- Department of Microbiology and Parasitology, Faculty of Pharmacy, University of Sevilla, Sevilla, Spain
Hypersaline soils are extreme environments that have received little attention until the last few years. Their halophilic prokaryotic population seems to be more diverse than those of well-known aquatic systems. Among those inhabitants, representatives of the family Balneolaceae (phylum Balneolota) have been described to be abundant, but very few members have been isolated and characterized to date. This family comprises the genera Aliifodinibius and Fodinibius along with four others. A novel strain, designated 1BSP15-2V2T, has been isolated from hypersaline soils located in the Odiel Saltmarshes Natural Area (Southwest Spain), which appears to represent a new species related to the genus Aliifodinibius. However, comparative genomic analyses of members of the family Balneolaceae have revealed that the genera Aliifodinibius and Fodinibius belong to a single genus, hence we propose the reclassification of the species of the genus Aliifodinibius into the genus Fodinibius, which was first described. The novel strain is thus described as Fodinibius salsisoli sp. nov., with 1BSP15-2V2T (=CCM 9117T = CECT 30246T) as the designated type strain. This species and other closely related ones show abundant genomic recruitment within 80–90% identity range when searched against several hypersaline soil metagenomic databases investigated. This might suggest that there are still uncultured, yet abundant closely related representatives to this family present in these environments. In-depth in-silico analysis of the metabolism of Fodinibius showed that the biotin biosynthesis pathway was present in the genomes of strain 1BSP15-2V2T and other species of the family Balneolaceae, which could entail major implications in their community role providing this vitamin to other organisms that depend on an exogenous source of this nutrient.
1. Introduction
The family Balneolaceae (Xia et al., 2016) belongs to the phylum Balneolota, class Balneolia (Munoz et al., 2016), and includes six genera: Aliifodinibius, Balneola, Fodinibius, Gracilimonas, Halalkalibaculum, and Rhodohalobacter (Parte et al., 2020), which are found in marine and hypersaline environments, such as deep-sea sediments, salt-mines, salterns, and saline soils (Urios et al., 2006; Choi et al., 2009; Wang et al., 2012, 2013; Cho et al., 2013; Xia et al., 2017; Wu et al., 2022a,b). The genus Fodinibius was proposed in 2012 based on a single species, Fodinibius salinus (Wang et al., 2012) and the genus Aliifodinibius was firstly described only one year later (Wang et al., 2013), including at that time two species, Aliifodinibius roseus (type species) and Aliifodinibius sediminis. These taxa shared low 16S rRNA gene sequence identities (<92.4%) with F. salinus, the most closely related species. However, no further genomic comparison was performed at that time. The aforementioned identity values, along with their differential fatty acids and polar lipids profiles, as well as some phenotypic features, were considered enough evidence to propose that A. roseus and A. sediminis represented a novel genus, different from Fodinibius. At the time of writing, five species names have been validly published within the genus Aliifodinibius: Aliifodinibius roseus and Aliifodinibius sediminis (Wang et al., 2013), Aliifodinibius halophilus (Xia et al., 2016), Aliifodinibius salicampi (Cho et al., 2017, 2018), and Aliifodinibius saliphilus (Cho and Whang, 2020). Besides, the species “Aliifodinibius salipaludis” (Zhao et al., 2020) has been described but its name has not been validly published so far. All these species were isolated from hypersaline environments, such as salt mines, salterns, and saline soils. They are Gram-stain-negative, rod-shaped, non-motile, and moderately halophilic bacteria, with a NaCl requirement for growing between 4 and 25% (w/v) and an optimum between 8 and 15%, except for “A. salipaludis,” that can grow optimally at 25% (w/v) NaCl (Zhao et al., 2020). The pH values supporting their growth range between pH 6.0 and 11.0, with an optimum at pH 7.0–8.0. They are able to produce catalase and oxidase but unable to hydrolyze starch and DNA (Wang et al., 2013; Xia et al., 2016; Cho et al., 2017; Cho and Whang, 2020; Zhao et al., 2020).
The Odiel Saltmarshes Natural Area is located in the estuary of the Odiel and Tinto rivers (Southwest Spain), and its hypersaline soils have been previously explored by Vera-Gargallo and Ventosa (2018). They obtained two metagenomes database of these area named SMO1 and SMO2. Their shotgun metagenomic analysis taxonomically annotated almost a 20% of the prokaryotic diversity as representatives of the phylum Balneolota. Genus-level taxonomic affiliation of 16S rRNA genes detected in the reads above 95% identity assigned 9.0 and 3.4% of the abundance to the genera Fodinibius and Gracilimonas, respectively, for the SMO1 metagenomic database, and 3.0 and 1.6% for the SMO2 metagenomic database. Other studies have also revealed the presence of this taxonomic group on different metagenomic datasets. The abundance of the uncultured members of the class Balneolia was up to 8% in the hypersaline soda lake sediment studied by Vavourakis et al. (2018), being one of the three predominant bacterial groups along with the phylum Bacillota and the class Gammaproteobacteria. Both studies recovered MAGs related to uncultured members of the family Balneolaceae (Vavourakis et al., 2018; Vera-Gargallo and Ventosa, 2018). Kimbrel et al. (2018) obtained a medium-quality MAG related to Gracilimonas tropica, with a 0.26% of abundance, from sediments with salinity of 7.5%. The thalassohaline brines located in Maras (Peru) showed a considerable abundance of up to 6% of Balneolota, mainly associated (5% out of the total) to the species A. roseus (Castelán-Sánchez et al., 2019). These previous studies indicate that the members of the family Balneolaceae are fairly abundant in hypersaline ecosystems, although the number of cultivated species is still scarce.
In the present study, we describe the isolation of a new strain, following a culturomic strategy, and we have carried out its exhaustive description as a novel species within the family Balneolaceae. Besides, we completed the phylogenomic analyses of the whole family, which revealed the need for merging two of its genera. Furthermore, we performed genome-based functional analyses of these bacteria, including the vitamin B7 pathway, to elucidate their potential ecological role and adaptative mechanisms to survive under harsh conditions of salinity and heavy metals, along with its distribution in hypersaline habitats.
2. Materials and methods
2.1. Strain isolation and physico-chemical features of the sampling site
The samples were collected from hypersaline soils located at the saltmarshes of the Odiel Natural Area in Huelva (Southwest Spain) as previously described (Vera-Gargallo and Ventosa, 2018; Vera-Gargallo et al., 2019). The pH and electrical conductivity of the saline soil was measured with the pHmeter CRISON BASIC 20 and the conductometer CRISON 35+, respectively, after a 1:5 dilution. Arsenic and cadmium concentrations were measured by Inductively Coupled Plasma Mass Spectrometry (ICP-MS); copper and zinc concentrations, by Inductively Coupled Plasma Optical Emission Spectroscopy (ICP-OES); and lead concentration, by atomic absorption spectroscopy. All those spectrometric assays were carried out by Innoagral Laboratories (Mairena del Aljarafe, Sevilla, Spain).
The strain 1BSP15-2V2T was isolated using the standard dilution-plating technique and grew on a low-nutrient medium enriched with pyruvate (SMM medium) at 28°C after 3 months of incubation. The colonies were subcultured until a pure culture was obtained. The composition of the low nutrients SMM medium was the following (g L−1): casein digest, 5.0; sodium pyruvate, 1.1; supplemented with up to 15% (w/v) salt concentration from a seawater (SW) stock ([g L−1] NaCl, 234.0; MgCl2·6H2O, 39.0; MgSO4·7H2O, 61.0; CaCl2, 1.0; KCl, 6.0; NaHCO3, 0.2; NaBr, 0.7); the pH was adjusted to 7.5; solidified with 2.0% (w/v) agar when needed. This medium was first used by León et al. (2016) to isolate the species of the genus Spiribacter, which is an abundant halophilic bacterium of the salterns community at intermediate salinities. The culture was preserved at −80°C in the same liquid medium with 40% (v/v) glycerol. Additionally, Marine Agar (MA, Difco 2216) medium, supplemented with 10% (w/v) NaCl, was used to determine the fatty acid profile of this strain for better comparative purposes with other species of the genus under study. In addition, reference type strains of the species of the genus Aliifodinibius were used for comparative phenotypic analyses. The following strains were obtained from the corresponding culture collections: A. halophilus KCTC 42497T, A. roseus DSM 21986T, A. salicampi KACC 19060T, “A. salipaludis” KCTC 52855T, A. saliphilus KACC 19126T, and A. sediminis DSM 21194T. These strains were grown and preserved in R2A medium supplemented with 7.5% (w/v) SW. Additionally, DNA of strain A. salicampi KACC 19060T was extracted and sequenced as detailed below as it was the only species of the genus Aliifodinibius whose genome was not publicly available.
2.2. DNA extraction and PCR amplification
Genomic DNA of strain 1BSP15-2V2T was extracted using the Marmur (1961) method modified for small volumes. The almost complete 16S rRNA gene sequence was amplified by PCR with universal primers 27F (5′-AGA GTT TGA TCM TGG CTC AG-3′) and 1492R (5′-GGT TAC CTT GTT ACG ACT T3′) (Lane, 1991). Both genomic DNA and PCR products were purified using MEGAquick-spin™ plus (iNtRON Biotechnology), following the manufacturer’s recommendations. The 16S rRNA gene was sequenced using Sanger technology by StabVida (Caparica, Portugal). The same protocol was followed for the DNA extraction of strain A. salicampi KACC 19060T.
2.3. Phylogenetic analysis
Identification of phylogenetic neighbors of strain 1BSP15-2V2T according to the 16S rRNA gene sequence identity was carried out searching the EzBioCloud database1 (Yoon et al., 2017). For further phylogenetic analysis, the type strains of the identified closely related taxa of the family Balneolaceae were used. The 16S rRNA gene sequences of the strains included in the analysis were obtained from SILVA and GenBank databases. Alignment of the primary and secondary structures of the selected 16S rRNA sequences was performed using the integrated fast aligner implemented in the ARB package (Ludwig et al., 2004). For phylogenetic tree reconstruction, maximum-likelihood (Felsenstein, 1981), maximum-parsimony (Felsenstein, 1983), and neighbor-joining (Saitou and Nei, 1987) algorithms integrated in the ARB software (Ludwig et al., 2004) were used, and the Jukes-Cantor model (Jukes and Cantor, 1969) was selected to correct the distance matrix. The robustness of the branch topology was checked by bootstrap analysis (1,000 pseudoreplicates) (Felsenstein, 1985). The script “gitana”2 was employed for formatting and imaging of the tree visualization.
2.4. Comparative genomic analyses
Whole shotgun sequencing of the genome of strain 1BSP15-2V2T was performed by Novogene Europe (Cambridge, United Kingdom) on the Illumina NovaSeq PE150 platform. Paired end reads were assembled with SPAdes v3.13.0 (Prjibelski et al., 2020). Quality control of the assembly was performed with QUAST v2.3 (Gurevich et al., 2013), which also calculated some genomic statistics, such as the DNA G + C content. The length-filtered scaffolds were screened by using Prodigal v2.60 (Hyatt et al., 2010), to extract the nucleotide coding sequences as well as their protein translation, and Prokka v1.12 (Seemann, 2014) for the standard GenBank annotation. CheckM v1.0.5 (Parks et al., 2015) allow us to verify genome completeness and contamination. The same pipeline was followed for the genome sequencing and analysis of strain A. salicampi KACC 19060T. Additionally, the available draft genomes of the type strains of species of the genera Aliifodinibius, Balneola, Fodinibius, Gracilimonas, Halalkalibaculum, and Rhodohalobacter were used for comparative genomic analyses (Supplementary Table S1).
Isoelectric point calculation of predicted proteins was carried out using the “iep” program implemented in the EMBOSS package v6.5.7.0 (Rice et al., 2000), and plotted with the R package “ggplot2” v3.3.3 (Wickham, 2009). Meanwhile, the R package “fmsb” v0.7.3 (Nakazawa, 2022) was used for displaying the spider (or radar) graph of amino acid frequency. In both cases, colors were chosen from the wide selection of the R package “paletteer” v.1.4.0 (Hvitfeldt, 2021). Functional annotation was performed with the online tool BlastKOALA (Kanehisa et al., 2016). The KEGG Orthology numbers (KO), corresponding to KEGG pathways and KEGG modules, were assigned to the identified coding sequences, and then plotted in a heatmap to identify the most abundant functions. KO definitions were manually inspected and compared among the species of the family Balneolaceae.
Following the proposed minimal standards for prokaryotic taxonomy (Chun et al., 2018), we used different Overall Genome Relatedness Indexes (OGRIs) to determine the status of strain 1BSP15-2V2T within the family Balneolaceae. Average Amino acid Identity (AAI) and Average Nucleotide Identity for orthologous sequences (orthoANI) were calculated using the Enveomics toolbox (Rodriguez-R and Konstantinidis, 2016) and OAU software v1.2 (Lee et al., 2016), respectively. For digital DNA–DNA hybridization (dDDH), the Genome-to-Genome Distance Calculator bioinformatic tool (GGDC v3.0) available from the Leibniz Institute DSMZ (Meier-Kolthoff et al., 2021) was used. For a more reliable determination of the relationship among the members of the Balneolaceae, a phylogenomic tree based on the core-proteome sequences of those strains was built. The orthologous protein clusters were identified after BLASTp v2.2.28+ search and extracted from the proteome by using the Markov Cluster Algorithm, as implemented in the Enveomics toolbox (Rodriguez-R and Konstantinidis, 2016). Muscle v3.8.31 (Edgar, 2004) was employed to align the orthologous sequences. The phylogeny was calculated based on 1,049 concatenated protein sequences with the approximately maximum-likelihood algorithm implemented in FastTreeMP v2.1.8 (Price et al., 2010), considering the Jones-Taylor-Thornton model of amino acid evolution (Jones et al., 1992). Furthermore, the Shimodaira-Hasegawa test (Shimodaira and Hasegawa, 1999) was used to check the reliability of each node. The visualization of the final topology was performed with the online tool iTOL v6 (Letunic and Bork, 2021), and the intersection of the shared orthologous proteins among the species under study was calculated with the R package “UpSetR” v1.4.0 (Conway et al., 2017).
2.5. Ecological distribution
With the aim to determine the presence and ecological distribution in hypersaline environments of strain 1BSP15-2V2T, as well as those of the previously described members of the genera Aliifodinibius, Balneola, Fodinibius, Gracilimonas, Halalkalibaculum, and Rhodohalobacter, a fragment recruitment analysis was performed against different environmental metagenomic datasets (Supplementary Table S2) showing similar metadata features to avoid bias due to them. The rRNA gene sequences of each of the analyzed genomes were masked due to the highly conserved nature of those genomic regions. Subsequently, the ≥30 bp high-quality metagenomic reads were BLASTn v2.2.28+ searched against each of the query genomes.
2.6. Fatty acids composition and phenotypic characterization
For the determination of the fatty acid profile, strain 1BSP15-2V2T was grown on MA medium supplemented with 10% (w/v) NaCl at 37°C for 5 days. Fatty acids were determined by gas chromatography with an Agilent 6850 system at the Spanish Type Culture Collection (CECT), Valencia, Spain. The protocol recommended by the MIDI Microbial Identification System was followed (Sasser, 1990), using the TSBA6 library (MIDI, 2008).
Colonial morphology and pigmentation of strain 1BSP15-2V2T were observed in the same SMM medium used for isolation supplemented with 7.5% (w/v) SW solution at pH 7.5, after 5 days of incubation at 37°C. Cell morphology and motility were examined by phase contrast microscopy (Olympus CX41). Anaerobic growth was determined after incubation on the above-mentioned medium, conditions, and time, using the AnaeroGen™ system (Oxoid).
The range and optimal salt concentration, pH, and temperature requirements for strain 1BSP15-2V2T were determined by monitoring the optical density. Absorbance at 600 nm was measured every 2 h for 72 h using an Infinite M Nano (Tecan, Grödig, Austria) microplate reader. The incubation was set at 37°C with linear shaking. For determination of the salinity requirements, the SMM liquid medium was supplemented with SW stock to give a final salt concentration of 0 (in this case only distilled water was added), 3, 5, 6, 7.5, 9, 10, 12, 14, 15, 16, 17, 19, 20, 22, and 25% (w/v). To determine pH values supporting growth, the pH was adjusted to 3.0, 4.0, 5.0, 6.0, 7.0, 7.5, 8.0, 9.0, and 10.0 using a buffered system. Optimal temperature and range were ascertained in the same SMM liquid medium at the optimal salinity and pH values for the growth of strain 1BSP15-2V2T at different temperatures: 4, 13, 14, 15, 20, 28, 34, 37, 40, 42, 43, 44, and 45°C. In this case, the optical density was measured in a Spectronic 20D+ (ThermoSpectronics, Cambridge, United Kingdom).
