- Department of Plant Production and Genetics, Malayer University, Malayer, Iran
As an essential nutrient for plant growth, nickel's (Ni) requirement is very low, and its augmented level causes environmental pollution and toxicity. Being a root endophytic fungus, Piriformospora indica (P. indica) can be beneficial to many plants under stress and non-stress conditions, particularly in terms of their improved growth performance. P. indica, as evidenced, enhances tolerance and resistance in most plants once they experience a range of stresses caused by biotic and abiotic factors, e.g., diseases and heavy metals. Against this background, the positive effects of P. indica on the tomato plants under Ni-induced stress (300, 600, and 900 mg L−1) were analyzed in three experiments at labs, at greenhouses, and via aeroponics in this study. The growth traits of the tomato plants, such as root length (RL) and root dry weight (RDW), were accordingly found to be positively boosted in the cases treated with P. indica compared to the non-treated ones. Treating with P. indica also thwarted the negative effects of Ni on some biochemical traits, including anthocyanin (Anth), proline (Pro), catalase (CAT), and glutathione peroxidase (GPx), while significantly minimizing the adverse impacts of this heavy metal at different levels on hydrogen peroxide (H2O2). Despite this, the Ni-stressed plants indicated much better traits in the presence of this fungus, compared with the non-treated ones, in most of the cases measured. Moreover, the photosynthetic pigments, i.e., chlorophyll a and b (Chl a & b) and carotenoid content (Carrot), were significantly higher in the tomato plants treated with P. indica under high Ni-induced stress as compared with the non-treated ones under non-Ni conditions, in which these pigments were low. The pro-production was further observed all through the P. indica inoculation, which could aid the treated plants in becoming Ni-stress-tolerant. Finally, the current study contributed to a better understanding of how to use the P. indica symbiosis to induce heavy metal tolerance in tomato plants, such as Ni, to meet the goals of sustainable agriculture.
1. Introduction
The association between the concentration of certain heavy metals in soils and plants has led to contamination that exceeds standard limits. The unprincipled disposal of sewage sludge and industrial effluents in farming fields has also given rise to nickel (Ni) accumulation in soils used for vegetable production and irrigated with untreated wastewater. Ni can thus harm some tissues, and it is further suspected of being responsible for some types of cancer. Moreover, no significant evidence indicates its nutritional value in higher organisms like humans (Ebrahimi et al., 2018). To date, no Ni-specific transporter has also been observed in plant cells for the uptake, but the Ni uptake has occurred by roots due to weak selective cation transporters, such as the Zrt/Irt-like proteins (ZIPs) (Van der Pas and Ingle, 2019). The increasing expression of various parts of the ZIP family has been additionally demonstrated in ribonucleic acid (RNA)-sequencing, whereas Senecio coronatus (Thunb.) Harv. in the family Asteraceae has shown the high expression of a ZIP transporter in roots, but this was not the considerable case in non-accumulating plants (Meier et al., 2018). However, the interpretation of the ZIP transporter behavior according to the sequence similarity models for plant ZIPs seems challenging due to the high rate of gene duplication in the ZIP family. The cytoplasmic chelation of Ni ions by ligands has also been introduced as one of the heavy-metal tolerance mechanisms, but the nature of the ligands, along with their importance, has not been identified. Besides, the amino acid histidine plays a significant role in the Ni transport from roots to shoots, owing to the high expression of the ATP-phosphoribosyl transferase in the amino acid histidine pathway (Sharma et al., 2021). Moreover, the epidermis of the shoot cells is the first location of Ni storage from the xylem, but the transporters for Ni unloading are not detected and may contain parts of the ZIP family (Visioli et al., 2014). Notably, the vacuole is the primary location of Ni deposition in cells.
Nevertheless, Ni has been spotted as an essential micronutrient for some microorganisms, such as algae, as it is used in some cofactors that are not detected in higher cells (Kumar et al., 2021). It is thus vital to plant growth and development and significantly contributes to various physiological processes, such as germination and yield performance. High Ni concentrations also change the metabolic processes of plants by preventing enzyme activity, inhibiting photosynthesis, and disturbing chlorophyll (Chl) biosynthesis (Shahzad et al., 2018). Under Ni-induced pollution, excess Ni, rather than a deficiency, is more commonly found. Its toxic effects on plants involve suppressed mitotic processes, lower plant growth rates, and reduced quantity and quality yield performance (Hassan et al., 2019).
Ni has been further mentioned to generate reactive oxygen species (ROS) in plants, thus causing oxidative stress and leading to the loss of biological processes as well as macromolecules (Barbouti et al., 2021). Even if plants have a defense mechanism to neutralize Ni-induced oxidative stress, such as antioxidant enzyme production, this defense system may not be adequate to moderate the impacts of Ni toxicity under high toxicity.
Notably, economic development has also contributed to environmental pollution by releasing high concentrations of toxic metals into the ecosystem, like Ni, which is a severe contaminant due to its widespread use in the agriculture and industry sectors (Rizwan et al., 2018). In Iran, good-quality tomatoes are grown in the open field because of a sunny environment and the differences between day and night temperatures. The application of phosphate fertilizers in tomato fields has thus led to Ni accumulation in the soils in this region and, consequently, the deposition of this heavy metal in tomatoes (Jalali and Karimi-Mojahed, 2020). The distribution of Ni in tomatoes is accordingly specified by the access to nutrients and the characteristics of soils and fertilizers applied for this purpose (Vittori-Antisari et al., 2015). Utilizing chemical fertilizers and disposing of industrial waste into the environment have further contaminated soils and water with Ni, which represents an environmental problem wherein this metal can be accumulated in crops and transferred to the food chain (Correia et al., 2018).
As evidenced, the inoculation of cherry tomato (Lycopersicon esculentum) with Piriformospora indica (P. indica), as a root endophytic fungus, had thus significantly improved tomato seedling growth under non-stress conditions (Varma et al., 2013; Anith et al., 2015). Nouh et al. (2020) additionally reported the positive effects of P. indica on salt stress tolerance and disease resistance in such plants. Similarly, Athira and Anith (2020) could increase the resistance of tomato plants to biotic stress caused by Fusarium wilt. Ghorbani et al. (2019) also observed the positive effects of P. indica on abiotic salinity tolerance and the growth of tomato plants under salinity conditions. Furthermore, P. indica has been so far used for heavy-metal toxicity tolerance in different plants, including tomatoes; for example, Baghaie and Aghili (2021) studied the effects of P. indica on lead toxicity in tomatoes and concluded that the antioxidant enzyme activities had been significantly amplified in roots in comparison to stems. However, it seems that the root treatment of different plants with useful fungi can promote some growth traits and enhance yield performance under non-stress conditions and even increase the resistance and tolerance of plants to biotic and abiotic stress, such as diseases and heavy metal toxicity (Aslam et al., 2019; Berni et al., 2019). Thus, this study investigated the effects of the root endophytic fungus, P. indica, on reducing Ni phytotoxicity in tomato plants, as the tomato fields are currently often being contaminated with sewage sludge and industrial effluents, and even irrigated with untreated wastewater.
2. Materials and methods
2.1. Fungus and plant inoculation
The fungus P. indica was gotten originally from the Department of Plant Production and Genetics, Faculty of Agriculture, Malayer University, Iran, and then grown on a complex medium. After the surface sterilization of the tomato cultivar ProSeed Urbana seeds with sodium hypochlorite (2%) for 10 min, they were germinated and inoculated with the suspension of P. indica spores and cultivated in pots containing sterile PittMoss. To generate spore suspension, the spores were released by gently scratching the fungus surface on the Petri dishes with a spatula. The spores were collected by centrifugation at 4000 rpm and then the pellet was rinsed three times in distilled water containing 0.02% Tween-20, sonicated to separate spores, and the spore concentration was finally adjusted to 5 × 105 spores per mL. Tomato seedlings were inoculated by immersing in the spore suspension solution with gentle shaking for 1–2 h in both experiments (soil and aeroponic culture). To prepare the fungal mycelium, the active disks were placed in a liquid medium and then incubated in a shaker incubator at 28 °C and 150 rpm for 7–10 days. Next, mycelium was filtered and washed several times with distilled water to remove the medium.
2.2. Experiments
2.2.1. Lab germination
The tomato seeds were treated with 5.0% sodium hypochlorite for 5 min and then with 70.0% ethanol for 30 s for surface sterilization. Then, they were washed three times with distilled water. In total, 30 seeds of tomato were used for each Ni nitrate [Ni(NO3)2] stress treatment (0, 300, 600, and 900 mg L−1) and were placed in Petri dishes containing two layers of Whatman No 2.0 filter paper moistened with 10 mL of solutions or distilled water as the control. The stock solutions of Ni nitrate were further prepared in distilled water at the desired concentrations and diluted with distilled water to obtain suitable concentrations. Double-distilled water was applied as the control treatment. For inoculation, 2 g of mycelium were thoroughly mixed with 100 mL of extracted spore extract, and 2 mL were added to the Petri dishes, except for the control treatments without P. indica. The Petri dishes were then located in a growth cabinet with a temperature of 25°C and a 16/8-h light/dark cycle. The measured traits also included germination rate (GR), shoot fresh weight (SFW), fresh root weight (RFW), shoot dry weight (SDW), root dry weight (RDW), shoot length (SL), root length (RL), proline (Pro), flavonoid content (Flav), phenol content (Phen), catalase (CAT), and glutathione peroxidase (GPx).
