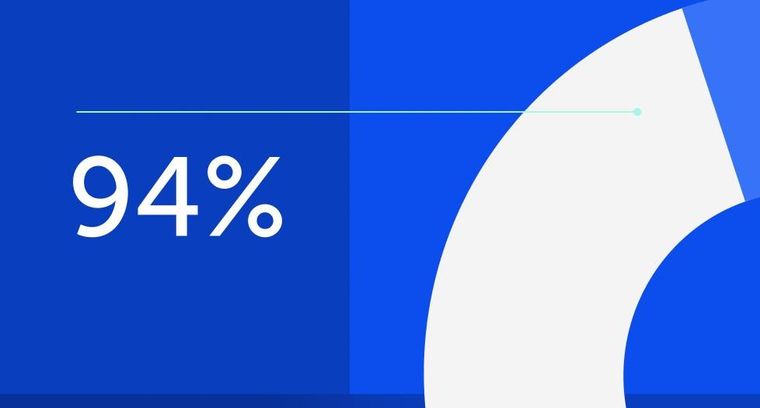
94% of researchers rate our articles as excellent or good
Learn more about the work of our research integrity team to safeguard the quality of each article we publish.
Find out more
REVIEW article
Front. Microbiol., 02 December 2022
Sec. Antimicrobials, Resistance and Chemotherapy
Volume 13 - 2022 | https://doi.org/10.3389/fmicb.2022.1061603
Excessive antibiotic prescriptions as well as their misuse in agriculture are the main causes of antimicrobial resistance which poses a growing threat to public health. It necessitates the search for novel chemicals to combat drug resistance. Since ancient times, naturally occurring medicines have been employed and the enormous variety of bioactive chemicals found in nature has long served as an inspiration for researchers looking for possible therapeutics. Secondary metabolites from microorganisms, particularly those from actinomycetes, have made it incredibly easy to find new molecules. Different actinomycetes species account for more than 70% of naturally generated antibiotics currently used in medicine, and they also produce a variety of secondary metabolites, including pigments, enzymes, and anti-inflammatory compounds. They continue to be a crucial source of fresh chemical diversity and a crucial component of drug discovery. This review summarizes some uncommon sources of antifungal metabolites and highlights the importance of further research on these unusual habitats as a source of novel antimicrobial molecules.
Fungal diseases are already a global threat that is getting worse in the time of the ongoing COVID-19 pandemic, especially in developing countries. This pandemic is a troubling reminder of the fact that vulnerability still exists in the modern world. Even today, communicable diseases continue to be the world’s leading cause of death (WHO, 2019). Unfortunately, healthcare authorities have underestimated some of these microbial hazards in the past, even though they endanger the lives of millions of people every year. An important example of such overlooked diseases is fungal infections. Even with significant developments in the field of medicine in the past few decades, the spread of fungal infections is continuously on the rise. Furthermore, the long-term therapeutic use of antifungal medicines in patients has led to the emergence of multi-drug resistant fungal strains, including the particularly aggressive, Candida auris. The field of clinical mycology is dynamic and continually shifting. New risk factors for atypical mycoses have emerged as a result of a new therapy for malignant and autoimmune disorders. In transplant patients or people with cancer, the use of antifungal agents (such as triazoles or echinocandin) has been successful in reducing the occurrence of invasive fungal diseases but at the same time, has increased the risk of infections caused by non-fumigatus Aspergillus sp. in such patients (Husain and Camargo, 2019). There has been an emergence of azole-resistant in clinical and environmental isolates because of the widespread use of triazole insecticides in agriculture (Price et al., 2015). Candida auris, a new yeast species that was unknown barely a decade ago, is now spreading across the globe causing massive healthcare-related outbreaks in about 40 countries on 6 continents (Du et al., 2020). There has also been a recurrence of a once-rare fungal infection caused by the opportunistic Fusarium sp. in immunocompromised patients worldwide due to developing resistance to various antifungal drugs (Taj-Aldeen, 2017). One important reason contributing to the dramatic rise of fungal infections is the increase in the vulnerable population, such as the elderly, critically ill, or immunocompromised individuals. The rising figures of AIDS, cancer, and transplant patients along with the widespread use of immune-modulating medications and antibiotics, are some risk factors for the spread of FIs (Friedman and Schwartz, 2019; Lockhart and Guarner, 2019). Also, as medical equipment like catheters become more widely used, the danger of biofilm formation increases (Khatoon et al., 2018). Biofilms are a collection of highly diverse and well-organized cells that protect against physical and chemical threats. Biofilms are generally resistant to present treatments and are thought to be a major contributor to the high mortality rates in invasive fungal infections (Pierce et al., 2015). In addition to their direct effects on people, fungi also endanger food security by wreaking havoc on the crops that provide food for billions of people and by creating chemicals that contaminate food sources and cause cancer (Fisher et al., 2012). Mass deaths of bats and amphibians are endangering biodiversity, and an unusual number of fungal diseases have recently been responsible for the extinction of wild species (Fisher et al., 2020). As global temperatures rise and pests and pathogens migrate northward, with fungi leading the way, climate change is likely to make matters worse (Lazar et al., 2022). The rate of developing antifungals has been much slower as compared to that of antibacterial agents. One of the main reasons for this is the fact that fungi are eukaryotic organisms and most agents that have antifungal properties are also toxic to their eukaryotic host, i.e., humans. The key requirement for an antifungal drug is that the eukaryotic machinery of the fungal pathogen is selectively disabled or destroyed while causing little or no damage to the host cellular activities (Odds et al., 2003). Despite this limitation, several agents have been developed in the past along with numerous ongoing studies. According to the figures from 2012, just 0.005 percent of new pharmaceuticals made from synthetic molecules are successful, compared to 0.6 percent and 1.6 percent, respectively, for the development of complete natural items and natural microbial products (Bérdy, 2012). The majority of medications on the market today were created through the synthesis or semi-synthesis of natural compounds and microbes continue to be a valuable source for the creation of new natural products (Newman and Cragg, 2020). Gene modification can be used to alter the bioactive molecules collected from bacteria to create better or ideal pharmaceuticals, which are then mass manufactured. The overview of the review is given in Figure 1.
Many superficial mycoses have been treated with a range of topical medicines that have had moderate efficacy in reducing common infections over the last century. The fact that there are limited antifungal medications available to treat systemic fungal infections increases the impact of fungus on human health (Perfect, 2017). There are only four primary classes of available antifungal drugs, which include- polyenes, flucytosine, azoles, and echinocandins (Carmona and Limper, 2017). Many opportunistic fungi such as Candida auris and Lomentospora prolificans are innately resistant to many antimycotics (Arastehfar et al., 2020). Some species, like C. glabrata, have strong intrinsic resistance to antifungals like azoles, while other species such as C. albicans, can acquire resistance to antifungals after being exposed to them, mainly during preventive treatments (Arastehfar et al., 2020). Despite the limited number of antimicrobials available, microorganisms have a remarkable capacity to develop resistance to them, posing a serious threat to public health. Fungal pathogens, which can only be treated with a limited number of antifungal medications and produce catastrophic invasive infections, are of particular concern. The rates of resistance for fungal infections vary by geography, species, and accessible treatments; nonetheless, the spread of drug-resistant fungus species is global. In general, fungal pathogens’ innate and acquired drug resistance strategies involve lowering the effective drug concentration, changing the drug target, or rerouting antifungal toxicity through metabolic change (Sanglard, 2016).
Some of the challenges that hinder antifungal drug development can be summarized as follows:
• Current antifungal medicines have not been as efficient to reduce mortality caused by fungal infections and the efficacy of available antifungal therapies has reached a stage of plateau.
• Also, there has to be a larger focus on developing drugs having faster fungicidal efficacy to improve clinical outcomes. The current treatment course for typical antifungal medicines is far too long, posing the risk of inadequate short-term fungicidal effectiveness, decreased patient compliance and/or tolerance, or even the formation of direct antifungal drug resistance (Cuenca-Estrella, 2014).
• There is a need to broaden the antifungal spectrum to include some developing, extremely drug-resistant fungi (like Candida auris) that are increasingly being seen in hospitalized patients who are immunosuppressed due to other serious underlying conditions.
• To boost potency and prevent the establishment of drug resistance, it is critical to have an optimum mix of therapeutic agents or classes. Some antifungal drug classes, such as azoles and echinocandins, are seeing an increase in resistant strains (Smith et al., 2015).
Many novel actinobacteria have been published with the hypothesis that novel strains from previously undiscovered sources could be great targets for the discovery of bioactive substances (van Bergeijk et al., 2020). Table 1 lists the novel natural antifungal compounds that have been isolated from actinomycetes since 2011. Several recent research on natural product discovery has concentrated on the examination of insect-microbe symbioses and especially, interactions between insects and actinobacteria because bacterial symbionts need small molecules to communicate with the host or take part in host defence (Chouvenc et al., 2018; Simon et al., 2022). The associations between endosymbiotic bacteria with arthropods can vary from facultative to obligate mutualisms; these bacteria can assist with nutrient uptake, promote resistance to plant secondary metabolite, and chemical pollutant and pesticide detoxification (Zhao et al., 2022), while some symbionts exude antifungals to inhibit the proliferation of the arthropods’ fungal antagonists (Kett et al., 2021). Firebugs and the European beewolf, which harbors antibiotic-producing Streptomyces in their antennae to help protect their larvae from fungus infections, are two examples of the importance of defensive secondary metabolites in insect-Actinobacteria symbiosis (Kaltenpoth et al., 2012; Salem et al., 2013). Similar to this, ants that cultivate fungi of the Attini species have symbiotic Pseudonocardia that aid in guarding the ants’ fungi against specific pathogens (Oh et al., 2009; Poulsen et al., 2010). Other insects, such as dung beetles (Scarabaeidae: Copris tripartitus), and fungus-growing termites, have also been documented to harbor insect-associated Actinobacteria (Kurtböke et al., 2015; Um et al., 2015).
Because the host must endure exposure to these substances, bacteria found in associations with animal hosts are likely to generate antimicrobial chemicals that are less harmful than those found in soil bacteria. Figure 2 depicts the distribution of microorganisms and their metabolites from different habitats mentioned in this review.
Cockroaches have been found to carry members of several related bacterial families, most notably the Enterobacteriaceae, Staphylococcaceae, and Mycobacteriaceae species. The most often reported genera from the cockroach gut were Streptomyces, Bacillus, Enterococcus, and Pseudomonas (Guzman and Vilcinskas, 2020). Feces of the cockroach Cryptocercus punctulatus demonstrated antifungal action linked to compounds made by gut microorganisms (Rosengaus et al., 2013). A species of actinobacteria, Streptomyces globisporus WA5-2-37 that produces Actinomycin X2 and Collismycin, was just recently discovered in the intestine of Periplaneta americana (Chen et al., 2020). Bacillus atrophaeus, Bacillus subtilis, and Pseudomonas reactans are a few bacterial strains that have been successfully isolated from the gut of Blatella germanica and have the ability to limit the growth of entomopathogenic fungus Beauveria bassiana (Huang et al., 2013).
