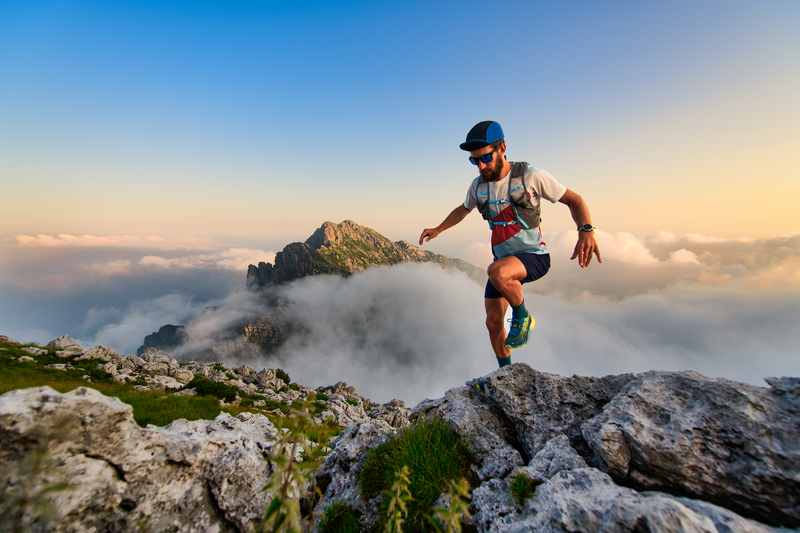
94% of researchers rate our articles as excellent or good
Learn more about the work of our research integrity team to safeguard the quality of each article we publish.
Find out more
REVIEW article
Front. Microbiol. , 28 November 2022
Sec. Virology
Volume 13 - 2022 | https://doi.org/10.3389/fmicb.2022.1065894
Lumpy skin disease is caused by lumpy skin disease virus (LSDV), which can induce cattle with high fever and extensive nodules on the mucosa or the scarfskin, seriously influencing the cattle industry development and international import and export trade. Since 2013, the disease has spread rapidly and widely throughout the Russia and Asia. In the past few decades, progress has been made in the study of LSDV. It is mainly transmitted by blood-sucking insects, and various modes of transmission with distinct seasonality. Figuring out how the virus spreads will help eradicate LSDV at its source. In the event of an outbreak, selecting the most effective vaccine to block and eliminate the threat posed by LSDV in a timely manner is the main choice for farmers and authorities. At present, a variety of vaccines for LSDV have been developed. The available vaccine products vary in quality, protection rate, safety and side effects. Early detection of LSDV can help reduce the cost of disease. In addition, because LSDV has a huge genome, it is currently also used as a vaccine carrier, forming a new complex with other viral genes through homologous recombination. The vaccine prepared based on this can have a certain preventive effect on many kinds of diseases. Clinical detection of disease including nucleic acid and antigen level. Each method varies in convenience, accuracy, cost, time and complexity of equipment. This article reviews our current understanding of the mode of transmission of LSDV and advances in vaccine types and detection methods, providing a background for further research into various aspects of LSDV in the future.
Lumpy skin disease (LSD) is an emerging viral transboundary disease which can spread beyond the outbreak area and become epidemic (Jordi et al., 2018; K, 2014; K et al., 2021). The most common clinical symptoms are nodular lesions on the surface of the skin and mucous membranes. Skin nodule lesions often appear on the outside of infected cattle, such as head, neck, back, perineum, breast, and other areas of the cattle (Molla et al., 2017). The affected cattle have varying degrees of edema and lameness in their legs (Salib and Osman, 2011). In vivo, they often present with mucosal ulcerations high fever and enlarged lymph nodes (Moyer et al., 2000). It is often manifested as mucopurulent nasal discharge (Lubinga et al., 2015; Elhaig et al., 2017). But this is not the characteristic clinical symptom of LSD. Although a large majority of the affected cattle could recover after a long period of illness, they will have long-term symptoms of mastitis, pneumonia, and deep holes in the hide (Selim et al., 2021a). As it is a fulminating infectious disease, the World Organization for Animal Health (OIE) stipulates LSD is the communicable disease that must be reported. LSDV can spread in many ways, such as indirect contact transmission between animals through vectors, lactation spread, blooding feeding insects, semen spread and iatrogenic transmission (Weiss, 1968; Carn and Kitching, 1995; Mullen and Durden, 2002; Annandale et al., 2010; European Food Safety Authority, 2018). Some researchers have conducted experiments to confirm that the disease is difficult to spread through direct contact (Carn and Kitching, 1995; Magori-Cohen et al., 2012; Mulatu and Feyisa, 2018). LSD was first reported in Zambia, South Africa in 1929 (Rhodesia, 1932). During the past decades, LSDV has spread widely and rapidly throughout the North Africa, Middle Eastern, Asia and other areas of the world, seriously influence the development of the cattle and water buffalo industry (Rgbe, 2014). Among them, the cattle with fine-skinned such as Holstein-Friesian and Jersey are the most susceptible to the virus. However, thick-skinned Bos indicus breeds including the Afrikander show less severe signs of the disease (Coetzer, 2004).
However, there are fewer effective preventive measures for LSD. Restricting the movement of the sick cattle, quarantine, sacrifice the cattle infected with LSDV are heavily recommended (Wolff et al., 2020b). Control and prevention of LSD in the countries like Albania, Bulgaria, Greece, Montenegro, FYROM, Serbia, Ethiopia relies extremely on vaccination (Gari et al., 2015; Klement et al., 2020). Because the LSDV has an intricate immune escape mechanism, no safe and efficient vaccines have been developed for this disease till now. Sheeppox virus (SPPV) and goatpox virus (GTPV) have antigenic homology and cross protection with LSDV; therefore, the vaccines of these two viruses can be used to prevented the LSD. Inevitably, the above two vaccines may have some potential risks because they are live-attenuated vaccines that derived from strains isolated in the field (Tuppurainen et al., 2014; Liu L. et al., 2021a). It is not recommended to use in the disease-free areas.
The diagnostic measures for LSD are mainly aimed at its nucleic acid sequence or corresponding antigen and antibody (Ireland and Binepal, 1998). The accuracy of each diagnostic method varies in a variety of occasions.
The aim of this study is to summarize the research progress of LSDV transmission modes, the types of vaccines used, and detection methods, and to sort out the characteristics of each vaccine and detection method. It will provide a reference for cutting off the spread of diseases, research on safe and efficient vaccines and the development of efficient and fast detection methods.
LSD is a viral contagious cattle disease caused by Lumpy skin disease virus (LSDV; Murphy et al., 2008). The virus is a large linear double-stranded DNA genomes of 151 kb and belongs to one of the Capripoxvirus genus, subfamily Chordopoxvirniae, family Poxviridae (Tulman et al., 2001; Bhanuprakash et al., 2006; Moss, 2007; K, 2014). Viruses of the Poxviridae family are very similar in morphological characteristics (Mcfadden, 2005). Since the researchers have not yet resolved the particle pattern diagram of LSDV, we draw the prediction diagram of LSDV structural pattern based on the pattern diagram of poxvirus for reference (Figure 1). The Capripoxvirus genus consists of SPPV, GTPV and LSDV (Tulman et al., 2001; Zare, 2010). These three viruses could cause transboundary disease with serious consequences among the ruminants, causing a major threat to the global animal husbandry (Sprygin et al., 2018a). They all have their own specific natural reservoir. The main hosts of the first two viruses are sheep and goat, while the LSDV mainly affects the cattle and water buffalo (Afonso et al., 2012; Fagbo et al., 2014; Lefkowitz et al., 2018). In addition, LSDV can also infect giraffes, impalas, and wildebeest (Young et al., 1970; Dao et al., 2022). Capripoxvirus genus is the most harmfully significant in the Poxviridae family affecting domestic ruminants in Africa and Asia. LSDV has more than 97% nucleotide sequence homology with GTPV (Gershon et al., 1989a; Upton, 2004). It is generally acknowledged that, the original pox virus may have originated from one or more basic species. They adapted by spreading the disease among the different kind of susceptible animals (Seet et al., 2003; Sohier et al., 2019). Homologous recombination is the key towards the evolution of the virion. A lot of viruses may have evolved from a common ancestor through genetic recombination within the virus itself to expand their host range and virulence (Gershon et al., 1989b). As a result, poxviruses of various animals were formed. The morphology, structure, biochemistry, and antigenicity of mammalian poxviruses in each genus are similar with each other. After infecting cells with LSDV in the 1960s, Alexander et al. (1957) and Plowright and Witcomb (1959) observed that the inclusion bodies produced in the cytoplasm were highly similar to those produced by other members of the Poxviridae family. Later, Munz and Owen (1966) observed that the morphological structure of LSDV and vaccinia virus was also very similar under the electron microscope. In recent years, researchers have observed the appearance of LSDV under the electron microscope, which is indeed similar to the appearance of other virus members in the Poxviridae that have been published (Sanz-Bernardo et al., 2020). The length of the virus genome is 151 kb, which consists of a central coding area and a 2.4 kb inverted terminal repeat sequence on both wings. According to the scientific prediction, LSDV has 156 putative genes. It has nine more genes than the other two viruses in the genus. Its morphological characteristics are similar to poxvirus, about 350 nanometers in length and 300 nanometers in width, with envelopes, but no clotting activity. This virus can be proliferated in primary cells, such as lamb and calf kidney or testicular cells, sheep embryonic kidney and lung cells, and chicken embryo fibroblasts. It also can multiply in Madin-Darby bovine kidney cells and baby hamster kidney cells (BHK-21), but the pathological changes are slower. Recent investigations have found that LSDV can hardly proliferate in African green monkey kidney (Vero) cells (Wang et al., 2022). Kumar et al. (2021) treated Vero cells with the product of virus amplification from primary goat kidney (PGK) cells, and it could obtain higher viral titer only after adapting to LSDV and continuing to pass generations. Higher virus titers can be produced in Vero which are cell adapted LSDV (Kumar et al., 2021). This also has new implications for the production of vaccines. In some experiments that need to ensure virulence stability, for example, the construction of recombinant viruses, we choose to perform in Vero cells. LSDV can proliferate on the chorioallantoic membrane of chicken embryo and cause acne-spots, and the virus does not cause the death of the embryo (Black et al., 1986; Upton, 2004; Kumar et al., 2021). The next year, Chinese scientists found that LSDV could produce higher viral titers in primary cattle testicular cells (Wang et al., 2022). When the wild-type virus was attenuated to prepare the vaccine strains, the state of the chicken embryo can be observed as a reference. Because it is a double stranded DNA virus, it has a certain thermal stability. Research shows that LSDV can be completely inactivated at 56°C, making it lose its infectivity (Wolff et al., 2020a).
Figure 1. The prediction diagram of LSDV structure mode. Mature virion of LSDV (MV), sometimes mature virion is surrounded by a lipid membrane derived from the endoplasmic reticulum (EV). The surface of the virus is envelope, which contains some entry-fusion complex. The virus contains lateral body, capsid and core. The surface of the EV has many surface microtubules.
In 1929, LSD was found in the Zambia, Southern Africa, then it spread north to the Middle East (Weiss, 1968). By the 1940s, the disease had spread across the southern African countries, affecting plenty of livestock. During the following decades, LSD transferred slowly northwards, and it is currently present throughout virtually the entire African continent, including Madagascar. Libya, Algeria, Morocco and Tunisia are the only African countries unaffected by the disease. The first LSD outbreak in Egypt was reported in May 1988 (Ali et al., 1990). In 1989 there was an LSD outbreak in Israel (Yeruham et al., 1994). This outbreak was the first instance of LSD north of the Sahara Desert and outside of the African continent. After the year 2000, more and more outbreaks were reported by Middle Eastern countries and currently LSD is considered as an endemic disease in the region. At the end of 2013, the disease invaded into Turkey and Iraq. Incursion the disease into Iran and Azerbaijan was reported in the year after that. In the late 2014, the first LSDV cases were reported in the northern part of an island in the eastern Mediterranean, Cyprus. Turkey serves as an important crossroads connecting the Eurasian continent, facilitated the spread of LSD to the Balkans and some parts of the European countries. And it eventually spread to the northeast countries in Asia. Spread to the Russian Federation in 2015, followed by Kazakhstan in 2016. Then it was spread into Yi li, Xinjiang Province, China in 2019. In the following 2 years, the disease spread to southern and eastern parts of China and the countries in South Asia, including Nepal, Bhutan, Vietnam, Thailand and Myanmar (Pseudo-urticaria, 1931; Mulatu and Feyisa, 2018; Arjkumpa et al., 2021; Punyapornwithaya et al., 2022). By 2022, the disease had spread east and north to Mongolia and Eastern Siberia (Figure 2).
Figure 2. The disease originated in Africa and subsequently spread to European and Asian countries. The transmission route is based on the time and location of LSD which reported by the OIE in the past two decades. https://wahis.woah.org/#/dashboards/country-or-disease-dashboard/ (accessed 26 September 2022).