2.2.2. Greenhouse trial
Similar to the aeroponic system, the inoculated seeds with the fungus P. indica were transferred into the pots with PittMoss (Gramoflor GmbH & Co. KG, Germany) and soil after 14 days in the greenhouse. This soil mixture was also autoclaved two times with a one-day interval to sterilize. The nutrition was further performed with the 2 g L−1 Hoagland nutrient solution, and the Ni nitrate stress treatments (0, 300, 600, and 900 mg L−1) were applied after 3 weeks. The plants were grown in a growth chamber with 70% humidity, a temperature of 25°C, and a 16/8-h light/dark cycle under two different inoculating systems, including uninoculated and inoculated P. indica. Each treatment combination also consisted of four replication plants grown in separate pots. The seedlings were also harvested after 70 days, and the traits measured were RL, root volume (RV), stem length (SL), RDW, stem dry weight (SDW), dry leaf weight (LDW), and leaf number (LN). Besides, some biochemical traits were measured on the leaf samples, such as chlorophyll a (Chl a, b), carotenoid content (Carrot), Phen, protein content (Prot), anthocyanin (Anth), Pro, Flav, CAT, GPx, hydrogen peroxide (H2O2), and malondialdehyde (MDA).
2.2.3. Aeroponic system
The aeroponic system was established in a growth chamber (each unit with 100 × 100 × 120 cm; depth × width × length) by controlling some environmental factors, such as temperature and photoperiod, which had a mobile front panel for managing and harvesting. The tomato roots were also sprayed with the Hoagland solution every 20 min for 20 s, the nutrient solution was renewed weekly, and the plants were staked out with a wire mesh if necessary. The P. indica-inoculated tomato seeds were further transferred into the aeroponic system after 14 days. The tomato seedlings were also maintained under environmental conditions with a 16/8 h photoperiod (day/night), 70% humidity, 350–400 μmol m−2 s−1 light intensity, and 25°C temperature. After 3 weeks, the P. indica infestation was verified, and the heavy-metal stress treatments (i.e., Ni nitrate) were applied in concentrations of 0, 300, 600, and 900 mg L−1. The seedlings were finally harvested after 40 days, and similar traits as in the greenhouse experiment were measured.
2.3. Protocols
All traits were measured in accordance with standard protocols. The seed germination properties were also recorded based on the International Rules for ISTA (1996). RL and SL were accordingly measured via a lab ruler, and RV was assessed with an overflow spout. The roots, stems, and leaves were also dried for 24 h separately, and then, RDW, SDW, and LDW were measured by a digital scale. In addition, LN per plant was counted in each experimental unit. The MDA content was further evaluated from the root tissue (Heath and Packer, 1968), and the Pro content was determined by Bates et al. (1973). Anth was additionally measured based on Gitelson et al. (2001), while the Flav estimation was done by the complementary colorimetric procedure introduced by Chang et al. (2002). The antioxidant enzymes were also assayed for CAT and GPx as well as for H2O2 with reference to the study of Mondal et al. (2004). Phen was further estimated based on the method developed by Zhu and Yao (2004), and total Prot was obtained according to the Bradford method (Kruger, 2009). The magnitudes of Chl a, b and Carrot were ultimately determined in line with Zhongfu et al. (2009).
2.4. Statistical analysis
The recorded datasets of three experiments were tested by the Anderson-Darling test for normality using the MINITAB (2017) (ver. 18.1) software package. The data from each experiment were also subjected to the analysis of variance (ANOVA) using Statistica version 10.0 (StatSoft, 2011). The SPSS version 23.0 (IBM, 2015) was also exercised to calculate the magnitude of the standard errors and the least significant difference (LSD) values (p < 0.05) if significant differences occurred in the treatment mean scores.
3. Results
3.1. Germination
The ANOVA results showed a significant difference (p < 0.01) for the main effects of P. indica as well as for various concentrations of Ni in all measured traits (results are not shown). Moreover, the interaction effects of P. indica on Ni were significant (p < 0.01) for all measured traits except for SL, Phen, Pro, CAT, and GPx. The treatment with (results are not shown) 0.0 mg L−1 Ni nitrate and the non-treatment with P. indica were also assumed to be controls. Treating the tomato seeds with P. indica accordingly led to an increase of 32, 30, 69, 12, and 76% in RL, RFW, SFW, RDW, and SDW, respectively, over the non-treated control plants under non-Ni stress, while the amount of Flav decreased (21%) upon applying P. indica (Table 1). The treatment with P. indica also made Ni's harmful impacts ineffective in the other measured traits, including SL, SFW, GR, Pro, Phen, Flav, and GPx (Table 1). Thus, P. indica could stop the effects of 300 mg L−1 of Ni-induced stress on germination or even improve some traits (Figure 1).
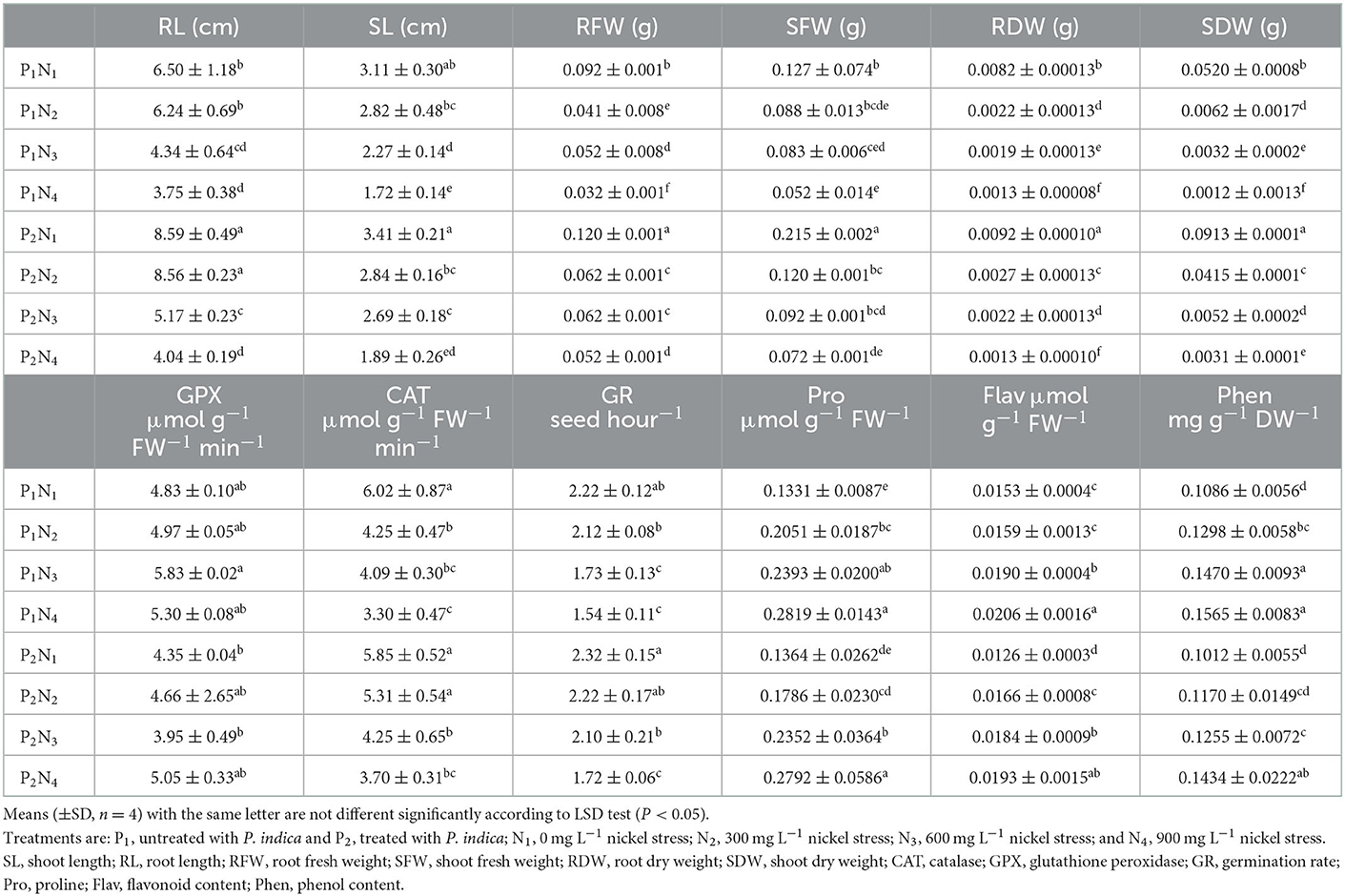
Table 1. The effect of P. indica inoculation on the measured traits of tomato subjected to nickel nitrate stress under lab germination experiment.
