- 1Key Laboratory of Medical Microbiology and Parasitology & Key Laboratory of Environmental Pollution Monitoring and Disease Control, Ministry of Education, School of Basic Medical Sciences, Guizhou Medical University, Guiyang, Guizhou, China
- 2Beijing Key Laboratory of Plant Gene Resources and Biotechnology for Carbon Reduction and Environmental Improvement, College of Life Sciences, Capital Normal University, Beijing, China
- 3Center for Yunnan Plateau Biological Resources Protection and Utilization, College of Biological Resource and Food Engineering, Qujing Normal University, Qujing, China
- 4Section of Genetics, Institute for Research and Development in Health and Social Care, Battaramulla, Sri Lanka
- 5National Institute of Fundamental Studies, Kandy, Sri Lanka
- 6State key Laboratory of Microbial Technology, Shandong University, Qingdao, China
- 7Department of Hospital Infection Management, Affiliated Hospital of Guizhou Medical University, Guiyang, Guizhou, China
- 8Chishui Riverside Jiangi-Flavour Baijiu Research Center, Guizhou Sunveen Liquor Co., Ltd, Guiyang, China
- 9Department of Biology, Faculty of Science, Chiang Mai University, Chiang Mai, Thailand
- 10Environmental Science Research Center, Faculty of Science, Chiang Mai University, Chiang Mai, Thailand
The efficiently renewable bioethanol can help to alleviate energy crisis and environmental pollution. Genetically modified strains for efficient use of xylose and developing lignocellulosic hydrolysates play an essential role in facilitating cellulosic ethanol production. Here we present a promising strain GRE3OE via GRE3 overexpressed in a previously reported Saccharomyces cerevisiae strain WXY70. A comprehensive evaluation of the fermentation level of GRE3OE in alkaline-distilled sweet sorghum bagasse, sorghum straw and xylose mother liquor hydrolysate. Under simulated corn stover hydrolysate, GRE3OE produced 53.39 g/L ethanol within 48 h. GRE3OE produced about 0.498 g/g total sugar in sorghum straw hydrolysate solution. Moreover, GRE3OE consumed more xylose than WXY70 in the high-concentration xylose mother liquor. Taken together, GRE3OE could be a candidate strain for industrial ethanol development, which is due to its remarkable fermentation efficiency during different lignocellulosic hydrolysates.
Introduction
With the higher demand for fossil fuel, the atmospheric CO2 level is rising. Thus, biofuels have become increasingly popular as sustainable and renewable alternative energy sources (e.g., bioethanol; Sheehan, 2009). Bioethanol is environmentally friendly and abundant. Hence, used for biofuel, which is an alternative to fossil fuel due to biodegradability and reduced toxicity (Baghel et al., 2015; Vasić et al., 2021). Corn and sugarcane are mainly used as inputs in the production of bioethanol in Brazil and the United States. As well as sustainability issues, there are limitations to the using of food resources (Greene et al., 2004; Farrell et al., 2006). It is necessary to increase ethanol production for green environmental and economic sustainability. Renewable cellulosic ethanol is gaining more attention due to its low cost and ability to reduce environmental pollution.
The two most abundant components in lignocellulose feedstock hydrolysates are glucose and xylose (Jeffries, 1985). In co-fermentations of glucose and xylose, industrially robust microbes have not been available. It has been found that Saccharomyces cerevisiae produces ethanol with a high yield and productivity, making it a good candidate for bioethanol production (Lee et al., 2016). Saccharomyces cerevisiae has been reported with high capacity for converting glucose into ethanol (Piskur et al., 2006). The artificial introduction of xylose metabolism genes (including XR/XDH pathway and XI pathway) into Saccharomyces cerevisiae results in efficiently mixed sugar co-fermentation for high ethanol production (Lee et al., 2021). In view of this, the development of high bioethanol-producing yeast strains from cellulose hydrolysates is a timely needed and a vital task.
However, wild-type Saccharomyces cerevisiae is incapable of utilizing xylose during the fermentation process. Therefore, a variety of strain development procedures have been used to create Saccharomyces cerevisiae that can quickly and effectively ferment xylose. Biomass is efficiently converted into ethanol, xylose utilization is more important to industries. Recently, genetically modified Saccharomyces cerevisiae have attracted great attention for their ability to efficiently utilize xylose (Lee et al., 2021). Saccharomyces cerevisiae utilized xylose through a xylose reductase/xylitol dehydrogenase (XR/XDH) pathway and a xylose isomerase (XI) pathway (Cao et al., 2014; Bae et al., 2021). Compared with strains using XI pathway, XR/XDH pathway strains consume xylose more efficiently and produce more ethanol production (Karhumaa et al., 2007). Previously, the XR/XDH expression strains were successfully constructed in-vitro and showed a trend towards increased ethanol production (Zhang et al., 2019; Sun and Jin, 2021). Through this pathway that xylitol can be converted to xylulose by the NADPH-dependent enzyme xylose reductase (XR), encoded by XYL1. By NAD+-dependent xylitol dehydrogenase (XDH) encoded by XYL2, xylulose is converted (Zha et al., 2012). The GRE3 gene encodes a non-specific aldose reductase containing NADPH as a co-factor (Romaní et al., 2015). The GRE3 genes of Candida albicans and Saccharomyces cerevisiae, and the XYL1 gene of Scheffersomyces stipitis, are highly related sequences encoding predicted xylose reductase activity (Harcus et al., 2013). The expression levels of GRE3, XYL2 and XYL3 could be optimized to further resolve the xylose redox imbalance (Kim et al., 2017). We speculate that GRE3 has similar potential to XYL1 and XYL2 in terms of xylose utilization and plays a critical role in xylose metabolism.
There is still much more endeavor for improvement in ethanol production due to the high concentration of mixed sugars and inhibitors of various microorganisms produced by industrial physicochemical pretreatment of lignocellulosic feedstocks. In this study, a gene closely associated with ethanol metabolism by introducing GRE3, into XR/XDH utilized yeast strain to improve the biofuel production from cellulose hydrolysates and to provide a potential industrial strain for the development of efficient cellulosic ethanol. In different hydrolysates obtained by different pretreatment methods, modification yeast strains were evaluated for fermentation efficiency. In this study, the overexpression of GRE3 can improve ethanol production in different hydrolysates, using the parental strain WXY70 as a reference. We finally confirm that GRE3OE is a promising strain in industrial applications.
Materials and methods
The construction of yeast strains and plasmids
Previously evolved strain CE7 that nos. 15,567 stored in China General Microbiological Culture Collection Center, the XR-XDH-XK pathway was introduced to obtain strain WXY70 by Zhang et al. (2019). An amplified copy of the GRE3 gene was linked to a linearized fragment T1 from plasmid pT1-0 (L1-PPGK1-TPGI-L2) using the Golden Gate assembly method to produce plasmid pT1-1 (Zhang et al., 2019). By linearizing pT1-1 with PCR following DpnI digestion, we obtained the fragments L1-PPGK1-GRE3-TPGI-L2. In addition, plasmids pT5-0 and pT3-0 contained linearized fragments CAT8up-AbA-L1 and L2-CAT8down. WXY70 was produced by co-transforming these three fragments. In the confirmed strain, PPGK1-GRE3-TPGI is integrated at the CAT8 site and drives overexpression of GRE3, which is known as GRE3OE.