In order to phenotypically characterize the new isolate, strain 1BSP15-2V2T was routinely grown in SMM medium supplemented with 7.5% (w/v) salts at pH 7.5 and 37°C for 5 days. However, the reference strains A. halophilus KCTC 42497T, A. roseus DSM 21986T, A. salicampi KACC 19060T, “A. salipaludis” KCTC 52855T, A. saliphilus KACC 19126T, and A. sediminis DSM 21194T were routinely grown in the medium recommended by the culture collections, that is, R2A medium supplemented with 7.5% (w/v) SW solution at the same pH, temperature, and time than those for strain 1BSP15-2V2T. All biochemical tests were accomplished under these culture conditions. Catalase activity was examined by adding a 3% (w/v) H2O2 solution to colonies (Cowan and Steel, 1965), and oxidase activity was determined with 1% (v/v) tetramethyl-p-phenylenediamine (Kovacs, 1956). The following tests were determined as described by Cowan and Steel (1965): hydrolysis of gelatin, starch, Tween 80, DNA, casein, and aesculin, production of indole, methyl red and Voges–Proskauer tests, Simmons’ citrate, nitrate, and nitrite reduction, H2S production, urease, and phenylalanine deaminase. Acid production from carbohydrates was determined using a modified phenol red base medium prepared with 7.5% (w/v) salts supplemented with 0.05% (w/v) yeast extract and 1% (w/v) of the filter-sterilized carbohydrate to be checked (Cowan and Steel, 1965; Ventosa et al., 1982). The medium described by Koser (1923), as modified by Ventosa et al. (1982), was used to assess the utilization of different substrates as sole carbon and energy sources or as sole carbon, nitrogen, and energy sources. The studied carbohydrates, alcohols, organic acids, and amino acids were added as filter-sterilized solutions to give a final concentration of 1 g L−1 for all of them, except for carbohydrates, whose final concentration was 2 g L−1.
3. Results and discussion
3.1. Heavy metal contamination of the studied samples
To our best knowledge there are no reference studies on the concentration of heavy metals in the hypersaline soils from the Odiel Saltmarshes National Area, but it is known that the Odiel river waters and sediments contain high concentrations of arsenic, cadmium, copper, lead, and zinc due to unregulated industrial and mining activities in the past (Sainz et al., 2002, 2004). The sampled hypersaline soil presented a pH value of 8.4 and an electrical conductivity of 24.57 mS/cm. The concentrations of arsenic, cadmium, copper, lead, and zinc were (mg kg−1): 14.86, 0.47, 106.40, 24.81, and 129.30, respectively. Arsenic, copper, and zinc presented values above the reference ranges for non-contaminated soils, according to the criteria of the Government of the region of Andalucía, where the Odiel Saltmarshes National Area is located (Consejería de Medio Ambiente, 1999), while cadmium and lead concentrations were within the reference ranges (Table 1).
3.2. The new isolated strain constitutes a novel species of the genus Fodinibius
The strain 1BSP15-2V2T was cultured in pure culture after an extensive screening of the prokaryotic diversity carried out in hypersaline soil samples obtained from the Odiel Saltmarshes Natural Area (Huelva, Southwest Spain), where more than 4,000 isolates were investigated. The 1,455 bp-long 16S rRNA gene sequence of this strain showed the closest relationship with A. roseus DSM 21986T and A. saliphilus ECH52T (95.02% sequence identity, in both cases). Reconstruction of the 16S rRNA gene-based phylogeny by the maximum-likelihood algorithm clustered strain 1BSP15-2V2T together to the species of the genus Aliifodinibius (Figure 1). The species Fodinibius salinus YIM D15T was also clustered within the other species of the genus Aliifodinibius, which suggests that the two genera are closely related. However, the bootstrap value for the node harboring strain 1BSP15-2V2T is below 70% and the topology differs sightly depending on the algorithms used (maximum-likelihood, maximum-parsimony, or neighbor-joining). A similar issue can be observed for the branch that accommodates Fodinibius salinus YIM D15T. Therefore, the robustness of this tree is not strong enough as to infer reliable relationship between the species of the genera Aliifodinibius and Fodinibius, and strain 1BSP15-2V2T, but offers an approach to their evolutionary history.
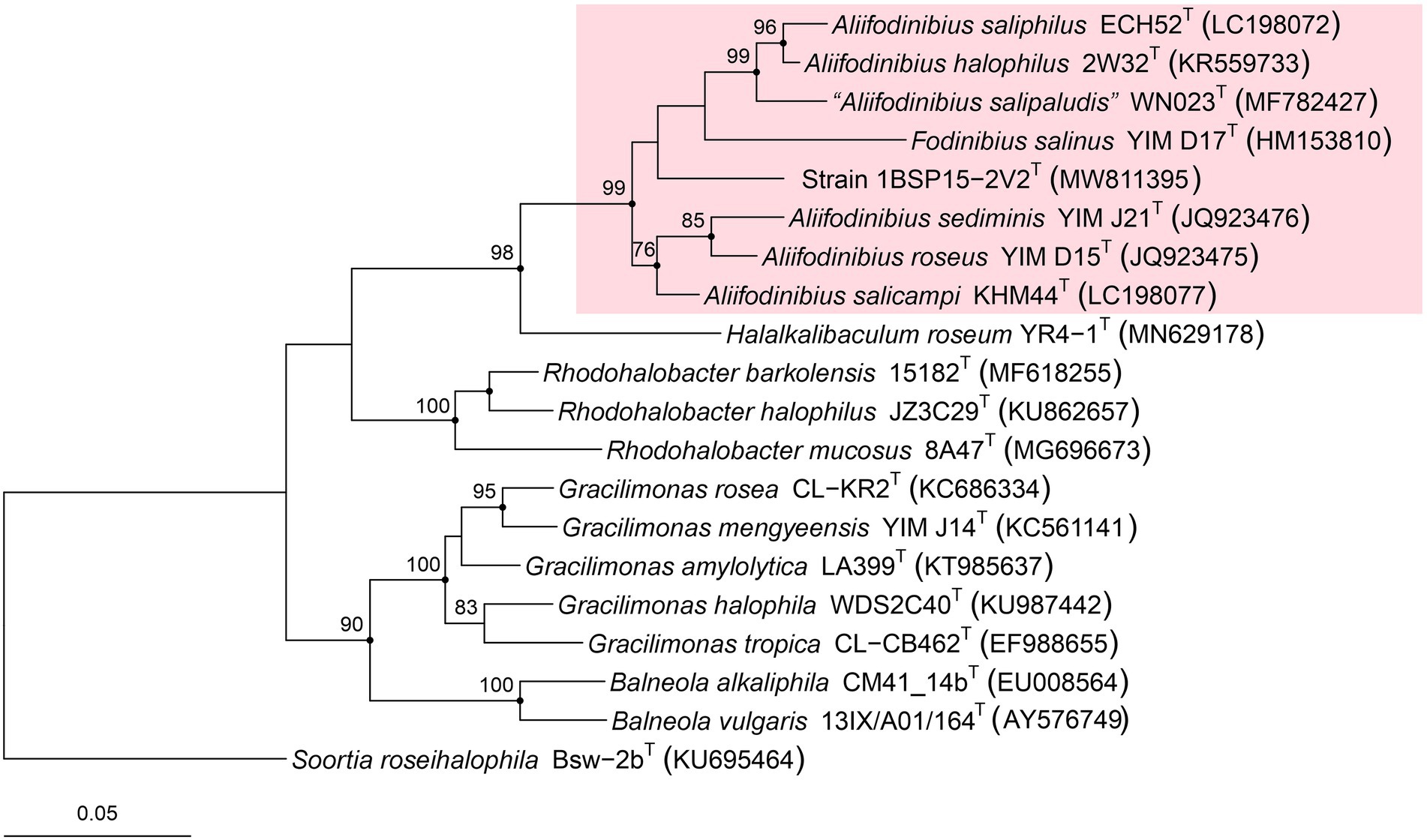
Figure 1. Maximum-likelihood phylogenetic tree based on the comparison of the 16S rRNA gene sequences showing the relationships among members of the family Balneolaceae. Bootstrap values equal or higher than 70% based on 1,000 pseudoreplicates are indicated at selected branch nodes. Filled circles indicate that the corresponding node was also obtained in the trees generated with the maximum-parsimony and neighbor-joining algorithms. The species Soortia roseihalophila, the closest relative to the family Balneolaceae, was selected as an outgroup. Bar, 0.05 substitutions per nucleotide position.
The draft genome sequence of strain 1BSP15-2V2T (GCF_026229185.1) was assembled into 43 contigs, with a total size of 4,850,852 bp, and a DNA G + C content of 44.47 mol%. We also obtained the genome sequence of the closely related species A. salicampi KACC 19060T (GCF_026228885.1), not previously available in public databases, which contains 13 contigs, slightly shorter size (3,937,193 bp), and a genomic G + C content of 42.75 mol%. The members of the genus Aliifodinibius possess genome sizes ranging from 3,583,276 bp (“A. salipaludis” WN023T) to 5,082,244 bp (A. roseus DSM 21986T) (Figure 2A; Supplementary Table S1). Fodinibius salinus DSM 21935T has one of the smallest genomes of the members within the family Balneolaceae (2,861,751 bp), but with similar G + C percentage than those of the genera Aliifodinibius, Gracilimonas, Halalkalibaculum, and Rhodohalobacter. On the contrary, the species of the genus Balneola, the type genus of the family Balneolaceae, have smaller average genome size than the rest of members of the family (except the genus Fodinibius), as well as the lowest G + C content (<40 mol%) (Figures 2A,B; Supplementary Table S1).
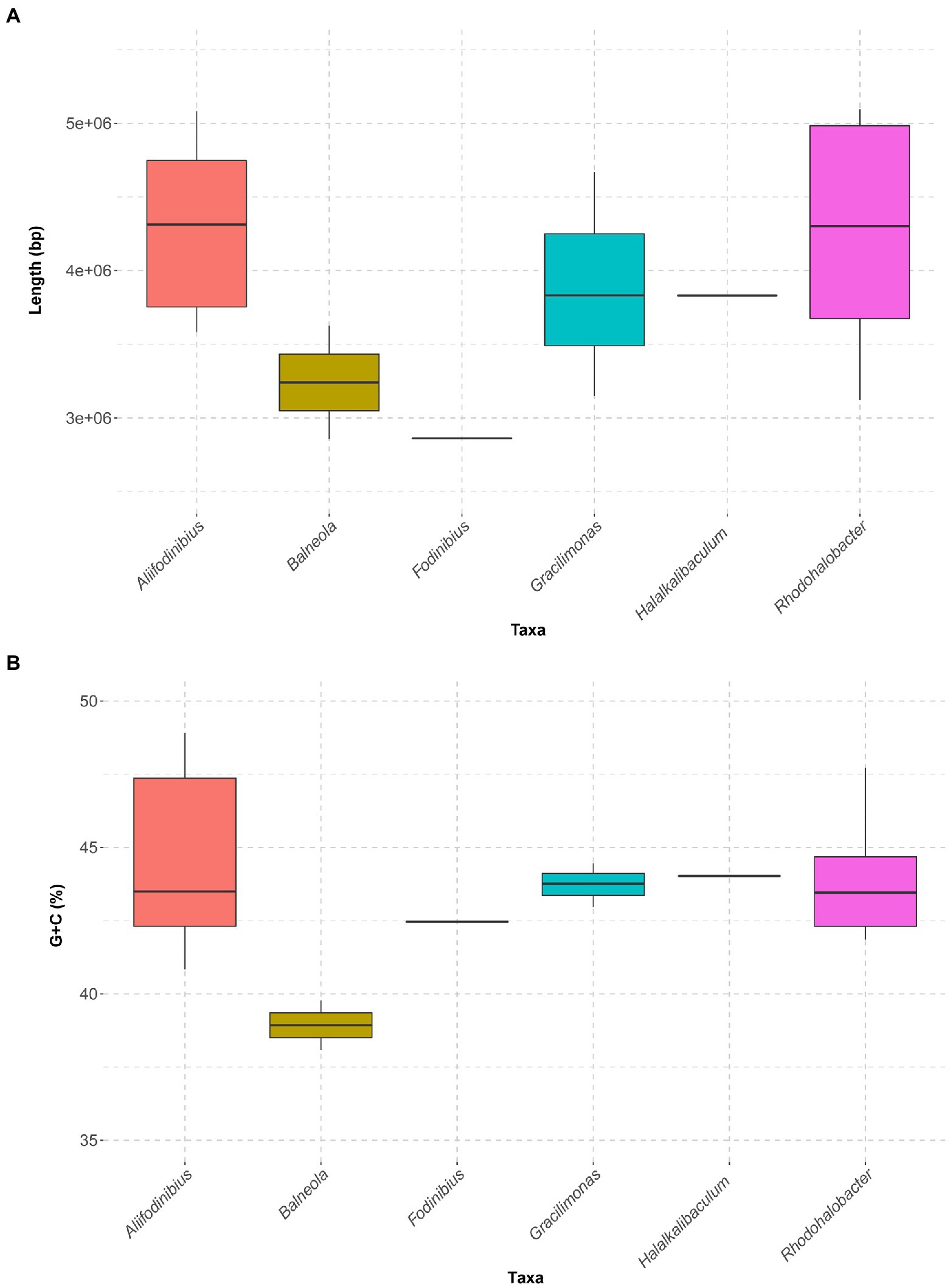
Figure 2. Boxplots summarizing genome size (A) and G + C content (B) of the genera of the family Balneolaceae, sorted alphabetically. The family Balneolaceae includes genera with very different genomes sizes, but their G + C percentages are mostly stable around 43 mol%, except for the type genus Balneola, below 40 mol%.
The approximated maximum-likelihood phylogenomic tree, based on the concatenation of 1,049 translated core genes, showed that the strain 1BSP15-2V2T clustered again with the species of the genus Aliifodinibius (Figure 3). This clustering was supported by a 100% bootstrap value, indicating the robustness of the group, which was unclear in the 16S rRNA gene-based phylogeny (Figure 1). Furthermore, Fodinibius salinus DSM 21935T, the only described species of this genus, also clustered with 100% branch support together to the species of the genus Aliifodinibius, rather than forming a separated and independent branch. This fact seems to indicate that members of the genera Aliifodinibius and Fodinibius could be actually merged into the single genus Fodinibius.
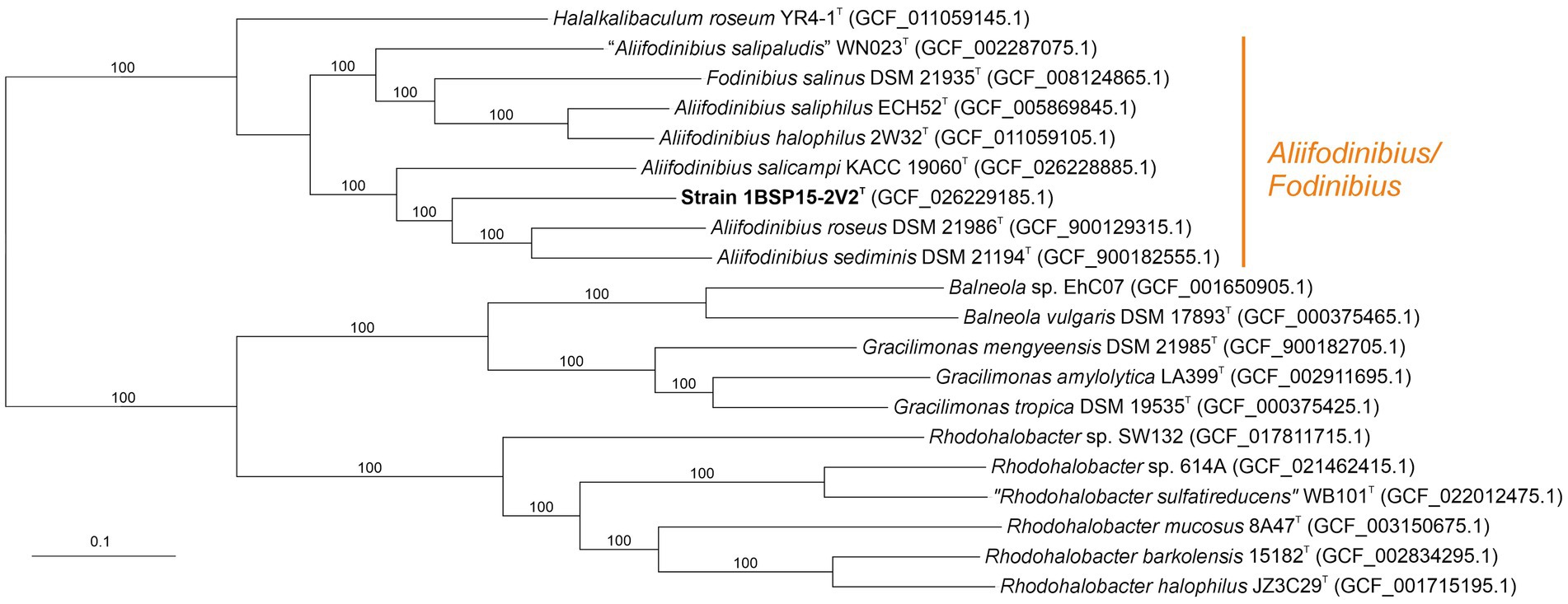
Figure 3. Approximately maximum-likelihood phylogenomic tree based on 1,049 concatenated core protein sequences of members of the family Balneolaceae. Bootstrap values ≥70% are indicated above the branches. Bar, 0.1 substitutions per nucleotide position.