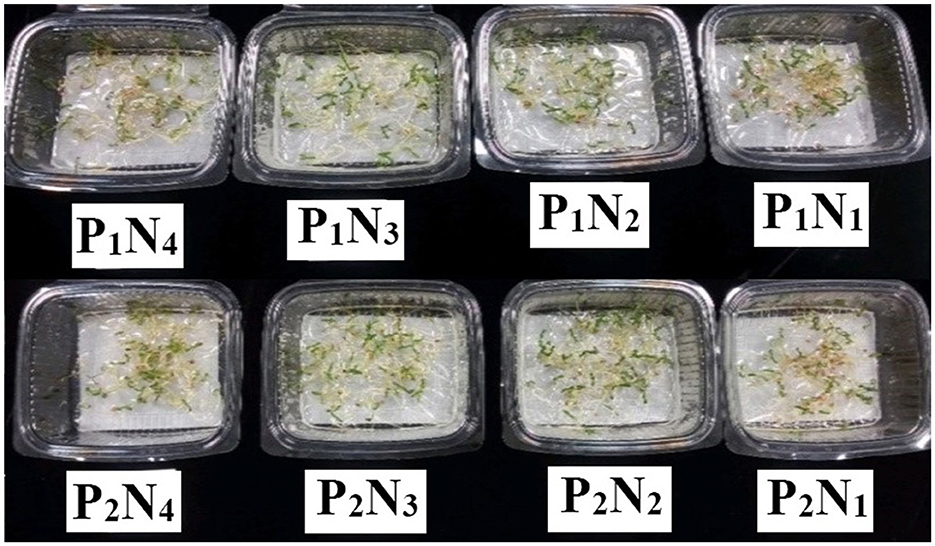
Figure 1. The germination experiment: the effect of P. indica treatment on the tomato germination under nickel stress, N1, N2, N3, and N4 (0, 300, 600, and 900 mg L−1). P1; untreated with P. indica and P2; treated with P. indica.
Under 600 mg L−1 of Ni, several germination traits such as SL, RFW, EDW, SDW, and GR were elevated due to the application of P. indica (Table 1). When subjected to severe stress from Ni (900 mg L−1), RFW and SDW also elevated in the P. indica-treated group, while such a treatment deteriorated the adverse effects of Ni-induced stress on the other recorded traits at the germination stage (Table 1). Using P. indica enhanced tomato seed germination under non-stress conditions. It thwarted its harmful effects under different levels of Ni stress on various seed germination properties.
3.2. Greenhouse seedling
The ANOVA results indicated significant differences (p < 0.01) for the main effects of P. indica and Ni on all measured traits, whereas their interaction effects were only significant (p < 0.01) for SL, RV, RDW, Chl b, and GPx. The treatment with 0.0 mg L−1 Ni nitrate and the non-treatment with P. indica were also assumed as the controls. In the greenhouse experiment, the P. indica-treated plants showed a significant increase in RL (20%), RV (56%), RDW (46%), SDW (33%), LDW (30%), LN (22%), CAT (21%), GPx (29%), Prot (15%), and Anth (4%) under non-stress conditions, while the magnitudes of Phen, Pro, and Chl a & b did not alter following the P. indica application (Table 2). Under 300 mg L−1 Ni stress, the magnitudes of RV (35%), SDW (21%), LDW (29%), Prot (69%), Anth (15%), Carrot (108%), and Chl b (105%) also enlarged due to the P. indica use (Table 2). Moreover, applying P. indica caused the negative impacts of Ni to be ineffective in RL, RDW, LN, CAT, GPx, and Chl a (Table 2). Under 600 mg L−1 of Ni stress, the quantities of RL (36%), RV (20%), RDW (44%), SDW (32%), LDW (33%), Prot (91%), Anth (5%), and Carrot (39%) correspondingly enhanced due to the P. indica application (Figure 2).
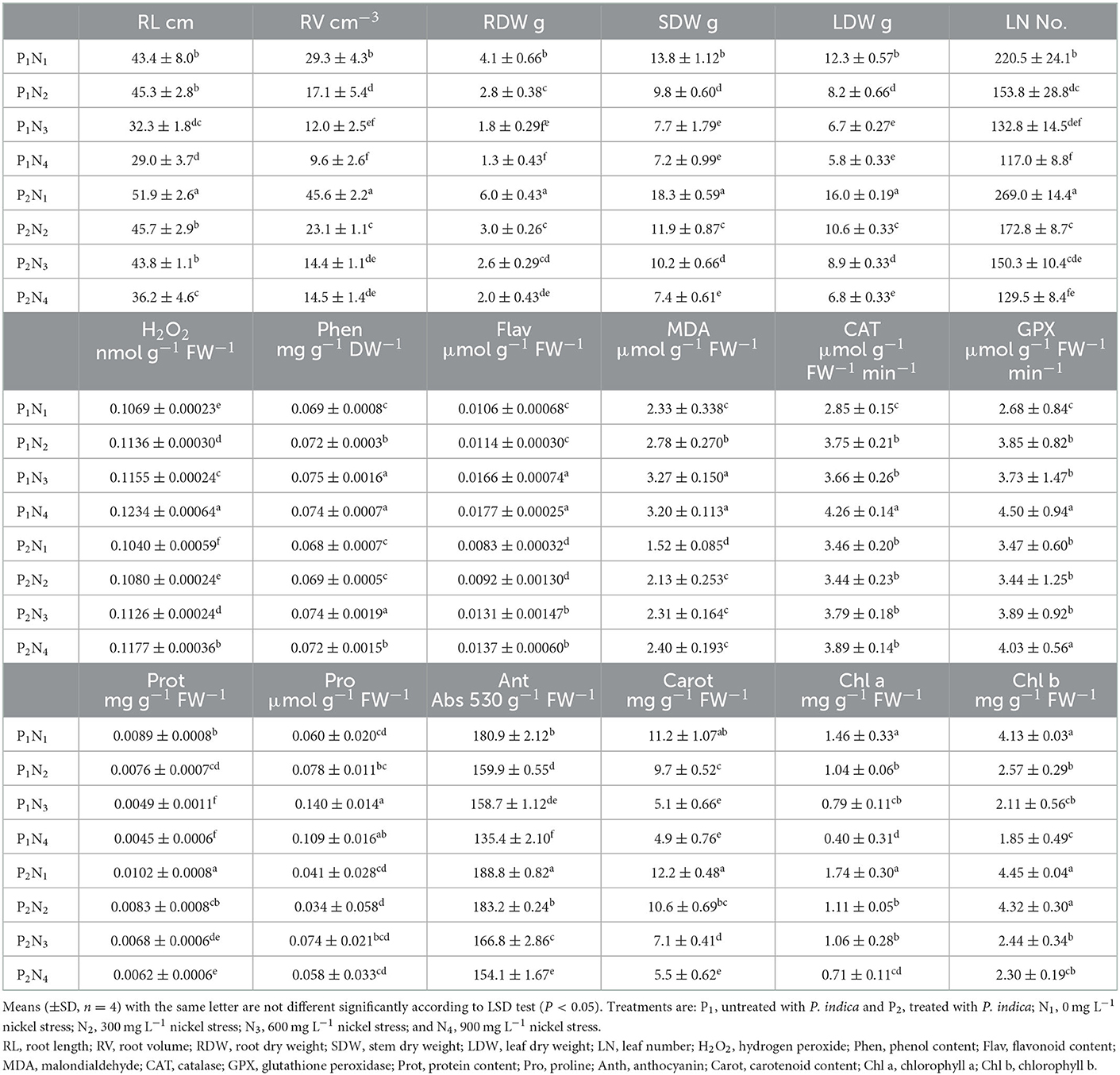
Table 2. The effect of P. indica inoculation on the measured traits of tomato subjected to nickel nitrate stress under greenhouse experiment.
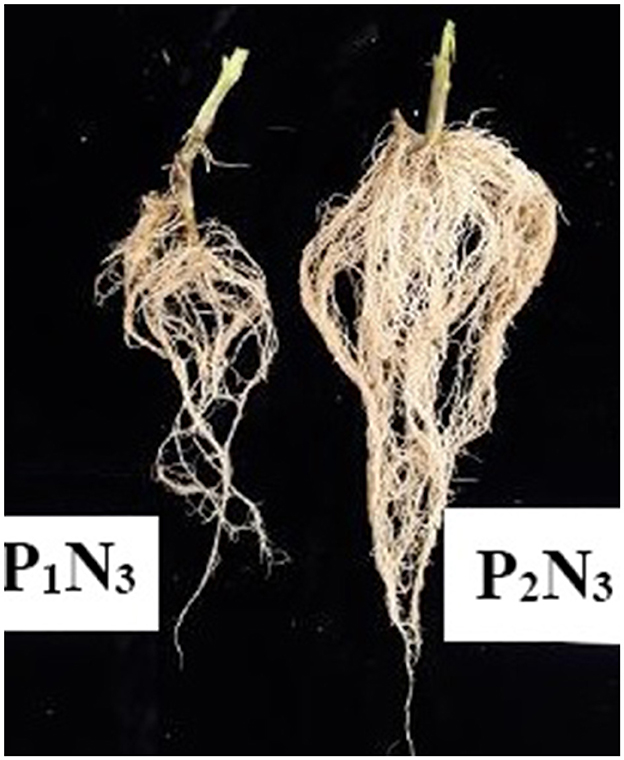
Figure 2. The greenhouse experiment: the effect of P. indica treatment on the tomato root under nickel stress, N3 (600 mg L−1). P1; untreated with P. indica and P2; treated with P. indica.
Under severe stress from Ni (900 mg L−1), the positive effects of P. indica were restricted to RL (25%), RV (51%), RDW (54%), and Anth (14%), while the use of P. indica frustrated the harmful impacts of Ni-induced stress on SDW, LDW, LN, GPx, Carrot, and Chl a, b (Table 2). In contrast, using P. indica could not eliminate the harmful effects of Ni stress at high concentrations (900 mg L−1) in H2O2, Phen, Flav, MDA, CAT, Prot, and Pro (Table 2). The activity of the GPx antioxidant enzyme did not also decrease, especially under severe Ni stress, denoting that certain rootstocks could mitigate Ni toxicity via detoxification in cells as a process toward the detoxification of H2O2 and lipid peroxides by reduced glutathione.