Simulated corn stover hydrolysate fermentation analysis
Yeast cells were grown in normal YPD (Difco, United States) medium shaking at 200 rpm for 24 h, then centrifuged for 5 min and washed twice with sterile water before collection. Inoculated yeast was inoculated into 250 ml shake flasks with 100 ml of simulated corn stover hydrolysate (80 g/l glucose, 40 g/l xylose, and 3 g/l acetate) at an OD600 value of 1 (Zhang et al., 2019). To reduce air exchange, fermentation was performed under anaerobic conditions at 30°C, 200 rpm, and three layers of parafilm. The samples were retrieved by syringe needle at every 12 h time interval for HPLC analysis. Three replicates were performed for each experiment.
Alkaline distillation of sweet sorghum bagasse hydrolysate fermentation
A solid-state fermentation produced sweet sorghum with a diameter of 1 to 2 mm and a length of 3 to 50 mm. The alkaline-distilled process is carried out by completely mixing approximately 4 kg of sweet sorghum bagasse with 10% (w/w DM) NaOH concentrate solution, which is then loaded into distillation stripper designed and manufactured by Tsinghua University. Hydrolysis method according to the described by Yu et al. (2014). A solid-to-liquid ratio of 1: 5 is achieved after hydrolysis, the insoluble solids removed by centrifugation and then supplemented 2 g/l KH2PO4, 2 g/l (NH4)2SO4, 1 g/l MgSO4 and 10 g/l yeast extract for fermentation. Initial fermentation cell density was OD600 = 1.0. Three replicates were performed for each experiment.
Sorghum straw hydrolysate fermentation
The pretreatment of sorghum straw hydrolysate solution was based on Wu et al. (2021). A collection of sorghum straw (SS) in the suburbs of Lianyungang (China) and processed through a 40 mesh sieve grinder. An enclosed conical flask with a liquid–solid ratio of 10: 1 (wt: wt) was used. SS was pretreated in dilute NaOH (0.75 wt%) solution and then placed in an autoclave at 121°C for 1 h (Wu et al., 2021). An exact ratio of choline chloride (ChCl) and lactic acid (LAC) was mixed. To reduce evaporation, 60°C was applied to the mixture in a conical flask with a stopper and stirred continuously (160 rpm) until a clear solvent was obtained. An oil bath at 150°C was used to mix 75.0 g of ChCl: LAC with 5.0 g of SS in a three-necked flask. Pretreated SS was also obtained by washing and dehydrating the mixture. A triplicate sample of the hydrolysis was prepared, while other hydrolysate samples were stored at 4°C for future use.
Analytical procedures for yeast fermentation processes
Determination of substrate concentrations in samples including mixed sugars and metabolites containing ethanol and xylitol using a 1,260 HPLC column HPX-87H (Bio-Rad, United States) at 30°C and a relative index of refraction detector at 50°C. The mobile phase used in the HPLC system was 5 mM H2SO4 flowing at a rate of 0.4 ml/min (Zhang et al., 2019). Xylose mother liquor with a sugar content of 120 g/l xylose (Jin et al., 2019). An analytical grade is used for all chemicals.
Result and discussion
Construction of GRE3OE strains and fermentation analysis
Our previously reported strain SFA1OE by expressing SFA1 gradually increased ethanol production in different lignocellulosic hydrolysates (Zhu et al., 2020). As shown in Figure 1A, we found differences in GRE3 expressed genes displayed upregulated transcription trend according to our transcriptome analysis in strains WXY70 and SFA1OE during the fermentation process, based on the efficient fermentation performance of SFA1OE and the excellent ethanol production, it is reasonable to hypothesize that GRE3 gene expression is positively correlated with ethanol production and could further improve fermentation in industrial settings.
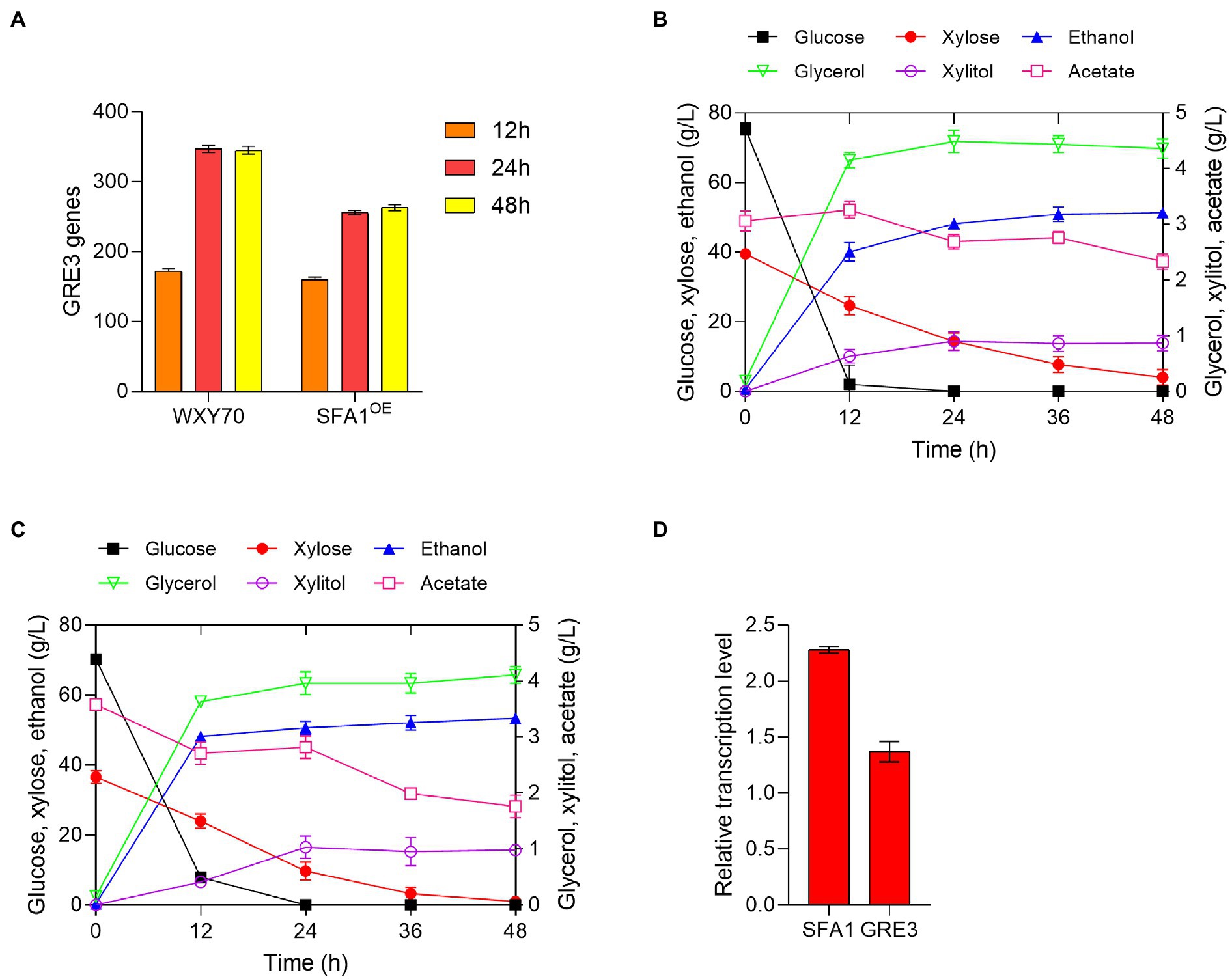
Figure 1. Analysis of transcript levels of engineered strains and fermentation in simulated corn stover hydrolysate solution. (A) Amount of GRE3 genes in strains WXY70 and SFA1OE. Fermentation profiles of the parental strain WXY70 (B), GRE3OE (C) in simulated corn stover hydrolysate within 48 h. (D) The transcriptional level analysis of SFA1OE and GRE3OE strains overexpressed genes.