In early Africa, LSD may be widely spread due to long-distance migration of cattle. There is plenty of experimental data to support that LSDV is transmitted through the arthropods such as mosquitoes or midges, and the hematophagus such as hard ticks (Burdin and Prydie, 1959; Carn and Kitching, 1995; Tuppurainen et al., 2011; El-Ansary et al., 2022). The latter are the main vector of the virus. Amblyomma hebraeum ticks can transmit the virus by the mechanical/intrastadial and transstadial transmission modes (Lubinga et al., 2015). LSDV can live in Aedes aegypti female mosquitoes for a minimum of 2 to 8 days and infect other healthy cattle by themselves (Chihota et al., 2001; Sanz-Bernardo et al., 2021). In addition to the blood-borne virus transmitted by tick bites, it can also be taken by the female insects of Amblyomma hebraeum and Rhipicephalus appendiculatus passing through their eggs (Jongejan et al., 1980; Lubinga et al., 2014). The virus DNA can be detected in blood samples and nodular lesion area near the skin of susceptible cattle bitten by the larva of the Rhipicehalus decoloratus ticks. The larvae came from female ticks that had fed the blood from experimentally infected donors, and then the healthy experimentally cattle bitten by these small worms have mild symptoms typical of LSD (Tuppurainen et al., 2013). LSDV can protect itself from being destroyed by the wintering habits of the individual tick species (Bryson et al., 2002). LSDV can be transmitted by Stomoxys calcitrans and Haematopota spp., which are tiny blood-sucking insects (Sohier et al., 2019; Issimov et al., 2020). They are the most probably LSDV transmission vectors (Gubbins, 2019; Sanz-Bernardo et al., 2021). No direct studies have shown that LSDV can further multiply in vectors, but the basic reproductive number of LSDV in hosts varies greatly after the virus transmitted by different vectors reaches susceptible animals. Stomoxys Calcitrans has the highest reproductive number of 19.1, while Aedes aegypti has the lowest reproductive number of 2.4. That’s nearly eight-fold difference. However, it has been suggested that LSDV can survive in vitro culture of tick cell lines for 35 days without loss of infectivity (Tuppurainen et al., 2015). In addition to the bite of ticks, the bite of some species of mosquitoes can transmit the virus too. The latest British study confirmed that LSDV can exist in the mouthparts of four blood sucking insects including Stomoxys calcitrans, Aedes aegypti, Culex quinquefasciatus, and Cubicoides nubeculosus, for about 9 days, and then spread the disease by biting healthy cattle. This is the main way for mosquitoes to transmit LSDV (Sanz-Bernardo et al., 2022). According to early investigation in South Africa, it also can be spread by the direct contact, but at a significantly lower transmission rates and efficiency (Mulatu and Feyisa, 2018). Due to the limitation of tick’s mobility, flies, which are good at flying, have become one of the most harmful arthropod pests to the cattle worldwide (Gubbins, 2019). During the dry and cold seasons, the rate of this disease infection drops obviously, however, in the warm and wet period, the rate increases, which is closely related to the plummeting insect population and mobility. There is no significant association between sex or different cattle populations and seroprevalence of LSD infection. Furthermore, the variety of cattle, age, season, water supply and feeding system, introduction of breeding stock, and exposure to other species such as birds and insects all play important role in the occurrence of LSDV infection of LSD (Burdin and Prydie, 1959; Selim et al., 2021b). In addition to the direct contact and bites from blood-sucking insects, close-range transmission may also occur through LSDV-contaminated medical devices (Ali et al., 2012). Some viruses of the Poxviridae family can be transmitted through aerosols (Aleksandr et al., 2020). As a member of it, LSDV has also been reported that it may spread to other areas through air transport (Klausner et al., 2017). This is the cause of repeated LSD outbreaks in some countries and regions in the Middle East. It is also possible that the blood-sucking insects travel long distances with the help of air currents (Greenberg et al., 2012). But there is little chance of it spreading further into Russia and parts of Europe (Klausner et al., 2017; Saegerman et al., 2018). When sick cattle which carrying LSDV share a food tank with the healthy cattle to drink water or feed troughs, the healthy cattle will have typical symptoms of this disease (Ali et al., 2012). Researchers have pointed out that LSDV is difficult to spread through direct contact between cattle (Weiss, 1968; Carn and Kitching, 1995; Mulatu and Feyisa, 2018). Aleksandr et al. (2020) found that LSDV could be transmitted without the presence of flying insects and ticks. They speculated that it might be the contact between the skin and mucous membrane of healthy cattle and infected cattle that caused the transmission of the virus. The complexity of communication has not been fully analyzed, which can be used as a future research direction. Researchers has confirmed that it should be caused by the polluted snot and saliva of the sick livestock. The reason why the cattle with symptoms are different is that the virulence level of the virus is low, and the symptoms will be more severe if they come into contact with more viruses, while eating the food with less virus, they will show mild fever, the surface of skin does not even appear nodular lesions (Dietze et al., 2018). In another study, the experimenters reported that the viral loads in oral and nasal mucosa are comparable to those in skin nodules. The virus is most likely to be found in droplets and aerosols formed by the infected cattle and spread further by air currents. Therefore, saliva and nasal swabs can be a more convenient sampling method for the detection of this disease (Babiuk et al., 2008; Dietze et al., 2018). Even though experiments have shown that LSDV may be transmitted vertically from mother to offspring through the uterus (Şevik and Doğan, 2017). However, there were wounds on the surface of the born calf, which did not rule out the infection of the virus after birth. So the conclusion of vertical transmission needs to be verified (Rouby et al., 2016). Vaccinated cows could detect vaccine virus shedding in secreted milk (Bedeković et al., 2018). Therefore, vaccinated cows cannot breastfeed during the withdrawal period. An earlier study had confirmed that the LSDV in bovine semen for a long-term excretion. The experimental animals in this study, including azoospermic or severely oligozoospermic bull, can also detect the nucleic acid of LSDV by PCR, indicating that LSDV may exist in other body fluids than the semen fraction (Osuagwuh et al., 2007; Figure 3). Even if the clinical signs of the bulls are not obvious, they will continue to expel LSDV to the outside environment (Irons et al., 2005; Annandale et al., 2014). The testicles and lymph nodes of infected cattle can carry the virus, which can spread the disease. If these unsterilized animal products are transported over long distance by plane or truck, or live cattle with asymptomatic infection, or even infected cattle with obvious symptoms, as mentioned above, the disease can spread to other countries and regions (Kononov et al., 2019; Table 1). Recently, a new technology was developed to forecast the incidence of LSDV infection by assessing meteorological and geological attributes (Afshari Safavi, 2022). If this technology can be improved to predict and prevent the infection with antiparasitic drugs or vaccines in time, the losses from infection can be greatly reduced.
Figure 3. Diagram of the transmission modes of lumpy skin disease. This picture shows the propagation mode of LSDV more intuitively. Each infection mode corresponds to the one which was introduced in the article.
Table 1. The modes of transmission of lumpy skin disease and the summary of the countries and regions.
The diseased cattle infected with LSD showed some clinical symptoms that could affect their health with naked eyes, such as edema of skin mucosa, decrease milk yield of cows, enlargement of lymph nodes, nodular lesions of different sizes on the skin surface, lameness of legs, etc. (Awad et al., 2010; Salib and Osman, 2011; Molla et al., 2017; Okur-Gumusova et al., 2020). The pathological changes of organs and tissues caused by LSDV infection in their bodies also affect their health.
Studies have shown that most of the organs and tissues of infected animals have pathological changes such as orchitis, cow mastitis, necrotic hepatitis, disseminated vasculitis, lymphadenitis, etc. A small number of cattle are accompanied by tracheitis, myocardial damage and other pathological changes, and can produce different intensity of injury induction in the affected animals, making LSDV more aggressive to the body (Ali et al., 2021). Ahmed et al. found in the clinical trial that the imbalance of oxidation antioxidation status in diseased cattle resulted in excessive increase of proinflammatory cytokine content and adverse effects on animals. Subsequently, the accumulation of metabolites in the liver, kidney and heart makes the organ function impaired, which leads to the occurrence of hypophosphatemia, and further aggravates the symptoms of hemolytic anemia (Ahmed and Dessouki, 2013). Abutarbush (2015) and Jalali et al. (2017) carried out the hematological and biochemical effect of LSD, the results showed that the blood of the affected cattle had pancytopenia, hyperproteinemia, hyperkalemia, hyperchloremia, and reduced creatinine concentration. It can be used as an index to evaluate the severity of the disease and to judge the prognosis.
Severe nodular lesions on the body surface of the cattle will cause holes in the skin, exposing the wound to the air. Affected cattle lack the effective protection of the first line of defense, and are prone to secondary infection with other bacterial or viral diseases, which may directly lead to their death. For susceptible cattle, timely prevention of disease and disinfection of diseased parts should be handled in place. Studies have shown that some nucleoside and nucleotide analogues can be used as anti-poxviruses drugs (De Clercq and Neyts, 2004). In the future, specific anti LSDV drugs should be developed. Under the premise of vaccination, drug assisted prevention will achieve better effects against the epidemic.
As mentioned above, LSDV mainly infects cattle and water buffalo, but also has been reported to infect wildebeest, impala and giraffe (Young et al., 1970; Dao et al., 2022). After all, the number of infections is very small compared with the first two. As the main host animal of LSDV, cattle and buffalo have different susceptibility to this virus.
House et al. (1990) pointed out in an investigation report that all sheep, goats and buffaloes survived the LSD outbreak in Suez and Ismalia, showing no clinical symptoms. In recent years, there is also experimental evidence that buffaloes have low susceptibility to this virus (Neamat-Allah and Mahmoud, 2019). Researchers speculate that the reason is that buffalo have thick skin, and the mouthparts of blood sucking insects such as mosquitoes, flies and ticks cannot easily pass through the skin of buffalo, so the transmission rate and susceptibility of this disease are low (Chihota et al., 2003; Neamat-Allah and Mahmoud, 2019). It is also possible that because buffalo have been living in the pond for a long time, their skin is exposed to the air for a much shorter time than other breeds of cattle. This makes it difficult for blood sucking insects to touch their skin, resulting in a lower susceptibility to LSD. Barnard (1997) detected that there was no LSD antibody in wild buffaloes in South Africa, which may also indicate that buffaloes are not sensitive to LSD. However, the number of subjects is not large enough, the reliability of this study remains to be discussed. Some clinical trials also showed that buffalo inoculated with LSD vaccine could not effectively stimulate the body to produce anti LSD antibodies (Okur-Gumusova et al., 2020). This requires national veterinary authorities to timely and effectively assess the effectiveness of vaccines and develop vaccination strategies.
For cattle with strong resistance, such as buffalo, it may be able to resist the invasion of LSDV. The researchers said that this may be due to the insensitivity of buffalo to LSDV, which is only its non-adapted host. It may also be caused by the life habits of buffalo and the structure of their skin is different from that of ordinary domestic cattle. They also found that there was capripoxviruses in the buffaloes of the test group, which may be the symptom caused by other viruses rather than LSDV, making the laboratory staff not accurate enough to detect the content of LSDV antibody (Elhaig et al., 2017). Therefore, the future research direction should be to confirm the true pathogen in buffaloes with LSDV symptoms. If LSDV is indeed the culprit, scientists need to develop LSDV vaccines that specifically target buffalo and can induce high-level antibodies.
The prevention and elimination of infectious diseases ultimately depend on the largescale use of corresponding vaccines. Smallpox virus, a member of Poxviridae family that damages human life and health, has been eradicated in the last century after high-density mass inoculation of live vaccinia vaccines (Bhanuprakash et al., 2012). LSD is no exception as an infectious disease that seriously endangers the development of cattle industry (Wolff et al., 2020b). As far as the development of vaccines is concerned, it has roughly gone through live attenuated vaccines, inactivated vaccines, recombinant vaccines, combined vaccines, genetic engineering vaccines (Francis, 2018; Wang et al., 2020). The various vaccines that are developed by scientists over the years have their own characteristics, advantages and disadvantages.
Live attenuated vaccines, also known as attenuated vaccines, refer to natural virulent strains of microorganisms that have lost or weakened their pathogenicity to the original host animal through physical, chemical or biological treatment, and have been continuously passaged and screened. Vaccines prepared from strains that maintain good immunogenicity and genetic characteristics, or attenuated strains selected and multiplied from nature and culture conditions with good immunogenicity (Gershon et al., 1984). However, the live vaccine itself also has limitations, such as clinical side-effects, the risk of detoxification, and the risk of contracting new diseases due to homologous recombination with other viruses of this genus (Lee et al., 2012; Sprygin et al., 2018b; Krotova et al., 2022). Therefore, it is not recommended to be used in the areas without this disease. Immunosuppression is a factor to be considered after vaccination with attenuated vaccine, and its consequences can lead to a weak immune response to the vaccinated vaccine, while increasing the risk of secondary infection (Harland et al., 1992).