Concerning the OGRIs analysis, AAI values between strain 1BSP15-2V2T and the other members of the genus Aliifodinibius ranged from 72.1% (with A. sediminis DSM 21194T) to 68.1% (with A. halophilus 2W32T) (Figure 4). These values were above the 65–72% threshold suggested to circumscribe members of the same genus (Konstantinidis and Tiedje, 2007; Konstantinidis et al., 2017). Similar AAI values were obtained for Fodinibius salinus DSM 21935T, which varied from 72.9% (with A. halophilus 2W32T) to 68.1% (with A. roseus DSM 21986T) with respect to the species of the genus Aliifodinibius, but were equal or lower than 64.9% for Halalkalibaculum roseum YR4-1T and the remaining species of the genera of the family Balneolaceae (Figure 4). Furthermore, orthoANI percentages among the analyzed genomes within the Balneolaceae were all below the 95% threshold established for species differentiation (Goris et al., 2007; Richter and Rosselló-Móra, 2009; Chun and Rainey, 2014), including strain 1BSP15-2V2T, which shares the highest similarity with A. roseus DSM 21986T and A. sediminis DSM 21194T (72.7% for both species) (Figure 5). These data undoubtedly indicate that all strains under study represented different species. The same conclusion can be inferred from dDDH results, where all values are far below the 70% cutoff defined for species delineation (Stackebrandt and Goebel, 1994; Auch et al., 2010) (Figure 5).
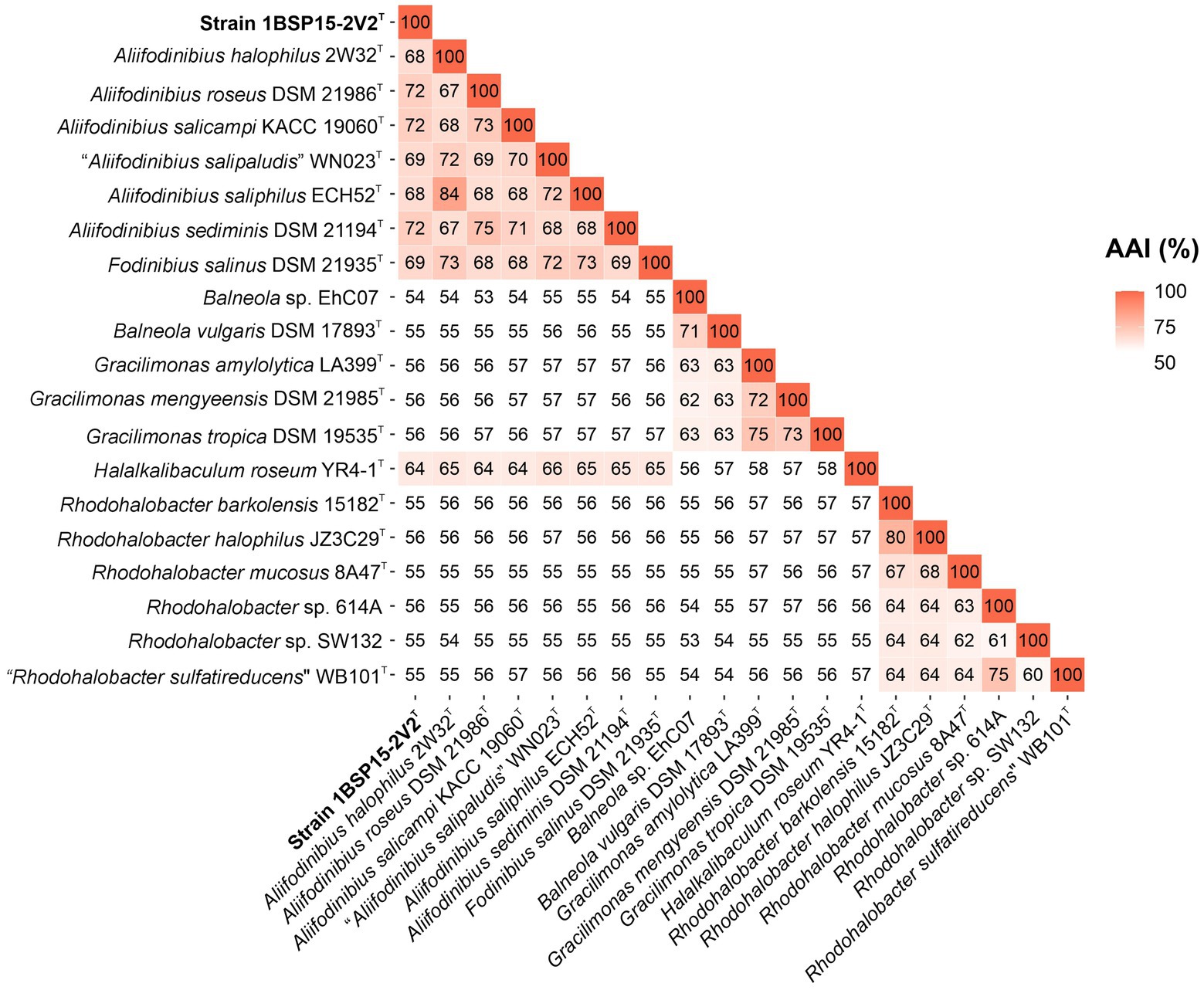
Figure 4. AAI matrix among the 20 studied genomes of the family Balneolaceae. AAI values among species of the cluster Fodinibius-Aliifodinibius were all higher than the threshold (65%) accepted for genus delineation. Isolate 1BSP15-2V2T shared values above 68% with species of the genera Aliifodinibius and Fodinibius, and equal or lower than 64% with other members of the family Balneolaceae, hinting at the affiliation of the novel strain to the genera Aliifodinibius/Fodinibius.
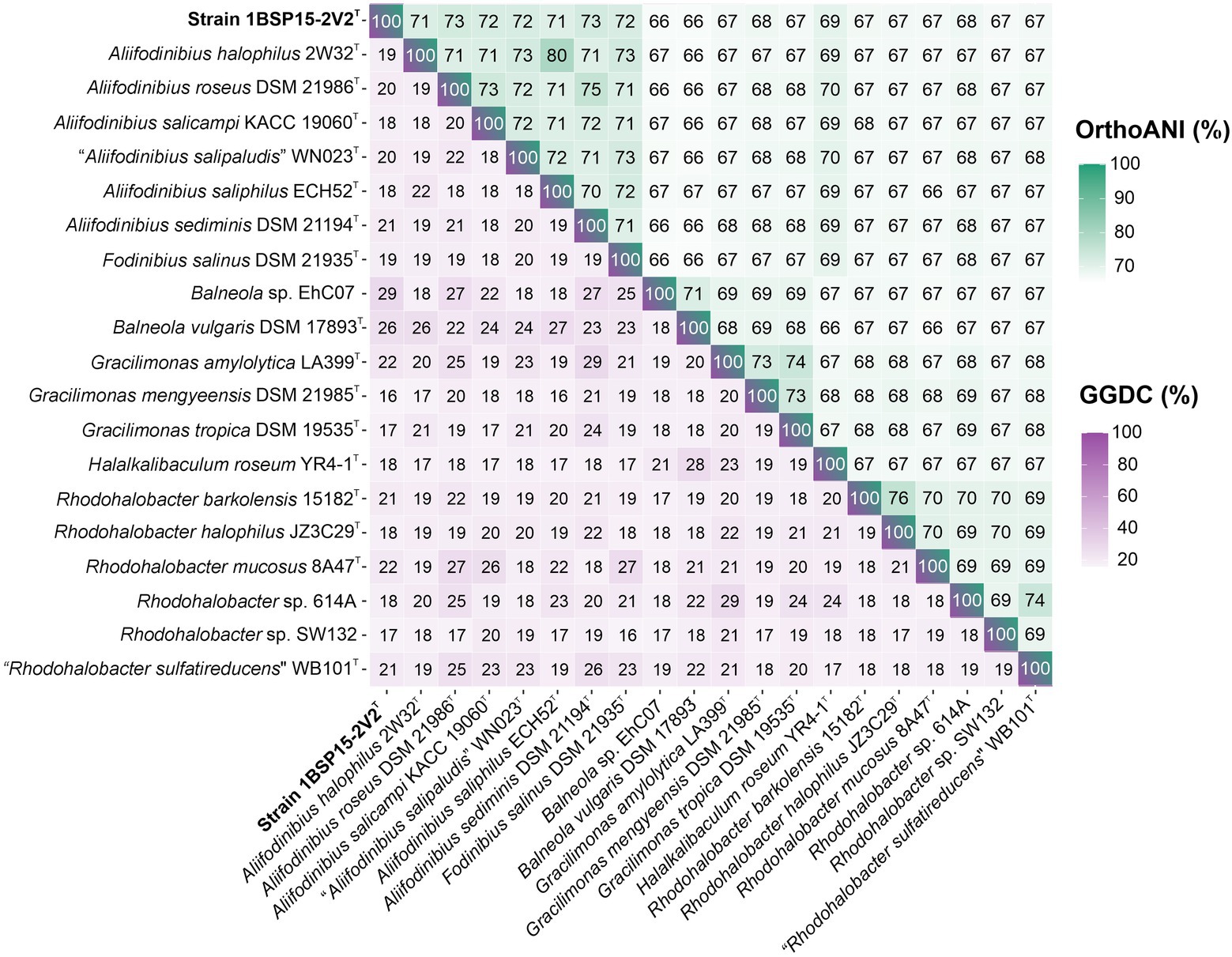
Figure 5. OrthoANI (upper triangle) and GGDC (lower triangle) matrix among the 20 studied genomes of the family Balneolaceae. All GGDC (purple) and orthoANI (green) values were below the cutoff for species delineation, indicating that all the genomes belong to different species.
The distribution of core, soft-core, and accessory genomic content among the 20 genomes of the family Balneolaceae investigated here are shown in Figure 6. All the studied genomes of the species of the family Balneolaceae share a total of 1,049 core genes. The species “Aliifodinibius salipaludis” WN023T possesses the highest number of unique genes (270), although it has the smaller genome size (3.58 Mb) and the lower number of CDS (3,143) (Supplementary Table S1). This could be related with the habitat where “Aliifodinibius salipaludis” WN023T dwells, since to date it is the only member of the family isolated from saline-alkaline soil instead of hypersaline sediments or salterns (Zhao et al., 2020). However, our new strain 1BSP15-2V2T was also isolated from saline soils and its exclusive genetic material is not high enough (12 unique genes) as to be displayed among the most frequent intersections (Figure 6). Strain 1BSP15-2V2T shares a total of 61 genes exclusively with the type species of the genus Aliifodinibius, A. roseus DSM 21986T, and 50 additional genes if the species A. sediminis DSM 21194T is also considered. In any case, strain 1BSP15-2V2T shares a higher number of genes with the species of the genus Aliifodinibius than with any other of the remaining genera of the family Balneolaceae. Moreover, a large cluster of 88 genes that are present solely within the genera Aliifodinibus and Fodinibius, as well as the genus Halalkalibaculum, points to their closed evolutionary relationships (Figure 6). Similarly, 97 genes are exclusively shared between the members of the genus Rhodohalobacter. In summary, we observed that the most closely related the species are the highest number of genes they share. Further differences in the protein functions are described in Section 3.4.
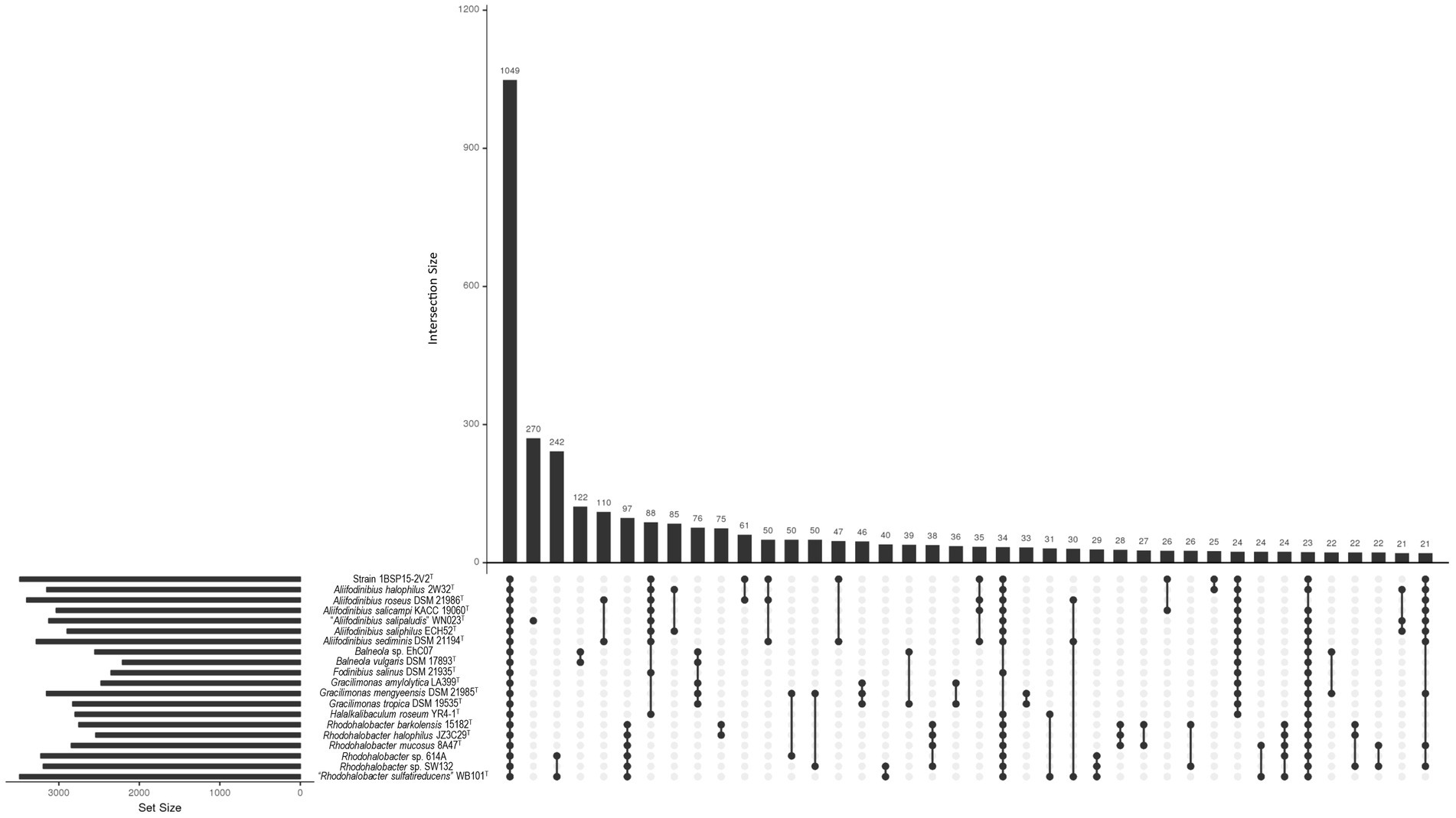
Figure 6. Upset plot showing the proteins shared among the 20 Balneolaceae genomes under study. Only the 40 most frequent intersecting sets are displayed. From a pan genome of 8,113 translated genes, 1,049 were encoded in all the genomes (core genome). “Aliifodinibius salipaludis” WN023T harbored the highest number of unique translated genes (270). The closely related genera Aliifodinibius, Fodinibius, and Halalkalibaculum shared 88 translated genes.