In this study, GRE3OE was constructed by expressing GRE3 in the parental strain WXY70 and fermented in the simulated corn stover hydrolysate (Table 1). At 12 h, WXY70 and GRE3OE consumed all glucose, and gradually increased ethanol production. During fermentation, the remaining xylose contents of WXY70 and GRE3OE were 4.03 and 0.96 g/l, respectively (Figures 1B,C). At 48 h, WXY70 and GRE3OE produced 51.37 and 53.39 g/l of the maximum ethanol concentrations, or ethanol yield of 0.447 and 0.498 g/g total sugars, approximately 87.65 and 97.65% of the theoretical value, proving that GRE3OE performed better during fermentation to produce ethanol and improved xylose metabolic capacities. In addition, WXY70 consumed less acetate (0.73 g/l) than GRE3OE (1.82 g/l). A mixture of glucose and xylose resulted in significant improvements in ethanol production by GRE3OE. The results suggest that applying the XR/XDH to engineered yeast strains during fermentation can be a promising strategy. In contrast, deletion of the GRE3 gene also yielded 67.8% of the theoretical value for ethanol in mixed sugar fermentation (Tanino et al., 2010). Additionally, Saccharomyces cerevisiae strain SR8 fermented xylose excellently, with an ethanol yield of 0.359 g/g total sugars (Shin et al., 2019). Above results suggest that the GRE3 is efficient in regulating the consume of sugars and has a positive effect on the efficient of glucose and xylose consumption as well as the rate of ethanol production.
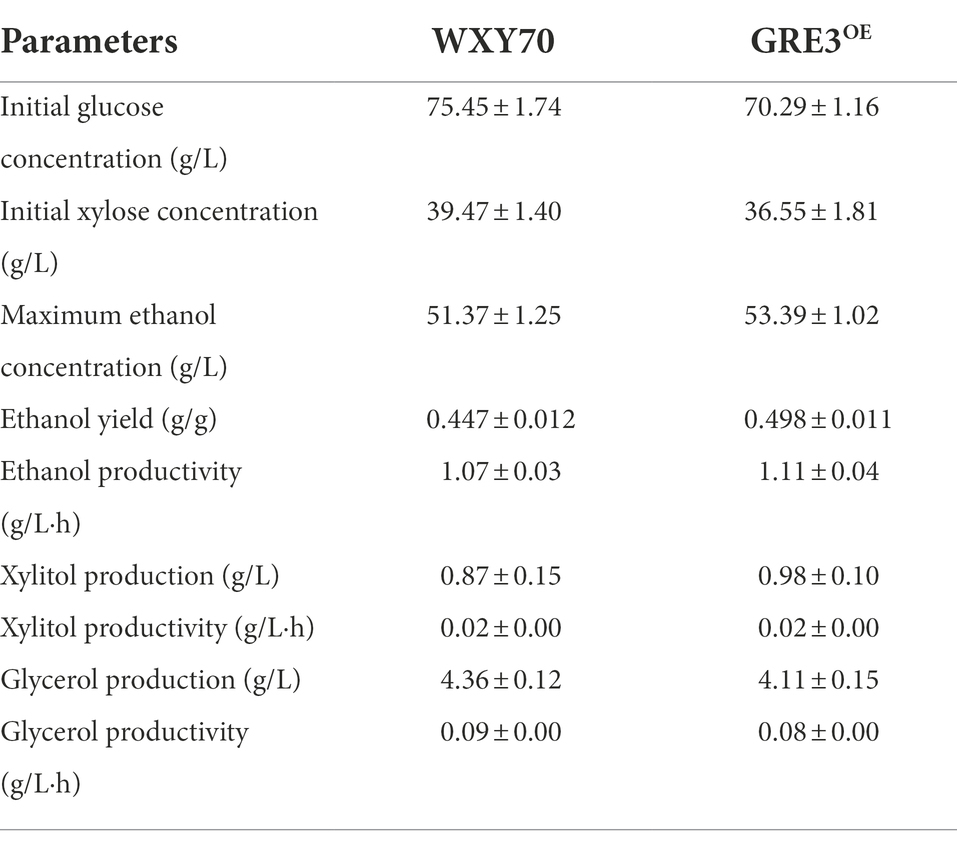
Table 1. Comparison of fermentation profile in simulated corn stover hydrolysate fermentation between WXY70 and GRE3OE of the 48 h.
To better validate these results, we examined the transcript levels of SFA1OE and GRE3OE as shown in Figure 1D. Interestingly, the expression levels of SFA1 and GRE3 were increased, which were 2.28 and 1.37 times of WXY70. Overexpression of GRE3 may further overcome the redox imbalance in xylose utilization, resulting in increased ethanol production. A transcriptomic analysis of yeast strains according to its foldchange revealed positive correlations between previously reported key genes SFA1, CCW12 and SPT2 expressions and the ethanol production improvements (Li et al., 2022). Based on the previously reported excellent ethanol production performance of the SFA1OE strain (Zhu et al., 2020), thus, it is speculated that high ethanol yield in GRE3OE is closely related to the changes in their expression levels. In summary, we speculated that expression of GRE3 resulted in upregulation of CDC19 which encoding enzymes involved in pyruvate to ethanol pathway, yielding high ethanol production. The above results motivated us to measure the fermentability of GRE3OE in a different of hydrolysates to further evaluation of the fermentation profile of the GRE3OE strain.
Evaluation of the fermentation capacity of GRE3OE in alkaline-distilled sweet sorghum bagasse
In addition to providing plenty of soluble sugars, sweet sorghum bagasse has a high lignocellulosic content, making it a potential source of energy crop for the production of bioethanol. During the integrated fermentation process, soluble mixture sugars from released sweet sorghum hydrolysate can be fermented through a proposed Advanced Solid-state Fermentation technology to produce efficient 1.5 generation bioethanol production (Li et al., 2013), an alkali-distillation process combines ethanol distillation and alkali pretreatment in order to convert sweet sorghum bagasse’s lignocellulose into ethanol (Li et al., 2013). This advanced technology increases the efficiency of sweet sorghum bagasse utilization through a green, low consumption process.
Considering that sweet sorghum bagasse with high mixed sugar content, the feasibility of producing cellulosic ethanol from alkaline-distilled sweet sorghum bagasse with GRE3OE was evaluated (Table 2). Within fermentation process of 12 h, both strains WXY70 and GRE3OE consumed almost all the glucose. The xylose was consumed at 8.79 g/l and 9.19 g/l for WXY70 and GRE3OE, respectively. GRE3OE has better xylose consumption capacity. At 12 h, WXY70 produced 7.43 g/l ethanol, with an ethanol yield of 0.310 g/g total sugars, or 60.70% of the theoretical value (Figure 2A), GRE3OE produced 10.77 g/l ethanol, with an ethanol yield of 0.431 g/g total sugars, or 84.47% of the theoretical value (Figure 2B). GRE3OE produced the maximum ethanol concentration earlier than WXY70. GRE3OE consumed more sugar and thus produced more ethanol than WXY70 at the same time. As shown in Figures 2A,B, acetate as an inhibitor can inhibit the growth of yeast cells, GRE3OE consumed slightly acetate (0.60 g/l) than WXY70 (0.58 g/l), indicating that GRE3OE contributes in the consumption inhibitor, allowing the strain to produce ethanol during the fermentation more effectively. Based on these results, we also observed that sugar alcohol conversion of GRE3OE was higher than WXY70. At 12 h, the sugar alcohol conversion of WXY70 and GRE3OE were 31.20 and 43.66% (Figure 2C). Within 36 h, the sugar alcohol conversion rate of GRE3OE was higher than that of WXY70, suggesting that GRE3OE is more efficient at converting sugar to ethanol in a short period of time. The increased sugar alcohol conversion of GRE3OE may be due to a further improvement in the redox imbalance in xylose metabolism. The above results show that GRE3OE had much better fermentation performance than WXY70.