3.3. Aeroponic system
The ANOVA results demonstrated significant differences (p < 0.01) for the main effects of P. indica and Ni in all measured traits. Besides, the interaction effects of P. indica by Ni were significant (p < 0.01) for all measured traits except for Anth. The treatment with 0.0 mg L−1 Ni nitrate and the non-treatment with P. indica were also assumed to be the controls. In the aeroponic experiment, the P. indica-treated plants accordingly indicated a significant upsurge in RL (17%), RV (12%), RDW (49%), SDW (35%), LDW (28%), LN (37%), CAT (22%), GPx (25%), Prot (25%), and Anth (10%) under non-stress conditions, while the magnitudes of MDA, Carrot, and Chl a & b did not change after treatment with P. indica (Table 3). Under 300 mg L−1 Ni-induced stress, the amounts of RL (19%), RV (7%), RDW (72%), SDW (25%), LDW (14%), LN (70%), Prot (27%), and Anth (13%) also elevated due to the P. indica application (Table 3).
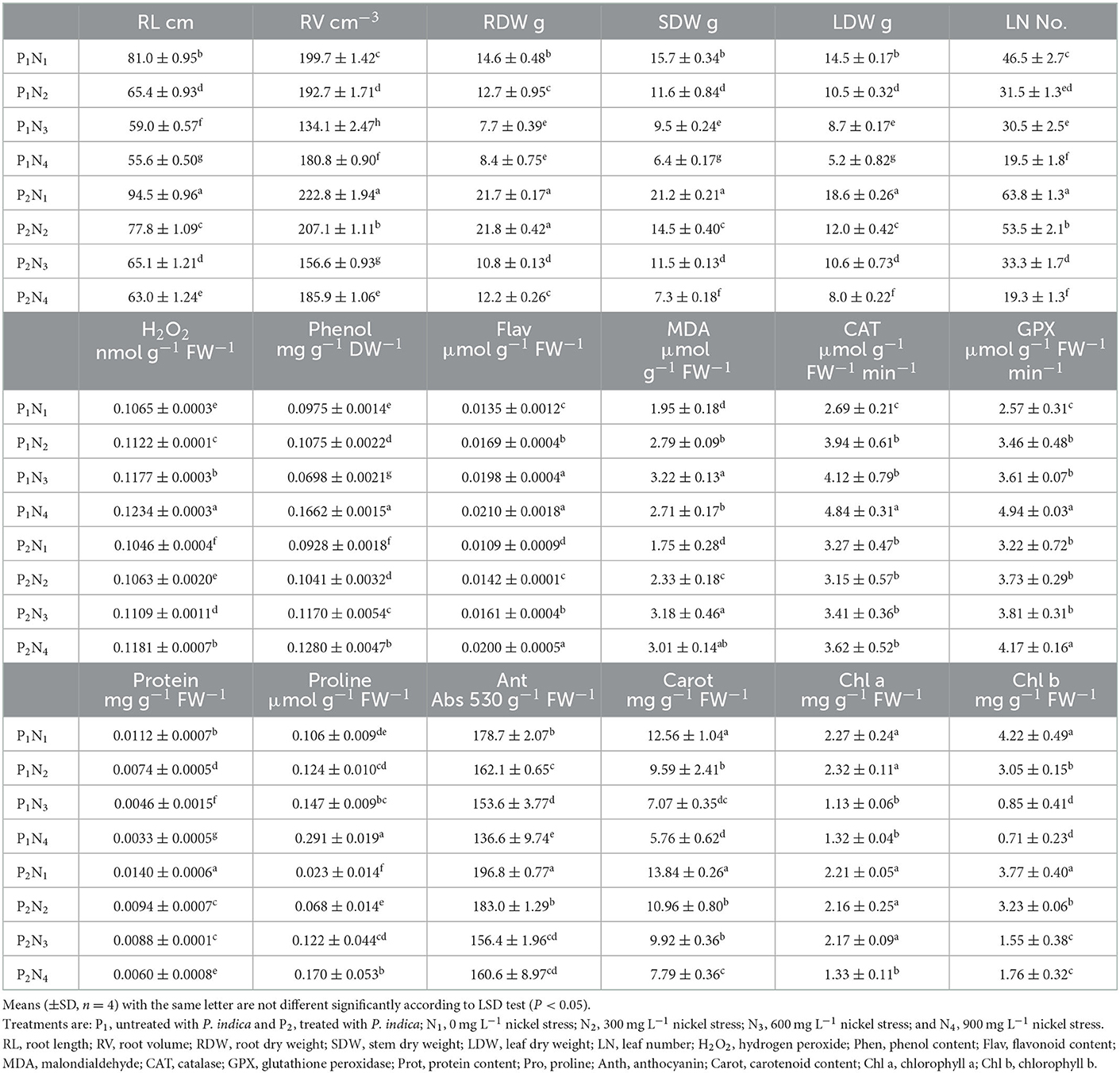
Table 3. The effect of P. indica inoculation on the measured traits of tomato subjected to nickel nitrate stress under aeroponic experiment.
Under the 600 mg L−1 of Ni stress, RL (10%), RV (17%), RDW (40%), SDW (21%), LFW (22%), LN (9%), Phen (68%), Prot (91%), Carrot (40%), Chl a (92%), and Chl b (82%) also augmented as the result of using P. indica (Table 3). Under severe stress from Ni (900 mg L−1), the positive effects of P. indica were also limited to RL (13%), RV (3%), RDW (45%), SDW (14%), LDW (54%), Prot (82%), and Chl b (148%), while the application of P. indica blocked the adverse impacts of Ni stress on LN, Flav, MDA, GPx, and Chl a (Figure 3).
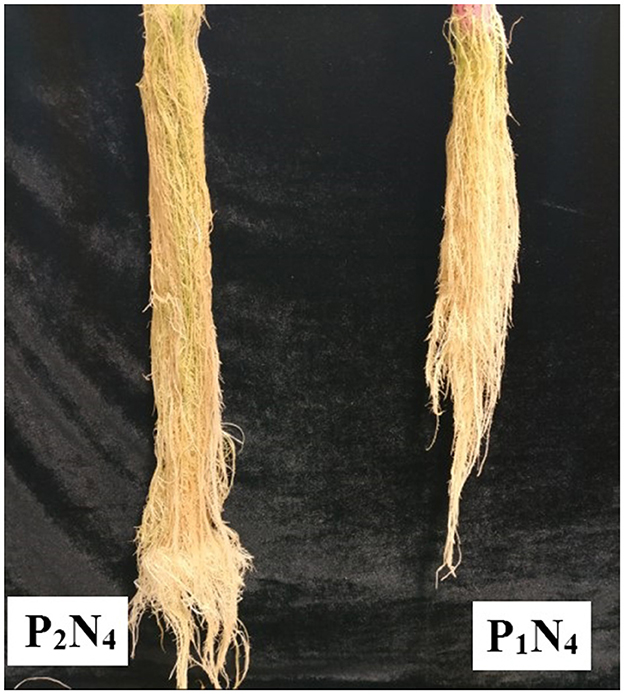
Figure 3. The aeroponic experiment: the effect of P. indica treatment on the tomato root under nickel stress, N4 (900 mg L−1). P1; untreated with P. indica and P2; treated with P. indica.
3.4. Trait associations
The results of the principal component analysis (PCA) based on the correlation matrices indicated that the two first PCs described 91, 92, and 90% of the variance in the lab germination, greenhouse, and aeroponic experiments, respectively. In the plot of the germination (Figure 4), the PC1 axis mainly distinguished the traits of Pro, Flav, Phen, and GPx from the others. Thus, the first PC could separate the traits into two main groups according to the Ni-induced stress conditions. Figure 4 shows the traits corresponding to Ni stress on the left and those based on normal or non-stress conditions, except for CAT, on the right. It further facilitates the visualization of the associations among the traits by approximating the correlation coefficient through the cosine of the angle between the vectors. Therefore, some of the most prominent associations, according to Figure 4, were as follows: (i) a strong positive association between RL and SL and between Pro and Flav, as illustrated by the small acute angles between their vectors (r = cos 0 = +1), (ii) a near-zero correlation between GPx and Pro and Flav, as indicated by the near perpendicular vectors (r = cos 90 = 0), and (iii) a strong negative association between Phen and RL and SL, and between CAT and SFW and Pro and Flav, as indicated by the large obtuse angles (r = cos 180 = −1).
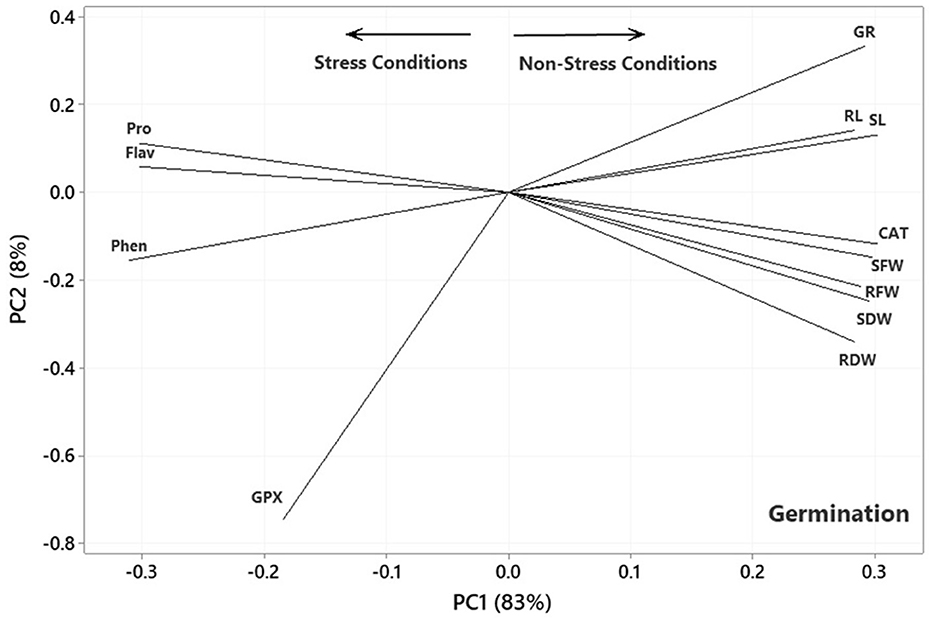
Figure 4. Plot showing the association among the measured traits subjected to four treatments (0, 300, 600, and 900 mg L−1) in the germination experiment based on the two first PCs. SL, shoot length; RL, root length; RFW, root fresh weight; SFW, shoot fresh weight; RDW, root dry weight; SDW, shoot dry weight; CAT, catalase; GPX, glutathione peroxidase; GR, germination rate; Pro, proline; Flav, flavonoid content; Phen, phenol content.
