- 1Grupo de Ecofisiología Térmica, Facultad de Ciencias Naturales y Oceanográficas, Departamento de Botánica, Universidad de Concepción, Concepción, Chile
- 2Cape Horn International Center (CHIC), Puerto Williams, Chile
- 3Rizoma, Centro de Estudios Agroecológicos y Botánicos, Valparaíso, Chile
- 4Laboratorio de Microbiología de Suelos, Departamento de Suelos y Recursos Naturales, Facultad de Agronomía, Universidad de Concepción, Concepción, Chile
- 5Laboratorio de Biopelículas y Microbiología Ambiental, Centro de Biotecnología, Universidad de Concepción, Concepción, Chile
- 6Laboratorio de Epigenética Vegetal, Facultad de Ciencias Forestales, Departamento de Silvicultura, Universidad de Concepción, Concepción, Chile
Introduction: Plants interact with plant growth-promoting bacteria (PGPB), especially under stress condition in natural and agricultural systems. Although a potentially beneficial microbiome has been found associated to plants from alpine systems, this plant- PGPB interaction has been scarcely studied. Nevados de Chillán Complex hold one of the southernmost xerophytic formations in Chile. Plant species living there have to cope with drought and extreme temperatures during the growing season period, microclimatic conditions that become harsher on equatorial than polar slopes, and where the interaction with PGPB could be key for plant survival. Our goal was to study the abundance and activity of different PGPB associated to two abundant plant species of Andean xerophytic formations on contrasting slopes.
Methods: Twenty individuals of Berberis empetrifolia and Azorella prolifera shrubs were selected growing on a north and south slope nearby Las Fumarolas, at 2,050 m elevation. On each slope, microclimate based on temperature and moisture conditions were monitored throughout the growing period (oct. – apr.). Chemical properties of the soil under plant species canopies were also characterized. Bacterial abundance was measured as Log CFU g−1 from soil samples collected from each individual and slope. Then, the most abundant bacterial colonies were selected, and different hormonal (indoleacetic acid) and enzymatic (nitrogenase, phosphatase, ACC-deaminase) mechanisms that promote plant growth were assessed and measured.
Results and Discussion: Extreme temperatures were observed in the north facing slope, recording the hottest days (41 vs. 36°C) and coldest nights (−9.9 vs. 6.6°C). Moreover, air and soil moisture were lower on north than on south slope, especially late in the growing season. We found that bacterial abundance was higher in soils on north than on south slope but only under B. empetrifolia canopy. Moreover, the activity of plant growth-promoting mechanisms varied between slopes, being on average higher on north than on south slope, but with plant species-dependent trends. Our work showed how the environmental heterogeneity at microscale in alpine systems (slope and plant species identity) underlies variations in the abundance and plant growth promoting activity of the microorganisms present under the plant canopy of the Andean xerophytic formations and highlight the importance of PGPB from harsh systems as biotechnological tools for restoration.
Introduction
Plant growth-promoting bacteria (hereafter PGPB) are a diverse group of microorganisms beneficial to free-living or endophytic plants and can inhabit different plant compartments (de Souza et al., 2015; Afzal et al., 2017). Different bacterial traits promote plant growth directly or indirectly through mechanisms that have been widely documented, especially in soil and plant rhizosphere bacteria (Gamalero and Glick, 2011; Ramakrishna et al., 2019). These mechanisms mainly include enzymatic activities, such as urease, phosphatases, β-glucosidase, catalase, and the production of bacterial phytohormones that promote plant growth (Glick, 2012). Among the most studied mechanisms are nitrogen fixation, phosphate solubilization, phytohormone production, siderophores, and enzymes activity such as ACC deaminase that promotes resistance to different stress factors (e.g., Drought, Ashry et al., 2022; salinity, Kumar et al., 2020; trace metals, Kong and Glick, 2017). For example, the bacterial enzyme nitrogenase catalyzes molecular nitrogen to ammonia, which is absorbed by the plants increasing crop yields (Franche et al., 2009). Likewise, bacteria with ACC deaminase enzyme activity can degrade 1-aminocyclopropane-1-carboxylate (ACC), an ethylene precursor, producing ammonia and α-ketobutyrate. The decrease in ethylene levels allows the plant to survive under stressful conditions (e.g., drought and salinity; Glick et al., 2007; Orozco-Mosqueda et al., 2021). Another interesting mechanism is phosphate solubilization by bacterial enzymes such as phosphatase, phytase, and C-P lyase, that solubilize organic phosphate into inorganic phosphate, which could be absorbed and used for plant metabolism (Rawat et al., 2021). Moreover, bacterial phytohormones such as indole acetic acid (IAA) stimulate plant cell division, enhancing growth in roots and aerial structures (Duca and Glick, 2020). All these bacterial mechanisms that promote plant growth depend on environmental factors (Olanrewaju et al., 2017).
The growth-promoting activity of soil PGPB is influenced by abiotic and biotic factors. For example, nitrogen fixation is favored by increasing phosphorus and carbon availability but decreases in the presence of trace metals (Liengen, 1999; Wakelin et al., 2010). Regarding phosphate solubilization, Mujahid et al. (2015) have reported that phosphate-solubilizing PGPB increase their halo in media with carbon and nitrogen sources. While Alemneh et al. (2022) correlated soil chemical properties and phosphate solubilization of PGPB, and determined that these did not benefit from soil carbon and nitrogen. We believe that the high availability of carbon and nitrogen in growing media, such as those observed by Mujahid et al. (2015) generate opposite trends in phosphate solubilization when compared to the availability of these nutrients in the soil. Moreover, the production of IAA correlates positively with the presence of heavy metals, and negatively with the availability of sugars, nitrogen, and phosphorus in the soil (Ahmad et al., 2005; Mendoza-Hernández et al., 2016; Alemneh et al., 2022). Finally, ACC-deaminase activity of several bacteria genus increases to deaminate ACC in the presence of copper, arsenic, and lead (Mendoza-Hernández et al., 2016). Alternatively, plant root exudates compound rich in sugars, which favor microbial metabolism, increasing growth-promoting activity and plant-PGPB interactions (Bais et al., 2006). In turn, root exudates releasing is also influenced by abiotic conditions to which plants and PGPB are exposed, such as soil moisture, nutrient availability, and temperature (Vives-Peris et al., 2018; Barbosa et al., 2020). For instance, the interaction between the coastal halophyte plant Limonium sinense with habitat-adapted, endophytic bacteria of the genus Bacillus favoring plant survival under salt stress and is mediated by root exudates (Xiong et al., 2020). Soil temperature and moisture have also been defined as drivers of bacterial growth-promoting activity (Rousk et al., 2018). The ideal temperature for phosphate solubilization is below 25°C (Mujahid et al., 2015), while higher temperatures coupled with higher soil acidity increase IAA production (Kanu and Dakora, 2009; Alemneh et al., 2022). Soil moisture, in turn, has been defined as an indispensable resource for the activity of nitrogen-fixing bacteria in cold climates such as the Arctic tundra (Rousk et al., 2018). Therefore, the abundance and activity of PGPB will depend on their environmental requirements and availability of resources such as those required by the plants with which they interact.
The benefits of PGPBs have been reported mainly in agricultural systems due to the global demand for food and cultivable land (Bhattacharyya and Jha, 2012; Ramakrishna et al., 2019). In contrast, the abundance and activity of PGPBs in natural systems have been scarcely explored, despite its great potential and novelty (Pérez-Jaramillo et al., 2018; Leontidou et al., 2020). For example, PGPBs associated to wild (or native plants) species have been reported in harsh environments, such as desert (Fuentes et al., 2020; Maldonado et al., 2022), alpine (Sezen et al., 2016; Adamczyk et al., 2019; Wang et al., 2020), and saline environments (Rueda-Puente et al., 2019). Additionally, some studies have explored the presence of PGPBs adapted to environments with extreme conditions, such as salinity, (e.g., PGPBs studied from the soils of Lonar Lake in India, Hingole and Pathak, 2016), drought (e.g., PGPBs from different desert regions of Egypt, Ashry et al., 2022), or low temperatures (e.g., isolated PGPBs from the Himalayas, Yadav et al., 2015). Undoubtedly, exploring “harsh” systems for different life forms offers a space to find microorganisms with great potential for plant production and restoration, due to their ability to cope with extreme conditions and to promote plant growth and survival.
Alpine systems represent a natural laboratory to explore the presence and activity of PGPBs because of their harsh climatic conditions for life (Körner, 2004; Li et al., 2020; Rahman et al., 2020). In alpine habitats, temperature, radiation, exposure, and precipitation vary with season, altitude, and exposure of slopes (Körner and Ohsawa, 2005), producing a spatial heterogeneity of environmental conditions (Siles and Margesin, 2016; Körner, 2021), which may affect from individual physiological responses to the distribution and community structure of plants (Chaturvedi and Shivaji, 2006; Rumpf et al., 2018; López-Angulo et al., 2019; Sklenář et al., 2021). Such environmental heterogeneity has been studied mainly along altitudinal gradients and contrasting slope exposures. Specifically, soils of pole-facing slopes tend to be wetter, colder and with higher organic matter than those of equatorial-facing slopes (Scherrer and Körner, 2011). These differences in environmental conditions propitiate greater plant species richness and cover in polar than equatorial facing slopes (Zeng et al., 2014; Måren et al., 2015; Viale et al., 2019). While the difference in plant diversity of high-elevation species between slopes has been widely studied (e.g., Zeng et al., 2014; Yang et al., 2020), differences for other organisms such as PGPBs have been almost unexplored. To date, bacterial diversity decreases with altitude (Adamczyk et al., 2019). Meanwhile, their relative abundance, especially in free-living psychrophiles, increases with elevation (Siles and Margesin, 2016; Rui et al., 2022). PGPBs natives to alpine systems are essential nowadays due to their ability to cope with low temperatures and to promote plant growth through different mechanisms under cold conditions and poor nutrient soils (Chaturvedi and Shivaji, 2006; Jaggi et al., 2020; Farooq et al., 2022). These constraint conditions for plants can be alleviated by PGPBs through the activity of enzymes and phytohormones that favor plant nutrient uptake and stress resistance (Haselwandter et al., 1983; Widawati and Suliasih, 2006; Joshi et al., 2014; Yarzábal, 2014; Kadioglu et al., 2018; Pandey and Yarzábal, 2019; Fan et al., 2021). Some studies have reported PGPBs in alpine ecosystems (Yadav et al., 2015), described their diversity in terms of factors structuring bacterial communities (Tang et al., 2020; Wang et al., 2020; Rui et al., 2022), and quantified their plant growth-promoting activity (Haselwandter et al., 1983; Widawati and Suliasih, 2006; Viruel et al., 2011; Yadav et al., 2015; Kadioglu et al., 2018). Although the interaction with beneficial microorganisms could be part of the adaptive strategy of plants in alpine systems (Farooq et al., 2022), little is known about their abundance and activity of PGPB in contrasting microhabitats within these natural systems.
The Nevados de Chillán volcanic complex is in a Mediterranean – Temperate climate transition zone (Arroyo et al., 2004), characterized by a complex topography (González-Ferrán, 1995; Dixon et al., 1999), and great plant diversity and endemism (Rodríguez et al., 2008). The lower portion of the alpine zone is dominated by xerophytic vegetation, which is one of the southernmost xerophytic formations in Chile (Squeo et al., 2008). Plant species of this Andean xerophytic formation must cope with low soil moisture and large-daily thermal oscillations during the snow-free period in the area, which could be even more extreme depending on slope exposure. The success of xerophytic plant species in alpine systems could be mediated by PGPBs-plant associations. However, knowledge is scarce, with only one study reporting PGPB isolated from the shrub species Parastrephia quadrangularis in the Andean Puna (Acuña et al., 2019). Thus, our general goal was to study the abundance and activity of different plant growth-promoting bacteria (PGPB) associated with two abundant plant species of Andean xerophytic formations on contrasting slopes. We expected that the abundance and activity of different PGPB associated with plant species will be greater on drier and or more thermally extreme slope.
Materials and methods
The study area was the Fumarolas sector (36°55′15”S, 71°26′25”W), nearby Nevados de Chillán Ski Resort, located at 80 km east of Chillán (Ñuble region, Chile). Is located in the transition zone between central Chile’s Mediterranean regions, with sclerophyllous and temperate forests plant elements of South Chile (Arroyo et al., 2004). This area is characterized by a past and current volcanic activity resulting in a manifold topography characterized by a complex relief and geology (González-Ferrán, 1995; Dixon et al., 1999). The combination of biogeographic situation, geomorphologic complexity, and climate change leads to an exceptional degree of botanical diversity and endemism (Rodríguez et al., 2008). In this area, the treeline is determined by Nothofagus pumilio and N. antarctica at approximately 2,100 m above sea level (Fajardo et al., 2011; Piper et al., 2016). Above there, the alpine zone comprises an elevation range from 2,100 to 2,700 m (Pfanzelt et al., 2008). Vegetation is short (<150 cm), and arranged in plant assemblages of shrubs, rosettes, grasses, and geophytes (Pfanzelt et al., 2008). For example, lower elevation portion (2100–2,300 m) comprises several shrubs (Berberis empetrifolia, Azorella prolifera) and herbaceous species (Adesmia emarginata, Grausa lateritia) assemblages, which coincides with one of the southmost xerophytic formations in Chile (Figure 1; Donoso, 1982; Squeo et al., 2001; Moreira and Cereceda, 2013). Upper elevation portion (2300–2,700 m) is dominated by herbaceous species (Viola volcanica, Nassauvia revoluta) and grasses (Bromus, Festuca, and Poa species), which forms a matrix of low plant cover between rocks and lava flows (Pfanzelt et al., 2008). In general, this Andean system is characterized by summer water shortage and great daily temperature fluctuations (Termas de Chillán climatic station, CN360042, 1708 m above sea level).1 During the snow free period, i.e., between October and April approximately, daily minimum and maximum air temperatures oscillate between −10°C to more than 41°C, respectively (Table 1), depending on slope and date. Moreover, soil water potential decrease from −0.8 MPa in October to <−2 MPa in March, especially in north-facing slopes (Figure 2).
