni, D., Mailhe, M., Cadoret, F., Vitton, V., Fournier, P. E., and Raoult, D. (2016). 'Colidextribacter massiliensis' gen. Nov., sp. nov., isolated from human right colon. New Microbes New Infect. 17, 27–29. doi: 10.1016/j.nmni.2016.11.023
Schwiertz, A., Taras, D., Schäfer, K., Beijer, S., Bos, N. A., Donus, C., et al. (2010). Microbiota and SCFA in lean and overweight healthy subjects. Obesity 18, 190–195. doi: 10.1038/oby.2009.167
Segata, N., Izard, J., Waldron, L., Gevers, D., Miropolsky, L., Garrett, W. S., et al. (2011). Metagenomic biomarker discovery and explanation. Genome Biol. 12:1. doi: 10.1186/gb-2011-12-6-r60
Sell, H., Habich, C., and Eckel, J. (2012). Adaptive immunity in obesity and insulin resistance. Nat. Rev. Endocrinol. 8, 709–716. doi: 10.1038/nrendo.2012.114
Shin, N.-R., Lee, J. C., Lee, H. Y., Kim, M. S., Whon, T. W., Lee, M. S., et al. (2014). An increase in the Akkermansia spp. population induced by metformin treatment improves glucose homeostasis in diet-induced obese mice. Gut 63, 727–735. doi: 10.1136/gutjnl-2012-303839
Song, T., Yang, Y., Zhou, Y., Wei, H., and Peng, J. (2017). GPR120: a critical role in adipogenesis, inflammation, and energy metabolism in adipose tissue. Cell. Mol. Life Sci. 74, 2723–2733. doi: 10.1007/s00018-017-2492-2
Song, X., Zhong, L., Lyu, N., Liu, F., Li, B., Hao, Y., et al. (2019). Inulin can alleviate metabolism disorders in Ob/Ob mice by partially restoring leptin-related pathways mediated by gut microbiota. Genomics Proteomics Bioinformatics 17, 64–75. doi: 10.1016/j.gpb.2019.03.001
Song, E.-J., Shin, N. R., Jeon, S., Nam, Y. D., and Kim, H. (2022). Impact of the herbal medicine, Ephedra sinica stapf, on gut microbiota and body weight in a diet-induced obesity model. Front. Pharmacol. 13:1042833. doi: 10.3389/fphar.2022.1042833
Stojanov, S., Berlec, A., and Štrukelj, B. (2020). The influence of probiotics on the Firmicutes/Bacteroidetes ratio in the treatment of obesity and inflammatory bowel disease. Microorganisms 8:1715. doi: 10.3390/microorganisms8111715
Tanagho, P. A., and Shohdy, K. S. (2016). GPR 120: the potential target for obesity treatment. Endocr. Metab. Immune Disord. Drug Targets 16, 8–11. doi: 10.2174/1871530316666151123115611
Turnbaugh, P. J., Ley, R. E., Mahowald, M. A., Magrini, V., Mardis, E. R., and Gordon, J. I. (2006). An obesity-associated gut microbiome with increased capacity for energy harvest. Nature 444, 1027–1031. doi: 10.1038/nature05414
Van Der Hee, B., and Wells, J. M. (2021). Microbial regulation of host physiology by short-chain fatty acids. Trends Microbiol. 29, 700–712. doi: 10.1016/j.tim.2021.02.001
Vijay-Kumar, M., Aitken, J. D., Carvalho, F. A., Cullender, T. C., Mwangi, S., Srinivasan, S., et al. (2010). Metabolic syndrome and altered gut microbiota in mice lacking toll-like receptor 5. Science 328, 228–231. doi: 10.1126/science.1179721
Vila, A.V., Collij, V., Sanna, S., Sinha, T., Imhann, F., Bourgonje, A. R., et al. (2020). Impact of commonly used drugs on the composition and metabolic function of the gut microbiota. Nat. Commun. 11, 1–11. doi: 10.1038/s41467-019-14177-z
Wang, Y., Yao, W., Li, B., Qian, S., Wei, B., Gong, S., et al. (2020). Nuciferine modulates the gut microbiota and prevents obesity in high-fat diet-fed rats. Exp. Mol. Med., 52, 1959–1975, doi: 10.1038/s12276-020-00534-2 PMC8080667
Wang, M., Zhang, S., Zhong, R., Wan, F., Chen, L., Liu, L., et al. (2021). Olive fruit extracts supplement improve antioxidant capacity via altering colonic microbiota composition in mice. Front. Nutr. 8:645099. doi: 10.3389/fnut.2021.645099
Wei, W., Jiang, W., Tian, Z., Wu, H., Ning, H., Yan, G., et al. (2021). Fecal g. Streptococcus and g. Eubacterium_coprostanoligenes_group combined with sphingosine to modulate the serum dyslipidemia in high-fat diet mice. Clin. Nutr. 40, 4234–4245. doi: 10.1016/j.clnu.2021.01.031
Wilson, I., and Nicholson, J. (2009). The role of gut microbiota in drug response. Curr. Pharm. Des. 15, 1519–1523. doi: 10.2174/138161209788168173
Yamada, H., Umemoto, T., Kakei, M., Momomura, S. I., Kawakami, M., Ishikawa, S. E., et al. (2017). Eicosapentaenoic acid shows anti-inflammatory effect via GPR120 in 3T3-L1 adipocytes and attenuates adipose tissue inflammation in diet-induced obese mice. Nutr. Metab. 14:33. doi: 10.1186/s12986-017-0188-0
Yesilbursa, D., Serdar, Z., Serdar, A., Sarac, M., Coskun, S., and Jale, C. (2005). Lipid peroxides in obese patients and effects of weight loss with orlistat on lipid peroxides levels. Int. J. Obes. 29, 142–145. doi: 10.1038/sj.ijo.0802794
Zeng, H., Ishaq, S. L., Zhao, F. Q., and Wright, A. D. G. (2016). Colonic inflammation accompanies an increase of β-catenin signaling and Lachnospiraceae/Streptococcaceae bacteria in the hind gut of high-fat diet-fed mice. J. Nutr. Biochem. 35, 30–36. doi: 10.1016/j.jnutbio.2016.05.015
Keywords: obesity, anti-obesity drug, gut microbiota, lorcaserin, phentermine
Citation: Song E-J, Shin NR, Jeon S, Nam Y-D and Kim H (2023) Lorcaserin and phentermine exert anti-obesity effects with modulation of the gut microbiota. Front. Microbiol. 13:1109651. doi: 10.3389/fmicb.2022.1109651
Edited by:
Jing-hua Wang, Daejeon University, South KoreaReviewed by:
Yingying Liu, University of North Dakota, United StatesChenhong Zhang, Shanghai Jiao Tong University, China
Copyright © 2023 Song, Shin, Jeon, Nam and Kim. This is an open-access article distributed under the terms of the Creative Commons Attribution License (CC BY). The use, distribution or reproduction in other forums is permitted, provided the original author(s) and the copyright owner(s) are credited and that the original publication in this journal is cited, in accordance with accepted academic practice. No use, distribution or reproduction is permitted which does not comply with these terms.
*Correspondence: Hojun Kim, ✉ a2lta2xhckBkb25nZ3VrLmFjLmty; Young-Do Nam, ✉ eW91bmdkbzk4QGtmcmkucmUua3I=; Songhee Jeon, ✉ anNvbmcwMzA0QGhhbm1haWwubmV0
†These authors have contributed equally to this work