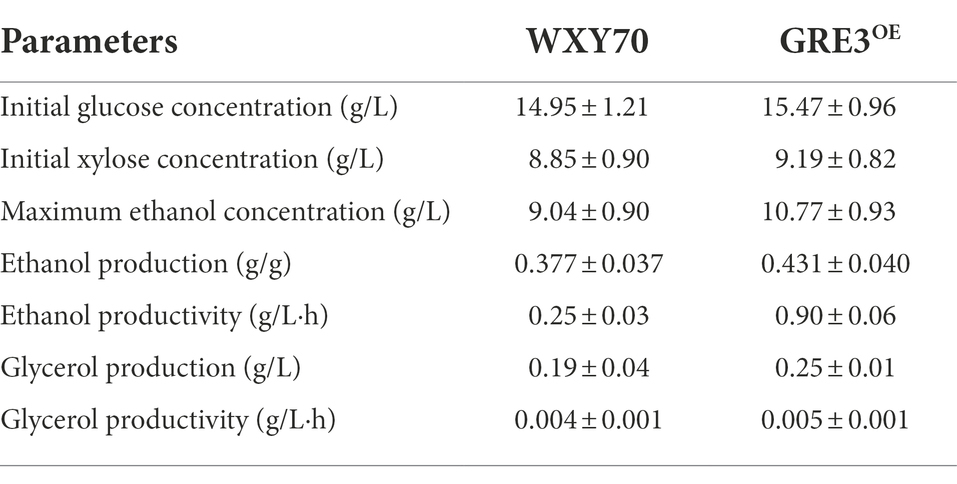
Table 2. Comparison of fermentation profile in alkaline-distilled sweet sorghum bagasse fermentation between WXY70 and GRE3OE of the 48 h.
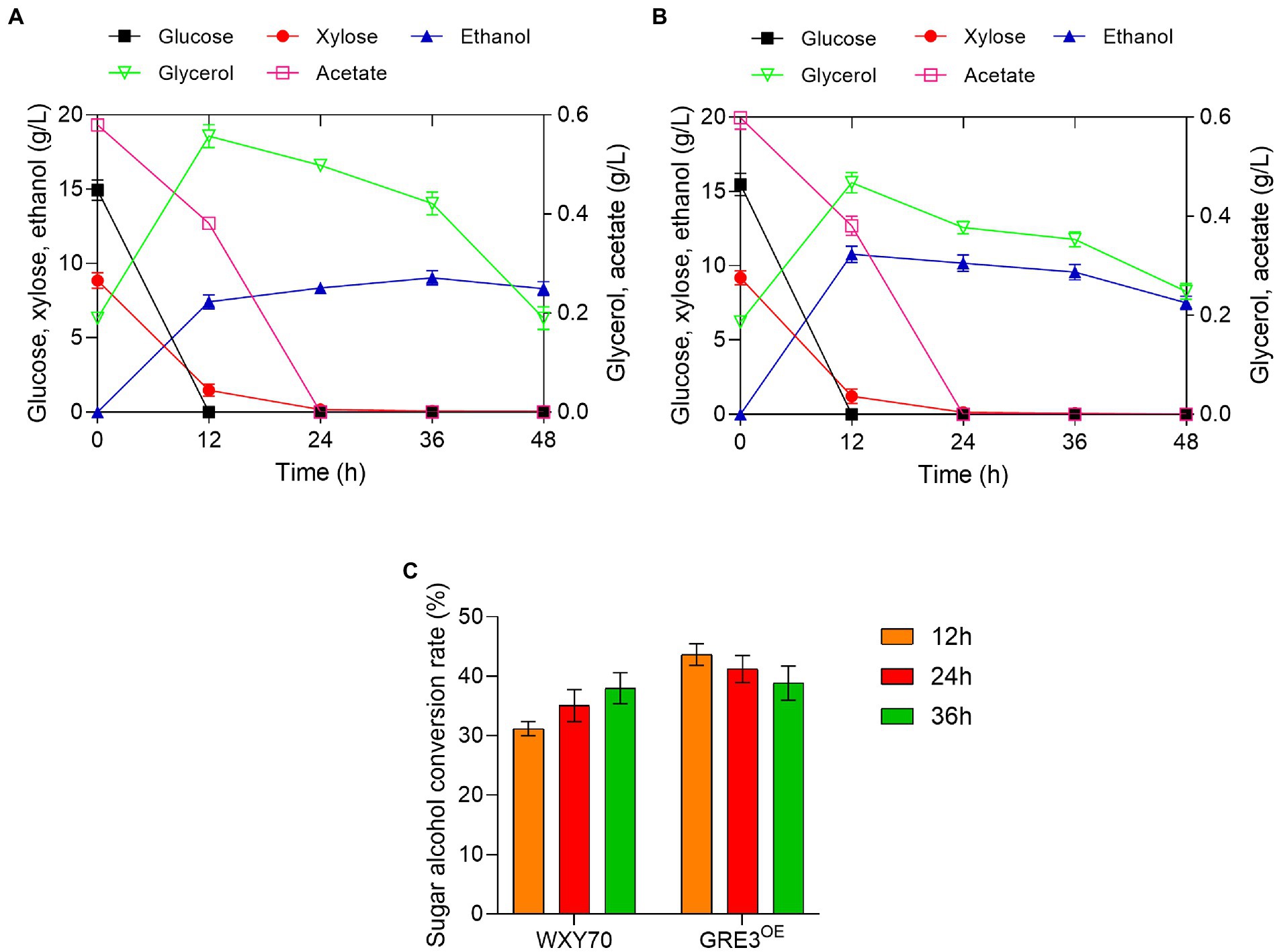
Figure 2. Profiles of strain WXY70 and GRE3OE during fermentation and growth in alkaline-distilled sweet sorghum bagasse hydrolysate solution. Fermentation and growth profile of WXY70 (A) and GRE3OE (B) within 48 h. (C) The sugar alcohol conversion rate of WXY70 and GRE3OE at 12 h, 24 h, and 36 h.
We previously reported an efficient engineered strain SFA1OE (Zhu et al., 2020), a yeast strain with efficient fermentation performance in alkaline-distilled sweet sorghum bagasse, we consider GRE3OE to be similar to SFA1OE. Thus, we evaluated the fermentation performance of GRE3OE under alkaline-distilled sweet sorghum bagasse. As expected, GRE3OE produced more cellulosic ethanol from alkaline-distilled sweet sorghum bagasse hydrolysate than control strain WXY70. Contrary to previous reports that strain Zymomonas mobilis TSH-01 produced 5.65 g/l ethanol, or 0.161 g/g total sugar under the same condition of alkaline-distilled sweet sorghum bagasse (Zhu et al., 2020). Above this overexpression of GRE3 genes in Saccharomyces cerevisiae improves the fermentation performance. The alkaline distillation of lignocellulosic materials is considered as a cost-effective method of producing bioethanol from 1.5 generations of biomass. For the production of bioethanol, GRE3OE strain ensures high efficacy and complete utilization of sweet sorghum bagasse.
Evaluation of the fermentation capacity of GRE3OE in sorghum straw hydrolysate solution
Straw from sorghum is a renewable feedstock that can be used to produce biofuels and biobased chemicals (Matsakas et al., 2014). We evaluated the fermentability of WXY70 and GRE3OE in sorghum straw hydrolysate solution (Table 3). Within fermentation process of 48 h, both strains WXY70 and GRE3OE consumed almost all the glucose, the control strain WXY70 produced 7.43 g/l ethanol production, with an ethanol yield of 0.437 g/g total sugars, or 85.70% of the theoretical maximum (Figure 3A). Meanwhile GRE3OE produced 7.73 g/l ethanol, with an ethanol yield of 0.455 g/g total sugars, or 89.16% of the theoretical maximum (Figure 3B). Our fermentation results showed that GRE3OE produced slightly ethanol production than WXY70 in sorghum straw hydrolysate solution.