Vertebrate skin microbiomes act as a defense against infections and can influence disease risk. Higher bacterial species richness, certain microbial community assemblages, and the presence of microorganisms that produce compounds that impede pathogen growth have all been linked to lower infection risk in vertebrates. Many amphibians’ population declines and extinctions have been attributed to the fungal pathogen Batrachochytrium dendrobatidis (Bd), which causes chytridiomycosis (Berger et al., 2016). Since these circumstances match with the pathogen’s ideal development range, chytridiomycosis has primarily afflicted tropical amphibian populations at high elevations and during cooler seasons (Sapsford et al., 2013). Amphibians have a variety of defence mechanisms, including the presence of skin bacteria that create antifungal metabolites. For example, the bacteria Janthinobacterium lividum, which lives in the salamander Plethodon cinereus, produces anti-Bd secondary metabolites such as violacein and indole-3-carboxaldehyde (Brucker et al., 2008).
Communities of symbiotic microorganisms are found in insect digestive systems, and these communities are the focus of research on microbial diversity and new bioactive microbial compounds (Breznak, 2003). Fungus-farming termites belonging to the subfamily Macrotermitinae, cultivate basidiomycetes (genus Termitomyces) as their main source of food (Lever et al., 2015) and these basidiomycetes are constantly susceptible to parasitism by other fungi. The termites probably use a variety of tactics to manage such antagonists, with the production of antifungals being one among them (Um et al., 2013). The gut flora of the Macrotermitinae has so far been found to contain seven different bacterial phyla (Otani et al., 2014). Bacillus strains are the most prevalent and can produce antifungals among them. An initial analysis of an extract of the Bacillus strain cultures using liquid chromatography and mass spectrometry identified a significant secondary metabolite: Bacillaene, a polyene polyketide that is present in all strains and which inhibits the growth of Pseudoxylaria, Trichoderma, and Fusarium (Um et al., 2013). From the nests of the French Guiana termite Nasutitermes corniger, the fungus Neonectria discophora SNB-CN63 was isolated (Sorres et al., 2018) that produced Ilicicolinic acid C and D. These are non-macrocyclic, non-polycyclic polyketide that showed antifungal activities against Trichophyton rubrum and Candida albicans (Nirma et al., 2015). There is proof that the hindgut microbiota of the termite Zootermopsis angusticollis produces many functionally active b-1,3-glucanases with a probable involvement in fungal pathogen protection (Rosengaus et al., 2014). Termites also support the Streptomyces species, which makes the antifungal Natalamycin (Kim et al., 2014). Other antibiotics known as microtermolides A and B are produced by the Streptomyces strain connected to the fungus-farming termites (Carr et al., 2012).
The solitary wasps Sceliphron caementarium and Chalybion californicum are associated with Streptomyces spp. that produce Streptochlorin, a range of Piericidin analogs, and other chemicals that protect their larvae against bacteria and fungi (Poulsen et al., 2011). Sceliphrolactam, an antifungal substance, was discovered from Streptomyces that was associated to the mud dauber wasp Sceliphron caementarium (Oh et al., 2011). The substance is a polyene macrocyclic lactam with antifungal action against Candida albicans that is resistant to amphotericin B (Oh et al., 2011). Kumar et al. (2012, 2014) also reported novel species of Streptomyces from solitary wasp producing antimicrobial agents showing promising activity against drug resistant bacterial and fungal pathogens.
The leaf cutter ants have many defense mechanisms against the risk of pathogenic infection of their garden fungus, including a tripartite mutualistic interaction in which they host bacteria that produce antibiotics on their bodies (Barke et al., 2010). Numerous of these bacteria have coevolved with their hosts, creating antifungals to inhibit parasitic fungi (Escovopsis spp. and allied taxa), and in exchange, the ants feed the bacteria through special exocrine glands inside elaborate cuticular crypts, which also provide the bacteria with their preferred microclimate (Currie et al., 2006). L. gongylophorus, a mutualist fungus, is the only source of nourishment for Acromyrmex, which also harbors Pseudonocardia bacteria in its metapleural glands (Heine et al., 2018). Another fungus, Escovopsis, parasitizes the L. gongylophorus cultivar (Yek et al., 2012). To suppress Escovopsis, the Pseudonocardia produce various variations of the broad-spectrum polyene antifungal nystatin P1 (Holmes et al., 2016). Additionally, it has recently been discovered that Pseudonocardia associated with the attines Apterostigma dentigerum and Trachymyrmex cornetzi create novel cyclic depsipeptide substances known as gerumycins A-C (Holmes et al., 2016).
Cave habitats encourage the development of antimicrobials due to their unique properties, such as darkness, high humidity, consistent low temperature, and lack of nutrition which influence the microflora that thrives there (Ghosh et al., 2017). Such extreme environments have caught the attention of researchers due to the presence of undiscovered microbial communities in these caves. Due to a scarcity of nutrients in cave habitats, microorganisms become more competitive and adopt survival tactics such as secreting metabolites (antibiotics and enzymes; Ghosh et al., 2017). Various caves have been studied for potential bioactive metabolites all over the world. The Streptomyces genus has been found in a variety of caves in various countries including Thailand (Nakaew et al., 2009a), Turkey (Yücel and Yamaç, 2010), Pakistan (Zada et al., 2016), India (Yogita et al., 2012), China (Jiang et al., 2015), Russia (Axenov-Gibanov et al., 2016; Voytsekhovskaya et al., 2018), Canada (Riquelme et al., 2017), and Poland (Herold et al., 2005). Other Actinobacteria genera have also been found in cave habitats including Arthrobacter, Nocardia, Saccharothrix, Lentzea, Micrococcus, Nonomuraea, Spirillospora, Micromonospora, Microbacterium, Cryobacterium, and Lysinibacter (Lee et al., 2000; Ningthoujam et al., 2009; Nakaew et al., 2009b). But only a few bioactive substances have been documented so far and the chemical characteristics of the majority of the reported metabolites released by particular bacteria are unknown. The identified bioactive substances include undecylprodigiosin (Stankovic et al., 2012), xiakemycin and huanglongmycin (Jiang et al., 2015; Jiang L. et al., 2018), chaxalactin B (Castro et al., 2018), diazepinomicin (Gosse et al., 2019) from Streptomyces sp., a mixture of antibiotics of Streptomyces sp., Bacillus sp. and Bacillaceae (Axenov-Gibanov et al., 2016), a mixture of polyene and non-polyene metabolites of Streptomyces sp. and Penicillium sp. (Belyagoubi et al., 2018), lanthipeptides, polymyxin B, paenicidin B, fusaricidin, tridecaptin, and colistin A of Paenibacillus (Baindara et al., 2020; Lebedeva et al., 2021), lipids of Toxopsis calypsus and Phormidium melanochroun (Lamprinou et al., 2015).
Exocrine glands in arthropods secrete a variety of secondary compounds. The outer cuticle of the insect serves as the first line of defence against fungal infection. The first spider AMPs, known as lycotoxins-I and II from Lycosa carolinensis, possessed both antibacterial and antifungal properties that were able to prevent the growth of bacteria and the Candida glabrata yeast (Yan and Adams, 1998). Venom can have neurotoxic effects, but it can also have analgesic, anticancer, antiarrhythmic, and most importantly, antibacterial properties. Spider venom contains salts, acylpolyamines, peptides, enzymes, amino acids, nucleotides, and low molecular mass molecules (Vassilevski et al., 2009). The venom of Ornithoctonus hainana also contained an antibacterial substance called Oh-defensin that was active against both Gram-positive and Gram-negative bacteria as well as fungi (Zhao et al., 2014). When extracted from the hemolymph of scorpions of the species Androctonus australis, the peptide androctonin was found to be effective against both bacteria and fungus (Ehret-Sabatier et al., 1996). Melectin and halictines are two antimicrobial substances that can be found in the venom of Hymenopterans (Slaninová et al., 2011). Ponericins from the venom of the ponerine ant Pachicondyla gueldi has been observed to be effective against bacteria and yeasts (Orivel et al., 2001). Unknown toxins in the venom of the paper wasp Polistes flavus are also effective against Candida and Aspergillus niger (Prajapati and Upadhyay, 2016). The antimicrobial properties of the venom of wasps and bees have also been demonstrated in some studies. For instance, the crude venom of the wasp Vespa orientalis effectively exhibited antifungal efficacy against the fungal strain Candida albicans (Farag and Swaby, 2018). The venom of Pseudopolybia vespiceps wasps contains another mastoparan peptide that exhibits substantial antifungal properties against Candida albicans and Candida neoformans (Silva et al., 2017). Other recently discovered peptides with certain antifungal activities obtained from natural sources, i.e., animal secretions or microbes, have been summarized in Table 2.
Figure 3 depicts the old as well as emerging fungal targets along with their respective antifungal agents that are either currently available for use or undergoing clinical trials. Some of the fungal targets that have recently gained attention of researchers to develop novel antifungals are described below.
An interesting potential target for developing novel antifungal drugs is the covalent attachment of proteins to glycosylphosphatidylinositol (GPI), which anchors them to the plasma membrane (Yadav and Khan, 2018). The Eisai Company in Japan developed the novel antifungal drug E1210 (fosmanogepix or APX001), which inhibits an initial stage of the GPI-dependent anchoring of mannosylated cell wall proteins linked to the polysaccharide wall component; the cell wall becomes weaker as a result of this interruption, which stops the growth of fungi. The fourth enzyme in the GPI-anchoring pathway, Gwp1p, which is in charge of inositol acylation, is the target of E1210 (the mammalian homolog is not inhibited; Watanabe et al., 2012). In vitro tests showed that E1210 was highly effective against the majority of fungi, including strains that were resistant to triazoles and polyenes as well as yeasts (Candida species other than Candida krusei) and molds (Aspergillus species, Fusarium species, and black moulds; Miyazaki et al., 2011; Pfaller et al., 2011). In a recent study, fosmanogepix was also found to be effective against Fusariosis in immunocompromised mice (Alkhazraji et al., 2020). A second GPI inhibitor named gepinacin (Phenoxyacetanilide), which is a monocarboxylic amide with antifungal activity against various yeasts and molds was found (Ivanov et al., 2022). An essential acyltransferase needed for the manufacture of fungal GPI anchors, Gwt1, is selectively inhibited by gepinacin (McLellan et al., 2012). The GPI anchor synthetic pathway enzyme Mcd4 is inhibited by M720, a phosphoethanolamine transferase-I inhibitor with in vivo activity against Candida albicans (Mann et al., 2015). The SPT14 gene (YPL175W), which encodes the fungal UDP-glycosyltransferase catalytic subunit known as Spt14, is crucial for the production of GPI. A natural substance called jawsamycin (FR-900848) contains oligomeric cyclopropyl that targets the catalytic component Spt14 (Fu et al., 2020). Jawsamycin targets the fungal Spt14 but not the human PIG-A, although the human homolog PIG-A and the S. cerevisiae Spt14 share 40% similarity (Fu et al., 2020). Jawsamycin demonstrated antifungal action with broad-spectrum and potency against Mucorales fungi, such as Rhizopus oryzae and Absidia corymbifera, as well as Fusarium species, Scedosporium species, and Mucor circinelloides (Fu et al., 2020).
