- 1College of Animal Science & Technology, Zhongkai University of Agriculture and Engineering, Guangzhou, China
- 2College of Veterinary Medicine, South China Agricultural University, Guangzhou, China
Mitochondria are important organelles involved in cell metabolism and programmed cell death in eukaryotic cells and are closely related to the innate immunity of host cells against viruses. Mitophagy is a process in which phagosomes selectively phagocytize damaged or dysfunctional mitochondria to form autophagosomes and is degraded by lysosomes, which control mitochondrial mass and maintain mitochondrial dynamics and cellular homeostasis. Innate immunity is an important part of the immune system and plays a vital role in eliminating viruses. Viral infection causes many physiological and pathological alterations in host cells, including mitophagy and innate immune pathways. Accumulating evidence suggests that some virus promote self-replication through regulating mitophagy-mediated innate immunity. Clarifying the regulatory relationships among mitochondria, mitophagy, innate immunity, and viral infection will shed new insight for pathogenic mechanisms and antiviral strategies. This review systemically summarizes the activation pathways of mitophagy and the relationship between mitochondria and innate immune signaling pathways, and then discusses the mechanisms of viruses on mitophagy and innate immunity and how viruses promote self-replication by regulating mitophagy-mediated innate immunity.
1 Introduction
1.1 Mitochondria and mitophagy
Mitochondria are dynamic, multifunctional organelles with double-layered membranes. Mitochondria not only provide energy for life activities and metabolism, but also serve as the primary site of aerobic respiration, which is known as the “powerhouse” of the cell (Siekevitz, 1957). Mitochondria are also involved in the physiological processes of adenosine triphosphate (ATP) and reactive oxygen species (ROS) production, regulating lipid metabolism and calcium signal transduction, and play a crucial role in the maintenance of normal life activities in the human body (Montava-Garriga and Ganley, 2020).
Mitophagy is a process in which phagosomes select damaged and excess mitochondria, and are subsequently degraded by autophagic autophagolysosomes. Mitophagy controls the quality of mitochondria, maintains the normal cellular function and physiological processes (Teresak et al., 2022). There are many factors that induce mitophagy, such as turnover of mitochondria during normal cell physiology, disease, excessive ROS, and viral infection (Lin et al., 2019).
1.2 Mechanisms of mitophagy activation
Mitophagy can be mediated by two pathways, PTEN-induced putative kinase 1 (PINK1)/Parkin-dependent pathway and PINK1/Parkin-independent pathway. Among them, Parkin-dependent pathway includes ubiquitin mediated mitophagy; PINK1/Parkin-independent pathway includes autophagy receptor-mediated and mitochondrial dynamics-mediated mitophagy (Figure 1).
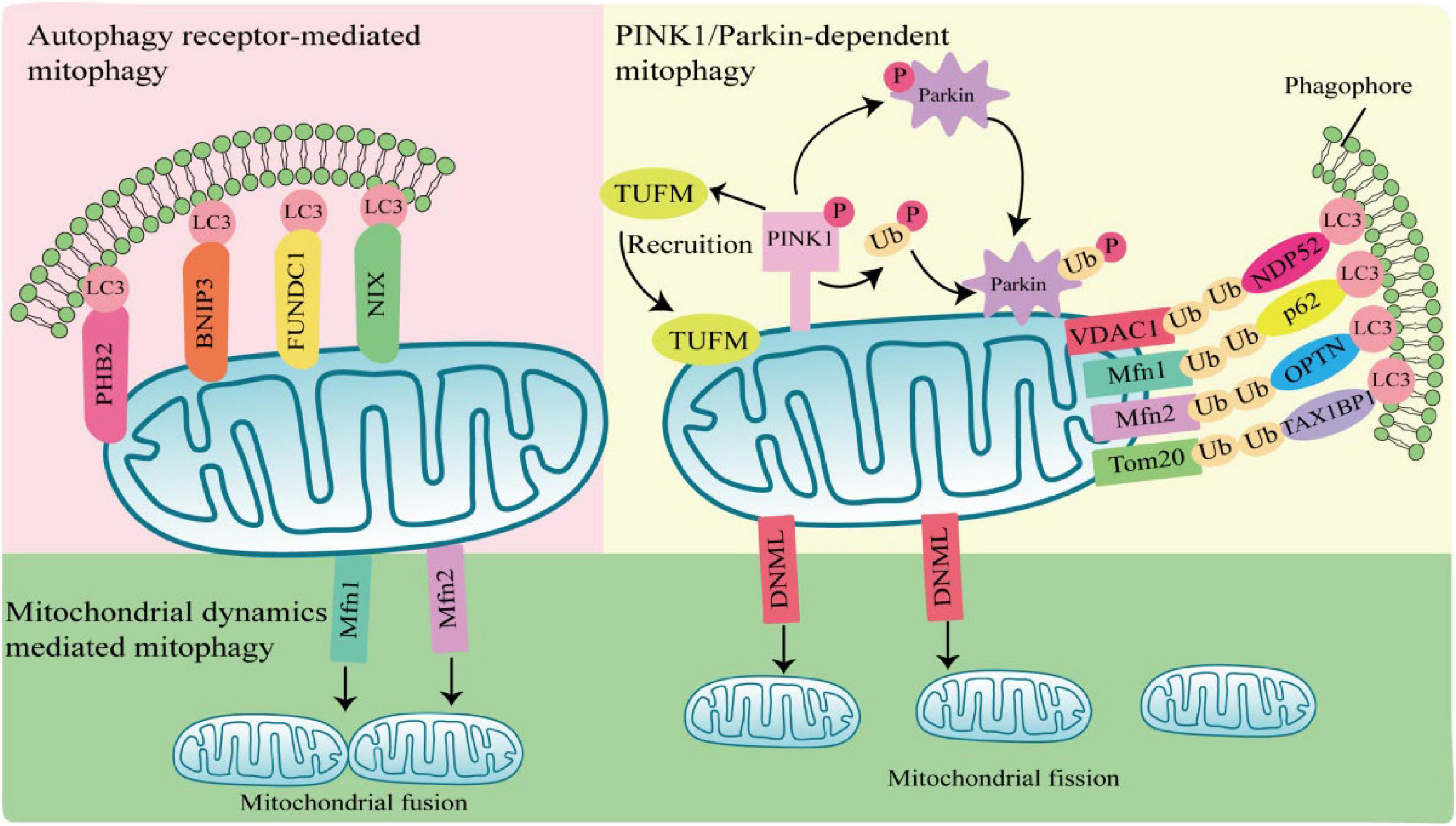
Figure 1. Mechanisms of mitophagy activation. Parkin-dependent mitophagy generally refers to ubiquitin-mediated mitophagy. PTEN-induced putative kinase 1 (PINK1) accumulates and is phosphorylation at the outer mitochondrial membrane (OMM) when mitochondria are damaged or depolarized. Phosphorylated PINK1 recruits Tu translation elongation factor (TUFM) to the OMM and leads to phosphorylation of Parkin and ubiquitin. Subsequently, activated Parkin ubiquitinates mitochondrial outer membrane proteins, which are recognized by autophagy receptors such as nuclear dot protein 52 (NDP52), sequestosome 1 (SQSTM1), optineurin (OPTN), and Tax1-Binding Protein 1 (TAX1BP1), and interact with light chain 3 (LC3) through the LIR region of autophagy receptors to induce autophagy. Parkin-independent mitophagy depends on several autophagy receptors, including prohibitin 2 (PHB2), E1B 19kDa-interacting protein 3 (BNIP3), FUN14 domain containing 1 (FUNDC1), and NIP3-like protein X (NIX), which directly anchor to the outer mitochondrial membrane, and bind to the LC3 do-main on autophagosomes in a ubiquitination independent manner to induces mitophagy. Mitochondrial dynamics-mediated mitophagy. When activated by pathogens, mitochondrial fission is regulated by dynamin 1-like (DNM1L), Mfn1, and mitochondrial fusion protein 2 (Mfn2) are activated and recruited to the mitochondria, inducing mitochondrial fission and fusion, thereby regulating mitophagy.
1.2.1 PINK1/Parkin-dependent mitophagy
PTEN-induced putative kinase 1/Parkin-dependent mitophagy usually occurs in response to mitochondrial damage or mitochondrial membrane depolarization. PINK1 is a serine/threonine kinase located in the inner mitochondrial membrane. In normal mitochondria, PINK1 is degraded by the proteasome after entering the inner mitochondrial membrane. When mitochondria are damaged or depolarized, PINK1 accumulates at the outer mitochondrial membrane (OMM) and phosphorylates ubiquitin (Ub) molecules at S65 (Xu et al., 2020). Parkin has a ring finger protein 1 (RING1) domain that binds to phosphorylated Ub and alters Parkin conformation, leading to Parkin activation (Kane et al., 2014; Wauer et al., 2015; Tang et al., 2017). Activated Parkin ubiquitinated mitochondrial outer membrane protein voltage-dependent anion channel 1 (VDAC1), mitochondrial fusion protein 1/2 (MFN1/2) and mitochondrial membrane translocase 20 (TOMM20) (Chan et al., 2011). Structurally, autophagy receptors sequestosome 1 (SQSTM1), nuclear dot protein 52 (NDP52), optineurin (OPTN), and Tax1-Binding Protein 1 (TAX1BP1), contain a ubiquitin-binding domain and a microtubule-associated protein 1 light chain 3 (LC3) interacting LIR region, which is a bridge between damaged or depolarized mitochondria and phagosomes (Hanna et al., 2012; Lazarou et al., 2015). Autophagy receptors link ubiquitinated outer mitochondrial membrane proteins and phagosomes at the beginning of mitophagy, prompting damaged or depolarized mitochondria to be engulfed by autophagosomes and then degraded by lysosomes (Harper et al., 2018; Pickles et al., 2018).
1.2.2 PINK1/Parkin-independent mitophagy
PTEN-induced putative kinase 1/Parkin-independent mitophagy is usually mediated by the autophagy receptors, such as NIP3-like protein X (Nix; also known as BNIP3L), prohibitin 2 (PHB2), E1B 19kDa-interacting protein 3 (BNIP3), and FUN14 domain containing 1 (FUNDC1). These autophagy receptors directly anchor to the outer mitochondrial membrane, and bind to the LC3 domain on autophagosomes in an ubiquitination independent manner to induces mitophagy (Hanna et al., 2012; Shi et al., 2014; Chen et al., 2017; Wei et al., 2017).