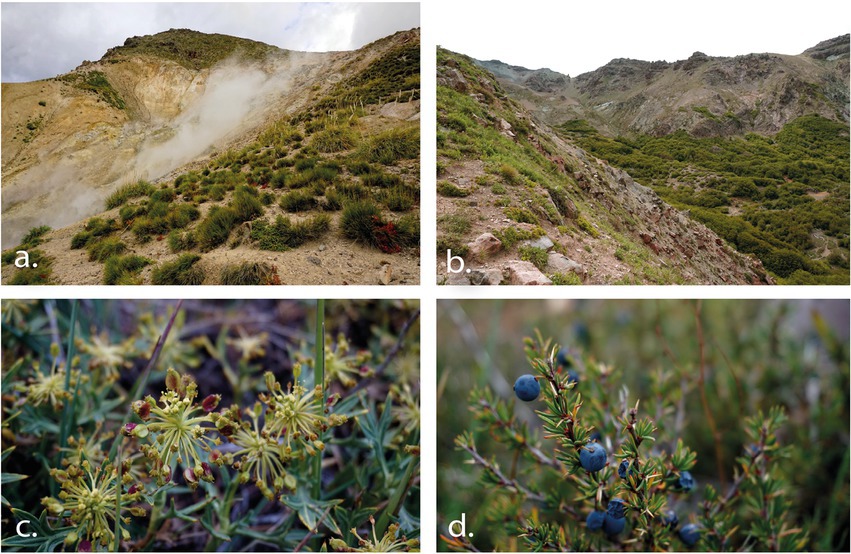
Figure 1. Study site located in the Nevados de Chillán Volcanic complex. Letters (A,B) indicate the north and south slopes, respectively. Selected species present on both slopes are indicated on the right side: (C). A. prolifera and (D). B. empetrifolia. Credits: Carla Aguilera-Torres.
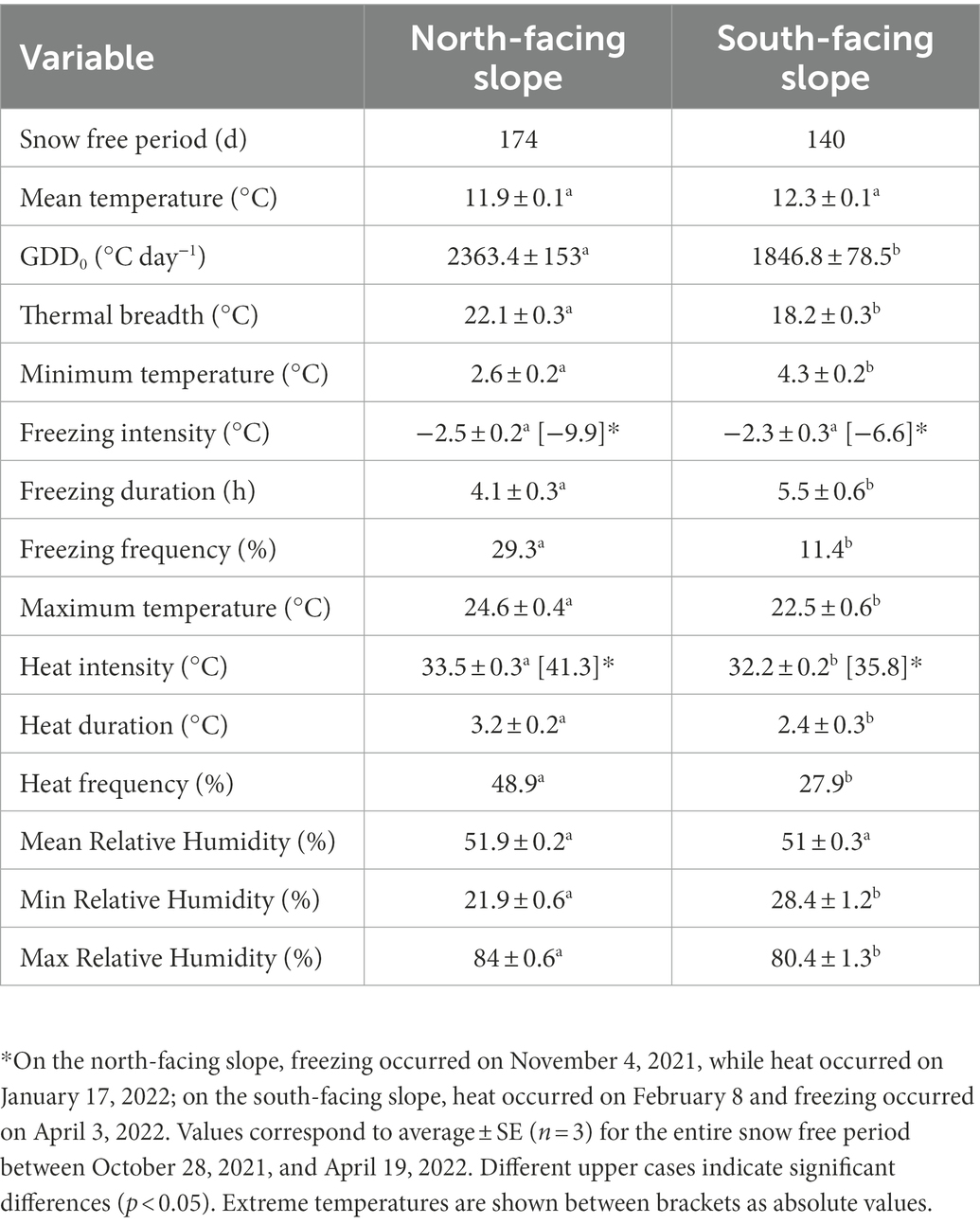
Table 1. Air temperature and moisture conditions measured on two contrasting slopes in the alpine zone of the Nevados de Chillán volcano complex.
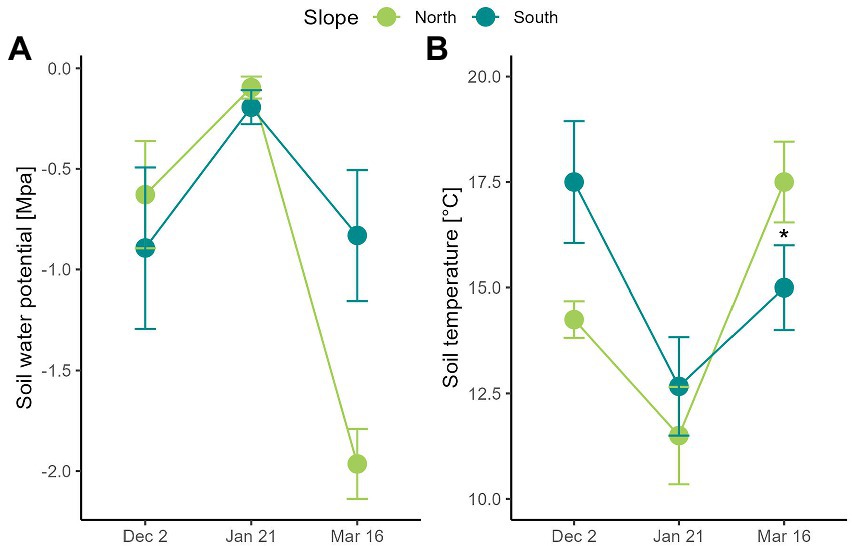
Figure 2. Soil climatic conditions measured in two contrasting slopes in the Nevados de Chillán Volcano complex. (A) Water potential (MPa) and (B) soil temperature (°C) was measured at 15 cm of soil depth on two contrasting slopes. Values correspond to average ± SE (n = 3). Dashed grey lines indicate temperature thresholds for plant physiology: heat (30°C) – freezing (0°C). Blue arrows indicate snowfall. Asterisks show significant differences (Repeated measures ANOVA, p < 0.05).
Species selection
A pre-selection of six target species was conducted, based on Herbarium data at the Universidad de Concepción (CONC, Chile). This information was crossed with the national distribution of plant xerophytic formation report elaborated by National Forestry Service, CONAF [Gestión de Recursos Naturales (GRN), 2022]. The combined information added to field observations enabled to select within the study area two dominant species in the Andean xerophytic formations: Berberis empetrifolia Lam. (Berberidaceae) and Azorella prolifera (Cav.) G.M. Plunkett & A.N. Nicolas (Apiaceae; Figure 1). B. empetrifolia is a spiny and dwarf shrub of 50 cm tall, that has small narrow entire leaves, and small, yellow-colored flowers and later freshy blue-black berries. It is distributed from 29° to 56° S along Chile and Argentina, and from sea level to 3,500 m elevation (Rodríguez et al., 2018). A. prolifera is a shrub or subshrub that is native to Argentina, Bolivia and Chile, that forms cushions up to 100 cm high, yellowish-green, sometimes glaucous, very aromatic, petiolate leaves measuring up to 50 mm long, with yellow flowers, hermaphrodite in the center and male at the edge, arranged in simple umbels protruding above the foliage, its fruit is a winged reddish-yellow schizocarp. A. prolifera is distributed from 26° to 56° S, and from o to above 1,500 m of elevation (Rodríguez et al., 2018). Both species are key for other plants recruitment in the southern Andes (Nuñez et al., 1999) and exhibit high resistance to different types of stress (Durante et al., 2011; Golluscio et al., 2011; Varas et al., 2013; Sierra-Almeida et al., 2016).
Microclimatic characterization of contrasting slopes
Target species were present in two contrasting slopes (Figure 1): north- (1,984 m, 36°54′24.25”S 71°24′10.16”W) and south-exposure (2,089 m, 36°54′16.90”S 71°23′58.83”W). On each slope, three air temperature (°C) and relative humidity (%) sensors were placed at 15 cm above the soil surface (U23 Hobo Pro v2 6′, Onset Comp, United States). They were programmed to record every 30 min during the entire snow free period. On the north-facing slope, sensors were installed on October 28, 2021, whilst on the south slope, they were installed on December 02 because it was covered by snow before this date. Air temperature recording were used to estimate several parameters: seasonal mean, maximum and minimum air temperatures; intensity, frequency, and duration of freezing (T < 0°C) and heat events (T > 30°C); growing degree days (GDDs), used as a measure of heat accumulation (in °C) above a base temperature (i.e., 0°C) to represent an index of the cumulate energy available to growing plants (McMaster and Wilhelm, 1997). It was calculated as:
GDD0 = [[(maximum daily temperature + minimum daily temperature)/2]] − base temperature.
The daily GDDs were summed per the entire growing season on each slope. We used 0°C as a conservative base growing temperature (threshold temperature above which plants can perform metabolic functions), because plants from cold climate generally vary in their absolute base growing temperature, and this value encompasses this variability (Körner, 2011).
Soil temperature and water potential were recorded by using psychrometers (PST-55, C52 Wescor Inc., Utah, United States), which were placed at 15 cm of soil depth (n = 3 per slope). These sensors were buried at the same date as air sensors. Soil temperature and moisture were manually recorded once per month using a microvoltmeter (HR 33 T; Wescor Inc., Utah, United States). In April, measurements of soil temperature and water potential were not carried out because started snowfall. All sensors were removed from the sites on April 19, 2022.
Given that soil chemical properties affect the bacterial abundance and activity (Preem et al., 2012; Chodak et al., 2015), on April 16, soil samples were collected under the plant canopy for their chemical characterization. A total of 12 soil samples of 1 kg (3 replicates × 2 species × 2 slopes) were placed into plastic bags, marked, and immediately transported into a cooler to our lab group at the University of Concepción (Concepción). Then, soil samples were sent for complete chemical analysis to the Soil and Plant Analysis Laboratory at Universidad de Concepción (Chillán).
Bacterial abundance
On April 19, soil samples were collected from surrounding roots of Azorella prolifera and Berberis empetrifolia. Three soil samples of 0,5 kg were collected per species and slope (total 12 samples). Samples soils were placed into a cooler and immediately transported to our lab, where they were stored at −20°C until the start of microbiological studies. Abundance of bacteria were estimated by Colony-Forming Unit (CFU) per species (A. prolifera and B. empetrifolia) and slope (North and South). For this, 1 g of soil per species and slope exposure was taken and serially diluted in Phosphate-Buffered Saline (PBS) medium under sterile conditions. Subsequently, the 10−2 and 10−3 dilutions were sown on plates with plate count agar medium (DIFCO), which were incubated for 2 days at 25°C. The CFU of each dilution was then counted to determine the most abundant and morphologically different culturable strains for plant growth-promoting trait assays. The CFU were transformed to their natural logarithm (Log CFU g−1).