As the most representative strain of LSDV, the Neethling strain in South Africa was originally known as a virus similar to vaccinia virus, and it was the real pathogen that caused the outbreaks in Botswana in 1943 and South Africa in 1945 and then it was purified and named Neethling type (Stephens, 1966; Hunter and Wallace, 2001). Weiss (1968) investigated in clinical trials that the live vaccine made of this strain attenuated could play a certain prevention role against LSD. Then Weiss serially passaged this strain in the challocyst membrane of chicken embryos, resulting in the attenuation of the virus virulence. By the 20th passage, the virus did not cause systemic rash or other typical symptoms, and only half of the inoculated cattle localized swelling at the site that resolves within the next 4 to 6 weeks without signs of necrosis. Some mild side effects from this vaccine are called Neethling disease. The disease was also reported after vaccinating cattle with Neethling strain (Yeruham et al., 1994). Those vaccinated with the live attenuated Neethling strain produced a local response and the antibodies in the cattle were maintained for more than 3 years, and both cattle were resistant to the virulent strain even in cattle without a local response. Field study in Israel in 2012 concluded that Neethling had a lower incidence of morbidity after vaccination (Ben-Gera et al., 2015). The use of passaging and attenuation methods should be appropriate. If the virulence is excessively weakened, the immune effect will be counterproductive. The Neethling vaccine produced in Ethiopia could not protect vaccinated cattle against the virus challenge in clinical trials, with a protection rate of only 30% (Gari et al., 2015). One survey on a dairy farm in Northern Greece showed that after inoculation of adult cows with the Neethling strain, swelling was seen in 12% of immunized cows, which then subsided. Small skin nodules less than 0.5 cm in 9% of them, not in calves. Mild viremia occurs in vaccinated herds, luckily this condition is of short duration (Katsoulos et al., 2018). According to the (European Food Safety Authority, 2017), in Croatia, less than 1% of the cattle were vaccinated with Neethling vaccine and had adverse reactions (Calistri et al., 2019). It has been reported that Ethiopian Neethling vaccine was not protective against the disease (Gari et al., 2015). A study carried out by Bedeković et al. (2018) found that the vaccine virus could be detected in milk from cows vaccinated with this vaccine strain. Adverse reactions may occur when using the Neethling vaccine. Therefore, vaccine efficacy and safety should be fully evaluated to achieve the desired immune effect.
Haegeman et al. (2021) conducted numerous clinical trials and compared LSDV homologous live attenuated vaccines including Lumpy Skin Disease Vaccine (South-Africa), Lumpyvax (South-Africa), Kenyavac (South-Africa), Herbivac LS (South-Africa) and Vaccin LSD Neethling O vivant (Morocco). The above vaccines could cause the body to have a fever, but none negatively affected feed intake and daily activities and general health in all groups. Swollen lymph nodes in the group receiving the Herbivac LS vaccine. The remaining three vaccines from South Africa showed symptoms of Neethling disease after being vaccinated. Small nodules developed in the group vaccinated with the Moroccan Neethling vaccine, not as large as those found in infected animals (Haegeman et al., 2021). Considering the aforementioned Greek study, the subjects of the two experiments are very different, so there is a certain deviation in the data of clinical symptoms (Katsoulos et al., 2018). After the first three vaccines were inoculated and challenged by virulent strains, the virus can be detected in the blood. It is considered that the virus is detected in the blood after being challenged with the virulent strains, which is not the true viremia. None virus was detected in the blood of the cattle which were vaccinated with the latter two vaccines. The pathogen of contagious bovine pleuropneumonia (CBPP) is Mycoplasma mycoides subsp. mycoides (Mmm). Safini et al. (2022) made a bivalent vaccine by attenuated this pathogen (strain obtained from CIRAD AF262936) and Neethling strain (ID: AF409138), which can induce inoculated cattle to produce high-level neutralizing antibodies against the two diseases without clinical adverse reactions. It is predicted that the vaccine can protect the two diseases. However, there is no challenge with virulent strains, and the future test direction should be inclined to verify the protection after the challenge of virulent strains.
LSDV shares more than 97% nucleic acid sequence homology with GTPV and SPPV (Tulman et al., 2001, 2002). Therefore, cross-immunization of goat pox or sheep pox live attenuated vaccine is usually used clinically to prevent LSD. Back in the 1990s, veterinarians in Egypt controlled outbreaks of LSD using a vaccine against a Romanian poxvirus strain (Ali et al., 1990). Gari et al. (2015) verified that a sheep and goat pox (KSGP) 0–180 strain vaccines prepared in Kenya did not provide LSDV protection in cattle (Gari et al., 2015). Brenner et al. (2009) developed a clinical response after re-exposure to LSDV infection in Yugoslav RM65-vaccinated cattle during an epidemic in 2006–2007. Bamouh et al. (2021) demonstrated that the KSGP O-180 and KSGP O-240 vaccine strains may have the problem of vaccine virus shedding, thereby infecting other unvaccinated or other healthy cattle. The Gorgan goatpox vaccine (Gorgan vaccines) developed by the Jordan Biological Center was used to prevent goat pox virus in the Middle East in 2010 (Abbas, 2010), and then Gari et al. (2015) used this vaccine to study against LSDV and found that it can significantly stimulate the cellular immune response of vaccinated cattle, proved that the vaccine is highly immunogenic against LSDV. For two decades from 1989 to 2009, the Israeli authorities had used the RM-65 vaccine strain to control LSD and sheeppox, but the vaccine did not eliminate both diseases (Brenner et al., 1992; Yeruham et al., 1995).
When live vaccines are used to protect animals against viral and bacterial infections, the exact nature of the genetic transformation that results in attenuation is unknown. Since attenuating mutations occur randomly, a single point mutation that causes a virulence return in animals will occur. These uncontrollable factors make the attenuated vaccine a time bomb that can be detonated at any time (Minor et al., 1986). The previous description mentioned that the effects of some vaccine strains in the last century and this century were significantly different, which may be due to the base pair mutation of the vaccine strains during the production process. The incidence of homologous recombination of double-strand DNA viruses is high, and the vaccine may not exert its original effect due to the enhanced virulence of the virus after inoculation and other viruses of the Poxviridae family. Therefore, in the clinical application of live attenuated vaccines, specific problems should be analyzed in detail, and more suitable vaccines should be selected according to the actual situation of the cows to be vaccinated (Table 2).
Inactivated vaccines refer to the inactivation of complete viruses (or bacteria) by physical, chemical and biological methods, so that the pathogens are sufficiently killed, infectivity or virulence is lost, and their immunogenicity is maintained. It has the advantages of low production cost, short development cycle and good usage effect. Compared with live attenuated vaccines, inactivated vaccines usually require booster immunization to prevent virus invasion (Bhanuprakash et al., 2004). Blackall (1988) reported in 1988 that the use of vaccine adjuvants can effectively enhance the effect of inactivated vaccines.
There are no reports of inactivated LSD vaccines circulating on the market. It was found that the use of Bi-ethylimine bromure (BEI) to inactivate the attenuated Neethling strain also provided good protection. A variety of antibodies can be detected and the virus neutralization test demonstrated that the antibody response rate of the inactivated vaccine was 37% higher than that of the live attenuated vaccine on the 28th day after vaccination (Hamdi et al., 2020). Y Es-sadeqy et al. developed a bivalent inactivated vaccine with oil adjuvants against the LSDV and Bluetongue virus (BTV) in 2020, and stimulated the production of high levels of neutralizing antibodies considered animals welfare and animal ethics, no challenge test to be conducted, so the specific clinical effect needs to be verified by further experiments (Es-Sadeqy et al., 2021). Wolff et al. (2020b) pointed out that different vaccine adjuvants can make inactivated vaccines more effective. The use of adjuvant A, whose main component is a low molecular weight copolymer, and adjuvant B is composed of amphotericin, Quil A and cholesterol, both adjuvants were used in the inactivated Neethling vaccine and Serbia vaccine have no adverse reactions. Adjuvant A can effectively stimulate the humoral immune response and the production of IFN-γ in vaccinated cattle, so it has become a clinically preferred adjuvant. Matsiela et al. (2022) inactivated Neethling strain with low concentration of BEI, used Montanide™ Gel-01 as vaccine adjuvant, and immunized rabbits to obtain a high level of neutralizing antibody. It has not been tested in cattle, and the new adjuvant developed can be used as a reference.
After the inactivated vaccine is injected into the animal, other proteins and antigen components in it will also induce the body to react. Therefore, in addition to interfering with the host’s immune response, it will also induce unwanted immune responses, which facilitates the antigen extraction process, optimizations and improvements (Stephens et al., 1984). One disadvantage of inactivated vaccines is that they generate a narrow immune spectrum, although they are excellent in inducing humoral immunity, they are rarely effective in inducing cell-mediated immune responses and mucosal immune responses. Therefore, it is still necessary to prepare a safer and more efficient inactivated vaccine against LSDV (Table 3).
Because the live attenuated vaccine can keep all relevant antigens in the vaccine, and the pathogen can replicate in the host, it can stimulate the host’s cellular immunity and humoral immunity, so it is considered to be the most ideal method. Unfortunately, traditional methods cannot attenuate all pathogens. As mentioned above, even if the virus is attenuated, virulence return may occur. In order to overcome these problems, some scientists had tried to identify the virulence genes of different pathogens, change the virulence of pathogens by directional mutation or deletion of these virulence genes, and achieve attenuated strain in a recombination way (Liang et al., 1991).
Deletion of the thymidine kinase and glycoprotein genes of herpes virus did not change its normal replication in vitro (Kitching et al., 1987). Then Romero et al. pointed out that the recombinant capripox virus vaccine expressing the rinderpest fusion protein gene was prepared by using homologous recombination to knock out the thymidine kinase gene of pox virus and then recombine with the fusion protein gene of rinderpest virus, which can effectively protect vaccinated cows from rinderpest and the threat of lumpy skin disease (Romero et al., 1993). Ten years later, Ngichabe et al. (2002) confirmed the reliability of the recombinant vaccine for rinderpest and goat pox through successive research results. A single vaccination protected cattle from rinderpest and lumpy skin disease virus for up to a year and the cattle in other experimental group can be protected for up to 3 years. Johnston and McFadden (2003) found that poxviruses can encode a homologue of interferon gamma to competitively block the binding of interferon gamma produced in the host to its natural receptor, thereby achieving the purpose of immune escape. Wendy et al. (2002) found that the aphthous virus gene can encode the production of interleukin-10-like, which subsequently produces immunosuppressive effects on host cells. On this basis, D. Kara et al. used homologous recombination technology to construct deletion of LSDV open reading frames 005 and 008, and then constructed recombinant vaccines, including LSDV-WB005KO and LSDV-WB008KO. Clinical trials after that, the aggregation of the two can effectively stimulate the neutralizing antibody level of the vaccinated cattle, which can effectively resist the invasion of LSDV. However, in the early stage of vaccination, there will be clinical reactions that are small and can be subsided (Kara et al., 2018). It was previously reported that LSDV-WB005KO also protected vaccinated animals from SPPV and GTPV (Boshra et al., 2015). Then the LSDV-WB005KO may be a better choice in clinical practice.
Recombinant poxviruses, like other vaccines, are concerned by regulatory authorities that vaccines made from recombinant viruses will also be released into the environment, posing a safety hazard to healthy animals. The solution to this problem is to study suicidal or non-replicating recombinant viruses. Graham and Prevec (1992) developed replication-deficient adenoviruses. These viruses can replicate in vitro in cells containing the E1 region, but cannot replicate in normal cells. Even if healthy animals are exposed to the virus, it is safe. In the following 2 years, Heffner and Peeters et al. reported that some herpes viruses must delete their glycoprotein genes to replicate in cell lines containing glycoprotein genes, but not in normal cells. The development in a direction of safe use also provides a new idea for the preparation of vaccines after recombination of LSDV with other viruses (Table 4; Heffner et al., 1993; Peeters et al., 1994).
Therapeutics that deliver DNA into the body to express the corresponding protein in some way is a long-term goal that will require the efforts of generations. Miller and Dusty (1992). published an article saying that from more difficult techniques such as transplantation of transfected cells (lymphocytes, myoblasts, hematopoietic stem cells), to more direct methods, such as the use of viral vectors (reverse transcription Viruses, adenoviruses, herpesviruses, parvoviruses) deliver DNA to target tissues in the body, thereby stimulating the activation of the body’s immune system. Poxviruses have been widely studied as vaccine vectors. Due to their large genome, vary in size from 130 to 375 kb (Holowczak, 1982). Such innate conditions allow them to tolerate the insertion of foreign genes of more than 25,000 base pairs. In the presence of a highly active promoter, the simultaneous expression of multiple exogenous genes can be achieved, and the humoral immunity and cellular immunity can be effectively activated (Zavala et al., 2001; Willey et al., 2003). In addition, it has a narrow host spectrum and is safe as a vaccine carrier (Hunter and Wallace, 2001). The virus is heat-resistant, which can reduce the cost of refrigerated storage. At the same time, a multivalent vaccine can prevent multiple diseases after injection, which is far safer and more cost-effective than multiple injections of a single vaccine to achieve the same effect of preventing multiple diseases (Prow et al., 2018).