1.2.3 Mitochondrial dynamics-mediated pathway
Most damaged mitochondria are cleared by mitophagy directly, but some with larger volumes cannot be encapsulated by autophagosomes and need to be cleaved into small particles to be phagocytosed and degraded. Mitochondria are in a balanced process of fusion and fission to maintain the stability of mitochondrial mass, which is called mitochondrial dynamics. Mitochondrial fusion is regulated by the mitochondrial fusion protein 2 (Mfn2), and mitochondrial fission is regulated by dynamin 1-like (DNM1L) (Wei et al., 2017). When activated by pathogens, DNM1L is recruited in OMM and assembled into oligomeric structures that drive the contraction and rupture of mitochondrial membranes, leading to mitochondrial fission. Fission mitochondria are engulfed by autophagosomes, which in turn are degraded by lysosomes. The whole process is called mitochondrial dynamics mediated-mitophagy (Rambold et al., 2011; Pernas and Scorrano, 2016).
2 Virus and innate immunity
2.1 Innate immune system
Innate immunity is the first line of defense against the invasion of exogenous microorganisms, which is a rapid response to pathogens or danger signals. Host’s innate immune system recognizes various pattern-associated molecular patterns (PAMPs) through a series of pattern recognition receptors (PRRs) to activate the downstream signaling pathway (Brennan and Bowie, 2010). These receptors are distributed on the cell membrane or in the cytoplasm, including Toll-like receptors (TLRs), retinoic acid-inducible gene-I-like receptors (RLRs), and NOD-like receptors (NLRs) (Bożek and Lengauer, 2010; Li and Wu, 2021). When pathogenic microorganisms invade the body, PAMPs are recognized by PRRs expressed by innate immune cells and induce multiple intracellular signaling cascades. These cascades of signaling lead primarily to the activation of the downstream nuclear factor kappa B (NF-κB), interferon regulatory factor 3 (IRF3) and interferon regulatory factor 7 (IRF7), which enable NF-κB, IRF3 and IRF7 enter the nucleus and promote secretion of proinflammatory cytokines and IFNs. Secreted IFNs bind to their corresponding interferon receptors on the cell membrane, and the expression of hundreds of interferon-stimulated genes (ISGs) is induced by activation of the JAK-STAT signaling pathway to defend against and clear pathogenic microorganisms (Bożek and Lengauer, 2010; Thompson et al., 2011; Li and Wu, 2021).
2.2 The mechanism of innate immunity responses to viral infection
There are multiple ways in which innate immune responses in viral infection, including the TLR pathway, RIG-I pathway and NLRs pathway (Figure 2).
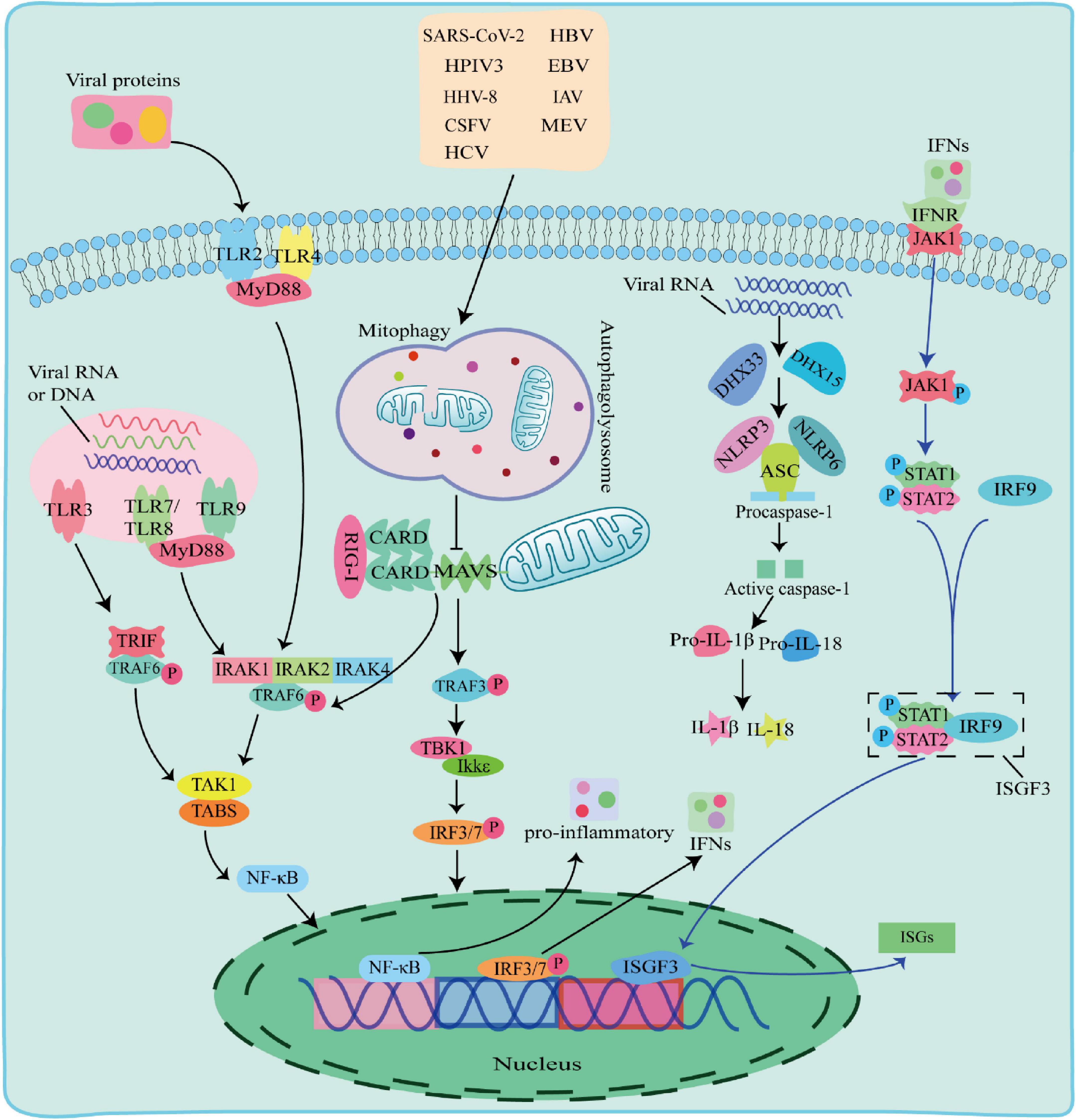
Figure 2. The Toll-like receptor (TLR) pathway, RIG-I pathway and NOD-like receptors (NLRs) pathway responses to viral infection. TLR pathway: TLR2 and TLR4 detected viral proteins on the membrane, TLR3, TLR7, TLR8, and TLR9 detected virus nucleic acids in endosomes. The TIR domain of TLR3 binds to the adaptor protein TRIF, and the TIR domain of other TLRs binds to the adaptor protein MyD88 to activate and induce nuclear factor kappa B (NF-κB) to enter the nucleus and promote the secretion of IFN-I and proinflammatory factors; RIG-I pathway: after the virus is stimulated by dsRNA, caspase recruitment domain (CARD-CARD) interaction between RIG-I and Mitochondrial antiviral-signaling protein (MAVS) initiates a signaling cascade that activates the TBK1-IRF3 or NF-κB pathway to induce the expression of IFN-I and multiple proinflammatory genes; NOD-like receptors (NLRs) pathway: When RNA viruses recognized by RNA helicases DHX33 or DHX15, which further activates to recruit downstream NLRs NOD-like receptors protein 3 (NLRP3) or NOD-like receptors protein 6 (NLRP6) to form NLRP3 or NLRP6 inflammasome complex with pro-cysteinyl aspartate specific proteinase-1 (pro-caspase-1) and apoptosis-associated speck-like protein containing a card (ASC), which in turn activates caspase-1 to form active IL-1β and IL-18, inducing the onset of inflammation.
2.2.1 TLR pathway against viruses
Toll-like receptors are pattern recognition receptors expressed by antigen-presenting cells (APCs) and exist on the cell surface or in the endosomes to sense PAMPs. TLRs on cell membrane recognize viral proteins, including TLR2 and TLR4 (Kurt-Jones et al., 2000; Bieback et al., 2002). Endosomal TLRs, including TLR3, TLR7, TLR8, and Toll-like receptor 9 (TLR9) recognize various viral nucleic acids, among which TLR3 recognizes double stranded RNA viruses (Alexopoulou et al., 2001), TLR7 and TLR8 recognize single stranded RNA viruses and (Diebold et al., 2004), TLR9 uniquely identifies unmethylated CpG DNA viruses. The TIR domain of TLR3 binds to the adaptor protein TRIF, and interacts with TNF receptor-associated factor 6 (TRAF6). The TIR domains of other TLRs bind to MyD88 adaptor protein, and recruits and phosphorylates downstream interleukin-1 receptor-associated kinase 1 (IRAK1), interleukin-1 receptor-associated kinase 2 (IRAK2), and interleukin-1 receptor-associated kinase 4 (IRAK4) (Janssens and Beyaert, 2003). Phosphorylated IRAK interacts with TRAF6 and activates a protein kinase complex consisting of TGF-beta-Activated Kinase 1 (TAK1) and TAK1-binding proteins (TABs) (Deng et al., 2000; Wang et al., 2001). The activated protein kinase complex activates NF-κB into the nucleus, leading to upregulation of inflammatory cytokines, including TNF, interleukin 1β (IL-1β), and IFN-γ. IFNs phosphorylate STAT1 and STAT2 to form a heterodimer by activating the JAK-STAT signaling pathway, which associates with interferon regulatory factor 9 (IRF9) to form a complex, interferon-stimulated gene factor 3 (ISGF3). ISGF3 entries into the nucleus and induces the expression of hundreds of ISGs, thereby play an antiviral role.