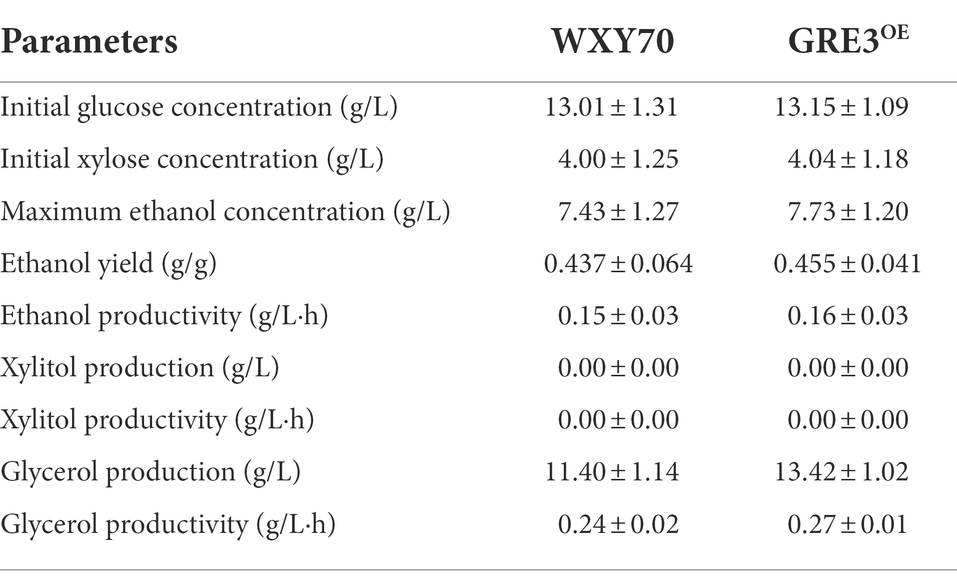
Table 3. Comparison of fermentation profile in sorghum straw hydrolysate fermentation between WXY70 and GRE3OE of the 48 h.
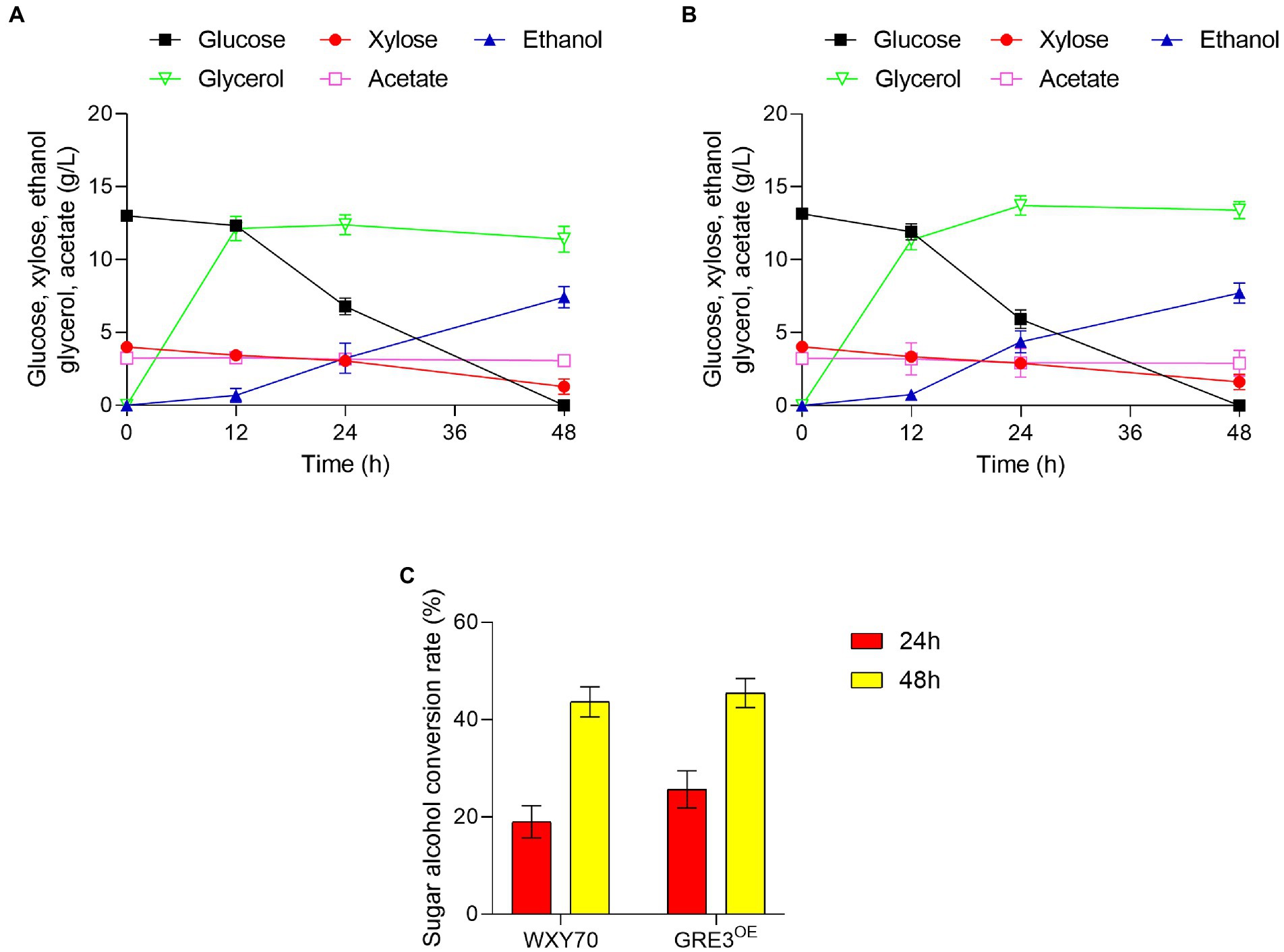
Figure 3. The growth and fermentation characteristics of strain WXY70 and GRE3OE in sorghum straw hydrolysate solution. Growth and fermentation characteristics of WXY70 (A) and GRE3OE (B) within 48 h. (C) The sugar alcohol conversion rate of WXY70 and GRE3OE at 24 h and 48 h.
However, WXY70 produced 11.40 g/l glycerol while GRE3OE produced 13.42 g/l at 48 h. We observed that the GRE3OE strain produced more glycerol than WXY70. The close contact due to GRE3 and GPD1, a gene that controls glycerol synthesis (Aguilera and Prieto, 2004). Research has been carried out to improve ethanol production by overexpressing GRE3 instead of XYL1 in Saccharomyces cerevisiae (Kim et al., 2013), and we reasonably hypothesize that GRE3 is correlated with XYL1 and both of them are aldose reductases. Overexpression of XYL1, regulated by the strong promoter of PGK1, increased the XR activity of the strains in the study, and an increase in glycerol production in strains with high XR activity was also observed (Jeppsson et al., 2003). We speculate that GRE3 overexpression increased XR activity and led to upregulation of GPD1 thus increasing ethanol and glycerol production. Acetate is the main inhibitor in industrial fermentation solutions. At the same time, WXY70 consumed 0.16 g/l acetate while GRE3OE consumed 0.34 g/l.