PGPB activity
The five most abundant and distinct colonies by species and slope were selected according to their bacterial cell shape and structural appearance. They were subculture two or three times until pure strains were obtained. Nineteen culturable bacterial strains were obtained and subjected to assays that evaluated the activity of four plant growth-mechanisms, including IAA production, ACC deaminase, phosphate solubilization and nitrogenase enzyme activities and then were compared between contrasting slopes.
a. To evaluate indole acetic acid (IAA) production, selected culturable strains were inoculated in generic nutrient broth [1 gD (+)-glucose, 15 g peptone, 6 g NaCl, 3 g yeast extract], with 0.15% (w/v) tryptophan in darkness for 96 h at 30°C with 120 rpm. IAA production was measured via high-performance liquid chromatography (HPLC; Primaide, Hitachi Co, Ltd., Tokyo, Japan; Gang et al., 2019). The calibration curve was prepared with serial solutions of IAA ranging from 0 to 50 ppm in methanol, 50 μl of the samples were injected onto a kromasil C-18 column equipped with a diode array detector, and retention times ranged from 5.7 to 7.9 min. All samples were analyzed in triplicate. The results were expressed in μg ml−1 of bacterial IAA.
b. The presence and activity of ACC-deaminase enzyme was studied following protocols described by Senthilkumar et al. (2021) and Penrose and Glick (2003) with modifications. Liquid DF minimal culture medium was prepared, where the bacteria of interest were inoculated at 28°C for 72 h; bacteria that showed turbidity were seeded in solid DF minimal medium to confirm the presence of ACC deaminase. In cultures that evidenced plate growth in solid DF medium, ACC deaminase enzymatic activity was measured by the production of α-ketobutyrate, which was determined by spectrophotometer (Spectroquant Prove 300) at 540 nm by comparison with the standard curve of α-ketobutyrate, which ranged from 0 to 100 μM (Honma and Shimomura, 1978). Higher concentrations of α-ketobutyrate were indicative of higher enzyme activity (Ali et al., 2014).
c. To determine nitrogenase enzyme activity, strains were incubated in a semi-solid nitrogen-free (NF) medium for 72 h at 28°C (Kifle and Laing, 2015). Samples showing evidence of turbidity were subjected to acetylene reduction assay (ARA; Hardy et al., 1968), where 10% of the atmosphere was removed with a syringe and replaced with acetylene. After 20 h, 4.4 ml of gas was taken for each vial to analyze the amount of ethylene formed through gas chromatography (GC 6890 N, Agilent Technologies), equipped with J&W HP-5 GC column, 30 m, 0.25 mm, 0.25 μm, H₂, N₂, and air detectors, with flow rates of 40, 24, and 450 ml/min, respectively. To determine the injection, detection and column temperatures, the ranges of Hara et al. (2009) were followed with modifications: Injection temperatures were 150°C, detection temperature was 150°C, and column temperature was 50°C. Retention times ranged from 1 to 1.5 min. 4–5 ml of samples were injected per 10 min. Each sample was prepared in triplicate for injection. The calibration curve was prepared with solutions composed of different ethylene concentrations expressed in nmol C₂H₄ d−1vial−1.
d. Phosphate solubilization, mineral phosphate solubilization was assayed according to Pikovskaya (1948), the mineral phosphate solubilization was assayed by seeding bacteria in a solid medium Pikovskaya (PVK) in plates containing insoluble tricalcium phosphate, that bacteria with phosphatase are capable of degrading. The plates were incubated at 28°C. The development of a clear zone around the colony was evaluated after 20 days. Samples were analyzed in triplicate.
Identification of bacterial strains by studying the 16S rRNA gene
To determine whether the selection of bacterial strains by cellular and structural appearance included different species, the following analysis were carried out. Ten bacterial strains randomly selected of 19 used for PGPB analysis were grown on semi-solid TBS medium. DNA extractions of individual colonies were performed using the DNeasy® Plant mini kit (QIAGEN, Dusseldorf, Germany) according to the manufacturer’s instructions. The integrity of the DNA samples was visualized by agarose gel electrophoresis, the concentration was determined by spectrophotometry (A260/A280) using Infinite® M200 Pro NanoQuant (Tecan®, Tecan Trading AG, Switzerland) and the DNA samples were kept at −20°C. PCR was performed to amplify and sequence part of the 16S rRNA gene. PCR reactions contained 1X GoTaq® reaction buffer (1.5 mM MgCl2), 200 mM dNTP, 0 2 μM of each primer (16S_27F, 5’-AGAGAGTTTGATCCTGCTCAG-3′ and 16S_805R, 5’-GACTACHVGGGGGTATCTAATCC-3′), 1 U of GoTaq DNA Polymerase (Promega, Madison, United States), 20 ng/μL−1 of DNA and filled to 20 μl with sterile filtered molecular grade water. Thermal conditions were achieved by cycling at 94°C for 3 min, followed by 35 cycles of DNA denaturation at 94°C for 60 s, primer annealing at 45°C for 40 s and DNA elongation at 72°C for 60 s, and a final extension at 72°C for 7 min. PCR products were run on 1% (w/v) agarose gels with SYBR Safe DNA Gel Stain (Thermo Fisher Scientific, Inc., Carlsbad, CA) in TAE buffer at 80 V for 45 min. The amplicons were visualized in a UV light transilluminator. The amplified DNA fragments were purified and directly sequenced in both directions (Macrogen, Seoul, South Korea). Sequencing results (ABI chromatograms) were analyzed in the free open-source software UGENE v.33. Nine out of 10 bacterial strains yielded analyzable chromatograms. The consensus fastq files were analyzed using the EzBioCloud 16S Database (Yoon et al., 2017). EzBiocloud is a species level resolution database made of 61 700 species/phylotypes, including 13 132 species/phylotypes with validly published names. A phylogenetic tree was constructed using the Seaview 4.0 program with the neighbor-joining method to determine relationships between bacterial strains. The resulting consensus tree was constructed using 1000 replicates.
Data analysis
Differences in soil conditions (i.e., temperature and water potential) throughout the growing season between north and south slopes were assessed by repeated measures ANOVAs (p < 0.05). Differences in mean, maximum, minimum air temperature and relative humidity, thermal breadth, intensity and duration of freezing and heat events between north and south-facing slopes, were assessed by t tests or non-parametric equivalent tests (p < 0.05) when data normality and homoscedasticity were not met. Differences in frequency of freezing and heat events and GDDs between slopes were assessed by Chi2 tests. Differences in soil properties were assessed by Factorial ANOVAs, where species and slope were predictors. When parametric requirements were not met these differences were assessed by Kruskal-Wallis ANOVA by ranks. Differences in PGPB abundance (Log CFUg−1) and in the activity of plant growth-promoting mechanisms (i.e., phytohormone and enzymes) were assessed by ANOVAs as well. All data were analyzed through the Statistica version 13.
Results
Microclimatic characterization of contrasting slopes
The North-facing slope was potentially more stressful than south-facing slope according to microclimatic data obtained during the complete snow free period (Table 1). Although air mean temperature was similar between slopes (t = 0.02, p = 0.985), GDD0 were 22% (χ2 = 3,133, p < 0.0001) and thermal breadth was 3.9°C greater (t = 7.3, p < 0.001) in north- than in south-facing slope. In addition, considerable differences in extreme temperatures were observed (Table 1). For example, the mean minimum temperature was lower in north- than in south-facing slope (2.5°C ± 0.2 vs. 4.3°C ± 0.2, t = 4.2, p < 0.0001). The intensity of freezing events averaged −2.4°C for both slopes (t = 0.11, p = 0.74), but their frequency was 2.4 times greater in north- than in south-facing slope (χ2 = 10.7, p = 0.001), with the lowest temperature of −9.9°C recorded on November 4, whilst south-facing slope was covered by snow. The duration of freezing events was 25% longer in south- than north-facing slope (t = 5, p = 0.028), but in both microsites they lasted on average more than 4 h (Table 1). At the other extreme, the mean maximum air temperature was 2.2°C higher in north- than in south-facing slope (Table 1, Z = 2.86, p = 0.004). In north-facing slope, heat events were 1.8 times more frequent (χ2 = 9.8, p = 0.002), 1.3 times longer (Z = 2.3, p = 0.022) and 1.3°C hotter (Z = 2.5, p = 0.011) than in the south-facing slope. In addition, heat events with temperatures over 40°C were recorded only in the north-facing slope (Table 1). Regarding air relative humidity (RH), averaged 51% for both slopes (t = 2.7, p = 0.077), but minimum RH was 6.5% lower (t = 3.9, p = 0.001) and maximum RH was 4% higher (t = 2.1, p = 0.034) in north- than in south-facing slopes.
Soil temperature was similar on north and the south-facing slope (Figure 2; slope F1,4 = 0.5, p = 0.519) with variations reported throughout the growing season (date F2,8 = 15.3, p = 0.002). In contrast, soil water potential was on average − 0.7 MPa and − 0.5 MPa in December and January on both slopes, respectively (Figure 2). However, its decrease was 2.2 times greater in the north- than in the south-facing slope (date x slope F2,8 = 9.3, p = 0.008). Hence, soils of north-facing slopes reported water potential of −1.96 MPa in March, while on the south-facing slope it was of −0.89 MPa (Figure 2). No recordings were carried out in April because snow started to cover soil moisture sensors.
Soil chemical properties varied between plant species and slope exposure (Table 2). For example, soil pH under Azorella prolifera was on average greater than of under Berberis empetrifolia (6.3 vs 5.3, H3,12 = 8.44, p < 0.038), independent of slope. Regarding organic matter, slope differences were observed for soils under both species but in opposite directions (Table 2; H3,12 = 9.15, p < 0.027). Under A. prolifera canopy, organic matter was a half lower in the north than in the south slope (Z = 1.96, p = 0.049). In contrast, organic matter was almost once greater in the north than in the south slope (Z = 1.96, p = 0.049) in soil collected under B. empetrifolia canopy (Table 2). Available K was 3 times greater in south than north slope for soils under A. prolifera (Z = 1.96, p = 0.049), whilst for soils under B. empetrifolia available K was similar between slopes (Z = 1.09, p = 0.275). Exchange Al was similar between slopes in soils under A. prolifera (Z = 0.86, p = 0.383), but it was almost 3 times greater in soils from north than south slopes under B. empetrifolia (Z = 1.96, p = 0.049). Al and Ca saturation varied between species but were similar between slopes (Table 2). For example, Al saturation was on average 15 times greater in soils under B. empetrifolia than in soils under A. prolifera (H3,12 = 9.26, p < 0.026). In contrast, Ca saturation was on average 26,4% greater in soils under A. prolifera than under B. empetrifolia (Table 2, F1,8 = 66.8, p < 0.0001). In the case of K Saturation, it was greater in soils from south slope independent of plant species (Table 2; H3,12 = 9.36, p < 0.025), with slope differences of 2.6 and 3.7 times in soils under A. prolifera and B. empetrifolia. Mg saturation exhibited similar percentages between slopes for soils under A. prolifera, but it was 8.2% greater in north than in south slope in soils under B. empetrifolia (Table 2; F1,8 = 10.9, p = 0.011). Similar pattern was observed in Fe (Table 2). No differences in Fe were found between slopes for soils under A. prolifera, but Fe was 44.2% greater in north than in south slope in soils under B. empetrifolia (Table 2; H 1,6 = 3.86, p = 0.049). Regarding S available, it was almost 9 times greater in south than in north slopes in soils under A. prolifera, but no differences between slopes were observed in soils under B. empetrifolia (Table 2; F1,8 = 12.9, p = 0.007).
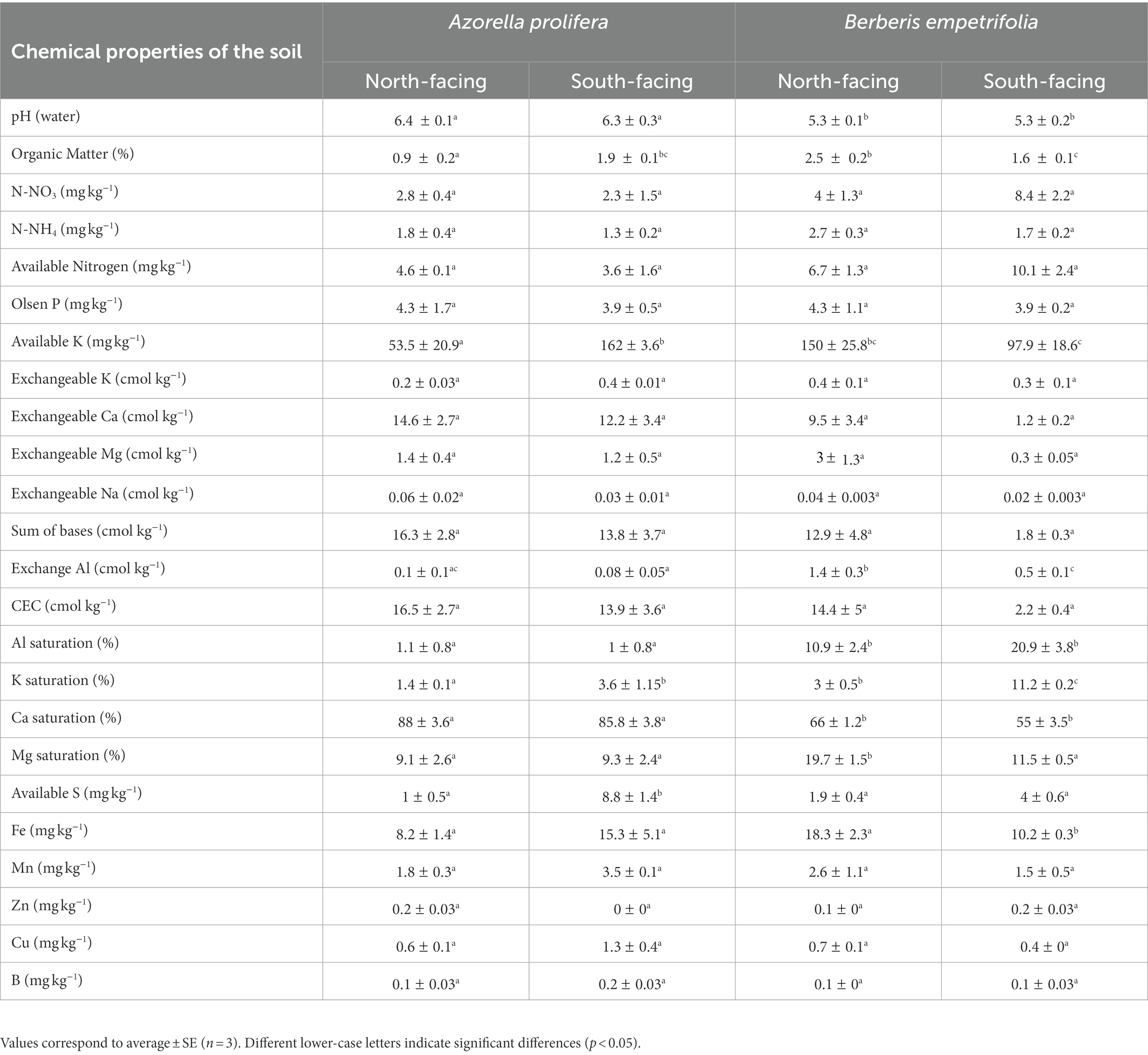
Table 2. Chemical characterization of the soils collected under plant canopy of Azorella prolifera and Berberis empetrifolia species growing in contrasting slopes.
Bacterial abundance and their identity from Andean xerophytic formations
Differences in CFU abundance between contrasting slopes depended on plant species where soil samples were obtained (H3,20 = 7.91, p = 0.048; Figure 3). While abundance in soils collected under Azorella prolifera was similar between slopes (Figure 3A; Z = 1.43, p = 0.28), CFU were more abundant in soils from north-facing slope when they were collected under Berberis empetrifolia canopy (Figure 3B; Z = 0.57, p = 0.021).