In the plot of the greenhouse experiment (Figure 5), the PC1 axis could further split the traits of Pro, MDA, Flav, Phen, H2O2, CAT, and GPx from the others. Thus, the PC1 could separate the traits based on Ni-induced stress, like that in the germination, even better due to the standing of CAT near other traits related to the stress conditions. Moreover, some of the most prominent relationships based on Figure 5 were (i) a strong positive association between CAT and GPx and between Phen and Flav, (ii) a near-zero correlation between GPx and CAT and Pro and MDA, and (iii) a strong negative association between RL and Phen and Flav, between H2O2 and Anth and Chl a & b. Most of the mentioned relationship predictions could also be verified from the original data because the PCA described the bulk of the total variances (over 90%). Figure 6 also shows that the PC1 axis sets apart the same traits in both aeroponic and greenhouse experiments, i.e., Pro, MDA, Flav, Phen, H2O2, CAT, and GPx in one group and the other traits in another group. Therefore, the PC1 could discriminate the traits based on the stress conditions in the aeroponic experiment, like those in lab germination and greenhouse ones. Some of the most prominent correlations based on Figure 6 were as follows: (i) a strong positive correlation between LN, Anth, and Carrot and Prot, and between CAT and Pro, (ii) a near-zero correlation between Phen and MDA, and (iii) a strong negative association between RV and MDA, and between LDW and SDW and Flav.
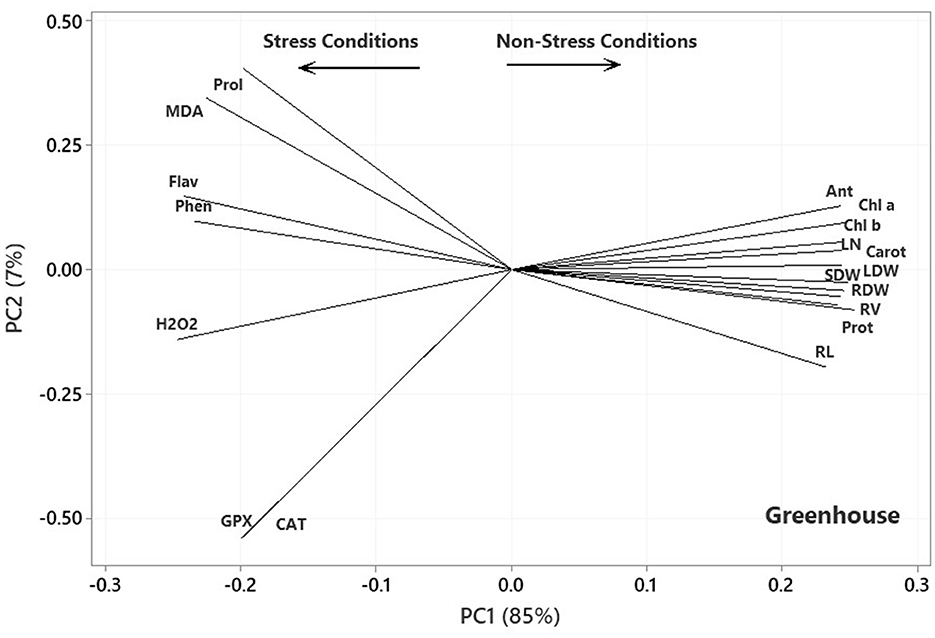
Figure 5. Plot showing the association among the measured traits subjected to four treatments (0, 300, 600, and 900 mg L−1) in the greenhouse experiment based on the two first PCs. RL, root length; RV, root volume; RDW, root dry weight; SDW, stem dry weight; LDW, leaf dry weight; LN, leaf number; H2O2, hydrogen peroxide; Phen, phenol content; Flav, flavonoid content; MDA, malondialdehyde; CAT, catalase; GPX, glutathione peroxidase; Prot, protein content; Pro, proline; Anth, anthocyanin; Carot, carotenoid content; Chl a, chlorophyll a; Chl b, chlorophyll b.
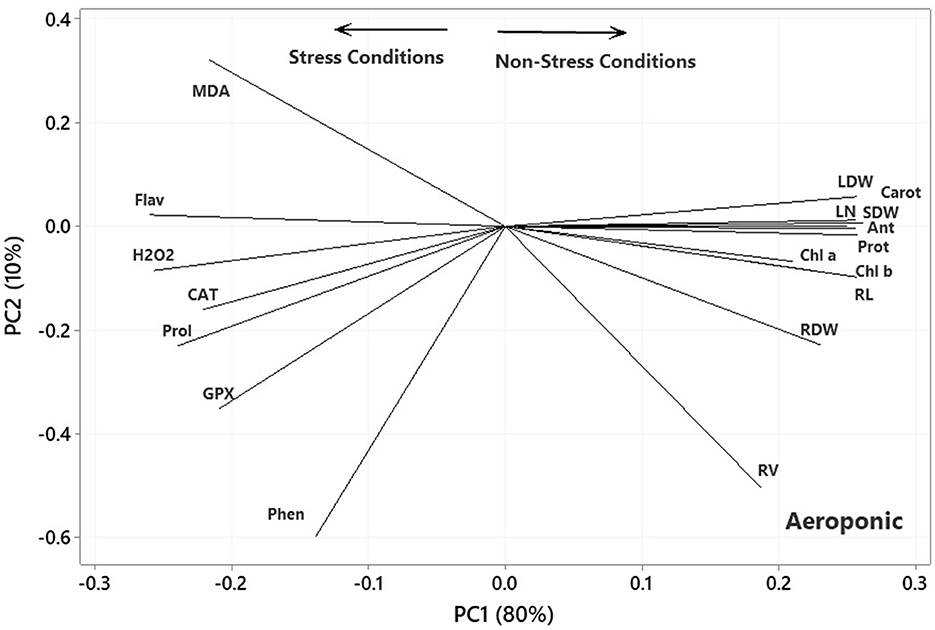
Figure 6. Plot showing the association among the measured traits subjected to four treatments (0, 300, 600, and 900 mg L−1) in the aeroponic experiment based on the two first PCs. RL, root length; RV, root volume; RDW, root dry weight; SDW, stem dry weight; LDW, leaf dry weight; LN, leaf number; H2O2, hydrogen peroxide; Phen, phenol content; Flav, flavonoid content; MDA, malondialdehyde; CAT, catalase; GPX, glutathione peroxidase; Prot, protein content; Pro, proline; Anth, anthocyanin; Carot, carotenoid content; Chl a, chlorophyll a; Chl b, chlorophyll b.
4. Discussion
The study results established that increasing Ni-induced stress from 0 to 900 mg L−1 reduced most traits in tomato plants due to the phytotoxicity of Ni stress. Similar results have been further reported in this respect (Nazir et al., 2019; Jahan et al., 2020). Notably, the Ni requirement in plants is very low, and its toxicity happens at 10 mg g−1 for the sensitive species, but the tolerant ones can bear 50 mg g−1 concentrations (Kumar et al., 2021). Hassan et al. (2019) had accordingly shown that the negative effects of Ni on plants could be due to the reduced uptake and the translocation of essential nutrients to their shoots induced by Ni phytotoxicity. The effects of Ni on tomato growth could be further related to the enhancement of lipid peroxidation because heavy metal ions can initiate lipid peroxidation in plants (Giannakoula et al., 2021). As the MDA production, the magnitude of lipid peroxidation was also elevated in the higher levels of Ni stress compared to the lower levels or under non-stress conditions.
In the present study, the significant, positive effect of the P. indica application on decelerating depression in most of the measured traits was observed in the lab, greenhouse, and aeroponic experiments, whereas RL was enhanced by 32, 20, and 17% following the P. indica use in comparison with non-Ni stress in the germination, greenhouse, and aeroponic experiments, respectively. In other words, P. indica application could decrease the rate of trait depression under Ni stress (Figure 7). Similarly, Bagde et al. (2011) reported that treating sunflowers with P. indica increased RL by 36% and RDW by 24%. Usman et al. (2019) further stated that the root and shoot characteristics had been harmfully affected by Ni stress because of Ni toxicity disorders in the metabolic system and had thus prevented the normal cell cycle process.