3.3. Chemotaxonomic and phenotypic characterization support the placement of the new isolate within the genus Fodinibius
The major fatty acids determined for strain 1BSP15-2V2T were iso-C15:0 (36.4%), C16:1 ω7c and/or C16:1 ω6c (24.7%), and iso-C17:1 ω9c and/or 10-methyl C16:0 (10.8%). The profile of this strain is similar to those of the type strains of the species of the genera Aliifodinibius and Fodinibius (Supplementary Table S3). No clear differential profiles were obtained for species of the genera Aliifodinibius and Fodinibius, supporting the hypothesis that they all belong to the same genus.
The strain 1BSP15-2V2T formed irregular, opaque, and orange-red pigmented colonies. Cells were Gram-stain-negative, non-motile, non-endospore-forming rods, with a size of 0.05–0.10 × 0.20–0.30 μm. The detailed phenotypic characteristics of strain 1BSP15-2V2T are included in the new species description. Besides, additional phenotypic data for the previously described species of the genera Aliifodinibius and Fodinibius included in this study are shown in Supplementary Table S4. The morphological and many of the biochemical characteristics of the isolate 1BSP15-2V2T agree with those reported for the other members of the genera Aliifodinibius and Fodinibius, and thus, support the placement of strain 1BSP15-2V2T within the genus Fodinibius (which should also include the described species of Aliifodinibius). Besides, strain 1BSP15-2V2T possesses several features that allow to distinguish it from the close-related species of the genera Aliifodinibius and Fodinibius, namely, the ability to use several amino acids as sole carbon, nitrogen, and energy sources (Supplementary Table S4).
3.4. Functional genomic analysis reveals the potential ecological role of the new strain as a biotin producer
BlastKOALA annotation identified a total of 1,986 different KO numbers across the 16 available genomes of the type strains of species of the family Balneolaceae. Those KO numbers were classified according to KEGG pathway database, and the 50 most abundant pathways are shown in Figure 7. Strain 1BSP15-2V2T seems to exhibit a functional profile like that of the already described species of the genera Aliifodinibius/Fodinibius and the rest of the genera within the family Balneolaceae. Nevertheless, it must be noted that 22 KO numbers were only present in strain 1BSP15-2V2T and not in any other member of the family. Among those unique functional orthologs, we detected frdA, frdB, frdC, and frdD genes in the genome of strain 1BSP15-2V2T, involved in the conversion of fumarate to succinate (Supplementary Table S5).
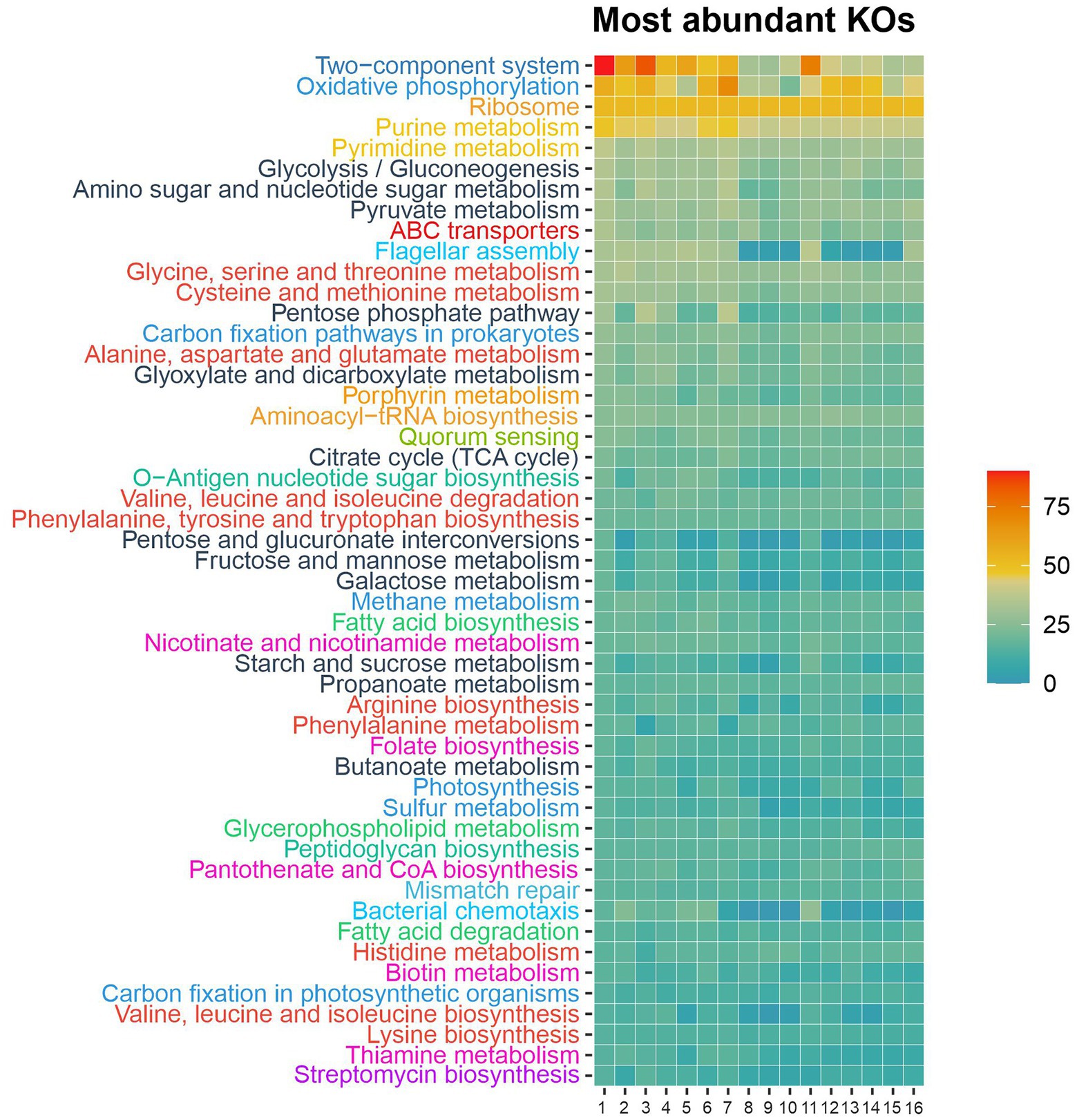
Figure 7. KO numbers grouped by KEGG categories annotated for the representative genomes of the family Balneolaceae. KEGG pathways are sorted according to their abundance in the strain 1BSP15-2V2T and only the 50 most abundant are displayed. The most prominent pathways detected in those genomes belonged the following KEGG global categories: signal transduction (dark blue), energy metabolism (blue), translation (orange), nucleotide metabolism (yellow), carbohydrate metabolism (black), membrane transport (dark red), cell motility (light blue), amino acid metabolism (light red), cellular community (light green), glycan biosynthesis and metabolism (dark green), lipid metabolism (green), metabolism of cofactors and vitamins (pink), and biosynthesis of other secondary metabolites (purple). 1. Strain 1BSP15-2V2T; 2. Aliifodinibius halophilus 2W32T; 3. Aliifodinibius roseus DSM 21986T; 4. Aliifodinibius salicampi KACC 19060T; 5. “Aliifodinibius salipaludis” WN023T; 6. Aliifodinibius saliphilus ECH52T; 7. Aliifodinibius sediminis DSM 21194T; 8. Fodinibius salinus DSM 21935T; 9. Balneola vulgaris DSM 17893T; 10. Gracilimonas amylolytica LA399T; 11. Gracilimonas mengyeensis DSM 21985T; 12. Gracilimonas tropica DSM 19535T; 13. Halalkalibaculum roseum YR4-1T; 14. Rhodohalobacter barkolensis 15182T; 15. Rhodohalobacter halophilus JZ3C29T; 16. Rhodohalobacter mucosus 8A47T.
The most prevalent pathways of strain 1BSP15-2V2T were included into energy, carbohydrate, and amino acid metabolism KEGG global categories. All the species of the genera Aliifodinibius and Fodinibius harbored genes involved in pyruvate metabolism, including the pyruvate oxidation pathway comprising KO numbers K00161 (pdhA, pyruvate dehydrogenase E1 component α-subunit), K00162 (pdhB, pyruvate dehydrogenase E1 component β-subunit), K00627 [pdhC, pyruvate dehydrogenase E2 component (dihydrolipoamide acetyltransferase)], and K00382 (pdhD, dihydrolipoamide dehydrogenase) (Supplementary Table S5). However, only strain 1BSP15-2V2T, A. halophilus KCTC 42497T, and A. roseus DSM 21986T were able to use pyruvate as sole carbon and energy source when tested experimentally (Supplementary Table S4). The other genera of the family Balneolaceae showed the same in-silico metabolism for pyruvate, but it was not confirmed in this study under laboratory conditions.
Strain 1BSP15-2V2T and the species of the genus Aliifodinibius possessed genes linked to flagellar assembly (Figure 7; Supplementary Table S5), in contrast to the genera Fodinibius, Balneola, Halalkalibaculum, and Rhodohalobacter, as well as the species Gracilimonas amylolytica LA399T and G. tropica DSM 19535T, although the route was incomplete in all the cases. This finding agrees with the fact that none of the descriptions of the species of the genus Aliifodinibius reported the cellular motility (Wang et al., 2013; Xia et al., 2016; Cho et al., 2017; Cho and Whang, 2020; Zhao et al., 2020). Cells of 1BSP15-2V2T did not show motility either in the present study. The presence of a broken pathway for flagellar motility seems to indicate that the ancestor of the family Balneolaceae may have had that feature, which was gradually lost as it was not required for their survival in the nature. Major differences between the functional profile of species of the genera Aliifodinibius and Fodinibius are related to bacterial motility, that is flagellar assembly and flagellar motor switch (two component system). This supports the theory proposed above about the loss of motility-related genes, which might have happened quicker in F. salinus. Other than those two pathways, F. salinus presents a mostly similar profile to the species of the genus Aliifodinibius. We can find minor differences between the genomes of the studied species of Aliifodinibius/Fodinibius, such as less abundance of functions related to oxidative phosphorylation and purine metabolism in the species “Aliifodinibius salipaludis” and F. salinus, though similar between them, and an oscillation in the number of genes encoding enzimatic activities in the pentose phosphate pathway. In any case, all the studied genomes are uncompleted, and their range size is broad among the members of the Aliifodinibius/Fodinibius. Hence, we can only speculate about the presence or lack of functions, as it may be due to a deficient annotation or assembly with the current bioinformatic tools, or to an adaptation of the bacteria to their particular environment.
The biosynthesis pathway for biotin (vitamin B7) appears to be present in the species of the family Balneolaceae. Though the understanding of biotin biosynthesis is still fragmentary, previous studies (Cronan, 2018; Manandhar and Cronan, 2018) propose two stages: the synthesis of a pimelate moiety, and the assembly of the bicyclic rings of the biotin molecule. The latter is mostly conserved, with four associated genes: bioF, bioA, bioD, and bioB, all of them present in strain 1BSP15-2V2T (Figure 8A; Supplementary Table S5). These genes were also detected in A. halophilus, A. saliphilus, Fodinibius salinus, Halalkalibaculum roseum, and Balneola vulgaris (Figure 8B; Supplementary Table S5). Only bioB and bioF were present in A. salicampi and “A. salipaludis.” However, the bioF gene, which performs the first step of the assembly of the bicyclic rings, can be found in all the studied species of the family Balneolaceae. On the contrary, there are at least three known pathways to accomplish the first stage of biotin biosynthesis: the BioC-BioH and the BioI-BioW pathways, studied in Escherichia coli and Bacillus subtilis, respectively (Lin and Cronan, 2011), and the most recently proposed BioZ pathway (Zhang et al., 2021). However, the BioC-BioH pathway seems to be the most prevalent of these three (Feng et al., 2014; Wei et al., 2019). Genes bioC, fabF, fabG, fabZ, and fabI from the BioC-BioH pathway are found in all the species of the genus Aliifodinibius, except the bioC gene lacking in A. roseus. These genes are also present in strain 1BSP15-2V2T and Fodinibius salinus, as well as in most species of the family Balneolaceae. Noteworthy, all studied genomes of the family seem to lack the bioH gene, which performs the last step of the BioC-BioH pathway. However, homologous genes have been found in other organisms as replacements (Rodionov et al., 2002), such as bioG in Haemophilus sp. (Shi et al., 2016), bioK in Synechococcus sp. (Shapiro et al., 2012), bioJ in Francisella sp. (Wei et al., 2019), and bioV in Helicobacter sp. (Bi et al., 2016). None of these alternative genes were found in the genomes under study, but we cannot discard the possibility that the family Balneolaceae harbors a different homologous gene that hydrolyzes the pimeloyl-ACP methyl ester into pimeloyl-ACP in the last step of the BioC-BioH pathway (Lin and Cronan, 2011). On the other hand, all the species of the family Balneolaceae present birA, accB, and accC genes in their genomes. The protein BirA has a dual function: attach biotin to AccB protein and down-regulate biotin biosynthesis in the absence of free AccB acceptor protein. AccB is a carrier protein that forms a complex with the AccC subunit (Choi-Rhee and Cronan, 2003), so both macromolecules indirectly regulate the transcription of the biotin operon. Low levels of available AccB protein, that is when AccB protein is biotinylated or unbiotinylated but bonded to AccC, avoid biotin transfer from BirA to AccB, resulting in higher levels of BirA ligand bound to biotin. The accumulation of BirA-bound biotin represses biotin operon transcription (Abdel-Hamid and Cronan, 2007). In summary, the genome of 1BSP15-2V2T, as well as those of the rest of the species of the family Balneolaceae, contain genes involved in the regulation of biotin biosynthesis. With a single exception, they also have the complete set of bioC, fabF, fabG, fabZ, and fabI genes that catalize the first steps of biotin biosynthesis, albeit all of them lack the bioH gene, which encodes the last enzyme of this first stage. Since several bioH homologs have been reported in the literature, the species of the family Balneolaceae are hypothesized to harbor one of those not-yet described homologous genes. Considering that the more conserved second stage (bioF, bioA, bioD, and bioB genes) is only present in the genomes of strain 1BSP15-2V2T and other five members of the family, the complete (or almost complete) biotin biosynthesis pathway was only identified in the genomes of strain 1BSP15-2V2T, A. halophilus, A. saliphilus, Fodinibius salinus, Halalkalibaculum roseum, and the type species of the family, Balneola vulgaris, whereas it was missing from the genera Gracilimonas and Rhodohalobacter. Since the biotin biosynthetic pathway is well conserved across biotin producers (Lin and Cronan, 2011), it may be explained the presence of at least some of those genes within all described species of the family Balneolaceae, even if the pathway is uncompleted. Biotin plays an important role in fatty acid synthesis, amino acid metabolism, and gluconeogenesis in bacteria, archaea, and eukaryotes (Knowles, 1989; Jitrapakdee and Wallace, 2003). The biosynthesis of biotin is exclusive of some prokaryotes, fungi, and plants but it is an essential cofactor for mammals too, which depend on the exogenous supply from the diet or the microbiota (Cronan et al., 2014). For example, vitamin B7 deficiency in humans is associated with neurological diseases (León-Del-Río, 2019). It is a high-costing metabolic pathway, especially the steps carried out by the proteins coded by bioC, bioA and bioB genes. Hence, most organisms prefer an exogenous uptake of this cofactor (Sirithanakorn and Cronan, 2021). The isolated species could play a role in its ecosystem by suppling biotin to those organisms that lack the mechanisms for de novo synthesis. Besides, it might be further explored as an interesting source of biotin for human deficiency with biotechnological application. Nowadays, biotin for pharmacological purposes is chemically synthetize since bacterial biosynthesis has given unsuccessful results to date (Sirithanakorn and Cronan, 2021). This is, partly, due to the uncomplete understanding of the biotin pathway in prokaryotes. Thus, the genomic identification of this route in strain 1BSP15-2V2T will allow its targeting as a possible candidate for further studies of the biotin biosynthesis.