As shown in Figure 3C, the sugar alcohol conversion of WXY70 and GRE3OE was 19.04 and 25.67% at 24 h. At 48 h the sugar alcohol conversion of WXY70 and GRE3OE was 43.68 and 45.46%. This fermentation result showed GRE3OE has higher sugar alcohol conversion than WXY70. Thus, our fermentation results suggested GRE3 enhances the sugar utilization efficiency in sorghum straw hydrolysates solution resulted in more ethanol production.
Evaluation of the fermentation capacity of GRE3OE in xylose mother liquor
Functional sugar alcohols can be produced from the xylose mother liquor (XML) generated during xylitol production (Jin et al., 2019). However, there is a great deal of difficulty in directly separating or reusing this xylose (Wang et al., 2016; Liu et al., 2018). We have previously determined the relative fermentation profiles of the highly efficient engineered strains SPT2OE and 8G-3 in 120 g/l xylose and they have good fermentation performance (Li et al., 2022). Thus, the relative fermentation profiles of WXY70 and GRE3OE under the same experimental conditions were determined (Table 4). As shown in Figures 4A,B, both strains consumed all the glucose at 24 h. During the fermentation process, the remaining xylose content of WXY70 and GRE3OE strains was 4.04 g/l and 2.80 g/l, suggesting that GRE3OE consumed more xylose to produce high ethanol production. At 36 h, the control strain WXY70 produced 37.57 g/l ethanol, with an ethanol yield of 0.310 g/g total sugar or 60.71% of the theoretical maximum (Figure 4A). In contrast, GRE3OE produced 37.66 g/l ethanol, with an ethanol yield of 0.315 g/g total sugar, or 62.00% of the theoretical maximum (Figure 4B). The above results indicated that GRE3OE strain accelerated xylose metabolism and improved xylose utilization efficiency to produce more ethanol production.
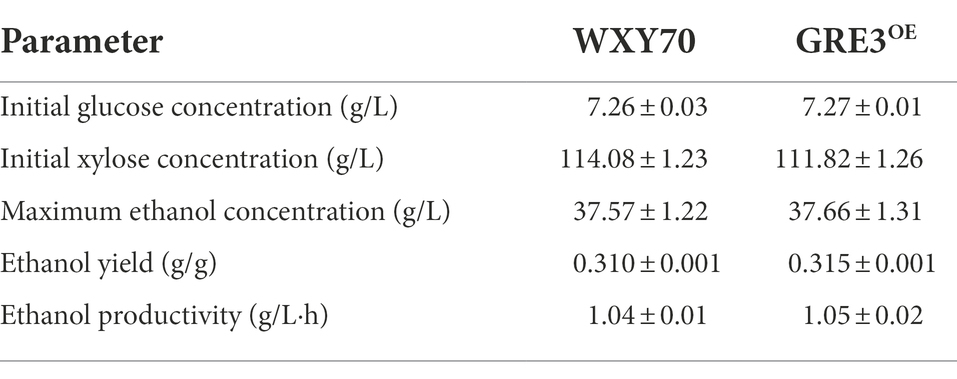
Table 4. Comparison of fermentation profile in xylose mother liquor hydrolysate fermentation between WXY70 and GRE3OE of the 48 h.
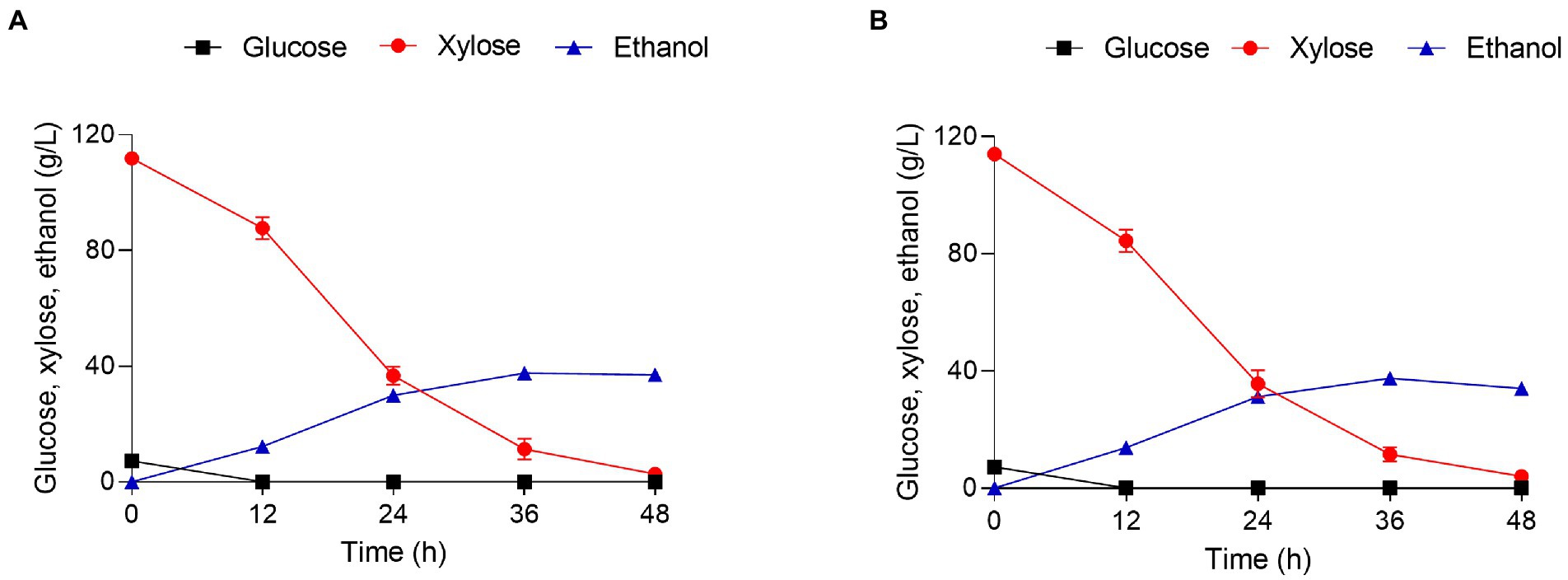
Figure 4. Xylose mother liquor hydrolysate fermentation and growth characteristics of strains WXY70 and GRE3OE. Growth and fermentation characteristics of WXY70 (A) and GRE3OE (B) within 48 h.
In addition, GRE3, XYL2, and XYL3 overexpressing strains exhibited decent XR and XDH activities (Kim et al., 2017). GRE3 has a potential function in xylose utilization and plays a critical role in xylose metabolism through XR/XDH pathway. An additional copy of XR resulted in a 1.7-fold increase in xylose consumption and a 55% decrease in xylitol yield in a previous study (Jeppsson et al., 2003). It is possible to improve xylose utilization by balancing the XR activity (Karhumaa et al., 2006). Meanwhile, engineered strains use the PGK1 promoter to increase XR activity, resulting in assimilating and metabolizing xylose faster (Zha et al., 2012). Our fermentation results in this study also using a stronger promoter PGK1 to express GRE3 further increase xylose consumption as well as increase ethanol production. Although previous studies reported that engineered strain with deletion of the GRE3 could increase ethanol production (Träff et al., 2001; Tanino et al., 2010). Their strains are based on the XI/XK pathway, while our strains are based on the XR/XDH pathway. We used different xylose metabolic pathways and our fermentation results showed that due to the XR/XDH pathway GRE3OE strains could also perform well in ethanol production.