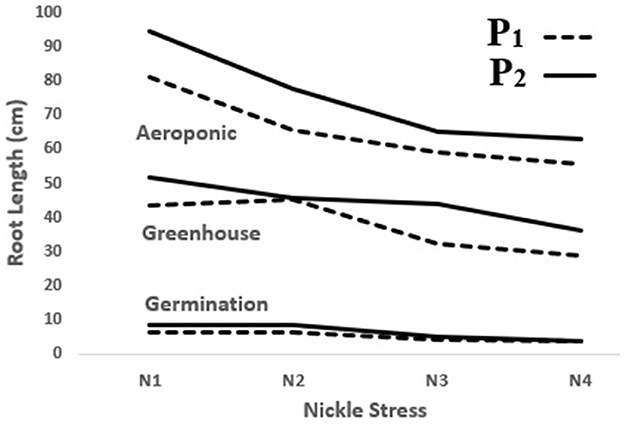
Figure 7. The effect of P. indica treatment on root length in the tomato subjected to nickel exposure (0, 300, 600, and 900 mg L−1). P1; untreated with P. indica and P2; treated with P. indica.
This study revealed that the tomato plants could withstand up to 300 or even 600 mg L−1 of Ni stress after the P. indica treatment and exhibited higher growth traits than the non-treated ones, such as RL and SL. In other words, applying P. indica could maintain the tomato plant's tolerance to Ni-induced stress in morphological traits and remove the harmful effects of heavy metal contamination. According to Sepehri and Khatabi (2021), the decrease in SDW and RDW stopped cadmium concentrations in the P. indica-inoculated alfalfa plants. Similarly, according to Hassan et al. (2019), Ni reduced SL and RL and even caused a remarkable decline in other morphological traits. Rajput et al. (2022) further reported that the seed germination of plants had been diminished by Ni stress, while GR had not been reduced under different levels of Ni stress due to the application of P. indica, but it increased from 5 to 21% in the present study. The P. indica-treatment further boosted the growth of the tomato plants under Ni stress, indicating the effective role of P. indica in protecting the plants against the effects of Ni. According to Adya et al. (2013), P. indica had elevated nutrient uptake, allowing the plants to survive under abiotic stress and admit tolerance to heavy metal toxicity, along with other useful effects, such as growth improvement and favorable yield performance. Similar investigations have also reported the positive role of P. indica in inducing the tolerance of plants to copper (Sabra et al., 2018) and arsenic (Ghorbani et al., 2021) toxicity. Besides, Ghorbani et al. (2021) found that P. indica had enhanced plant growth with the inhibition of huge damage occurring to photosynthetic organs because arsenic could be immobilized in the roots and had not transferred to the plant shoots.
Treating P. indica correspondingly thwarted the harmful effects of Ni on H2O2 and decreased the adverse effects of Ni. The H2O2 magnitude also increased in the leaf tissue of Solanum Lycopersicum L. under Ni stress (Nazir et al., 2019), and it was expected that compounding the Ni concentrations could significantly elevate the quantities of H2O2, but treating the tomato plants with P. indica prevented its production in cells. The H2O2 production under heavy metal stress could also do considerable damage to cells and even lead to death (Das and Roychoudhury, 2014), while the antioxidant enzymes, like CAT and GPx, could scavenge H2O2, cause cell death, and provide defense against disbalances in the redox position of the stressed plants (García-Caparrós et al., 2021).
Furthermore, the study findings revealed that CAT and GPx significantly restricted H2O2 production under Ni-induced stress, but positive associations existed. The application of P. indica on tomato plants seemed to stimulate their defense mechanisms, like the high production of antioxidant enzymes. Reduction in CAT activity under Ni stress was also expected because the Ni excess could reduce some micronutrients, like iron or zinc, contributing to the structure of metalloenzymes, such as CAT (Altaf et al., 2021). The decrease in CAT activity recorded in this study was thus in agreement with the reports by Nazir et al. (2019) on tomatoes and Ghorbani et al. (2021) on rice.
Moreover, Chl a, b, as well as Carrot in the Ni-stressed plants treated with P. indica, were analyzed, suggesting that the amount of these pigments was significantly higher in the treated plants as compared with the non-treated ones grown under the same conditions. It seemed that P. indica could maintain the process of photosynthesis under Ni stress by inducing the defense system of the antioxidant enzymes, which could subside the Ni-induced oxidative stress. Such similar results have already been reported for tomatoes (Solanum Lycopersicum L.) (Ghorbani et al., 2018) and Dimocarpus longan seedlings (Cheng et al., 2022). There are also some antioxidant enzymes in plants, and it is expected that the P. indica inoculation can stimulate some of them, though the CAT activity decreased. However, only the enzyme activity was considered here, so the amount of enzyme might have amplified in the periods after inoculation through the rise in the expression of the related genes.
Given that antioxidant enzymes alone may not be very effective in cleaning ROS, the possibility of augmenting other enzymes that could scavenge ROS besides CAT is very high. Antioxidant enzymes and other non-enzymatic antioxidants also work together to reduce oxidative stress. Therefore, downgrading or escalating the activity of an antioxidant enzyme cannot be the reason for the success or failure of a plant defense process under oxidative stress. Therefore, P. indica aided the tomato plants by protecting them against Ni stress, but further investigations are required to grasp the mechanism of how P. indica is useful for plants. Although most studies have not simulated field conditions, the report by Khalid et al. (2019) supported the idea of using P. indica under field conditions for inducing tolerance to abiotic stress. Comparatively, the higher growth characteristics in the P. indica-treated plants could be attributed to their capacity to maintain higher Chl contents because the treated plants could delay photoinhibition under stress conditions and prevent the Ni interference in the photosynthetic electron transport chain and the light-harvesting complex II (Dubey, 2018). Moreover, the lower Chl a, b ratio recorded in the greenhouse and aeroponic experiments here under 300 mg L−1 of Ni stress and the greenhouse experiment under 900 mg L−1 of Ni stress demonstrated less damage to Chl b, indicating the better protection of the photosystem II (PSII) under the Ni-induced stress conditions (Janečková et al., 2019; Muhammed et al., 2022).
One other way for plants to protect themselves against Ni stress is the synthesis of osmolytes, such as Pro, which stabilizes proteins and membranes (Khan et al., 2020). In this study, the Pro quantities increased as Ni stress rose from 0 to 900 mg L−1, consistent with previous reports (Nazir et al., 2019). The increase in Pro may be further associated with reduced oxidative stress, resulting in the prevention of protein hydrolysis. The P. indica application augments this natural response under different Ni stress conditions. The changes in the membrane lipid composition were also found in this investigation since MDA augmented when the tomato plants were exposed to different Ni concentrations, as reported earlier on pigeon peas and cucumbers (Khoshgoftarmanesh et al., 2014). The lowest magnitudes of the MDA accumulation recorded in P. indica-treated plants also verified that this fungus could significantly contribute to mitigating the oxidative damage caused by Ni by enhancing the antioxidant capacity and reducing Pro and MDA in leaf cells.
The PCA of all experiments also indicated that the PC1 axis divided the traits into main groups, i.e., Pro, MDA, Flav, Phen, H2O2, CAT, and GPx in one group as an indicator of the Ni stress condition and the other traits in another group as a sign of normal or non-stress conditions. These results were in good agreement with the reports of Kirova et al. (2021), investigating wheat genotypes with similar templates of drought stress response associated with Pro, H2O2, Phen, Flav, CAT, and GPx. Although some traits in each group indicated weak or near-zero correlations, all had the same characteristics, such as common behavior under stress or non-stress conditions. Similarly, Plazas et al. (2019) reported that Pro, MDA, Flav, CAT, and GPx were involved in the stress response. The higher magnitudes of CA and GPx had also been recorded in the leaves of tolerant cultivars under drought stress, and these H2O2-scavenging enzymes were important in stress conditions (Kirova et al., 2021). The study results provide relevant information about the responses of the tomato plants to Ni stress, as well as the significant, positive effects of P. indica treatment on the removal of the harmful impacts of stress under different growing systems. It was thus suggested to evaluate commercial tomato cultivars inoculated with P. indica under Ni or other heavy metal stress conditions in farming fields to demonstrate the good effects of P. indica on meeting the goals of sustainable agriculture.
5. Conclusion
This study revealed that P. indica treatment significantly and positively enhanced most measured traits, especially photosynthetic factors, and improved the growth performance of tomato plants under Ni toxicity stress. The application of P. indica also decreased the content of MDA and H2O2 and, as a result, increased the tolerance of the tomato plants under Ni exposure. The PCA also separated Pro, MDA, Flav, Phen, H2O2, CAT, and GPx as the stress-related traits from the others under Ni-induced stress and normal conditions (Figure 8). Considering the positive effects of P. indica on tomato tolerance to Ni toxicity, its application can thus be recommended as an eco-friendly tool and a plant biostimulant in Ni-contaminated regions.