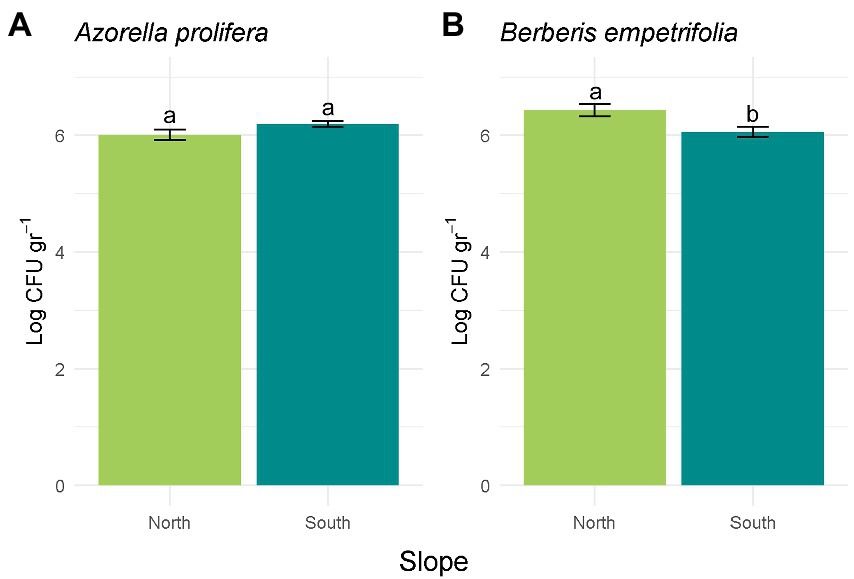
Figure 3. Bacterial abundance calculated in Log CFU g−1 for A. prolifera (A) and B. empetrifolia (B) soil. Values indicate average ± SE (n = 3). Letters indicate significant differences between slopes (Wilcoxon test, p > 0.05).
Slope differences in activity plant growth-promoting mechanisms
Of the 19 bacterial isolates present in the soils under the canopies of A. prolifera and B. empetrifolia on the north and south slopes, 89.47% of the isolates had activity in the production of IAA. Of the 17 isolates with activity in the indole acetic acid production mechanism, 52.95% of the isolates were from the northern slope and 47.05% were from the southern slope. For the ACC deaminase mechanism, 52.6% of the isolates had activity corresponding to 10 bacterial isolates, of which 70% corresponded to the northern slope and 30% to the southern slope. As for nitrogen fixation, 73.6%, corresponding to 14 bacterial isolates, had activity for this mechanism, where 64.28% corresponded to the northern slope and 35.73% had activity on the southern slope. 89.47% of the isolates corresponding to 17 bacterial isolates could solubilize phosphate, among the isolates with activity, we detected that 47.05% belonged to the northern slope and 52.95% (Supplementary Table S1; Supplementary material).The activity of four PGP mechanisms were compared between slopes. The hormonal PGP mechanism; Indole acetic acid (IAA) is presented in Figure 4. All isolates on the north slope of both A. prolifera and B. empetrifolia produced IAA, while on the south slope only 80% of the isolates produced this phytohormone. The highest value of IAA production was from an isolate from the north slope and A. prolifera soil (Supplementary Table S1; Supplementary material). When comparing the averages of IAA production on the north and south slopes for A. prolifera and B. empetrifolia species, we did not find differences between slopes, with averages IAA production of 1.4 μg ml−1 for A. prolifera (Figure 4A; F1,22 = 16.49, p = 0.9), and 1.6 μg ml−1 for B. empetrifolia (Figure 4B; F1,25 = 0,89, p = 0.46).
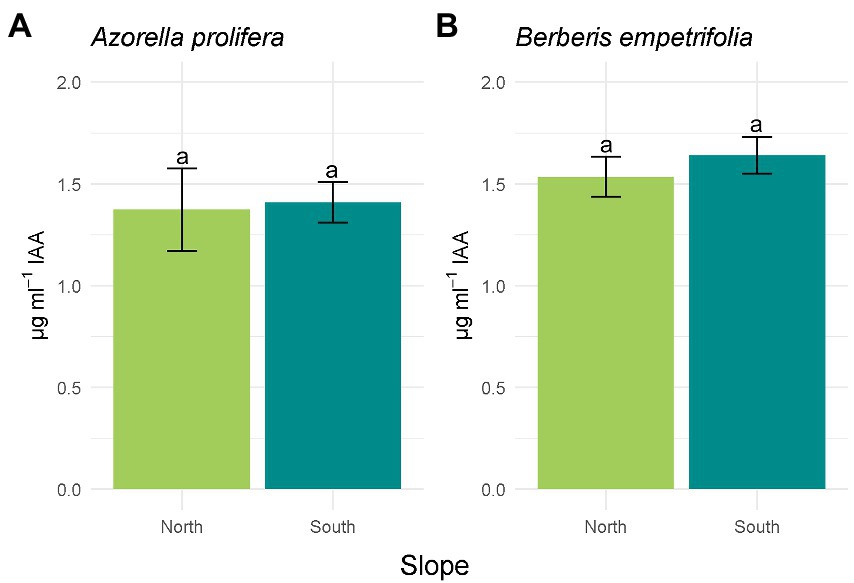
Figure 4. Indoleacetic acid (IAA) production of bacteria isolated from 2 species of Andean xerophytic formations on contrasting slopes: Azorella prolifera (A) and Berberis empetrifolia (B). For each species, north slope is shown in green and south slope in light blue. Values indicate average ±SE. Equal letters indicate no differences (p > 0.05).
The activity of enzymatic PGP mechanisms was on average higher in north than south slopes (Figure 5). On the north slope 77.7% of the bacterial isolates under the canopies of A. prolifera and B. empetrifolia possessed ACC-deaminase enzymatic mechanism activity, whereas, on the south slope, only 30% of the bacterial isolates from this slope possessed ACC-deaminase activity (Supplementary Table S1; Supplementary material). The highest activity of ACC deaminase was observed in a bacterial strain isolated from A. prolifera, which was 5 times higher in soils from north- than south slopes (7.1 vs. 1 μM; Figure 5A; F1,7 = 0.8 p = 0.01). In the case of B. empetrifolia, ACC deaminase had a similar activity in soils from both slopes (3.39 vs. 2.42 μM; Figure 5B; F1,8 = 0.04, p = 0. 41).
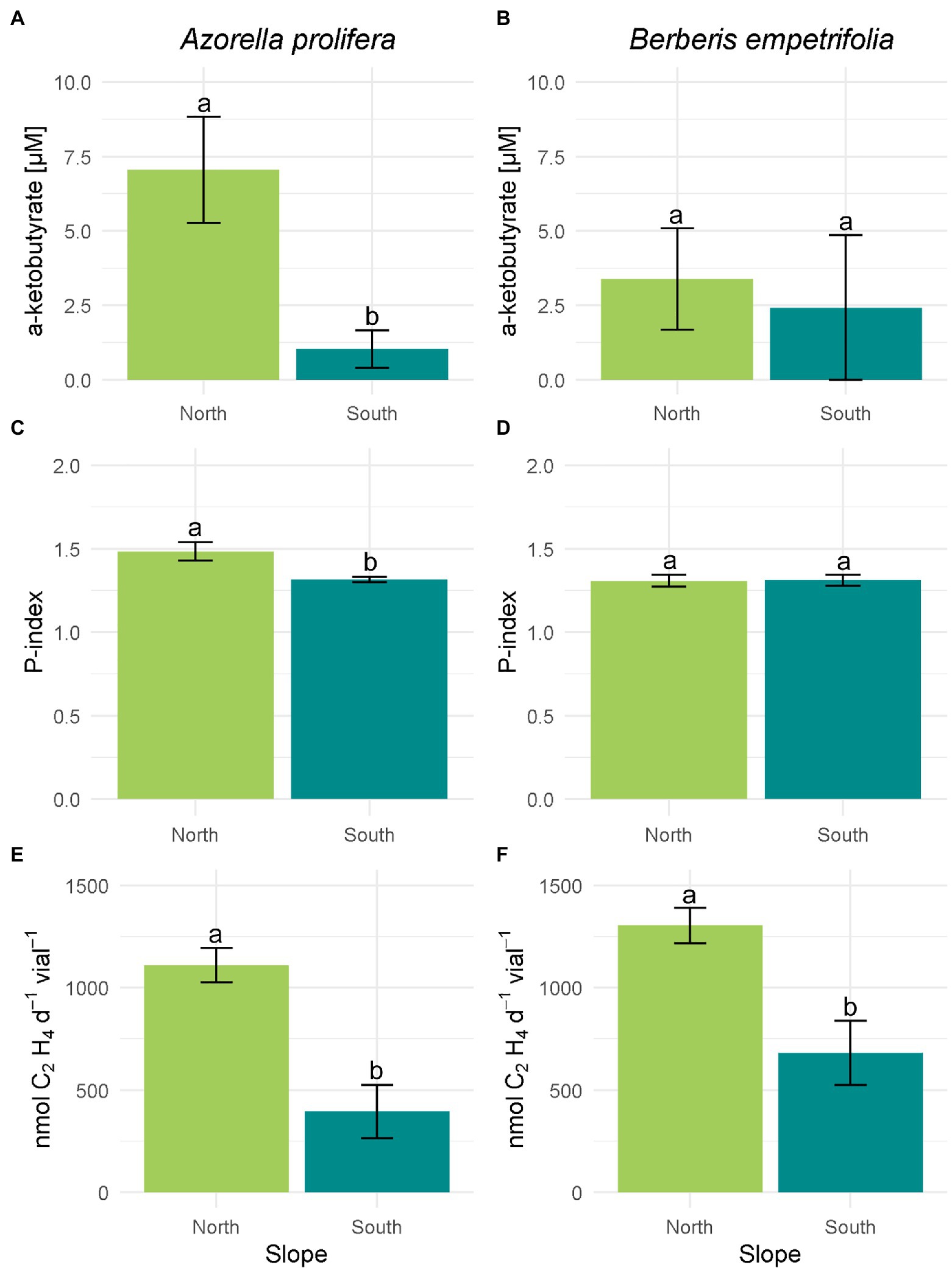
Figure 5. Enzymatic plant growth promoting mechanisms of bacteria isolated from 2 species of Andean xerophytic formations on contrasting slopes. For all mechanisms the columns were divided by species: A. prolifera on the left and B. empetrifolia on the rights. For each species the slopes are indicated with colors: green for the north slope and light blue for the south slope. ACC deaminase enzyme activity (A,B) are presented as μM of α-ketobutyrate. Phosphate solubilization (C,D) is presented as Index P. Nitrogen fixation (E,F) is given as nmoles C₂H₄ d−1 vial−1. Values indicate average ± SE. Different letters above error bars indicate significant differences between slopes (T test or nonparametric equivalent, p < 0.05).
Among the north slope isolates, bacterial colonies with phosphate solubilizing mechanism were 88.8%, while on the south slope it was 90% (Supplementary Table S1; Supplementary material). However, the ability to solubilize phosphorus was higher on the north slope (H3,20 = 9.66, p = 0.022), particularly under the A. prolifera canopy, where, the solubilization Phosphorus index was 12% greater for culturable bacteria obtained in soils under from north than from south slope (Figure 5C; Z = 1.7, p = 0.01). In contrast, solubilization P index was similar between slopes for bacteria obtained from soil under B. empetrifolia (1,31 vs. 1,31; Figure 5D; Z = 0.21, p = 0.87).
Nitrogenase enzyme activity on the north slope occurred for all isolates, both for soils under A. prolifera canopy and B. empetrifolia soils. On the southern slope, on the other hand, nitrogenase activity only occurred for half of the bacterial isolates (Supplementary Table S1; Supplementary material). A greater nitrogenase activity was observed in bacteria obtained from north slope soils independent of plant species (Figures 5E,F). For example, for bacteria isolated from A. prolifera soils, ethylene production averaged 396 nmoles C₂H₄ d−1vial−1 on the south slope, whilst on the north slope it was 1,109 nmoles C₂H₄ d−1vial−1 (Figure 5E; F1,25 = 0.95, p = 0.007). For bacteria isolated from B. empetrifolia soils, the nitrogenase activity was 3.3 times greater in the north than in the south slope (Figure 5F; F1,25 = 3.5, p = 0.001).
Molecular identification of bacterial strains
Randomly selected bacterial strains used to determine species identity of colonies and correspond to five genus and six species (Table 3). Regarding phylogenetic tree, four clades were identified and correspond with genus detected (Figure 6). Only two bacterial strains were assigned to the same species (2HBER4 and 4SBER4) and would correspond to Pseudomonas atacamensis. These bacterial strains where isolated from B. empetrifolia. Five bacterial strains were assigned to species belonging to the genera Pseudarthrobacter and Arthrobacter, detecting only almost identical (2SAZO6 and 6SAZO5) both from under A. prolifera on the south slope. The other two entities are made up of single species, respectively.