2.2.2 RIG-I pathway against viruses
Retinoic acid-inducible gene-I-like receptors are cytoplasmic RNA helicases that act as intracellular receptors for dsRNA viruses, including retinoic acid-inducible gene I (RIG-I), melanoma differentiation-associated gene 5 (MDA5) and laboratory of genetics and physiology 2 (LGP2). RIG-I and MDA5 contain a DExD/H-box RNA helicase domain, a C-terminal inhibitory domain (RD), and two tandem caspase recruitment domains (CARDs) at the N terminus, whereas LGP2 lacks CARDs domains (Jia et al., 2021). LGP2 reported to regulate RIG-I and MDA5 depending on the type of infectious RNA virus (Fu et al., 2020; Kong et al., 2021). Mitochondrial antiviral-signaling protein (MAVS) is a CARD domain-containing transmembrane protein localized to the outer mitochondrial membrane. The CARD domain of RIG-I in the cytoplasm cannot send signals to downstream pathways under normal circumstances. Upon dsRNA viral stimulation, the CARD domain is activated and the card-card interaction between RIG-I and MAVS initiates a signaling cascade. This signal activates TRAF6 and TRAF3, TRAF6 induces TAK1 protein kinase complex, and TRAF3 activates TBK1 and IKKε protein kinases, which phosphorylate and activate NF-κB and IRF3, respectively, and induce the expression of IFN-I and several proinflammatory genes, thereby playing antiviral effects (Chiang et al., 2014; Huang et al., 2021).
2.2.3 NLRs pathway against viruses
NOD-like receptors are one type of PRRs receptors that recognize PAMPs in the cytoplasm. The nucleotide-binding oligomerization domain-like receptor protein 3 (NLRP3), apoptosis-associated speck-like protein containing a card (ASC) and pro-cysteinyl aspartate specific proteinase-1 (pro-caspase-1) form an inflammasome complex to regulate IL-1β maturation and secretion with interleukin18 (IL-18) (Agostini et al., 2004; Allen et al., 2009). When RNA viruses including reovirus and rotavirus invade cells, their PAMPs dsRNA could be recognized by RNA helicases DHX33 or DHX15, which further activates to recruit downstream NLRs NLRP3 or NLRP6 to form NLRP3 or NLRP6 inflammasome complex with pro-caspase-1 and ASC, followed by activation of IL-1β and IL-18 for inducing the onset of inflammation (Mitoma et al., 2013; Shi et al., 2019; Xing et al., 2021; Zhang et al., 2022).
3 Mitochondria and innate immunity pathway
3.1 Mitochondrial proteins and innate immunity pathway
Studies have shown that MAVS is a key node protein in innate immunity antiviral signal transduction, which anchored to mitochondria and is a key adaptor protein in RIG-I like receptor signaling. RIG-I-MAVS signal transduction pathway plays a key role in the immune response of cells in response to RNA virus infection (Zhou et al., 2021). Studies have shown that MAVS molecules, after being activated by upstream RIG-I, will form prion-like aggregates and further activate downstream signaling pathways, eventually activating transcription factors IRF3 and NF-κB and inducing cells to express IFN-β, thereby inhibiting the proliferation of the virus in the body (Liu et al., 2020; Zou et al., 2021). Further studies found that Postmastectomy Radiation Therapy 9 (PMRT9), localized to mitochondria, directly binds to MAVS and catalyzes the symmetric dimethylation of MAVS, thereby inhibiting the aggregation and activation of MAVS. RNA virus infection attenuates the mitochondrial localization of PRMT9 and its inhibitory effect on MAVS, decreases the methylation modification of MAVS, and then promotes the aggregation and activation of MAVS, and up-regulates the expression of type I interferon and the antiviral immune response (Bai et al., 2022). In addition, in the pathogenesis of Non-alcoholic fatty liver disease (NAFLD), MAVS plays an important role in mitochondrial metabolism and energy regulation, and is a key protein that mediates the interaction between immune response and metabolic homeostasis by maintaining mitochondrial homeostasis (Fu et al., 2022). Studies have shown that the mitochondrial protein Era-like 1 (ERAL1) also positively regulates RLR-mediated innate antiviral immune responses. On the one hand, ERAL1 binds to MAVS and promotes MAVS activation; on the other hand, ERAL1 is transported from mitochondria to cytoplasm and promotes K63-linked ubiquitination of RIG-I/MDA5 (Li et al., 2021).
3.2 Mitochondrial DNA (mtDNA) and innate immunity pathway
Mitochondria have emerged as key factor of innate immunity, which works on anti-viruses. NLRX1, TRAF6, NLRP3, IRGM, and other innate immune molecules have also been confirmed to be associated with mitochondria (Arnoult et al., 2011). Meanwhile, the release of mitochondrial DNA (mtDNA) activates a series of innate immune signaling pathways such as STING, TLR9 and NLRP3.
3.2.1 mtDNA-cGAS-STING
The mitochondrial genomic DNA differs from the nuclear DNA. One of the significant differences is that mtDNA is generally less methylated than nuclear DNA, which makes mtDNA very similar to bacterial DNA and usually acts as heterologous DNA to activate the immune response. In the event of mitochondrial stress, mtDNA releases into the cytoplasm through BAX/BAK-dependent mitochondrial outer membrane permeabilization (MOMP) or mitochondrial permeability transition pore (mPTP) (Cosentino et al., 2022). The mtDNA binds to cGAS, activating the downstream STING signaling pathway and inducing the production of IFN-I (White et al., 2014). Under pathological conditions, abnormal activation of mtDNA-cGAS-STING signaling pathway causes the overexpression of IFN-I and related inflammatory genes, which is closely related to the occurrence and development of various diseases (West et al., 2015).
3.2.2 mtDNA activates TLR9
Toll-like receptor 9 recognizes hypomethylated CpG motifs in DNA through the adaptor protein myeloid differentiation primary response protein 88 (MYD88) (Zhang et al., 2010), which activates MAPKs and NF-κB to trigger inflammatory responses or activates interferon regulatory factor 7 (IRF7) to enhance type I interferon responses in immune cells. Studies have demonstrated that CpG motifs in mitochondrial DNA activate TLR9 signaling, thereby activating p38 and p42-44 MAPK activity. In addition, mitochondrial DNA in the plasma of trauma patients and other non-infectious injuries activate TLR9 through an extracellular release mechanism, while promoting inflammation by activating TLR9 through an intracellular binding mechanism (Oka et al., 2012). Furthermore, oxidized mitochondrial DNA has been shown to induce TLR9 and IRF7 dependent IFN-α expression, thereby enhancing the type I interferon response in plasmacytoid dendritic cells (Caielli et al., 2016).
3.2.3 mtDNA and inflammasome activation
Mammalian inflammasomes are an important part of the host’s innate immune system, and substantial evidence suggests that mitochondrial DNA is an endogenous agonist of inflammasomes (Riley and Tait, 2020). Studies have shown that when mouse bone marrow-derived macrophages are stimulated by lipopolysaccharide (LPS) and ATP, mitochondrial DNA released into the cytoplasm, which promotes the activation of NLRP3 inflammasome, thereby causing the secretion of IL-1β and IL-18 (Xian et al., 2021). Moreover, studies also have shown that mitochondrial DNA directly activates the NLRC4 inflammasomes, and oxidized mitochondrial DNA released into the cytoplasm during apoptosis can binds to the NLRP3 inflammasome (Jabir et al., 2015).
3.3 Mitochondrial double stranded RNA (mtdsRNA) and innate immunity pathway
dsRNAs are usually found in the cytoplasm, and one source is mitochondrial double-stranded RNA (mtdsRNA), which is produced by bidirectional transcription of mtDNA (Borowski et al., 2013; Dhir et al., 2018). PNPase and hSuv3, two helicases of mtdsRNA, are extremely important in limiting mtdsRNA levels. Deletion of either of them will result in a substantial accumulation of mitochondrial dsRNA that escapes into the cytoplasm (Borowski et al., 2013). Studies have shown that MDA5 is a major sensor of mtdsRNA. The immune response induced by mtdsRNA is mediated through the MDA5-MAVS axis and results in the expression of IFN-I and proinflammatory cytokine genes (Wiatrek et al., 2019). It has also been shown that the accumulation of dsRNA can be detected in virus-infected mammalian cells. Therefore, it is plausible that mtdsRNA accumulation and escape into the cytoplasm trigger an antiviral response upon viral infection (Weber et al., 2006; Dhir et al., 2018).
4 Effects of viral infection on mitochondrial function and mitophagy
Viruses are intracellular parasites without cellular structures, which target mitochondria in many ways to disrupt the homeostasis of mitochondria and in turn affect the metabolism and physiology of the host cell, promoting the replication and propagation of the virus itself in the host cell (Hanada et al., 2007). At the same time, viruses directly regulate mitophagy through their own viral factors or indirectly regulate mitophagy through different means (Figure 3; Kane et al., 2014; Ding et al., 2017; Sin et al., 2017).