Aspden et al. (2002) recombined the glycoprotein gene of rabies virus into LSDV as a vector (rLSDV-RG), this recombinant virus can stimulate the humoral immune response after clinical trials, the results show that 75% of cattle can resist rabies virus significantly stronger than the control group. Subsequently, the same author in 2003 published a paper confirming that the vaccination of non-ruminant animals such as rabbits and mice with this vaccine induce rabies virus-neutralizing antibodies twice as high as reported by the World Health Organization (WHO). The level of neutralizing antibodies produced after vaccination of the mice was comparable to that of the commercially available rabies vaccine (Aspden et al., 2003). Wallace and Viljoen (2005) found that two viral recombinants were constructed by using lumpy skin disease virus as a vector and the thymidine kinase gene located on it as the insertion site of foreign genes. They are the structural glycoprotein gene expressing Bovine Epizootic fever (LSDV-BEFV) and the two genes expressing Rift Valley fever glycoprotein (LSDV-RVFV). These two virus recombinants can induce the production of neutralizing antibodies in experimental animals. After LSDV-BEFV stimulation test, high levels of neutralizing antibodies can be stimulated. Mice inoculated with LSDV-RVFV are resistant to RVFV up to 100%.
The genes recombined with LSDV listed above are all from RNA viruses (Rabies virus, Bovine Epizootic fever virus, Rift Valley fever virus), so this other multivalent vaccine brings new ideas. At the same time, it also provides a reference for the development of vaccines for lumpy skin disease.
The on-site diagnosis of LSD often relies on clinical symptoms to determine whether the cattle are infected with the disease. However, in the early stages of the disease, affected cattle often show only fever and very few skin lesions, which greatly reduces the accuracy of the diagnosis. According to the researchers’ findings, the clinical symptoms of lumpy skin disease are similar to those of bovine herpesvirus infection, demodicosis, bovine viral diarrhea-mucosal disease and bovine malignant catarrhal fever (Reid et al., 1984; Deregt and Loewen, 1995; Reddy and Sivajothi, 2016). The presence of these factors complicates field diagnosis. Therefore, more accurate detection methods such as directly targeting the pathogen in the laboratory are needed (Perry, 2010). Sometimes after vaccination, animals still suffer from the disease. In consequence, judging whether animals were infected with the more virulent wild strain or the vaccine strain adversely affected the animals. Then it is particularly important to distinguish whether there is a wild virus or a vaccine strain in the animal (Chibssa et al., 2018; Fawzi et al., 2022).
Heine et al. (1999) found that SPPV and LSDV in the genus Capripoxvirus have a P32 gene with a nucleic acid sequence similarity of more than 98%. Two nucleotide site mutations in the P32 gene of LSDV lead to two EcoR V sites are missing. Therefore, the researchers used this idea for clinical testing, using specific primers B68 and B69 to amplify the P32 gene by polymerase chain reaction (PCR) in the viral samples collected from the field, followed by restriction endonuclease digestion susceptibility to determine whether the cattle infected with LSDV or SPPV. If this feature is used as a diagnostic method, more Capripoxviruses need to be sequenced to confirm that the EcoR V locus is specific for all SPPV. Babiuk et al. found that the presence of LSDV could be detected using real-time PCR. Compared with the oral and nasal mucosa, the detection rate of the diseased material in the skin nodule injury site was higher. This provides a reference for the collection of clinical patient materials in the future (Babiuk et al., 2008). In October of the same year, Stram et al. (2008) reported that they analyzed the non-homologous sequences of the three viruses (GTPV, SPPV and LSDV) in the genus Capripoxvirus, found a nucleic acid sequence that only exists in LSDV, and then designed PCR primers for this sequence to distinguish LSDV. A comparative experiment by Awad et al. (2010) in October of the following year showed that the detection rate of virus in the blood of infected cow skin biopsy specimens and infected animals by PCR can reach 100%, and the detection rate of virus in the blood of febrile cows can reach 77.8%. All were significantly higher than the virus detection rate of virus isolation, dot blot hybridization and indirect enzyme-linked immunosorbent assay (ELISA). Therefore, PCR method can be used as a fast and efficient tool for LSDV field infection diagnosis (Awad et al., 2010). In 2016, a portable, simple, and rapid method called recombinase polymerase amplification (RPA) assay for the field detection of the genome of LSDV (Shalaby et al., 2016). Yana et al. (2018) published a technique based on real-time high-resolution fusing PCR. The nucleic acid sequence of the viruses (SPPV, GTPV, LSDV) in the disease materials collected on site was amplified by PCR, and then the three viruses were distinguished according to the melting temperature of the generated amplicons. The gene that specifically targets LSDV is LSDV-ORF010, which has the unique species-specific nucleotide differences. Subsequently, Modise et al. (2021) found on this basis that the type of virus can be analyzed by the high-resolution melting (HRM) assay of PCR amplification products generated after genus-specific primers amplify sample viral DNA and bind dyes. Some farms do not have expensive and high-precision instruments, such as PCR machines, and the staff that on the farm may not have the skills to operate PCR machines, so a cheaper, convenient, reliable and easy-to-operate method is needed to replace PCR. Mwanandota et al. (2018) explored a novel method for the detection of LSDV, named loop-mediated isothermal amplification (LAMP). This method target the poly (A) polymerase small subunit (VP39) gene because of its higher detection rate and sensitivity. It is possible to detect extremely small amounts of nucleic acid substances present in the air with experimental error. Zeedan et al. (2019) compared PCR, real-time PCR (qPCR), fluorescent antibody technology (FAT), indirect FAT (iFAT) and indirect ELISA (iELISA) for the detection of LSDV and the positive rate of LSDV antibodies. In the skin disease material detection group, the detection rate of qPCR was better than that of conventional PCR, which could reach 39.13%. The virus detection rate of the FAT method was the lowest at 32.6%. All were higher than the antibody positive rate detected in blood. For the detection of LSDV antibodies in serum, iELISA was 3.45 percentage points higher than iFAT. This also confirms what was mentioned above, suggesting that when collecting disease materials on the spot, the skin nodule lesion area is preferentially collected as a test sample (Zeedan et al., 2019). Then, Haegeman et al. (2020) developed a novel high-sensitivity, high-specificity assay, the Immunoperoxidase Monolayer Assay (IPMA), which can detect LSDV antibodies early in vaccination and disease infection. This technology can be used in simple and crude environment detection, with high safety and can be processed in ordinary biosafety level laboratory. The same year, a novel study was reported by Milovanovic et al. (2020) using ELISA to detect LSDV-specific antibodies in milk. The advantage of this new technology is non-invasive sampling, which can collect a wide range of samples and can be used for large-scale screening. Sequence differences in three genes, RPO30, P32, and GPCR, were analyzed using single-nucleotide polymorphism using nanopore sequencing technology to build a database to distinguish GTPV, SPPV, and LSDV. The ease of replication of this database makes it more widely used. The advantages of this technology are that it is suitable for complex clinical environments, short detection time, and strong portability (Eltom et al., 2021). Lesions caused by LSDV in other species can also be used as a differential diagnosis method, for example, the isolation of the virus into embryonated chicken eggs (ECEs) can cause characteristic pitting lesions on the chorioallantoic membrane (El-Ansary et al., 2022). The pathological sections made from the skin lesions can be diagnosed by immunohistochemical (IHC) methods, and the distribution of pathogenic antigens can be detected by specific anti-LSDV antibodies. Changes in the dermis and epidermis of the skin after infection with the virus can be observed under the microscope, including watery degeneration, granulomatous reaction, dystrophic calcification of the dermis, and the formation of inflammatory cells (El-Neweshy et al., 2013; Sanz-Bernardo et al., 2020; Amin et al., 2021). Ali et al. observed inclusion bodies in the cytoplasm of bovine skin capsule through histopathological examination, and confirmed that these inclusion bodies were characteristic pathological lesions related to LSD. In recent years, some researchers confirmed this view in clinical tests (Ali et al., 1990; Ahmed and Dessouki, 2013; Neamat-Allah and Mahmoud, 2019). A new rapid diagnostic technique for LSDV-ORF068 gene targeting using recombinase polymerase amplification assay (RPA) combined with CRISPR-Cas12a-based fluorescence assay (RPA-Cas12a-fluorescence assay). It can be detected in trace amounts with excellent accuracy and sensitivity. There is no cross-reactivity with other common bovine viruses (Jiang et al., 2022). A rapid diagnostic tool of colorimetric sandwich-type lateral flow immunoassay (LFIA) was established using two monoclonal antibodies against different epitopes of P32 structural protein of LSDV and gold nanoparticles (Cavalera et al., 2022). The sensitivity of this new method is similar to that of ELISA, but it has not been widely used in clinical diagnosis, and its specificity needs to be determined after clinical trials. All the above methods require instruments and power equipment to detect. Korthase et al. (2022) invented a method of extracting nucleic acid without electricity, namely TripleE, which can extract nucleic acid from 8 samples within 10 min and ensure sensitivity. It can be applied to the place without good experimental conditions for diagnosis. For the diagnostic methods of detecting a specific gene to determine the type of virus, we need to screen a large number of viral nucleic acid types to ensure that the above-mentioned specific gene is not in the local affected cows with similar symptoms due to the homologous recombination or gene mutation (Table 5).
Different diagnostic methods can be selected according to the stage of the disease in clinical application. In the early stage of LSDV infection, when the clinical symptoms are not obvious, some highly sensitive detection methods can be used, such as nucleic acid level, antigen antibody level detection methods. Early diagnosis, detection and treatment can effectively prevent and treat in advance. When the clinical symptoms obviously, the characteristic pathological changes can be used for differential diagnosis.
Menasherow et al. (2014) found three methods to detect vaccine strains versus wild strains. First, the Neethling vaccine strain is 27 bases shorter than the Israeli virulent strain of the enveloped virions (EEV) gene, which can be distinguished by genetic sequencing based on this finding. The second method is to use primers to amplify the genome of the virus, and then use specific upstream and downstream primers to amplify by nested PCR, and determine the composition of the virus according to the difference in annealing temperature. The last method is to digest the amplicon of the PCR reaction according to the Mbo I enzyme cleavage site. The vaccine strain samples can be digested by Mbo I enzyme, but the virulent strain cannot be digested. Clinically, the second and third identification methods are more rapidly and widely used (Menasherow et al., 2014). Agianniotaki et al. (2017) developed a dual real-time PCR method in 2017, targeting GPCR genes, and the amplification efficiency of wild-type virus and the vaccine virus was 91.3% and 90.7%. According to the amplification efficiency of the sample to identify whether it is a wild-type virus or a vaccine virus. Several detection methods that have been researched and discovered so far need to analyze the obtained data to get the results. Möller et al. (2019) developed a new technology based on TaqMan probe-based independent double-stranded real-time qPCR (real-time quantitative PCR) detection method to distinguish virulent strains from vaccine strains with 100% analytical sensitivity and specificity. Also targeting the EEV gene, Agianniotaki et al. (2021) developed a novel surveillance tool, duplex real-time PCR, to specifically detect the presence of wild-type LSDV in samples containing high titer vaccine against LSDV. In the presence and absence of LSDV vaccine virus, the amplification efficiencies of virus samples were 99.0% and 98.6%. The experimenters set β-actin as an internal amplification control to increase the detection accuracy (Table 6).
Prevention and control should be carried out according to the local disease transmission mechanism and the living habits of species that are often used as transmission vectors. Eliminate those species in the right season to reduce their exposure to susceptible animals. Although there is substantial evidence of widespread vector-borne disease, LSD also spreads during periods when insects are inactive. Studies by Klausner et al. (2017) have reported that the virus may be transmitted through the atmosphere with aerosols, but it is not completely certain. Therefore, further investigation of the role of airflow on the transmission of LSDV is required. After the epidemic, there may be problems such as immune recessive infection and continuous detoxification, and it is necessary to further clarify and standardize the treatment of the same herd of cattle. High temperature fumigation can also effectively avoid the breeding of LSDV and eliminate the hotbed of virus.