Enolase catalyzes the conversion of 2-phosphoglycerate (2-PG) and phosphoenolpyruvate in the penultimate phase of glycolysis (Ji et al., 2016). A genetic knockout of enolase in C. albicans has been demonstrated to decrease germination tube and hyphal development, leading to decreased virulence and growth rate, which is consistent with its participation in core metabolic activities (Ji et al., 2016). It’s interesting to note that while this enzyme performs its glycolytic functions in the cytoplasm, many bacterial and fungal species also express it on their cell surfaces. This has been seen in Aspergillus flavus, A. terrus, A. nidulans, Candida glabrata, Saccharomyces cerevisiae (Funk et al., 2016). The potential of A. fumigatus surface-expressed enolase to interact with human immunological regulators, such as factor H, factor-H-like protein 1 (FHL-1), C4b-binding protein (C4BP), and plasminogen, was demonstrated in a thorough investigation (Dasari et al., 2019). When human A549 epithelial cells or an epithelial monolayer were exposed to swollen A. fumigatus conidia covered with human plasminogen, cell retraction, as well as cellular metabolic activity, was observed to be lowered significantly (Dasari et al., 2019). The structure-guided design of a peptidomimetic or small molecule inhibitor that precisely targets the enolase plasminogen docking site is a potential route for novel therapies (Dasari et al., 2019). A thorough understanding of the interactions between the two proteins is necessary for this method. Although full-length human Type II plasminogen (4DUR) and fungal enolase from Saccharomyces cerevisiae (3ENL) have high-resolution crystal structures, the interface for their interactions has not yet been established (Law et al., 2012).
A vital mechanism that promotes fungal virulence and survivability is mannitol production. Mannitol is acyclic, six-carbon sterol alcohol that is produced by the major biosynthetic enzymes mannitol-2-dehydrogenase (M2DH) and mannitol-1-phosphate 5-dehydrogenase (M1P5DH). Mannitol is a molecule that is found in almost all the structures of fungi, including mycelia, fruiting bodies, and conidia. It functions as a molecule that stores carbohydrates, an osmolyte, and a source of reducing power (Meena et al., 2015). Mannitol synthesis is boosted during pathogenesis to take advantage of its capacity to squelch reactive oxygen species (ROS) and evade host defenses (Wyatt et al., 2014; Meena et al., 2015). Interest in the underlying mechanism of mannitol’s manufacture and secretion during fungal infection has increased because of the special and varied features of mannitol, particularly concerning the human disease. The fact that humans do not manufacture mannitol and hence lack corresponding enzymes adds to the appeal of targeting mannitol production pathways in fungus. The discovery and development of inhibitory drugs that selectively target these fungal enzymes are made much easier by the lack of a human equivalent.
Due to its involvement in vital activities like DNA and RNA synthesis, energy metabolism, and signal transmission, disruption of the purine and pyrimidine biosynthesis pathway using small molecules has been investigated as a potential strategy to produce antimicrobials (Trapero et al., 2018). It is crucial in the drug design process to achieve species-selective targeting of the biosynthesis enzymes since many of these are shared by humans, fungi, and bacteria (Chitty and Fraser, 2017). Thus, the creation of high potency and selectivity inhibitors will benefit greatly from a thorough understanding of the structural and kinetic properties of the fungal and human homologs of these enzymes. A novel substance known as ECC1385 that was discovered in a library of synthetic compounds has been demonstrated to inhibit C. neoformans and C. albicans GMP synthase activity (Rodriguez-Suarez et al., 2007). It exhibited whole cell action against a wide range of fungi and bacteria in vitro, including various species of Candida, A. fumigatus, C. neoformans and Staphylococcus aureus (Rodriguez-Suarez et al., 2007). Antiretroviral medicine azidothymidine (AZT; brand name RETROVIR) is being used to treat HIV demonstrated antifungal efficacy in vivo and in vitro for A. flavus (Wang X. et al., 2019). Since AZT has FDA approval, there is potential to employ the AZT scaffold to enhance its antimycotic capabilities or to repurpose it as an antifungal medication. F901318 (olorofim), which was found using an Aspergillus nidulans library screen, inhibits the enzyme dihydroorotate dehydrogenase (DHODH), which is responsible for catalyzing the fourth enzymatic stage of pyrimidine biosynthesis (Oliver et al., 2016). Despite being ineffective against Mucorales and Candida species, F901318 is quite effective in vitro against triazole-resistant Aspergillus, Scedosporium, Madurella, and Fusarium species (Law, 2016). Mammalian cells also have DHODH, however, F901318 is unable to inhibit the human version of the enzyme. In addition, olorofim exhibits effectiveness both in vitro and in vivo against A. fumigatus isolates that are resistant to other antifungal medications (Du Pré et al., 2018). The FDA designated olorofim as a “breakthrough drug therapy” in November 2019. It was subsequently given the orphan drug designation for the treatment of invasive aspergillosis, Lomentospora/Scedosporium infection (March 2020), and coccidiosis (June 2020), and just recently became an approved medication for the treatment of infectious diseases (June 2020; Wiederhold, 2020).
In eukaryotes, Hsp90 is a crucial molecular chaperone that controls the proper transport, maturation, and destruction of client proteins. In addition, Hsp90 controls the growth of mycelium, which is essential for virulence. Drug-resistant fungi are becoming an increasingly serious problem, and Hsp90 may offer a fresh way to address this growing danger (Arastehfar et al., 2020). Hsp90 impacts the survival, pathogenicity, and drug resistance of many diseases. The nucleotide-binding domains (NBD) of Hsp90 are conformationally flexible, according to research by Whitesell L. and colleagues in which they created CMLD013075, which had a more than 25-fold binding selectivity for fungal Hsp90 even though Hsp90 is extremely conserved (Huang et al., 2019; Whitesell et al., 2019). In contrast to previous Hsp90 inhibitors, CMLD013075 demonstrated little toxicity to mammalian cell lines (Whitesell et al., 2019). When used against resistant clinical isolates of Candida albicans, CMLD013075 was able to reduce the development of Candida albicans, and also improved the antifungal effects of azole antifungals. As a highly selective fungal Hsp90 inhibitor, CMLD013075 is a successful illustration of how IFIs can be treated by preventing fungal Hsp90 from doing its job. Histone deacetylase 2 (Hos2) controls the deacetylation of Hsp90, which is necessary for the interaction of Hsp90 with specific co-chaperones and the stability of several Hsp90 client proteins (van Daele et al., 2019). MGCD290 is a fungus Hos2 inhibitor created by MethylGene, Inc. of Montreal, Canada which not only prevents the deacetylation of histone proteins but also that of non-histone proteins like Hsp90 (Pfaller et al., 2015). Although MGCD290 only exhibits mild anti-Candida activity it exhibits good synergistic antifungal activity with azoles against a variety of Candida species strains that are resistant to several drugs in an in vitro setting (Pfaller et al., 2015). To increase the antifungal effectiveness of antifungal medications against drug-resistant pathogenic fungi, MGCD290 can be used as an adjuvant. It is necessary to confirm the antifungal efficacy of MGCD290 utilizing additional animal models of IFIs and, ultimately, in carefully planned clinical studies.
Chitin is produced by a family of membrane-localized chitin synthase enzymes and is necessary for cell survival (Chs1 to 3, and Chs8 in C. albicans). Due to their structural similarity, polyoxins and nikkomycins are powerful inhibitors of the Chs enzyme that compete with the Chs substrate UDP-GlcNAc for Chs binding, although they only have a little impact on whole cells (Shubitz et al., 2014; Ibe and Munro, 2021). Streptomyces tendae and Streptomyces ansochromogenes both generate the structurally related antifungal drugs nikkomycin X and nikkomycin Z, which have activity against a range of human fungal infections. The respiratory infections caused by Coccidioides species can be treated with nikkomycin Z (Shubitz et al., 2014). Recent research has led to the development of two new nikkomycin analogs, nikkomycin Px and Pz, which share the same antifungal properties as nikkomycin X and Z but exhibit significantly greater pH and temperature stability (Feng et al., 2014). Despite having specialized roles, the enzymes involved in the manufacture of chitin might become redundant in certain circumstances. The creation of effective chitin synthase inhibitors has also been hampered by minute variations in protein structure across members of the Chs family.
The lack of access to specific diagnostic tests and the restricted availability of antifungal drugs are the major challenges faced by clinicians to manage drug-resistant pathogens in present times. Also, the vast diversity of the fungal kingdom ensures an endless supply of new pathogens as well as new variants of known pathogens that are capable of adapting and evolving resistance themselves when exposed to antifungal agents. The key issue is that mostly in-vitro experiments have been used to investigate the activity of newly discovered antifungals. As the novel antifungal research moves forward, more studies using in-vivo infection models and subsequent clinical studies are required. Nevertheless, several naturally available agents all around us show promise in fending against the growing threat posed by fungi. There has been significant progress in identifying possible targets for new agents that take advantage of intrinsic dissimilarities between humans and fungi. Even while many of these targets are still hypothetical, some compounds have just recently started their early stages of clinical research. By increasing funding for research, exploring unexplored as well as underexplored sources of natural antimicrobials, taking into account novel alternative targets, repurposing already-existing non-antifungal medications, fortifying ties between academia and industry, and offering financial incentives for the development of new therapeutic options to treat these uncommon but deadly infections, we must address this unmet need.
MC and BN: data curation, writing–original draft preparation, and reviewing and editing. VijK: conceptualization, writing– original draft preparation, reviewing and editing, and supervision. AV: original draft preparation and reviewing, and editing. PS: original draft preparation, reviewing, and editing. VivK and SG: original draft preparation, reviewing, and editing. All authors contributed to the article and approved the submitted version.
MC thankful to Uttarakhand State Council for Science and Technology (UCOST) for providing fellowship and PEJS is thankful to the Helsinki University, Helsinki, Finland for Open access support.
The authors declare that the research was conducted in the absence of any commercial or financial relationships that could be construed as a potential conflict of interest.
All claims expressed in this article are solely those of the authors and do not necessarily represent those of their affiliated organizations, or those of the publisher, the editors and the reviewers. Any product that may be evaluated in this article, or claim that may be made by its manufacturer, is not guaranteed or endorsed by the publisher.