In this study, these fermentation results indicated our metabolic engineering modification strategies are rational. The engineered industrial strain demonstrated efficacious xylose utilization. Besides, we observed that the engineered Saccharomyces cerevisiae overexpressing GRE3 exhibited better xylose fermentation and ethanol production than the control strain, which is also consistent with previous studies (Träff-Bjerre et al., 2003; Kim et al., 2013). Taken together, GRE3OE exhibited superior fermentation properties in comparison with that of WXY70 in complex hydrolysate environments, resulting in its potential industrial ethanol application.
Conclusion
The target strain GRE3OE was obtained by metabolic and evolutionary engineering. Alkaline-distilled sweet sorghum bagasse, sorghum straw, and xylose mother liquor was used to evaluate GRE3OE contribution to cellulosic ethanol production. GRE3OE outperformed the control strain in simulated corn stover hydrolysate within 48 h, achieving an over 53 g/l of ethanol. GRE3OE produced ethanol yield of 0.498 g/g total sugars within 48 h in sorghum straw hydrolysate solution. These results indicate that GRE3OE outperformed control strain in alkaline-distilled sweet sorghum bagasse, sorghum straw and xylose mother liquor hydrolysate solution. As a result of this study, a new pathway for efficient bioethanol production has opened up and a potential industrial strain has been identified. In the future, we can positively focus on the expression of the GRE3 to contribute ethanol production. Based on our obtained results in this study, GRE3OE has a high glycerol production, we can achieve transformation by combining GPD1 and GRE3 expressing. The further research work promotes the conversion of glycerol into ethanol by expressing glycerol dehydrogenase gene and pull more carbon towards ethanol rather than the by-products, which increasing the yield and rate of ethanol.
Data availability statement
The original contributions presented in the study are included in the article/supplementary material; further inquiries can be directed to the corresponding author.
Author contributions
HW: data curation and writing original draft. LC: conceptualization and writing review and editing. QL: formal analysis and data curation. NW, XL, IP, and Q-RL: writing and review. JZ and MC: data curation. Y-QK: funding acquisition and writing and review. All authors contributed to the article and approved the submitted version.
Funding
This work was supported by the 111 Project (D20009); National Natural Science Foundation of China (NSFC; no. 32060034 and 22278273); State Key Laboratory of Microbial Technology Open Projects Fund (Project NO. M2022-09); Guizhou Scientific Plan Project ([2020]4Y220); Talent Base Project of Guizhou Province, China [RCJD2018-22]; Guizhou Provincial Academician Workstation of Microbiology and Health (No. [2020]4004); High-level Innovation Talent Project of Guizhou Province (GCC [2022]036–1); and Major Science and Technology Projects of China Tobacco [No.110202101048 (LS-08)].
Acknowledgments
The authors sincerely appreciate Prof. Yucai He, Prof. Shihui Yang, and Shizhong Li for kind helps on providing raw materials and writing guidance. IP is grateful to Chiang Mai University for partial support this research.
Conflict of interest
XL was employed by company Guizhou Sunveen Liquor Co., Ltd.
The remaining authors declare that the research was conducted in the absence of any commercial or financial relationships that could be construed as a potential conflict of interest.
Publisher’s note
All claims expressed in this article are solely those of the authors and do not necessarily represent those of their affiliated organizations, or those of the publisher, the editors and the reviewers. Any product that may be evaluated in this article, or claim that may be made by its manufacturer, is not guaranteed or endorsed by the publisher.
References
Aguilera, J., and Prieto, J. A. (2004). Yeast cells display a regulatory mechanism in response to methylglyoxal. FEMS Yeast Res. 4, 633–641. doi: 10.1016/j.femsyr.2003.12.007
Bae, J. H., Kim, M. J., Sung, B. H., Jin, Y. S., and Sohn, J. H. (2021). Directed evolution and secretory expression of xylose isomerase for improved utilisation of xylose in Saccharomyces cerevisiae. Biotechnol. Biofuels 14:223. doi: 10.1186/s13068-021-02073-y
Baghel, R. S., Trivedi, N., Gupta, V., Neori, A., Reddy, C. R. K., Lali, A., et al. (2015). Biorefining of marine macroalgal biomass for production of biofuel and commodity chemicals. Green Chem. 17, 2436–2443. doi: 10.1039/C4GC02532F
Cao, L., Tang, X., Zhang, X., Zhang, J., Tian, X., Wang, J., et al. (2014). Two-stage transcriptional reprogramming in Saccharomyces cerevisiae for optimizing ethanol production from xylose. Metab. Eng. 24, 150–159. doi: 10.1016/j.ymben.2014.05.001
Farrell, A. E., Plevin, R. J., Turner, B. T., Jones, A. D., O’Hare, M., and Kammen, D. M. (2006). Ethanol can contribute to energy and environmental goals. Science 311, 506–508. doi: 10.1126/science.1121416
Greene, N., Celik, F. E., Jackson, M., Jayawardhana, K., Larson, D., Mackenzie, D., et al. (2004). Growing energy: How Biofuels Can Help End America’s Oil Dependence. New York: Natural Resources Defense Council.
Harcus, D., Dignard, D., Lépine, G., Askew, C., Raymond, M., Whiteway, M., et al. (2013). Comparative xylose metabolism among the Ascomycetes C. albicans, S. stipitis and S. cerevisiae. PLoS One 11:e80733. doi: 10.1371/journal.pone.0080733
Jeffries, T. W. (1985). Emerging technology for fermenting D-xylose. Trends Biotechnol. 3, 208–212. doi: 10.1016/0167-7799(85)90048-4
Jeppsson, M., Träff, K., Johansson, B., Hahn-Hägerdal, B., and Gorwa-Grauslund, M. F. (2003). Effect of enhanced xylose reductase activity on xylose consumption and product distribution in xylose-fermenting recombinant Saccharomyces cerevisiae. FEMS Yeast Res. 3, 167–175. doi: 10.1016/S1567-1356(02)00186-1
Jin, L. Q., Yang, B., Xu, W., Chen, X. X., Jia, D. X., Liu, Z. Q., et al. (2019). Immobilization of recombinant Escherichia coli whole cells harboring xylose reductase and glucose dehydrogenase for xylitol production from xylose mother liquor. Bioresour. Technol. 285:121344. doi: 10.1016/j.biortech.2019.121344
Karhumaa, K., Fromanger, R., Hahn-Hägerdal, B., and Gorwa-Grauslund, M. F. (2006). High activity of xylose reductase and xylitol dehydrogenase improves xylose fermentation by recombinant Saccharomyces cerevisiae. Appl. Microbiol. Biotechnol. 73, 1039–1046. doi: 10.1007/s00253-006-0575-3
Karhumaa, K., Garcia Sanchez, R., Hahn-Hägerdal, B., and Gorwa-Grauslund, M. F. (2007). Comparison of the xylose reductase-xylitol dehydrogenase and the xylose isomerase pathways for xylose fermentation by recombinant Saccharomyces cerevisiae. Microb. Cell Factories 6:5. doi: 10.1186/1475-2859-6-5