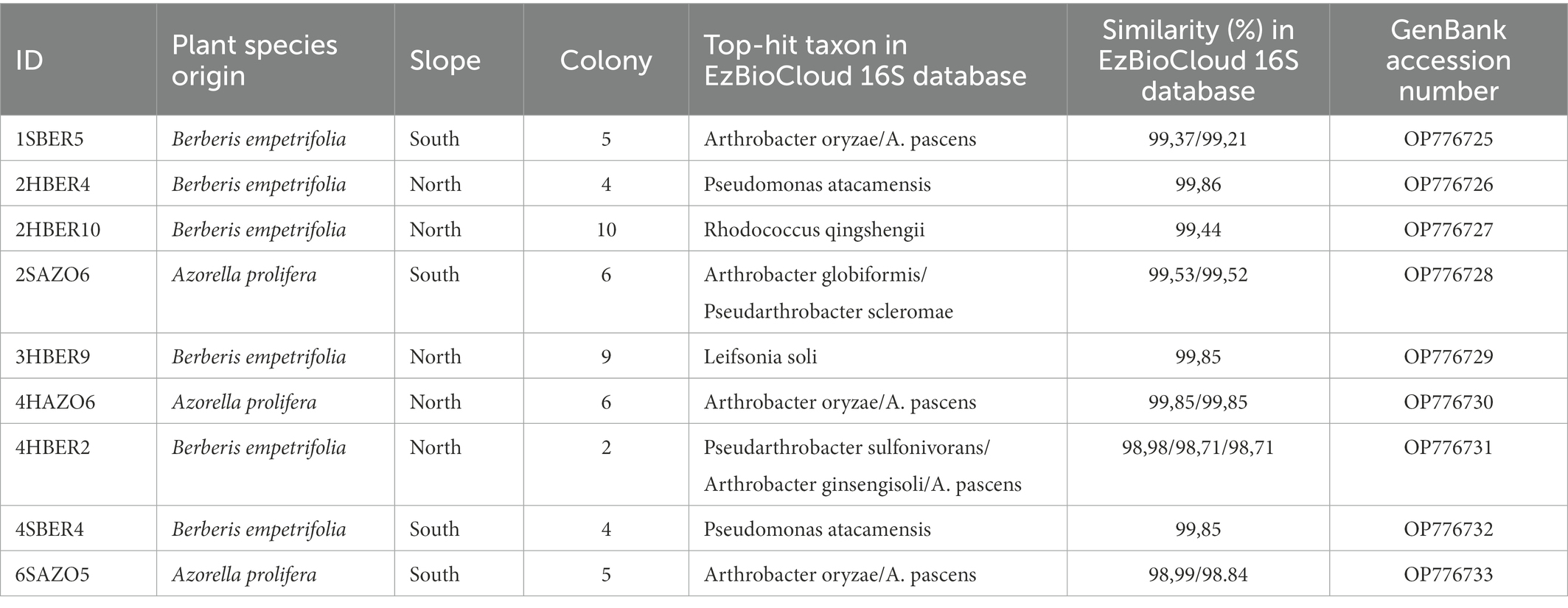
Table 3. Bacterial sequences obtained for 9 of the most abundant colonies isolated from soil under plant xerophytics species in the Nevados de Chillán Volcano Complex. See Materials and Methods for details.
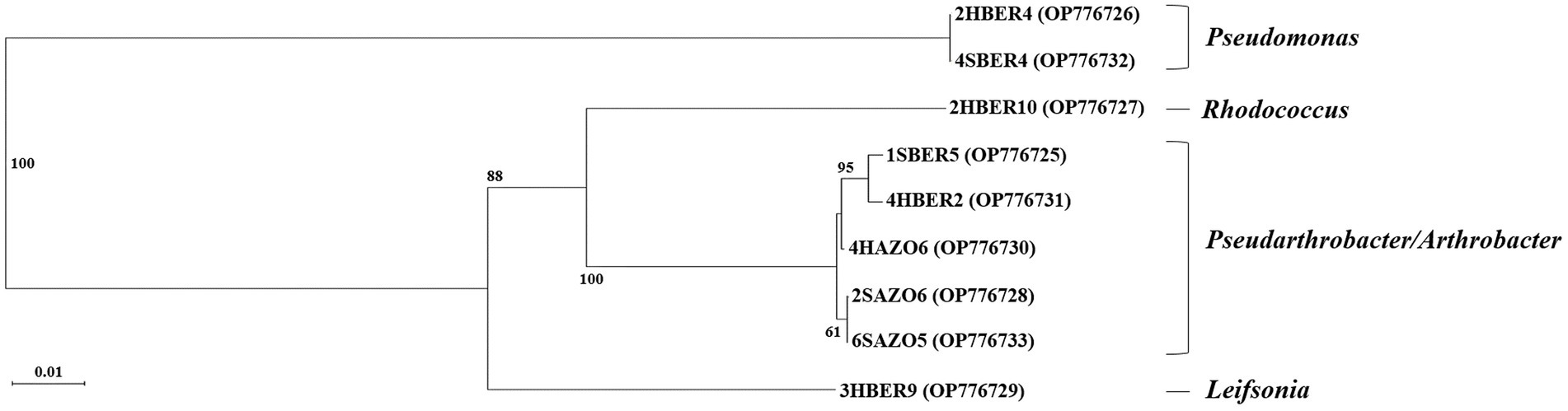
Figure 6. Phylogenetic tree based on sequences of the 16S genes (790 bp amplicon size) of 9 bacterial isolates obtained from A. prolifera and B. empetrifolia species from the north and south slopes of the Nevados de Chillán volcanic complex. 2HBER4, 2HBER10, 4HBER2, 3HBER9 correspond to isolates of the species B. empetrifolia on the south slope. 4SBER4, 1SBER5 correspond to isolates of the specie B. empetrifolia on the north slope. 4HAZO6 correspond to isolates of the specie A. prolifera on the south slope. 2SAZO6, and 6SAZO5 correspond to isolates of the species A. prolifera on north slope.
Discussion
This work quantified the abundance and activity of bacteria present in the south-central zone of the Andes Mountains. In addition, we observed how environmental variability in water availability, thermal oscillation, and plant identity impacted the different indicators evaluated. An important finding in the present investigation was that a higher abundance and enzyme activity was observed on the drier slope compared to the southern slope. In addition, two of the three enzymatic mechanisms showed differences between slopes, but only for bacteria associated with Azorella prolifera, not for Berberis empetrifolia. Thus, species identity is key when exploring the beneficial potential of plant growth-promoting bacteria in alpine systems.
First, the ranges obtained for bacterial abundance seem to coincide with the literature. For example, works such as those of Sinegani and Younessi (2017) have obtained ranges close to 3 Log CFU g−1 for contaminated sites, and values between 6.3 and 8.3 Log CFU g−1 for pastures and agricultural systems, the most common being close to 6 Log CFU g−1. Kieft et al. (1993) have obtained ranges between 2 and 6.7 Log CFU g−1 for soils of natural arid systems in the United States. In relation to the activity of PGPBs from Andean xerophytic formations reached low levels compared to those reported for agricultural systems. For example, our PGPBs produced indole acetic acid in a range of 1.4 μg ml−1 to 1.7 μg ml−1, whilst in agricultural systems IAA productions fluctuate between 8.5 μg ml−1 and 69.7 μg ml−1 (Herlina et al., 2017). Likewise, while phosphate solubilization in agricultural systems fluctuates between 4 and 5 (Schoebitz et al., 2013; Pande et al., 2017), in our Andean xerophytic system it did not exceed 1.5. While the highest nitrogenase enzyme activity in our assay was 1.4 μmol C₂H₄ d−1vial−1, assays with industrial bacteria ranged between 6 and 108 μmol C₂H₄ d−1 vial−1 (Li et al., 2017). Most studies evaluating the potential benefits of PGPBs are conducted in agricultural systems, with bacteria that have been optimized for years, so the low enzyme activity observed in bacteria from natural systems is not surprising. Interestingly, this pattern changes when we compare our results with the plant growth-promoting activity of bacteria from natural systems. For example, Kadioglu et al. (2018), isolated cold-tolerant bacteria with ACC deaminase activity from different native alpine plants in Erzurum (between 1,760 and 2,720 m above sea level). The activity of these bacteria ranged from 0.9 to 1.2 μM α-ketobutyrate, almost six times less than the activity obtained in our study. Most studies on the abundance and activity of PGPBs in alpine systems are generally from the Himalayas, where the bacteria interact with cultivated plants and in highly degraded soils (e.g., Majeed et al., 2018; Kumar et al., 2019). The level of disturbance in a system is also known to affect PGPB activity (Bergottini et al., 2015). This makes it difficult to draw comparisons that evidence the potential of the findings embodied in this study.
Bacterial abundance and growth-promoting mechanisms related to slopes
We observed that bacterial abundance and enzyme activity was higher on the north than on the south slope. The climatic conditions could be behind this trend. Generally, the north slope tends to be drier and warmer than the south slope, as previously described for its equatorially oriented slopes (Scherrer and Körner, 2011). In addition, more intense and frequent heat and freezing events occurred on the north slope (Table 1), with greater intra-seasonal thermal fluctuations than on the south slope. This means that plant-PGPB interactions should be more likely to be found on the north slope, and the benefits, represented as the activity of PGP mechanisms, should be greater on this “hard” slope.
Regarding the influence of each environmental factor on bacterial abundance, what might contribute to the differences observed on the north slope. Studies have described the influence of each environmental factor independently, such as temperature (Chen et al., 2015), soil moisture (Naylor and Coleman-Derr, 2018), and soil chemical properties (Ai et al., 2020). For example, increasing temperature favors bacterial abundance, especially in cold regions (Chen et al., 2015). As for soil moisture, it affects in a more dynamic and integrated way, because it affects soil chemical properties and plant performance (Naylor and Coleman-Derr, 2018), which in turn affects bacteria. However, the identity and adaptations of bacteria determine whether they will survive and grow under extreme conditions (Pérez-Jaramillo et al., 2018). Furthermore, their response will depend on how stable the communities they compose are; stable bacterial communities do not change in drier soils (Stres et al., 2008). A clear example is a work of Chodak et al. (2015), who evaluated the response of drought-adapted bacteria that were subsequently rewetted, resulting in a stress-related decrease in gram-negative bacteria and an increase in gram-positive bacteria. Although the higher bacterial abundance observed on the north-facing slope responded to climatic factors, we believe that the response of bacterial abundance depends also on the characteristics of the bacteria (i.e., adaptations and identity), their community and the plants with which they interact.
The identity of the most abundant and active bacteria coincides with that reported for other systems with extreme conditions. All bacterial genera identified in this study have been identified in climatically limiting environments such as the Himalayas (e.g., Pseudomonas, Selvakumar et al., 2008; Rhodococcus, Ruberto et al., 2005; Leifsonia, Reddy et al., 2008), the Andes mountain range (e.g., Pseudomonas Viruel et al., 2011; Vega-Celedón et al., 2021), Antarctica (e.g., Arthrobacter, Dsouza et al., 2015 Pseudarthrobacter; Shin et al., 2020; Leifsonia, Ganzert et al., 2011), as well as in extremely dry regions (e.g., Pseudoarthrobacter isolated from African deserts, Buckley et al., 2019). In terms of their activity, the detected genera have been characterized as PGPB. For example, the production of IAA by bacteria of the genus Leifsonia (Kang et al., 2016), or the ACC deaminase, phosphatase, and nitrogenase activity of Pseudomonas (Georgieva et al., 2018; Adhikari et al., 2021; Vega-Celedón et al., 2021), or bacteria of the genera Arthrobacter and Pseudoarthrobacter that have been reported with multiple growth-promoting activities (Lahsini et al., 2022). It is believed that the climatic limitations of our study area favored a select group of PGPB that possess adaptations that allow them to survive and grow in conditions that for other bacterial groups would be lethal.
The influence of climatic variability concerning temperature and water availability on the enzyme activity of PGPB has been limitedly studied. For example, for ACC deaminase enzyme activity, we know that it is an enzyme characteristic of saline and drought-exposed soils (Glick, 2004; Brockett et al., 2012). In general, both the number of ACC deaminase isolates, and their activity were higher on the north slope than on the south slope, which was much less active. Considering our results, it could be intuited that in more extreme environments there could be a higher activity of this enzyme. The phosphatase activity has been detected in psychrotolerant bacteria, which also solubilize phosphate under low-temperature conditions [e.g., Pseudomonas spp. isolated from Hilamaya, Adhikari et al. (2021)]. Our studied bacteria likely possess the same qualities, but studies are lacking to understand the influence of temperature on phosphate solubilization of our isolates. About nitrogen fixation, which is also higher on the north slope, we know that nitrogenase enzyme activity is favored in humid and cold south conditions (Rousk et al., 2018). However, our results showed that nitrogenase enzyme activity was higher on the drier and more temperature fluctuating north slope. Probably, a specific group of nitrogen-fixing bacteria adapted to such microclimatic conditions prevails on the north slope. The exposure of the slope favored the activity of the three enzymatic mechanisms on the north slope. However, it was not enough to create differences between the two species, so we believe that plant identity played a key role in our results.
Bacterial abundance and growth-promoting mechanisms related to species identity
Bacterial abundance was influenced by slope exposure, and unexpectedly by species identity. Initially, we thought that trends in abundance and activity should respond only to slope exposure and should coincide for the two species since both were present under the same temperature and moisture conditions. By looking at the chemical properties of the soil, there were noticeable differences between species. A clear example was the alteration of pH by plant identity, which exacerbated the differences in bacterial abundance only in B. empetrifolia soils. Soils of B. empetrifolia and A. prolifera had differences in pH, with the former being more acidic. Soil pH is usually altered by root exudates of plant identity (Youssef and Chino, 1989). However, studies that have evaluated bacterial abundance as a function of pH claim that increasing pH should increase bacterial abundance (Rousk et al., 2018). On the other hand, research such as that of Yang et al. (2019), conducted in alpine systems disturbed by agricultural activity, mentions that the abundance of groups such as Actinobacteria (e.g., Rhodococcus associated with B. empetrifolia on the North Slope) is not influenced by changes in pH (Yang et al., 2019). While we believe that the combination of slope exposure coupled with pH changes in B. empetrifolia soils benefited the bacterial abundance of a group of microorganisms, studies are lacking to secure these insights.
Linking plant identity and associated abundant bacteria. We found that for the genus Azorella, it is the first time that bacteria of the genus Pseudoarthrobacter are detected. Previously, works such as those of Rodríguez-Echeverría et al. (2021) had detected abundant phyla such as actinobacteria for A. compacta and A. madreporica. However, among the bacteria identified, no actinobacteria were reported for A. prolifera. Vega-Celedón et al. (2021) had previously detected bacteria of the genus Arthrobacter associated with B. microphylla in Patagonia. Genera such as Pseudomonas, Rhodococcus, and Leifsonia had not been previously detected, so this is the first record in this regard.