Alkhazraji, S., Gebremariam, T., Alqarihi, A., Gu, Y., Mamouei, Z., Singh, S., et al. (2020). Fosmanogepix (APX001) is effective in the treatment of immunocompromised mice infected with invasive pulmonary scedosporiosis or disseminated fusariosis. Antimicrob. Agents Chemother. 64, e01735–e01719. doi: 10.1128/AAC.01735-19
Arastehfar, A., Gabaldón, T., Garcia-Rubio, R., Jenks, J. D., Hoenigl, M., Salzer, H. J., et al. (2020). Drug-resistant fungi: an emerging challenge threatening our limited antifungal armamentarium. Antibiotics 9:877. doi: 10.3390/antibiotics9120877
Asolkar, R. N., Singh, A., Jensen, P. R., Aalbersberg, W., Carté, B. K., Feussner, K. D., et al. (2017). Marinocyanins, cytotoxic bromo-phenazinone meroterpenoids from a marine bacterium from the Streptomycete clade MAR4. Tetrahedron 73, 2234–2241. doi: 10.1016/j.tet.2017.03.003
Axenov-Gibanov, D. V., Voytsekhovskaya, I. V., Tokovenko, B. T., Protasov, E. S., Gamaiunov, S. V., Rebets, Y. V., et al. (2016). Actinobacteria isolated from an underground lake and moonmilk speleothem from the biggest conglomeratic karstic cave in Siberia as sources of novel biologically active compounds. PLoS One 11:e0149216. doi: 10.1371/journal.pone.0149216
Ayroza, G., Ferreira, I. L., Sayegh, R. S., Tashima, A. K., and da Silva Jr, P. I. (2012). Juruin: an antifungal peptide from the venom of the Amazonian pink toe spider, Avicularia juruensis, which contains the inhibitory cystine knot motif. Front. Microbiol. 3:324. doi: 10.3389/fmicb.2012.00324
Bae, M., Kim, H., Moon, K., Nam, S. J., Shin, J., Oh, K. B., et al. (2015). Mohangamides a and B, new dilactone-tethered pseudo-dimeric peptides inhibiting Candida albicans isocitrate lyase. Org. Lett. 17, 712–715. doi: 10.1021/ol5037248
Baindara, P., Nallabelli, N., and Korpole, S. (2020). Whole genome mining reveals a diverse repertoire of lanthionine synthetases and lanthipeptides among the genus Paenibacillus. J. Appl. Microbiol. 128, 473–490. doi: 10.1111/jam.14495
Barke, J., Seipke, R. F., Grüschow, S., Heavens, D., Drou, N., Bibb, M. J., et al. (2010). A mixed community of actinomycetes produce multiple antibiotics for the fungus farming ant Acromyrmex octospinosus. BMC Biol. 8:109. doi: 10.1186/1741-7007-8-109
Beemelmanns, C., Ramadhar, T. R., Kim, K. H., Klassen, J. L., Cao, S., Wyche, T. P., et al. (2017). Macrotermycins A–D, glycosylated macrolactams from a termite-associated Amycolatopsis sp. M39. Org. Lett. 19, 1000–1003. doi: 10.1021/acs.orglett.6b03831
Belguesmia, Y., Choiset, Y., Rabesona, H., Baudy-Floc'h, M., Le Blay, G., Haertlé, T., et al. (2013). Antifungal properties of durancins isolated from Enterococcus durans A 5-11 and of its synthetic fragments. Lett. Appl. Microbiol. 56, 237–244. doi: 10.1111/lam.12037
Belmadani, A., Semlali, A., and Rouabhia, M. (2018). Dermaseptin-S1 decreases Candida albicans growth, biofilm formation and the expression of hyphal wall protein 1 and aspartic protease genes. J. Appl. Microbiol. 125, 72–83. doi: 10.1111/jam.13745
Belyagoubi, L., Belyagoubi-Benhammou, N., Jurado, V., Dupont, J., Lacoste, S., Djebbah, F., et al. (2018). Antimicrobial activities of culturable microorganisms (actinomycetes and fungi) isolated from Chaabe Cave, Algeria. Int. J. Speleol. 47, 189–199. doi: 10.5038/1827-806X.47.2.2148
Bérdy, J. (2012). Thoughts and facts about antibiotics: where we are now and where we are heading. J. Antibiot. 65, 385–395. doi: 10.1038/ja.2012.27
Berger, L., Roberts, A. A., Voyles, J., Longcore, J. E., Murray, K. A., and Skerratt, L. F. (2016). History and recent progress on chytridiomycosis in amphibians. Fungal Ecol. 19, 89–99. doi: 10.1016/j.funeco.2015.09.007
Breznak, J. A. (2003). Invertebrates—insects. Micro. Div. Bioprospet., 191–203. doi: 10.1128/9781555817770.ch19
Brucker, R. M., Harris, R. N., Schwantes, C. R., Gallaher, T. N., Flaherty, D. C., Lam, B. A., et al. (2008). Amphibian chemical defense: antifungal metabolites of the microsymbiont Janthinobacterium lividum on the salamander Plethodon cinereus. J. Chem. Ecol. 34, 1422–1429. doi: 10.1007/s10886-008-9555-7
Bunyapaiboonsri, T., Yoiprommarat, S., Suriyachadkun, C., Supothina, S., Chanthaket, R., Chutrakul, C., et al. (2017). Actinomadurone, a polycyclic tetrahydroxanthone from Actinomadura sp. BCC 35430. Tetrahedron Lett. 58, 3223–3225. doi: 10.1016/j.tetlet.2017.07.008
Cao, Y., Pi, H., Chandrangsu, P., Li, Y., Wang, Y., Zhou, H., et al. (2018). Antagonism of two plant-growth promoting Bacillus velezensis isolates against Ralstonia solanacearum and Fusarium oxysporum. Sci. Rep. 8, 1–14. doi: 10.1038/s41598-018-22782-z
Carmona, E. M., and Limper, A. H. (2017). Overview of treatment approaches for fungal infections. Clin. Chest Med. 38, 393–402. doi: 10.1016/j.ccm.2017.04.003
Carr, G., Poulsen, M., Klassen, J. L., Hou, Y., Wyche, T. P., Bugni, T. S., et al. (2012). Microtermolides a and B from termite-associated Streptomyces sp. and structural revision of vinylamycin. Org. Lett. 14, 2822–2825. doi: 10.1021/ol301043p
Castro, J. F., Razmilic, V., Gomez-Escribano, J. P., Andrews, B., Asenjo, J., and Bibb, M. (2018). The ‘gifted’actinomycete Streptomyces leeuwenhoekii. Antonie Van Leeuwenhoek 111, 1433–1448. doi: 10.1007/s10482-018-1034-8
Chen, Y., Liu, R. H., Li, T. X., Huang, S. S., Kong, L. Y., and Yang, M. H. (2017). Enduspeptides AF, six new cyclic depsipeptides from a coal mine derived Streptomyces sp. Tetrahedron 73, 527–531. doi: 10.1016/j.tet.2016.12.033
Chen, Z., Ou, P., Liu, L., and Jin, X. (2020). Anti-MRSA activity of actinomycin X2 and collismycin a produced by Streptomyces globisporus WA5-2-37 from the intestinal tract of American cockroach (Periplaneta americana). Front. Microbiol. 11:555. doi: 10.3389/fmicb.2020.00555
Chevrette, M. G., Carlson, C. M., Ortega, H. E., Thomas, C., Ananiev, G. E., Barns, K. J., et al. (2019). The antimicrobial potential of Streptomyces from insect microbiomes. Nature Commun. 10:516. doi: 10.1038/s41467-019-08438-0
Chitty, J. L., and Fraser, J. A. (2017). Purine acquisition and synthesis by human fungal pathogens. Microorganisms 5:33. doi: 10.3390/microorganisms5020033
Chouvenc, T., Elliott, M. L., Šobotník, J., Efstathion, C. A., and Su, N. Y. (2018). The termite fecal nest: a framework for the opportunistic acquisition of beneficial soil Streptomyces (Actinomycetales: Streptomycetaceae). Environ. Entomol. 47, 1431–1439. doi: 10.1093/ee/nvy152
Cuenca-Estrella, M. (2014). Antifungal drug resistance mechanisms in pathogenic fungi: from bench to bedside. Clin. Microbiol. Infect. 20, 54–59. doi: 10.1111/1469-0691.12495
Currie, C. R., Poulsen, M., Mendenhall, J., Boomsma, J. J., and Billen, J. (2006). Coevolved crypts and exocrine glands support mutualistic bacteria in fungus-growing ants. Science 311, 81–83. doi: 10.1126/science.1119744
Dasari, P., Koleci, N., Shopova, I. A., Wartenberg, D., Beyersdorf, N., Dietrich, S., et al. (2019). Enolase from Aspergillus fumigatus is a moonlighting protein that binds the human plasma complement proteins factor H, FHL-1, C4BP, and plasminogen. Front. Immunol. 10:2573. doi: 10.3389/fimmu.2019.02573
Dash, R., and Bhattacharjya, S. (2021). Thanatin: an emerging host defense antimicrobial peptide with multiple modes of action. Int. J. Mol. Sci. 22:1522. doi: 10.3390/ijms22041522
Ding, N., Jiang, Y., Han, L., Chen, X., Ma, J., Qu, X., et al. (2016). Bafilomycins and odoriferous sesquiterpenoids from Streptomyces albolongus isolated from Elephas maximus feces. J. Nat. Prod. 79, 799–805. doi: 10.1021/acs.jnatprod.5b00827
Du, H., Bing, J., Hu, T., Ennis, C. L., Nobile, C. J., and Huang, G. (2020). Candida auris: epidemiology, biology, antifungal resistance, and virulence. PLoS Pathog. 16:e1008921. doi: 10.1371/journal.ppat.1008921
Du Pré, S., Beckmann, N., Almeida, M. C., Sibley, G. E., Law, D., Brand, A. C., et al. (2018). Effect of the novel antifungal drug F901318 (olorofim) on growth and viability of Aspergillus fumigatus. Antimicrob. Agents Chemother. 62, e00231–e00218. doi: 10.1128/AAC.00231-18
Ehret-Sabatier, L., Loew, D., Goyffon, M., Fehlbaum, P., Hoffmann, J. A., van Dorsselaer, A., et al. (1996). Characterization of novel cysteine-rich antimicrobial peptides from scorpion blood. J. Biol. Chem. 271, 29537–29544. doi: 10.1074/jbc.271.47.29537
Farag, R., and Swaby, S. (2018). Antimicrobial effects of wasp (Vespa orientalis) venom. Egypt. Pharm. J. 17, 218–222.