For remote areas, it may not be financially feasible to fully vaccinate and eradicate disease, but vaccinated cattle should be permanently marked at least. These vaccinated and non-vaccinated cattle can be managed together, and using herd immunity to reduce the losses from outbreaks. At present, live attenuated vaccines are more commonly used. However, such live vaccines have the risk of homologous recombination with other viruses, causing the vaccinated animals to be infected with other new viruses. An inactivated vaccine targeting LSDV has not yet been developed. Researchers have developed a live attenuated vaccine that can be inactivated and used with adjuvants to achieve a good preventive effect, but it has not been widely used in clinical practice (Hamdi et al., 2020). For countries and regions without this disease, the problem of inactivated vaccines still needs to be solved urgently. There are no reports on subunit vaccines with higher safety profile. LSDV has been used in the laboratory as a vector for recombinant subunit vaccines for some diseases (Aspden et al., 2002; Wallace and Viljoen, 2005). It has been studied that the H3L gene of vaccinia virus is the main immunodominant envelope protein of the mature virus in cells, which can induce the production of neutralizing antibodies (Kumar et al., 2017). In addition, the LSDV14 gene is similar to the K3L gene of vaccinia virus and the M156 gene of myxoma virus, which inhibit the phosphorylation of protein kinases, thereby inhibiting the production of interferon (Beattie et al., 1995; Peng et al., 2016). These may prompt the production of safe and efficient vaccines in other ways. In order to overcome the risk of virulence reversion and homologous recombination of attenuated vaccines, the development of safer and more efficient recombinant vaccines may be the direction of LSDV vaccine development in the future. By inserting a gene at a specific location in the LSDV genome, it can replicate in a specific cell line, but not in the host, resulting in higher safety (Klupp et al., 1992; Heffner et al., 1993). Therefore, it is necessary to deeply study the pathogenic mechanism of LSDV, and explore the virulence genes and immunosuppressive genes, so that the pathogen can be weakened to different degrees through targeted mutation or deletion. Studies have shown that ivermectin (IVM) can inhibit the viral titer of LSDV and attenuate the transmission of LSDV (Toker et al., 2022). As an insect-borne disease, LSDV can be targeted to study whether other anti-parasitic drugs can be used in conjunction with vaccines to effectively block the spread of LSDV.
Currently used diagnostic methods with highly sensitivity, such as LAMP and RPA-Cas12a-fluorescence assay, are all targeting viral genes. However, these detection methods are easily affected by aerosol contamination, which affects the accuracy of the diagnostic results (Liu S. et al., 2021). Therefore, future research should focus on how to overcome the pollution caused by these aerosols.
Since 1929, LSD has endangered the healthy development of the global cattle industry for nearly a 100 years. Scientists are doing everything they can to eliminate this pathogen. The feasible prevention and diagnosis methods that have been developed need to be verified by a large number of clinical trials. Therefore, cutting off effective transmission routes, large-scale safe and efficient use of vaccines, and correct detection methods are the directions of efforts in the future. However, we still do not have a deep understanding of the pathogenic mechanism of this disease. This review highlighted the current research progress of this disease, and puts forward prospects for the insufficiency of research and future research trends, providing information for the elimination of the disease.
ZL, YS, XY and XW conceived and designed the study. ZL, KY, SW, JY, XM, YS, XY wrote the manuscript. All authors listed have made a substantial, direct, and intellectual contribution to the work and approved it for publication.
This study was supported by the grants from the Key Development and Research Foundation of Gansu (grant no. 21YF5WA153), Natural Science Foundation of Gansu Province (21JR7RA022), and Natural Science Foundation Project of China (grant no. 32202779).
We appreciate the other members of the Y. S. lab for their constructive comments.
The authors declare that the research was conducted in the absence of any commercial or financial relationships that could be construed as a potential conflict of interest.
All claims expressed in this article are solely those of the authors and do not necessarily represent those of their affiliated organizations, or those of the publisher, the editors and the reviewers. Any product that may be evaluated in this article, or claim that may be made by its manufacturer, is not guaranteed or endorsed by the publisher.
Abbas, F. (2010). Production of goat pox virus vaccine from a live attenuated goat pox virus strain. JAPS 20, 315–317.
Abutarbush, S. M. (2015). Hematological and serum biochemical findings in clinical cases of cattle naturally infected with lumpy skin disease. J. Infect. Dev. Ctries. 9, 283–288. doi: 10.3855/jidc.5038
Afonso, P. P., Silva, P. M., Schnellrath, L. C., Jesus, D. M., Hu, J., Yang, Y., et al. (2012). Biological characterization and next-generation genome sequencing of the unclassified Cotia virus SPAn232 (Poxviridae). J. Virol. 86, 5039–5054. doi: 10.1128/JVI.07162-11
Afshari Safavi, E. (2022). Assessing machine learning techniques in forecasting lumpy skin disease occurrence based on meteorological and geospatial features. Trop. Anim. Health Prod. 54:55. doi: 10.1007/s11250-022-03073-2
Agianniotaki, E. I., Chaintoutis, S. C., Haegeman, A., De Clercq, K., Chondrokouki, E., and Dovas, C. I. (2021). A Taq man probe-based multiplex real-time PCR method for the specific detection of wild type lumpy skin disease virus with beta-actin as internal amplification control. Mol. Cell. Probes 60:101778. doi: 10.1016/j.mcp.2021.101778
Agianniotaki, E. I., Chaintoutis, S. C., Haegeman, A., Tasioudi, K. E., De Leeuw, I., Katsoulos, P. D., et al. (2017). Development and validation of a Taq Man probe-based real-time PCR method for the differentiation of wild type lumpy skin disease virus from vaccine virus strains. J. Virol. Methods 249, 48–57. doi: 10.1016/j.jviromet.2017.08.011
Ahmed, A. M., and Dessouki, A. A. (2013). Abattoir-based survey and histopathological findings of lumpy skin disease in cattle at Ismailia abattoir. Int. J. Biosci. Biochem. Bioinformat. 3. doi: 10.7763/IJBBB.2013.V3.235
Aleksandr, K., Olga, B., David, W. B., Pavel, P., Yana, P., Svetlana, K., et al. (2020). Non-vector-borne transmission of lumpy skin disease virus. Sci. Rep. 10:7436. doi: 10.1038/s41598-020-64029-w
Alexander, R., Plowright, W., and Haig, D. (1957). Cytopathogenic agents associated with lumpy skin disease of cattle. Bull. Epizoot. Dis. Afr. 5, 489–492.
Ali, H., Ali, A. A., Atta, M. S., and Cepica, A. (2012). Common, eEmerging, vector-borne and infrequent abortogenic virus infections of cattle. Transbound. Emerg. Dis. 59, 11–25. doi: 10.1111/j.1865-1682.2011.01240.x
Ali, A. A., Esmat, M., Attia, H., Selim, A., and Abdel-Hamid, Y. M. (1990). Clinical and pathological studies on lumpy skin disease in Egypt. Vet. Rec. 127, 549–550.
Ali, A. A., Neamat-Allah, A. N. F., Sheire, H. A. E., and Mohamed, R. I. (2021). Prevalence, intensity, and impacts of non-cutaneous lesions of lumpy skin disease among some infected cattle flocks in Nile Delta governorates, Egypt. Comp. Clin. Path. 30, 693–700. doi: 10.1007/s00580-021-03264-7
Al-Salihi, K. A. (2014). Lumpy skin disease: review of the literature. Mirror Res. Vet. Sci. Ani. 3, 6–23.
Amin, D. M., Shehab, G., Emran, R., Hassanien, R. T., Alagmy, G. N., Hagag, N. M., et al. (2021). Diagnosis of naturally occurring lumpy skin disease virus infection in cattle using virological, molecular, and immunohistopathological assays. Vet. World 14, 2230–2237. doi: 10.14202/vetworld.2021.2230-2237
Annandale, C. H., Holm, D. E., Ebersohn, K., and Venter, E. H. (2014). Seminal transmission of lumpy skin disease virus in heifers. Transbound. Emerg. Dis. 61, 443–448. doi: 10.1111/tbed.12045
Annandale, C. H., Irons, P. C., Bagla, V. P., Osuagwuh, U. I., and Venter, E. H. (2010). Sites of persistence of lumpy skin disease virus in the genital tract of experimentally infected bulls. Reprod. Domest. Anim. 45, 250–255. doi: 10.1111/j.1439-0531.2008.01274.x
Arjkumpa, O., Suwannaboon, M., Boonrod, M., Punyawan, I., Liangchaisiri, S., Laobannue, P., et al. (2021). The first lumpy skin disease outbreak in Thailand (2021): epidemiological features and spatio-temporal analysis. Front. Vet. Sci. 8:799065. doi: 10.3389/fvets.2021.799065
Aspden, K., Dijk, A., Bingham, J., Cox, D., and Williamson, A. L. (2002). Immunogenicity of a recombinant lumpy skin disease virus (neethling vaccine strain) expressing the rabies virus glycoprotein in cattle. Vaccine 20, 2693–2701. doi: 10.1016/S0264-410X(02)00203-7
Aspden, K., Passmore, J. A., Tiedt, F., and Williamson, A. L. (2003). Evaluation of lumpy skin disease virus, a capripoxvirus, as a replication-deficient vaccine vector. J. Gen. Virol. 84, 1985–1996. doi: 10.1099/vir.0.19116-0
Awad, W. S., Ibrahim, A. K., Mahran, K., Fararh, K. M., and Abdel Moniem, M. I. (2010). Evaluation of different diagnostic methods for diagnosis of lumpy skin disease in cows. Trop. Anim. Health Prod. 42, 777–783. doi: 10.1007/s11250-009-9486-5
Babiuk, S., Bowden, T. R., Parkyn, G., Dalman, B., Manning, L., Neufeld, J., et al. (2008). Quantification of lumpy skin disease virus following experimental infection in cattle. Transbound. Emerg. Dis. 55, 299–307. doi: 10.1111/j.1865-1682.2008.01024.x
Bamouh, Z., Hamdi, J., Fellahi, S., Khayi, S., and Elharrak, M. (2021). Investigation of post vaccination reactions of two live attenuated vaccines against lumpy skin disease of cattle. Vaccine (Basel) 6, 5–17. doi: 10.3390/vaccines9060621
Barnard, B. J. (1997). Antibodies against some viruses of domestic animals in southern African wild animals. Onderstepoort J. Vet. Res. 64, 95–110.
Beattie, E., Paoletti, E., and Tartaglia, J. (1995). Distinct patterns of IFN sensitivity observed in cells infected with vaccinia K3L-and E3L-mutant viruses. Virology 210, 254–263. doi: 10.1006/viro.1995.1342
Bedeković, T., Šimić, I., Krešić, N., and Lojkić, I. (2018). Detection of lumpy skin disease virus in skin lesions, blood, nasal swabs and milk following preventive vaccination. Transbound. Emerg. Dis. 65, 491–496. doi: 10.1111/tbed.12730
Ben-Gera, J., Klement, E., Khinich, E., Stram, Y., and Shpigel, N. Y. (2015). Comparison of the efficacy of Neethling lumpy skin disease virus and x10RM65 sheep-pox live attenuated vaccines for the prevention of lumpy skin disease—the results of a randomized controlled field study. Vaccine 33, 4837–4842.
Bhanuprakash, V., Hosamani, M., Venkatesan, G., Balamurugan, V., Yogisharadhya, R., and Singh, R. K. (2012). Animal poxvirus vaccines: a comprehensive review. Expert Rev. Vaccines 11, 1355–1374. doi: 10.1586/erv.12.116
Bhanuprakash, V., Indrani, B. K., Hegde, R., Kumar, M. M., and Moorthy, A. (2004). A classical live attenuated vaccine for sheep pox. Trop. Anim. Health. Prod. 36, 307–320. doi: 10.1023/b:trop.0000026661.88631.50
Bhanuprakash, V., Indrani, B. K., Hosamani, M., and Singh, R. K. (2006). The current status of sheep pox disease. Comp. Immunol. Microbiol. Infect. Dis. 29, 27–60. doi: 10.1016/j.cimid.2005.12.001
Black, D. N., Hammond, J. M., and Kitching, R. P. (1986). Genomic relationship between capripoxviruses. Virus Res. 5, 277–292. doi: 10.1016/0168-1702(86)90024-9
Blackall, P. J. (1988). Further comparison of adjuvants for an inactivated infectious coryza vaccine. Avian Dis. 32.
Boshra, H., Truong, T., Nfon, C., Bowden, T. R., Gerdts, V., Tikoo, S., et al. (2015). A lumpy skin disease virus deficient of an IL-10 gene homologue provides protective immunity against virulent capripoxvirus challenge in sheep and goats. Antivir. Res. 123, 39–49. doi: 10.1016/j.antiviral.2015.08.016
Brenner, J., Bellaiche, M., Gross, E., Elad, D., Oved, Z., Haimovitz, M., et al. (2009). Appearance of skin lesions in cattle populations vaccinated against lumpy skin disease: statutory challenge. Vaccine 27, 1500–1503. doi: 10.1016/j.vaccine.2009.01.020
Brenner, J., David, D., Avraham, A., Klopferorgad, U., Samina, I., and Peleg, B.A. (1992). Experimental infection with local lumpy skin disease virus in cattle vaccinated with sheep pox vaccine.
Bryson, N. R., Tice, G. A., Horak, I. G., Stewart, C. G., and du Plessis, B. J. (2002). Ixodid ticks on cattle belonging to small-scale farmers at 4 communal grazing areas in South Africa. J. S. Afr. Vet. Assoc. 73, 98–103. doi: 10.4102/jsava.v73i3.568
Burdin, M., and Prydie, J. (1959). Observations on the first outbreak of lumpy skin disease in Kenya. Bull. Epizoot. Dis. Afr. 7, 21–26.