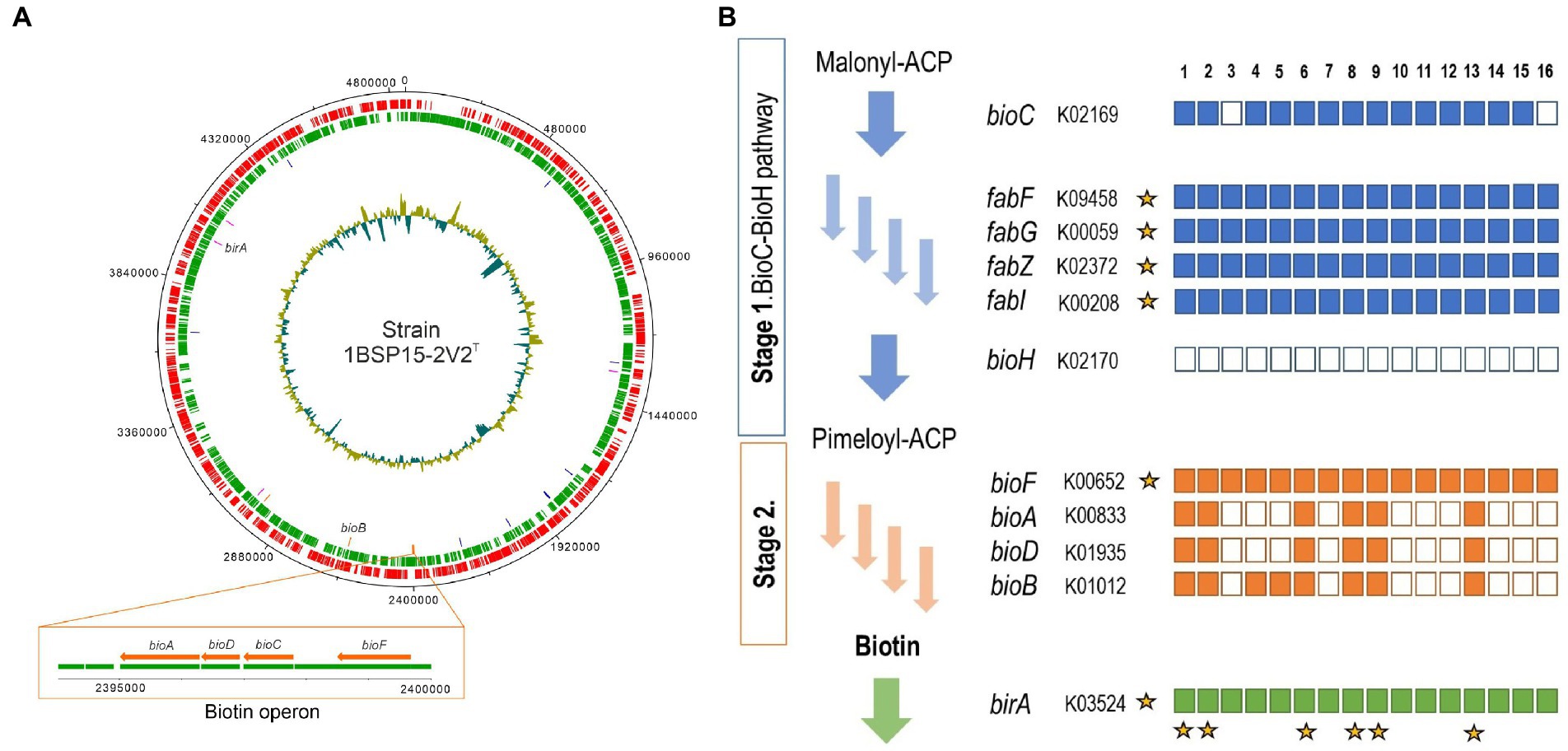
Figure 8. (A) Circular genome map of strain 1BSP15-2V2T annotated by Prokka. The graph was generated with “DNAPlotter” (Carver et al., 2009). Circles indicate, from outside inwards: coding sequences in the leading strand (red); coding sequences in the lagging strand (green); coding sequences related to the biotin biosynthesis; G + C content. Biotin pathway-related genes are displayed in colors: stage 1 of biosynthesis, blue; stage 2 of biosynthesis, orange; regulation and transport, pink. A diagram of the biotin operon arrangement is shown in the box. Genes bioA, bioD, bioC, and bioF are located in the same region of the genome with a hypothetical protein encoded between bioF and the rest of the bioADC genes. The organization in form of operon allows the cotranscript of their proteins in the correct stoichiometry (Sirithanakorn and Cronan, 2021). bioB and birA genes are placed in a different region of the chromosome. (B) Biotin biosynthesis pathway for members of the family Balneolaceae. Although almost the complete BioC-BioH pathway was detected in most of the genomes, bioH gene was missing for all of them. Stars indicate either that the gene is present in all the genomes or that the pathway (except bioH gene) is complete for the corresponding genome. 1. Strain 1BSP15-2V2T; 2. Aliifodinibius halophilus 2W32T; 3. Aliifodinibius roseus DSM 21986T; 4. Aliifodinibius salicampi KACC 19060T; 5. “Aliifodinibius salipaludis” WN023T; 6. Aliifodinibius saliphilus ECH52T; 7. Aliifodinibius sediminis DSM 21194T; 8. Fodinibius salinus DSM 21935T; 9. Balneola vulgaris DSM 17893T; 10. Gracilimonas amylolytica LA399T; 11. Gracilimonas mengyeensis DSM 21985T; 12. Gracilimonas tropica DSM 19535T; 13. Halalkalibaculum roseum YR4-1T; 14. Rhodohalobacter barkolensis 15182T; 15. Rhodohalobacter halophilus JZ3C29T; 16. Rhodohalobacter mucosus 8A47T.
In addition to the biotin biosynthesis, strain 1BSP15-2V2T also presents functional orthologs related to other cofactors and vitamins, such as folate, pantothenate and CoA biosynthesis, and metabolism of thiamine, nicotinate, and nicotinamide (Figure 7; Supplementary Table S5). On the other hand, only the species of the genera Aliifodinibius and Fodinibius, including the strain 1BSP15-2V2T, showed genes involved in the cobalamin (vitamin B12) transport system (btuC, btuD, and btuF), and all members of the family Balneolaceae harbors btuB, a vitamin B12 transporter. Moreover, a previous study has reported the presence of cobalamin-dependent enzymes in the metagenomic dataset SMO2, obtained from hypersaline soils at the Odiel Saltmarshes National Area (Durán-Viseras et al., 2019). These enzymes corresponded to the KO numbers K00525 (nrdA, ribonucleoside-diphosphate reductase α-chain), K01848 (mcmA1, methylmalonyl-CoA mutase, N-terminal domain), and K00548 (metH, 5-methyltetrahydrofolate–homocysteine methyltransferase). Two of them, K00525 and K00548, along with K01847 (MUT, methylmalonyl-CoA mutase) and K11942 (icmF, isobutyryl-CoA mutase), were found in the genomes of strain 1BSP15-2V2T and members of the family Balneolaceae. However, none of them encoded the cobalamin biosynthesis pathway, which is not surprising, as it is a high-cost metabolic mechanism (Morris, 2012; Doxey et al., 2015). Thus, it seems to indicate that strain 1BSP15-2V2T as well as the rest of the family Balneolaceae depend on an external source of vitamin B12, such as species of the haloarchaeal genera Halobacterium (Woodson et al., 2003) and Halonotius (Durán-Viseras et al., 2019).
3.5. Metalloresistant abilities to bear up against contaminated soils
As stated above, cadmium and lead concentrations in our sampled hypersaline soils were within the reference ranges for a non-contaminated soil, whereas arsenic, copper, and zinc concentrations were above the maximum thresholds (Table 1). Therefore, we can classify our samples as arsenic, copper, and zinc contaminated soils.
Several genes related to heavy metal resistance were found in the genome of strain 1BSP15-2V2T. The ars operon (KO numbers K03325, K03741, and K03892) (Supplementary Table S5) is responsible for reduction of arsenate, As (V), to arsenite, As (III), and its excretion from the cell (Chauhan et al., 2019; Islam et al., 2022). Arsenic resistance is believed to be a widespread mechanism among prokaryotes due to the high concentration of this metalloid in the early period of the Earth (Chen et al., 2020). On the other hand, the CzcCBA efflux system (K15725, K15726, K15727, and K16264) (Supplementary Table S5) provides resistance to cadmium, copper, and zinc (Legatzki et al., 2003). This pump has been found in many metallotolerant Gram-negative bacteria (Manara et al., 2012; Khan et al., 2015; Alquethamy et al., 2021). Furthermore, the zntA gene (K01534) (Supplementary Table S5) for Zn2+/Cd2+-exporting (Rensing et al., 1997; Noll and Lutsenko, 2000) was also detected in the genome of strain 1BSP15-2V2T. Previous studies have demonstrated the ability of CzcCBA efflux system to efficiently remove zinc and cadmium from the cytoplasm (Legatzki et al., 2003). Thus, the existence of an additional zntA gene-encoding pump in strain 1BSP15-2V2T seems to be an adaptative mechanism to cope with the harsh metal conditions of the Odiel Saltmarshes National Area hypersaline soils. Nevertheless, it must be noted that our predictions are entirely based on in-silico functional annotation analysis and, therefore, further laboratory research is necessary to confirm the heavy metal resistance of this bacterium.
3.6. Genomic information unveils a salt-out osmoregulation strategy
Two main osmoregulatory mechanisms allow prokaryotes to dwell in high-salt concentration environments. The salt-in strategy is typical of extreme halophiles, mostly haloarchaea (Youssef et al., 2014) and some bacteria, such as those of the widely studied genus Salinibacter (Antón et al., 2002). To maintain the structure and activity of their proteins under high cytoplasmic ion concentrations these microorganisms possess a more acidic proteome (Oren, 2008). However, this kind of proteome has also been described in microorganisms with a salt-out mechanism (Elevi Bardavid and Oren, 2012; Oren, 2013), which is the most extended strategy across prokaryotes allowing adaptation to wider variations in the surrounding osmotic pressure (Oren, 2008).
All genomes under study have a similar isoelectric profile (Figure 9A) and amino acid frequency distribution (Figure 9B), showing a less acidic proteome than those of the extreme haloarchaeon Haloarcula vallismortis and the bacterium Salinibacter ruber. Concerning the genome of strain 1BSP15-2V2T, it harbors Ktr potassium importer genes (K03498, K03499) that may contribute to a fast response against osmotic stress, as it has been previously observed in Bacillus subtilis (Holtmann et al., 2003; Hoffmann and Bremer, 2016) and Synechocystis sp. (Zulkifli et al., 2010). Another ion homeostasis-related protein annotated in the genome of strain 1BSP15-2V2T (K03313) is the NhaA Na+/H+ antiporter, which plays a relevant role to avoid the toxicity of high cytoplasmatic Na+ concentrations (Krulwich et al., 2011; Patiño-Ruiz et al., 2022). However, potassium and sodium fluxes are impractical mechanisms to deal with sustained high osmolarity (Hoffmann and Bremer, 2017). For that reason, strain 1BSP15-2V2T also presents compatible solute transporters of the Opu family (K05845, K05846, K05847), involved in the ATP-dependent uptake of a wide variety of compatible solutes into the cytoplasm, such as glycine betaine, choline, and proline betaine (Hoffmann and Bremer, 2017; Teichmann et al., 2018). Sudden decrease of osmolarity could also trigger stress in bacteria. Accordingly, strain 1BSP15-2V2T encodes Msc mechanosensitive channels (K03282, K03442) which release ions and organic molecules if an osmotic down shock occurs (Booth and Blount, 2012; Booth, 2014). The different strategies to balance the osmotic pressure encoded in the genome of strain 1BSP15-2V2T (Supplementary Table S5) indicate that this microorganism can thrive on a range of salt concentrations, which is consistent with the physiological traits tested under laboratory conditions (Supplementary Table S4). Consequently, strain 1BSP15-2V2T can be defined as a moderately halophilic bacterium with a salt-out osmoregulation mechanism.
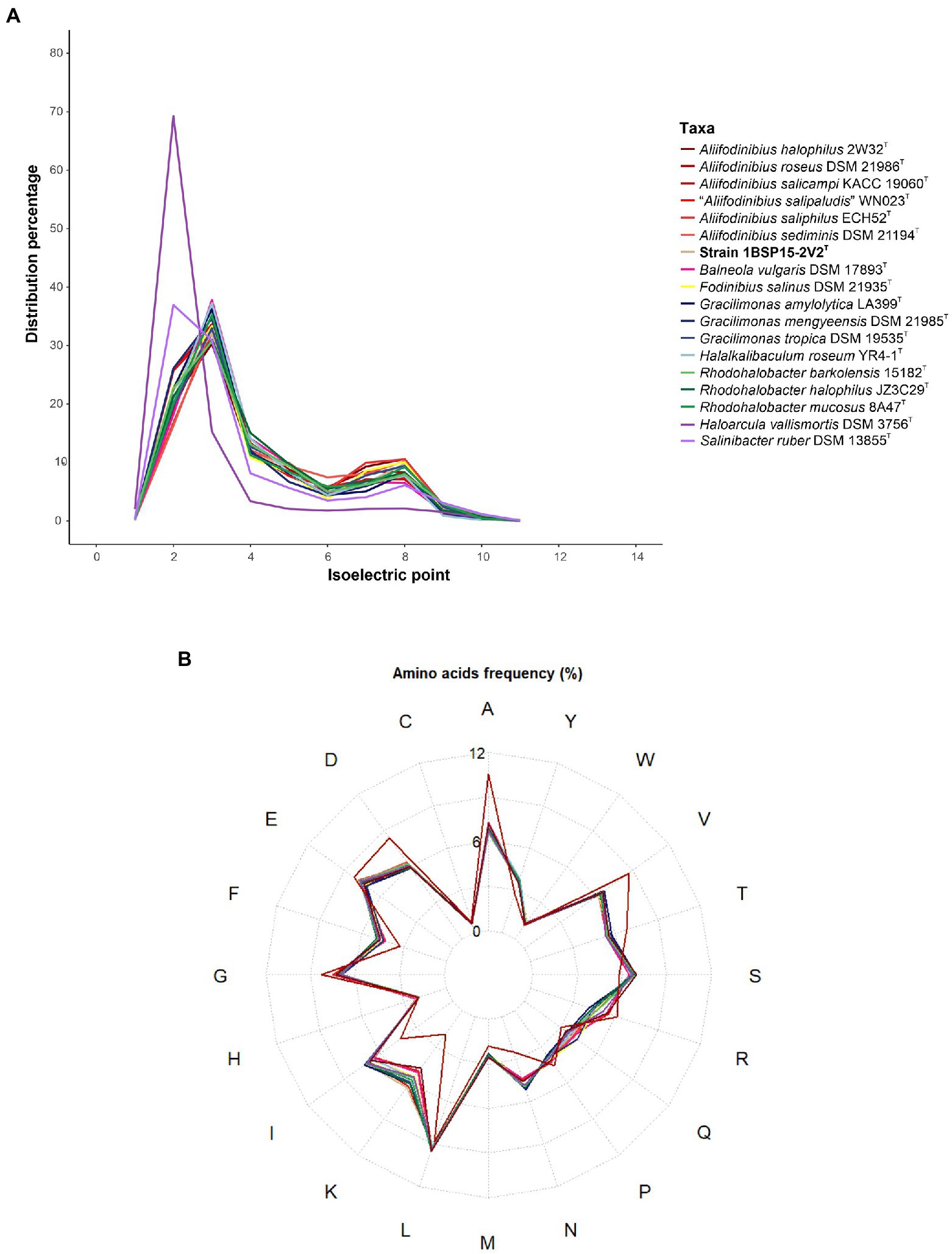
Figure 9. Isoelectric profile (A) and amino acid frequency (B) of the type strains of each species of the family Balneolaceae. The haloarchaeon Haloarcula vallismortis DSM 3756T (GCF_900106715.1) and the bacterium Salinibacter ruber DSM 13855T (GCF_000013045.1) have been included to compare our results with those of extreme halophiles with salt-in osmoregulatory mechanism. The genomes under study possessed a less acidic proteome, suggesting a salt-out strategy, though further in vivo analyses are necessary to confirm this hypothesis.