Feng, C., Ling, H., Du, D., Zhang, J., Niu, G., and Tan, H. (2014). Novel nikkomycin analogues generated by mutasynthesis in Streptomyces ansochromogenes. Microb. Cell Factories 13:59. doi: 10.1186/1475-2859-13-59
Fernandes, K. E., and Carter, D. A. (2017). The antifungal activity of lactoferrin and its derived peptides: mechanisms of action and synergy with drugs against fungal pathogens. Front. Microbiol. 8:2. doi: 10.3389/fmicb.2017.00002
Fisher, M. C., Gurr, S. J., Cuomo, C. A., Blehert, D. S., Jin, H., Stukenbrock, E. H., et al. (2020). Threats posed by the fungal kingdom to humans, wildlife, and agriculture. MBio 11, e00449–e00420. doi: 10.1128/mBio.00449-20
Fisher, M. C., Henk, D., Briggs, C. J., Brownstein, J. S., Madoff, L. C., McCraw, S. L., et al. (2012). Emerging fungal threats to animal, plant and ecosystem health. Nature 484, 186–194. doi: 10.1038/nature10947
Freire, D. O., da Cunha, N. B., Leite, M. L., Kostopoulos, A. G., da Silva, S. N., de Souza, A. C., et al. (2020). Wasp venom peptide, synoeca-MP, from Synoeca surinama shows antimicrobial activity against human and animal pathogenic microorganisms. Pept. Sci. 112:e24141. doi: 10.1002/pep2.24141
Friedman, D. Z., and Schwartz, I. S. (2019). Emerging fungal infections: new patients, new patterns, and new pathogens. J. Fungi 5:67. doi: 10.3390/jof5030067
Fu, Y., Estoppey, D., Roggo, S., Pistorius, D., Fuchs, F., Studer, C., et al. (2020). Jawsamycin exhibits in vivo antifungal properties by inhibiting Spt14/Gpi3-mediated biosynthesis of glycosylphosphatidylinositol. Nat. Commun. 11:3387. doi: 10.1038/s41467-020-17221-5
Funk, J., Schaarschmidt, B., Slesiona, S., Hallström, T., Horn, U., and Brock, M. (2016). The glycolytic enzyme enolase represents a plasminogen-binding protein on the surface of a wide variety of medically important fungal species. Int. J. Med. Microbiol. 306, 59–68. doi: 10.1016/j.ijmm.2015.11.005
Garrigues, S., Gandía, M., Castillo, L., Coca, M., Marx, F., Marcos, J. F., et al. (2018). Three antifungal proteins from Penicillium expansum: different patterns of production and antifungal activity. Front. Microbiol. 9:2370. doi: 10.3389/fmicb.2018.02370
Garrigues, S., Gandía, M., Popa, C., Borics, A., Marx, F., Coca, M., et al. (2017). Efficient production and characterization of the novel and highly active antifungal protein AfpB from Penicillium digitatum. Sci. Rep. 7:14663. doi: 10.1038/s41598-017-15277-w
Ghosh, S., Kuisiene, N., and Cheeptham, N. (2017). The cave microbiome as a source for drug discovery: reality or pipe dream? Biochem. Pharmacol. 134, 18–34. doi: 10.1016/j.bcp.2016.11.018
Gosse, J. T., Ghosh, S., Sproule, A., Overy, D., Cheeptham, N., and Boddy, C. N. (2019). Whole genome sequencing and metabolomic study of cave Streptomyces isolates ICC1 and ICC4. Front. Microbiol. 10:1020. doi: 10.3389/fmicb.2019.01020
Gupta, R., and Srivastava, S. (2014). Antifungal effect of antimicrobial peptides (AMPs LR14) derived from lactobacillus plantarum strain LR/14 and their applications in prevention of grain spoilage. Food Microbiol. 42, 1–7. doi: 10.1016/j.fm.2014.02.005
Guzman, J., and Vilcinskas, A. (2020). Bacteria associated with cockroaches: health risk or biotechnological opportunity? Appl. Microbiol. Biotechnol. 104, 10369–10387. doi: 10.1007/s00253-020-10973-6
Han, J., Wang, F., Gao, P., Ma, Z., Zhao, S., Lu, Z., et al. (2017). Mechanism of action of AMP-jsa9, a LI-F-type antimicrobial peptide produced by Paenibacillus polymyxa JSa-9, against Fusarium moniliforme. Fungal Genet. Biol. 104, 45–55. doi: 10.1016/j.fgb.2017.05.002
Hara, S., Ishikawa, N., Hara, Y., Nehira, T., Sakai, K., Gonoi, T., et al. (2017). Nabscessins A and B, aminocyclitol derivatives from Nocardia abscessus IFM 10029T. J. Nat. Prod. 80, 565–568. doi: 10.1021/acs.jnatprod.6b00935
Harunari, E., Imada, C., and Igarashi, Y. (2019). Konamycins a and B and rubromycins CA1 and CA2, aromatic polyketides from the tunicate-derived Streptomyces hyaluromycini MB-PO13T. J. Nat. Prod. 82, 1609–1615. doi: 10.1021/acs.jnatprod.9b00107
Heine, D., Holmes, N. A., Worsley, S. F., Santos, A. C. A., Innocent, T. M., Scherlach, K., et al. (2018). Chemical warfare between leafcutter ant symbionts and a co-evolved pathogen. Nat. Commun. 9:2208. doi: 10.1038/s41467-018-04520-1
Herold, K., Gollmick, F. A., Groth, I., Roth, M., Menzel, K. D., Möllmann, U., et al. (2005). Cervimycin A–D: a polyketide glycoside complex from a cave bacterium can defeat vancomycin resistance. Chem. Eur. J. 11, 5523–5530. doi: 10.1002/chem.200500320
Holmes, N. A., Innocent, T. M., Heine, D., Bassam, M. A., Worsley, S. F., Trottmann, F., et al. (2016). Genome analysis of two Pseudonocardia phylotypes associated with Acromyrmex leafcutter ants reveals their biosynthetic potential. Front. Microbiol. 7:2073. doi: 10.3389/fmicb.2016.02073
Holzknecht, J., Kühbacher, A., Papp, C., Farkas, A., Váradi, G., Marcos, J. F., et al. (2020). The Penicillium chrysogenum Q176 antimicrobial protein PAFC effectively inhibits the growth of the opportunistic human pathogen Candida albicans. J. Fungi 6:141. doi: 10.3390/jof6030141
Hong-Ling, Y. A. N. G., Zhi-Qiang, S. H. E. N., Xuan, L. I. U., and Yi, K. O. N. G. (2016). Two novel antimicrobial peptides from skin venoms of spadefoot toad Megophrys minor. Chin. J. Nat. Med. 14, 294–298. doi: 10.1016/S1875-5364(16)30030-9
Hoshino, S., Wong, C. P., Ozeki, M., Zhang, H., Hayashi, F., Awakawa, T., et al. (2018). Umezawamides, new bioactive polycyclic tetramate macrolactams isolated from a combined-culture of Umezawaea sp. and mycolic acid-containing bacterium. J. Antibiot. 71, 653–657. doi: 10.1038/s41429-018-0040-4
Huang, D. S., LeBlanc, E. V., Shekhar-Guturja, T., Robbins, N., Krysan, D. J., Pizarro, J., et al. (2019). Design and synthesis of fungal-selective resorcylate aminopyrazole Hsp90 inhibitors. J. Med. Chem. 63, 2139–2180. doi: 10.1021/acs.jmedchem.9b00826
Huang, Y. H., Wang, X. J., Zhang, F., Huo, X. B., Fu, R. S., Liu, J. J., et al. (2013). The identification of a bacterial strain BGI-1 isolated from the intestinal flora of Blattella germanica, and its anti-entomopathogenic fungi activity. J. Econ. Entomol. 106, 43–49. doi: 10.1603/ec12120
Huber, A., Hajdu, D., Bratschun-Khan, D., Gáspári, Z., Varbanov, M., Philippot, S., et al. (2018). New antimicrobial potential and structural properties of PAFB: a cationic, cysteine-rich protein from Penicillium chrysogenum Q176. Sci. Rep. 8, 1–16. doi: 10.1038/s41598-018-20002-2
Husain, S., and Camargo, J. F. (2019). Invasive Aspergillosis in solid-organ transplant recipients: guidelines from the American Society of Transplantation infectious diseases Community of Practice. Clin. Transpl. 33:e13544. doi: 10.1111/ctr.13544
Ibe, C., and Munro, C. A. (2021). Fungal cell wall: an underexploited target for antifungal therapies. PLoS Pathog. 17:e1009470. doi: 10.1371/journal.ppat.1009470
Igarashi, M., Sawa, R., Yamasaki, M., Hayashi, C., Umekita, M., Hatano, M., et al. (2017). Kribellosides, novel RNA 5′-triphosphatase inhibitors from the rare actinomycete Kribbella sp. MI481-42F6. J. Antibiot. 70, 582–589. doi: 10.1038/ja.2016.161
Ivanov, M., Ćirić, A., and Stojković, D. (2022). Emerging antifungal targets and strategies. Int. J. Mol. Sci. 23:2756. doi: 10.3390/ijms23052756
Ji, H., Wang, J., Guo, J., Li, Y., Lian, S., Guo, W., et al. (2016). Progress in the biological function of alpha-enolase. Anim. Nutr. 2, 12–17. doi: 10.1016/j.aninu.2016.02.005
Jia, F., Wang, J., Peng, J., Zhao, P., Kong, Z., Wang, K., et al. (2018). The in vitro, in vivo antifungal activity and the action mode of Jelleine-I against Candida species. Amino Acids 50, 229–239. doi: 10.1007/s00726-017-2507-1
Jiang, Z. K., Guo, L., Chen, C., Liu, S. W., Zhang, L., Dai, S. J., et al. (2015). Xiakemycin A, a novel pyranonaphthoquinone antibiotic, produced by the Streptomyces sp. CC8-201 from the soil of a karst cave. J. Antibiot. 68, 771–774. doi: 10.1038/ja.2015.70
Jiang, L., Pu, H., Xiang, J., Su, M., Yan, X., Yang, D., et al. (2018). Huanglongmycin AC, cytotoxic polyketides biosynthesized by a putative type II polyketide synthase from Streptomyces sp. CB09001. Front. Chem. 6:254. doi: 10.3389/fchem.2018.00254
Jiang, Y. J., Zhang, D. S., Zhang, H. J., Li, J. Q., Ding, W. J., Xu, C. D., et al. (2018). Medermycin-type naphthoquinones from the marine-derived Streptomyces sp. XMA39. J. Nat. Prod. 81, 2120–2124. doi: 10.1021/acs.jnatprod.8b00544
Kaltenpoth, M., Yildirim, E., Gürbüz, M. F., Herzner, G., and Strohm, E. (2012). Refining the roots of the beewolf-Streptomyces symbiosis: antennal symbionts in the rare genus Philanthinus (Hymenoptera, Crabronidae). Appl. Environ. Microbiol. 78, 822–827. doi: 10.1128/AEM.06809-11
Kang, B. R., Park, J. S., and Jung, W. J. (2020). Antifungal evaluation of fengycin isoforms isolated from Bacillus amyloliquefaciens PPL against Fusarium oxysporum f. sp. lycopersici. Microb. Pathog. 149:104509. doi: 10.1016/j.micpath.2020.104509
Karim, M. R. U., In, Y., Zhou, T., Harunari, E., Oku, N., and Igarashi, Y. (2021). Nyuzenamides a and B: bicyclic peptides with antifungal and cytotoxic activity from a marine-derived Streptomyces sp. Org. Lett. 23, 2109–2113. doi: 10.1021/acs.orglett.1c00210
Kett, S., Pathak, A., Turillazzi, S., Cavalieri, D., and Marvasi, M. (2021). Antifungals, arthropods and antifungal resistance prevention: lessons from ecological interactions. Proc. R. Soc. B 288:20202716. doi: 10.1098/rspb.2020.2716
Khatoon, Z., McTiernan, C. D., Suuronen, E. J., Mah, T. F., and Alarcon, E. I. (2018). Bacterial biofilm formation on implantable devices and approaches to its treatment and prevention. Heliyon 4:e01067. doi: 10.1016/j.heliyon.2018.e01067
Kim, H. J., Han, C. Y., Park, J. S., Oh, S. H., Kang, S. H., Choi, S. S., et al. (2018). Nystatin-like Pseudonocardia polyene B1, a novel disaccharide-containing antifungal heptaene antibiotic. Sci. Rep. 8:13584. doi: 10.1038/s41598-018-31801-y
Kim, K. H., Ramadhar, T. R., Beemelmanns, C., Cao, S., Poulsen, M., Currie, C. R., et al. (2014). Natalamycin A, an ansamycin from a termite-associated Streptomyces sp. Chem. Sci. 5, 4333–4338. doi: 10.1039/C4SC01136H
Komatsu, A., Satoh, T., Wakabayashi, H., and Ikeda, F. (2015). Effects of bovine lactoferrin to oral Candida albicans and Candida glabrata isolates recovered from the saliva in elderly people. Odontology 103, 50–55. doi: 10.1007/s10266-013-0135-0
Kumar, V., Bharti, A., Gupta, V. K., Gusain, O., and Bisht, G. S. (2012). Actinomycetes from solitary wasp mud nest and swallow bird mud nest: isolation and screening for their antibacterial activity. World J. Microbiol. Biotechnol. 28, 871–880. doi: 10.1007/s11274-011-0884-2
Kumar, V., Naik, B., Gusain, O., and Bisht, G. S. (2014). An actinomycete isolate from solitary wasp mud nest having strong antibacterial activity and kills the Candida cells due to the shrinkage and the cytosolic loss. Front. Microbiol. 5:446. doi: 10.3389/fmicb.2014.00446
Kurtböke, D. İ., French, J. R., Hayes, R. A., and Quinn, R. J. (2015). Eco-taxonomic insights into actinomycete symbionts of termites for discovery of novel bioactive compounds. Biotechnol. Appl. Biodivers. 147, 111–135. doi: 10.1007/10_2014_270
Lacret, R., Oves-Costales, D., Gómez, C., Díaz, C., De la Cruz, M., Pérez-Victoria, I., et al. (2014). New ikarugamycin derivatives with antifungal and antibacterial properties from Streptomyces zhaozhouensis. Mar. Drugs 13, 128–140. doi: 10.3390/md13010128
Lamprinou, V., Tryfinopoulou, K., Velonakis, E. N., Vatopoulos, A., Antonopoulou, S., Fragopoulou, E., et al. (2015). Cave cyanobacteria showing antibacterial activity. Int. J. Speleol. 44, 231–238. doi: 10.5038/1827-806X.44.3.2
Law, D. (2016). The efficacy of F901318 a novel antifungal drug in an animal model of aspergillosis. ICAAC-American Society for Microbiology Boston, MA.