Calistri, P., Declercq, K., Gubbins, S., Klement, E., Stegeman, A., Abrahantes, J. C., et al. (2019). Lumpy skin disease: III. Data collection and analysis. EFSA J. 17. doi: 10.2903/j.efsa.2019.5638
Carn, V. M., and Kitching, R. P. (1995). An investigation of possible routes of transmission of lumpy skin disease virus (Neethling). Epidemiol. Infect. 114, 219–226. doi: 10.1017/S0950268800052067
Cavalera, S., Pezzoni, G., Grazioli, S., Brocchi, E., Baselli, S., Lelli, D., et al. (2022). Investigation of the “antigen hook effect,” in lateral flow Sandwich immunoassay: the case of lumpy skin disease virus detection. Biosensors (Basel) 12:739. doi: 10.3390/bios12090739
Chibssa, T. R., Grabherr, R., Loitsch, A., Settypalli, T. B. K., Tuppurainen, E., Nwankpa, N., et al. (2018). A gel-based PCR method to differentiate sheeppox virus field isolates from vaccine strains. Virol. J. 15:59. doi: 10.1186/s12985-018-0969-8
Chihota, C. M., Rennie, L. F., Kitching, R. P., and Mellor, P. S. (2003). Attempted mechanical transmission of lumpy skin disease virus by biting insects. Med. Vet. Entomol. 17, 294–300. doi: 10.1046/j.1365-2915.2003.00445.x
Chihota, C. M., Rennie, L. F., Kitching, R. P., and Mellor, P. S. (2001). Mechanical transmission of lumpy skin disease virus by Aedes aegypti (Diptera: Culicidae). Epidemiol. Infect. 126, 317–321.
Coetzer, J.A.W. (2004). Infectious diseases of livestock. Cape Town, South Africa: Oxford University Press pp. 1268–1276.
Dao, T. D., Tran, L. H., Nguyen, H. D., Hoang, T. T., Nguyen, G. H., Tran, K. V. D., et al. (2022). Characterization of lumpy skin disease virus isolated from a giraffe in Vietnam. Transbound. Emerg. Dis. e3268–e3271. doi: 10.1111/tbed.14583
De Clercq, E., and Neyts, J. (2004). Therapeutic potential of nucleoside/nucleotide analogues against poxvirus infections. Rev. Med. Virol. 14, 289–300. doi: 10.1002/rmv.439
Deregt, D., and Loewen, K. G. (1995). Bovine viral diarrhea virus: biotypes and disease. Can. Vet. J. 36, 371–378.
Dietze, K., Moritz, T., Alexandrov, T., Krstevski, K., Schlottau, K., Milovanovic, M., et al. (2018). Suitability of group-level oral fluid sampling in ruminant populations for lumpy skin disease virus detection. Vet. Microbiol. 221, 44–48. doi: 10.1016/j.vetmic.2018.05.022
El-Ansary, R. E., El-Dabae, W. H., Bream, A. S., and El Wakil, A. (2022). Isolation and molecular characterization of lumpy skin disease virus from hard ticks, Rhipicephalus (Boophilus) annulatus in Egypt. BMC Vet. Res. 18:302. doi: 10.1186/s12917-022-03398-y
Elhaig, M. M., Selim, A., and Mahmoud, M. (2017). Lumpy skin disease in cattle: frequency of occurrence in a dairy farm and a preliminary assessment of its possible impact on Egyptian buffaloes. Onderstepoort J. Vet. Res. 84, e1–e6. doi: 10.4102/ojvr.v84i1.1393
El-Neweshy, M. S., El-Shemey, T. M., and Youssef, S. A. (2013). Pathologic and immunohistochemical findings of natural lumpy skin disease in Egyptian cattle. Pak. Vet. J. 33, 60–64.
Eltom, K. H., Althoff, A. C., Hansen, S., Böhlken-Fascher, S., Yousif, A., El-Sheikh, H. A., et al. (2021). Differentiation of Capripox viruses by Nanopore sequencing. Vaccines (Basel) 9:351. doi: 10.3390/vaccines9040351
Es-Sadeqy, Y., Bamouh, Z., Ennahli, A., Safini, N., and Harrak, M. E. (2021). Development of an inactivated combined vaccine for protection of cattle against lumpy skin disease and bluetongue viruses. Vet. Microbiol. 256:109046. doi: 10.1016/j.vetmic.2021.109046
European Food Safety Authority (2017). Lumpy skin disease: I. Data collection and analysis. Efsa J 15:e04773. doi: 10.2903/j.efsa.2017.4773
European Food Safety Authority (2018). Lumpy skin disease II. Data collection and analysis. EFSA J. 16:e05176. doi: 10.2903/j.efsa.2018.5176
Fagbo, S., Coetzer, J. A., and Venter, E. H. (2014). Seroprevalence of Rift Valley fever and lumpy skin disease in African buffalo (Syncerus caffer) in the Kruger National Park and Hluhluwe-iMfolozi park, South Africa. J. S. Afr. Vet. Assoc. 85, e1–e7. doi: 10.4102/jsava.v85i1.1075
Fawzi, E. M., Morsi, A. M., and Abd-Elfatah, E. B. (2022). Molecular diagnosis of three outbreaks during three successive years (2018, 2019, and 2020) of lumpy skin disease virus in cattle in Sharkia governorate. Egypt. Open Vet J 12, 451–462. doi: 10.5455/OVJ.2022.v12.i4.6
Francis, M. J. (2018). Recent advances in vaccine technologies. Vet. Clin. North Am. Small Anim. Pract. 48, 231–241. doi: 10.1016/j.cvsm.2017.10.002
Gari, G., Abie, G., Gizaw, D., Wubete, A., Kidane, M., Asgedom, H., et al. (2015). Evaluation of the safety, immunogenicity and efficacy of three capripoxvirus vaccine strains against lumpy skin disease virus. Vaccine 33, 3256–3261. doi: 10.1016/j.vaccine.2015.01.035
Gershon, P. D., Ansell, D. M., and Black, D. N. (1989a). A comparison of the genome organization of capripoxvirus with that of the orthopoxviruses. J. Virol. 63, 4703–4708.
Gershon, P. D., Paul Kitching, R., Hammond, J. M., and Black, D. N. (1989b). Poxvirus genetic recombination during natural virus transmission. J. Gen. Virol. 70:485.
Gershon, A. A., Steinberg, S. P., Gelb, L., Galasso, G., Borkowsky, W., LaRussa, P., et al. (1984). Live attenuated varicella vaccine. Efficacy for children with leukemia in remission. JAMA 252:355.
Graham, F. L., and Prevec, L. (1992). Adenovirus-based expression vectors and recombinant vaccines. Biotechnology 20, 363–390.
Greenberg, J. A., DiMenna, M. A., Hanelt, B., and Hofkin, B. V. (2012). Analysis of post-blood meal flight distances in mosquitoes utilizing zoo animal blood meals. J. Vector Ecol. 37, 83–89. doi: 10.1111/j.1948-7134.2012.00203.x
Gubbins, S. (2019). Using the basic reproduction number to assess the risk of transmission of lumpy skin disease virus by biting insects. Transbound. Emerg. Dis. 66, 1873–1883. doi: 10.1111/tbed.13216
Haegeman, A., De Leeuw, I., Mostin, L., Van Campe, W., Aerts, L., Vastag, M., et al. (2020). An Immunoperoxidase monolayer assay (IPMA) for the detection of lumpy skin disease antibodies. J. Virol. Methods 277:113800. doi: 10.1016/j.jviromet.2019.113800
Haegeman, A., Leeuw, I. D., Mostin, L., Campe, W. V., and Clercq, K. D. (2021). Comparative evaluation of lumpy skin disease virus-based live attenuated vaccines. Vaccines (Basel) 9:473. doi: 10.3390/vaccines9050473
Hamdi, J., Boumart, Z., Daouam, S., Arkam, A. E., and Harrak, M. E. (2020). Development and evaluation of an inactivated lumpy skin disease vaccine for cattle. Vet. Microbiol. 245:108689. doi: 10.1016/j.vetmic.2020.108689
Harland, R. J., Potter, A. A., Donkersgoed, J. V., Parker, M. D., Zamb, T. J., and Janzen, E. D. (1992). The effect of subunit or modified live bovine herpesvirus-1 vaccines on the efficacy of a recombinant Pasteurella haemolytica vaccine for the prevention of respiratory disease in feedlot calves. Can. Vet. J. 33, 734–741.
Heffner, S., Kovacs, F., Klupp, B. G., and Mettenleiter, T. C. (1993). Glycoprotein gp 50-negative pseudorabies virus: a novel approach toward a nonspreading live herpesvirus vaccine. J. Virol. 67, 1529–1537. doi: 10.1128/jvi.67.3.1529-1537.1993
Heine, H. G., Stevens, M. P., Foord, A. J., and Boyle, D. B. (1999). A capripoxvirus detection PCR and antibody ELISA based on the major antigen P32, the homolog of the vaccinia virus H3L gene. J. Immunol. Methods. 187–196. doi: 10.1016/s0022-1759(99)00072-1
House, J. A., Wilson, T. M., el Nakashly, S., Karim, I. A., Ismail, I., el Danaf, N., et al. (1990). The isolation of lumpy skin disease virus and bovine herpesvirus-4 from cattle in Egypt. J. Vet. Diagn. Investig. 2, 111–115. doi: 10.1177/104063879000200205
Hunter, P., and Wallace, D. (2001). Lumpy skin disease in southern Africa: a review of the disease and aspects of control. J. S. Afr. Vet. Assoc. 72, 68–71. doi: 10.4102/jsava.v72i2.619
Ireland, D. C., and Binepal, Y. S. (1998). Improved detection of capripoxvirus in biopsy samples by PCR. J. Virol. Methods 74, 1–7.
Irons, P. C., Tuppurainen, E. S., and Venter, E. H. (2005). Excretion of lumpy skin disease virus in bull semen. Theriogenology 63, 1290–1297. doi: 10.1016/j.theriogenology.2004.06.013
Issimov, A., Kutumbetov, L., Orynbayev, M. B., Khairullin, B., Myrzakhmetova, B., Sultankulova, K., et al. (2020). Mechanical transmission of lumpy skin disease virus by Stomoxys Spp (Stomoxys calsitrans, Stomoxys sitiens, Stomoxys indica), Diptera: Muscidae. Animals (Basel) 10:477. doi: 10.3390/ani10030477
Jalali, S. M., Rasooli, A., Seifi Abad Shapuri, M., and Daneshi, M. (2017). Clinical, hematologic, and biochemical findings in cattle infected with lumpy skin disease during an outbreak in Southwest Iran. Arch. Razi Inst. 72, 255–265. doi: 10.22092/ari.2017.113301
Jiang, C., Tao, D., Geng, Y., Yang, H., Xu, B., Chen, Y., et al. (2022). Sensitive and specific detection of lumpy skin disease virus in cattle by CRISPR-Cas 12a fluorescent assay coupled with Recombinase polymerase amplification. Genes (Basel) 13:734. doi: 10.3390/genes13050734
Johnston, J. B., and McFadden, G. (2003). Poxvirus immunomodulatory strategies: current perspectives. J. Virol. 77, 6093–6100.
Jongejan, F., Perié, N., Franssen, F. F., and Uilenberg, G. (1980). Artificial infection of Rhipicephalus appendiculatus with Theileria parva by percutaneous injection. Res. Vet. Sci. 29, 320–324.
Jordi, C., Alberto, A., Aleksandra, M., Ledi, P., Blagojco, T., Dimitar, T., et al. (2018). Economic cost of lumpy skin disease outbreaks in three Balkan countries: Albania, Bulgaria and the former Yugoslav Republic of Macedonia (2016–2017). Transbound. Emerg. Dis. 65, 1680–1688. doi: 10.1111/tbed.12926
Kara, P. D., Mather, A. S., Alri, P., Thireshni, C., Shawn, B., and Wallace, D. B. (2018). Characterisation of putative immunomodulatory gene knockouts of lumpy skin disease virus in cattle towards an improved vaccine. Vaccine 36, 4708–4715. doi: 10.1016/j.vaccine.2018.06.017
Katsoulos, P. D., Chaintoutis, S. C., Dovas, C. I., Polizopoulou, Z. S., Brellou, G., Agianniotaki, E. I., et al. (2018). Investigation on the incidence of adverse reactions, viraemia and haematological changes following field immunization of cattle using a live attenuated vaccine against lumpy skin disease. Transbound. Emerg. Dis. 174–185. doi: 10.1111/tbed.12646
Kitching, R. P., Hammond, J. M., and Taylor, W. P. (1987). A single vaccine for the control of capripox infection in sheep and goats. Res. Vet. Sci. 42, 53–60.