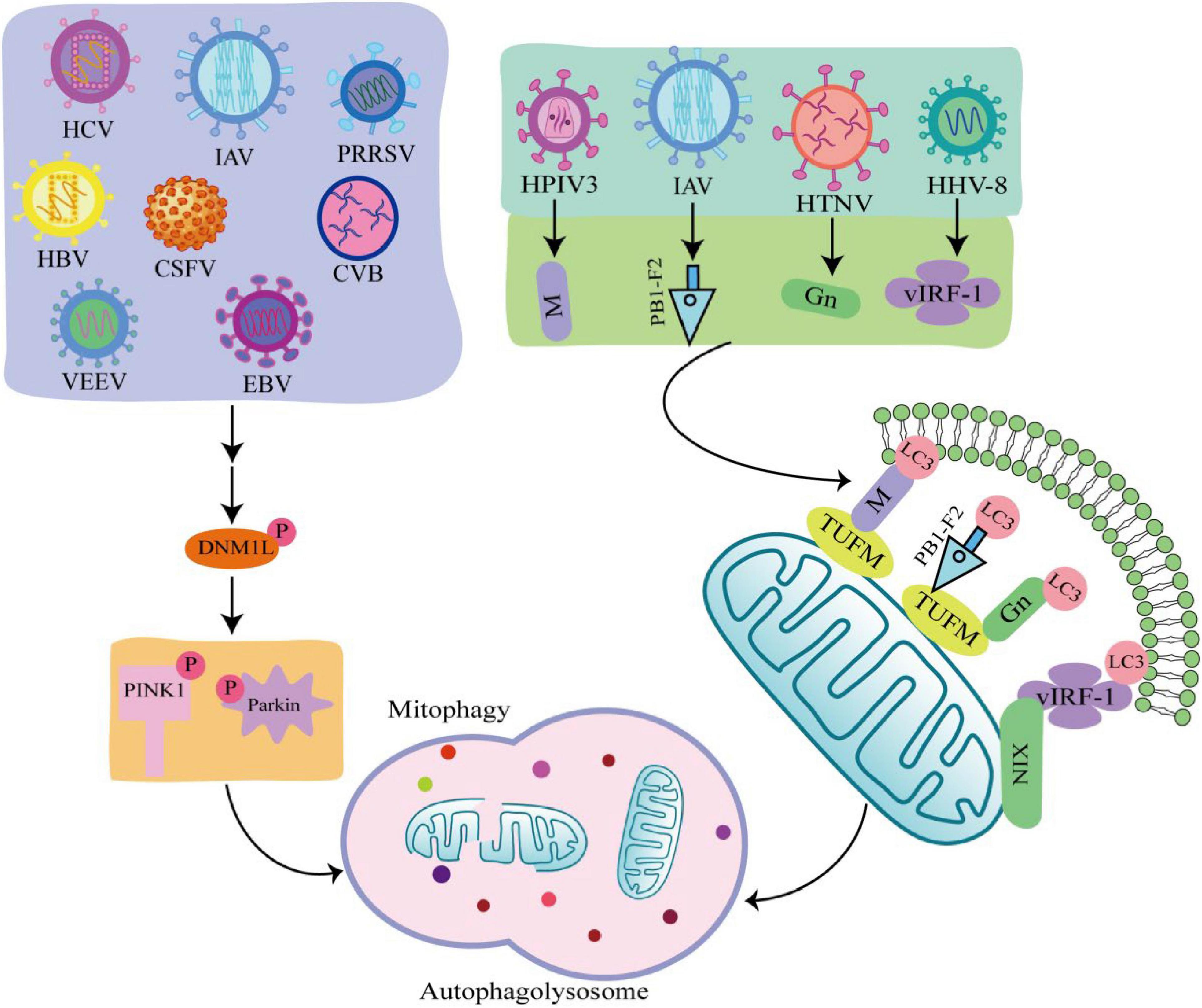
Figure 3. Viral infection induces mitophagy through different pathways. Some viruses such as hepatitis C virus (HCV), influenza A virus (IAV), porcine reproductive and respiratory syndrome virus (PRRSV), Hepatitis B virus (HBV), classical swine fever virus (CSFV), coxsackie virus B (CVB), venezuelan equine encephalitis virus (VEEV), and Epstein-Barr virus (EBV) phosphorylate and recruit mitochondrial fission is regulated by dynamin 1-like (DNM1L) to mitochondria, promoting mitochondrial fission in turn causing PTEN-induced putative kinase 1 (PINK1)/Parkin dependent mitophagy. There are also viruses such as human herpes virus 8 (HHV-8), HPIV3, IAV, hantaan virus (HTNV) utilize encode proteins that act as mitophagy adaptors to bind mitophagy receptors and light chain 3 (LC3), initiating mitophagy.
4.1 Virus regulates mitophagy through PINK1/Parkin-dependent pathway
Many viruses are shown to initiate mitophagy by activating the PINK1-Parkin pathway. Hepatitis B virus (HBV) and hepatitis C virus (HCV) promote Dynamin 1 Like (DNM1L) gene expression and recruitment to mitochondria by stimulating DNM1L (ser616) phosphorylation, leading to mitochondrial fission (Kim et al., 2013a,b). After mitochondrial fission, PINK1 and Parkin genes are up-regulated, and Parkin is translocated to mitochondria, followed by mitophagy. This is consistent with the detected changes in Parkin levels in liver tissue samples from HBV and HCV patients (Jassey et al., 2019). In addition, when DNM1L is knocked out, mitochondrial division and mitophagy are induced by the PB1-F2 protein of influenza A virus (IAV), indicating that IAV also induces mitochondrial division by stimulating DNM1L phosphorylation, leading to Parkin-dependent mitophagy (Yoshizumi et al., 2014; Wang H. et al., 2021). Similarly, Epstein-Barr virus (EBV), coxsackie virus B (CVB), venezuelan equine encephalitis virus (VEEV), classical swine fever virus (CSFV), and porcine reproductive and respiratory syndrome virus (PRRSV) all promote DNM1L phosphorylation and induce mitophagy through the PINK1-Parkin pathway (Li et al., 2016; Gou et al., 2017; Keck et al., 2017; Xie et al., 2020; Wang R. et al., 2021).
4.2 Virus regulates mitophagy through PINK1-Parkin independent pathway
Some viruses initiate mitophagy independent of the PINK1-Parkin signaling pathway. PINK-Parkin-independent mitophagy is usually mediated by autophagy receptors, such as NIX, BNIP3, FUNDC1, and PHB2 (Liu et al., 2014). These autophagy receptors anchored on the outer mitochondrial membrane, interact with LC3 through the LC3 interacting region, promoting mitophagy. Viruses use their encoded proteins as mitophagy adapters to bind to autophagy receptors and LC3 to initiate mitophagy (Birgisdottir et al., 2013). For example, the vIRF-1 protein encoded by human herpes virus 8 (HHV-8) directly bind to NIX and LC3 to activate mitophagy. Tu translation elongation factor (TUFM) is a protein that exists in the cytoplasm and mitochondria (Lin et al., 2020). The matrix protein of human parainfluenza virus type 3 (HPIV3) is transferred to mitochondria through interaction with TUFM and interacts with LC3 to mediate mitophagy (Ding et al., 2017). Moreover, both PB1-F2 protein of IAV and GN protein of hantaan virus (HTNV) promote mitophagy in this way (Wang et al., 2019; Wang R. et al., 2021). It was found that West Nile virus (WNV) infection induce LC3 lipidation and the formation of LC3-labeled autophagic vacuoles in Vero cells, but the link between WNV and mitophagy is unclear (Beatman et al., 2012). The autophagy-receptor-dependent, rather than PINK1-Parkin-dependent mitophagy also plays an important role in viral replication, suggesting that autophagy receptors may be critical targets against viral infection.
5 Viruses promote self-replication by mtDNA-cGAS-STING
5.1 DNA virus and mtDNA-cGAS-STING signaling pathway
During DNA virus infection, cGAS recognize the genomic DNA of the virus, and activate the downstream STING signaling pathway by binding to mtDNA. Accordingly, viruses have evolved corresponding mechanisms to evade the host’s immune surveillance. For example, Herpes Simplex Virus Type 1 (HSV-1) virus can rapidly degrade the mtDNA of host cells through its encoded conserved nuclease UL12.5, resulting in complete loss of mtDNA in infected cells, thereby evading the immune response of host cells and facilitating its own replication (Duguay and Smiley, 2013).
5.2 RNA viruses and mtDNA-cGAS-STING signaling pathway
The activation of cGAS-STING signaling pathway has been observed during the infection of a variety of RNA viruses, and studies have shown that the activation of cGAS-STING signaling pathway is related to the release of mtDNA caused by RNA virus infection. For example, dengue virus (DENV) stimulates the release of IL-1β from host cells, which stimulate adjacent cells to increase mitochondrial volume, reduce mitochondrial membrane potential, and finally promote mtDNA release and activate cGAS-STING pathway (Aarreberg et al., 2019). In addition, DENV promotes the release of mtDNA by inducing the production of ROS in host cells, and activates the innate immune signaling pathway mediated by TLR9 and cGAS (Lai et al., 2018). Studies have shown that the influenza and Zika viruses manipulate mtDNA to escape host immune response (Newby et al., 2007; Zheng et al., 2018). Influenza virus binds to mtDNA via the RNA-binding domain of the non-structural protein (NS1) protein to evade cGAS-STING-dependent antiviral immune responses, while Zika virus enhances the stability of cysteine-containing aspartate-specific proteinase-1 (caspase-1) through its encoded NS1, in order to promote the cleavage of cGAS by caspase-1, and achieve the effect of inhibiting the host immune response.
6 Viruses mediate immune escape by inducing mitophagy
6.1 Viruses promote self-replication by triggering mitophagy to inhibit IFN-I
Interferon is a glycoprotein produced in response to stimulation by viruses or other interferon inducers. The generation of IFN- I (IFNα and IFNβ) is the core element of anti-virus, which is crucial to the survival of host cells during virus infection (Perry et al., 2005). During viral infection, viral PAMP is recognized by RLRs receptors and binds to MAVS on the outer mitochondrial membrane to activate MAVS, which induce IFN-I secretion by recruiting and activating TRAF6, TBK1, and TABS (Castanier et al., 2010). Studies have shown that some viruses, including Mesles virus (MEV), HHV-8, CSFV, EBV, HBV, HCV, IAV, SARS-CoV-2, and HPIV3, antagonize IFN-I production through mitophagy. Virus-induced mitophagy promotes the degradation of MAVS, and low levels of MAVS block its downstream signaling pathways to inhibit the secretion of IFN–induced mitophagy promotes the degradation of MAVS, and low levels of MAVS block its downstream stream signaling pathways to inhibit the secretion of IFN-I, which in turn inhibits the production of antiviral proteins and promotes viral replication (Figure 4; Xia et al., 2014a). In this process, viruses use different mechanisms to induce mitophagy to inhibit IFN-I production. For example, MEV infection induces SQSTM1-mediated mitophagy to degrade MAVS, which attenuates the RLR signaling pathway and promotes viral replication (Xia et al., 2014a). Similarly, CSFV infection also inhibits IFN-I by inducing mitophagy through the autophagy receptor NDP52 and affecting mitochondrial dynamics (Kim et al., 2014; Fan et al., 2019). In addition, HBV induced Parkin dependent mitophagy, and recruited linear ubiquitin assembly complex (LUBAC) to mitochondria and disrupt MAVS signalosome, which in turn disrupts IFN-I synthesis (Khan et al., 2016). Viral proteins also play important roles in the induction of mitophagy and the inhibition of IFN-I. EBV uses its own encoded protein BHRF1 to act on mitochondria to control innate immunity. BHRF1 recruited DNM1L to induce mitochondrial fission, which forms small particles and captured by autolysosome, rendering the innate immune signaling adaptor MAVS degraded and IFN-fission (Bekisz et al., 2010). HPIV3 matrix protein (M) and IAV matrix protein PB1-F2 induced mitophagy by interacting with autophagy receptor TUFM on mitochondria and LC3, which in turn inhibited IFN-I secretion (Alymova et al., 2014; Ding et al., 2014). HHV-8 vIRF-1 protein and SARS-CoV-2 ORF10 protein activated mitophagy by binding to the autophagy receptor Nix on mitochondria (Vo et al., 2019; Li et al., 2022), which leads to MAVS degradation, attenuates the ability of the innate antiviral response, and promotes viral self-replication (Shi et al., 2020).