Law, R. H., Caradoc-Davies, T., Cowieson, N., Horvath, A. J., Quek, A. J., Encarnacao, J. A., et al. (2012). The X-ray crystal structure of fulllength human plasminogen. Cell Rep. 1, 185–190. doi: 10.1016/j.celrep.2012.02.012
Lazar, N., Mesarich, C. H., Petit-Houdenot, Y., Talbi, N., Li de la Sierra-Gallay, I., Zélie, E., et al. (2022). A new family of structurally conserved fungal effectors displays epistatic interactions with plant resistance proteins. PLoS Pathog. 18:e1010664. doi: 10.1128/microbiolspec.FUNK-0027-2016
Lebedeva, J., Jukneviciute, G., Čepaitė, R., Vickackaite, V., Pranckutė, R., and Kuisiene, N. (2021). Genome mining and characterization of biosynthetic gene clusters in two cave strains of Paenibacillus sp. Front. Microbiol. 11:612483. doi: 10.3389/fmicb.2020.612483
Lee, S. D., Kim, E. S., Roe, J. H., Kim, J. H., Kang, S. O., and Hah, Y. C. (2000). Saccharothrix violacea sp. nov., isolated from a gold mine cave, and Saccharothrix albidocapillata comb. nov. Int. J. Syst. Evol. Microbiol. 50, 1315–1323. doi: 10.1099/00207713-50-3-1315
Lever, M. A., Torti, A., Eickenbusch, P., Michaud, A. B. Å., Antl-Temkiv, T., and JãRgensen, B. B. (2015). A modular method for the extraction of DNA and RNA, and the separation of DNA pools from diverse environmental sample types. Front. Microbiol. 6:476. doi: 10.3389/fmicb.2015.00476
Liu, Z., Yuan, K., Zhang, R., Ren, X., Liu, X., Zhao, S., et al. (2016). Cloning and purification of the first termicin-like peptide from the cockroach Eupolyphaga sinensis. J. Venom. Anim. Toxins Incl. Trop. Dis. 22:5. doi: 10.1186/s40409-016-0058-7
Lockhart, S. R., and Guarner, J. (2019). Emerging and reemerging fungal infections. Semin. Diagn. Pathol. 36, 177–181. doi: 10.1053/j.semdp.2019.04.010
Mann, P. A., McLellan, C. A., Koseoglu, S., Si, Q., Kuzmin, E., Flattery, A., et al. (2015). Chemical genomics-based antifungal drug discovery: targeting glycosylphosphatidylinositol (GPI) precursor biosynthesis. ACS Infect. Dis. 1, 59–72. doi: 10.1021/id5000212
Manns, D. C., Churey, J. J., and Worobo, R. W. (2015). Variable efficacy of the proteinaceous antifungal YvgO in select fruit juices and teas as a complement with UV methods of food protection. J. Food Prot. 78, 1851–1860. doi: 10.4315/0362-028X.JFP-15-128
McLellan, C. A., Whitesell, L., King, O. D., Lancaster, A. K., Mazitschek, R., and Lindquist, S. (2012). Inhibiting GPI anchor biosynthesis in fungi stresses the endoplasmic reticulum and enhances immunogenicity. ACS Chem. Biol. 7, 1520–1528. doi: 10.1021/cb300235m
Meena, M., Prasad, V., Zehra, A., Gupta, V. K., and Upadhyay, R. S. (2015). Mannitol metabolism during pathogenic fungal-host interactions under stressed conditions. Front. Microbiol. 6:1019. doi: 10.3389/fmicb.2015.01019
Memariani, H., and Memariani, M. (2020). Anti-fungal properties and mechanisms of melittin. Appl. Microbiol. Biotechnol. 104, 6513–6526. doi: 10.1007/s00253-020-10701-0
Miyazaki, M., Horii, T., Hata, K., Watanabe, N. A., Nakamoto, K., Tanaka, K., et al. (2011). In vitro activity of E1210, a novel antifungal, against clinically important yeasts and molds. Antimicrob. Agents Chemother. 55, 4652–4658. doi: 10.1128/AAC.00291-11
Moghaddam, M. R. B., Vilcinskas, A., and Rahnamaeian, M. (2017). The insect-derived antimicrobial peptide metchnikowin targets Fusarium graminearum β (1, 3) glucanosyltransferase Gel1, which is required for the maintenance of cell wall integrity. Biol. Chem. 398, 491–498. doi: 10.1515/hsz-2016-0295
Muhialdin, B. J., Hassan, Z., Bakar, F. A., and Saari, N. (2016). Identification of antifungal peptides produced by lactobacillus plantarum IS10 grown in the MRS broth. Food Control 59, 27–30. doi: 10.1016/j.foodcont.2015.05.022
Nakaew, N., Pathom-aree, W., and Lumyong, S. (2009a). Generic diversity of rare actinomycetes from Thai cave soils and their possible use as new bioactive compounds. Actinomycetologica 23, 21–26. doi: 10.3209/saj.SAJ230201
Nakaew, N., Pathom-Aree, W., and Lumyong, S. (2009b). First record of the isolation, identification and biological activity of a new strain of Spirillospora albida from Thai cave soil. Actinomycetologica 23, 1–7. doi: 10.3209/saj.SAJ230102
Newman, D. J., and Cragg, G. M. (2020). Natural products as sources of new drugs over the nearly four decades from 01/1981 to 09/2019. J. Nat. Prod. 83, 770–803. doi: 10.1021/acs.jnatprod.9b01285
Ningthoujam, D. S., Sanasam, S., and Nimaichand, S. (2009). Screening of actinomycete isolates from niche habitats in Manipur for antibiotic activity. Am. J. Biochem. Biotechnol. 5, 221–225. doi: 10.3844/ajbbsp.2009.221.225
Nirma, C., Eparvier, V., and Stien, D. (2015). Antibacterial ilicicolinic acids C and D and ilicicolinal from Neonectria discophora SNB-CN63 isolated from a termite nest. J. Nat. Prod. 78, 159–162. doi: 10.1021/np500080m
Odds, F. C., Brown, A. J., and Gow, N. A. (2003). Antifungal agents: mechanisms of action. Trends Microbiol. 11, 272–279. doi: 10.1016/s0966-842x(03)00117-3
Oh, D. C., Poulsen, M., Currie, C. R., and Clardy, J. (2009). Dentigerumycin: a bacterial mediator of an ant-fungus symbiosis. Nat. Chem. Biol. 5, 391–393. doi: 10.1038/nchembio.159
Oh, D. C., Poulsen, M., Currie, C. R., and Clardy, J. (2011). Sceliphrolactam, a polyene macrocyclic lactam from a wasp-associated Streptomyces sp. Org. Lett. 13, 752–755. doi: 10.1021/ol102991d
Oliver, J., Law, D., Sibley, G., Kennedy, A., and Birch, M. (2016). F901318, a novel antifungal agent from the orotomide class: discovery and mechanism of action. Poster 749 advances against Aspergillosis.