K, L. K., B, S., and K, R. (2021). Clinico-molecular diagnosis and characterization of bovine lumpy skin disease virus in Andhra Pradesh, India. Trop. Anim. Health Prod. 53:424. doi: 10.1007/s11250-021-02872-3
Klausner, Z., Fattal, E., and Klement, E. (2017). Using synoptic Systems' typical wind trajectories for the analysis of potential atmospheric long-distance dispersal of lumpy skin disease virus. Transbound. Emerg. Dis. 64, 398–410. doi: 10.1111/tbed.12378
Klement, E., Broglia, A., Antoniou, S. E., Tsiamadis, V., Plevraki, E., Petrovic, T., et al. (2020). Neethling vaccine proved highly effective in controlling lumpy skin disease epidemics in the Balkans. Prev. Vet. Med. 181:104595. doi: 10.1016/j.prevetmed.2018.12.001
Klupp, B. G., Visser, N., and Mettenleiter, T. C. (1992). Identification and characterization of pseudorabies virus glycoprotein H. J. Virol. 66:3048.
Kononov, A., Prutnikov, P., Shumilova, I., Kononova, S., Nesterov, A., Byadovskaya, O., et al. (2019). Determination of lumpy skin disease virus in bovine meat and offal products following experimental infection. Transbound. Emerg. Dis. 66, 1332–1340. doi: 10.1111/tbed.13158
Korthase, C., Elnagar, A., Beer, M., and Hoffmann, B. (2022). Easy express extraction (triple E)-A universal, electricity-free nucleic acid extraction system for the lab and the pen. Microorganisms 10:1074. doi: 10.3390/microorganisms10051074
Krotova, A., Byadovskaya, O., Shumilova, I., van Schalkwyk, A., and Sprygin, A. (2022). An in-depth bioinformatic analysis of the novel recombinant lumpy skin disease virus strains: from unique patterns to established lineage. BMC Genomics 23:396. doi: 10.1186/s12864-022-08639-w
Kumar, N., Chander, Y., Kumar, R., Khandelwal, N., Riyesh, T., Chaudhary, K., et al. (2021). Isolation and characterization of lumpy skin disease virus from cattle in India. PLoS One 16:e0241022. doi: 10.1371/journal.pone.0241022
Kumar, A., Yogisharadhya, R., Venkatesan, G., Bhanuprakash, V., Pandey, A. B., and Shivachandra, S. B. (2017). Co-administration of recombinant major envelope proteins (rA27L and rH3L) of buffalopox virus provides enhanced immunogenicity and protective efficacy in animal models. Antivir. Res. 141, 174–178. doi: 10.1016/j.antiviral.2017.02.017
Lee, S. W., Markham, P. F., Coppo, M. J., Legione, A. R., Markham, J. F., Noormohammadi, A. H., et al. (2012). Attenuated vaccines can recombine to form virulent field viruses. Science 337:188. doi: 10.1126/science.1217134
Lefkowitz, E. J., Dempsey, D. M., Hendrickson, R. C., Orton, R. J., Siddell, S. G., and Smith, D. B. (2018). Virus taxonomy: the database of the international committee on taxonomy of viruses (ICTV). Nucleic Acids Res. 46, D708–D717. doi: 10.1093/nar/gkx932
Liang, X. P., Babiuk, L. A., Hurk, L., Fitzpatrick, D. R., and Zamb, T. J. (1991). Bovine herpesvirus 1 attachment to permissive cells is mediated by its major glycoproteins gI, gIII, and gIV. J. Virol. 65, 1124–1132. doi: 10.1128/jvi.65.3.1124-1132.1991
Liu, S., Tao, D., Liao, Y., Yang, Y., Sun, S., Zhao, Y., et al. (2021). Highly sensitive CRISPR/Cas12a-based fluorescence detection of porcine reproductive and respiratory syndrome virus. ACS Synth. Biol. 10, 2499–2507. doi: 10.1021/acssynbio.1c00103
Liu, L., Wang, X., Mao, R., Zhou, Y., Yin, J., Sun, Y., et al. (2021). Research progress on live attenuated vaccine against African swine fever virus. Microb. Pathog. 158:105024. doi: 10.1016/j.micpath.2021.105024
Lubinga, J. C., Tuppurainen, E. S., Coetzer, J. A., Stoltsz, W. H., and Venter, E. H. (2014). Transovarial passage and transmission of LSDV by Amblyomma hebraeum, Rhipicephalus appendiculatus and Rhipicephalus decoloratus. Exp. Appl. Acarol. 62, 67–75. doi: 10.1007/s10493-013-9722-6
Lubinga, J. C., Tuppurainen, E. S., Mahlare, R., Coetzer, J. A., Stoltsz, W. H., and Venter, E. H. (2015). Evidence of transstadial and mechanical transmission of lumpy skin disease virus by Amblyomma hebraeum ticks. Transbound. Emerg. Dis. 62, 174–182. doi: 10.1111/tbed.12102
Magori-Cohen, R., Louzoun, Y., Herziger, Y., Oron, E., Arazi, A., Tuppurainen, E., et al. (2012). Mathematical modelling and evaluation of the different routes of transmission of lumpy skin disease virus. Vet. Res. 43:1. doi: 10.1186/1297-9716-43-1
Matsiela, M. S., Naicker, L., Dibakwane, V. S., Ntombela, N., Khoza, T., and Mokoena, N. (2022). Improved safety profile of inactivated Neethling strain of the lumpy skin disease vaccine. Vaccine X 12:100209. doi: 10.1016/j.jvacx.2022.100209
Menasherow, S., Rubinstein-Giuni, M., Kovtunenko, A., Eyngor, Y., Fridgut, O., Rotenberg, D., et al. (2014). Development of an assay to differentiate between virulent and vaccine strains of lumpy skin disease virus (LSDV). J. Virol. Methods 199, 95–101. doi: 10.1016/j.jviromet.2013.12.013
Miller,, and Dusty, A. (1992). Human gene therapy comes of age. Nature 357, 455–460. doi: 10.1038/357455a0
Milovanovic, M., Milicevic, V., Radojicic, S., Valcic, M., Hoffmann, B., and Dietze, K. (2020). Suitability of individual and bulk milk samples to investigate the humoral immune response to lumpy skin disease vaccination by ELISA. Virol. J. 17:28. doi: 10.1186/s12985-020-01298-x
Minor, P. D., John, A., Ferguson, M., and Icenogle, J. P. (1986). Antigenic and molecular evolution of the vaccine strain of type 3 poliovirus during the period of excretion by a primary vaccinee. J. Gen. Virol. 67, 693–706. doi: 10.1099/0022-1317-67-4-693
Modise, B. M., Settypalli, T. B. K., Kgotlele, T., Xue, D., Ntesang, K., Kumile, K., et al. (2021). First molecular characterization of poxviruses in cattle, sheep, and goats in Botswana. Virol. J. 18:167. doi: 10.1186/s12985-021-01634-9
Molla, W., de Jong Mart, C. M., Gari, G., and Frankena, K. (2017). Economic impact of lumpy skin disease and cost effectiveness of vaccination for the control of outbreaks in Ethiopia. Prev. Vet. Med. 147, 100–107. doi: 10.1016/j.prevetmed.2017.09.003
Möller, J., Moritz, T., Schlottau, K., Krstevski, K., Hoffmann, D., Beer, M., et al. (2019). Experimental lumpy skin disease virus infection of cattle: comparison of a field strain and a vaccine strain. Arch. Virol. 164, 2931–2941. doi: 10.1007/s00705-019-04411-w
Moyer, R.W., Arif, B.M., Black, D.N., Boyle, D.B., Buller, R.M., Dumbell, K.R., et al. (2000). Family Poxviridae.
Mulatu, E., and Feyisa, A. (2018). Review: Lumpy Skin Disease. J. Vet. Sci. Technol. 09:3. doi: 10.4172/2157-7579.1000535
Mullen, G.R., and Durden, L.A. (2002). Medical and Veterinary Entomology. New York: Elsevier M, 15–27.
Munz, E. K., and Owen, N. C. (1966). Electron microscopic studies on lumpy skin disease virus type "Neethling". Onderstepoort J. Vet. Res. 33, 3–8.
Murphy, F. A., Fauquet, C. M., Bishop, D., Ghabrial, S. A., and Summers, M. D. (2008). Virus taxonomy: classification and nomenclature of viruses. Encyclopedia Virol. 140, 9–23.
Mwanandota, J. J., Macharia, M., Ngeleja, C. M., Sallu, R. S., Yongolo, M. G., Mayenga, C., et al. (2018). Validation of a diagnostic tool for the diagnosis of lumpy skin disease. Vet. Dermatol. 29, 532–e178. doi: 10.1111/vde.12690
Neamat-Allah, A. N. F., and Mahmoud, E. A. (2019). Assessing the possible causes of hemolytic anemia associated with lumpy skin disease naturally infected buffaloes. Comp. Clin. Pathol. 28, 747–753. doi: 10.1007/s00580-019-02952-9
Ngichabe, C. K., Wamwayi, H. M., Ndungu, E. K., Mirangi, P. K., Bostock, C. J., Black, D. N., et al. (2002). Long term immunity in African cattle vaccinated with a recombinant capripox-rinderpest virus vaccine. Epidemiol. Infect 128, 343–349.
Okur-Gumusova, S., Tamer, C., Ozan, E., Cavunt, A., Kadi, H., Muftuoglu, B., et al. (2020). An investigation of the seroprevalence of Crimean Congo hemorrhagic fever and lumpy skin disease in domesticated water buffaloes in northern Turkey. Trop. Biomed. 37, 165–173.
Osuagwuh, U. I., Bagla, V., Venter, E. H., Annandale, C. H., and Irons, P. C. (2007). Absence of lumpy skin disease virus in semen of vaccinated bulls following vaccination and subsequent experimental infection. Vaccine 25, 2238–2243. doi: 10.1016/j.vaccine.2006.12.010
Peeters, B., Bouma, A., Bruin, T. D., Moormann, R., and Kimman, T. (1994). Non-transmissible pseudorabies virus gp 50 mutants: a new generation of safe live vaccines. Vaccine 12, 375–380. doi: 10.1016/0264-410X(94)90104-X
Peng, C., Haller, S. L., Rahman, M. M., McFadden, G., and Rothenburg, S. (2016). Myxoma virus M156 is a specific inhibitor of rabbit PKR but contains a loss-of-function mutation in Australian virus isolates. Proc. Natl. Acad. Sci. U. S. A. 113, 3855–3860. doi: 10.1073/pnas.1515613113
Perry, B. (2010). Infectious diseases of livestock with special reference to southern Africa: J.A.W. Coetzer, G.R. Thomson and R.C. Tustin (editors), Oxford university press, 1994, 2 vols, 1, 605 + viiipp, $245.00, ISBN 0-1957-0506-8. Aust. Vet. J. 73:198.
Plowright, W., and Witcomb, M. A. (1959). The growth in tissue cultures of a virus derived from lumpy-skin disease of cattle. J. Pathol. Bacteriol. 78, 397–407. doi: 10.1002/path.1700780207
Prow, N. A., Jimenez Martinez, R., Hayball, J. D., Howley, P. M., and Suhrbier, A. (2018). Poxvirus-based vector systems and the potential for multi-valent and multi-pathogen vaccines. Expert Rev. Vaccines 17, 925–934. doi: 10.1080/14760584.2018.1522255
Punyapornwithaya, V., Seesupa, S., Phuykhamsingha, S., Arjkumpa, O., Sansamur, C., and Jarassaeng, C. (2022). Spatio-temporal patterns of lumpy skin disease outbreaks in dairy farms in northeastern Thailand. Front. Vet. Sci. 9:957306. doi: 10.3389/fvets.2022.957306
Reddy, B. S., and Sivajothi, S. (2016). Clinical management of demodicosis in Ongole cattle. J. Parasit. Dis. 40, 1311–1312. doi: 10.1007/s12639-015-0677-x
Reid, H. W., Buxton, D., Berrie, E., Pow, I., and Finlayson, J. (1984). Malignant catarrhal fever. Vet. Rec. 114, 581–583. doi: 10.1136/vr.114.24.581
Rgbe, H. (2014). Lumpy skin disease (LSD): Outbreak investigation, isolation and molecular detection of lumpy skin disease in selected areas of eastern Shewa, Ethiopia. Doctoral dissertation. AAU, 72.
Rhodesia, N. (1932). Annual bulletin, Department of Animal Health 1931. Annual bulletin Department of Animal Health.