In this study, plant identity influenced two of the three evaluated mechanisms of PGPB. Interestingly, bacterial isolates present on the north slope under the A. prolifera plant canopies differed in activity for two of the four mechanisms evaluated between the north and south slopes. At the same time, these bacterial isolates were the only ones that presented activity in all the hormonal and enzymatic mechanisms evaluated (Supplementary Table S1; Supplementary material). Phosphatase activity was high in soils under the A. prolifera canopy, which in parallel exhibited the lowest percentage of organic matter on the north slope. Coincidentally, Alemneh et al. (2022) reported a negative correlation between phosphate solubilization and soil carbon, which explains our results. Concerning ACC deaminase enzyme activity, we believe that it is not linked to soil chemical properties and is related to the plant in a more complex way. We know that the plant through its root exudates modifies its microbiome benefiting one group of microorganisms over others for its benefit (Glick et al., 2007). A. prolifera was likely more stressed than B. empetrifolia on the north slope, and for this reason, we only had differences in ACC deaminase activity for one species. As for nitrogenase enzyme activity, this was higher on the north slope for both species, so we believe that at least this mechanism is not influenced by species identity. Our results evidence a notorious relationship between plants and PGPB activity, where interactions do not seem to occur randomly.
The combination of slope exposure and plant identity evidenced a clear influence on PGPB enzyme activity, where the harshness of the alpine system favored PGPB and its benefits when conditions became more extreme on the north slope. Studies indicate that stressed plants modify their root exudates, altering their soil microbiome and favoring the presence of PGPB (Li et al., 2020; Glick and Gamalero, 2021; Chen et al., 2022). The composition of root exudates depends on soil chemical properties, plant genotype, and plant age (Vives-Peris et al., 2018). Perhaps, climatically limiting conditions “enhanced” A. prolifera root exudation, increasing the activity of PGPBs. Further studies need to consider plant responses by incorporating root exudates among predictors of bacterial abundance and activity.
Conclusion
Our work brings us closer to understanding what factors affect the abundance and activity of PGPB in hard systems such as those present in our system. Before our research, studies related to PGPB present in the soils of the Andes Mountains had evidenced their great biotechnological potential and their novelty for the detection of new species, in areas such as the Atacama Desert and the altiplano, with preliminary characterizations. The present work enriches the existing information related to the presence of PGPB from alpine systems in regions that had not been previously explored.
Our work showed how the environmental heterogeneity of the alpine systems, given by the contrasting slopes and its thermal and moisture oscillations, added to the identity of the species, generating variations in the abundance and plant growth promoting activity of the microorganisms present under the plant canopy of the xerophytic formations. It would be interesting to determine how and to what extent each factor contributes to the benefit provided by PGPBs. We believe that incorporating the influence of factors such as plant condition, plant identity, and microclimatic variations on changes in PGPB abundance and activity would highlight the importance of these interactions that do not occur randomly, especially when conditions become more extreme.
Data availability statement
The original contributions presented in the study are included in the article/Supplementary material, further inquiries can be directed to the corresponding author.
Author contributions
Plants may interact with soil bacteria that promote their survival and growth (PGPB) through a variety of mechanisms, especially under harsh climatic conditions. Plants that live in alpine systems have to be able to cope with harsh climatic conditions such as temperature extremes. Likely, plant-bacteria interactions are behind of plant strategies through they can grow in alpine systems. However, these interactions are poorly known. One of the southernmost xerophytic formations is in the Andes of central-southern Chile. In this system, plants are exposed to drought and extreme temperatures during the growing season, being harsher on equatorial than polar slopes. Thus, we compared the abundance and activity of PGPB under two plant species canopy that live in contrasting slopes. We found that bacterial abundance and enzymatic activity of PGPB was higher on the driest and with greatest thermal oscillation slope. Unexpectedly, we also found that abundance and activity of bacteria depended on plant identity. We believe that microclimatic conditions such as humidity, temperature, soil nutrients, and plant identity explain our findings, which are not random, especially when conditions become more extreme.
Funding
This work was supported by CONAF-FIBN 047/2020 (RH), FONDECYT N°1220425 (MS), VRID-UDEC 2021000184INV (ASA) Grants, and ANID-Chile Technological Centres of Excellence with Basal Financing Project [CHIC–ANID PIA/BASAL PFB210018]. Carla Aguilera-Torres holds an ANID Master Fellowship.
Acknowledgments
María Dolores López and Felipe Noriega (Laboratorio de Química de Productos Naturales), José Becerra and Claudia Pérez (Laboratorio de Química de Productos Naturales), Matías Betancur (Laboratorio de Microbiología de Suelos), Adrián Garrido (Laboratorio de Epigenética Vegetal) and GET team for their help in the field work.
Conflict of interest
The authors declare that the research was conducted in the absence of any commercial or financial relationships that could be construed as a potential conflict of interest.
Publisher’s note
All claims expressed in this article are solely those of the authors and do not necessarily represent those of their affiliated organizations, or those of the publisher, the editors and the reviewers. Any product that may be evaluated in this article, or claim that may be made by its manufacturer, is not guaranteed or endorsed by the publisher.
Supplementary material
The Supplementary material for this article can be found online at: https://www.frontiersin.org/articles/10.3389/fmicb.2022.1062414/full#supplementary-material
Footnotes
References
Acuña, J. J., Campos, M., Mora, M. D. L. L., Jaisi, D. P., and Jorquera, M. A. (2019). ACCD-producing rhizobacteria from an Andean Altiplano native plant (Parastrephia quadrangularis) and their potential to alleviate salt stress in wheat seedlings. Appl. Soil Ecol. 136, 184–190. doi: 10.1016/j.apsoil.2019.01.005
Adamczyk, M., Hagedorn, F., Wipf, S., Donhauser, J., Vittoz, P., Rixen, C., et al. (2019). The soil microbiome of GLORIA Mountain summits in the Swiss Alps. Front. Microbiol. 10:1080. doi: 10.3389/fmicb.2019.01080
Adhikari, P., Jain, R., Sharma, A., and Pandey, A. (2021). Plant growth promotion at low temperature by phosphate-solubilizing pseudomonas Spp. isolated from high-altitude Himalayan soil. Microb. Ecol. 82, 677–687. doi: 10.1007/s00248-021-01702-1
Afzal, I., Iqrar, I., Shinwari, Z. K., and Yasmin, A. (2017). Plant growth-promoting potential of endophytic bacteria isolated from roots of wild Dodonaea viscosa L. Plant Growth Regul. 81, 399–408. doi: 10.1007/s10725-016-0216-5
Ahmad, F., Ahmad, I., and Khan, M. S. (2005). Indole acetic acid production by the indigenous isolates of Azotobacter and fluorescent pseudomonas in the presence and absence of tryptophan. Turk. J. Biol. 29, 29–34.
Ai, S., Chen, J., Gao, D., and Ai, Y. (2020). Distribution patterns and drivers of artificial soil bacterial community on cut-slopes in alpine mountain area of Southwest China. Catena 194:104695. doi: 10.1016/j.catena.2020.104695
Alemneh, A. A., Zhou, Y., Ryder, M. H., and Denton, M. D. (2022). Soil environment influences plant growth-promotion traits of isolated rhizobacteria. Pedobiologia 90:150785. doi: 10.1016/j.pedobi.2021.150785
Ali, S. Z., Sandhya, V., and Rao, L. V. (2014). Isolation and characterization of drought-tolerant ACC deaminase and exopolysaccharide-producing fluorescent pseudomonas sp. Ann. Microbiol. 64, 493–502. doi: 10.1007/s13213-013-0680-3
Arroyo, M. T. K., Squeo, F., Cavieres, L. A., and Marticorena, C. (2004). “Chilenische Anden” in Gebirge der Erde. Landschaft, Klima, Pflanzenwelt. eds. C. A. Burga, F. Klötzli, and G. Grabherr (Ulmer, Stuttgart, Germany: Ulmer Eugen Verlag), 210–219.
Ashry, N. M., Alaidaroos, B. A., Mohamed, S. A., Badr, O. A. M., El-Saadony, M. T., and Esmael, A. (2022). Utilization of drought-tolerant bacterial strains isolated from harsh soils as a plant growth-promoting rhizobacteria (PGPR): utilization of drought-tolerant bacterial strains. Saudi J. Biological Sciences 29, 1760–1769. doi: 10.1016/j.sjbs.2021.10.054
Bais, H. P., Weir, T. L., Perry, L. G., Gilroy, S., and Vivanco, J. M. (2006). The role of root exudates in rhizosphere interactions with plants and other organisms. Annu. Rev. Plant Biol. 57, 233–266. doi: 10.1146/annurev.arplant.57.032905.105159
Barbosa, M. S., Rodrigues, E. P., Stolf-Moreira, R., Tischer, C. A., and de Oliveira, A. L. M. (2020). Root exudate supplemented inoculant of Azospirillum brasilense Ab-V5 is more effective in enhancing rhizosphere colonization and growth of maize. Environmental Sustain. 3, 187–197. doi: 10.1007/s42398-020-00103-3
Bergottini, V. M., Otegui, M. B., Sosa, D. A., Zapata, P. D., Mulot, M., Rebord, M., et al. (2015). Bio-inoculation of yerba mate seedlings (Ilex paraguariensis St. hill.) with native plant growth-promoting rhizobacteria: a sustainable alternative to improve crop yield. Biol. Fertil. Soils 51, 749–755. doi: 10.1007/s00374-015-1012-5
Bhattacharyya, P. N., and Jha, D. K. (2012). Plant growth-promoting rhizobacteria (PGPR): emergence in agriculture. World J. Microbiol. Biotechnol. 28, 1327–1350. doi: 10.1007/s11274-011-0979-9
Brockett, B. F. T., Prescott, C. E., and Grayston, S. J. (2012). Soil moisture is the major factor influencing microbial community structure and enzyme activities across seven biogeoclimatic zones in western Canada. Soil Biol. Biochem. 44, 9–20. doi: 10.1016/j.soilbio.2011.09.003
Buckley, E., Lee, K. C., Higgins, C. M., and Seale, B. (2019). Crossm whole-genome sequences of one Arthrobacter strain and desert. Microbiology Resource Announcements 8, 18–19. doi: 10.1128/MRA.00885-19
Chaturvedi, P., and Shivaji, S. (2006). Exiguobacterium indicum sp. Nov., a psychrophilic bacterium from the Hamta glacier of the Himalayan mountain ranges of India. Int. J. Syst. Evol. Microbiol. 56, 2765–2770. doi: 10.1099/ijs.0.64508-0
Chen, J., Luo, Y., Xia, J., Jiang, L., Zhou, X., Lu, M., et al. (2015). Stronger warming effects on microbial abundances in colder regions. Sci. Rep. 5, 1–10. doi: 10.1038/srep18032
Chen, C., Wang, M., Zhu, J., Tang, Y., Zhang, H., Zhao, Q., et al. (2022). Long-term effect of epigenetic modification in plant–microbe interactions: modification of DNA methylation induced by plant growth-promoting bacteria mediates promotion process. Microbiome 10, 36–19. doi: 10.1186/s40168-022-01236-9
Chodak, M., Golebiewski, M., Morawska-Ploskonka, J., Kuduk, K., and Niklinska, M. (2015). Soil chemical properties affect the reaction of forest soil bacteria to drought and rewetting stress. Ann. Microbiol. 65, 1627–1637. doi: 10.1007/s13213-014-1002-0
de Souza, R., Ambrosini, A., and Passaglia, L. M. P. (2015). Plant growth-promoting bacteria as inoculants in agricultural soils. Genet. Mol. Biol. 38, 401–419. doi: 10.1590/S1415-475738420150053
Dixon, H. J., Murphy, M. D., Sparks, S. J., Chávez, R., Naranjo, J. A., Dunkley,, et al. (1999). La geología del volcán Nevados de Chillán, Chile. Rev. Geol. Chile 26, 227–253.
Donoso, C. (1982). Reseña ecológica de los bosques mediterráneos de Chile. Bosque 4, 117–146. doi: 10.4206/bosque.1982.v4n2-04
Dsouza, M., Taylor, M. W., Turner, S. J., and Aislabie, J. (2015). Genomic and phenotypic insights into the ecology of Arthrobacter from Antarctic soils. BMC Genom. 16, 1–18. doi: 10.1186/s12864-015-1220-2
Duca, D. R., and Glick, B. R. (2020). Indole-3-acetic acid biosynthesis and its regulation in plant-associated bacteria. Appl. Microbiol. Biotechnol. 104, 8607–8619. doi: 10.1007/s00253-020-10869-5
Durante, M., Maseda, P. H., and Fernández, R. J. (2011). Xylem efficiency vs. safety: acclimation to drought of seedling root anatomy for six Patagonian shrub species. J. Arid Environ. 75, 397–402. doi: 10.1016/j.jaridenv.2010.12.001
Fajardo, A., Piper, F. I., and Cavieres, L. A. (2011). Distinguishing local from global climate influences in the carbon status variation with altitude of a treeline species. Glob. Ecol. Biogeogr. 20, 307–318. doi: 10.1111/j.1466-8238.2010.00598.x
Fan, S., Sun, H., Yang, J., Qin, J., Shen, D., and Chen, Y. (2021). Variations in soil enzyme activities and microbial communities along an altitudinal gradient on the eastern Qinghai–Tibetan plateau. Forests 12, 1–14. doi: 10.3390/f12060681
Farooq, S., Nazir, R., Ganai, B. A., Mushtaq, H., and Dar, G. J. (2022). Psychrophilic and psychrotrophic bacterial diversity of Himalayan Thajwas glacial soil. India. Biologia 77, 203–213. doi: 10.1007/s11756-021-00915-6
Franche, C., Lindström, K., and Elmerich, C. (2009). Nitrogen-fixing bacteria associated with leguminous and non-leguminous plants. Plant Soil 321, 35–59. doi: 10.1007/s11104-008-9833-8
Fuentes, A., Herrera, H., Charles, T. C., and Arriagada, C. (2020). Fungal and bacterial microbiome associated with the rhizosphere of native plants from the Atacama desert. Microorganisms 8:209. doi: 10.3390/microorganisms8020209
Gamalero, E., and Glick, B. R. (2011). in Mechanisms used by plant growth-promoting bacteria BT – Bacteria in agrobiology: Plant nutrient management. ed. D. K. Maheshwari (Berlin Heidelberg: Springer), 17–46.