3.7. Members of the family Balneolaceae prefer saline soil versus aquatic hypersaline environments
Genomic fragment recruitment analysis was performed against nine metagenomic datasets (Supplementary Table S2) to assess the environmental distribution of strain 1BSP15-2V2T and the other species of the family Balneolaceae. SMO1 and SMO2 metagenomes come from the same hypersaline soils used in this study. The other seven metagenomic databases originated from hypersaline aquatic environments (salterns and lakes) were included in this study to determine the distribution of the new taxon in different saline habitats (soils and water). The selected datasets ranged from 13 to 37% (w/v) salt concentration. Of these, SS13 metagenome has the closest salinity (13% [w/v] salts) to the optimal salt concentration defined for strain 1BSP15-2V2T (9% [w/v] salts). Our results show few reads (<0.01%) recruited at ≥95% identity from all species of the genera Aliifodinibius/Fodinibius for all metagenomic datasets. As nucleotide sequences are being compared, the 95% ANI threshold is considered for species delineation. Hence, the isolated species seems to be not highly abundant in the studied environments. However, soil and aquatic environments seem to follow different patterns, with a huge read recruitment at 80–90% identity for SMO1 and SMO2 (soils) datasets (Figure 10) not observed in water metagenomes (Figure 11). Barco et al. (2020) proposed an ANI cutoff value of 73.98% for genus demarcation, and thus, on this basis, it would suggest the existence of a taxon or group of taxa very closely related to the genera Aliifodinibius and Fodinibius (that could represent different species or even genera) abundant in those hypersaline soil environments. On the contrary, the scarce recruitment at 80–90% identity for the aquatic hypersaline databases might indicate that closed relatives of the genera Aliifodinibius/Fodinibius have a preference for hypersaline soils over their aquatic counterparts.
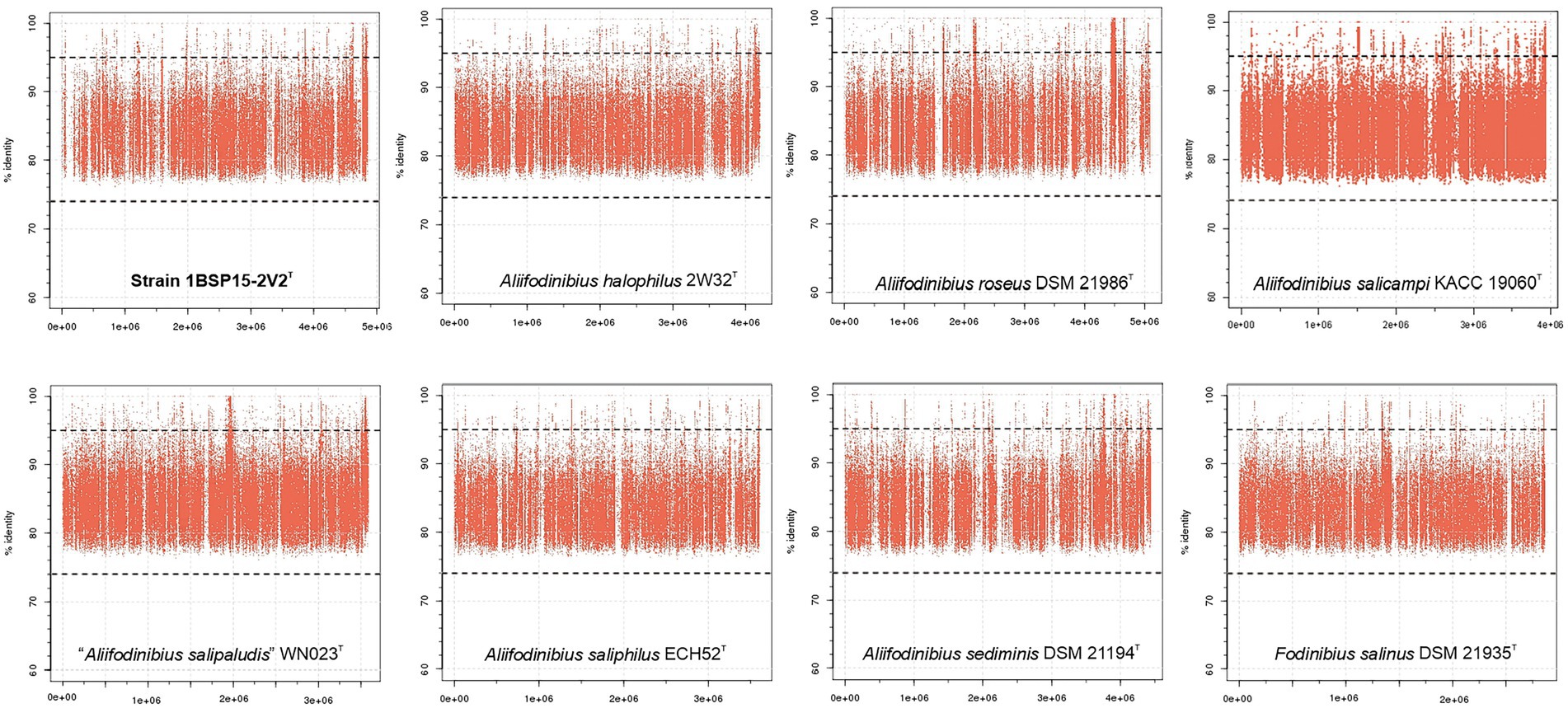
Figure 10. Genomic recruitment plots for the isolated strain 1BSP15-2V2T and other type strains of species of the genera Aliifodinibius and Fodinibius against SMO1 hypersaline soil metagenomic reads. X-axis represents the genome length and Y-axis represents the percentage of identity of the reads matching the genome. Red dots above the 95% threshold line indicate read assignment at the species level. As it can be seen, most of the reads matches the genome at 80%–90% identity (above the proposed 73.98% for genus delineation), meaning they cannot be affiliated to any of the described Aliifodinibius/Fodinibius species, but they probably belong to a closed relative well represented in the SMO1 hypersaline soil.
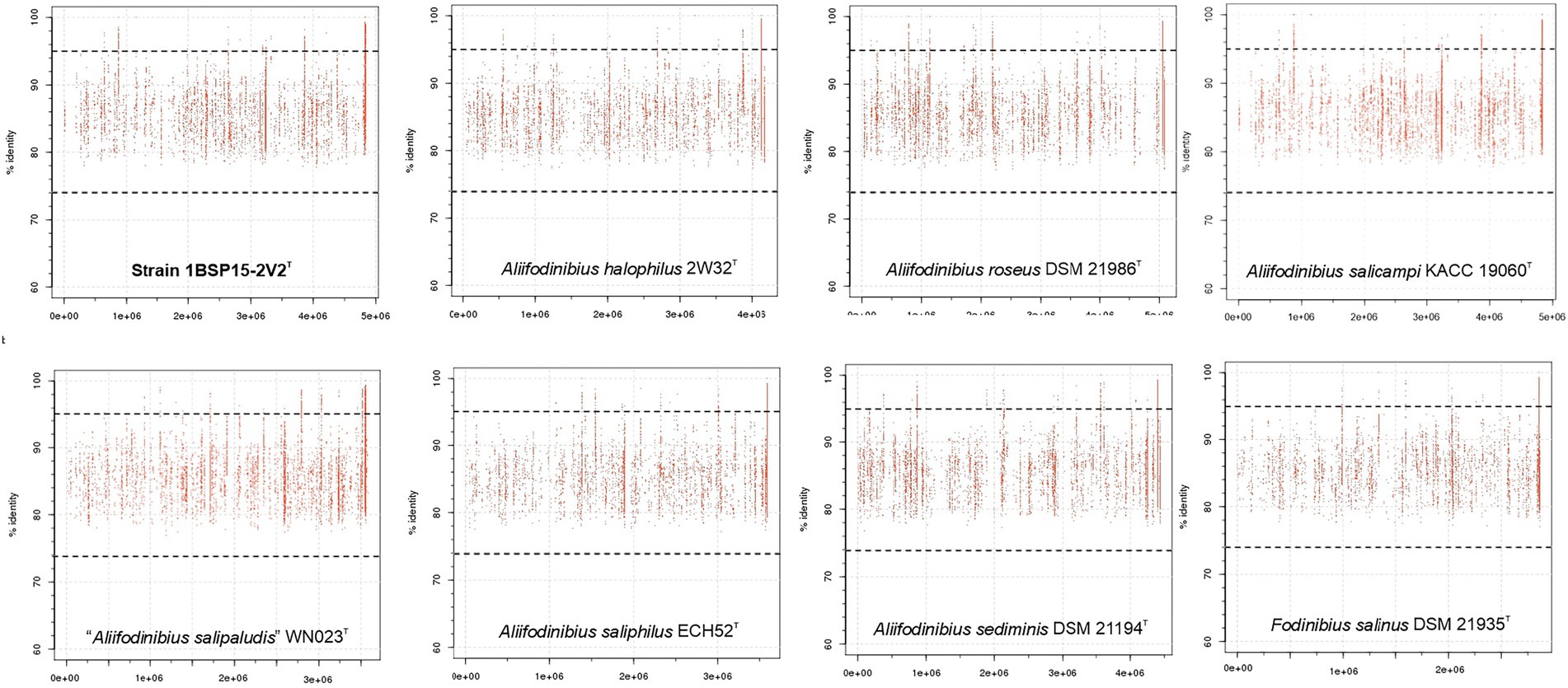
Figure 11. Genomic recruitment plots for the isolated strain 1BSP15-2V2T and other type strains of species of the genera Aliifodinibius and Fodinibius against SS13 saltern metagenomic reads. X-axis represents the genome length and Y-axis represents the percentage of identity of the reads matching the genome. Red dots above the 95% threshold line indicate read assignment at the species level. A genus delineation line is also shown at 73.98% identity. The scarce read recruitment by all the species analyzed demonstrates their low abundance in this aquatic environment.
4. Conclusion
Phylogenetic and genomic evidence, fatty acid profiles, and phenotypic characteristics of the microorganisms under study show unequivocally that the species of the genera Aliifodinibius and Fodinibius should be placed into a single genus. We propose the reclassification of the members of the genus Aliifodinibius into the genus Fodinibius, since the genus name Fodinibius was validly published first and, thus, it has priority over the genus name Aliifodinibius, according to the International Code of Nomenclature of Prokaryotes (Parker et al., 2019). Additionally, strain 1BSP15-2V2T represents a new species of the genus Fodinibius, for which the name Fodinibius salsisoli sp. nov. is proposed. The description of the new species, as well as the reclassification of the species of Aliifodinibius into the genus Fodinibius and, consequently, the emended description of the genus Fodinibius are enclosed below.
Description of Fodinibius salsisoli sp. nov.
Fodinibius salsisoli (sal.si.so’li. L. masc. adj. salsus salty; L.; neut. n. solum soil; N.L. gen. n. salsisoli of salty soil).
Cells are Gram-stain-negative, non-endospore-forming, non-motile rods with a size of 0.05–0.10 × 0.20–0.30 μm. Colonies are irregular, opaque, and orange-red pigmented in SMM medium supplemented with 7.5% (w/v) salts after 5 days of incubation at 37°C. Grows between 3 and 20% (w/v) salt concentration (optimally at 9% [w/v]), pH 5.0–8.0 (optimally at pH 6.0), and 14–43°C (optimally at 37°C). Growth is not observed under anaerobic conditions. Catalase positive and oxidase negative. Reduces nitrate and nitrite. Positive for methyl red test but negative for Voges-Proskauer test, indicating that it uses the mixed acid pathway for glucose fermentation. Simmons’ citrate and phenylalanine deaminase are positive. Aesculin is hydrolyzed, but casein, DNA, gelatin, Tween 80, starch, and urea are not. H2S is produced, but not indole. Acids are produced from D-arabinose, D-fructose, D-glucose, maltose, sucrose, and D-xylose but not from D-galactose, glycerol, lactose, mannitol, and D-trehalose. Utilizes amygdaline, D-cellobiose, D-fructose, D-galactose, D-glucose, D-lactose, D-maltose, D-mannose, D-melezitose, ribose, D-raffinose, salicin, sucrose, D-trehalose, D-xylose, dulcitol, ethanol, glycerol, mannitol, D-sorbitol, xylitol, fumarate, hippurate, malate, propionate, and pyruvate as sole sources of carbon and energy, but not L-arabinose, starch, butanol, methanol, propranolol, acetate, benzoate, butyrate, citrate, formate, and valerate. Utilizes L-asparagine, aspartic acid, cysteine, L-glutamine, L-methionine, ornithine, L-phenylalanine, and L-serine as the sole sources of carbon, nitrogen, and energy, but not L-alanine, arginine, L-glutamate, glycine, L-isoleucine, lysine, L-threonine, tryptophan, and valine. The major fatty acids are iso-C15:0, C16:1 ω7c and/or C16:1 ω6c, and iso-C17:1 ω9c and/or 10-methyl C16:0. The genome of the type strain has an approximate size of 4.85 Mb and its G + C content is 44.5 mol%.
The type strain, 1BSP15-2V2T (=CCM 9117T = CECT 30246T), was isolated from hypersaline soils at the Odiel Saltmarshes Natural Area in Huelva (Southwest Spain). The accession number for the 16S rRNA gene sequence is MW811395 and that of the genome sequence GCF_026229185.1.
Description of Fodinibius halophilus comb. nov.
Basonym: Aliifodinibius halophilus Xia et al. 2016.
The description is the same as for Aliifodinibius halophilus (Xia et al., 2016). The genome of the type strain has an approximate size of 4.19 Mb and its G + C content is 42.5 mol%. The accession number for the 16S rRNA gene sequence is KR559733 and that of the genome sequence GCF_011059105.1.
Type strain: 2W32T (=CICC 23869T = KCTC 42497T).
Description of Fodinibius roseus comb. nov.
Basonym: Aliifodinibius roseus Wang et al. 2013.
The description is the same as for Aliifodinibius roseus (Wang et al., 2013). The genome of the type strain has an approximate size of 5.08 Mb and its G + C content is 48.3 mol%. The accession number for its 16S rRNA gene sequence is JQ923475 and that of the genome sequence GCF_900129315.1.
Type strain: YIM D15T (=ACCC 10715T = DSM 21986T = KCTC 23442T).
Description of Fodinibius salicampi comb. nov.
Basonym: Aliifodinibius salicampi Cho et al. 2017.
The description is the same as for Aliifodinibius salicampi (Cho et al., 2017, 2018). The genome of the type strain has an approximate size of 3.94 Mb and its G + C content is 42.8 mol%. The accession number for its 16S rRNA gene sequence is LC198077 and that of the genome sequence GCF_026228885.1.
Type strain: NBRC 112531T (=KACC 19060T = KHM44T).
Description of Fodinibius saliphilus comb. nov.
Basonym: Aliifodinibius saliphilus Cho and Whang 2020.
The description is the same as for Aliifodinibius saliphilus (Cho and Whang, 2020). The genome of the type strain has an approximate size of 3.60 Mb and its G + C content is 40.8 mol%. The accession number for its 16S rRNA gene sequence is LC198072 and that of the genome sequence GCF_005869845.1.
Type strain: ECH52T (=KACC 19126T = NBRC 112664T).
Description of Fodinibius sediminis comb. nov.
Basonym: Aliifodinibius sediminis Wang et al. 2013.
The description is the same as for Aliifodinibius sediminis (Wang et al., 2013). The genome of the type strain has an approximate size of 4.43 Mb and its G + C content is 48.1 mol%. The accession number for its 16S rRNA gene sequence is JQ923475 and that of the genome sequence GCF_900182555.1.
Type strain: YIM J21T (=ACCC 10714T = DSM 21194T).
Emended description of the genus Fodinibius Wang et al. 2012
Cells are Gram-stain-negative, non-endospore-forming, non-motile rods, 0.05–1.5 μm in width and 0.2–5.5 μm in length (Wang et al., 2012). Colonies are salmon pink, pink, rose red, and reddish pigmented, circular, convex, and opaque with regular margins, and sometimes viscid. Some species are strictly aerobic bacteria, while others possess a facultatively anaerobic metabolism. Temperature range for growth between 14 and 45°C (optimum 37°C). Growth occurs at 3–25% (w/v) NaCl (optimum at 8–10%) and at pH values 5.0–10.0 (optimum at 6.0–8.0). All species produce catalase and most of them also oxidase. All species are unable to hydrolyze starch and DNA, and they cannot produce indole from tryptophane. Major fatty acids are iso-C15:0, iso-C17:1 ω9c, anteiso-C15:0, C16:1 ω7c and/or C16:1 ω6c, C16:1 ω7c and/or iso-C15:0 2-OH, and iso-C17:1 ω9c and/or 10-methyl C16:0. The DNA G + C content ranges between 40.8–48.3 mol% (genome).