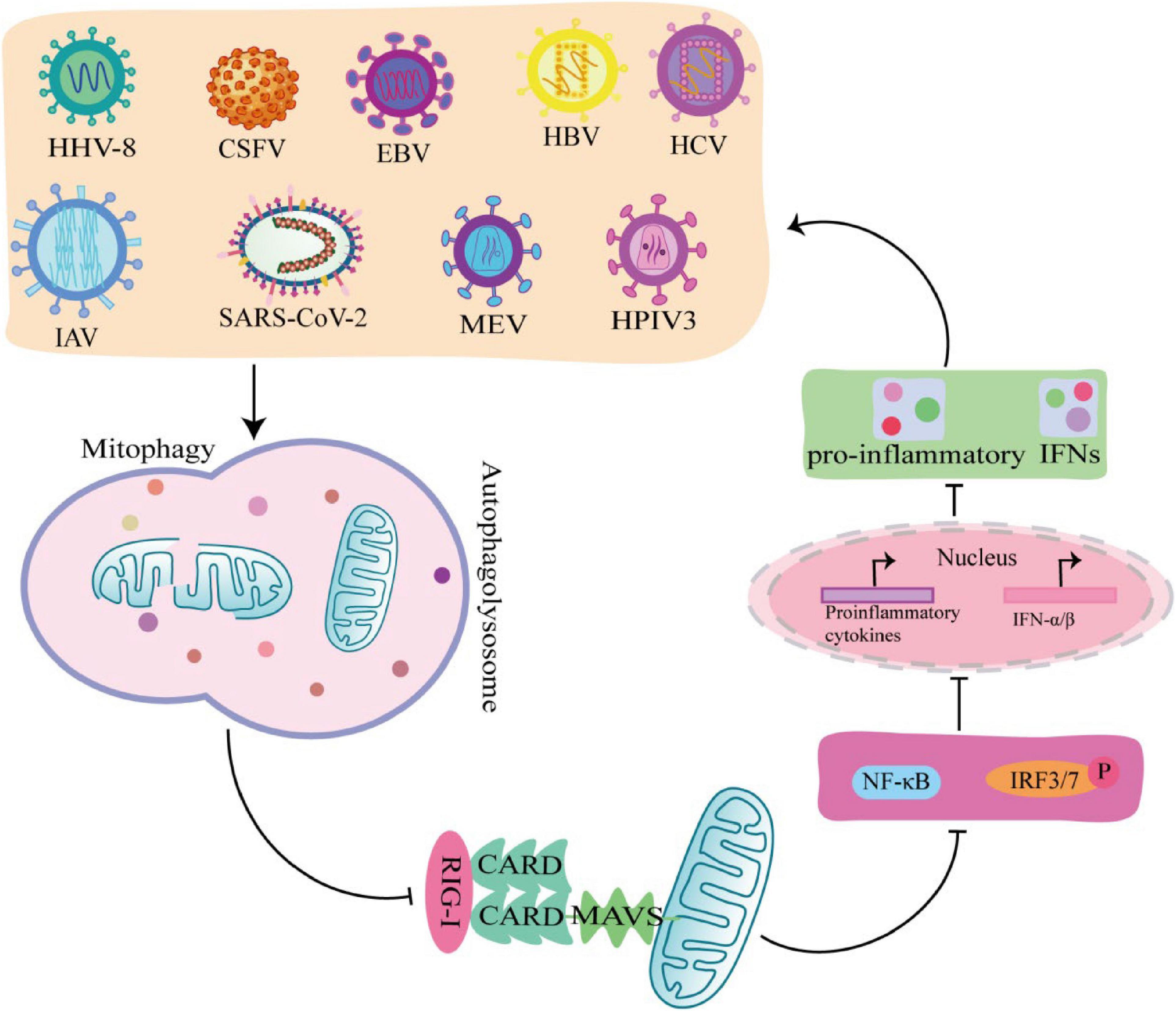
Figure 4. Viruses promote self-replication by suppressing innate immunity through mitophagy. MeV, EBV, HCV, HPIV3, IAV, HHV-8, SARS-COV-2, Hepatitis B virus (HBV), and classical swine fever virus (CSFV) induce mitophagy in different ways, rendering the innate immune signal transduction adaptor Mitochondrial antiviral-signaling protein (MAVS) degradation, which in turn inhibits the secretion of IFN-I and promotes viral self-replication.
6.2 Viruses promote self-replication by triggering mitophagy to suppress inflammation
NOD-like receptors protein 3, one of the PRRs receptors, acts as a sensor protein that, upon activation, induces the secretion of IL-1β and IL-18 and initiates the death of proinflammatory cells. Accumulating evidence showed that mitophagy plays an important role in NLRP3 inflammasome activation (Kim et al., 2016). Study has shown that some viruses inhibit the activation of the NLRP3 inflammasome by inducing mitophagy, thereby helping the virus to escape host immune defenses (Zhang et al., 2018). For example, MeV activates NLRP3 and induces IL-1β via mitophagy in THP-1 cells, whereas MeV non-structural V protein decrease NLRP3 and IL-1β through mitophagy (Komune et al., 2011). IAV infection phosphorylated the mitophagy-inducing factor unc-51 like autophagy activating kinase 1 (ULK1), which triggers serine/threonine kinase 2 (RIPK2)-mediated mitophagy for negatively regulating NLRP3 signaling pathway, and finally reduced the antiviral immunity (Lupfer et al., 2013; Chen and Takeshi, 2015). However, the links between virus induced mitophagy and inflammasome signaling are still unclear, and mining the connections among them would be a major breakthrough.
6.3 Viruses promote self-replication by triggering mitophagy to inhibit apoptosis
Apoptosis is a way in which the host defends against viral infection. Immune evasion and inhibition of apoptosis are required for successful virus infection. Interestingly, inhibition of apoptosis reduces the antiviral immune response and thus supports viral infection. Accordingly, viruses are also constantly evolving various ways to regulate apoptosis for their replication. It had shown that MeV triggers mitophagy to prevent apoptosis and cause damage to the organism (Djavaheri-Mergny et al., 2010). Edmonston B (MV-Edm) infection induced mitophagy and reduced the release of cytochrome c (CYCS) to the cytoplasm and thus blocked the pro-apoptotic cascade, thereby maintaining MeV replication in non-small lung cancer cell (Yang et al., 2010; Xia et al., 2014b). In addition, HBV, HCV, VEEV, CSFV, PRRSV, NDV, and TGEV all can induce mitophagy through different mechanisms and inhibit apoptosis for persistent infection (Kim et al., 2013a,b; Meng et al., 2014; Li et al., 2016; Zhu et al., 2016; Gou et al., 2017; Keck et al., 2017). On the contrary, some studies have shown that in Vero cells, WNV with low infection can trigger the mitochondrial mediated apoptosis pathway, which is initiated by the release of Cyt-c and the formation of apoptosome (Chu and Ng, 2003; Pan et al., 2021). It was found that WNV infection induce LC3 lipidation and the formation of LC3-labeled autophagic vacuoles in Vero cells, but the detailed mechanism is still unclear.
6.4 Viruses promote self-replication by inhibiting mitophagy to regulate inflammation
Studies have shown that most viruses increase mitophagy to evade the innate immune response (Zhang et al., 2018). But there are also studies found that some flaviviruses inhibit mitophagy to promote viral invasion into tissues. For example, Zika virus (ZIKV) inhibits mitophagy to maintain damaged mitochondria in cells and amplifies inflammatory signaling cascades. ZIKV NS5 protein antagonizes mitophagy by preventing its translocation onto depolarized mitochondria through binding to host protein Ajuba (Jia et al., 2020). ZIKV antagonizes mitophagy, which in turn causes specific chemokine amplification and thus increases DAMP signaling, increasing the spread of the virus to tissues (Ponia et al., 2021).
7 Viruses disrupt innate immunity by affecting mitochondrial dynamics
Some viruses disrupt innate immunity by affecting mitochondrial dynamics, which in turn promotes self-replication. For example, in DENV infection, DENV NS4B (non-structural protein 4B) and DENV protease NS2B3 disrupt innate immunity by perturbing mitochondrial dynamics (Strack and Cribbs, 2012; Yu et al., 2015). DENV NS4B induces mitochondrial elongation by inactivating DNM1L, which alters mitochondrial morphology, inhibits interferon production, and promotes DENV replication. DENV NS2B3, a protease essential for viral protein processing. Studies have shown that DENV NS2B3 has two novel cellular targets, MFN1 and MFN2. DENV NS2B3 impairs mitochondrial dynamics and impairs IFN responses by targeting MFNS, ultimately promoting DENV replication and inducing cell death (Yu et al., 2015).
8 Conclusion and perspectives
During viral infection, mitochondrial dynamics, autophagy activity and innate immune response of host cells are altered to promote viral replication. Revealing the mechanisms of mitochondria and innate immunity in host cells during virus infection will provide new strategies and new targets for antiviral drugs. At present, although some roles of mitophagy in antiviral immunity have been revealed, many questions remain unexplained and remain to be explored. Future studies need to explore more links of viral replication and mitophagy, particularly between viral proteins and host mitophagy specific proteins. Exploring the mechanism of mitophagy under viral infection and discovering the corresponding targets will provide new therapeutic strategies for combating viral infection.
Author contributions
CF and ZY: writing—original draft preparation. ZZ and SF: writing—review and editing. NC and WL: conceptualization, supervision, and project administration. WZ: contributed to the conception and design of the work. CF and SF: contributed to the topic selection, intention, and grammar editing. All authors have read and agreed to the published version of the manuscript.
Funding
This work was supported by grants from the Guangdong “Climbing” Program (No. KG210310110), the Program of National Natural Science Foundation of China (Nos. 32172824 and 32102643), and Outstanding Young Talents Project of South China Agricultural University.
Conflict of interest
The authors declare that the research was conducted in the absence of any commercial or financial relationships that could be construed as a potential conflict of interest.
Publisher’s note
All claims expressed in this article are solely those of the authors and do not necessarily represent those of their affiliated organizations, or those of the publisher, the editors and the reviewers. Any product that may be evaluated in this article, or claim that may be made by its manufacturer, is not guaranteed or endorsed by the publisher.