Orivel, J., Redeker, V., Le Caer, J. P., Krier, F., Revol-Junelles, A. M., Longeon, A., et al. (2001). Ponericins, new antibacterial and insecticidal peptides from the venom of the ant Pachycondyla goeldii. J. Biol. Chem. 276, 17823–17829. doi: 10.1074/jbc.M100216200
Otani, S., Mikaelyan, A., Nobre, T., Hansen, L. H., Koné, N. G. A., Sørensen, S. J., et al. (2014). Identifying the core microbial community in the gut of fungus-growing termites. Mol. Ecol. 23, 4631–4644. doi: 10.1111/mec.12874
Patiño, B., Vázquez, C., Manning, J. M., Roncero, M. I. G., Córdoba-Cañero, D., Di Pietro, A., et al. (2018). Characterization of a novel cysteine-rich antifungal protein from Fusarium graminearum with activity against maize fungal pathogens. Int. J. Food Microbiol. 283, 45–51. doi: 10.1016/j.ijfoodmicro.2018.06.017
Pérez, M., Schleissner, C., Fernández, R., Rodríguez, P., Reyes, F., Zuñiga, P., et al. (2016). PM100117 and PM100118, new antitumor macrolides produced by a marine Streptomyces caniferus GUA-06-05-006A. J. Antibiot. 69, 388–394. doi: 10.1038/ja.2015.121
Pérez-Victoria, I., Oves-Costales, D., Lacret, R., Martín, J., Sánchez-Hidalgo, M., Díaz, C., et al. (2019). Structure elucidation and biosynthetic gene cluster analysis of caniferolides A–D, new bioactive 36-membered macrolides from the marine-derived Streptomyces caniferus CA-271066. Org. Biomol. Chem. 17, 2954–2971. doi: 10.1039/c8ob03115k
Perfect, J. R. (2017). The antifungal pipeline: a reality check. Nat. Rev. Drug Discov. 16, 603–616. doi: 10.1038/nrd.2017.46
Pfaller, M. A., Rhomberg, P. R., Messer, S. A., and Castanheira, M. (2015). In vitro activity of a Hos2 deacetylase inhibitor, MGCD290, in combination with echinocandins against echinocandin-resistant Candida species. Diagn. Microbiol. Infect. Dis. 81, 259–263. doi: 10.1016/j.diagmicrobio.2014.11.008
Pfaller, M. A., Watanabe, N., Castanheira, M., Messer, S. A., and Jones, R. N. (2011). Pre-clinical development of antifungal susceptibility test methods for the testing of the novel antifungal agent E1210 versus Candida: comparison of CLSI and European committee on antimicrobial susceptibility testing methods. J. Antimicrob. Chemother. 66, 2581–2584. doi: 10.1093/jac/dkr342
Pierce, C. G., Srinivasan, A., Ramasubramanian, A. K., and López-Ribot, J. L. (2015). From biology to drug development: new approaches to combat the threat of fungal biofilms. Microbiol. Spectr. 3:3. doi: 10.1128/microbiolspec.MB-0007-2014
Poulsen, M., Cafaro, M. J., Erhardt, D. P., Little, A. E., Gerardo, N. M., Tebbets, B., et al. (2010). Variation in Pseudonocardia antibiotic defence helps govern parasite-induced morbidity in Acromyrmex leaf-cutting ants. Environ. Microbiol. Rep. 2, 534–540. doi: 10.1111/j.1758-2229.2009.00098.x
Poulsen, M., Oh, D. C., Clardy, J., and Currie, C. R. (2011). Chemical analyses of wasp-associated Streptomyces bacteria reveal a prolific potential for natural products discovery. PLoS One 6:e16763. doi: 10.1371/journal.pone.0016763
Prajapati, K. K., and Upadhyay, R. K. (2016). Antimicrobial activity of purified toxins from yellow wasp Polistes flavus (Vespidae) against certain bacteria and fungi. J. Biosci. Med. 4, 95–104. doi: 10.4236/jbm.2016.47010
Price, C. L., Parker, J. E., Warrilow, A. G., Kelly, D. E., and Kelly, S. L. (2015). Azole fungicides–understanding resistance mechanisms in agricultural fungal pathogens. Pest Manag. Sci. 71, 1054–1058. doi: 10.1002/ps.4029
Puri, S., and Edgerton, M. (2014). How does it kill?: understanding the candidacidal mechanism of salivary histatin 5. Eukaryot. Cell 13, 958–964. doi: 10.1128/EC.00095-14
Rangel, M., dos Santos Cabrera, M. P., Kazuma, K., Ando, K., Wang, X., Kato, M., et al. (2011). Chemical and biological characterization of four new linear cationic α-helical peptides from the venoms of two solitary eumenine wasps. Toxicon 57, 1081–1092. doi: 10.1016/j.toxicon.2011.04.014
Riquelme, C., Enes Dapkevicius, M. D. L., Miller, A. Z., Charlop-Powers, Z., Brady, S., Mason, C., et al. (2017). Biotechnological potential of Actinobacteria from Canadian and Azorean volcanic caves. Appl. Microbiol. Biotechnol. 101, 843–857. doi: 10.1007/s00253-016-7932-7
Rodriguez-Suarez, R., Xu, D., Veillette, K., Davison, J., Sillaots, S., Kauffman, S., et al. (2007). Mechanism-of-action determination of GMP synthase inhibitors and target validation in Candida albicans and Aspergillus fumigatus. Chem. Biol. 14, 1163–1175. doi: 10.1016/j.chembiol.2007.09.009
Rogozhin, E. A., Sadykova, V. S., Baranova, A. A., Vasilchenko, A. S., Lushpa, V. A., Mineev, K. S., et al. (2018). A novel lipopeptaibol emericellipsin a with antimicrobial and antitumor activity produced by the extremophilic fungus Emericellopsis alkalina. Molecules 23:2785. doi: 10.3390/molecules23112785
Rosengaus, R. B., Mead, K., Du Comb, W. S., Benson, R. W., and Godoy, V. G. (2013). Nest sanitation through defecation: antifungal properties of wood cockroach feces. Naturwissenschaften 100, 1051–1059. doi: 10.1007/s00114-013-1110-x
Rosengaus, R. B., Schultheis, K. F., Yalonetskaya, A., Bulmer, M. S., DuComb, W. S., Benson, R. W., et al. (2014). Symbiont-derived β-1, 3-glucanases in a social insect: mutualism beyond nutrition. Front. Microbiol. 5:607. doi: 10.3389/fmicb.2014.00607
Salem, H., Kreutzer, E., Sudakaran, S., and Kaltenpoth, M. (2013). A ctinobacteria as essential symbionts in firebugs and cotton stainers (H emiptera, P yrrhocoridae). Environ. Microbiol. 15, 1956–1968. doi: 10.1111/1462-2920.12001
Sanglard, D. (2016). Emerging threats in antifungal-resistant fungal pathogens. Front. Med. 3:11. doi: 10.3389/fmed.2016.00011
Santos, D. M., Verly, R. M., Piló-Veloso, D., De Maria, M., De Carvalho, M. A. R., Cisalpino, P. S., et al. (2010). LyeTx I, a potent antimicrobial peptide from the venom of the spider Lycosa erythrognatha. Amino Acids 39, 135–144. doi: 10.1007/s00726-009-0385-x
Sapsford, S. J., Alford, R. A., and Schwarzkopf, L. (2013). Elevation, temperature, and aquatic connectivity all influence the infection dynamics of the amphibian chytrid fungus in adult frogs. PLoS One 8:e82425. doi: 10.1371/journal.pone.0082425
Sato, S., Iwata, F., Yamada, S., and Katayama, M. (2012). Neomaclafungins A–I: oligomycin-class macrolides from a marine-derived actinomycete. J. Nat. Prod. 75, 1974–1982. doi: 10.1021/np300719g
Shehata, M. G., Badr, A. N., and Sohaimy, S. A. E. (2018). Novel antifungal Bacteriocin from Lactobacillus paracasei KC39 with AntiMycotoxigenic properties. Biosci. Res. 15, 4171–4183.
Shubitz, L. F., Trinh, H. T., Perrill, R. H., Thompson, C. M., Hanan, N. J., Galgiani, J. N., et al. (2014). Modeling nikkomycin Z dosing and pharmacology in murine pulmonary coccidioidomycosis preparatory to phase 2 clinical trials. J. Infect. Dis. 209, 1949–1954. doi: 10.1093/infdis/jiu029
Silva, J. C., Neto, L. M., Neves, R. C., Gonçalves, J. C., Trentini, M. M., Mucury-Filho, R., et al. (2017). Evaluation of the antimicrobial activity of the mastoparan Polybia-MPII isolated from venom of the social wasp Pseudopolybia vespiceps testacea (Vespidae, Hymenoptera). Int. J. Antimicrob. Agents 49, 167–175. doi: 10.1016/j.ijantimicag.2016.11.013
Simon, S., Chai, K., Drescher, M., and Chaves-Campos, J. (2022). Bacteria associated with leaf-cutter ants drive natural antibiotic resistance in soil bacteria. J. Trop. Ecol. 38, 410–415. doi: 10.1017/S0266467422000323
Slaninová, J., Putnová, H., Borovičková, L., Šácha, P., Čeřovský, V., Monincová, L., et al. (2011). The antifungal effect of peptides from hymenoptera venom and their analogs. Open Life Sci. 6, 150–159. doi: 10.2478/s11535-010-0111-4
Smith, K. D., Achan, B., Hullsiek, K. H., McDonald, T. R., Okagaki, L. H., Alhadab, A. A., et al. (2015). Increased antifungal drug resistance in clinical isolates of Cryptococcus neoformans in Uganda. Antimicrob. Agents Chemother. 59, 7197–7204. doi: 10.1128/AAC.01299-15
Sorres, J., Sabri, A., Brel, O., Stien, D., and Eparvier, V. (2018). Ilicicolinic acids and ilicicolinal derivatives from the fungus Neonectria discophora SNB-CN63 isolated from the nest of the termite Nasutitermes corniger found in French Guiana show antimicrobial activity. Phytochemistry 151, 69–77. doi: 10.1016/j.phytochem.2018.04.003
Stankovic, N., Radulovic, V., Petkovic, M., Vuckovic, I., Jadranin, M., Vasiljevic, B., et al. (2012). Streptomyces sp. JS520 produces exceptionally high quantities of undecylprodigiosin with antibacterial, antioxidative, and UV-protective properties. Appl. Microbiol. Biotechnol. 96, 1217–1231. doi: 10.1007/s00253-012-4237-3
Sugiyama, R., Nishimura, S., Ozaki, T., Asamizu, S., Onaka, H., and Kakeya, H. (2015). 5-Alkyl-1, 2, 3, 4-tetrahydroquinolines, new membrane-interacting lipophilic metabolites produced by combined culture of Streptomyces nigrescens and Tsukamurella pulmonis. Org. Lett. 17, 1918–1921. doi: 10.1021/acs.orglett.5b00607
Taj-Aldeen, S. J. (2017). Reduced multidrug susceptibility profile is a common feature of opportunistic Fusarium species: Fusarium multi-drug resistant pattern. J. Fungi 3:18. doi: 10.3390/jof3020018
Thery, T., Tharappel, J. C., Kraszewska, J., Beckett, M., Bond, U., and Arendt, E. K. (2016). Antifungal activity of a synthetic human β-defensin 3 and potential applications in cereal-based products. Innovative Food Sci. Emerg. Technol. 38, 160–168. doi: 10.1016/j.ifset.2016.09.018
Tian, H., Shafi, J., Ji, M., Bi, Y., and Yu, Z. (2017). Antimicrobial metabolites from Streptomyces sp. SN0280. J. Nat. Prod. 80, 1015–1019. doi: 10.1021/acs.jnatprod.6b01016
Tonk, M., Cabezas-Cruz, A., Valdés, J. J., Rego, R. O., Grubhoffer, L., Estrada-Pena, A., et al. (2015). Ixodes ricinus defensins attack distantly-related pathogens. Dev. Comp. Immunol. 53, 358–365. doi: 10.1016/j.dci.2015.08.001