Gang, S., Sharma, S., Saraf, M., Buck, M., and Schumacher, J. (2019). Analysis of indole-3-acetic acid (IAA) production in Klebsiella by LC-MS/MS and the Salkowski method. Bio-Protocol 9, e3230–e3239. doi: 10.21769/bioprotoc.3230
Ganzert, L., Bajerski, F., Mangelsdorf, K., Lipski, A., and Wagner, D. (2011). Leifsonia psychrotolerans sp. nov., a psychrotolerant species of the family microbacteriaceae from Livingston Island, Antarctica. Int. J. Syst. Evol. Microbiol. 61, 1938–1943. doi: 10.1099/ijs.0.021956-0
Georgieva, T., Evstatieva, Y., Savov, V., Bratkova, S., and Nikolova, D. (2018). Assessment of plant growth promoting activities of five rhizospheric pseudomonas strains. Biocatal. Agric. Biotechnol. 16, 285–292. doi: 10.1016/j.bcab.2018.08.015
Gestión de Recursos Naturales (GRN). (2022). Mapa de Áreas con Presencia de Formaciones Xerofíticas. Reviewed from https://www.grn.cl/Mapa%20de%20Areas%20con%20Presencia%20de%20Formaciones%20Xerofiticas.pdf.
Glick, B. R. (2004). Bacterial ACC deaminase and the alleviation of plant stress. Adv. Appl. Microbiol. 56, 291–312. doi: 10.1016/S0065-2164(04)56009-4
Glick, B. R. (2012). Plant growth-promoting bacteria: mechanisms and applications. Scientifica (Cairo). 2012:963401, 1–15. doi: 10.6064/2012/963401
Glick, B. R., and Gamalero, E. (2021). Recent developments in the study of plant microbiomes. Microorganisms 9, 1–18. doi: 10.3390/microorganisms9071533
Glick, B. R., Todorovic, B., Czarny, J., Cheng, Z., Duan, J., and McConkey, B. (2007). Promotion of plant growth by bacterial ACC deaminase. Crit. Rev. Plant Sci. 26, 227–242. doi: 10.1080/07352680701572966
Golluscio, R. A., Cavagnaro, F. P., and Valenta, M. D. (2011). Shrubs of the Patagonian steppe: adapted to tolerate drought or grazing? Austral Ecol. 21, 61–70.
Hara, S., Hashidoko, Y., Desyatkin, R. V., Hatano, R., and Tahara, S. (2009). High rate of N2 fixation by East Siberian cryophilic soil bacteria as determined by measuring acetylene reduction in nitrogen-poor medium solidified with gellan gum. Appl. Environ. Microbiol. 75, 2811–2819. doi: 10.1128/AEM.02660-08
Hardy, R. W. F., Holsten, R. D., Jackson, E. K., and Burns, R. C. (1968). The acetylene-ethylene assay for N2 fixation: laboratory and field evaluation 1. Plant Physiol. 43, 1185–1207. doi: 10.1104/pp.43.8.1185
Haselwandter, K., Hofmann, A., Holzmann, H. P., and Read, D. J. (1983). Availability of nitrogen and phosphorus in the nival zone of the Alps. Oecologia 57, 266–269. doi: 10.1007/BF00379589
Herlina, L., Pukan, K. K., and Mustikaningtyas, D. (2017). The endophytic bacteria producing IAA (Indole acetic acid) in Arachis hypogaea. Cell Biology and Develop. 1, 31–35. doi: 10.13057/cellbioldev/v010106
Hingole, S. S., and Pathak, A. P. (2016). Saline soil microbiome: a rich source of halotolerant PGPR. J. Crop. Sci. Biotechnol. 19, 231–239. doi: 10.1007/s12892-016-0035-2
Honma, M., and Shimomura, T. (1978). Metabolism of 1-aminocyclopropane-1-carboxylic acid. Agric. Biol. Chem. 42, 1825–1831. doi: 10.1080/00021369.1978.10863261
Jaggi, V., Brindhaa, N. T., and Sahgal, M. (2020). in Microbial diversity in northwestern Himalayan Agroecosystems: Functions and applications BT - microbiological advancements for higher Altitude Agro-Ecosystems & Sustainability. eds. R. Goel, R. Soni, and D. C. Suyal (Singapore: Springer), 135–161.
Joshi, P., Joshi, G. K., Tanuja,, Mishra, P. K., Bisht, J. K., and Bhatt, J. C. (2014). Diversity of cold tolerant phosphate solubilizing microorganisms from North Western Himalayas. 1, 227–264. doi: 10.1007/978-3-319-05936-5_10
Kadioglu, G. B., Koseoglu, M. S., Ozdal, M., Sezen, A., Ozdal, O. G., and Algur, O. F. (2018). Isolation of cold tolerant and ACC Deaminase producing plant growth promoting Rhizobacteria from high altitudes. Romanian Biotechnological Letters 23, 13479–13486.
Kang, S. M., Asaf, S., Kim, S. J., Yun, B. W., and Lee, I. J. (2016). Complete genome sequence of plant growth-promoting bacterium Leifsonia xyli SE134, a possible gibberellin and auxin producer. J. Biotechnol. 239, 34–38. doi: 10.1016/j.jbiotec.2016.10.004
Kanu, S., and Dakora, F. D. (2009). Thin-layer chromatographic analysis of lumichrome, riboflavin and indole acetic acid in cell-free culture filtrate of Psoralea nodule bacteria grown at different pH, salinity and temperature regimes. Symbiosis 48, 173–181. doi: 10.1007/BF03179996
Kieft, T. L., Amy, P. S., Brockman, F. J., Fredrickson, J. K., Bjornstad, B. N., and Rosacker, L. L. (1993). Microbial abundance and activities in relation to water potential in the vadose zones of arid and semiarid sites. Microb. Ecol. 26, 59–78. doi: 10.1007/BF00166030
Kifle, M. H., and Laing, M. D. (2015). Isolation and screening of bacteria for their Diazotrophic potential and their influence on growth promotion of maize seedlings in greenhouses. Front. Plant Sci. 6:1225. doi: 10.3389/fpls.2015.01225
Kong, Z., and Glick, B. R. (2017). The role of plant growth-promoting bacteria in metal phytoremediation. Adv. Microb. Physiol. 71, 97–132. doi: 10.1016/bs.ampbs.2017.04.001
Körner, C. (2004). Mountain biodiversity, its causes and function. Ambio 33, 11–17. doi: 10.1007/0044-7447-33.sp13.11
Körner, C. (2011). Coldest places on earth with angiosperm plant life. Alp. Bot. 121, 11–22. doi: 10.1007/s00035-011-0089-1
Körner, C. (2021). Life under and in snow: protection and limitation. Alpine Plant Life. 89–118. doi: 10.1007/978-3-030-59538-8_5
Körner, C., and Ohsawa, M. (2005). “Mountain systems” in Ecosystems and human well-being: Current state and trends. eds. R. Hassan, R. Scholes, and N. Ash, vol. 1 (Washington DC: Island press), 681–716.
Kumar, N., Kumar, A., Jeena, N., Singh, R., and Singh, H. (2020). Factors influencing soil ecosystem and agricultural productivity at higher altitudes. 55–70. doi: 10.1007/978-981-15-1902-4_4,
Kumar, A., Kumari, M., Swarupa, P., and Shireen, (2019). Characterization of pH dependent growth response of agriculturally important microbes for development of plant growth promoting bacterial consortium. J. Pure and Applied Microbiol. 13, 1053–1061. doi: 10.22207/JPAM.13.2.43
Lahsini, A., Sallami, A., Ait-ouakrim, E. H., El, H., Obtel, M., Douira, A., et al. (2022). Rhizosphere isolation and molecular identification of an indigenous abiotic stress-tolerant plant growth-promoting rhizobacteria from the rhizosphere of the olive tree in southern Morocco. Rhizosphere 23:100554. doi: 10.1016/j.rhisph.2022.100554
Leontidou, K., Genitsaris, S., Papadopoulou, A., Kamou, N., Bosmali, I., Matsi, T., et al. (2020). Plant growth promoting rhizobacteria isolated from halophytes and drought-tolerant plants: genomic characterisation and exploration of phyto-beneficial traits. Sci. Rep. 10, 14857–14815. doi: 10.1038/s41598-020-71652-0
Li, J., Li, C., Kou, Y., Yao, M., He, Z., and Li, X. (2020). Distinct mechanisms shape soil bacterial and fungal co-occurrence networks in a mountain ecosystem. FEMS Microbiol. Ecol. 96, 1–12. doi: 10.1093/femsec/fiaa030
Li, H. B., Singh, R. K., Singh, P., Song, Q. Q., Xing, Y. X., Yang, L. T., et al. (2017). Genetic diversity of nitrogen-fixing and plant growth promoting pseudomonas species isolated from sugarcane rhizosphere. Front. Microbiol. 8, 1–20. doi: 10.3389/fmicb.2017.01268
Liengen, T. (1999). Environmental factors influencing the nitrogen fixation activity of free-living terrestrial cyanobacteria from a high arctic area, Spitsbergen. Can. J. Microbiol. 45, 573–581. doi: 10.1139/w99-040
López-Angulo, J., Pescador, D. S., Sánchez, A. M., Luzuriaga, A. L., Cavieres, L. A., and Escudero, A. (2019). Alpine vegetation dataset from three contrasting mountain ranges differing in climate and evolutionary history. Data Brief 27:104816. doi: 10.1016/j.dib.2019.104816
Majeed, A., Muhammad, Z., and Ahmad, H. (2018). Plant growth promoting bacteria: role in soil improvement, abiotic and biotic stress management of crops. Plant Cell Rep. 37, 1599–1609. doi: 10.1007/s00299-018-2341-2
Maldonado, J. E., Gaete, A., Mandakovic, D., Aguado-Norese, C., Aguilar, M., Gutiérrez, R. A., et al. (2022). Partners to survive: Hoffmannseggia doellii root-associated microbiome at the Atacama Desert. New Phytol. 234, 2126–2139. doi: 10.1111/nph.18080
Måren, I. E., Karki, S., Prajapati, C., Yadav, R. K., and Shrestha, B. B. (2015). Facing north or south: does slope aspect impact forest stand characteristics and soil properties in a semiarid trans-Himalayan valley? J. Arid Environ. 121, 112–123. doi: 10.1016/j.jaridenv.2015.06.004
McMaster, G. S., and Wilhelm, W. W. (1997). Growing degree-days: one equation, two interpretations. Agriculture, Forest and Meteorology. 87, 291–300. doi: 10.1016/S0168-1923(97)00027-0
Mendoza-Hernández, J. C., Perea-Vélez, S., Arriola Morales, J., Martínez-Simón, S. M., and Pérez-Osorio, G. (2016). Assessing the effects of heavy metals in ACC deaminase and IAA production on plant growth-promoting bacteria. Microbiol. Res. 188-189, 53–61. doi: 10.1016/j.micres.2016.05.001
Moreira, A., and Cereceda, P. (2013). Diversidad y fragilidad del paisaje botánico de chile mediterráneo. Revista Chagual, XI 11, 30–40.
Mujahid, T. Y., Subhan, S. A., Wahab, A., Masnoon, J., Ahmed, N., and Abbas, T. (2015). Effects of different physical and chemical parameters on phosphate solubilization activity of plant growth promoting bacteria isolated from indigenous soil. J. Pharmacy and Nutrition Sciences 5, 64–70. doi: 10.6000/1927-5951.2015.05.01.10
Naylor, D., and Coleman-Derr, D. (2018). Drought stress and root-associated bacterial communities. Front. Plant Sci. 8, 1–16. doi: 10.3389/fpls.2017.02223
Nuñez, C. I., Aizen, M. A., and Ezcurra, C. (1999). Species associations and nurse plant effects in patches of high-Andean vegetation. J. Veg. Sci. 10, 357–364. doi: 10.2307/3237064
Olanrewaju, O. S., Glick, B. R., and Babalola, O. O. (2017). Mechanisms of action of plant growth promoting bacteria. World J. Microbiol. Biotechnol. 33, 197–116. doi: 10.1007/s11274-017-2364-9
Orozco-Mosqueda, M. D. C., Flores, A., Rojas-Sánchez, B., Urtis-Flores, C. A., Morales-Cedeño, L. R., Valencia-Marin, M. F., et al. (2021). Plant growth-promoting bacteria as bioinoculants: attributes and challenges for sustainable crop improvement. Agronomy 11, 1–15. doi: 10.3390/agronomy11061167
Pande, A., Pandey, P., Mehra, S., Singh, M., and Kaushik, S. (2017). Phenotypic and genotypic characterization of phosphate solubilizing bacteria and their efficiency on the growth of maize. J. Genetic Engineering and Biotechn. 15, 379–391. doi: 10.1016/j.jgeb.2017.06.005
Pandey, A., and Yarzábal, L. A. (2019). Bioprospecting cold-adapted plant growth promoting microorganisms from mountain environments. Appl. Microbiol. Biotechnol. 103, 643–657. doi: 10.1007/s00253-018-9515-2
Penrose, D. M., and Glick, B. R. (2003). Methods for isolating and characterizing ACC deaminase-containing plant growth-promoting rhizobacteria. Physiol. Plant. 118, 10–15. doi: 10.1034/j.1399-3054.2003.00086.x
Pérez-Jaramillo, J. E., Carrión, V. J., de Hollander, M., and Raaijmakers, J. M. (2018). The wild side of plant microbiomes. Microbiome 6, 143–149. doi: 10.1186/s40168-018-0519-z
Pfanzelt, S., Grau, J., and Rodríguez, R. (2008). A vegetation map of Nevados De Chillan volcanic complex, Bio-Bio region, Chile. Gayana. Botánica 65, 209–219. doi: 10.4067/s0717-66432008000200007
Pikovskaya, R. I. (1948). Mobilization of phosphorus in soil in connection with vital activity of some microbial species. Mikrobiologiya 17, 362–370.