References
Aarreberg, L. D., Esser-Nobis, K., Driscoll, C., Shuvarikov, A., Roby, J. A., and Gale, M. J. (2019). Interleukin-1beta induces mtDNA release to activate innate immune signaling via cGAS-STING. Mol. Cell 74, 801–815. doi: 10.1016/j.molcel.2019.02.038
Agostini, L., Martinon, F., Burns, K., McDermott, M. F., Hawkins, P. N., and Tschopp, J. (2004). NALP3 forms an IL-1beta-processing inflammasome with increased activity in muckle-wells autoinflammatory disorder. Immunity 20, 319–325. doi: 10.1016/s1074-7613(04)00046-9
Alexopoulou, L., Holt, A. C., Medzhitov, R., and Flavell, R. A. (2001). Recognition of double-stranded RNA and activation of NF-kappab by toll-like receptor 3. Nature 413, 732–738. doi: 10.1038/35099560
Allen, I. C., Scull, M. A., Moore, C. B., Holl, E. K., McElvania-TeKippe, E., Taxman, D. J., et al. (2009). The NLRP3 inflammasome mediates in vivo innate immunity to influenza a virus through recognition of viral RNA. Immunity 30, 556–565. doi: 10.1016/j.immuni.2009.02.005
Alymova, I. V., Samarasinghe, A., Vogel, P., Green, A. M., Weinlich, R., and McCullers, J. A. (2014). A novel cytotoxic sequence contributes to influenza A viral protein PB1-F2 pathogenicity and predisposition to secondary bacterial infection. J. Virol. 88, 503–515. doi: 10.1128/JVI.01373-13
Arnoult, D., Soares, F., Tattoli, I., and Girardin, S. E. (2011). Mitochondria in innate immunity. EMBO Rep. 12, 901–910. doi: 10.1038/embor.2011.157
Bai, X., Sui, C., Liu, F., Chen, T., Zhang, L., Zheng, Y., et al. (2022). The protein arginine methyltransferase PRMT9 attenuates MAVS activation through arginine methylation. Nat. Commun. 13:5016. doi: 10.1038/s41467-022-32628-y
Beatman, E., Oyer, R., Shives, K. D., Hedman, K., Brault, A. C., Tyler, K. L., et al. (2012). West Nile virus growth is independent of autophagy activation. Virology 433, 262–272. doi: 10.1016/j.virol.2012.08.016
Bekisz, J., Baron, S., Balinsky, C., Morrow, A., and Zoon, K. C. (2010). Antiproliferative properties of type I and type II interferon. Pharmaceuticals (Basel) 3, 994–1015. doi: 10.3390/ph3040994
Bieback, K., Lien, E., Klagge, I. M., Avota, E., Schneider-Schaulies, J., Duprex, W. P., et al. (2002). Hemagglutinin protein of wild-type measles virus activates toll-like receptor 2 signaling. J. Virol. 76, 8729–8736. doi: 10.1128/jvi.76.17.8729-8736.2002
Birgisdottir, A. B., Lamark, T., and Johansen, T. (2013). The LIR motif - crucial for selective autophagy. J. Cell Sci. 126(Pt 15), 3237–3247. doi: 10.1242/jcs.126128
Borowski, L. S., Dziembowski, A., Hejnowicz, M. S., Stepien, P. P., and Szczesny, R. J. (2013). Human mitochondrial RNA decay mediated by PNPase-hsuv3 complex takes place in distinct foci. Nucleic Acids Res. 41, 1223–1240. doi: 10.1093/nar/gks1130
Bożek, K., and Lengauer, T. (2010). Positive selection of HIV host factors and the evolution of Lentivirus genes. BMC Evol. Biol. 10:186. doi: 10.1186/1471-2148-10-186
Brennan, K., and Bowie, A. G. (2010). Activation of host pattern recognition receptors by viruses. Curr. Opin. Microbiol. 13, 503–507. doi: 10.1016/j.mib.2010.05.007
Caielli, S., Athale, S., Domic, B., Murat, E., Chandra, M., Banchereau, R., et al. (2016). Oxidized mitochondrial nucleoids released by neutrophils drive type I interferon production in human lupus. J. Exp. Med. 213, 697–713. doi: 10.1084/jem.20151876
Castanier, C., Garcin, D., Vazquez, A., and Arnoult, D. (2010). Mitochondrial dynamics regulate the RIG-I-like receptor antiviral pathway. EMBO Rep. 11, 133–138. doi: 10.1038/embor.2009.258
Chan, N. C., Salazar, A. M., Pham, A. H., Sweredoski, M. J., Kolawa, N. J., Graham, R. L., et al. (2011). Broad activation of the ubiquitin-proteasome system by Parkin is critical for mitophagy. Hum. Mol. Genet. 20, 1726–1737. doi: 10.1093/hmg/ddr048
Chen, I. Y., and Takeshi, I. (2015). Response of host inflammasomes to viral infection. Trends Microbiol. 23, 55–63.
Chen, Z., Liu, L., Cheng, Q., Li, Y., Wu, H., Zhang, W., et al. (2017). Mitochondrial E3 ligase MARCH5 regulates FUNDC1 to fine-tune hypoxic mitophagy. EMBO Rep. 18, 495–509. doi: 10.15252/embr.201643309
Chiang, J. J., Davis, M. E., and Gack, M. U. (2014). Regulation of RIG-I-like receptor signaling by host and viral proteins. Cytokine Growth Factor Rev. 25, 491–505. doi: 10.1016/j.cytogfr.2014.06.005
Chu, J. J. H., and Ng, M. L. (2003). The mechanism of cell death during west Nile virus infection is dependent on initial infectious dose. J Gen. Virol. 84(Pt 12), 3305–3314. doi: 10.1099/vir.0.19447-0
Cosentino, K., Hertlein, V., Jenner, A., Dellmann, T., Gojkovic, M., Pena-Blanco, A., et al. (2022). The interplay between BAX and BAK tunes apoptotic pore growth to control mitochondrial-dna-mediated inflammation. Mol. Cell 82, 933–949.e9. doi: 10.1016/j.molcel.2022.01.008
Deng, L., Wang, C., Spencer, E., Yang, L., Braun, A., You, J., et al. (2000). Activation of the IkappaB kinase complex by TRAF6 requires a dimeric ubiquitin-conjugating enzyme complex and a unique polyubiquitin chain. Cell 103, 351–361. doi: 10.1016/s0092-8674(00)00126-4
Dhir, A., Dhir, S., Borowski, L. S., Jimenez, L., Teitell, M., Rotig, A., et al. (2018). Mitochondrial double-stranded RNA triggers antiviral signalling in humans. Nature 560, 238–242. doi: 10.1038/s41586-018-0363-0
Diebold, S. S., Kaisho, T., Hemmi, H., Akira, S., and Reis, E. S. C. (2004). Innate antiviral responses by means of TLR7-mediated recognition of single-stranded RNA. Science 303, 1529–1531. doi: 10.1126/science.1093616
Ding, B., Zhang, G., Yang, X., Zhang, S., Chen, L., Yan, Q., et al. (2014). Phosphoprotein of human parainfluenza virus type 3 blocks autophagosome-lysosome fusion to increase virus production. Cell Host Microbe 15, 564–577. doi: 10.1016/j.chom.2014.04.004
Ding, B., Zhang, L., Li, Z., Zhong, Y., Tang, Q., Qin, Y., et al. (2017). The matrix protein of human parainfluenza virus type 3 induces mitophagy that suppresses interferon responses. Cell Host Microbe 21, 538–547. doi: 10.1016/j.chom.2017.03.004
Djavaheri-Mergny, M., Maiuri, M. C., and Kroemer, G. (2010). Cross talk between apoptosis and autophagy by caspase-mediated cleavage of Beclin 1. Oncogene 29, 1717–1719.
Duguay, B. A., and Smiley, J. R. (2013). Mitochondrial nucleases ENDOG and EXOG participate in mitochondrial DNA depletion initiated by herpes simplex virus 1 UL12.5. J. Virol. 87, 11787–11797. doi: 10.1128/JVI.02306-13
Fan, S., Wu, K., Luo, C., Li, X., Zhao, M., Song, D., et al. (2019). Dual NDP52 function in persistent CSFV infection. Front. Microbiol. 10:2962. doi: 10.3389/fmicb.2019.02962
Fu, C., Ye, S., Liu, Y., and Li, S. (2020). Role of CARD region of MDA5 gene in canine influenza virus infection. Viruses 12:307. doi: 10.3390/v12030307
Fu, J., Hu, F., Ma, T., Zhao, W. J., Tian, H., Zhang, Y., et al. (2022). A conventional immune regulator mitochondrial antiviral signaling protein blocks hepatic steatosis by maintaining mitochondrial homeostasis. Hepatology 75, 403–418. doi: 10.1002/hep.32126
Gou, H., Zhao, M., Xu, H., Yuan, J., He, W., Zhu, M., et al. (2017). CSFV induced mitochondrial fission and mitophagy to inhibit apoptosis. Oncotarget 8, 39382–39400. doi: 10.18632/oncotarget.17030
Hanada, T., Noda, N. N., Satomi, Y., Ichimura, Y., Fujioka, Y., Takao, T., et al. (2007). The Atg12-Atg5 conjugate has a novel E3-like activity for protein lipidation in autophagy. J. Biol. Chem. 282, 37298–37302. doi: 10.1074/jbc.C700195200
Hanna, R. A., Quinsay, M. N., Orogo, A. M., Giang, K., Rikka, S., and Gustafsson, A. B. (2012). Microtubule-associated protein 1 light chain 3 (LC3) interacts with Bnip3 protein to selectively remove endoplasmic reticulum and mitochondria via autophagy*. J. Biol. Chem. 287, 19094–19104. doi: 10.1074/jbc.M111.322933
Harper, J. W., Ordureau, A., and Heo, J. (2018). Building and decoding ubiquitin chains for mitophagy. Nat. Rev. Mol. Cell Biol. 19, 93–108. doi: 10.1038/nrm.2017.129
Huang, S., Li, Z., Wu, Z., Liu, C., Yu, M., Wen, M., et al. (2021). Ddah2 suppresses RLR-MAVS-mediated innate antiviral immunity by stimulating nitric oxide-activated, Drp1-induced mitochondrial fission. Sci. Signal. 14:eabc7931. doi: 10.1126/scisignal.abc7931
Jabir, M. S., Hopkins, L., Ritchie, N. D., Ullah, I., Bayes, H. K., Li, D., et al. (2015). Mitochondrial damage contributes to Pseudomonas aeruginosa activation of the inflammasome and is downregulated by autophagy. Autophagy 11, 166–182. doi: 10.4161/15548627.2014.981915