Romero, C. H., Barrett, T., Evans, S. A., Kitching, R. P., Gershon, P. D., Bostock, C., et al. (1993). Single capripoxvirus recombinant vaccine for the protection of cattle against rinderpest and lumpy skin disease. Vaccine 11, 737–742. doi: 10.1016/0264-410X(93)90258-Y
Rouby, S., and Aboulsoud, E. (2016). Evidence of intrauterine transmission of lumpy skin disease virus. Vet. J. 193–195. doi: 10.1016/j.tvjl.2015.11.010
Saegerman, C., Bertagnoli, S., Meyer, G., Ganiere, J. P., Caufour, P., De Clercq, K., et al. (2018). Risk of introduction of lumpy skin disease in France by the import of vectors in animal trucks. PLoS One 13:e0198506. doi: 10.1371/journal.pone.0198506
Safini, N., Elmejdoub, S., Bamouh, Z., Jazouli, M., Hamdi, J., Boumart, Z., et al. (2022). Development and evaluation of a combined contagious bovine Pleuropneumonia (CBPP) and lumpy skin disease (LSD) live vaccine. Viruses 14:372. doi: 10.3390/v14020372
Salib, F. A., and Osman, A. H. (2011). Incidence of lumpy skin disease among Egyptian cattle in Giza governorate. Egypt. Veterinary World 4, 162–167.
Sanz-Bernardo, B., Haga, I. R., Wijesiriwardana, N., Basu, S., Larner, W., Diaz, A. V., et al. (2021). Quantifying and modeling the acquisition and retention of lumpy skin disease virus by Hematophagus insects reveals clinically but not subclinically affected cattle are promoters of viral transmission and key targets for control of disease outbreaks. J. Virol. 95:e02239-20. doi: 10.1128/JVI.02239-20
Sanz-Bernardo, B., Haga, I. R., Wijesiriwardana, N., Hawes, P. C., and Beard, P. M. (2020). Lumpy skin disease is characterized by severe multifocal dermatitis with necrotizing Fibrinoid Vasculitis following experimental infection. Vet. Pathol. 57, 388–396. doi: 10.1177/0300985820913268
Sanz-Bernardo, B., Suckoo, R., Haga, I. R., Wijesiriwardana, N., Harvey, A., Basu, S., et al. (2022). The acquisition and retention of lumpy skin disease virus by blood-feeding insects is influenced by the source of virus, the insect body part, and the time since feeding. J. Virol. 96:e0075122. doi: 10.1128/jvi.00751-22
Seet, B. T., Johnston, J. B., Brunetti, C. R., Barrett, J. W., Everett, H., Cameron, C., et al. (2003). Poxviruses and immune evasion. Annu. Rev. Immunol. 21, 377–423. doi: 10.1146/annurev.immunol.21.120601.141049
Selim, A., Manaa, E., and Khater, H. (2021a). Molecular characterization and phylogenetic analysis of lumpy skin disease in Egypt. Comp. Immunol. Microbiol. Infect. Dis. 79:101699. doi: 10.1016/j.cimid.2021.101699
Selim, A., Manaa, E., and Khater, H. (2021b). Seroprevalence and risk factors for lumpy skin disease in cattle in northern Egypt. Trop. Anim. Health Prod. 53:350. doi: 10.1007/s11250-021-02786-0
Şevik, M., and Doğan, M. (2017). Epidemiological and molecular studies on lumpy skin disease outbreaks in Turkey during 2014-2015. Transbound. Emerg. Dis. 64, 1268–1279. doi: 10.1111/tbed.12501
Shalaby, M. A., El-Deeb, A., El-Tholoth, M., Hoffmann, D., Czerny, C. P., Hufert, F. T., et al. (2016). Recombinase polymerase amplification assay for rapid detection of lumpy skin disease virus. BMC Vet. Res. 12:244. doi: 10.1186/s12917-016-0875-5
Sohier, C., Haegeman, A., Mostin, L., De Leeuw, I., Campe, W. V., De Vleeschauwer, A., et al. (2019). Experimental evidence of mechanical lumpy skin disease virus transmission by Stomoxys calcitrans biting flies and Haematopota spp. horseflies. Sci. Rep. 9:20076. doi: 10.1038/s41598-019-56605-6
Sprygin, A., Artyuchova, E., Babin, Y., Prutnikov, P., Kostrova, E., Byadovskaya, O., et al. (2018a). Epidemiological characterization of lumpy skin disease outbreaks in Russia in 2016. Transbound. Emerg. Dis. 65, 1514–1521. doi: 10.1111/tbed.12889
Sprygin, A., Babin, Y., Pestova, Y., Kononova, S., Wallace, D. B., Van Schalkwyk, A., et al. (2018b). Analysis and insights into recombination signals in lumpy skin disease virus recovered in the field. PLoS One 13:e0207480. doi: 10.1371/journal.pone.0207480
Stephens, L. R., Little, P. B., Wilkie, B. N., and Barnum, D. A. (1984). Isolation of Haemophilus somnus antigens and their use as vaccines for prevention of bovine thromboembolic meningoencephalitis. Am. J. Vet. Res. 45, 234–239.
Stram, Y., Kuznetzova, L., Friedgut, O., Gelman, B., Yadin, H., and Rubinstein-Guini, M. (2008). The use of lumpy skin disease virus genome termini for detection and phylogenetic analysis. J. Virol. Methods 151, 225–229. doi: 10.1016/j.jviromet.2008.05.003
Toker, E. B., Ates, O., and Yeşilbağ, K. (2022). Inhibition of bovine and ovine capripoxviruses (lumpy skin disease virus and Sheeppox virus) by ivermectin occurs at different stages of propagation in vitro. Virus Res. 310:198671. doi: 10.1016/j.virusres.2021.198671
Tulman, E. R., Afonso, C. L., Lu, Z., Zsak, L., Kutish, G. F., and Rock, D. L. (2001). Genome of lumpy skin disease virus. J. Virol. 75, 7122–7130. doi: 10.1128/JVI.75.15.7122–7130.2001
Tulman, E. R., Afonso, C. L., Lu, Z., Zsak, L., Sur, J. H., Sandybaev, N. T., et al. (2002). The genomes of Sheeppox and Goatpox viruses. J. Virol. 76:6054. doi: 10.1128/jvi.76.12.6054-6061.2002
Tuppurainen, E. S., Lubinga, J. C., Stoltsz, W. H., Troskie, M., Carpenter, S. T., Coetzer, J. A., et al. (2013). Evidence of vertical transmission of lumpy skin disease virus in Rhipicephalus decoloratus ticks. Ticks Tick Borne Dis. 4, 329–333. doi: 10.1016/j.ttbdis.2013.01.006
Tuppurainen, E. S., Pearson, C. R., Bachanek-Bankowska, K., Knowles, N. J., Amareen, S., Frost, L., et al. (2014). Characterization of sheep pox virus vaccine for cattle against lumpy skin disease virus. Antivir. Res. 109, 1–6. doi: 10.1016/j.antiviral.2014.06.009
Tuppurainen, E. S., Stoltsz, W. H., Troskie, M., Wallace, D. B., Oura, C. A., Mellor, P. S., et al. (2011). A potential role for ixodid (hard) tick vectors in the transmission of lumpy skin disease virus in cattle. Transbound. Emerg. Dis. 58, 93–104. doi: 10.1111/j.1865-1682.2010.01184.x
Tuppurainen, E. S., Venter, E. H., Coetzer, J. A., and Bell-Sakyi, L. (2015). Lumpy skin disease: attempted propagation in tick cell lines and presence of viral DNA in field ticks collected from naturally-infected cattle. Ticks Tick Borne Dis. 6, 134–140. doi: 10.1016/j.ttbdis.2014.11.002
Upton, C. (2004). Poxvirus bioinformatics. Methods Mol. Biol. 269, 347–370. doi: 10.1385/1-59259-789-0:347
Wallace, D. B., and Viljoen, G. J. (2005). Immune responses to recombinants of the south African vaccine strain of lumpy skin disease virus generated by using thymidine kinase gene insertion. Vaccine 23, 3061–3067. doi: 10.1016/j.vaccine.2004.10.006
Wang, C. L., Li, M., and Lyu, X. J. (2020). Classification and production process of human vaccine. Zhonghua Yu Fang Yi Xue Za Zhi 54, 1017–1025. doi: 10.3760/cma.j.cn112150-20200520-00756
Wang, J., Xu, Z., Wang, Z., Li, Q., Liang, X., Ye, S., et al. (2022). Isolation, identification and phylogenetic analysis of lumpy skin disease virus strain of outbreak in Guangdong, China. Transbound. Emerg. Dis. 69:e2291-e 2301. doi: 10.1111/tbed.14570
Wendy, I., Catherine, A. M., Andrew, A. M., David, H., and Stephen, B. F. (2002). Orf virus-encoded interleukin-10 stimulates the proliferation of murine mast cells and inhibits cytokine synthesis in murine peritoneal macrophages. J. Gen. Virol. 83, 1049–1058. doi: 10.1099/0022-1317-83-5-1049
Willey, R. L., Byrum, R., Piatak, M., Kim, Y. B., Cho, M. W., Rossio, J. L., et al. (2003). Control of viremia and prevention of simian-human immunodeficiency virus-induced disease in rhesus macaques immunized with recombinant vaccinia viruses plus inactivated simian immunodeficiency virus and human immunodeficiency virus type 1 particles. J. Virol. 77, 1163–1174. doi: 10.1128/jvi.77.2.1163-1174.2003
Wolff, J., Beer, M., and Hoffmann, B. (2020a). Thermal inactivation of different Capripox virus isolates. Microorganisms 8:2053. doi: 10.3390/microorganisms8122053
Wolff, J., Moritz, T., Schlottau, K., Hoffmann, D., Beer, M., and Hoffmann, B. (2020b). Development of a safe and highly efficient inactivated vaccine candidate against lumpy skin disease virus. Vaccines (Basel) 9:4. doi: 10.3390/vaccines9010004
Yana, P., Olga, B., Alexander, K., and Alexander, S. (2018). A real time high-resolution melting PCR assay for detection and differentiation among sheep pox virus, goat pox virus, field isolates and vaccine strains of lumpy skin disease virus. Mol. Cell. Probes 41:S0890850818301488. doi: 10.1016/j.mcp.2018.08.003
Yeruham, I., Nir, O., Braverman, Y., Davidson, M., Grinstein, H., Haymovitch, M., et al. (1995). Spread of lumpy skin disease in Israeli dairy herds. Vet. Rec. 137:91.
Yeruham, I., Perl, S., Nyska, A., Abraham, A., and Grinstein, H. (1994). Adverse reactions in cattle to a capripox vaccine. Vet. Rec. 135, 330–332. doi: 10.1136/vr.135.14.330
Young, E., Basson, P. A., and Weiss, K. E. (1970). Experimental infection of game animals with lumpy skin disease virus (prototype strain Neethling). Onderstepoort J. Vet. Res. 37, 79–87.
Zavala, F., Rodrigues, M., Rodriguez, D., Rodriguez, J. R., Nussenzweig, R. S., and Esteban, M. (2001). A striking property of recombinant poxviruses: efficient inducers of in vivo expansion of primed CD8+ T cells. Virology 280, 155–159. doi: 10.1006/viro.2000.0792
Zeedan, G. S. G., Mahmoud, A. H., Abdalhamed, A. M., El-Razik, K., Khafagi, M. H., and Zeina, H. (2019). Detection of lumpy skin disease virus in cattle using real-time polymerase chain reaction and serological diagnostic assays in different governorates in Egypt in 2017. Vet World 12, 1093–1100. doi: 10.14202/vetworld.2019.1093-1100
Keywords: lumpy skin disease, lumpy skin disease virus, epidemiology, vaccine, etiology, diagnosis
Citation: Liang Z, Yao K, Wang S, Yin J, Ma X, Yin X, Wang X and Sun Y (2022) Understanding the research advances on lumpy skin disease: A comprehensive literature review of experimental evidence. Front. Microbiol. 13:1065894. doi: 10.3389/fmicb.2022.1065894
Received: 10 October 2022; Accepted: 27 October 2022;
Published: 28 November 2022.
Edited by:
Pragya Dhruv Yadav, ICMR-National Institute of Virology, IndiaReviewed by:
Ahmed N. F. Neamat-Allah, Zagazig University, EgyptCopyright © 2022 Liang, Yao, Wang, Yin, Ma, Yin, Wang and Sun. This is an open-access article distributed under the terms of the Creative Commons Attribution License (CC BY). The use, distribution or reproduction in other forums is permitted, provided the original author(s) and the copyright owner(s) are credited and that the original publication in this journal is cited, in accordance with accepted academic practice. No use, distribution or reproduction is permitted which does not comply with these terms.
*Correspondence: Yuefeng Sun, c3VueXVlZmVuZ0BjYWFzLmNu; Xiangwei Wang, d2FuZ3hpYW5nd2VpQGNhYXMuY24=; Xiangping Yin, eWlueGlhbmdwaW5nQGNhYXMuY24=
Disclaimer: All claims expressed in this article are solely those of the authors and do not necessarily represent those of their affiliated organizations, or those of the publisher, the editors and the reviewers. Any product that may be evaluated in this article or claim that may be made by its manufacturer is not guaranteed or endorsed by the publisher.
Research integrity at Frontiers
Learn more about the work of our research integrity team to safeguard the quality of each article we publish.