Kim, S. R., Kwee, N. R., Kim, H., and Jin, Y. S. (2013). Feasibility of xylose fermentation by engineered Saccharomyces cerevisiae overexpressing endogenous aldose reductase (GRE3), xylitol dehydrogenase (XYL2), and xylulokinase (XYL3) from Scheffersomyces stipitis. FEMS Yeast Res. 13, 312–321. doi: 10.1111/1567-1364.12036
Kim, H., Lee, H. S., Park, H., Lee, D. H., Boles, E., Chung, D., et al. (2017). Enhanced production of xylitol from xylose by expression of Bacillus subtilis arabinose: H+symporter and Scheffersomyces stipitis xylose reductase in recombinant Saccharomyces cerevisiae. Enzym. Microb. Technol. 107, 7–14. doi: 10.1016/j.enzmictec.2017.07.014
Lee, Y. G., Jin, Y. S., Cha, Y. L., and Seo, J. H. (2016). Bioethanol production fromcellulosic hydrolysates by engineered industrial Saccharomyces cerevisiae. Bioresour. Technol. 228, 355–361. doi: 10.1016/j.biortech.2016.12.042
Lee, S. B., Tremaine, M., Place, M., Liu, L., Pier, A., Krause, D. J., et al. (2021). Crabtree/Warburg-like aerobic xylose fermentation by engineered Saccharomyces cerevisiae. Metab. Eng. 68, 119–130. doi: 10.1016/j.ymben.2021.09.008
Li, J., Li, S., Han, B., Yu, M., Li, G., and Jiang, Y. (2013). A novel cost-effective technology to convert sucrose and homocelluloses in sweet sorghum stalks into ethanol. Biotechnol.Biofuels. 6:174. doi: 10.1186/1754-6834-6-174
Li, X., Zhao, R., Li, S., Wang, Y., Wang, X., Yang, W., et al. (2022). Global reprogramming of xylose metabolism in Saccharomyces cerevisiae efficiently produces ethanol from lignocellulose hydrolysates. Ind. Crop. Prod. 179:114666. doi: 10.1016/j.indcrop.2022.114666
Liu, X., Lin, L., Xu, X., Zhang, H., Wu, L., Zhu, P., et al. (2018). Two-step economical welan gum production by Sphingomonas sp. HT-1 from sugar industrial by-products. Carbohydr. Polym. 181, 412–418. doi: 10.1016/j.carbpol.2017.09.033
Matsakas, L., Sterioti, A. A., Rova, U., and Christakopolos, P. (2014). Use of dried sweet sotghum for the efficient production of lipids by the yeast Lipomyces starkeyi CBS 1807. Ind. Crop. Prod. 62, 367–372. doi: 10.1016/j.indcrop.2014.09.011
Piskur, J., Rozpedowska, E., Polakova, S., Merico, A., and Compagno, C. (2006). How did saccharomyces evolve to become a good brewer? Trends Genet. 22, 183–186. doi: 10.1016/j.tig.2006.02.002
Romaní, A., Pereira, F., Johansson, B., and Domingues, L. (2015). Metabolic engineering of Saccharomyces cerevisiae ethanol strains PE-2 and CAT-1 for efficient lignocellulosic fermentation. Bioresour. Technol. 179, 150–158. doi: 10.1016/j.biortech.2014.12.020
Sheehan, J. (2009). Engineering direct conversion of CO2 to biofuel. Nat. Biotechnol. 27, 1128–1129. doi: 10.1038/nbt1209-1128
Shin, M., Kim, J. W., Ye, S., Kim, S., Jeong, D., Lee, D. Y., et al. (2019). Comparative global metabolite profiling of xylose-fermenting Saccharomyces cerevisiae SR8 and Scheffersomyces stipitis. Appl. Microbiol. Biotechnol. 103, 5435–5446. doi: 10.1007/s00253-019-09829-5
Sun, L., and Jin, Y. S. (2021). Xylose assimilation for the efficient production of biofuels and chemicals by engineered Saccharomyces cerevisiae. Biotechnol. J. 16:e2000142. doi: 10.1002/biot.202000142
Tanino, T., Hotta, A., Ito, T., Ishii, J., Yamada, R., Hasunuma, T., et al. (2010). Construction of a xylose-metabolizing yeast by genome integration of xylose isomerase gene and investigation of the effect of xylitol on fermentation. Appl. Microbiol. Biotechnol. 88, 1215–1221. doi: 10.1007/s00253-010-2870-2
Träff, K. L., Otero Cordero, R. R., Van Zyl, W. H., and Hahn-Hägerdal, B. (2001). Deletion of the GRE3 aldose reductase gene and its influence on xylose metabolism in recombinant strains of Saccharomyces cerevisiae expressing the xylA and XKS1 genes. Appl. Environ. Microbiol. 67, 5668–5674. doi: 10.1128/AEM.67.12.5668-5674.2001
Träff-Bjerre, K. L., Jeppsson, M., Hahn-Hägerdal, B., and Gorwa-Grauslund, M. F. (2003). Endogenous NADPH-dependent aldose reductase activity influences product formation during xylose consumption in recombinant Saccharomyces cerevisiae. Yeast 21, 141–150. doi: 10.1002/yea.1072
Vasić, K., Knez, Ž., and Leitgeb, M. (2021). Bioethanol production by enzymatic hydrolysis from different lignocellulosic sources. Molecules 26:753. doi: 10.3390/molecules26030753
Wang, H. W., Li, L. J., Zhang, L. B., An, J., Cheng, H. R., and Deng, Z. X. (2016). Xylitol production from waste xylose mother liquor containing miscellaneous sugars and inhibitors: one-pot biotransformation by Candida tropicalis and recombinant Bacillus subtilis. Microb. Cell Factories 15, 1–12. doi: 10.1186/s12934-016-0480-0
Wu, M., Gong, L., Ma, C., and He, Y. C. (2021). Enhanced enzymatic saccharification of sorghum straw by effective delignification via combined pretreatment with alkali extraction and deep eutectic solvent soaking. Bioresour. Technol. 340:125695. doi: 10.1016/j.biortech.2021.125695
Yu, M., Li, J., Li, S., Du, R., Jiang, Y., Fan, G., et al. (2014). A cost-effective integrated process to convert solid-state fermented sweet sorghum bagasse into cellulosic ethanol. Appl. Energy 115, 331–336. doi: 10.1016/j.apenergy.2013.11.020
Zha, J., Hu, M. L., Shen, M. H., Li, B. Z., Wang, J. Y., and Yuan, Y. J. (2012). Balance of XYL1 and XYL2 expression in different yeast chassis for improved xylose fermentation. Front. Microbiol. 3:355. doi: 10.3389/fmicb.2012.00355
Zhang, C., Xue, Q., Hou, J., Mohsin, A., Zhang, M., Guo, M., et al. (2019). In-depth two-stage transcriptional reprogramming and evolutionary engineering of Saccharomyces cerevisiae for efficient bioethanol production from xylose w35ith acetate. J. Agric. Food Chem. 67, 12002–12012. doi: 10.1021/acs.jafc.9b05095
Keywords: ethanol, GRE3, lignocellulosic hydrolysates, Saccharomyces cerevisiae, xylose
Citation: Wang H, Cao L, Li Q, Wijayawardene NN, Zhao J, Cheng M, Li Q-R, Li X, Promputtha I and Kang Y-Q (2022) Overexpressing GRE3 in Saccharomyces cerevisiae enables high ethanol production from different lignocellulose hydrolysates. Front. Microbiol. 13:1085114. doi: 10.3389/fmicb.2022.1085114
Edited by:
Gen Zou, Shanghai Academy of Agricultural Sciences, ChinaReviewed by:
Nilesh Kumar Sharma, Worcester Polytechnic Institute, United StatesBhagwan Narayan Rekadwad, Yenepoya University, India
Changbin Chen, Chinese Academy of Sciences, China
Copyright © 2022 Wang, Cao, Li, Wijayawardene, Zhao, Cheng, Li, Li, Promputtha and Kang. This is an open-access article distributed under the terms of the Creative Commons Attribution License (CC BY). The use, distribution or reproduction in other forums is permitted, provided the original author(s) and the copyright owner(s) are credited and that the original publication in this journal is cited, in accordance with accepted academic practice. No use, distribution or reproduction is permitted which does not comply with these terms.
*Correspondence: Ying-Qian Kang, a2FuZ3lpbmdxaWFuQGdtYy5lZHUuY24=
†These authors have contributed equally to this work