Tóth, L., Kele, Z., Borics, A., Nagy, L. G., Váradi, G., Virágh, M., et al. (2016). NFAP2, a novel cysteine-rich anti-yeast protein from Neosartorya fischeri NRRL 181: isolation and characterization. AMB Express 6:75. doi: 10.1186/s13568-016-0250-8
Trapero, A., Pacitto, A., Singh, V., Sabbah, M., Coyne, A. G., Mizrahi, V., et al. (2018). Fragment-based approach to targeting Inosine-5′-monophosphate dehydrogenase (IMPDH) from mycobacterium tuberculosis. J. Med. Chem. 61, 2806–2822. doi: 10.1021/acs.jmedchem.7b01622
Tu, C. Y., Chen, Y. P., Yu, M. C., Hwang, E., Wu, D. Y., and Liaw, L. L. (2016). Characterization and expression of the antifungal protein from Monascus pilosus and its distribution among various Monascus species. J. Biosci. Bioeng. 122, 27–33. doi: 10.1016/j.jbiosc.2015.12.009
Um, S., Fraimout, A., Sapountzis, P., Oh, D. C., and Poulsen, M. (2013). The fungus-growing termite Macrotermes natalensis harbors bacillaene-producing bacillus sp. that inhibit potentially antagonistic fungi. Sci. Rep. 3:3250. doi: 10.1038/srep03250
Um, S., Park, S. H., Kim, J., Park, H. J., Ko, K., Bang, H. S., et al. (2015). Coprisamides a and B, new branched cyclic peptides from a gut bacterium of the dung beetle Copris tripartitus. Org. Lett. 17, 1272–1275. doi: 10.1021/acs.orglett.5b00249
van Bergeijk, D. A., Terlouw, B. R., Medema, M. H., and van Wezel, G. P. (2020). Ecology and genomics of Actinobacteria: new concepts for natural product discovery. Nat. Rev. Microbiol. 18, 546–558. doi: 10.1038/s41579-020-0379-y
Van Daele, R., Spriet, I., Wauters, J., Maertens, J., Mercier, T., Van Hecke, S., et al. (2019). Antifungal drugs: what brings the future? Med. Mycol. 57, S328–S343. doi: 10.1093/mmy/myz012
Vassilevski, A. A., Kozlov, S. A., and Grishin, E. V. (2009). Molecular diversity of spider venom, Molecular diversity of spider venom. Biochemistry 74, 1505–1534. doi: 10.1134/S0006297909130069
Vicente dos Reis, G., Abraham, W. R., Grigoletto, D. F., de Campos, J. B., Marcon, J., da Silva, J. A., et al. (2019). Gloeosporiocide, a new antifungal cyclic peptide from Streptomyces morookaense AM25 isolated from the Amazon bulk soil. FEMS Microbiol. Lett. 366:fnz175. doi: 10.1093/femsle/fnz175
Voytsekhovskaya, I. V., Axenov-Gribanov, D. V., Murzina, S. A., Pekkoeva, S. N., Protasov, E. S., Gamaiunov, S. V., et al. (2018). Estimation of antimicrobial activities and fatty acid composition of actinobacteria isolated from water surface of underground lakes from Badzheyskaya and Okhotnichya caves in Siberia. PeerJ 6:e5832. doi: 10.7717/peerj.5832
Wang, F., Fu, S. N., Bao, Y. X., Yang, Y., Shen, H. F., Lin, B. R., et al. (2017). Kitamycin C, a new antimycin-type antibiotic from Streptomyces antibioticus strain 200-09. Nat. Prod. Res. 31, 1819–1824. doi: 10.1080/14786419.2017.1295240
Wang, H., Tian, R., Tian, Q., Yan, X., Huang, L., and Ji, Z. (2019). Investigation on the antifungal ingredients of Saccharothrix yanglingensis Hhs. 015, an antagonistic endophytic actinomycete isolated from cucumber plant. Molecules 24:3686. doi: 10.3390/molecules24203686
Wang, X., Zhao, J., Ragupathy, V., and Hewlett, I. (2019). The effects of MAPK p38α on AZT resistance against reactivating HIV-1 replication in ACH2 cells. Molecular and Cellular Biochemistry 462, 41–50. doi: 10.1007/s11010-019-03608-6
Watanabe, N. A., Miyazaki, M., Horii, T., Sagane, K., Tsukahara, K., and Hata, K. (2012). E1210, a new broad-spectrum antifungal, suppresses Candida albicans hyphal growth through inhibition of glycosylphosphatidylinositol biosynthesis. Antimicrob. Agents Chemother. 56, 960–971. doi: 10.1128/AAC.00731-11
Wen, C., Guo, W., and Chen, X. (2014). Purification and identification of a novel antifungal protein secreted by Penicillium citrinum from the Southwest Indian Ocean. J. Microbiol. Biotechnol. 24, 1337–1345. doi: 10.4014/jmb.1405.05008
Whitesell, L., Robbins, N., Huang, D. S., McLellan, C. A., Shekhar-Guturja, T., LeBlanc, E. V., et al. (2019). Structural basis for species-selective targeting of Hsp90 in a pathogenic fungus. Nat. Commun. 10, 1–17. doi: 10.1038/s41467-018-08248-w
WHO (2019). The top 10 causes of death. Available at: https://www.who.int/news-room/fact-sheets/detail/the-top-10-causes-of-death [Accessed July 23, 2022].
Wiederhold, N. P. (2020). Review of the novel investigational antifungal Olorofim. J. Fungi 6:122. doi: 10.3390/jof6030122
Wyatt, T. T., van Leeuwen, M. R., Wösten, H. A., and Dijksterhuis, J. (2014). Mannitol is essential for the development of stress-resistant ascospores in Neosartorya fischeri (Aspergillus fischeri). Fungal Genet. Biol. 64, 11–24. doi: 10.1016/j.fgb.2013.12.010
Wyche, T. P., Piotrowski, J. S., Hou, Y., Braun, D., Deshpande, R., McIlwain, S., et al. (2014). Forazoline a: marine-derived polyketide with antifungal in vivo efficacy. Angew. Chem. Int. Ed. 53, 11583–11586. doi: 10.1002/anie.201405990
Yadav, U., and Khan, M. A. (2018). Targeting the GPI biosynthetic pathway. Pathog. Glob. Health 112, 115–122. doi: 10.1080/20477724.2018.1442764
Yamamoto, K., Futamura, Y., Uson-Lopez, R. A., Aono, H., Shimizu, T., and Osada, H. (2019). YO-001A, a new antifungal agent produced by Streptomyces sp. YO15-A001. J. Antibiot. 72, 986–990. doi: 10.1038/s41429-019-0239-z
Yan, L., and Adams, M. E. (1998). Lycotoxins, antimicrobial peptides from venom of the wolf spider Lycosa carolinensis. J. Biol. Chem. 273, 2059–2066. doi: 10.1074/jbc.273.4.2059
Yang, X., Wang, Y., Lee, W. H., and Zhang, Y. (2013). Antimicrobial peptides from the venom gland of the social wasp Vespa tropica. Toxicon 74, 151–157. doi: 10.1016/j.toxicon.2013.08.056
Yang, J., Yang, Z., Yin, Y., Rao, M., Liang, Y., and Ge, M. (2016). Three novel polyene macrolides isolated from cultures of Streptomyces lavenduligriseus. J. Antibiot. 69, 62–65. doi: 10.1038/ja.2015.76
Yek, S. H., Boomsma, J. J., and Poulsen, M. (2012). Towards a better understanding of the evolution of specialized parasites of fungus-growing ant crops. Psyche 2012:239392. doi: 10.1155/2012/239392
Yin, C., Jin, L., Li, S., Xu, X., and Zhang, Y. (2019). Diversity and antagonistic potential of Actinobacteria from the fungus-growing termite Odontotermes formosanus. 3. Biotech 9, 45–47. doi: 10.1007/s13205-019-1573-3
Yogita, R., Jayant, B., and Vibhuti, R. (2012). Potentiality test in antimicrobial activity and antibiotic sensitivity of subterranean Streptomyces strains isolated from Kotumsar cave of India. Int. J. Biol. Chem. 6, 53–60.
Yücel, S. E. M. R. A., and Yamaç, M. U. S. T. A. F. A. (2010). Selection of Streptomyces isolates from Turkish karstic caves against antibiotic resistant microorganisms. Pak. J. Pharm. Sci. 23, 1–6.
Zada, S., Naseem, A. A., Lee, S. J., Rafiq, M., Khan, I., Shah, A. A., et al. (2016). Geochemical and mineralogical analysis of Kashmir cave (smast), Buner, Pakistan, and isolation and characterization of bacteria having antibacterial activity. J. Cave Karst Stud. 78, 94–109. doi: 10.4311/2014MB0110
Zare-Zardini, H., Taheri-Kafrani, A., Ordooei, M., Ebrahimi, L., Tolueinia, B., and Soleimanizadeh, M. (2015). Identification and biochemical characterization of a new antibacterial and antifungal peptide derived from the insect Sphodromantis viridis. Biochem. Mosc. 80, 433–440. doi: 10.1134/S0006297915040069
Zeng, X. C., Wang, S., Nie, Y., Zhang, L., and Luo, X. (2012). Characterization of BmKbpp, a multifunctional peptide from the Chinese scorpion Mesobuthus martensii Karsch: gaining insight into a new mechanism for the functional diversification of scorpion venom peptides. Peptides 33, 44–51. doi: 10.1016/j.peptides.2011.11.012
Zhao, H., Kong, Y., Wang, H., Yan, T., Feng, F., Bian, J., et al. (2011). A defensin-like antimicrobial peptide from the venoms of spider, Ornithoctonus hainana. J. Pept. Sci. 17, 540–544. doi: 10.1002/psc.1370
Zhao, M., Lin, X., and Guo, X. (2022). The role of insect symbiotic bacteria in metabolizing phytochemicals and agrochemicals. Insects 13:583. doi: 10.3390/insects13070583
Keywords: antifungal agents, novel targets, drug resistant, unusual habitats, peptides
Citation: Choudhary M, Kumar V, Naik B, Verma A, Saris PEJ, Kumar V and Gupta S (2022) Antifungal metabolites, their novel sources, and targets to combat drug resistance. Front. Microbiol. 13:1061603. doi: 10.3389/fmicb.2022.1061603
Received: 04 October 2022; Accepted: 08 November 2022;
Published: 02 December 2022.
Edited by:
Guoqing Niu, Southwest University, ChinaReviewed by:
Elisa Binda, University of Insubria, ItalyCopyright © 2022 Choudhary, Kumar, Naik, Verma, Saris, Kumar and Gupta. This is an open-access article distributed under the terms of the Creative Commons Attribution License (CC BY). The use, distribution or reproduction in other forums is permitted, provided the original author(s) and the copyright owner(s) are credited and that the original publication in this journal is cited, in accordance with accepted academic practice. No use, distribution or reproduction is permitted which does not comply with these terms.
*Correspondence: Vijay Kumar, dmlqYXlna3BAZ21haWwuY29t; Per Erik Joakim Saris, cGVyLnNhcmlzQGhlbHNpbmtpLmZp
Disclaimer: All claims expressed in this article are solely those of the authors and do not necessarily represent those of their affiliated organizations, or those of the publisher, the editors and the reviewers. Any product that may be evaluated in this article or claim that may be made by its manufacturer is not guaranteed or endorsed by the publisher.
Research integrity at Frontiers
Learn more about the work of our research integrity team to safeguard the quality of each article we publish.