Piper, F. I., Viñegla, B., Linares, J. C., Camarero, J. J., Cavieres, L. A., and Fajardo, A. (2016). Mediterranean and temperate treelines are controlled by different environmental drivers. J. Ecol. 104, 691–702. doi: 10.1111/1365-2745.12555
Preem, J.-K., Truu, J., Truu, M., Mander, Ü., Oopkaup, K., Lõhmus, K., et al. (2012). Bacterial community structure and its relationship to soil physico-chemical characteristics in alder stands with different management histories. Ecol. Eng. 49, 10–17. doi: 10.1016/j.ecoleng.2012.08.034
Rahman, I. U., Afzal, A., Iqbal, Z., Hart, R., Abd Allah, E. F., Alqarawi, A. A., et al. (2020). Response of plant physiological attributes to altitudinal gradient: plant adaptation to temperature variation in the Himalayan region. Sci. Total Environ. 706:135714. doi: 10.1016/j.scitotenv.2019.135714
Ramakrishna, W., Yadav, R., and Li, K. (2019). Plant growth promoting bacteria in agriculture: two sides of a coin. Appl. Soil Ecol. 138, 10–18. doi: 10.1016/j.apsoil.2019.02.019
Rawat, P., Das, S., Shankhdhar, D., and Shankhdhar, S. C. (2021). Phosphate-solubilizing microorganisms: mechanism and their role in phosphate Solubilization and uptake. J. Soil Sci. Plant Nutr. 21, 49–68. doi: 10.1007/s42729-020-00342-7
Reddy, G. S. N., Prabagaran, S. R., and Shivaji, S. (2008). Leifsonia pindariensis sp. nov., isolated from the Pindari glacier of the Indian Himalayas, and emended description of the genus Leifsonia. Int. J. Syst. Evol. Microbiol. 58, 2229–2234. doi: 10.1099/ijs.0.65715-0
Rodríguez, R., Grau, J., Baeza, C., and Davies, A. (2008). Lista comentada de las plantas vasculares de Nevados de Chillán, Chile. Gayana Botanica 65, 153–197. doi: 10.4067/S0717-66432008000200005
Rodríguez, R., Marticorena, C., Alarcón, D., Baeza, C., Cavieres, L., Finot, V. L., et al. (2018). Catálogo de las plantas vasculares de Chile. Gayana Botánica 75, 1–430. doi: 10.4067/S0717-66432018000100001
Rodríguez-Echeverría, S., Delgado-Baquerizo, M., Morillo, J. A., Gaxiola, A., Manzano, M., Marquet, P. A., et al. (2021). Azorella cushion plants and aridity are important drivers of soil microbial communities in Andean ecosystems. Ecosystems 24, 1576–1590. doi: 10.1007/s10021-021-00603-1
Rousk, K., Sorensen, P. L., and Michelsen, A. (2018). What drives biological nitrogen fixation in high arctic tundra: moisture or temperature. Ecosphere 9:2117. doi: 10.1002/ecs2.2117
Ruberto, L. A. M., Vazquez, S., Lobalbo, A., and Mac Cormack, W. P. (2005). Psychrotolerant hydrocarbon-degrading Rhodococcus strains isolated from polluted Antarctic soils. Antarct. Sci. 17, 47–56. doi: 10.1017/S0954102005002415
Rueda-Puente, E. O., Bianciotto, O., Farmohammadi, S., Zakeri, O., Elías, J. L., Hernández-Montiel, L. G., et al. (2019). “Plant growth-promoting bacteria associated to the halophyte Suaeda maritima (L.)” in Abbas, Iran BT - Sabkha ecosystems. eds. B. Gul, B. Böer, M. A. Khan, M. Clüsener-Godt, and A. Hameed, vol. VI (New York: Springer International Publishing), 289–300.
Rui, J., Hu, J., Wang, F., Zhao, Y., and Li, C. (2022). Altitudinal niches of symbiotic, associative and free-living diazotrophs driven by soil moisture and temperature in the alpine meadow on the Tibetan plateau. Environ. Res. 211:113033. doi: 10.1016/j.envres.2022.113033
Rumpf, S. B., Hülber, K., Klonner, G., Moser, D., Schütz, M., Wessely, J., et al. (2018). Range dynamics of mountain plants decrease with elevation. Proc. Natl. Acad. Sci. U. S. A. 115, 1848–1853. doi: 10.1073/pnas.1713936115
Scherrer, D., and Körner, C. (2011). Topographically controlled thermal-habitat differentiation buffers alpine plant diversity against climate warming. J. Biogeogr. 38, 406–416. doi: 10.1111/j.1365-2699.2010.02407.x
Schoebitz, M., Ceballos, C., and Ciampi, L. (2013). Effect of immobilized phosphate solubilizing bacteria on wheat growth and phosphate uptake. J. Soil Sci. Plant Nutr. 13, 1–10. doi: 10.4067/s0718-95162013005000001
Selvakumar, G., Kundu, S., Joshi, P., Nazim, S., Gupta, A. D., Mishra, P. K., et al. (2008). Characterization of a cold-tolerant plant growth-promoting bacterium Pantoea dispersa 1A isolated from a sub-alpine soil in the North Western Indian Himalayas. World J. Microbiol. Biotechnol. 24, 955–960. doi: 10.1007/s11274-007-9558-5
Senthilkumar, M., Amaresan, N., and Sankaranarayanan, A. (2021). “Estimation of malondialdehyde (MDA) by Thiobarbituric acid (TBA) assay” in Plant-microbe interactions (Humana, New York, NY: Springer Protocols Handbooks)
Sezen, A., Ozdal, M., Kubra, K. O. C., and Algur, O. F. (2016). Isolation and characterization of plant growth promoting rhizobacteria (PGPR) and their effects on improving growth of wheat. J. Appl. Biol. Sci. 10, 41–46.
Shin, Y., Lee, B. H., Lee, K. E., and Park, W. (2020). Pseudarthrobacter psychrotolerans sp. nov., a cold-adapted bacterium isolated from Antarctic soil. Int. J. Syst. Evol. Microbiol. 70, 6106–6114. doi: 10.1099/ijsem.0.004505
Sierra-Almeida, A., Reyes-Bahamonde, C., and Cavieres, L. A. (2016). Drought increases the freezing resistance of high-elevation plant species from central Chilean Andes. Oecologia 181, 1011–1023. doi: 10.1007/s00442-016-3622-5
Siles, J. A., and Margesin, R. (2016). Abundance and diversity of bacterial, Archaeal, and fungal communities along an altitudinal gradient in alpine Forest soils: what are the driving factors? Microb. Ecol. 72, 207–220. doi: 10.1007/s00248-016-0748-2
Sinegani, A. A. S., and Younessi, N. (2017). Antibiotic resistance of bacteria isolated from heavy metal-polluted soils with different land uses. J. Global Antimicrobial Resistance 10, 247–255. doi: 10.1016/j.jgar.2017.05.012
Sklenář, P., Romoleroux, K., Muriel, P., Jaramillo, R., Bernardi, A., Diazgranados, M., et al. (2021). Distribution changes in Páramo plants from the equatorial high Andes in response to increasing temperature and humidity variation since 1880. Alp. Bot. 131, 201–212. doi: 10.1007/s00035-021-00270-x
Squeo, F. A., Arancio, G., and Gutiérrez, J. R. (2001). Libro rojo de la flora nativa y de los sitios prioritarios para su conservación: Región de Coquimbo. eds. G. Arancio and J. R. Gutiérrez (Gutiérrez La Serena, Chile: Ediciones Universidad de La Serena), 137–163.
Squeo, F. A., Arancio, G., Gutiérrez, J. R., Letelier, L., Arroyo, M. T. K., León-Lobos, P. Y., et al. (2008). Flora amenazada de la Región de Atacama y estrategias para su conservación. Anexo acuerdos taller de la Ley de Bosque Nativo de la Macro Zona Norte. Ediciones de la Universidad de La Serena, 71–72.
Stres, B., Danevčič, T., Pal, L., Fuka, M. M., Resman, L., Leskovec, S., et al. (2008). Influence of temperature and soil water content on bacterial, archaeal and denitrifying microbial communities in drained fen grassland soil microcosms. FEMS Microbiol. Ecol. 66, 110–122. doi: 10.1111/j.1574-6941.2008.00555.x
Tang, M., Li, L., Wang, X., You, J., Li, J., and Chen, X. (2020). Elevational is the main factor controlling the soil microbial community structure in alpine tundra of the Changbai Mountain. Sci. Rep. 10, 12442–12415. doi: 10.1038/s41598-020-69441-w
Varas, B., Castro, M. H., Rodriguez, R., Von Baer, D., Mardones, C., and Hinrichsen, P. (2013). Identification and characterization of microsatellites from calafate (Berberis microphylla, Berberidaceae). Applications in Plant Sciences 1:1200003. doi: 10.3732/apps.1200003
Vega-Celedón, P., Bravo, G., Velásquez, A., Cid, F. P., Valenzuela, M., Ramírez, I., et al. (2021). Microbial diversity of psychrotolerant bacteria isolated from wild flora of Andes mountains and Patagonia of Chile towards the selection of plant growth-promoting bacterial consortia to alleviate cold stress in plants. Microorganisms 9, 1–28. doi: 10.3390/microorganisms9030538
Viale, M., Bianchi, E., Cara, L., Ruiz, L. E., Villalba, R., Pitte, P., et al. (2019). Contrasting climates at both sides of the Andes in Argentina and Chile 7, 1–15. doi: 10.3389/fenvs.2019.00069,
Viruel, E., Lucca, M. E., and Siñeriz, F. (2011). Plant growth promotion traits of phosphobacteria isolated from Puna, Argentina. Arch. Microbiol. 193, 489–496. doi: 10.1007/s00203-011-0692-y
Vives-Peris, V., Molina, L., Segura, A., Gómez-Cadenas, A., and Pérez-Clemente, R. M. (2018). Root exudates from citrus plants subjected to abiotic stress conditions have a positive effect on rhizobacteria. J. Plant Physiol. 228, 208–217. doi: 10.1016/j.jplph.2018.06.003
Wakelin, S. A., Gupta, V. V. S. R., and Forrester, S. T. (2010). Regional and local factors affecting diversity, abundance and activity of free-living, N2-fixing bacteria in Australian agricultural soils. Pedobiologia 53, 391–399. doi: 10.1016/j.pedobi.2010.08.001
Wang, C., Michalet, R., Liu, Z., Jiang, X., Wang, X., Zhang, G., et al. (2020). Disentangling large- and small-scale abiotic and biotic factors shaping soil microbial communities in an alpine cushion plant system. Front. Microbiol. 11, 1–17. doi: 10.3389/fmicb.2020.00925
Widawati, S., and Suliasih, S. (2006). The population of phosphate solubilizing bacteria (PSB) from Cikaniki, Botol Mountain, and Ciptarasa area, and the ability of PSB to solubilize insoluble P in solid pikovskaya medium. Biodiversitas J. Biological Diversity 7, 109–113. doi: 10.13057/biodiv/d070203
Xiong, Y. W., Li, X. W., Wang, T. T., Gong, Y., Zhang, C. M., Xing, K., et al. (2020). Root exudates-driven rhizosphere recruitment of the plant growth-promoting rhizobacterium Bacillus flexus KLBMP 4941 and its growth-promoting effect on the coastal halophyte Limonium sinense under salt stress. Ecotoxicol. Environ. Saf. 194:110374. doi: 10.1016/j.ecoenv.2020.110374
Yadav, A. N., Sachan, S. G., Verma, P., and Saxena, A. K. (2015). Prospecting cold deserts of northwestern Himalayas for microbial diversity and plant growth promoting attributes. J. Biosci. Bioeng. 119, 683–693. doi: 10.1016/j.jbiosc.2014.11.006
Yang, J., El-Kassaby, Y. A., and Guan, W. (2020). The Effect of slope aspect on vegetation attributes in a mountainous dry valley, Southwest China. Sci. Rep., 10, 1–11. doi: 10.1038/s41598-020-73496-0
Yang, F., Niu, K., Collins, C. G., Yan, X., Ji, Y., Ling, N., et al. (2019). Grazing practices affect the soil microbial community composition in a Tibetan alpine meadow. Land Degrad. Dev. 30, 49–59. doi: 10.1002/ldr.3189
Yarzábal, L. A. (2014). “Cold-tolerant phosphate-solubilizing microorganisms and agriculture development in mountainous regions of the world” in Phosphate solubilizing microorganisms eds. K. Mohammad Saghir, Z. Almas, and M. Javed. (Cham: Springer), 113–135.
Yoon, S. H., Ha, S. M., Kwon, S., Lim, J., Kim, Y., Seo, H., et al. (2017). Introducing EzBioCloud: a taxonomically united database of 16S rRNA and whole genome assemblies. Int. J. Syst. Evol. Microbiol. 67, 1613–1617. doi: 10.1099/ijsem.0.001755
Youssef, R. A., and Chino, M. (1989). Root-induced changes in the rhizosphere of plants. I. pH changes in relation to the bulk soil. Soil Sci. Plant Nutr. 35, 461–468. doi: 10.1080/00380768.1989.10434779
Keywords: alpine, ACC deaminase, extreme temperatures, IAA, nitrogenase, soil bacteria, xerophytic plants
Citation: Aguilera-Torres C, Riveros G, Morales LV, Sierra-Almeida A, Schoebitz M and Hasbún R (2023) Relieving your stress: PGPB associated with Andean xerophytic plants are most abundant and active on the most extreme slopes. Front. Microbiol. 13:1062414. doi: 10.3389/fmicb.2022.1062414
Edited by:
Paola Grenni, National Research Council, ItalyReviewed by:
Maria Ludovica Saccà, Council for Agricultural and Economics Research (CREA), ItalyNi Luh Suriani, Udayana University, Indonesia
Copyright © 2023 Aguilera-Torres, Riveros, Morales, Sierra-Almeida, Schoebitz and Hasbún. This is an open-access article distributed under the terms of the Creative Commons Attribution License (CC BY). The use, distribution or reproduction in other forums is permitted, provided the original author(s) and the copyright owner(s) are credited and that the original publication in this journal is cited, in accordance with accepted academic practice. No use, distribution or reproduction is permitted which does not comply with these terms.
*Correspondence: Angela Sierra-Almeida, YW5nZWxhc2llcnJhQHVkZWMuY2w=
†These authors have contributed equally to this work