The type species is Fodinibius salinus. The genome of the type strain of this type species has an approximate size of 2.86 Mb and its G + C content is 42.5 mol%. The genus is member of the family Balneolaceae, order Balneolales, class Balneolia, phylum Balneolota.
Data availability statement
The datasets presented in this study can be found in online repositories. The names of the repository/repositories and accession number(s) can be found at: https://www.ncbi.nlm.nih.gov/genbank/, JAGGJA000000000 https://www.ncbi.nlm.nih.gov/genbank/, JAJNDC000000000 https://www.ncbi.nlm.nih.gov/genbank/, MW811395.
Author contributions
AV, CS-P, and RRH conceived the study. CG, CS-P, and AV acquired the environmental samples. CG performed the laboratory experiments and bioinformatic analysis, supported by CS-P and RRH, respectively. CG drafted the manuscript. CG, CS-P, RRH, and AV revised the manuscript. All authors contributed to the article and approved the submitted version.
Funding
This study was supported by grants PID2020-118136GB-I00 funded by MCIN/AEI/10.13039/501100011033 and ERDF A way of making Europe, and from the Junta de Andalucía (P20_01066 and BIO-213), both with FEDER funds. CG was a recipient of a predoctoral fellowship (PRE2018-083242) from the Spanish Ministry of Science and Innovation. RRH was a recipient of a short-stay grant (PRX21/00598) from the Spanish Ministry of Universities.
Acknowledgments
We thank A. Oren (The Hebrew University of Jerusalem) for his help on the nomenclature of the new species.
Conflict of interest
The authors declare that the research was conducted in the absence of any commercial or financial relationships that could be construed as a potential conflict of interest.
Publisher’s note
All claims expressed in this article are solely those of the authors and do not necessarily represent those of their affiliated organizations, or those of the publisher, the editors and the reviewers. Any product that may be evaluated in this article, or claim that may be made by its manufacturer, is not guaranteed or endorsed by the publisher.
Supplementary material
The Supplementary material for this article can be found online at: https://www.frontiersin.org/articles/10.3389/fmicb.2022.1101464/full#supplementary-material
Footnotes
References
Abdel-Hamid, A. M., and Cronan, J. E. (2007). Coordinate expression of the acetyl coenzyme a carboxylase genes, accB and accC, is necessary for normal regulation of biotin synthesis in Escherichia coli. J. Bacteriol. 189, 369–376. doi: 10.1128/JB.01373-06
Alquethamy, S. F., Adams, F. G., Maharjan, R., Delgado, N. N., Zang, M., Ganio, K., et al. (2021). The molecular basis of Acinetobacter baumannii cadmium toxicity and resistance. Appl. Environ. Microbiol. 87:e0171821. doi: 10.1128/AEM.01718-21
Antón, J., Oren, A., Benlloch, S., Rodríguez-Valera, F., Amann, R., and Rosselló-Mora, R. (2002). Salinibacter ruber gen. nov., sp. nov., a novel, extremely halophilic member of the bacteria from saltern crystallizer ponds. Int. J. Syst. Evol. Microbiol. 52, 485–491. doi: 10.1099/00207713-52-2-485
Auch, A. F., Klenk, H. P., and Göker, M. (2010). Standard operating procedure for calculating genome-to-genome distances based on high-scoring segment pairs. Stand. Genomic Sci. 2, 142–148. doi: 10.4056/sigs.541628
Barco, R. A., Garrity, G. M., Scott, J. J., Amend, J. P., Nealson, K. H., and Emerson, D. (2020). A genus definition for Bacteria and Archaea based on a standard genome relatedness index. mBio 11, e02475–e02419. doi: 10.1128/mBio.02475-19
Bi, H., Zhu, L., Jia, J., and Cronan, J. E. (2016). A biotin biosynthesis gene restricted to Helicobacter. Sci. Rep. 6:21162. doi: 10.1038/srep21162
Booth, I. R. (2014). Bacterial mechanosensitive channels: progress towards an understanding of their roles in cell physiology. Curr. Opin. Microbiol. 18, 16–22. doi: 10.1016/j.mib.2014.01.005
Booth, I. R., and Blount, P. (2012). The MscS and MscL families of mechanosensitive channels act as microbial emergency release valves. J. Bacteriol. 194, 4802–4809. doi: 10.1128/JB.00576-12
Carver, T., Thomson, N., Bleasby, A., Berriman, M., and Parkhill, J. (2009). DNAPlotter: circular and linear interactive genome visualization. Bioinformatics 25, 119–120. doi: 10.1093/bioinformatics/btn578
Castelán-Sánchez, H. G., Elorrieta, P., Romoacca, P., Liñan-Torres, A., Sierra, J. L., Vera, I., et al. (2019). Intermediate-salinity systems at high altitudes in the Peruvian Andes unveil a high diversity and abundance of bacteria and viruses. Genes (Basel) 10:891. doi: 10.3390/genes10110891
Chauhan, D., Srivastava, P. A., Agnihotri, V., Yennamalli, R. M., and Priyadarshini, R. (2019). Structure and function prediction of arsenate reductase from Deinococcus indicus DR1. J. Mol. Model. 25:15. doi: 10.1007/s00894-018-3885-3
Chen, S. C., Sun, G. X., Yan, Y., Konstantinidis, K. T., Zhang, S. Y., Deng, Y., et al. (2020). The great oxidation event expanded the genetic repertoire of arsenic metabolism and cycling. Proc. Natl. Acad. Sci. U. S. A. 117, 10414–10421. doi: 10.1073/pnas.2001063117
Cho, Y., Chung, H., Jang, G. I., Choi, D. H., Noh, J. H., and Cho, B. C. (2013). Gracilimonas rosea sp. nov., isolated from tropical seawater, and emended description of the genus Gracilimonas. Int. J. Syst. Evol. Microbiol. 63, 4006–4011. doi: 10.1099/ijs.0.052340-0
Cho, G. Y., Lee, J. C., and Whang, K. S. (2017). Aliifodinibius salicampi sp. nov., a moderately halophilic bacterium isolated from a grey saltern. Int. J. Syst. Evol. Microbiol. 67, 2598–2603. doi: 10.1099/ijsem.0.001981
Cho, G. Y., Lee, J. C., and Whang, K. S. (2018). Erratum: Aliifodinibius salicampi sp. nov., a moderately halophilic bacterium isolated from a grey saltern. Int. J. Syst. Evol. Microbiol. 68:692. doi: 10.1099/ijsem.0.002572
Cho, G. Y., and Whang, K. S. (2020). Aliifodinibius saliphilus sp. nov., a moderately halophilic bacterium isolated from sediment of a crystallizing pond of a saltern. Int. J. Syst. Evol. Microbiol. 70, 358–363. doi: 10.1099/ijsem.0.003765
Choi, D. H., Zhang, G. I., Noh, J. H., Kim, W.-S., and Cho, B. C. (2009). Gracilimonas tropica gen. nov., sp. nov., isolated from a Synechococcus culture. Int. J. Syst. Evol. Microbiol. 59, 1167–1172. doi: 10.1099/ijs.0.005512-0
Choi-Rhee, E., and Cronan, J. E. (2003). The biotin carboxylase-biotin carboxyl carrier protein complex of Escherichia coli acetyl-CoA carboxylase. J. Biol. Chem. 278, 30806–30812. doi: 10.1074/jbc.M302507200
Chun, J., Oren, A., Ventosa, A., Christensen, H., Arahal, D. R., da Costa, M. S., et al. (2018). Proposed minimal standards for the use of genome data for the taxonomy of prokaryotes. Int. J. Syst. Evol. Microbiol. 68, 461–466. doi: 10.1099/ijsem.0.002516
Chun, J., and Rainey, F. A. (2014). Integrating genomics into the taxonomy and systematics of the Bacteria and Archaea. Int. J. Syst. Evol. Microbiol. 64, 316–324. doi: 10.1099/ijs.0.054171-0
Consejería de Medio Ambiente (1999). Los criterios y estándares para declarar un suelo contaminado en Andalucía y la metodología y técnicas de toma de muestra y análisis para su investigación. Sevilla: Junta de Andalucía.
Conway, J. R., Lex, A., and Gehlenborg, N. (2017). UpSetR: an R package for the visualization of intersecting sets and their properties. Bioinformatics 33, 2938–2940. doi: 10.1093/bioinformatics/btx364
Cowan, S. T., and Steel, K. J. (1965). Manual for the Identification of Medical Bacteria. Cambridge: University Press.
Cronan, J. E. (2018). Advances in synthesis of biotin and assembly of lipoic acid. Curr. Opin. Chem. Biol. 47, 60–66. doi: 10.1016/j.cbpa.2018.08.004
Cronan, J. E., Stewart, V., and Begley, T. (2014). Biotin and lipoic acid: synthesis, attachment, and regulation. EcoSal Plus 6:2012. doi: 10.1128/ecosalplus.ESP-0001-2012
Doxey, A. C., Kurtz, D. A., Lynch, M. D. J., Sauder, L. A., and Neufeld, J. D. (2015). Aquatic metagenomes implicate Thaumarchaeota in global cobalamin production. ISME J. 9, 461–471. doi: 10.1038/ismej.2014.142
Durán-Viseras, A., Andrei, A.-S., Ghai, R., Sánchez-Porro, C., and Ventosa, A. (2019). New Halonotius species provide genomics-based insights into cobalamin synthesis in haloarchaea. Front. Microbiol. 10:1928. doi: 10.3389/fmicb.2019.01928
Edgar, R. C. (2004). MUSCLE: multiple sequence alignment with high accuracy and high throughput. Nucleic Acids Res. 32, 1792–1797. doi: 10.1093/nar/gkh340
Elevi Bardavid, R., and Oren, A. (2012). Acid-shifted isoelectric point profiles of the proteins in a hypersaline microbial mat: an adaptation to life at high salt concentrations? Extremophiles 16, 787–792. doi: 10.1007/s00792-012-0476-6
Felsenstein, J. (1981). Evolutionary trees from DNA sequences: a maximum likelihood approach. J. Mol. Evol. 17, 368–376. doi: 10.1007/BF01734359
Felsenstein, J. (1983). Parsimony in systematics: biological and statistical issues. Annu. Rev. Ecol. Syst. 14, 313–333. doi: 10.1146/annurev.es.14.110183.001525
Felsenstein, J. (1985). Confidence limits on phylogenies: an approach using the bootstrap. Evolution 39, 783–791. doi: 10.1111/j.1558-5646.1985.tb00420.x
Feng, Y., Napier, B. A., Manandhar, M., Henke, S. K., Weiss, D. S., and Cronan, J. E. (2014). A Francisella virulence factor catalyses an essential reaction of biotin synthesis. Mol. Microbiol. 91, 300–314. doi: 10.1111/mmi.12460
Goris, J., Konstantinidis, K. T., Klappenbach, J. A., Coenye, T., Vandamme, P., and Tiedje, J. M. (2007). DNA-DNA hybridization values and their relationship to whole-genome sequence similarities. Int. J. Syst. Evol. Microbiol. 57, 81–91. doi: 10.1099/ijs.0.64483-0
Gurevich, A., Saveliev, V., Vyahhi, N., and Tesler, G. (2013). QUAST: quality assessment tool for genome assemblies. Bioinformatics 29, 1072–1075. doi: 10.1093/bioinformatics/btt086
Hoffmann, T., and Bremer, E. (2016). “Management of osmotic stress by Bacillus subtilis: genetics and physiology” in Stress and Environmental Regulation of Gene Expression and Adaptation in Bacteria. ed. F. J. de Bruijn (New York: John Wiley & Sons), 657–676.
Hoffmann, T., and Bremer, E. (2017). Guardians in a stressful world: the Opu family of compatible solute transporters from Bacillus subtilis. Biol. Chem. 398, 193–214. doi: 10.1515/hsz-2016-0265
Holtmann, G., Bakker, E. P., Uozumi, N., and Bremer, E. (2003). KtrAB and KtrCD: two K+ uptake systems in Bacillus subtilis and their role in adaptation to hypertonicity. Society 185, 1289–1298. doi: 10.1128/JB.185.4.1289
Hvitfeldt, E. (2021). Paletteer: Comprehensive Collection of Color Palettes. Available at: https://github.com/EmilHvitfeldt/paletteer
Hyatt, D., Chen, G.-L., Locascio, P. F., Land, M. L., Larimer, F. W., and Hauser, L. J. (2010). Prodigal: prokaryotic gene recognition and translation initiation site identification. BMC Bioinformatics 11:119. doi: 10.1186/1471-2105-11-119
Islam, M. N., Suzauddula, M., Ahamed, Z., Rabby, M. G., Hossen, M. M., Biswas, M., et al. (2022). Phylogenetic analysis and characterization of arsenic (As) transforming bacterial marker proteins following isolation of As-tolerant indigenous bacteria. Arch. Microbiol. 204:660. doi: 10.1007/s00203-022-03270-5
Jitrapakdee, S., and Wallace, J. C. (2003). The biotin enzyme family: conserved structural motifs and domain rearrangements. Curr. Protein Pept. Sci. 4, 217–229. doi: 10.2174/1389203033487199
Jones, D. T., Taylor, W. R., and Thornton, J. M. (1992). The rapid generation of mutation data matrices from protein sequences. Comput. Appl. Biosci. 8, 275–282. doi: 10.1093/bioinformatics/8.3.275
Jukes, T. H., and Cantor, C. R. (1969). “Evolution of protein molecules” in Mammalian Protein Metabolism. ed. H. N. Munro (London: Academic Press), 21–132.
Kanehisa, M., Sato, Y., and Morishima, K. (2016). BlastKOALA and GhostKOALA: KEGG tools for functional characterization of genome and metagenome sequences. J. Mol. Biol. 428, 726–731. doi: 10.1016/j.jmb.2015.11.006
Khan, Z., Nisar, M. A., Hussain, S. Z., Arshad, M. N., and Rehman, A. (2015). Cadmium resistance mechanism in Escherichia coli P4 and its potential use to bioremediate environmental cadmium. Appl. Microbiol. Biotechnol. 99, 10745–10757. doi: 10.1007/s00253-015-6901-x
Kimbrel, J. A., Ballor, N., Wu, Y. W., David, M. M., Hazen, T. C., Simmons, B. A., et al. (2018). Microbial community structure and functional potential along a hypersaline gradient. Front. Microbiol. 9:1492. doi: 10.3389/fmicb.2018.01492
Knowles, J. R. (1989). The mechanism of biotin-dependent enzymes. Annu. Rev. Biochem. 58, 195–221. doi: 10.1146/annurev.bi.58.070189.001211
Konstantinidis, K. T., Rosselló-Móra, R., and Amann, R. (2017). Uncultivated microbes in need of their own taxonomy. ISME J. 11, 2399–2406. doi: 10.1038/ismej.2017.113
Konstantinidis, K. T., and Tiedje, J. M. (2007). Prokaryotic taxonomy and phylogeny in the genomic era: advancements and challenges ahead. Curr. Opin. Microbiol. 10, 504–509. doi: 10.1016/j.mib.2007.08.006
Koser, S. A. (1923). Utilization of the salts of organic acids by the colon-aerogenes group. J. Bacteriol. 8, 493–520. doi: 10.1128/jb.8.5.493-520.1923
Kovacs, N. (1956). Identification of Pseudomonas pyocyanea by the oxidase reaction. Nature 178:703. doi: 10.1038/178703a0
Krulwich, T. A., Sachs, G., and Padan, E. (2011). Molecular aspects of bacterial pH sensing and homeostasis. Nat. Rev. Microbiol. 9, 330–343. doi: 10.1038/nrmicro2549
Lane, D. (1991). “16S/23S rRNA sequencing” in Nucleic acid Techniques in Bacterial Systematics. ed. E. Stackebrandt (New York: Wiley), 115–175.