Janssens, S., and Beyaert, R. (2003). Functional diversity and regulation of different interleukin-1 receptor-associated kinase (IRAK) family members. Mol. Cell 11, 293–302. doi: 10.1016/s1097-2765(03)00053-4
Jassey, A., Liu, C. H., Changou, C. A., Richardson, C. D., Hsu, H. Y., and Lin, L. T. (2019). Hepatitis C virus non-structural protein 5A (NS5A) disrupts mitochondrial dynamics and induces mitophagy. Cells 8:290. doi: 10.3390/cells8040290
Jia, H., Peng, H., and Hou, Z. (2020). Ajuba: An emerging signal transducer in oncogenesis. Pharmacol. Res. 151:104546. doi: 10.1016/j.phrs.2019.104546
Jia, J., Fu, J., and Tang, H. (2021). Activation and evasion of RLR signaling by DNA virus infection. Front. Microbiol. 12:804511. doi: 10.3389/fmicb.2021.804511
Kane, L. A., Lazarou, M., Fogel, A. I., Li, Y., Yamano, K., Sarraf, S. A., et al. (2014). PINK1 phosphorylates ubiquitin to activate parkin E3 ubiquitin ligase activity. J. Cell Biol. 205, 143–153. doi: 10.1083/jcb.201402104
Keck, F., Brooks-Faulconer, T., Lark, T., Ravishankar, P., Bailey, C., Salvador-Morales, C., et al. (2017). Altered mitochondrial dynamics as a consequence of Venezuelan Equine encephalitis virus infection. Virulence 8, 1849–1866. doi: 10.1080/21505594.2016.1276690
Khan, M., Syed, G. H., Kim, S. J., and Siddiqui, A. (2016). Hepatitis B virus-induced parkin-dependent recruitment of linear ubiquitin assembly complex (LUBAC) to mitochondria and attenuation of innate immunity. PLoS Pathog. 12:e1005693. doi: 10.1371/journal.ppat.1005693
Kim, M. J., Yoon, J. H., and Ryu, J. H. (2016). Mitophagy: A balance regulator of NLRP3 inflammasome activation. BMB Rep. 49, 529–535. doi: 10.5483/bmbrep.2016.49.10.115
Kim, S. J., Khan, M., Quan, J., Till, A., Subramani, S., and Siddiqui, A. (2013a). Hepatitis B virus disrupts mitochondrial dynamics: Induces fission and mitophagy to attenuate apoptosis. PLoS Pathog. 9:e1003722. doi: 10.1371/journal.ppat.1003722
Kim, S. J., Syed, G. H., and Siddiqui, A. (2013b). Hepatitis C virus induces the mitochondrial translocation of Parkin and subsequent mitophagy. PLoS Pathog. 9:e1003285. doi: 10.1371/journal.ppat.1003285
Kim, S. J., Syed, G. H., Khan, M., Chiu, W. W., Sohail, M. A., Gish, R. G., et al. (2014). Hepatitis C virus triggers mitochondrial fission and attenuates apoptosis to promote viral persistence. Proc. Natl. Acad. Sci. U.S.A. 111, 6413–6418. doi: 10.1073/pnas.1321114111
Komune, N., Ichinohe, T., Ito, M., and Yanagi, Y. (2011). Measles virus V protein inhibits NLRP3 inflammasome-mediated interleukin-1beta secretion. J. Virol. 85, 13019–13026. doi: 10.1128/JVI.05942-11
Kong, F., You, H., Zheng, K., Tang, R., and Zheng, C. (2021). The crosstalk between pattern-recognition receptor signaling and calcium signaling. Int. J. Biol. Macromol. 192, 745–756. doi: 10.1016/j.ijbiomac.2021.10.014
Kurt-Jones, E. A., Popova, L., Kwinn, L., Haynes, L. M., Jones, L. P., Tripp, R. A., et al. (2000). Pattern recognition receptors TLR4 and CD14 mediate response to respiratory syncytial virus. Nat. Immunol. 1, 398–401. doi: 10.1038/80833
Lai, J. H., Wang, M. Y., Huang, C. Y., Wu, C. H., Hung, L. F., Yang, C. Y., et al. (2018). Infection with the dengue RNA virus activates TLR9 signaling in human dendritic cells. EMBO Rep. 19:e46182. doi: 10.15252/embr.201846182
Lazarou, M., Sliter, D. A., Kane, L. A., Sarraf, S. A., Wang, C., Burman, J. L., et al. (2015). The ubiquitin kinase PINK1 recruits autophagy receptors to induce mitophagy. Nature 524, 309–314. doi: 10.1038/nature14893
Li, D., and Wu, M. (2021). Pattern recognition receptors in health and diseases. Signal Transduct. Target Ther. 6:291. doi: 10.1038/s41392-021-00687-0
Li, S., Kuang, M., Chen, L., Li, Y., Liu, S., Du, H., et al. (2021). The mitochondrial protein ERAL1 suppresses RNA virus infection by facilitating rig-i-like receptor signaling. Cell Rep. 34:108631. doi: 10.1016/j.celrep.2020.108631
Li, S., Wang, J., Zhou, A., Khan, F. A., Hu, L., and Zhang, S. (2016). Porcine reproductive and respiratory syndrome virus triggers mitochondrial fission and mitophagy to attenuate apoptosis. Oncotarget 7, 56002–56012. doi: 10.18632/oncotarget.10817
Li, X., Hou, P., Ma, W., Wang, X., Wang, H., Yu, Z., et al. (2022). SARS-CoV-2 ORF10 suppresses the antiviral innate immune response by degrading MAVS through mitophagy. Cell. Mol. Immunol. 19, 67–78. doi: 10.1038/s41423-021-00807-4
Lin, J., Chen, K., Chen, W., Yao, Y., Ni, S., Ye, M., et al. (2020). Paradoxical mitophagy regulation by PINK1 and TUFm. Mol. Cell 80, 607–620. doi: 10.1016/j.molcel.2020.10.007
Lin, Q., Li, S., Jiang, N., Shao, X., Zhang, M., Jin, H., et al. (2019). PINK1-Parkin pathway of mitophagy protects against contrast-induced acute kidney injury via decreasing mitochondrial ROS and NLRP3 inflammasome activation. Redox Biol. 26:101254. doi: 10.1016/j.redox.2019.101254
Liu, L., Sakakibara, K., Chen, Q., and Okamoto, K. (2014). Receptor-mediated mitophagy in yeast and mammalian systems. Cell Res. 24, 787–795. doi: 10.1038/cr.2014.75
Liu, X., Zhu, C., Zha, H., Tang, J., Rong, F., Chen, X., et al. (2020). SIRT5 impairs aggregation and activation of the signaling adaptor MAVS through catalyzing lysine desuccinylation. EMBO J. 39:e103285. doi: 10.15252/embj.2019103285
Lupfer, C., Thomas, P. G., Anand, P. K., Vogel, P., Milasta, S., Martinez, J., et al. (2013). Receptor interacting protein kinase 2-mediated mitophagy regulates inflammasome activation during virus infection. Nat. Immunol. 14, 480–488. doi: 10.1038/ni.2563
Meng, G., Xia, M., Wang, D., Chen, A., Wang, Y., Wang, H., et al. (2014). Mitophagy promotes replication of oncolytic Newcastle disease virus by blocking intrinsic apoptosis in lung cancer cells. Oncotarget 5, 6365–6374. doi: 10.18632/oncotarget.2219
Mitoma, H., Hanabuchi, S., Kim, T., Bao, M., Zhang, Z., Sugimoto, N., et al. (2013). The DHX33 RNA helicase senses cytosolic RNA and activates the NLRP3 inflammasome. Immunity 39, 123–135. doi: 10.1016/j.immuni.2013.07.001
Montava-Garriga, L., and Ganley, I. G. (2020). Outstanding questions in mitophagy: What we do and do not know. J. Mol. Biol. 432, 206–230. doi: 10.1016/j.jmb.2019.06.032
Newby, C. M., Sabin, L., and Pekosz, A. (2007). The RNA binding domain of influenza A virus NS1 protein affects secretion of tumor necrosis factor alpha, interleukin-6, and interferon in primary murine tracheal epithelial cells. J. Virol. 81, 9469–9480. doi: 10.1128/JVI.00989-07
Oka, T., Hikoso, S., Yamaguchi, O., Taneike, M., Takeda, T., Tamai, T., et al. (2012). Mitochondrial DNA that escapes from autophagy causes inflammation and heart failure. Nature 485, 251–255. doi: 10.1038/nature10992
Pan, Y., Cheng, A., Wang, M., Yin, Z., and Jia, R. (2021). The dual regulation of apoptosis by flavivirus. Front. Microbiol. 12:654494. doi: 10.3389/fmicb.2021.654494
Pernas, L., and Scorrano, L. (2016). Mito-morphosis: Mitochondrial fusion, fission, and cristae remodeling as key mediators of cellular function. Annu. Rev. Physiol. 78, 505–531. doi: 10.1146/annurev-physiol-021115-105011
Perry, A. K., Chen, G., Zheng, D., Tang, H., and Cheng, G. (2005). The host type I interferon response to viral and bacterial infections. Cell Res. 15, 407–422. doi: 10.1038/sj.cr.7290309
Pickles, S., Vigie, P., and Youle, R. J. (2018). Mitophagy and quality control mechanisms in mitochondrial maintenance. Curr. Biol. 28, R170–R185. doi: 10.1016/j.cub.2018.01.004
Ponia, S. S., Robertson, S. J., McNally, K. L., Subramanian, G., Sturdevant, G. L., Lewis, M., et al. (2021). Mitophagy antagonism by ZIKV reveals Ajuba as a regulator of PINK1 signaling, PKR-dependent inflammation, and viral invasion of tissues. Cell Rep. 37:109888. doi: 10.1016/j.celrep.2021.109888
Rambold, A. S., Kostelecky, B., Elia, N., and Lippincott-Schwartz, J. (2011). Tubular network formation protects mitochondria from autophagosomal degradation during nutrient starvation. Proc. Natl. Acad. Sci. U.S.A. 108, 10190–10195. doi: 10.1073/pnas.1107402108
Riley, J. S., and Tait, S. W. (2020). Mitochondrial DNA in inflammation and immunity. EMBO Rep. 21:e49799. doi: 10.15252/embr.201949799
Shi, C. S., Nabar, N. R., Huang, N. N., and Kehrl, J. H. (2019). SARS-Coronavirus open reading frame-8b triggers intracellular stress pathways and activates NLRP3 inflammasomes. Cell Death Discov. 5:101. doi: 10.1038/s41420-019-0181-7