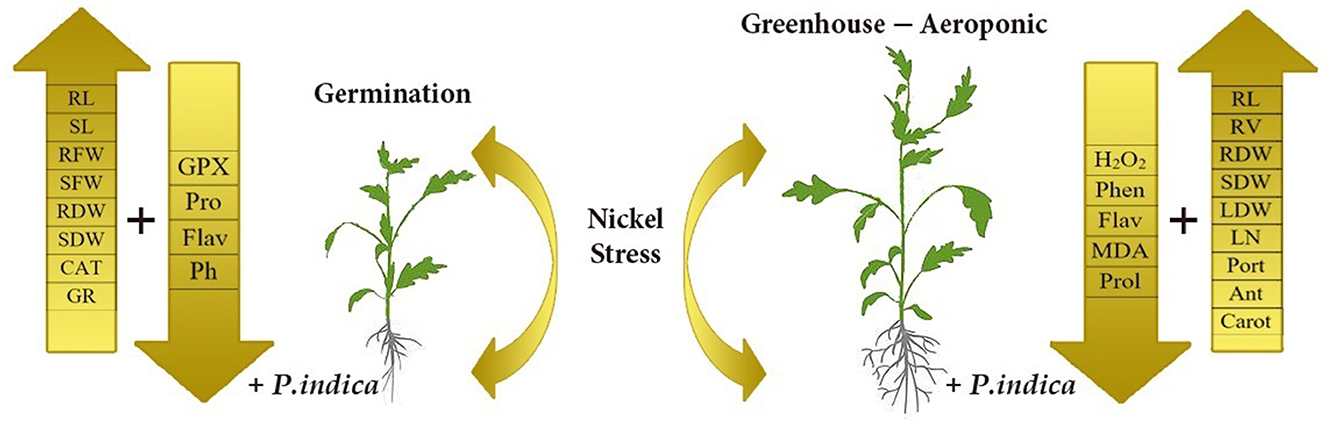
Figure 8. Schematic representation depicts morphological and physiological differences between P. indica colonized and uncolonized tomato plants. The overall health and productivity are enhanced P. indica infested tomato plants as compared to the non-infested ones under nickel stress.
Data availability statement
The datasets presented in this article are not readily available because there is no restrictions. Requests to access the datasets should be directed to emFocmFfbW92YWhlZGlfMzEyQHlhaG9vLmNvbQ==.
Author contributions
ZM and MG: conceptualization, methodology, and analysis. NM, ZM, and MG: validation and investigation. ZM: writing the original draft, reviewing, and editing the manuscript. All authors have read and agreed to the published version of the manuscript.
Conflict of interest
The authors declare that the research was conducted in the absence of any commercial or financial relationships that could be construed as a potential conflict of interest.
Publisher's note
All claims expressed in this article are solely those of the authors and do not necessarily represent those of their affiliated organizations, or those of the publisher, the editors and the reviewers. Any product that may be evaluated in this article, or claim that may be made by its manufacturer, is not guaranteed or endorsed by the publisher.
References
Adya, A. K., Gautam, A., Zhang, L., and Varma, A. (2013). “Characterization of Piriformospora indica culture filtrate,” in Sebacinales-Forms, Functions, and Biotechnological Applications, Soil Biology Series No. 33, eds A. Varma, G. Kost, and R. Oelmuller (Berlin: Springer-Verlag), 345–375.
Altaf, M. A., Shahid, R., Ren, M. X., Altaf, M. M., Jahan, M. S., and Khan, L. U. (2021). Melatonin mitigates nickel toxicity by improving nutrient uptake fluxes, root architecture system, photosynthesis, and antioxidant potential in tomato seedlings. J. Plant Nutr. Soil Sci. 21, 1842–1855. doi: 10.1007/s42729-021-00484-2
Anith, K. N., Sreekumar, A., and Sreekumar, J. (2015). The growth of tomato seedlings inoculated with co-cultivated Piriformospora indica and Bacillus pumilus. Symbiosis 65, 9–16. doi: 10.1007/s13199-015-0313-7
Aslam, M. M., Karanja, J., and Bello, S. K. (2019). Piriformospora indica colonization reprograms plants to improved P-uptake, enhanced crop performance, and biotic/abiotic stress tolerance. Physiol. Mol. Plant Pathol. 106, 232–237. doi: 10.1016/j.pmpp.2019.02.010
Athira, S., and Anith, K. N. (2020). Plant growth promotion and suppression of bacterial wilt incidence in tomato by rhizobacteria, bacterial endophytes and the root endophytic fungus Piriformospora indica. Indian Phytopath 73, 629–642. doi: 10.1007/s42360-020-00283-2
Bagde, U. S., Prasad, R., and Varma, A. (2011). Influence of culture filtrate of Piriformospora indica on growth and yield of seed oil in Helianthus annus. Symbiosis 53, 83–88. doi: 10.1007/s13199-011-0114-6
Baghaie, A. H., and Aghili, F. (2021). Contribution of Piriformospora indica on improving the nutritional quality of greenhouse tomato and its resistance against cu toxicity after humic acid addition to soil. Environ. Sci. Pollut. Res. 28, 64572–64585. doi: 10.1007/s11356-021-15599-3
Barbouti, A., Lagopati, N., Veroutis, D., Goulas, V., Evangelou, K., Kanavaros, P., et al. (2021). Implication of dietary iron-chelating bioactive compounds in molecular mechanisms of oxidative stress-induced cell ageing. Antioxidants 10, 491. doi: 10.3390/antiox10030491
Bates, L. S., Waldren, R. P., and Teare, I. D. (1973). Rapid determination of free proline for water-stress studies. Plant Soil 39, 205–207. doi: 10.1007/BF00018060
Berni, R., Guerriero, G., and Cai, G. (2019). “One for all and all for one! Increased plant heavy metal tolerance by growth-promoting microbes: a metabolomics standpoint,” in Plant Metallomics and Functional Omics (Cham: Springer), 39–54.
Chang, C. C., Yang, M. H., Wen, H. M., and Chern, J. C. (2002). Estimation of total flavonoid content in propolis by two complementary colorimetric methods. J. Food Drug Anal. 10, 2748. doi: 10.38212/2224-6614.2748
Cheng, C., Li, D., Wang, B., Liao, B., Qu, P., Liu, W., et al. (2022). Piriformospora indica colonization promotes the root growth of Dimocarpus longan seedlings. Sci. Hort 301, 111–137. doi: 10.1016/j.scienta.2022.111137
Correia, L., Marrocos, P., Montalván-Olivares, D. M., Velasco, F. G., Luzardo, F. H. M., and Mota de Jesus, R. (2018). Bioaccumulation of nickel in tomato plants: risks to human health and agro-environmental impacts. Environ. Monit. Assess. 190, 1–12. doi: 10.1007/s10661-018-6658-7
Das, K., and Roychoudhury, A. (2014). Reactive oxygen species (ROS) and response of antioxidants as ROS-scavengers during environmental stress in plants. Front. Environ. Sci 2, 1–13 doi: 10.3389/fenvs.2014.00053
Dubey, R. S. (2018). “Photosynthesis in plants under stressful conditions,” in Handbook of Photosynthesis (Boca Raton, FL: CRC Press), 629–649.
Ebrahimi, R., Hayati, B., Shahmoradi, B., Rezaee, R., Safari, M., Maleki, A., et al. (2018). Adsorptive removal of nickel and lead ions from aqueous solutions by poly (amidoamine) (PAMAM) dendrimers (G4). Environ. Technol. Innovat. 12, 261–272. doi: 10.1016/j.eti.2018.10.001
García-Caparrós, P., De Filippis, L., Gul, A., Hasanuzzaman, M., Ozturk, M., Altay, V., et al. (2021). Oxidative stress and antioxidant metabolism under adverse environmental conditions: a review. Bot. Rev. 87, 421–466. doi: 10.1007/s12229-020-09231-1
Ghorbani, A., Omran, V. O. G., Razavi, S. M., Pirdashti, H., and Ranjbar, M. (2019). Piriformospora indica confers salinity tolerance on tomato (Lycopersicon esculentum Mill.) through amelioration of nutrient accumulation, K+/Na+ homeostasis and water status. Plant Cell Rep. 38, 1151–1163. doi: 10.1007/s00299-019-02434-w
Ghorbani, A., Razavi, S. M., Ghasemi Omran, V. O., and Pirdashti, H. (2018). Piriformospora indica inoculation alleviates the adverse effect of NaCl stress on growth, gas exchange and chlorophyll fluorescence in tomato (Solanum lycopersicum L.). Plant Bio 20, 729–736. doi: 10.1111/plb.12717
Ghorbani, A., Tafteh, M., Roudbari, N., Pishkar, L., Zhang, W., and Wu, C. (2021). Piriformospora indica augments arsenic tolerance in rice (Oryza sativa) by immobilizing arsenic in roots and improving iron translocation to shoots. Ecotoxicol. Environ. Saf. 209, 111793. doi: 10.1016/j.ecoenv.2020.111793
Giannakoula, A., Therios, I., and Chatzissavvidis, C. (2021). Effect of lead and copper on photosynthetic apparatus in citrus (Citrus aurantium L.) plants. The role of antioxidants in oxidative damage as a response to heavy metal stress. Plants 10, 155. doi: 10.3390/plants10010155
Gitelson, A. A., Merzlyak, M. N., and Chivkunova, O. B. (2001). Optical properties and nondestructive estimation of anthocyanin content in plant leaves. Photochem. Photobiol. 74, 38–45. doi: 10.1562/0031-8655(2001)074<0038:opaneo>2.0.co;2
Hassan, M. U., Chattha, M. U., Khan, I., Chattha, M. B., Aamer, M., Nawaz, M., et al. (2019). Nickel toxicity in plants: reasons, toxic effects, tolerance mechanisms, and remediation possibilities—a review. Environ. Sci. Pollut. Res. 26, 12673–12688. doi: 10.1007/s11356-019-04892-x
Heath, R. L., and Packer, L. (1968). Photoperoxidation in isolated chloroplasts: I. Kinetics and stoichiometry of fatty acid peroxidation. Arch. Biochem. Biophys. 125, 189–198. doi: 10.1016/0003-9861(68)90654-1
ISTA (1996). International Seed Testing Association. International rules for seed testing. Seed Sci. Tech. 21, 46.