Lee, I., Ouk Kim, Y., Park, S.-C., and Chun, J. (2016). OrthoANI: an improved algorithm and software for calculating average nucleotide identity. Int. J. Syst. Evol. Microbiol. 66, 1100–1103. doi: 10.1099/ijsem.0.000760
Legatzki, A., Grass, G., Anton, A., Rensing, C., and Nies, D. H. (2003). Interplay of the Czc system and two P-type ATPases in conferring metal resistance to Ralstonia metallidurans. J. Bacteriol. 185, 4354–4361. doi: 10.1128/JB.185.15.4354-4361.2003
León, M. J., Vera-Gargallo, B., Sánchez-Porro, C., and Ventosa, A. (2016). Spiribacter roseus sp. nov., a moderately halophilic species of the genus Spiribacter from salterns. Int. J. Syst. Evol. Microbiol. 66, 4218–4224. doi: 10.1099/ijsem.0.001338
León-Del-Río, A. (2019). Biotin in metabolism, gene expression, and human disease. J. Inherit. Metab. Dis. 42, 647–654. doi: 10.1002/jimd.12073
Letunic, I., and Bork, P. (2021). Interactive Tree Of Life (iTOL) v5: an online tool for phylogenetic tree display and annotation. Nucleic Acids Res. 49, W293–W296. doi: 10.1093/nar/gkab301
Lin, S., and Cronan, J. E. (2011). Closing in on complete pathways of biotin biosynthesis. Mol. BioSyst. 7, 1811–1821. doi: 10.1039/c1mb05022b
Ludwig, W., Strunk, O., Westram, R., Richter, L., Meier, H., Yadhukumar,, et al. (2004). ARB: a software environment for sequence data. Nucleic Acids Res. 32, 1363–1371. doi: 10.1093/nar/gkh293
Manandhar, M., and Cronan, J. E. (2018). A canonical biotin synthesis enzyme, 8-amino-7-oxononanoate synthase (BioF), utilizes different acyl chain donors in Bacillus subtilis and Escherichia coli. Appl. Environ. Microbiol. 84, e02084–e02017. doi: 10.1128/AEM.02084-17
Manara, A., DalCorso, G., Baliardini, C., Farinati, S., Cecconi, D., and Furini, A. (2012). Pseudomonas putida response to cadmium: changes in membrane and cytosolic proteomes. J. Proteome Res. 11, 4169–4179. doi: 10.1021/pr300281f
Marmur, J. (1961). A procedure for the isolation of deoxyribonucleic acid from micro-organisms. J. Mol. Biol. 3, 208–218. doi: 10.1016/S0022-2836(61)80047-8
Meier-Kolthoff, J. P., Carbasse, J. S., Peinado-Olarte, R. L., and Göker, M. (2021). TYGS and LPSN: a database tandem for fast and reliable genome-based classification and nomenclature of prokaryotes. Nucleic Acids Res. 50, D801–D807. doi: 10.1093/nar/gkab902
MIDI (2008). Sherlock Microbial Identification System Operating Manual, version 6.1. Newark, DE: MIDI Inc.
Morris, M. S. (2012). The role of B vitamins in preventing and treating cognitive impairment and decline. Adv. Nutr. 3, 801–812. doi: 10.3945/an.112.002535
Munoz, R., Rosselló-Móra, R., and Amann, R. (2016). Revised phylogeny of Bacteroidetes and proposal of sixteen new taxa and two new combinations including Rhodothermaeota phyl. nov. Syt. Appl. Microbiol. 39, 281–296. doi: 10.1016/j.syapm.2016.04.004
Nakazawa, M. (2022). Fmsb: Functions for Medical Statistics Book with Some Demographic Data. R Package Version 0.3. Available at: https://cran.r-project.org/package=fmsb
Noll, M., and Lutsenko, S. (2000). Expression of ZntA, a zinc-transporting P1-type ATPase, is specifically regulated by zinc and cadmium. IUBMB Life 49, 297–302. doi: 10.1080/15216540050033168
Oren, A. (2008). Microbial life at high salt concentrations: phylogenetic and metabolic diversity. Saline Syst. 4:2. doi: 10.1186/1746-1448-4-2
Oren, A. (2013). Life at high salt concentrations, intracellular KCl concentrations, and acidic proteomes. Front. Microbiol. 4:315. doi: 10.3389/fmicb.2013.00315
Parker, C. T., Tindall, B. J., and Garrity, G. M. (2019). International code of nomenclature of prokaryotes. Int. J. Syst. Evol. Microbiol. 69, S1–S111. doi: 10.1099/ijsem.0.000778
Parks, D. H., Imelfort, M., Skennerton, C. T., Hugenholtz, P., and Tyson, G. W. (2015). CheckM: assessing the quality of microbial genomes recovered from isolates, single cells, and metagenomes. Genome Res. 25, 1043–1055. doi: 10.1101/gr.186072.114
Parte, A. C., Sardà Carbasse, J., Meier-Kolthoff, J. P., Reimer, L. C., and Göker, M. (2020). List of prokaryotic names with standing in nomenclature (LPSN) moves to the DSMZ. Int. J. Syst. Evol. Microbiol. 70, 5607–5612. doi: 10.1099/ijsem.0.004332
Patiño-Ruiz, M., Ganea, C., and Călinescu, O. (2022). Prokaryotic Na+/H+ exchangers – transport mechanism and essential residues. Int. J. Mol. Sci. 23:9156. doi: 10.3390/ijms23169156
Price, M. N., Dehal, P. S., and Arkin, A. P. (2010). FastTree 2 – approximately maximum-likelihood trees for large alignments. PLoS One 5:e9490. doi: 10.1371/journal.pone.0009490
Prjibelski, A., Antipov, D., Meleshko, D., Lapidus, A., and Korobeynikov, A. (2020). Using SPAdes de novo assembler. Curr. Protoc. Bioinformatics 70:e102. doi: 10.1002/cpbi.102
Rensing, C., Mitra, B., and Rosen, B. P. (1997). The zntA gene of Escherichia coli encodes a Zn(II)-translocating P-type ATPase. Proc. Natl. Acad. Sci. U. S. A. 94, 14326–14331. doi: 10.1073/pnas.94.26.14326
Rice, P., Longden, I., and Bleasby, A. (2000). EMBOSS: the European molecular biology open software suite. Trends Genet. 16, 276–277. doi: 10.1016/S0168-9525(00)02024-2
Richter, M., and Rosselló-Móra, R. (2009). Shifting the genomic gold standard for the prokaryotic species definition. Proc. Natl. Acad. Sci. U. S. A. 106, 19126–19131. doi: 10.1073/pnas.0906412106
Rodionov, D. A., Mironov, A. A., and Gelfand, M. S. (2002). Conservation of the biotin regulon and the BirA regulatory signal in Eubacteria and Archaea. Genome Res. 12, 1507–1516. doi: 10.1101/gr.314502
Rodriguez-R, L. M., and Konstantinidis, K. T. (2016). The Enveomics collection: a toolbox for specialized analyses of microbial genomes and metagenomes. PeerJ. Prepr. 4:e1900v1. doi: 10.7287/peerj.preprints.1900v1
Sainz, A., Grande, J. A., and de la Torre, M. L. (2004). Characterization of heavy metal discharge into the ria of Huelva. Environ. Int. 30, 557–566. doi: 10.1016/j.envint.2003.10.013
Sainz, A., Grande, J. A., de La Torre, M. L., and Sánchez-Rodas, D. (2002). Characterization of sequential leachate discharges of mining waste rock dumps in the Tinto and Odiel rivers. J. Environ. Manag. 64, 345–353. doi: 10.1006/jema.2001.0497
Saitou, N., and Nei, M. (1987). The neighbor-joining method: a new method for reconstructing phylogenetic trees. Mol. Biol. Evol. 4, 406–425. doi: 10.1093/oxfordjournals.molbev.a040454
Sasser, M. (1990). Identification of bacteria by gas chromatography of cellular fatty acids. Technical Note 101, 1–6.
Seemann, T. (2014). Prokka: rapid prokaryotic genome annotation. Bioinformatics 30, 2068–2069. doi: 10.1093/bioinformatics/btu153
Shapiro, M. M., Chakravartty, V., and Cronan, J. E. (2012). Remarkable diversity in the enzymes catalyzing the last step in synthesis of the pimelate moiety of biotin. PLoS One 7:e49440. doi: 10.1371/journal.pone.0049440
Shi, J., Cao, X., Chen, Y., Cronan, J. E., and Guo, Z. (2016). An atypical α/β-hydrolase fold revealed in the crystal structure of pimeloyl-acyl carrier protein methyl esterase BioG from Haemophilus influenzae. Biochemistry 55, 6705–6717. doi: 10.1021/acs.biochem.6b00818
Shimodaira, H., and Hasegawa, M. (1999). Multiple comparisons of log-likelihoods with applications to phylogenetic inference. Mol. Biol. Evol. 16, 1114–1116. doi: 10.1093/oxfordjournals.molbev.a026201
Sirithanakorn, C., and Cronan, J. E. (2021). Biotin, a universal and essential cofactor: synthesis, ligation and regulation. FEMS Microbiol. Rev. 45:fuab003. doi: 10.1093/femsre/fuab003
Stackebrandt, E., and Goebel, B. M. (1994). Taxonomic note: a place for DNA-DNA reassociation and 16S rRNA sequence analysis in the present species definition in bacteriology. Int. J. Syst. Evol. Microbiol. 44, 846–849. doi: 10.1099/00207713-44-4-846
Teichmann, L., Kümmel, H., Warmbold, B., and Bremer, E. (2018). OpuF, a new Bacillus compatible solute ABC transporter with a substrate-binding protein fused to the transmembrane domain. Appl. Environ. Microbiol. 84:01718. doi: 10.1128/AEM.01728-18
Urios, L., Agogué, H., Lesongeur, F., Stackebrandt, E., and Lebaron, P. (2006). Balneola vulgaris gen. nov., sp. nov., a member of the phylum Bacteroidetes from the North-Western Mediterranean Sea. Int. J. Syst. Evol. Microbiol. 56, 1883–1887. doi: 10.1099/ijs.0.64285-0
Vavourakis, C. D., Andrei, A. S., Mehrshad, M., Ghai, R., Sorokin, D. Y., and Muyzer, G. (2018). A metagenomics roadmap to the uncultured genome diversity in hypersaline soda lake sediments. Microbiome 6:168. doi: 10.1186/s40168-018-0548-7
Ventosa, A., Quesada, E., Rodriguez-Valera, F., Ruiz-Berraquero, F., and Ramos-Cormenzana, A. (1982). Numerical taxonomy of moderately halophilic Gram-negative rods. Microbiology 128, 1959–1968. doi: 10.1099/00221287-128-9-1959
Vera-Gargallo, B., Chowdhury, T. R., Brown, J., Fansler, S. J., Durán-Viseras, A., Sánchez-Porro, C., et al. (2019). Spatial distribution of prokaryotic communities in hypersaline soils. Sci. Rep. 9:1769. doi: 10.1038/s41598-018-38339-z
Vera-Gargallo, B., and Ventosa, A. (2018). Metagenomic insights into the phylogenetic and metabolic diversity of the prokaryotic community dwelling in hypersaline soils from the Odiel saltmarshes (SW Spain). Genes (Basel) 9:152. doi: 10.3390/genes9030152
Wang, Y. X., Liu, J. H., Xiao, W., Ma, X. L., Lai, Y. H., Li, Z. Y., et al. (2013). Aliifodinibius roseus gen. nov., sp. nov., and Aliifodinibius sediminis sp. nov., two moderately halophilic bacteria isolated from salt mine samples. Int. J. Syst. Evol. Microbiol. 63, 2907–2913. doi: 10.1099/ijs.0.043869-0
Wang, Y. X., Liu, J. H., Xiao, W., Zhang, X. X., Li, Y. Q., Lai, Y. H., et al. (2012). Fodinibius salinus gen. nov., sp. nov., a moderately halophilic bacterium isolated from a salt mine. Int. J. Syst. Evol. Microbiol. 62, 390–396. doi: 10.1099/ijs.0.025502-0
Wei, W., Guan, H., Zhu, T., Zhang, S., Fan, C., Ouyang, S., et al. (2019). Molecular basis of BioJ, a unique gatekeeper in bacterial biotin synthesis. iScience. 19, 796–808. doi: 10.1016/j.isci.2019.08.028
Woodson, J. D., Peck, R. F., Krebs, M. P., and Escalante-Semerena, J. C. (2003). The cobY gene of the archaeon Halobacterium sp. strain NRC-1 is required for de novo cobamide synthesis. J. Bacteriol. 185, 311–316. doi: 10.1128/JB.185.1.311-316.2003
Wu, S., Wang, J., Wang, J., Du, X., Ran, Q., Chen, Q., et al. (2022a). Halalkalibacterium roseum gen. nov., sp. nov., a new member of the family Balneolaceae isolated from soil. Int. J. Syst. Evol. Microbiol. 72:005339. doi: 10.1099/ijsem.0.005339
Wu, S., Wang, J., Wang, J., Du, X., Ran, Q., Chen, Q., et al. (2022b). Corrigendum: Halalkalibacterium roseum gen. nov., sp. nov., a new member of the family Balneolaceae isolated from soil. Int. J. Syst. Evol. Microbiol. 72:005451. doi: 10.1099/ijsem.0.005451
Xia, J., Ling, S. K., Wang, X. Q., Chen, G. J., and Du, Z. J. (2016). Aliifodinibius halophilus sp. nov., a moderately halophilic member of the genus Aliifodinibius, and proposal of Balneolaceae fam. nov. Int. J. Syst. Evol. Microbiol. 66, 2225–2233. doi: 10.1099/ijsem.0.001012
Xia, J., Xie, Z.-H., Dunlap, C. A., Rooney, A. P., and Du, Z.-J. (2017). Rhodohalobacter halophilus gen. nov., sp. nov., a moderately halophilic member of the family Balneolaceae. Int. J. Syst. Evol. Microbiol. 67, 1281–1287. doi: 10.1099/ijsem.0.001806
Yoon, S.-H., Ha, S.-M., Kwon, S., Lim, J., Kim, Y., Seo, H., et al. (2017). Introducing EzBioCloud: a taxonomically united database of 16S rRNA gene sequences and whole-genome assemblies. Int. J. Syst. Evol. Microbiol. 67, 1613–1617. doi: 10.1099/ijsem.0.001755
Youssef, N. H., Savage-Ashlock, K. N., McCully, A. L., Luedtke, B., Shaw, E. I., Hoff, W. D., et al. (2014). Trehalose/2-sulfotrehalose biosynthesis and glycine-betaine uptake are widely spread mechanisms for osmoadaptation in the Halobacteriales. ISME J. 8, 636–649. doi: 10.1038/ismej.2013.165
Zhang, S., Xu, Y., Guan, H., Cui, T., Liao, Y., Wei, W., et al. (2021). Biochemical and structural characterization of the BioZ enzyme engaged in bacterial biotin synthesis pathway. Nat. Commun. 12:2056. doi: 10.1038/s41467-021-22360-4
Zhao, X., Miao, S., Sun, Y., Gong, Q., Zhao, J., Wang, J., et al. (2020). Aliifodinibius salipaludis sp. nov., isolated from saline-alkaline soil. Curr. Microbiol. 77, 1328–1333. doi: 10.1007/s00284-019-01863-w
Keywords: Balneolaceae, hypersaline soils, phylogenomics, biotin biosynthesis, genomic analysis, moderate halophile, taxonomic reclassification
Citation: Galisteo C, de la Haba RR, Sánchez-Porro C and Ventosa A (2023) Biotin pathway in novel Fodinibius salsisoli sp. nov., isolated from hypersaline soils and reclassification of the genus Aliifodinibius as Fodinibius. Front. Microbiol. 13:1101464. doi: 10.3389/fmicb.2022.1101464
Edited by:
Sumit Kumar, Amity Institute of Biotechnology, Amity University, IndiaReviewed by:
Aharon Oren, Hebrew University of Jerusalem, IsraelXueWei Xu, Ministry of Natural Resources, China
Copyright © 2023 Galisteo, de la Haba, Sánchez-Porro and Ventosa. This is an open-access article distributed under the terms of the Creative Commons Attribution License (CC BY). The use, distribution or reproduction in other forums is permitted, provided the original author(s) and the copyright owner(s) are credited and that the original publication in this journal is cited, in accordance with accepted academic practice. No use, distribution or reproduction is permitted which does not comply with these terms.
*Correspondence: Cristina Sánchez-Porro, ✉ c2FucG9yQHVzLmVz; Antonio Ventosa, ✉ dmVudG9zYUB1cy5lcw==