Shi, R. Y., Zhu, S. H., Li, V., Gibson, S. B., Xu, X. S., and Kong, J. M. (2014). BNIP3 interacting with LC3 triggers excessive mitophagy in delayed neuronal death in stroke. CNS Neurosci. Ther. 20, 1045–1055. doi: 10.1111/cns.12325
Shi, Y., Wu, J., Zhong, T., Zhu, W., She, G., Tang, H., et al. (2020). Upstream ORFs prevent MAVS spontaneous aggregation and regulate innate immune homeostasis. iScience 23:101059. doi: 10.1016/j.isci.2020.101059
Sin, J., McIntyre, L., Stotland, A., Feuer, R., and Gottlieb, R. A. (2017). Coxsackievirus B escapes the infected cell in ejected mitophagosomes. J. Virol. 91:e01347-17. doi: 10.1128/JVI.01347-17
Strack, S., and Cribbs, J. T. (2012). Allosteric modulation of DRP1 mechanoenzyme assembly and mitochondrial fission by the variable domain. J. Biol. Chem. 287, 10990–11001. doi: 10.1074/jbc.M112.342105
Tang, M. Y., Vranas, M., Krahn, A. I., Pundlik, S., Trempe, J. F., and Fon, E. A. (2017). Structure-guided mutagenesis reveals a hierarchical mechanism of Parkin activation. Nat. Commun. 8:14697. doi: 10.1038/ncomms14697
Teresak, P., Lapao, A., Subic, N., Boya, P., Elazar, Z., and Simonsen, A. (2022). Regulation of PRKN-independent mitophagy. Autophagy 18, 24–39. doi: 10.1080/15548627.2021.1888244
Thompson, M. R., Kaminski, J. J., Kurt-Jones, E. A., and Fitzgerald, K. A. (2011). Pattern recognition receptors and the innate immune response to viral infection. Viruses 3, 920–940. doi: 10.3390/v3060920
Vo, M. T., Smith, B. J., Nicholas, J., and Choi, Y. B. (2019). Activation of NIX-mediated mitophagy by an interferon regulatory factor homologue of human Herpesvirus. Nat. Commun. 10:3203. doi: 10.1038/s41467-019-11164-2
Wang, C., Deng, L., Hong, M., Akkaraju, G. R., Inoue, J., and Chen, Z. J. (2001). TAK1 is a ubiquitin-dependent kinase of MKK and IKK. Nature 412, 346–351. doi: 10.1038/35085597
Wang, H., Zheng, Y., Huang, J., and Li, J. (2021). Mitophagy in antiviral immunity. Front. Cell. Dev. Biol. 9:723108. doi: 10.3389/fcell.2021.723108
Wang, K., Ma, H., Liu, H., Ye, W., Li, Z., Cheng, L., et al. (2019). The glycoprotein and nucleocapsid protein of hantaviruses manipulate autophagy flux to restrain host innate immune responses. Cell Rep. 27, 2075–2091. doi: 10.1016/j.celrep.2019.04.061
Wang, R., Zhu, Y., Ren, C., Yang, S., Tian, S., Chen, H., et al. (2021). Influenza A virus protein PB1-F2 impairs innate immunity by inducing mitophagy. Autophagy 17, 496–511. doi: 10.1080/15548627.2020.1725375
Wauer, T., Simicek, M., Schubert, A., and Komander, D. (2015). Mechanism of phospho-ubiquitin-induced Parkin activation. Nature 524, 370–374. doi: 10.1038/nature14879
Weber, F., Wagner, V., Rasmussen, S. B., Hartmann, R., and Paludan, S. R. (2006). Double-stranded RNA is produced by positive-strand RNA viruses and DNA viruses but not in detectable amounts by negative-strand RNA viruses. J. Virol. 80, 5059–5064.
Wei, Y., Chiang, W. C., Sumpter, R. J., Mishra, P., and Levine, B. (2017). Prohibitin 2 is an inner mitochondrial membrane mitophagy receptor. Cell 168, 224–238. doi: 10.1016/j.cell.2016.11.042
West, A. P., Khoury-Hanold, W., Staron, M., Tal, M. C., Pineda, C. M., Lang, S. M., et al. (2015). Mitochondrial DNA stress primes the antiviral innate immune response. Nature 520, 553–557. doi: 10.1038/nature14156
White, M. J., McArthur, K., Metcalf, D., Lane, R. M., Cambier, J. C., Herold, M. J., et al. (2014). Apoptotic caspases suppress mtDNA-induced STING-mediated type I IFN production. Cell 159, 1549–1562. doi: 10.1016/j.cell.2014.11.036
Wiatrek, D. M., Candela, M. E., Sedmik, J., Oppelt, J., Keegan, L. P., and O’Connell, M. A. (2019). Activation of innate immunity by mitochondrial dsRNA in mouse cells lacking p53 protein. RNA 25, 713–726. doi: 10.1261/rna.069625.118
Xia, M., Gonzalez, P., Li, C., Meng, G., Jiang, A., Wang, H., et al. (2014a). Mitophagy enhances oncolytic measles virus replication by mitigating DDX58/RIG-I-like receptor signaling. J. Virol. 88, 5152–5164. doi: 10.1128/JVI.03851-13
Xia, M., Meng, G., Jiang, A., Chen, A., Dahlhaus, M., Gonzalez, P., et al. (2014b). Mitophagy switches cell death from apoptosis to necrosis in NSCLC cells treated with oncolytic measles virus. Oncotarget 5, 3907–3918. doi: 10.18632/oncotarget.2028
Xian, H., Liu, Y., Rundberg, N. A., Gatchalian, R., Crother, T. R., Tourtellotte, W. G., et al. (2021). Metformin inhibition of mitochondrial ATP and DNA synthesis abrogates NLRP3 inflammasome activation and pulmonary inflammation. Immunity 54, 1463–1477. doi: 10.1016/j.immuni.2021.05.004
Xie, L., Shi, F., Li, Y., Li, W., Yu, X., Zhao, L., et al. (2020). Drp1-dependent remodeling of mitochondrial morphology triggered by EBV-LMP1 increases cisplatin resistance. Signal Transduct. Target Ther. 5:56. doi: 10.1038/s41392-020-0151-9
Xing, J., Zhou, X., Fang, M., Zhang, E., Minze, L. J., and Zhang, Z. (2021). DHX15 is required to control RNA virus-induced intestinal inflammation. Cell Rep. 35:109205. doi: 10.1016/j.celrep.2021.109205
Xu, Y., Tang, Y., Lu, J., Zhang, W., Zhu, Y., Zhang, S., et al. (2020). PINK1-mediated mitophagy protects against hepatic ischemia/reperfusion injury by restraining NLRP3 inflammasome activation. Free Radic. Biol. Med. 160, 871–886. doi: 10.1016/j.freeradbiomed.2020.09.015
Yang, Y., Xing, D., Zhou, F., and Chen, Q. (2010). Mitochondrial autophagy protects against heat shock-induced apoptosis through reducing cytosolic cytochrome c release and downstream caspase-3 activation. Biochem. Biophys. Res. Commun. 395, 190–195. doi: 10.1016/j.bbrc.2010.03.155
Yoshizumi, T., Ichinohe, T., Sasaki, O., Otera, H., Kawabata, S., Mihara, K., et al. (2014). Influenza A virus protein PB1-F2 translocates into mitochondria via Tom40 channels and impairs innate immunity. Nat. Commun. 5:4713. doi: 10.1038/ncomms5713
Yu, C. Y., Liang, J. J., Li, J. K., Lee, Y. L., Chang, B. L., Su, C. I., et al. (2015). Dengue virus impairs mitochondrial fusion by cleaving mitofusins. PLoS Pathog. 11:e1005350. doi: 10.1371/journal.ppat.1005350
Zhang, E., Fang, M., Jones, C., Minze, L. J., Xing, J., and Zhang, Z. (2022). Mechanisms involved in controlling RNA virus-induced intestinal inflammation. Cell. Mol. Life Sci. 79:313. doi: 10.1007/s00018-022-04332-z
Zhang, L., Qin, Y., and Chen, M. (2018). Viral strategies for triggering and manipulating mitophagy. Autophagy 14, 1665–1673. doi: 10.1080/15548627.2018.1466014
Zhang, Q., Itagaki, K., and Hauser, C. J. (2010). Mitochondrial DNA is released by shock and activates neutrophils via p38 map kinase. Shock 34, 55–59. doi: 10.1097/SHK.0b013e3181cd8c08
Zheng, Y., Liu, Q., Wu, Y., Ma, L., Zhang, Z., Liu, T., et al. (2018). Zika virus elicits inflammation to evade antiviral response by cleaving cGAS via NS1-caspase-1 axis. Embo J. 37:e99347. doi: 10.15252/embj.201899347
Zhou, L., He, R., Fang, P., Li, M., Yu, H., Wang, Q., et al. (2021). Hepatitis B virus RIGS the cellular metabolome to avoid innate immune recognition. Nat. Commun. 12:98. doi: 10.1038/s41467-020-20316-8
Zhu, L., Mou, C., Yang, X., Lin, J., and Yang, Q. (2016). Mitophagy in TGEV infection counteracts oxidative stress and apoptosis. Oncotarget 7, 27122–27141. doi: 10.18632/oncotarget.8345
Keywords: mitophagy, mitochondria, innate immunity, viral infection, mechanisms
Citation: Fu C, Cao N, Liu W, Zhang Z, Yang Z, Zhu W and Fan S (2022) Crosstalk between mitophagy and innate immunity in viral infection. Front. Microbiol. 13:1064045. doi: 10.3389/fmicb.2022.1064045
Received: 07 October 2022; Accepted: 29 November 2022;
Published: 16 December 2022.
Edited by:
Saurabh Chattopadhyay, The University of Toledo, United StatesReviewed by:
Junji Xing, Houston Methodist Research Institute, United StatesGayatri Subramanian, National Institute of Allergy and Infectious Diseases (NIH), United States
Siddhesh Aras, Wayne State University, United States
Copyright © 2022 Fu, Cao, Liu, Zhang, Yang, Zhu and Fan. This is an open-access article distributed under the terms of the Creative Commons Attribution License (CC BY). The use, distribution or reproduction in other forums is permitted, provided the original author(s) and the copyright owner(s) are credited and that the original publication in this journal is cited, in accordance with accepted academic practice. No use, distribution or reproduction is permitted which does not comply with these terms.
*Correspondence: Wenhui Zhu, NDEzMTM4NTVAcXEuY29t; Shuangqi Fan, c2hxZmFuQHNjYXUuZWR1LmNu