Jahan, M. S., Guo, S., Baloch, A. R., Sun, J., Shu, S., Wang, Y., et al. (2020). Melatonin alleviates nickel phytotoxicity by improving photosynthesis, secondary metabolism and oxidative stress tolerance in tomato seedlings. Ecotoxicol. Environ. Saf. 197, 110593. doi: 10.1016/j.ecoenv.2020.110593
Jalali, M., and Karimi-Mojahed, J. (2020). Assessment of the health risks of heavy metals in soils and vegetables from greenhouse production systems in Iran. Int. J. Phytoremediat. 22, 834–848. doi: 10.1080/15226514.2020.1715917
Janečková, H., Husičková, A., Lazár, D., Ferretti, U., Pospíšil, P., and Špundová, M. (2019). Exogenous application of cytokinin during dark senescence eliminates the acceleration of photosystem II impairment caused by chlorophyll b deficiency in barley. Plant Physiol. Biochem. 136, 43–51. doi: 10.1016/j.plaphy.2019.01.005
Khalid, M., Rahman, S. U., and Huang, D. (2019). Molecular mechanism underlying Piriformospora indica-mediated plant improvement/protection for sustainable agriculture. Acta Biochim. Biophys. Sin. 51, 229–242. doi: 10.1093/abbs/gmz004
Khan, M. I. R., Jahan, B., AlAjmi, M. F., Rehman, M. T., and Khan, N. A. (2020). Ethephon mitigates nickel stress by modulating antioxidant system, glyoxalase system and proline metabolism in Indian mustard. Physiol. Mol. Biol. Plants 26, 1201–1213. doi: 10.1007/s12298-020-00806-1
Khoshgoftarmanesh, A. H., Bahmanziari, H., and Sanaeiostovar, A. (2014). Responses of cucumber to deficient and toxic amounts of nickel in nutrient solution containing urea as nitrogen source. Biol. Plant 58, 524–530. doi: 10.1007/s10535-014-0415-8
Kirova, E., Pecheva, D., and Simova-Stoilova, L. (2021). Drought response in winter wheat: protection from oxidative stress and mutagenesis effect. Acta Physiol. Plant. 43, 1–11. doi: 10.1007/s11738-020-03182-1
Kruger, N. J. (2009). “The Bradford method for protein quantitation,” in The Protein Protocols Handbook (Totowa, NJ: Springer), 17–24.
Kumar, A., Jigyasu, D. K., Subrahmanyam, G., Mondal, R., Shabnam, A. A., Cabral-Pinto, M. M. S., et al. (2021). Nickel in terrestrial biota: Comprehensive review on contamination, toxicity, tolerance and its remediation approaches. Chemosphere 2021, 129996. doi: 10.1016/j.chemosphere.2021.129996
Meier, S. K., Adams, N., Wolf, M., Balkwill, K., Muasya, A. M., Gehring, C. A., et al. (2018). Comparative RNA-seq analysis of nickel hyperaccumulating and non-accumulating populations of Senecio coronatus (Asteraceae). Plant J. 95, 1023–1038. doi: 10.1111/tpj.14008
Mondal, K., Sharma, N. S., Malhotra, S. P., Dhawan, K., and Singh, R. (2004). Antioxidant systems in ripening tomato fruits. Biol. Plant 48, 49–53. doi: 10.1023/B:BIOP.0000024274.43874.5b
Muhammed, M. A., Mohamed, A. K. S., Qayyum, M. F., Haider, G., and Ali, H. A. (2022). Physiological response of mango transplants to phytohormones under salinity stress. Sci. Hort. 296, 110918. doi: 10.1016/j.scienta.2022.110918
Nazir, F., Hussain, A., and Fariduddin, Q. (2019). Interactive role of epibrassinolide and hydrogen peroxide in regulating stomatal physiology, root morphology, photosynthetic and growth traits in Solanum lycopersicum L. under nickel stress. Environ. Exp. Bot. 162, 479–495. doi: 10.1016/j.envexpbot.2019.03.021
Nouh, F. A. A., Nahas, H. H. A., and Abdel-Azeem, A. M. (2020). Piriformospora indica: endophytic fungus for salt stress tolerance and disease resistance. Agric. Important Fungi Sustain. Agric. 102, 261–283. doi: 10.1007/978-3-030-48474-3_9
Plazas, M., Nguyen, H. T., González-Orenga, S., Fita, A., Vicente, O., Prohens, J., et al. (2019). Comparative analysis of the responses to water stress in eggplant (Solanum melongena) cultivars. Plant Physiol. Biochem. 143, 72–82. doi: 10.1016/j.plaphy.2019.08.031
Rajput, S., Sengupta, P., Kohli, I., Varma, A., Singh, P. K., and Joshi, N. C. (2022). “Role of Piriformospora indica in inducing soil microbial communities and drought stress tolerance in plants,” in New and Future Developments in Microbial Biotechnology and Bioengineering (Amsterdam: Elsevier), 93–110.
Rizwan, M., Mostofa, M. G., Ahmad, M. Z., Imtiaz, M., Mehmood, S., Adeel, M., et al. (2018). Nitric oxide induces rice tolerance to excessive nickel by regulating nickel uptake, reactive oxygen species detoxification and defense-related gene expression. Chemosphere 191, 23–35. doi: 10.1016/j.chemosphere.2017.09.068
Sabra, M., Aboulnasr, A., Franken, P., Perreca, E., Wright, L. P., and Camehl, I. (2018). Beneficial root endophytic fungi increase growth and quality parameters of sweet basil in heavy metal contaminated soil. Front. Plant Sci. 9, 1726. doi: 10.3389/fpls.2018.01726
Sepehri, M., and Khatabi, B. (2021). Combination of siderophore-producing bacteria and Piriformospora indica provides an efficient approach to improve cadmium tolerance in alfalfa. Microb. Ecol. 81, 717–730. doi: 10.1007/s00248-020-01629-z
Shahzad, B., Tanveer, M., Rehman, A., Cheema, S. A., Fahad, S., Rehman, S., et al. (2018). Nickel; whether toxic or essential for plants and environment-A review. Plant Physiol. Biochem. 132, 641–651. doi: 10.1016/j.plaphy.2018.10.014
Sharma, P., Ngo, H. H., Khanal, S., Larroche, C., Kim, S. H., and Pandey, A. (2021). Efficiency of transporter genes and proteins in hyperaccumulator plants for metals tolerance in wastewater treatment: Sustainable technique for metal detoxification. Environ. Tech. Innov. 23, 101725. doi: 10.1016/j.eti.2021.101725
StatSoft (2011). Statistica (Data Analysis Software System), v. 10.0. Available online at: www.statsoft.com
Usman, K., Al-Ghouti, M. A., and Abu-Dieyeh, M. H. (2019). The assessment of cadmium, chromium, copper, and nickel tolerance and bioaccumulation by shrub plant Tetraena qataranse. Sci. Rep. 9, 1–11. doi: 10.1038/s41598-019-42029-9
Van der Pas, L., and Ingle, R. A. (2019). Towards an understanding of the molecular basis of nickel hyperaccumulation in plants. Plants 8, 11. doi: 10.3390/plants8010011
Varma, A., Bajaj, R., Agarwal, A., Asthana, S., Rajpal, K., Das, A., et al. (2013). Memoirs of ‘Rootonic'-the Magic Fungus. Gurgaon: Amity University Press.
Visioli, G., Gullì, M., and Marmiroli, N. (2014). Noccaea caerulescens populations adapted to grow in metalliferous and non-metalliferous soils: Ni tolerance, accumulation and expression analysis of genes involved in metal homeostasis. Environ. Exp. Bot. 105, 10–17. doi: 10.1016/j.envexpbot.2014.04.001
Vittori-Antisari, L., Carbone, S., Gatti, A., and Vianello, G. P. (2015). Uptake and translocation of metals and nutrients in tomato grown in soil polluted with metal oxide (CeO2, Fe3O4, SnO2, TiO2) or metallic (Ag, Co, Ni) engineered nanoparticles. Environ. Sci. Pollut. Res. 22, 1841–1853. doi: 10.1007/s11356-014-3509-0
Zhongfu, N. I., Eun-Deok, K., and Jeffrey Chen, Z. (2009). “Chlorophyll and starch assays,” in Chen Lab (Austin, TX: The University of Texas at Austin), 2677.
Keywords: nickel, Piriformospora indica, root, antioxidant enzymes, hydrogen peroxidase
Citation: Mahmoodi N, Movahedi Z and Ghabooli M (2023) Impact of Piriformospora indica on various characteristics of tomatoes during nickel nitrate stress under aeroponic and greenhouse conditions. Front. Microbiol. 13:1091036. doi: 10.3389/fmicb.2022.1091036
Received: 06 November 2022; Accepted: 28 December 2022;
Published: 03 February 2023.
Edited by:
Manoj Kumar Solanki, University of Silesia in Katowice, PolandReviewed by:
Kaushik Das, University of Calcutta, IndiaM. H. M. Borhannuddin Bhuyan, Bangladesh Agricultural Research Institute, Bangladesh
Copyright © 2023 Mahmoodi, Movahedi and Ghabooli. This is an open-access article distributed under the terms of the Creative Commons Attribution License (CC BY). The use, distribution or reproduction in other forums is permitted, provided the original author(s) and the copyright owner(s) are credited and that the original publication in this journal is cited, in accordance with accepted academic practice. No use, distribution or reproduction is permitted which does not comply with these terms.
*Correspondence: Zahra Movahedi, emFocmFfbW92YWhlZGlfMzEyQHlhaG9vLmNvbQ==